- Department of Aerospace and Mechanical Engineering, University of Notre Dame, Notre Dame, IN, United States
In this Perspective, we provide our insights and opinions about the contribution—and potential co-regulation—of mechanics and metabolism in incurable breast cancer brain metastasis. Altered metabolic activity can affect cancer metastasis as high glucose supply and demand in the brain microenvironment favors aerobic glycolysis. Similarly, the altered mechanical properties of disseminating cancer cells facilitate migration to and metastatic seeding of the brain, where local metabolites support their progression. Cancer cells in the brain and the brain tumor microenvironment often possess opposing mechanical and metabolic properties compared to extracranial cancer cells and their microenvironment, which inhibit the ease of extravasation and metastasis of these cells outside the central nervous system. We posit that the brain provides a metabolic microenvironment that mechanically reinforces the cellular structure of cancer cells and supports their metastatic growth while restricting their spread from the brain to external organs.
Introduction
Cancer genetic and metabolic aberrations are linked via oncogenes and tumor suppressors that play a key role in cell metabolism (1–3). They largely affect three major metabolic pathways: aerobic glycolysis, glutaminolysis, and one-carbon metabolism (4–8). These alterations make it possible for cancer cells to transition from simple adenosine triphosphate (ATP) production to the generation of large quantities of nucleotides, fatty acids, amino acids, and other intermediates necessary for rapid mitosis, proliferation, and cell growth (9–11). Cancer cells can even alter their metabolic programs to maintain cell-autonomous proliferation in the often nutrient-poor conditions of the tumor microenvironment (12).
Considering that cellular energy metabolism greatly impacts neoplasia and can determine cell fate (e.g., proliferation versus apoptosis), high energy metabolism in the brain could be largely responsible for the propensity and aggressiveness of metastasis to and within that organ (13). The brain is a highly vascularized structure, and its cells are largely dependent on circulating glucose for energy production (14). Normal brain cells derive most of their energy from aerobic oxidation of glucose, while metastatic cancer cells possess metabolic flexibility and depend not only on glucose for energy but also on glutamine and acetate, irrespective of their origin or subtype (8). This metabolic adaptation promotes the rapid growth of cancer in the brain (8).
Besides metabolism, the brain is also mechanically distinct from other organs. The increased energy demand in the brain tumor microenvironment supports hyper-vascularization and a leaky blood–brain barrier, which causes increased fluid pressure and increased shear stress within the tumor microenvironment (15, 16). The mechanical properties of brain tissue, such as its stiffness (i.e., Young’s modulus), are lower compared to other tissues in the body. This is in part due to the increased fluid pressure from leaky vasculature and excessive loss of cell mass in the brain caused by the tumor and chronic inflammation (16–19).
In brain tumors, there is a relatively elevated production of some ECM proteins (proteoglycans, hyaluronic acid, glycosaminoglycans, and collagen), which increase tumor stiffness and play an important role in tumor progression (20, 21). This is in part due to the increased metabolic stress and elevated YAP-TAZ signaling (20, 21). But unlike extracranial cancers that are typically stiffer than their host tissue, primary brain tumors (e.g., glioblastoma) are often more compliant than their surrounding tissues, as shown in Table 1 (17). Mechanical forces are also strikingly altered in the presence of a brain tumor (28). For example, edema, which is a commonly observed clinical pathology, is a result of excess fluid pressure in and around brain tumors (29). Solid stress—exerted by growth-induced forces—from brain tumors is exerted not only within the tumor mass itself but externally as well, compressing the surrounding normal tissue, thereby reducing perfusion and inducing neuronal loss (30–32). These mechanical stresses are believed to be a major cause of the clinical symptoms seen in brain cancer patients (31).
Cancer cells themselves have also altered physical properties; their mechanical integrity tends to be lower than that of normal cells in the brain and decreases with increasing tumor progression and metastasis (33–37). Tumorigenesis, for example, induces actin cortex remodeling, which in turn makes cancerous cells softer—a key advantage for uncontrolled division, migration, and infiltration. Reduced cell membrane stiffness enhances the ability of cancer cells to migrate from the primary tumor to secondary sites, with each organ hosting its own unique mechanical properties and forces (38).
In this Perspective, we explore how the brain microenvironment regulates unique relationships between metabolism and mechanics (Figure 1), both at the cellular and tissue levels. We propose that exploring mechano-metabolic interplay may reveal new targetable vulnerabilities.
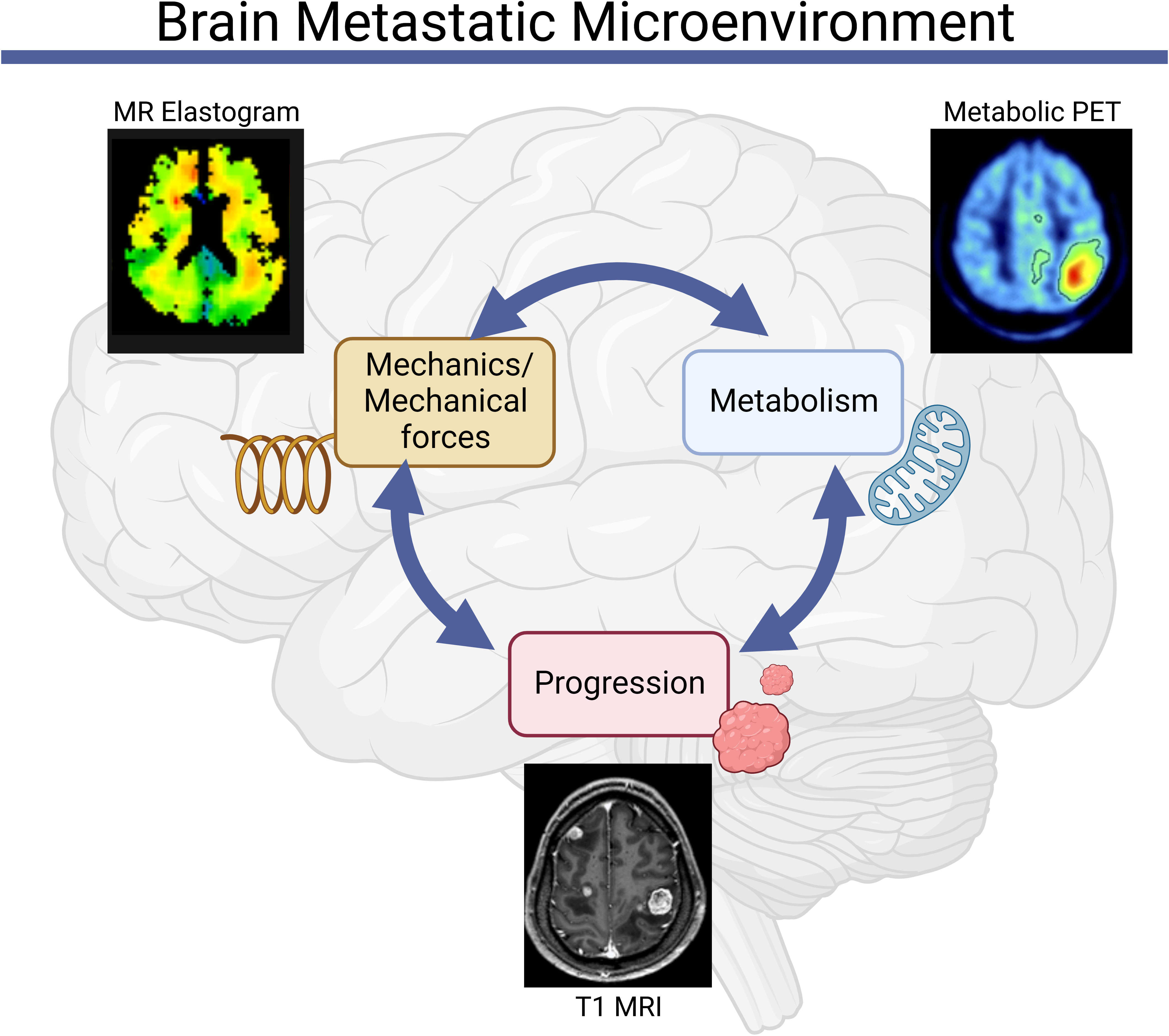
Figure 1 Mechanics and metabolism are linked in the brain metastatic microenvironment. Abnormalities in tissue and cell-scale physical properties influence and are influenced by metabolic processes, both of which can contribute to the initiation and progression of brain metastases [Clinical images reproduced from (39–41)].
Cancer mechanics and metabolism in the brain
Mechanical signals from the brain and tumor microenvironment (TME) modulate cell and tumor mechanics and influence cell metabolism to promote the aggressiveness of cancer (42, 43). Cancer cells mechanically interact with their environment via mechanotransduction by converting mechanical cues into biochemical outputs (44). Mechanical stresses—sensed through conserved mechanotransduction pathways—can alter the metabolism and behavior of cancer cells and can cause cancer cells to attain stem-like properties, thus driving cancer progression and metastasis (42). Mechanotransduction also leads to cytoskeletal reorganization and changes in cell stiffness, another important player in the mechanical–metabolic feedback system. As shown in Table 2, several other factors influence the mechanical–metabolic feedback system in the body.
In most cancers, the mechanically and spatially heterogeneous TME induces metabolic alterations, facilitating cancer cells to dynamically tune energy generation in response to fluctuating energy needs (45). Proliferation rates and propensity for migration are higher in cancerous cells than in normal cells, which requires more energy and allows them to preferentially respond to environments with higher nutrient and energy production (46, 47). Mechanotransduction provides signals to control cell proliferation, differentiation, and death and requires the metabolism of nutrients for both energy generation and biosynthesis of macromolecules (48). Not only do these functions depend on cell mechanics, but they also largely depend on the mechanical properties and functions of the extracellular matrix (ECM) (44).
In tumors, ECM stiffness is largely governed by the deposition and crosslinking of collagen as well as the presence of hyaluronic acid (49–52). Many cell activities are influenced by the properties of the ECM, including metabolic reprogramming (47). The mechanical properties of individual single cells and the sensing of external forces induce metabolic changes in the cells, which in turn regulate cell and tissue mechanics (e.g., cytoskeletal changes, ECM production) (53). For example, normal cells in softer ECM (such as the brain, which lacks collagen and other stiffening molecules) have fewer bundled actin fibers than those near stiff ECM structures (54). Cells within soft ECM facilitate optimal glycolysis by mediating TRIM21 (tripartite motif containing-21, a ubiquitin ligase) and inducing subsequent degradation of phosphofructokinase (PFK), a rate-limiting glycolytic enzyme (54). In contrast, cells surrounded by stiff ECM have increased cell-surface tension, which has promoted highly bundled actin fibers that entrap TRIM21, rendering it inactive and increasing the rate of glycolysis (54). In most cancers, increasing ECM stiffness upregulates the number of glucose transport proteins in the cell membrane, increases glycolytic enzymes and glycose synthase activity, induces the expression of gluconeogenic genes, and enhances the pentose phosphate pathway, all of which increase cancer cell metabolism (47). Thus, changes in cell and/or ECM stiffness—influenced by microenvironmental factors and mechanobiological signaling—play an important role in cell growth, proliferation, migration, and malignancy (36, 55, 56).
While ECM stiffness and cell–ECM adhesion are important regulators of tumor cell invasion, ECM degradation through enzymes such as matrix metalloproteinases (MMPs) is also critical for cell migration (57). The acidic tumor microenvironment resulting from overactive cell metabolism is favorable for MMP activation (58). The resulting metabolic shift toward aerobic glycolysis in cancer cells supports the production of MMP2, for example, which can help clear a path through the ECM (58). Thus, metabolism contributes to protease-enabled cell migration.
The transcriptional regulators YAP and TAZ are largely responsible for the effects of ECM stiffness on cellular glucose metabolism (47). They integrate mechanical cues and responses to soluble signals and metabolic pathways to control several aspects of cell behavior, including proliferation and migration. In normal development, the Hippo pathway serves to regulate YAP/TAZ activity to control cell proliferation and stemness. Mechanosensory processes are integrated into the Hippo pathway, linking mechanical stress to the transcriptional response of the cell. For example, stiffened ECM causes actin polymerization within the cell, which inhibits the downstream Hippo pathway, allowing YAP/TAZ to migrate to the nucleus and function as transcription factors to promote proliferation (59). YAP/TAZ activation also regulates metabolic processes, promoting aerobic glycolysis and responding to local glucose levels (60). In breast tumors, YAP activation drives cancer growth and metastasis (61). YAP, which is found to be localized in the nucleus, is highly expressed in breast cancer tissues and increases metabolic transcriptional activity in breast cancer cells (62). Hence, aberrant YAP/TAZ activation can specifically promote cancer cell metastasis, such as breast cancer brain metastases (63, 64).
In contrast to ECM stiffness, cellular stiffness is primarily influenced by the structural composition of the cytoskeleton, i.e., increasing structural protein density (e.g., actin, keratins) can increase cell stiffness (37, 65). However, in response to the aberrant biomechanical tumor microenvironment, cancer cell cytoskeletal proteins undergo significant degradation and reduction, thus rendering these cells more mechanically compliant (37, 65–68). This is the case for most metastatic cancers (including breast cancers), where the rigidity of the cytoskeleton decreases with tumor progression, especially in highly metastatic cells (37, 65, 66).
Cell cytoskeletal composition and their dynamic alterations during motility can also contribute to metabolic alterations. The focal adhesion proteins activated during cell adhesion and detachment guide mitochondrial regulation and govern the rate of ATP production (48, 69). In the brain, for example, they can catabolize gamma-aminobutyric acid (GABA) to create nicotinamide adenine dinucleotide + hydrogen (NADH) for the support of biosynthetic processes for sustained proliferation and migration (8). Highly proliferative metastatic cancer cells with low adhesion, higher PI3K expression, and loss of PTEN tend to use alternative endogenous substrates for their metabolism and continued proliferation (8, 70). Thus, cytoskeletal protein polymerization and cell mechanics are intrinsically connected and can largely be affected by the microenvironment (53).
The phosphatidylinositol 3-kinase (PI3K) signaling pathway, which plays a role in the regulation of glucose metabolism and renders the cells dependent on high levels of glucose flux, is activated via integrin-mediated activation of focal adhesion kinase (FAK) (71–73). However, PI3K is dysregulated through various mechanisms, including loss or inactivation of the tumor suppressor PTEN, mutation or amplification of PI3K, and activation of tyrosine kinase growth factor receptors or oncogenes upstream of PI3K (73). PI3K is active in brain metastases, including those from breast cancer (71–73). Activation of the PI3K pathway initiates a cascade that results in the formation of new actin fibers and branching of existing fibers. This actin polymerization leads to protrusions at the cell membrane, such as lamellipodia and invadopodia. Mature invadopodia mediate cell interaction with and movement within their microenvironment and involve both cytoskeletal structures that facilitate cell movement and the delivery of matrix-degrading proteases to clear a path (74). There is also emerging evidence that cytoskeletal processes in turn regulate PI3K activity, potentially completing a positive feedback loop that results in cancer cell invasion (75).
In the brain, the mechanical properties of cancer cells and tumors in the metastatic environment are opposing (e.g., tumor versus host stiffness). Cellular stiffness is generally higher than that of the ECM, and the overall tumor generally softens with cancer progression (16). This undoubtedly affects and is affected by cell metabolism and may partially explain the comparatively low metastatic rate of cancerous cells from the supportive brain microenvironment to extracranial sites, particularly in the context of primary brain tumors (53, 76). We, therefore, hypothesize that due to increased glucose supply in the brain, metastatic cancer cells often migrate to the brain, while brain cancer cells preferentially invade locally rather than extravasate and metastasize to other parts of the body.
Cancer metabolism and metastasis in the brain
Cancer cells consume excess nutrients and energy compared with benign cells. The Warburg effect alters cancer cell metabolism by increasing glucose uptake and the fermentation of glucose to lactate (77, 78) (Figure 2). This process, known as aerobic glycolysis, is less efficient than the complete mitochondrial respiration cycle that occurs in normal cells, but it may provide comparable amounts of energy and even confer a survival advantage to cancer cells. In glioblastoma, for example, which is the most common and malignant primary adult brain tumor, a metabolic shift is observed toward aerobic glycolysis (13). Glioma cells adapt to maximize their ability to synthesize substrates for membrane lipids, nucleic acids, and proteins for increased proliferation and migration (13). In breast cancer brain metastases, fatty acid synthesis is elevated compared to the metabolism at extracranial sites, resulting in a site-specific metabolic dependency (79). A recent study by Parida et al. showed that brain-tropic Her2+ breast cancer cell metabolic diversity and plasticity shape their metastatic fitness (80). These cells outcompete proximate cells in the brain for glucose uptake, metabolize lactate, hinder immune surveillance, and successfully seed brain metastases (80). This metastatic process requires de novo serine synthesis to proliferate in the brain microenvironment due to reduced amino acid levels in the brain relative to the plasma (81). However, because biochemical factors such as oxygen and glucose influence cell migration and metabolism, a well-nourished brain promotes cancer cell seeding and invasion (82). Initial metabolic plasticity also supports metastasis to and survival in the brain, where cancer cells lose some of their metabolic flexibility compared to the primary tumor upon colonization (82).
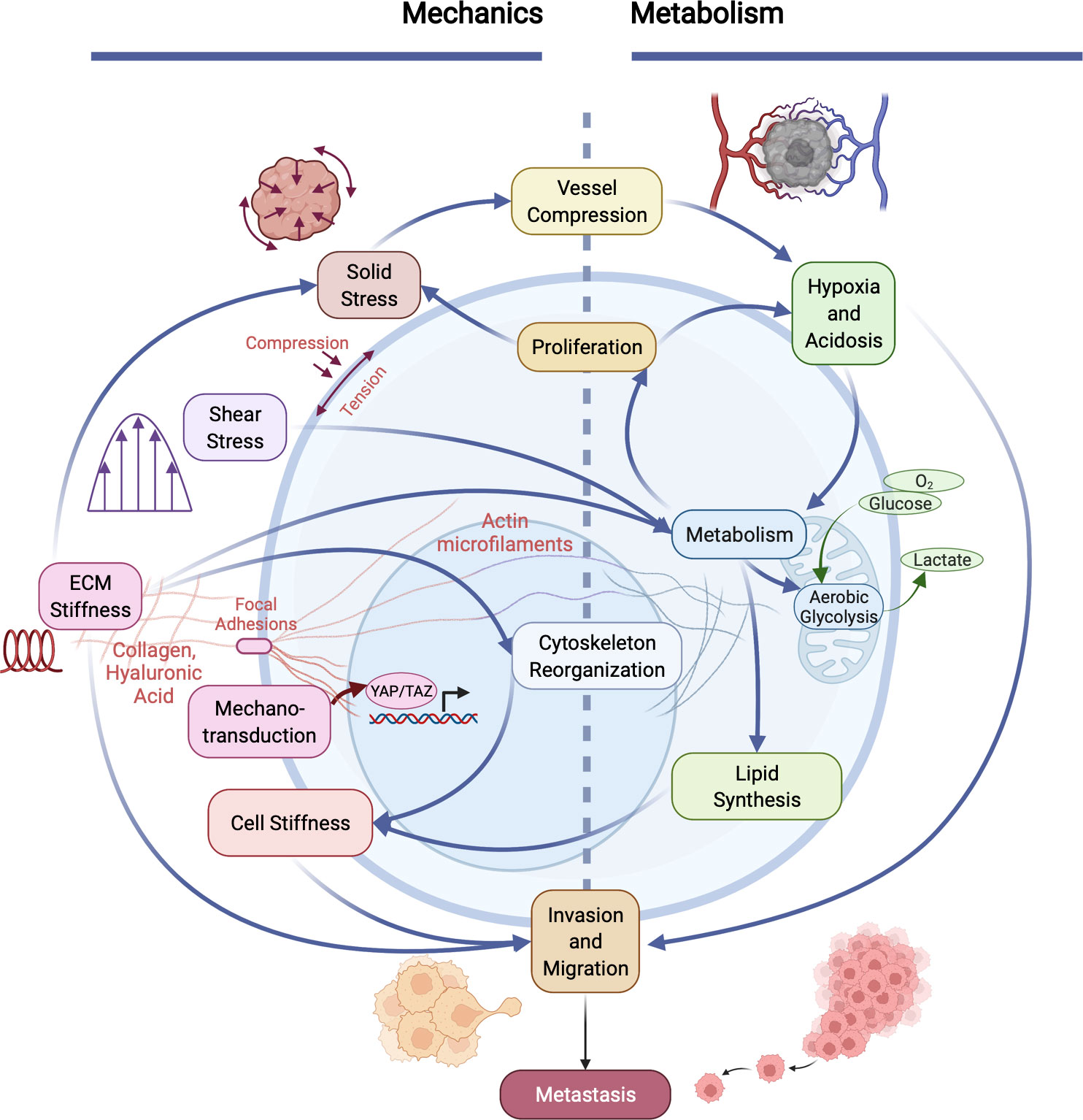
Figure 2 Cancer invasiveness and metastatic potential are regulated by the cooperation between aberrant cellular/tissue mechanics and altered metabolism in tumors. The physical and metabolic characteristics of tumors and their microenvironment interact in many distinct ways. Heightened solid stress (compressive and tensile) compresses tumor blood vessels, exacerbating hypoxia and acidosis within the microenvironment, which influences metabolism. Increased cell metabolism (e.g., aerobic glycolysis and lactate production) enables cell proliferation, which in turn causes an increase in solid stress. Metabolism is also influenced by increased fluid shear stress and elevated ECM stiffness, which results in altered cytoskeletal organization and reduced cancer cell membrane stiffness. Mechanical properties and forces are sensed via mechanotransduction pathways (e.g., focal adhesion kinase (FAK), resulting in translocation of YAP/TAZ to the nucleus which influences many cells physiological properties). Cell stiffness is also influenced by lipid metabolism in cancer cells. Together, co-regulated metabolic and mechanical alterations in cancer cells directly promote invasion and metastasis.
Intrinsic and extrinsic factors affect cancer metabolism and metastasis (8, 83). Intrinsically, mutations and changes in gene expression support metabolic shifts and can directly alter the levels or activity of metabolic enzymes within cancer cells (8). The loss of tumor suppressor genes such as phosphate and TENsin homolog deleted on chromosome 10 (PTEN) correlates with a significant increase in the risk of brain metastases in melanoma, breast, and lung cancer patients (83–86). Extrinsically, interactions with the extracellular matrix, surrounding cells, and available nutrients affect cell metabolism. For example, ECM alterations due to primary therapies (radiotherapies and chemotherapies) increase metabolism and energy production (ATP and GTP) and can create migratory tracts to promote intracranial tumor migration, invasion, and recurrence (87–89). Since about 20% of glucose-derived energy products in the body are consumed in the brain, the local rates of glucose supply and demand provide an ideal nutrient-rich environment to fuel the growth of primary and metastatic tumors (90). It has been recently shown that breast cancer metastases feature higher expression of glycolysis-related proteins (Glut-1, hexokinase II, CAIX, and MCT4) in the brain than in other organs (bone, liver, or lung) (91). Due to enhanced gluconeogenesis and glutamine oxidation (77), brain metastatic breast cancer cells have also been shown to develop the ability to survive and metastasize independently of glucose availability.
Breast cancer cells that metastasize to the brain have a genetic predisposition for adaptability and the ability to crosstalk with host cells, influencing de novo metabolic changes (92). For example, during early metastatic brain colonization, the blood–brain-barrier (BBB) is selectively disrupted via cancer cell trafficking and the inhibition of the docosahexaenoic acid (DHA) transporter expressed by endothelial cells. This loss can induce BBB leakage, reduce DHA transport, and alter metastatic lipid metabolism (83, 93). Because glutamine and glutamate are stored in the brain microenvironment, cancer cells also use these amino acids to support their continuous proliferation and biosynthesis of macromolecules (94). The PTEN pathway is suppressed in breast metastatic cells in the brain microenvironment by astrocytes via the activity of exosome-delivered miRNAs that inhibit PTEN expression, thus promoting tumor growth and progression (83). Hence, the metabolic soil of the brain is primed to support the growth of the metastatic cancer cell seeds (95).
Cancer cells also increase the synthesis of cholesterol, an integral part of the cell membrane (96). This is a common characteristic of breast cancer cells, as cholesterol must be constructed into new cell membranes in dividing cells (97). However, cholesterol synthesis is particularly upregulated in the brain, in part because cholesterol cannot cross the BBB (96). It has been shown that both tumor tissues and individual tumor cells have increased membrane cholesterol levels, across a range of cancer types (98). While there are contradictory findings on the effects of membrane cholesterol and cholesterol depletion in cancer cells, membrane cholesterol plays an important role in cell membrane stiffness (98). Brain metastatic cancer cells also increase total fatty acid content to support membrane biosynthesis, though unsaturated fatty acid synthesis is decreased (96, 98). Interestingly, unsaturated fatty acids lead to a more rigid cell membrane, i.e., a decrease in unsaturated fatty acid content may correspond to a decrease in cancer cell fluidity (99). Together, cholesterol and lipid synthesis provide yet another link between the biological and mechanical characteristics of brain metastatic cells. Indeed, the brain appears to provide a metabolic microenvironment that mechanically reinforces the cellular structure of metastatic cancer cells.
Cancer mechanics and metastasis in the brain
Numerous studies have shown the interdependency of mechanics and metastatic behavior of cancer cells (65, 66, 100, 101). The mechanical properties (e.g., stiffness, viscosity) of most cancer cells, including those in the breast, are lower than their counterparts in normal cells (67, 102, 103). Most cancerous cells have altered viscoelastic properties (lower stiffness and viscosity) that allow cells to move easily through the interstitium and tumor microarchitecture on their way to metastatic sites (104). In contrast, brain cancer cells within softer tumors may be less likely to systemically metastasize and may be partially constricted by the stiffer tissue of the surrounding host brain compared to the tumor stiffness, as well as the tumor mechanical stresses housed and amplified within the skull, all of which reduce the ease of migration (105). As described earlier, at the cellular level, brain cancer cells are stiffer than normal glial cells (76).
Besides well-known mechanical abnormalities such as cell and matrix stiffness and interstitial fluid pressure, tumors also generate solid stress due to the solid elements of the tumor (30). These solid stresses promote tumor progression and hinder the delivery and efficacy of anti-cancer therapies by compressing the blood vessels and contributing to intratumoral hypoxia (30). Growth-induced stresses enhance epithelial-to-mesenchymal (EMT) transition and cancer cell migration, in part via activation of β-catenin, AKT, and Erk pathways, all of which are metabolically related (105, 106). Notably, reducing solid stress (e.g., via angiotensin receptor blockade) in breast cancer mouse models reduces lung metastatic burden (107). However, direct links between this mechanical force and cancer cell membrane stiffness and metabolic activity have yet to be determined. Nevertheless, altered mechanics at the cellular and tissue levels can drive cancer metastasis.
Discussion
The interplay of mechanics and metabolism is largely unexplored but undoubtedly plays a major role even in the early stages of the metastatic cascade from primary sites such as breast tumors. Hypoxia and acidosis in the primary tumor microenvironment emerge due to rapid cell division, a poorly functioning vasculature, and high rates of glycolysis and lactate production in tumor cells (108). These conditions promote metabolic pathway alterations, including a switch from oxidative phosphorylation to aerobic glycolysis, to aid cancer cell proliferation (109, 110). The need for higher energy levels causes these cells to invade nearby and distal organs (e.g., the brain) where glucose levels are high and can sustain cancer growth and spread. These aggressive primary breast cancer cells have low membrane stiffness and viscosity compared to normal epithelial cells, so they can easily detach from their substrate and intravasate (65, 66, 68). The reduction in cancer cell membrane stiffness is in part due to the interactions between cell metabolism and cytoskeletal structure (109). Resistance of the cytoskeleton in response to altered mechanical cues in variable microenvironments can enable the altered energy metabolism, e.g., the persistence of high glycolytic rates in cancer cells despite chronic mechanical changes in the tumor tissue (109, 110).
The invasion occurs from the primary invasive tumor site (breast), through the circulation (blood vessels), to the secondary metastatic site (brain). Cancer cells experience distinct mechanical and metabolic microenvironments at each stage, leading to biophysical adaptations. While in circulation within the blood vessel, metastatic cancer cells experience varying mechanical forces such as shear stress, which can activate genetic programs associated with cytoskeletal remodeling and altered cell–cell adhesion (36). Shear stress also activates ATOH8, a fluid mechanosensor that transcriptionally promotes glycolysis and reduces reactive oxygen species (ROS) (111). This promotes cancer cell survival in the bloodstream by enabling metabolic flexibility. Notably, most cancer cells do not survive the circulation, which may be due in part to the varying mechanical microenvironment and/or altered metabolism within the blood vessel (112, 113). Krog et al. also suggested that the mechanical fragility of circulating cancer cells is not necessarily due to the magnitude and duration of exposure to fluid shear stresses during circulation but possibly due to other secondary causes such as their exposure to immune attack in the blood vessels and lack of matrix attachment (113). They also concluded that these cancer cells may be as likely to withstand hemodynamic stresses as other blood cells during circulation (113). Cancer cells that survive the circulation, however, acquire energy via alternate carbon sources than they do in the solid tumor microenvironment (114).
Once in the brain, breast cancer cells may assume brain-like properties to survive, e.g., by using GABA to synthesize NADH for energy production (115). Exposure to new mechanical properties and forces drives further metabolic and mechanical changes during metastasis, including the stiffening of the disseminated cancer cell membrane relative to those in the primary breast tumor (116, 117). Metabolically (e.g., via production of cytoskeletal proteins), mechanical cues from the tumor microenvironment can lead to increased cell stiffness (110). Reinforcement of the cytoskeletal structures and rigidity of the cell viscoelasticity in the brain may prevent secondary brain tumors from seeding tertiary extracranial metastases (or primary brain tumors from seeding initial secondary metastases). Further exploration into the co-regulation of mechanics and metabolism in the cerebral microenvironment may provide new insights and reveal new targetable vulnerabilities for breast cancer brain metastases.
Conclusion
The interaction between mechanics and metabolism is multifaceted and plays a pivotal role in cancer progression, as seen in the case of breast cancer metastasis to the brain. The brain microenvironment provides a favorable mechanical and metabolic microenvironment for disseminated cancer cells, helping promote tumor growth and invasion. However, it is challenging to fully understand the relationship between mechanics and metabolism due to heterogeneity within a tumor, between different tumors in one patient, between different patients of the same tumor type, and between different patients with different cancers. Current methods for measuring cell and tissue stiffness, such as atomic force microscopy and magnetic resonance elastography, could be improved to provide more accurate and high-resolution information. Understanding the complete metabolic milieu of a cell requires the acquisition of a complex range of metabolomics data, which is time-sensitive and cost-intensive and may not always capture the true in vivo (particularly dynamic) state. Further advancements in metabolic analysis methods combined with mechanical probing would aid in revealing the dynamic and heterogeneous metabolic states within a tumor and how they correlate with metastasis and patient outcomes. In the future, information about the mechanics and metabolism of patient-derived cancer cells could also serve as valuable biomarkers of metastasis.
Data availability statement
The original contributions presented in the study are included in the article/supplementary material. Further inquiries can be directed to the corresponding author.
Author contributions
KO and MD conceptualized and wrote the manuscript. KO and AB revised the manuscript and created the figures. All authors listed have made a substantial, direct, and intellectual contribution to the work and approved it for publication.
Acknowledgments
The authors thank Guest Editor Dr. Gino B. Ferraro for the invitation to contribute this article to the special issue. Figures were created with BioRender.com. This work was supported by the National Cancer Institute (NIH/NCI K22 CA258410 to MD).
Conflict of interest
The authors declare that the research was conducted in the absence of any commercial or financial relationships that could pose potential conflicts of interest.
Publisher’s note
All claims expressed in this article are solely those of the authors and do not necessarily represent those of their affiliated organizations, or those of the publisher, the editors and the reviewers. Any product that may be evaluated in this article, or claim that may be made by its manufacturer, is not guaranteed or endorsed by the publisher.
References
1. Boroughs LK, Deberardinis RJ. Metabolic pathways promoting cancer cell survival and growth. Nat Cell Biol (2015) 17(4):351–9. doi: 10.1038/ncb3124
2. Iurlaro R, León-Annicchiarico CL, Muñoz-Pinedo C. Regulation of cancer metabolism by oncogenes and tumor suppressors. Methods Enzymol (2014) 542:59–80. doi: 10.1016/B978-0-12-416618-9.00003-0
3. Jones RG, Thompson CB. Tumor suppressors and cell metabolism: A recipe for cancer growth. Genes Dev (2009) 23(5):537–48. doi: 10.1101/gad.1756509
4. Schiliro C, Firestein BL. Mechanisms of metabolic reprogramming in cancer cells supporting enhanced growth and proliferation. Cells (2021) 10(5):1–41. doi: 10.3390/cells10051056
5. Gaglio D, Metallo CM, Gameiro PA, Hiller K, Danna LS, Balestrieri C, et al. Oncogenic K-ras decouples glucose and glutamine metabolism to support cancer cell growth. Mol Syst Biol (2011) 7(523):1–15. doi: 10.1038/msb.2011.56
6. Ferreira LMR, Hebrant A, Dumont JE. Metabolic reprogramming of the tumor. Oncogene (2012) 31(36):3999–4011. doi: 10.1038/onc.2011.576
7. Phan LM, Yeung SCJ, Lee MH. Cancer metabolic reprogramming: importance, main features, and potentials for precise targeted anti-cancer therapies. Cancer Biol Med (2014) 11(1):1–19. doi: 10.7497/j.issn.2095-3941.2014.01.001
8. Ciminera AK, Jandial R, John T, Termini J. Metabolic advantages and vulnerabilities in brain metastases. Physiol Behav (2017) 176(3):139–48. doi: 10.1007/s10585-017-9864-8
9. Wishart DS. Is cancer a genetic disease or a metabolic disease? EBioMedicine (2015) 2(6):478–9. doi: 10.1016/j.ebiom.2015.05.022
10. DeBerardinis RJ, Lum JJ, Hatzivassiliou G, Thompson CB. The biology of cancer: Metabolic reprogramming fuels cell growth and proliferation. Cell Metab (2008) 7(1):11–20. doi: 10.1016/j.cmet.2007.10.002
11. Bauer DE, Harris MH, Plas DR, Lum JJ, Hammerman PS, Rathmell JC, et al. Cytokine stimulation of aerobic glycolysis in hematopoietic cells exceeds proliferative demand. FASEB J (2004) 18(11):1303–5. doi: 10.1096/fj.03-1001fje
12. Faubert B, Solmonson A, DeBerardinis RJ. Metabolic reprogramming and cancer progression. Sci Am Assoc Advancement Sci (2020) 368:152. doi: 10.1126/science.aaw5473
13. Kazue S, Marie N, Mieko S, Shinjo O. Metabolism and brain canceer. Clinics (2011) 66:33–43. doi: 10.1590/S1807-59322011001300005
14. Mergenthaler P, Lindauer U, Dienel GA, Meisel A. Sugar for the brain: The role of glucose in physiological and pathological brain function. Trends Neurosci (2013) 36(10):587–97. doi: 10.1016/j.tins.2013.07.001
15. Jin J, Fang F, Gao W, Chen H, Wen J, Wen X, et al. The structure and function of the glycocalyx and its connection with blood-brain barrier. Front Cell Neurosci (2021) ;15(October):1–9. doi: 10.3389/fncel.2021.739699
16. Streitberger KJ, Lilaj L, Schrank F, Braun J, Hoffmann KT, Reiss-Zimmermann M, et al. How tissue fluidity influences brain tumor progression. Proc Natl Acad Sci U S A (2020) 117(1):128–34. doi: 10.1073/pnas.1913511116
17. Fløgstad Svensson S, Fuster-Garcia E, Latysheva A, Fraser-Green J, Nordhøy W, Isam Darwish O, et al. Decreased tissue stiffness in glioblastoma by MR elastography is associated with increased cerebral blood flow. Eur J Radiol (2022) 147:147. doi: 10.1016/j.ejrad.2021.110136
18. Barnes JM, Przybyla L, Weaver VM. Tissue mechanics regulate brain development, homeostasis and disease. J Cell Sci Company Biologists Ltd; (2017) 130:71–82. doi: 10.1242/jcs.191742
19. Ativie F, Komorowska JA, Beins E, Albayram Ö, Zimmer T, Zimmer A, et al. Cannabinoid 1 receptor signaling on hippocampal GABAergic neurons influences microglial activity. Front Mol Neurosci (2018) 11(August):1–14. doi: 10.3389/fnmol.2018.00295
20. Payne LS, Huang PH. Europe PMC funders group the pathobiology of collagens in glioma. Mol Cancer Res (2013) 11(10):1–21. doi: 10.1158/1541-7786.mcr-13-0236
21. Khoonkari M, Liang D, Kamperman M, Kruyt FAE, van Rijn P. Physics of brain cancer: Multiscale alterations of glioblastoma cells under extracellular matrix stiffening. Pharmaceutics (2022) 14(5):1031. doi: 10.3390/pharmaceutics14051031
22. Metwally MK, El-Gohary SH, Han SM, Byun KM, Kim TS. Influence of the anisotropic mechanical properties of the breast cancer on photoacoustic imaging. In: Proceedings of the 7th Cairo international biomedical engineering conference, CIBEC 2014 Egypt: CIBEC, Cairo, vol. . (2015). p. 34–8.
23. Jamin Y, Boult JKR, Li J, Popov S, Garteiser P, Ulloa JL, et al. Exploring the biomechanical properties of brain malignancies and their pathologic determinants in vivo with magnetic resonance elastography. Cancer Res (2015) 75(7):1216–24. doi: 10.1158/0008-5472.CAN-14-1997
24. Miyazawa A, Ito S, Asano S, Tanaka I, Sato M, Kondo M, et al. Regulation of PD-L1 expression by matrix stiffness in lung cancer cells. Biochem Biophys Res Commun (2018) 495(3):2344–9. doi: 10.1016/j.bbrc.2017.12.115
25. Ghasemi H, Mousavibahar SH, Hashemnia M, Karimi J, Khodadadi I, Mirzaei F, et al. Tissue stiffness contributes to YAP activation in bladder cancer patients undergoing transurethral resection. Ann N Y Acad Sci (2020) 1473(1):48–61. doi: 10.1111/nyas.14358
26. Mueller S. Liver stiffness: a novel parameter for the diagnosis of liver disease. Hepatic Medicine: Evid Res (2010) 49:49–67. doi: 10.2147/HMER.S7394
27. Itoh Y, Takehara Y, Kawase T, Terashima K, Ohkawa Y, Hirose Y, et al. Feasibility of magnetic resonance elastography for the pancreas at 3T. J Magnetic Resonance Imaging (2016) 43(2):384–90. doi: 10.1002/jmri.24995
28. Wirtz D, Konstantopoulos K, Searson PC. The physics of cancer: The role of physical interactions and mechanical forces in metastasis. Nat Rev Cancer (2011) 11(7):512–22. doi: 10.1038/nrc3080
29. Michinaga S, Koyama Y. Pathogenesis of brain edema and investigation into anti-edema drugs. Int J Mol Sci MDPI AG; (2015) 16:9949–75. doi: 10.3390/ijms16059949
30. Nia HT, Datta M, Seano G, Zhang S, Ho WW, Roberge S, et al. In vivo compression and imaging in mouse brain to measure the effects of solid stress. Nat Protoc (2020) 15(8):2321–40. doi: 10.1038/s41596-020-0328-2
31. Seano G, Nia HT, Emblem KE, Datta M, Ren J, Krishnan S, et al. Solid stress in brain tumours causes neuronal loss and neurological dysfunction and can be reversed by lithium. Nat Biomed Engineering (2019) 3(3):230–45. doi: 10.1038/s41551-018-0334-7
32. Nia HT, Liu H, Seano G, Datta M, Jones D, Rahbari N, et al. Solid stress and elastic energy as measures of tumour mechanopathology. Nat Biomed Engineering (2017) 1(1):1–11. doi: 10.1038/s41551-016-0004
33. Moeendarbary E, Harris AR. Cell mechanics: Principles, practices, and prospects. Wiley Interdiscip Reviews: Syst Biol Med (2014) 6(5):371–88. doi: 10.1002/wsbm.1275
34. Lynch ME, Neu CP, Seelbinder B, McCreery KP. “Chapter 1.4 - The Role of Mechanobiology in Cancer Metastasis” in Mechanobiology Niebur GL Ed. Elsevier (2020), 65–78. doi: 10.1016/B978-0-12-817931-4.00004-2
35. Davies PF, Tripathi SC. Mechanical stress mechanisms and the cell: An endothelial paradigm. Circ Res (1993) 72(2):239–45. doi: 10.1161/01.RES.72.2.239
36. Kumar S, Weaver VM. Mechanics, malignancy, and metastasis: The force journey of a tumor cell. Cancer Metastasis Rev (2009) 28(1–2):113–27. doi: 10.1007/s10555-008-9173-4
37. Fletcher DA, Mullins RD. Cell mechanics and the cytoskeleton. Nature (2010) 463(7280):485–92. doi: 10.1038/nature08908
38. van Zijl F, Krupitza G, Mikulits W. Initial steps of metastasis: Cell invasion and endothelial transmigration. Mutat Res - Rev Mutat Res (2011) 728(1–2):23–34. doi: 10.1016/j.mrrev.2011.05.002
39. Verry C, Sancey L, Dufort S, le Duc G, Mendoza C, Lux F, et al. Treatment of multiple brain metastases using gadolinium nanoparticles and radiotherapy: NANO-RAD, a phase I study protocol. BMJ Open (2019) 9(2):e023591. doi: 10.1136/bmjopen-2018-023591
40. Prieto E, Martí-Climent JM, Domínguez-Prado I, Garrastachu P, Díez-Valle R, Tejada S, et al. Voxel-based analysis of dual-time-point 18F-FDG PET images for brain tumor identification and delineation. J Nucl Med (2011) 52(6):865–72. doi: 10.2967/jnumed.110.085324
41. Murphy MC, Huston J 3rd, Jack CR Jr, Glaser KJ, Senjem ML, Chen J, et al. Measuring the characteristic topography of brain stiffness with magnetic resonance elastography. PLoS One (2013) 8(12):e81668. doi: 10.1371/journal.pone.0081668
42. Torrino S, Grasset EM, Audebert S, Belhadj I, Lacoux C, Haynes M, et al. Mechano-induced cell metabolism promotes microtubule glutamylation to force metastasis. Cell Metab (2021) 33(7):1342–1357.e10. doi: 10.1016/j.cmet.2021.05.009
43. Hampp E, Botah R, Odusanya OS, Anuku N, Malatesta KA, Soboyejo WO. Biosynthesis and adhesion of gold nanoparticles for breast cancer detection and treatment. J Mater Res (2012) 27(22):2891–901. doi: 10.1557/jmr.2012.317
44. Chin LK, Xia Y, Discher DE, Janmey PA. Mechanotransduction in cancer. Curr Opin Chem Engineering Elsevier Ltd; (2016) 11:77–84. doi: 10.1016/j.coche.2016.01.011
45. Zanotelli MR, Zhang J, Reinhart-King CA. Mechanoresponsive metabolism in cancer cell migration and metastasis. Cell Metab Cell Press (2021) 33:1307–21. doi: 10.1016/j.cmet.2021.04.002
46. DelNero P, Hopkins BD, Cantley LC, Fischbach C. Cancer metabolism gets physical. Sci Trans Med Am Assoc Advancement Sci (2018) 10:eaaq1011. doi: 10.1126/scitranslmed.aaq1011
47. Ge H, Tian M, Pei Q, Tan F, Pei H. Extracellular matrix stiffness: New areas affecting cell metabolism. Front Oncol Front Media S.A. (2021) 11. doi: 10.3389/fonc.2021.631991
48. Romani P, Valcarcel-Jimenez L, Frezza C, Dupont S. Crosstalk between mechanotransduction and metabolism. Nat Rev Mol Cell Biol Nat Res (2021) 22:22–38. doi: 10.1038/s41580-020-00306-w
49. Jones MG, Andriotis OG, Roberts JJW, Lunn K, Tear VJ, Cao L, et al. Nanoscale dysregulation of collagen structure-function disrupts mechano-homeostasis and mediates pulmonary fibrosis. Elife (2018) 7:e36354. doi: 10.7554/eLife.36354
50. Gkretsi V, Stylianopoulos T. Cell adhesion and matrix stiffness: Coordinating cancer cell invasion and metastasis. Front Oncol Front Media S.A. (2018) 8. doi: 10.3389/fonc.2018.00145
51. Levental KR, Yu H, Kass L, Lakins JN, Egeblad M, Erler JT, et al. Matrix crosslinking forces tumor progression by enhancing integrin signaling. Cell (2009) 139(5):891–906. doi: 10.1016/j.cell.2009.10.027
52. Acerbi I, Cassereau L, Dean I, Shi Q, Au A, Park C, et al. Human breast cancer invasion and aggression correlates with ECM stiffening and immune cell infiltration. Integr Biol (United Kingdom) (2015) 7(10):1120–34. doi: 10.1039/c5ib00040h
53. Evers TMJ, Holt LJ, Alberti S, Mashaghi A. Reciprocal regulation of cellular mechanics and metabolism. Nat Metab Nat Res (2021) 3:456–68. doi: 10.1038/s42255-021-00384-w
54. Fernie AR, Zhang Y, Sampathkumar A. Cytoskeleton architecture regulates glycolysis coupling cellular metabolism to mechanical cues. Trends Biochem Sci Elsevier Ltd; (2020) 45:637–8. doi: 10.1016/j.tibs.2020.04.003
55. Teti A. Regulation of cellular functions by extracellular matrix. J Am Soc Nephrol (1992) 2:83–7. doi: 10.1681/ASN.V210s83
56. Elgundi Z, Papanicolaou M, Major G, Cox TR, Melrose J, Whitelock JM, et al. Cancer metastasis: The role of the extracellular matrix and the heparan sulfate proteoglycan perlecan. Front Oncol (2020) ;9(January). doi: 10.3389/fonc.2019.01482
57. Hegedüs B, Marga F, Jakab K, Sharpe-Timms KL, Forgacs G. The interplay of cell-cell and cell-matrix interactions in the invasive properties of brain tumors. Biophys J (2006) 91(7):2708–16. doi: 10.1529/biophysj.105.077834
58. Han T, Kang D, Ji D, Wang X, Zhan W, Fu M, et al. How does cancer cell metabolism affect tumor migration and invasion? Cell Adhesion Migration (2013) 7(5):395–403. doi: 10.4161/cam.26345
59. Cai X, Wang KC, Meng Z. Mechanoregulation of YAP and TAZ in cellular homeostasis and disease progression. Front Cell Dev Biol (2021) 9(May):1–12. doi: 10.3389/fcell.2021.673599
60. Misra JR, Irvine KD. The hippo signaling network and its biological functions. Annu Rev Genet (2018) 52:65–87. doi: 10.1146/annurev-genet-120417-031621
61. Zhang X, Zhao H, Li Y, Xia D, Yang L, Ma Y, et al. The role of YAP/TAZ activity in cancer metabolic reprogramming. Mol Cancer BioMed Cent Ltd (2018) 17:134. doi: 10.1186/s12943-018-0882-1
62. Nokin MJ, Durieux F, Peixoto P, Chiavarina B, Peulen O, Blomme A, et al. Methylglyoxal, a glycolysis side-product, induces Hsp90 glycation and YAP-mediated tumor growth and metastasis. elife (2016) 5:e19375. doi: 10.7554/eLife.19375.034
63. Totaro A, Panciera T, Piccolo S. YAP/TAZ upstream signals and downstream responses. Nat Cell Biol Nat Publishing Group; (2018) 20:888–99. doi: 10.1038/s41556-018-0142-z
64. Lamar JM, Xiao Y, Norton E, Jiang ZG, Gerhard GM, Kooner S, et al. SRC tyrosine kinase activates the YAP/TAZ axis and thereby drives tumor growth and metastasis. J Biol Chem (2019) 294(7):2302–17. doi: 10.1074/jbc.RA118.004364
65. Onwudiwe K, Obayemi J, Hu J, Oparah J, Onyekanne C, Nwazojie C, et al. Investigation of creep properties and the cytoskeletal structures of non-tumorigenic breast cells and triple-negative breast cancer cells. J Biomed Mater Res Part A (2021) :1–17. doi: 10.1002/jbm.a.37348
66. Hu J, Zhou Y, Obayemi JD, Du J, Soboyejo WO. An investigation of the viscoelastic properties and the actin cytoskeletal structure of triple negative breast cancer cells. J Mechanical Behav Biomed Materials. (2018) ;86(May):1–13. doi: 10.1016/j.jmbbm.2018.05.038
67. Pegoraro AF, Janmey P, Weitz DA. Mechanical properties of the cytoskeleton and cells. Cold Spring Harbor Perspect Biol (2017) 9(11):a022038. doi: 10.1101/cshperspect.a022038
68. Onwudiwe K, Hu J, Obayemi J, Uzonwanne V, Ani C, Nwazojie C, et al. Actin cytoskeletal structure and the statistical variations of the mechanical properties of non-tumorigenic breast and triple-negative breast cancer cells. J Mechanical Behav Biomed Mater (2021) 119(May 2020):104505. doi: 10.1016/j.jmbbm.2021.104505
69. Taufalele PV, Reinhart-King CA. Matrix stiffness primes cells for future oxidative stress. Trends Cancer (2021) 7(10):883–5. doi: 10.1016/j.trecan.2021.08.003
70. Aziz SA, Davies M, Pick E, Zito C, Jilaveanu L, Camp RL, et al. Phosphatidylinositol-3-kinase as a therapeutic target in melanoma. Clin Cancer Res (2009) 15(9):3029–36. doi: 10.1158/1078-0432.CCR-08-2768
71. Tung JC, Barnes JM, Desai SR, Sistrunk C, Conklin MW, Schedin P, et al. Tumor mechanics and metabolic dysfunction. Free Radical Biol Med Elsevier Inc.; (2015) 79:269–80. doi: 10.1016/j.freeradbiomed.2014.11.020
72. Adamo B, Deal AM, Burrows E, Geradts J, Hamilton E, Blackwell KL, et al. Phosphatidylinositol 3-kinase pathway activation in breast cancer brain metastases. Breast Cancer Res (2011) 13(6):R125. doi: 10.1186/bcr3071
73. Blazquez R, Wlochowitz D, Wolff A, Seitz S, Wachter A, Perera-Bel J, et al. PI3K: A master regulator of brain metastasis-promoting macrophages/microglia. GLIA (2018) 66(11):2438–55. doi: 10.1002/glia.23485
74. Gozzelino L, de Santis MC, Gulluni F, Hirsch E, Martini M. PI(3,4)P2 signaling in cancer and metabolism. Front Oncol (2020) 10(March):1–12. doi: 10.3389/fonc.2020.00360
75. Deng S, Leong HC, Datta A, Gopal V, Kumar AP, Yap CT. PI3K/AKT signaling tips the balance of cytoskeletal forces for cancer progression. Cancers (Basel). (2022) 14(23):1652. doi: 10.3390/cancers14071652
76. Khan ZS, Vanapalli SA. Probing the mechanical properties of brain cancer cells using a microfluidic cell squeezer device. Biomicrofluidics (2013) 7(1):011806. doi: 10.1063/1.4774310
77. Chen J, Lee HJ, Wu X, Huo L, Kim SJ, Xu L, et al. Gain of glucose-independent growth upon metastasis of breast cancer cells to the brain. Cancer Res (2015) 75(3):554–65. doi: 10.1158/0008-5472.CAN-14-2268
78. Liberti MV, Locasale JW. The warburg effect: How does it benefit cancer cells? Trends Biochem Sci (2016) 41(3, SI):211–87. doi: 10.1016/j.tibs.2016.01.004
79. Ferraro GB, Ali A, Luengo A, Kodack DP, Deik A, Abbott KL, et al. Fatty acid synthesis is required for breast cancer brain metastasis. Nat Cancer (2021) 2(4):414–28. doi: 10.1038/s43018-021-00183-y
80. Parida PK, Marquez-Palencia M, Nair V, Kaushik AK, Kim K, Sudderth J, et al. Metabolic diversity within breast cancer brain-tropic cells determines metastatic fitness. Cell Metab (2022) 34(1):90–105.e7. doi: 10.1016/j.cmet.2021.12.001
81. Ngo B, Kim E, Osorio-Vasquez V, Doll S, Bustraan S, Liang RJ, et al. Limited environmental serine and glycine confer brain metastasis sensitivity to PHGDH inhibition. Cancer Discov (2020) 10(9):1352–73. doi: 10.1158/2159-8290.CD-19-1228
82. Mosier JA, Schwager SC, Boyajian DA, Reinhart-King CA. Cancer cell metabolic plasticity in migration and metastasis. Clin Exp Metastasis Springer Sci Business Media BV; (2021) 38:343–59. doi: 10.1007/s10585-021-10102-1
83. Tiwary S, Morales JE, Kwiatkowski SC, Lang FF, Rao G, McCarty JH. Metastatic brain tumors disrupt the blood-brain barrier and alter lipid metabolism by inhibiting expression of the endothelial cell fatty acid transporter Mfsd2a. Sci Rep (2018) 8(1):1–13. doi: 10.1038/s41598-018-26636-6
84. Bucheit AD, Chen G, Siroy A, Tetzlaff M, Broaddus R, Milton D, et al. Complete loss of PTEN protein expression correlates with shorter time to brain metastasis and survival in stage IIIB/C melanoma patients with BRAFV600 mutations. Clin Cancer Res (2014) 20(21):5527–36. doi: 10.1158/1078-0432.CCR-14-1027
85. Kang Y, Jin Y, Li Q, Yuan X. Advances in lung cancer driver genes associated with brain metastasis. Front Oncol (2021) 10. doi: 10.3389/fonc.2020.606300
86. Wikman H, Lamszus K, Detels N, Uslar L, Wrage M, Benner C, et al. Relevance of PTEN loss in brain metastasis formation in breast cancer patients. Breast Cancer Res (2012) 14(2):R49. doi: 10.1186/bcr3150
87. Gupta K. “Chapter 13 - The molecular and cellular effects of radiotherapy-induced microenvironment changes on potential chemoresistance in glioblastoma,” in Glioblastoma Resistance to Chemotherapy: Molecular Mechanisms and Innovative Reversal Strategies Paulmurugan R, Massoud TF Eds. vol. 15 Academic Press, (2021), 335–64. doi: 10.1016/B978-0-12-821567-8.00035-X
88. Berg TJ, Pietras A. Radiotherapy-induced remodeling of the tumor microenvironment by stromal cells. Semin Cancer Biol (2022). doi: 10.1016/j.semcancer.2022.02.011
89. Gupta K, Vuckovic I, Zhang S, Xiong Y, Carlson BL, Jacobs J, et al. Radiation induced metabolic alterations associate with tumor aggressiveness and poor outcome in glioblastoma. Front Oncol (2020) 10(May):1–19. doi: 10.3389/fonc.2020.00535
90. Magistretti PJ, Allaman I. A cellular perspective on brain energy metabolism and functional imaging. Neuron Cell Press (2015) 86:883–901. doi: 10.1016/j.neuron.2015.03.035
91. Kim HM, Jung WH, Koo JS. Site-specific metabolic phenotypes in metastatic breast cancer. J Trans Med (2014) 12(1):354. doi: 10.1186/s12967-014-0354-3
92. Witzel I, Oliveira-Ferrer L, Pantel K, Müller V, Wikman H. Breast cancer brain metastases: Biology and new clinical perspectives. Breast Cancer Res (2016) 18(1):1–9. doi: 10.1186/s13058-015-0665-1
93. Pifferi F, Laurent B, Plourde M. Lipid transport and metabolism at the blood-brain interface: Implications in health and disease. Front Physiol (2021) 12. doi: 10.3389/fphys.2021.645646
94. Natarajan SK, Venneti S. Glutamine metabolism in brain tumors. Cancers (2019) 11:1628. doi: 10.3390/cancers11111628
95. Tanner K. Perspective: The role of mechanobiology in the etiology of brain metastasis. APL Bioeng (2018) 2:031801. doi: 10.1063/1.5024394
96. Santana-Codina N, Marcé-Grau A, Muixí L, Nieva C, Marro M, Sebastián D, et al. Grp94 is involved in the lipid phenotype of brain metastatic cells. Int J Mol Sci (2019) 20(16):3383. doi: 10.3390/ijms20163883
97. Ehmsen S, Pedersen MH, Wang G, Terp MG, Arslanagic A, Hood BL, et al. Increased cholesterol biosynthesis is a key characteristic of breast cancer stem cells influencing patient outcome. Cell Rep (2019) 27(13):3927–38. doi: 10.1016/j.celrep.2019.05.104
98. Lei K, Kurum A, Kaynak M, Bonati L, Han Y, Cencen V, et al. Cancer-cell stiffening via cholesterol depletion enhances adoptive T-cell immunotherapy. Nat Biomed Engineering (2021) 5(12):1411–25. doi: 10.1038/s41551-021-00826-6
99. Chen M, Huang J. The expanded role of fatty acid metabolism in cancer: new aspects and targets. Precis Clin Med (2019) 2(3):183–91. doi: 10.1093/pcmedi/pbz017
100. Ragazzon MRP, Gravdahl JT, Vagia M. Viscoelastic properties of cells: Modeling and identification by atomic force microscopy. Mechatronics (2018) ;50(September):271–81. doi: 10.1016/j.mechatronics.2017.09.011
101. Runel G, Lopez-Ramirez N, Chlasta J, Masse I. Biomechanical properties of cancer cells. Cells (2021) 10(4):1–14. doi: 10.3390/cells10040887
102. Cao Y, Bly R, Moore W, Gao Z, Cuitino AM, Soboyejo W. Investigation of the viscoelasticity of human osteosarcoma cells using a shear assay method. J Mater Res (2006) 21(8):1922–30. doi: 10.1557/jmr.2006.0235
103. Ravetto A, Anderson PDA, Bouten CVC, den Toonder JMJ. Microfluidics for single cell mechanical Characterization: A review. SM J Biomed Engineering (2017) 3(2):1016.
104. Barriga EH, Mayor R. Adjustable viscoelasticity allows for efficient collective cell migration. Semin Cell Dev Biol (2019) 93:55–68. doi: 10.1016/j.semcdb.2018.05.027
105. Kalli M, Voutouri C, Minia A, Pliaka V, Fotis C, Alexopoulos LG, et al. Mechanical compression regulates brain cancer cell migration through MEK1/Erk1 pathway activation and GDF15 expression. Front Oncol (2019) 9(SEP). doi: 10.3389/fonc.2019.00992
106. Fernández-Sánchez ME, Barbier S, Whitehead J, Béalle G, Michel A, Latorre-Ossa H, et al. Mechanical induction of the tumorigenic β-catenin pathway by tumour growth pressure. Nature (2015) 523(7558):92–5. doi: 10.1038/nature14329
107. Chauhan VP, Chen IX, Tong R, Ng MR, Martin JD, Naxerova K, et al. Reprogramming the microenvironment with tumorselective angiotensin blockers enhances cancer immunotherapy. Proc Natl Acad Sci U S A (2019) 166(22):10674–80. doi: 10.1073/pnas.1819889116
108. Sørensen BS, Busk M, Overgaard J, Horsman MR, Alsner J. Simultaneous hypoxia and low extracellular pH suppress overall metabolic rate and protein synthesis in vitro. PloS One (2015) 10(8):e0134955. doi: 10.1371/journal.pone.0134955
109. Park HA, Brown SR, Kim Y. Cellular mechanisms of circulating tumor cells during breast cancer metastasis. Int J Mol Sci (2020) 21(14):1–19. doi: 10.3390/ijms21145040
110. Park JS, Burckhardt CJ, Lazcano R, Solis LM, Isogai T, Li L, et al. Mechanical regulation of glycolysis via cytoskeleton architecture. Nature (2020) 578(7796):621–6. doi: 10.1038/s41586-020-1998-1
111. Huang Q, Li S, Hu X, Sun M, Wu Q, Dai H, et al. Shear stress activates ATOH8 via autocrine VEGF promoting glycolysis dependent-survival of colorectal cancer cells in the circulation. J Exp Clin Cancer Res (2020) 39(1):25. doi: 10.1186/s13046-020-1533-0
112. Perea Paizal J, Au SH, Bakal C. Squeezing through the microcirculation: survival adaptations of circulating tumour cells to seed metastasis. Br J Cancer (2021) 124:58–65. doi: 10.1038/s41416-020-01176-x
113. Krog BL, Henry MD. “Biomechanics of the circulating tumor cell microenvironment”. In: Adv Exp Med Biol (2018) 1092:209–33. doi: 10.1007/978-3-319-95294-9_11
114. Bergers G, Fendt SM. The metabolism of cancer cells during metastasis. Nat Rev Cancer (2021) 21(3):162–80. doi: 10.1038/s41568-020-00320-2
115. Neman J, Termini J, Wilczynski S, Vaidehi N, Choy C, Kowolik CM, et al. Human breast cancer metastases to the brain display GABAergic properties in the neural niche. Proc Natl Acad Sci U S A (2014) 111(3):984–9. doi: 10.1073/pnas.1322098111
116. Watson AW, Grant AD, Parker SS, Hill S, Whalen MB, Chakrabarti J, et al. Breast tumor stiffness instructs bone metastasis via maintenance of mechanical conditioning. Cell Rep (2021) 35(13):109293. doi: 10.1016/j.celrep.2021.109293
Keywords: tumor metabolism, glycolysis, fatty acid synthesis, tumor mechanics, extracellular matrix, mechanotransduction, cell stiffness, brain metastatic microenvironment
Citation: Onwudiwe K, Burchett AA and Datta M (2022) Mechanical and metabolic interplay in the brain metastatic microenvironment. Front. Oncol. 12:932285. doi: 10.3389/fonc.2022.932285
Received: 29 April 2022; Accepted: 26 July 2022;
Published: 18 August 2022.
Edited by:
Gino B Ferraro, Harvard Medical School, United StatesReviewed by:
Kshama Gupta, Mayo Clinic, United StatesCopyright © 2022 Onwudiwe, Burchett and Datta. This is an open-access article distributed under the terms of the Creative Commons Attribution License (CC BY). The use, distribution or reproduction in other forums is permitted, provided the original author(s) and the copyright owner(s) are credited and that the original publication in this journal is cited, in accordance with accepted academic practice. No use, distribution or reproduction is permitted which does not comply with these terms.
*Correspondence: Meenal Datta, bWRhdHRhQG5kLmVkdQ==