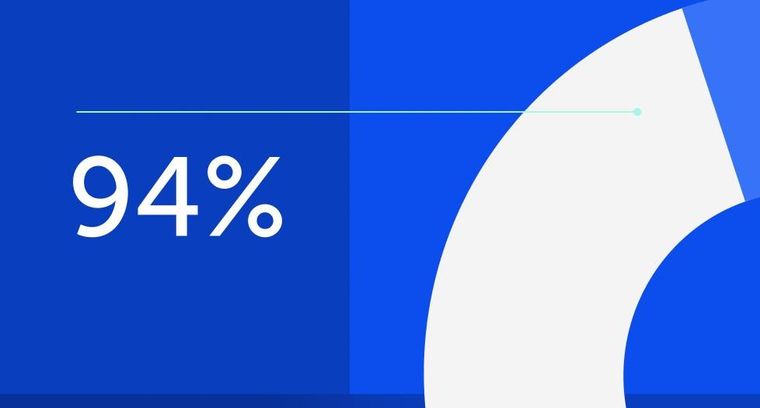
94% of researchers rate our articles as excellent or good
Learn more about the work of our research integrity team to safeguard the quality of each article we publish.
Find out more
REVIEW article
Front. Oncol., 07 July 2022
Sec. Pharmacology of Anti-Cancer Drugs
Volume 12 - 2022 | https://doi.org/10.3389/fonc.2022.933125
This article is part of the Research TopicNanomaterials and Multimodal Tumor TherapyView all 45 articles
Recent advances in nanotechnologies for cancer diagnosis and treatment have received considerable attention worldwide. Nanoparticles are being used to create nanodrugs and probes to diagnose and treat a variety of diseases, including cancer. Nanomedicines have unique advantages, such as increased surface-to-volume ratios, which enable them to interact with, absorb, and deliver small biomolecules to a very specific target, thereby improving the effectiveness of both probes and drugs. Nanoprobe biotechnology also plays an important role in the discovery of novel cancer biomarkers, and nanoprobes have become an important part of early clinical diagnosis of cancer. Various organic and inorganic nanomaterials have been developed as biomolecular carriers for the detection of disease biomarkers. Thus, we designed this review to evaluate the advances in nanoprobe technology in tumor diagnosis.
Cancer is currently one of the leading causes of death worldwide, with the number of cancer patients expected to increase over the next 50 years as demographic changes, such as population aging and growth, strongly influence cancer incidence and trends across different regions. Assuming that the latest incidence trends of major cancer types continue, the combined incidence of all cancers would double by 2070 (1). Cancer is often fatal, with early diagnosis generally acting as the deciding factor in therapeutic response. Thus, novel cancer prevention strategies and diagnostic tools must be developed to effectively reduce the number of future cancer cases and save more cancer patients.
Cancer is characterized by abnormal cell differentiation and proliferation, uncontrolled growth, invasion, and metastasis, and its occurrence is a complex multi-factor and multi-step process (2). Earlier cancer detection improves survival rate; however, about 50% of cancers are already in advanced stages of pathogenesis at their time of diagnosis (3–5). Early detection of cancer or precancerous lesions allows early intervention attempting to slow or prevent cancer progression and mortality. In addition to a better understanding of risk susceptibility for certain cancers, the biology and trajectory of precancerous and early cancer lesions must be assessed in order to identify secondary diseases that may require intervention. These efforts are best accelerated by early detection research translated into sensitive and specific early detection technologies (6). This need is supported by the observations following the COVID-19 pandemic, which has had a significant impact on cancer patients across the globe. Pandemic responses have resulted in delayed diagnosis and disruptions in treatment and follow-up care, increasing overall infection rates and premature deaths (7–9). This has highlighted the need to reduce the delays in cancer diagnosis associated with traditional diagnostic models and to address the inaccuracy and disruptions in diagnosis caused by COVID-19 (10). In this review, we aim to explore the application of tumor nanotechnology in tumor diagnosis.
Nanomedicine is an emerging science often applied in cancer therapy, as it is characterized by tumor-specific drug delivery, conferring a significant therapeutic advantage over traditional interventions (11–15). The integration of imaging and nanoprobes for cancer diagnosis and treatment may facilitate better responses and reduced side effects in normal tissues (14, 16, 17). This means that nano-biosensors are likely to be critical to the development of novel cancer therapies and diagnostics, as they can be used to detect cancer biomarkers, map cancer cells, and monitor metastasis in response to different substrates and conditions (18, 19). Thus, the cancer diagnosis and treatment landscape has expanded considerably over the last decade, propelled by advances in novel therapies and improved diagnostics (14, 16, 17, 20). This recent success in nanomedicine research has also paved the way for accurate diagnosis through the interaction of nanoprobes with specific biological systems (21, 22).
Optical tumor detection is becoming more and more common in biomedical research, but its limitations, including light penetration depth and signal attenuation in tissues, need to be overcome (23). Therefore, different optical imaging methods using nanoprobe technology have been developed for application in the fields of fluorescence, phosphorescence, and photoacoustic (PA) imaging. These methods are more sensitive than traditional techniques, providing higher resolution images and making it easier to get information pointing to anoxic areas.
Fluorescent probes are most common in optical sensors. Fluorescent probes bound to selected nanocarriers can produce dense hydrophobic aggregates following self-assembly in aqueous solutions. The probes can be activated by stimulus-specific “off-on” activation, improving the signal-to-noise ratio within the region of interest, resulting in extremely high sensitivity and increased resolution. Many of these probes are used to evaluate multiple tissues and subcellular structures (24).
Aggregation-caused quenching (ACQ) refers to the strong coupling reactions between ground state fluorophores that form a stable non-influenza fluorescence complex with a unique absorption spectrum (25), which is a typical activatable design. Yang et al. (26) took advantage of this simplicity to develop a scalable hypoxic-responsive human serum albumin-triggered nanosystem, consisting of human serum albumin, the near-infrared imaging photosensitizer chlore6, an oxaliplatin precursor, and a hypoxic-sensitive linker, azobenzene 4,4 ‘dicarboxylic acid. When exposed to a hypoxic tumor microenvironment, the nanosystem is cleaved by nitrogen reductase, breaking up the ultra-small human serum albumin aggregates and restoring chlore6 fluorescence, facilitating clear hypoxic imaging (27). Although the ACQ probe is simple to use, the quenching state of the dye largely depends on the assembly state of the nanocarrier. Thus, the status of the ACQs in any given carrier may be influenced by a variety of in vivo factors, including protein binding and enzyme degradation, which may lead to decreased selectivity and specificity for tumors.
In addition to general activatable designs, imaging strategies targeting tumor biomarkers via in situ luminescence can also be used to effectively image tumors in vivo. For example, the HIF-1α-induced transmembrane protein CAIX, which is a biomarker for hypoxic environments, is widely expressed on the surface of hypoxic cells (28). Huang et al. (29) evaluated the application of a CAIX-specific IRDye 800CW probe (CAIX-800) for hypoxia detection in a mouse model of orthotopic nasopharyngeal carcinoma (NPC). Their data revealed that a combination of this dye and fluorescence molecular tomography or computed tomography could be used to accurately locate early-stage NPC tumors, with detection as early as 2 weeks. Lymph node metastases from advanced NPC (6 weeks) were then observed using multispectral PA imaging. Taken together, the results of this study show that molecular conjugation combining appropriate targeting groups and near-infrared dyes can facilitate the selective imaging of specific anoxic analytes in vivo.
In addition, anaerobe integration in nanocarriers can also facilitate tumor targeting, as these microbes can only survive in anoxic environments; thus, their inclusion would force aggregation to the hypoxic areas of the tumor. In addition, bacterial migration to hypoxic tumors can also be facilitated by external stimulation. F. Chen et al. (30) attached lipid nanoparticles loaded with indocyanine green to bacterial surfaces to target and ablate hypoxic tumors through photothermal therapy. Fluorescent probes based on nanocarriers are used more and more frequently in tumor diagnosis.
Following photoexcitation, an excited electron passes through the excited triplet state between the systems, inducing a spin transition, which returns a singlet to the ground state and emits photons in the form of phosphorescence. Phosphorescence can then be converted to pO2 by calibrating the probe using the Sterne-Volmer equation. Several research groups have gone on to develop phosphorescence probes for various applications, including direct pO2 measurement (31, 32). Phosphorescent probes have the advantages of high spatial resolution and direct and reversible pO2 quantitative analysis. Yoshihara et al. (33) reported that Ru (II) complexes could be coupled with oligodeoxy nucleotides containing pyrene and nitroimidazole ligands, and that the hydrophilicity of the modified metal complex molecules could facilitate the induction of nanoaggregates, which could then be used to support qualitative analysis of tumor burden. In addition, Liu et al. (34) recently combined complementary imaging technology with nanoparticles to achieve high-quality, reliable, and quantitative hypoxia detection in several different cancer models. In this study, the research team encapsulated benzene-substituted Pd (II) porphyrin (PdTPTBP) into dSPE-PEG 2K phospholipid micelles (Pd-) in MX, to produce a phosphorescent nanoprobe, which could then be applied as a time-resolved lifetime imaging system. By combining the wide field of view of luminescent lifetime imaging with O2-sensitive nanoprobes, this group were able to quantify O2 localization in tumors and thus identify hotspots for likely tumor formation.
PA tomography may be another potential approach for addressing the limitations of modern imaging associated with maximum tissue penetration depth. This system works by converting excited light energy to heat/sound energy, facilitating a significant increase in penetration depth while maintaining near-microscopic spatial resolution. PA signals are generated by photon absorption, which causes rapid thermoelastic expansion and sound wave propagation. These pressure waves can be detected by transducers, and PA images can then be produced. These images are characterized by improved tissue penetration depth (35). A recent study (36) used endogenous PA imaging to detect tumor hypoxia in a multicancer model, providing anatomical and functional information on hypoxia. Knox et al. (37) developed a hyper-1-based hypoxic response probe for radiographic PA imaging, which utilized the aza-Bodipy platform and a dialkyl aniline substitutive group, facilitating further oxidation of the system to produce the single n-oxide probe RHP-1. Probe evaluations revealed a two-fold increase in PA 820 nm/PA 770 nm emissions when these sensors were placed under hypoxic conditions. Furthermore, in vivo PA imaging revealed that the hypoxic regions of the tumor could be mapped for 3D reconstruction when using a RHP-1 probe. Recently, M. Chen et al. (38) applied the bioreducible N-oxide hypoxia-sensitive probe Hyper-650 with enhanced molar absorption to high resolution PA microscopy. This system facilitated the simultaneous and constant monitoring of vascular sO2 and tissue oxygenation, following its combination with endogenous contrast agents. These images revealed that the identified tumor hypoxia center signals were consistent with those identified using traditional sO2 imaging, supporting their clinical value.
The tumor microenvironment is characterized by gradual changes in both spatial and temporal heterogeneity, which can be used to explore its diagnostic application in tumors. Fluorescent imaging probes responsive to various conditions, including hypoxia, pH, and protease expression, may be used to evaluate and diagnose specific tumor conditions (39–42). In precision medicine, molecular imaging is primarily used to identify cancer-specific targets, design therapeutic methods, and monitor drug administration responses. DCs, for example, are the most adept antigen-presenting cells, transmitting information to the cells in the adaptive immune system (27, 28). Accurate antigen delivery and effective activation of immune pathways are key to the development of DC anticancer vaccines and successful immunotherapy. However, DC viability, function, and their ability to migrate in vivo remain unknown. Superparamagnetic iron oxide (SPION) is an excellent MRI contrast agent that can be easily absorbed by DCs and tracked using MRI. Thus, MRI using SPIONs can provide more anatomical information and more detailed visualization than other methods, but at lower sensitivity (43, 44). To overcome this limitation Y.C. Chen et al. (45) pioneered the development of a novel dual-mode nanoprobe, SPIONIR797, for tracking DC migration in vivo and detection via a non-invasive combined method. Thus, leveraging the advantages of high sensitivity, high spatial resolution, and relatively simple operation, it is becoming increasingly easy to visualize various diseases and use these imaging techniques to draw critical diagnostic conclusions. Many of these systems focus on a variety of disease models, including tumors, inflammation, and atherosclerosis.
In addition to the technologies described above, other research groups continue to work on a variety of alternatives. For example, gold, silver, and bimetallic and magnetic nanoparticles are widely used in the manufacture of sensing tools due to their unique optical properties and biocompatibility (22, 46–51). The functionalization of these nanoparticles with different components provides an excellent opportunity to assemble selective and sensitive sensing materials for the detection of various cancer-related biomolecules. This was exemplified in the Zhang et al. (52) study, where a multifunctional ASnFAp : Gd/Tb system was synthesized using a new bionic strategy. Subsequent in vitro and in vivo experiments then confirmed improved tumor imaging and recognition ability, compared with conventional methods. The generated nanoparticles could be used as drug carriers for tumor imaging and treatment with good tumor recognition, treatment capacity, and superior biocompatibility. Thus, this study supports the very important potential clinical application of nanomaterials in diagnosis.
Given the complexity of the tumor microenvironment and the diversity of analytes that contribute to global hypoxia, there is currently no “gold standard” for measuring tumor hypoxia (53). Nevertheless, several novel methods designed to detect and evaluate tumor hypoxia have been developed, with several entering clinical evaluations. These include oxygen electrodes, electron paramagnetic resonance oximetry, positron emission tomography (PET), and more.
One example is the system created by de Georgia (54), which facilitates pO2 evaluation in invasive tumors via electrochemical probes. This system has been successfully employed in critical care settings focused on retaining nerve function. However, when the oxygen is completely consumed, the electrode undergoes electrochemical reduction, resulting in reduced signal sensitivity and increasing inaccurate readings. This system is also invasive and can lead to edema and hemorrhage in some tissues. Daimiel et al. (55) developed an alternative technique for repeatable quantitative pO2 measurement in tumors using electron paramagnetic resonance oximetry. This non-invasive method requires the use of a paramagnetic probe, which responds to the oxygen in any given environment and evaluates pO2 levels by measuring relaxation rates.
In addition, recent developments in 2-nitroimidazole-based radiotracers may facilitate an increase in PET application as an alternative non-invasive technique for detecting tumor hypoxia. Relatively short-lived radionuclides, such as 18F, can be easily bound via isotope exchange reactions to produce novel probes. Several studies have evaluated 18F LA-Beled FMISO, pentafluorinated etanidazole, hydrophilic fluoridazole, and arabinosfluorroazomycin as PET probes for hypoxia imaging (56).
However, the development of a new probe must always be followed by preclinical evaluation and scaled-up good manufacturing practices to facilitate clinical trials. Obstacles in each specific area must be overcome before probes can be incorporated into routine clinical practice. Despite all the immediate challenges of clinical transformation, nanoprobe imaging offers significant opportunities to provide improved non-invasive diagnostic tools. Combining optical imaging with other biomedical imaging methods may also facilitate multimodal imaging, providing even better tumor detection.
Nanoprobes have a unique set of physical advantages, including flexible biocompatibility and pharmacokinetics, and most present various unique nanoscale properties, providing more convenient tumor imaging. Nanostructures can also promote the production of organic dye aggregates. Thus, cancer diagnostic specialists have continued to focus on biomedical sensing and imaging. Nanosensors can also provide a protective substrate to prevent unnecessary interactions within the biological environment and improve in vivo circulation time and system delivery. With improved understanding of the basic physical phenomena resulting from nanoconstraints, nanosensors can be designed to facilitate both assembly and disassembly in response to specific physicochemical conditions, providing the possibility for the further development of “smart” responsive nanosensors.
In addition, the high degree of heterogeneity between and within tumors can result in complex diagnostic and evaluation issues, including a large discrepancy in imaging results (57). This highlights the importance of further studies on different tumor types. Considering the temporal and spatial variability of tumors, the development of sensors that can accurately track treatment dynamics in real time is a promising concept. Thus, we believe that nanoprobe sensors are likely to play a fundamental role in our understanding of tumors and are almost guaranteed to become increasingly important in the detection and treatment of various cancers due to their physical, pharmacokinetic, and nanoscopic properties.
All authors contributed to the design of the study and writing of the manuscript. QZ, KH, and HC undertook the research. YW and NZ wrote the main manuscript text. QZ and YW revised the article critically for important intellectual content and granted final approval of the submitted version. All authors reviewed the manuscript.
The authors declare that the research was conducted in the absence of any commercial or financial relationships that could be construed as a potential conflict of interest.
All claims expressed in this article are solely those of the authors and do not necessarily represent those of their affiliated organizations, or those of the publisher, the editors and the reviewers. Any product that may be evaluated in this article, or claim that may be made by its manufacturer, is not guaranteed or endorsed by the publisher.
ACQ, Aggregation-caused quenching; NPC, nasopharyngeal carcinoma; PA, Photoacoustic; PET, Positron emission tomography; SPION, Superparamagnetic iron oxide
1. Soerjomataram I, Bray F. Planning for Tomorrow: Global Cancer Incidence and the Role of Prevention 2020-2070. Nat Rev Clin Oncol (2021) 18(10):663–72. doi: 10.1038/s41571-021-00514-z
2. Yang Y, Huang Q, Xiao Z, Liu M, Zhu Y, Chen Q, et al. Nanomaterial-Based Biosensor Developing as a Route Toward In Vitro Diagnosis of Early Ovarian Cancer. Mater Today Bio (2022) 13:100218. doi: 10.1016/j.mtbio.2022.100218
3. Crosby D, Bhatia S, Brindle KM, Coussens LM, Dive C, Emberton M, et al. Early Detection of Cancer. Science (2022) 375(6586):eaay9040. doi: 10.1126/science.aay9040
4. Hricak H, Ward ZJ, Atun R, Abdel-Wahab M, Muellner A, Scott AM. Increasing Access to Imaging for Addressing the Global Cancer Epidemic. Radiology (2021) 301(3):543–6. doi: 10.1148/radiol.2021211351
5. Liu Y, Ai K, Lu L. Nanoparticulate X-Ray Computed Tomography Contrast Agents: From Design Validation to In Vivo Applications. Acc Chem Res (2012) 45(10):1817–27. doi: 10.1021/ar300150c
6. Wang J, Sui L, Huang J, Miao L, Nie Y, Wang K, et al. MoS2-Based Nanocomposites for Cancer Diagnosis and Therapy. Bioact Mater (2021) 6(11):4209–42. doi: 10.1016/j.bioactmat.2021.04.021
7. Hesary FB, Salehiniya H. The Impact of the COVID-19 Epidemic on Diagnosis, Treatment, Concerns, Problems, and Mental Health in Patients With Gastric Cancer. J Gastrointest Cancer (2021) 1–8. doi: 10.1007/s12029-021-00692-0
8. Tuech JJ, Gangloff A, Di Fiore F, Benyoucef A, Michel P, Schwarz L. The Day After Tomorrow: How Should We Address Health System Organization to Treat Cancer Patients After the Peak of the COVID-19 Epidemic? Oncology (2020) 98(12):827–35. doi: 10.1159/000509650
9. Huynh I. When COVID-19 Saves Lives: Accidental Cancer Diagnosis in an Epidemic Context. Ethics Med Public Health (2021) 18:100666. doi: 10.1016/j.jemep.2021.100666
10. Parihar A, Singhal A, Kumar N, Khan R, Khan MA, Srivastava AK. Next-Generation Intelligent MXene-Based Electrochemical Aptasensors for Point-Of-Care Cancer Diagnostics. Nanomicro Lett (2022) 14(1):100. doi: 10.1007/s40820-022-00845-1
11. Zhu D, Liu Z, Li Y, Huang Q, Xia L, Li K. Delivery of Manganese Carbonyl to the Tumor Microenvironment Using Tumor-Derived Exosomes for Cancer Gas Therapy and Low Dose Radiotherapy. Biomaterials (2021) 274:120894. doi: 10.1016/j.biomaterials.2021.120894
12. Zeng H, Li J, Hou K, Wu Y, Chen H, Ning Z. Melanoma and Nanotechnology-Based Treatment. Front Oncol (2022) 12:858185. doi: 10.3389/fonc.2022.858185
13. Qi P, Zhang J, Bao Z, Liao Y, Liu Z, Wang J. A Platelet-Mimicking Single-Atom Nanozyme for Mitochondrial Damage-Mediated Mild-Temperature Photothermal Therapy. ACS Appl Mater Interfaces (2022) 14(17):19081–90. doi: 10.1021/acsami.1c22346
14. Huang C, Liu Z, Chen M, Du L, Liu C, Wang S, et al. Tumor-Derived Biomimetic Nanozyme With Immune Evasion Ability for Synergistically Enhanced Low Dose Radiotherapy. J Nanobiotechnol (2021) 19(1):457. doi: 10.1186/s12951-021-01182-y
15. Zhao T, Wu W, Sui L, Huang Q, Nan Y, Liu J, et al. Reactive Oxygen Species-Based Nanomaterials for the Treatment of Myocardial Ischemia Reperfusion Injuries. Bioact Mater (2022) 7:47–72. doi: 10.1016/j.bioactmat.2021.06.006
16. Huang C, Ding S, Jiang W, Wang FB. Glutathione-Depleting Nanoplatelets for Enhanced Sonodynamic Cancer Therapy. Nanoscale (2021) 13(8):4512–8. doi: 10.1039/d0nr08440a
17. Huang C, Zhu C, Chen J, Huang K, Li F, Ding S, et al. Nano-Platelets as an Oxygen Regulator for Augmenting Starvation Therapy Against Hypoxic Tumor. Front Bioeng Biotechnol (2020) 8:571993. doi: 10.3389/fbioe.2020.571993
18. Shahbazi N, Zare-Dorabei R, Naghib SM. Multifunctional Nanoparticles as Optical Biosensing Probe for Breast Cancer Detection: A Review. Mater Sci Eng C Mater Biol Appl (2021) 127:112249. doi: 10.1016/j.msec.2021.112249
19. Mohammadpour Z, Majidzadeh AK. Applications of Two-Dimensional Nanomaterials in Breast Cancer Theranostics. ACS Biomater Sci Eng (2020) 6(4):1852–73. doi: 10.1021/acsbiomaterials.9b01894
20. Huang C, Wang FB, Liu L, Jiang W, Liu W, Ma W, et al. Hypoxic Tumor Radiosensitization Using Engineered Probiotics. Adv Healthc Mater (2021) 10(10):e2002207. doi: 10.1002/adhm.202002207
21. Kundu P, Singh D, Singh A, Sahoo SK. Cancer Nanotheranostics: A Nanomedicinal Approach for Cancer Therapy and Diagnosis. Anticancer Agents Med Chem (2020) 20(11):1288–99. doi: 10.2174/1871520619666190820145930
22. Xiao Z, Huang Q, Yang Y, Liu M, Chen Q, Huang J, et al. Emerging Early Diagnostic Methods for Acute Kidney Injury. Theranostics (2022) 12(6):2963–86. doi: 10.7150/thno.71064
23. Ntziachristos V. Going Deeper Than Microscopy: The Optical Imaging Frontier in Biology. Nat Methods (2010) 7(8):603–14. doi: 10.1038/nmeth.1483
24. Shashkova S, Leake MC. Single-Molecule Fluorescence Microscopy Review: Shedding New Light on Old Problems. Biosci Rep (2017) 37(4):BSR20170031. doi: 10.1042/BSR20170031
25. Luby BM, Walsh CD, Zheng G. Advanced Photosensitizer Activation Strategies for Smarter Photodynamic Therapy Beacons. Angew Chem Int Ed Engl (2019) 58(9):2558–69. doi: 10.1002/anie.201805246
26. Yang G, Phua SZF, Lim WQ, Zhang R, Feng L, Liu G, et al. A Hypoxia-Responsive Albumin-Based Nanosystem for Deep Tumor Penetration and Excellent Therapeutic Efficacy. Adv Mater (2019) 31(25):e1901513. doi: 10.1002/adma.201901513
27. Cui D, Huang J, Zhen X, Li J, Jiang Y, Pu K. A Semiconducting Polymer Nano-Prodrug for Hypoxia-Activated Photodynamic Cancer Therapy. Angew Chem Int Ed Engl (2019) 58(18):5920–4. doi: 10.1002/anie.201814730
28. Tafreshi NK, Lloyd MC, Proemsey JB, Bui MM, Kim J, Gillies RJ, et al. Evaluation of CAIX and CAXII Expression in Breast Cancer at Varied O2 Levels: CAIX is the Superior Surrogate Imaging Biomarker of Tumor Hypoxia. Mol Imaging Biol (2016) 18(2):219–31. doi: 10.1007/s11307-015-0885-x
29. Huang W, Wang K, An Y, Meng H, Gao Y, Xiong Z, et al. In Vivo Three-Dimensional Evaluation of Tumour Hypoxia in Nasopharyngeal Carcinomas Using FMT-CT and MSOT. Eur J Nucl Med Mol Imaging (2020) 47(5):1027–38. doi: 10.1007/s00259-019-04526-x
30. Chen F, Zang Z, Chen Z, Cui L, Chang Z, Ma A, et al. Nanophotosensitizer-Engineered Salmonella Bacteria With Hypoxia Targeting and Photothermal-Assisted Mutual Bioaccumulation for Solid Tumor Therapy. Biomaterials (2019) 214:119226. doi: 10.1016/j.biomaterials.2019.119226
31. O'Riordan TC, Fitzgerald K, Ponomarev GV, Mackrill J, Hynes J, Taylor C, et al. Sensing Intracellular Oxygen Using Near-Infrared Phosphorescent Probes and Live-Cell Fluorescence Imaging. Am J Physiol Regul Integr Comp Physiol (2007) 292(4):R1613–20. doi: 10.1152/ajpregu.00707.2006
32. Cheng MHY, Mo Y, Zheng G. Nano Versus Molecular: Optical Imaging Approaches to Detect and Monitor Tumor Hypoxia. Adv Healthc Mater (2021) 10(2):e2001549. doi: 10.1002/adhm.202001549
33. Yoshihara K, Takagi K, Son A, Kurihara R, Tanabe K. Aggregate Formation of Oligonucleotides That Assist Molecular Imaging for Tracking of the Oxygen Status in Tumor Tissue. Chembiochem (2017) 18(16):1650–8. doi: 10.1002/cbic.201700116
34. Liu Y, Gu Y, Yuan W, Zhou X, Qiu X, Kong M, et al. Quantitative Mapping of Liver Hypoxia in Living Mice Using Time-Resolved Wide-Field Phosphorescence Lifetime Imaging. Adv Sci (Weinh) (2020) 7(11):1902929. doi: 10.1002/advs.201902929
35. Li M, Tang Y, Yao J. Photoacoustic Tomography of Blood Oxygenation: A Mini Review. Photoacoustics (2018) 10:65–73. doi: 10.1016/j.pacs.2018.05.001
36. Ron A, Dean-Ben XL, Gottschalk S, Razansky D. Volumetric Optoacoustic Imaging Unveils High-Resolution Patterns of Acute and Cyclic Hypoxia in a Murine Model of Breast Cancer. Cancer Res (2019) 79(18):4767–75. doi: 10.1158/0008-5472.CAN-18-3769
37. Knox HJ, Kim TW, Zhu Z, Chan J. Photophysical Tuning of N-Oxide-Based Probes Enables Ratiometric Photoacoustic Imaging of Tumor Hypoxia. ACS Chem Biol (2018) 13(7):1838–43. doi: 10.1021/acschembio.8b00099
38. Chen M, Knox HJ, Tang Y, Liu W, Nie L, Chan J, et al. Simultaneous Photoacoustic Imaging of Intravascular and Tissue Oxygenation. Opt Lett (2019) 44(15):3773–6. doi: 10.1364/OL.44.003773
39. Wu Y, Zhang W, Li J, Zhang Y. Optical Imaging of Tumor Microenvironment. Am J Nucl Med Mol Imaging (2013) 3(1):1–15.
40. Li Y, Shen B, Zou G, Hu R, Pan Y, Qu J, et al. Super-Multiplex Nonlinear Optical Imaging Unscrambles the Statistical Complexity of Cancer Subtypes and Tumor Microenvironment. Adv Sci (Weinh) (2022) 9(5):e2104379. doi: 10.1002/advs.202104379
41. Vakoc BJ, Lanning RM, Tyrrell JA, Padera TP, Bartlett LA, Stylianopoulos T, et al. Three-Dimensional Microscopy of the Tumor Microenvironment In Vivo Using Optical Frequency Domain Imaging. Nat Med (2009) 15(10):1219–23. doi: 10.1038/nm.1971
42. Penet MF, Krishnamachary B, Chen Z, Jin J, Bhujwalla ZM. Molecular Imaging of the Tumor Microenvironment for Precision Medicine and Theranostics. Adv Cancer Res (2014) 124:235–56. doi: 10.1016/B978-0-12-411638-2.00007-0
43. Long CM, van Laarhoven HW, Bulte JW, Levitsky HI. Magnetovaccination as a Novel Method to Assess and Quantify Dendritic Cell Tumor Antigen Capture and Delivery to Lymph Nodes. Cancer Res (2009) 69(7):3180–7. doi: 10.1158/0008-5472.CAN-08-3691
44. Kobukai S, Baheza R, Cobb JG, Virostko J, Xie J, Gillman A, et al. Magnetic Nanoparticles for Imaging Dendritic Cells. Magn Reson Med (2010) 63(5):1383–90. doi: 10.1002/mrm.22313
45. Chen YC, Wen S, Shang SA, Cui Y, Luo B, Teng GJ. Magnetic Resonance and Near-Infrared Imaging Using a Novel Dual-Modality Nano-Probe for Dendritic Cell Tracking In Vivo. Cytotherapy (2014) 16(5):699–710. doi: 10.1016/j.jcyt.2013.09.006
46. Lee SJ, Kim HJ, Huh YM, Kim IW, Jeong JH, Kim JC, et al. Functionalized Magnetic PLGA Nanospheres for Targeting and Bioimaging of Breast Cancer. J Nanosci Nanotechnol (2018) 18(3):1542–7. doi: 10.1166/jnn.2018.14220
47. Zong J, Cobb SL, Cameron NR. Peptide-Functionalized Gold Nanoparticles: Versatile Biomaterials for Diagnostic and Therapeutic Applications. Biomater Sci (2017) 5(5):872–86. doi: 10.1039/c7bm00006e
48. Azharuddin M, Zhu GH, Das D, Ozgur E, Uzun L, Turner APF, et al. A Repertoire of Biomedical Applications of Noble Metal Nanoparticles. Chem Commun (Camb) (2019) 55(49):6964–96. doi: 10.1039/c9cc01741k
49. Li X, Yang T, Lin J. Spectral Analysis of Human Saliva for Detection of Lung Cancer Using Surface-Enhanced Raman Spectroscopy. J BioMed Opt (2012) 17(3):37003. doi: 10.1117/1.JBO.17.3.037003
50. Ravalli A, Marrazza G. Gold and Magnetic Nanoparticles-Based Electrochemical Biosensors for Cancer Biomarker Determination. J Nanosci Nanotechnol (2015) 15(5):3307–19. doi: 10.1166/jnn.2015.10038
51. Li RD, Yin BC, Ye BC. Ultrasensitive, Colorimetric Detection of microRNAs Based on Isothermal Exponential Amplification Reaction-Assisted Gold Nanoparticle Amplification. Biosens Bioelectron (2016) 86:1011–6. doi: 10.1016/j.bios.2016.07.042
52. Zhang W, Zhou R, Yang Y, Peng S, Xiao D, Kong T, et al. Aptamer-Mediated Synthesis of Multifunctional Nano-Hydroxyapatite for Active Tumour Bioimaging and Treatment. Cell Prolif (2021) 54(9):e13105. doi: 10.1111/cpr.13105
53. Tatum JL, Kelloff GJ, Gillies RJ, Arbeit JM, Brown JM, Chao KS, et al. Hypoxia: Importance in Tumor Biology, Noninvasive Measurement by Imaging, and Value of its Measurement in the Management of Cancer Therapy. Int J Radiat Biol (2006) 82(10):699–757. doi: 10.1080/09553000601002324
54. De Georgia MA. Brain Tissue Oxygen Monitoring in Neurocritical Care. J Intensive Care Med (2015) 30(8):473–83. doi: 10.1177/0885066614529254
55. Daimiel I. Insights Into Hypoxia: Non-Invasive Assessment Through Imaging Modalities and Its Application in Breast Cancer. J Breast Cancer (2019) 22(2):155–71. doi: 10.4048/jbc.2019.22.e26
56. Fleming IN, Manavaki R, Blower PJ, West C, Williams KJ, Harris AL, et al. Imaging Tumour Hypoxia With Positron Emission Tomography. Br J Cancer (2015) 112(2):238–50. doi: 10.1038/bjc.2014.610
Keywords: nanomaterial, nanotechnology, cancer, diagnosis, nanotech probes
Citation: Zhang Q, Hou K, Chen H, Zeng N and Wu Y (2022) Nanotech Probes: A Revolution in Cancer Diagnosis. Front. Oncol. 12:933125. doi: 10.3389/fonc.2022.933125
Received: 30 April 2022; Accepted: 14 June 2022;
Published: 07 July 2022.
Edited by:
Kelong Ai, Central South University, ChinaCopyright © 2022 Zhang, Hou, Chen, Zeng and Wu. This is an open-access article distributed under the terms of the Creative Commons Attribution License (CC BY). The use, distribution or reproduction in other forums is permitted, provided the original author(s) and the copyright owner(s) are credited and that the original publication in this journal is cited, in accordance with accepted academic practice. No use, distribution or reproduction is permitted which does not comply with these terms.
*Correspondence: Yiping Wu, d3V5aXBpbmd0akAxNjMuY29t; Ning Zeng, emVuZ25pbmd3aHRqQDE2My5jb20=
†These authors have contributed equally to this work
Disclaimer: All claims expressed in this article are solely those of the authors and do not necessarily represent those of their affiliated organizations, or those of the publisher, the editors and the reviewers. Any product that may be evaluated in this article or claim that may be made by its manufacturer is not guaranteed or endorsed by the publisher.
Research integrity at Frontiers
Learn more about the work of our research integrity team to safeguard the quality of each article we publish.