- 1Edmond H. Fischer Translational Medical Research Laboratory, Scientific Research Center, The Seventh Affiliated Hospital, Sun Yat-Sen University, Shenzhen, China
- 2Department of Pediatrics, The Seventh Affiliated Hospital of Sun Yat-Sen University, Shenzhen, China
Acute myeloid leukemia (AML) is a hematologic malignancy. The overall prognosis is poor and therapeutic strategies still need to be improved. Studies have found that abnormalities in metabolisms promote the survival of AML cells. In recent years, an increasing number of studies have reported the effectiveness of a protein synthesis inhibitor, homoharringtonine (HHT), for the treatment of AML. In this study, we demonstrated that HHT effectively inhibited AML cells, especially MV4-11, a cell line representing human AML carrying the poor prognostic marker FLT3-ITD. We analyzed the transcriptome of MV4-11 cells treated with HHT, and identified the affected metabolic pathways including the choline metabolism process. In addition, we generated a line of MV4-11 cells that were resistant to HHT. The transcriptome analysis showed that the resistant mechanism was closely related to the ether lipid metabolism pathway. The key genes involved in these processes were AL162417.1, PLA2G2D, and LPCAT2 by multiple intergroup comparison and Venn analysis. In conclusion, we found that the treatment of HHT significantly changed metabolic signatures of AML cells, which may contribute to the precise clinical use of HHT and the development of novel strategies to treat HHT-resistant AML.
Introduction
Acute myeloid leukemia (AML) is a heterogeneous hematologic malignancy featured by a proliferation of myeloblasts that are unable to undergo normal differentiation (1). It is the most prevalent form of leukemia in adults and the second major type of acute leukemia in children (2, 3). The disease progresses rapidly, with a survival of only a few weeks to a few months if untreated. In the United States, the 5-year overall survival rate for adults with relapsed/refractory AML (excluding APL) is approximately 10% (4).
Homoharringtonine (HHT) is a plant alkaloid that inhibits protein synthesis and has anti-tumor properties. In October 2012, HHT was approval by the U.S. Food and Drug Administration (FDA) for the treatment of chronic or accelerated CML after failure treatment of 2 or more tyrosine kinase inhibitors (5, 6). HHT has been used in China for more than 40 years to treat AML (7). A multicenter phase 3 trial found that the HAA (homoharringtonine-cytarabine-aclarubicin) regimen could be used as an alternative induction therapy for untreated AML, particularly in those patients with favorable and intermediate cytogenetics (8). Previous studies have found multiple mechanisms of HHT in treating AML, including targeting FLT3 pathway, antioxidant defense, SP1/TET1/5hmC pathway, TRAIL and p53 pathway, NF-κB pathway and the expression of myosin-9 and eIF4E (9–15). The mechanisms of resistance to HHT in AML include the reactivation of PI3K/AKT signaling pathway and the overexpression of MDR1, MRP1, P170-glycoprotein, MCL-1, and MAP4K1 (16–20). In addition, an increasing number of studies have reported synergistic effects of HHT combined with FLT3 inhibitors for the treatment of AML with FLT3-ITD (9, 21, 22). Recent research has shown that the treatment of FLT3 inhibitor gilteritinib leads to reprogramming of glycolipid metabolism in the tumor microenvironment, resulting in early and late drug resistance (23). Also, HHT in combination with glutaminase inhibitor CB-839 exacerbates AML mitochondrial reactive oxygen species and apoptosis (24). Venetoclax is a potent and selective oral inhibitor of BCL-2, a key regulator of mitochondrial energy metabolism that plays an important role in the survival of AML stem cells (25, 26). A study has shown a strong synergistic effect of venetoclax and HHT in diffuse large B-cell lymphoma in vitro and in vivo (27). Although a major progress has been made, the detailed mechanisms of effectiveness and resistance in HHT-based therapy in AML still need to be explored. And, the mechanisms of HHT metabolism-related studies in AML have not been reported.
Deregulating cellular metabolism is a hallmark of cancer (28). In AML, knockdown of ANRIL leads to decreased glucose uptake and inhibits survival of leukemia cells (29). Inhibition of glycolysis attenuates the proliferation of AML cells and enhances the cytotoxicity of Ara-C (30). Also, acetyl-CoA carboxylase 1(ACC1) was found to be the rate-limiting enzyme for fatty acid synthesis, and upregulation of ACC1 protein expression has the potential to be an effective strategy for the treatment of AML (31). Therefore, metabolic abnormalities in AML are highly involved in disease progression and resistance to the treatment, which need to be further investigated. In this study, we explored the change in transcriptome of HHT-treated AML cells and identified key metabolism pathways that may affect sensitivity and resistance of AML cells to HHT.
Materials and Methods
Cell Culture
Human AML cell lines MOLM-13 and MV4-11 were obtained from DSMZ (Braunschweig, Germany) and ATCC (VA, USA). The cells were maintained in RPMI 1640 (Hyclone, Thermo Fisher Scientific, USA) supplemented with 10% fetal bovine serum (FBS) (Gemini, USA), 100 U/ml penicillin, and 100µg/ml streptomycin (Hyclone, Thermo Fisher Scientific, USA) at 37°C in a humidified atmosphere containing 5% CO2.
Cell Viability Assay and Flow Cytometry
For the cell viability assay, 6 × 104 cells were plated in each well in a 96-well plate with complete RPMI 1640 supplemented with 10% FBS. The plate was incubated for 24 hours before detection. Cell viability was measured by the cell counting kit-8 (CCK-8, Solaribio, Beijing, China). For the apoptotic assay, cells were seeded in 24-well plates at 0.75× 106 cells/well and incubated for 18 hours and subjected to flow cytometry. The Annexin V-FITC Apoptosis Detection Kit was obtained from DOJINDO (Japan) (AD10-50). Flow cytometric analysis was performed by a Cytoflex flow cytometer (Beckman, USA).
Transcriptome Analysis
We obtained the resistant MV4-11 strain (R) by gradually increasing the concentration of HHT and kept the cells in culture with 10 nM for about 10 months. After obtaining the resistant strain, we cultured the resistant strain off-drug for about 3 months to obtain the detoxified strain (O). Together with the parental MV4-11 cells (N), R and O cells were cultured with or without 10 nM HHT for 6 hours. The samples were designated N0 and N6 for parent cells without or with HHT, O0 and O6 for off-drug cells without or with HHT, and R0 and R6 for resistant R cells with or without HHT, respectively. Cells were collected in lyophilization tubes and frozen in liquid nitrogen for 10 minutes. The isolation of RNA and next-generation of sequencing were performed by Genedenovo Biotechnology Co., Ltd (Guangzhou, China). Raw data and normalized gene expression data are deposited in the sequence read archive database under accession numbers PRJNA832421. Gene Ontology (GO) and Kyoto Encyclopedia of Genes and Genomes (KEGG) pathways were performed by Omicshare tools (https://www.omicshare.com/tools/). Gene Set Enrichment Analysis (GESA) was performed by using software GSEA and MSigDB (32).
Statistics
Data visualizing and statistical analysis were performed using GraphPad Prism 8.0 (GraphPad Software Inc., CA, USA). Differences between experimental groups were analyzed using unpaired Student t test. p value < 0.05 was considered significant.
Results
HHT Significantly Induced Apoptosis of MV4-11 and MOLM13
In the cell viability assay, we found that MV4-11 and MOLM13 were sensitive to the treatment of HHT, with IC50 of 7.92 nM and 12.98 nM, respectively (Figure 1A). Flow cytometry showed that HHT induced significant apoptosis in MV4-11 and MOLM13 (Figure 1B). To explore the resistant mechanism in HHT-treated AML cells, we established MV4-11 that resisted to HHT by gradually increasing the concentration of HHT in the medium. The cell viability assay showed that MV4-11 resistant strain and off-drug strain were less sensitive to the treatment of HHT, compared with the original MV4-11 (Figure 1C).
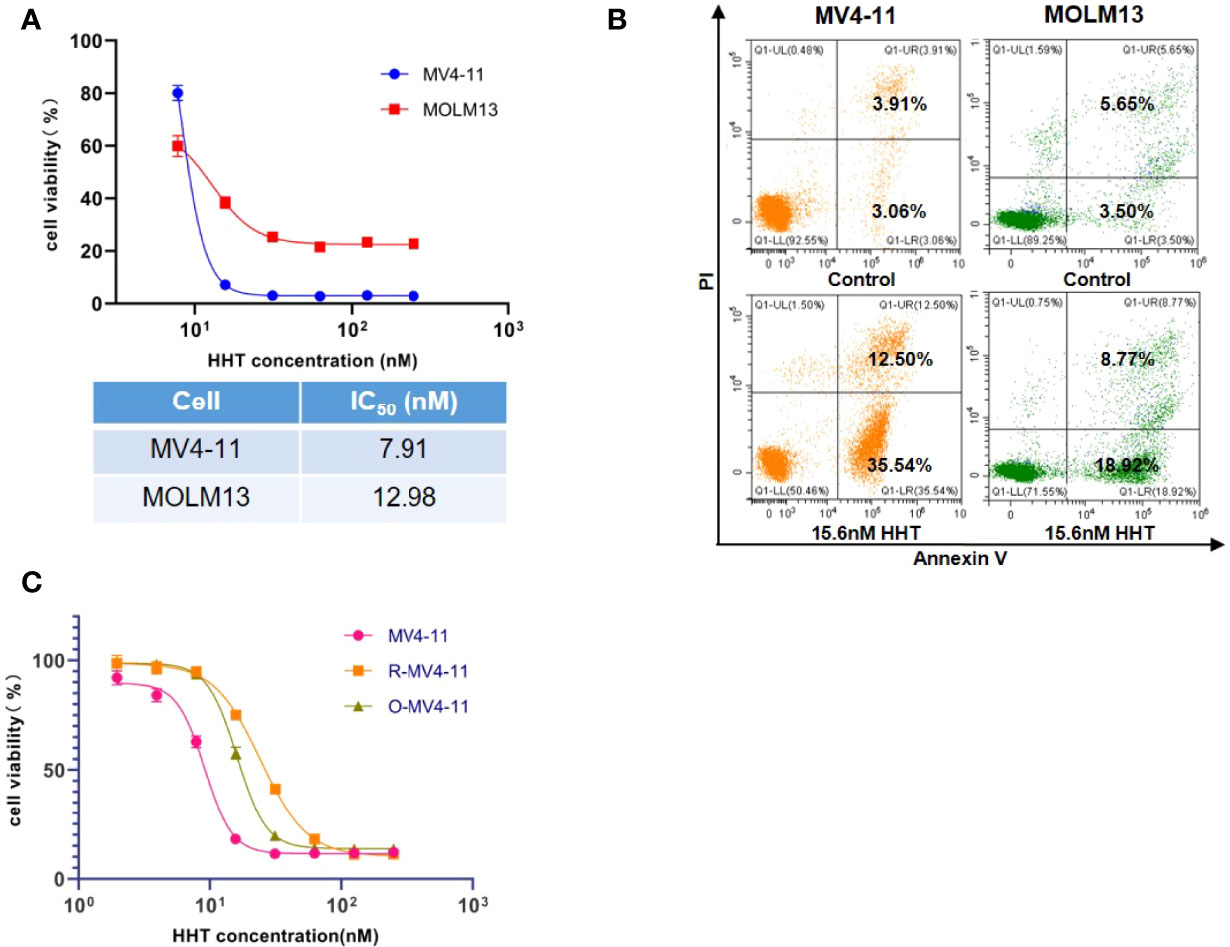
Figure 1 AML cell lines were sensitive to HHT and the establishment of HHT-resistant cells. MV4-11, MOLM13 cells were treated with different concentrations of HHT for 24 hours, and cell viability was detected by CCK-8 (A). AML cells were treated without or with 15.6 nM HHT for 18 hours, and analyzed by flow cytometry (B). Cells were treated with different concentrations of HHT for 24 hours. The cell viability of MV4-11, MV4-11 resistant strain (R-MV4-11), and off-drug strain (O-MV4-11) was detected by CCK-8 (C).
Choline Metabolism Was Associated With HHT Treatment in AML
We performed transcriptome analysis in 3 strains of MV4-11 cells treated by HHT or out of HHT, including the original strain (N0 and N6), the HHT-resistant strain (R0 and R6), and the off-drug strain (O0 and O6). The genetic heat map analysis and principal component analysis revealed a large inter-group variability and a small intra-group variability (Figures 2A, B), which was in accordance with the number of up- and down-regulated genes in each group (Figure 2C). These data indicate an ideal cell line modeling in our transcriptome analysis.
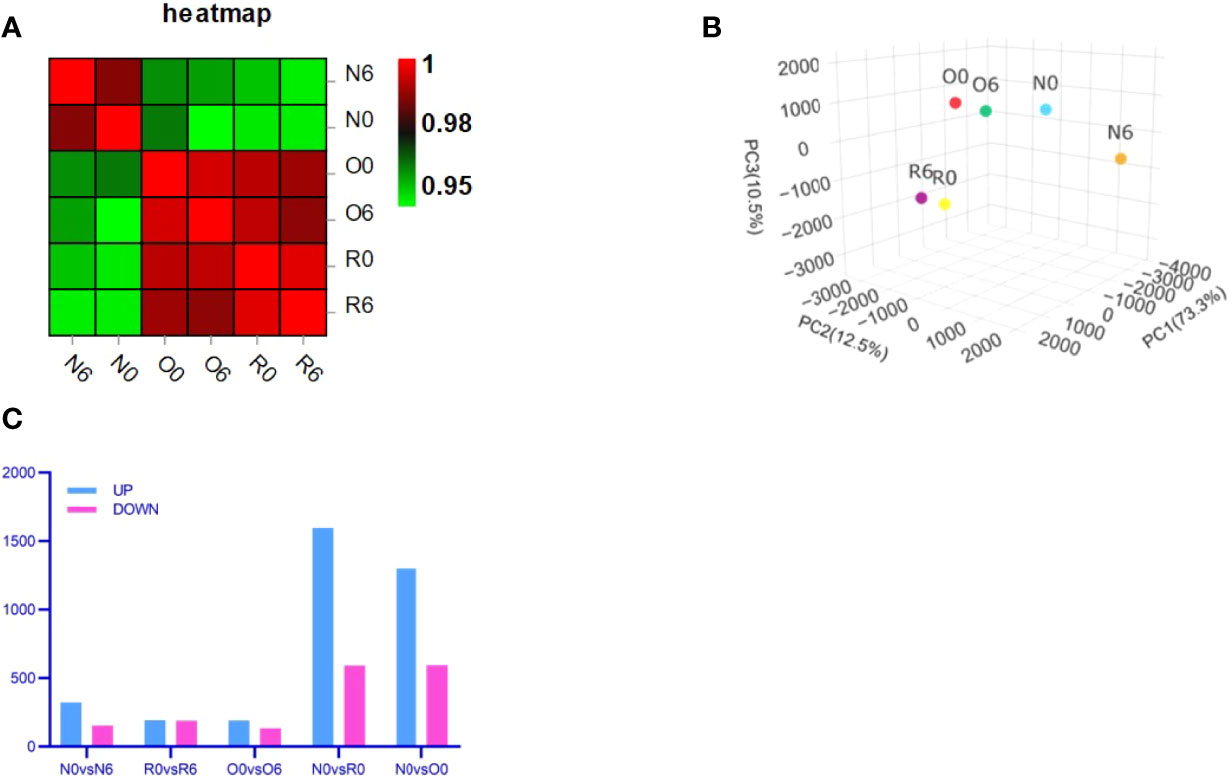
Figure 2 Transcriptome analysis showed an ideal cell line modeling. Correlation heat map (A) and Principal Component Analysis (PCA) (B) were used to assess intra- and inter-group differences in the samples. Bar charts showed the number of significantly different genes between groups (FDR < 0.05, multiple of difference greater than or equal to 2) (C).
In groups of N0 vs N6, O0 vs O6, and R0 vs R6, genes in metabolism processes were found to be significantly enriched by GO enrichment analysis (Figures 3A–C). To clarify the specific metabolic pathways, we performed KEGG enrichment analysis and found that genes in the choline metabolism pathway were significantly enriched after short-term intervention with HHT or out of HHT (N0 vs N6, p = 0.01; O0 vs O6, p = 0.02; R0 vs R6, p = 0.001) (Figures 3D–F). In contrast, this metabolic pathway was not significantly enriched in groups of long-term intervention of HHT (Figures 4C, D). Therefore, we speculated that the choline metabolism pathway is involved in the anti-leukemia effect of HHT in AML.
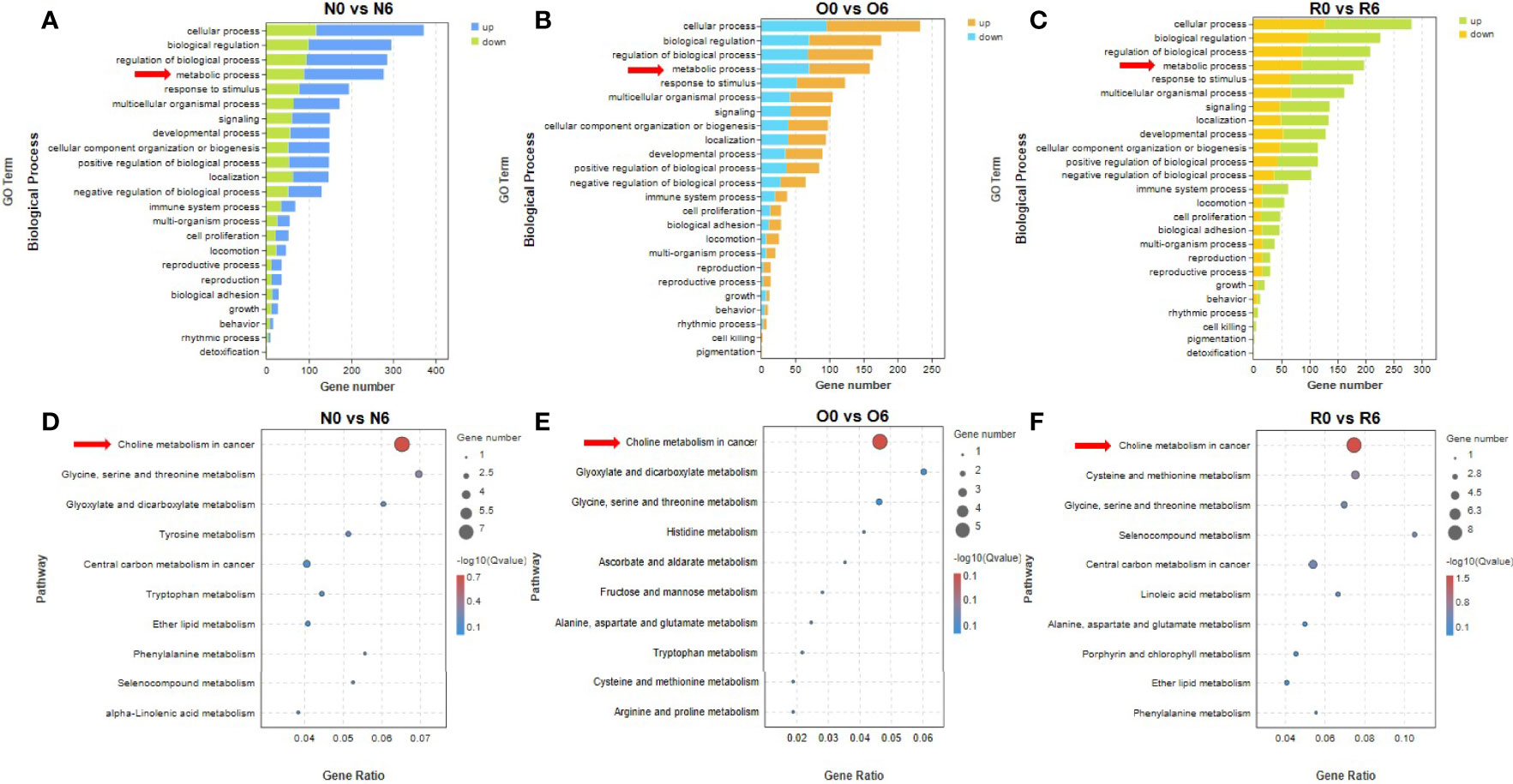
Figure 3 The drug efficacy mechanism of HHT was related to choline metabolism. Biological processes significantly affected in different cell lines with 10 nM HHT treatment for 6 hours (A, B) or HHT withdraw for 6 hours in the resistant line were found by GO enrichment analysis (C). HHT was found to significantly affect metabolism-related signaling pathways by KEGG enrichment analysis (D–F).
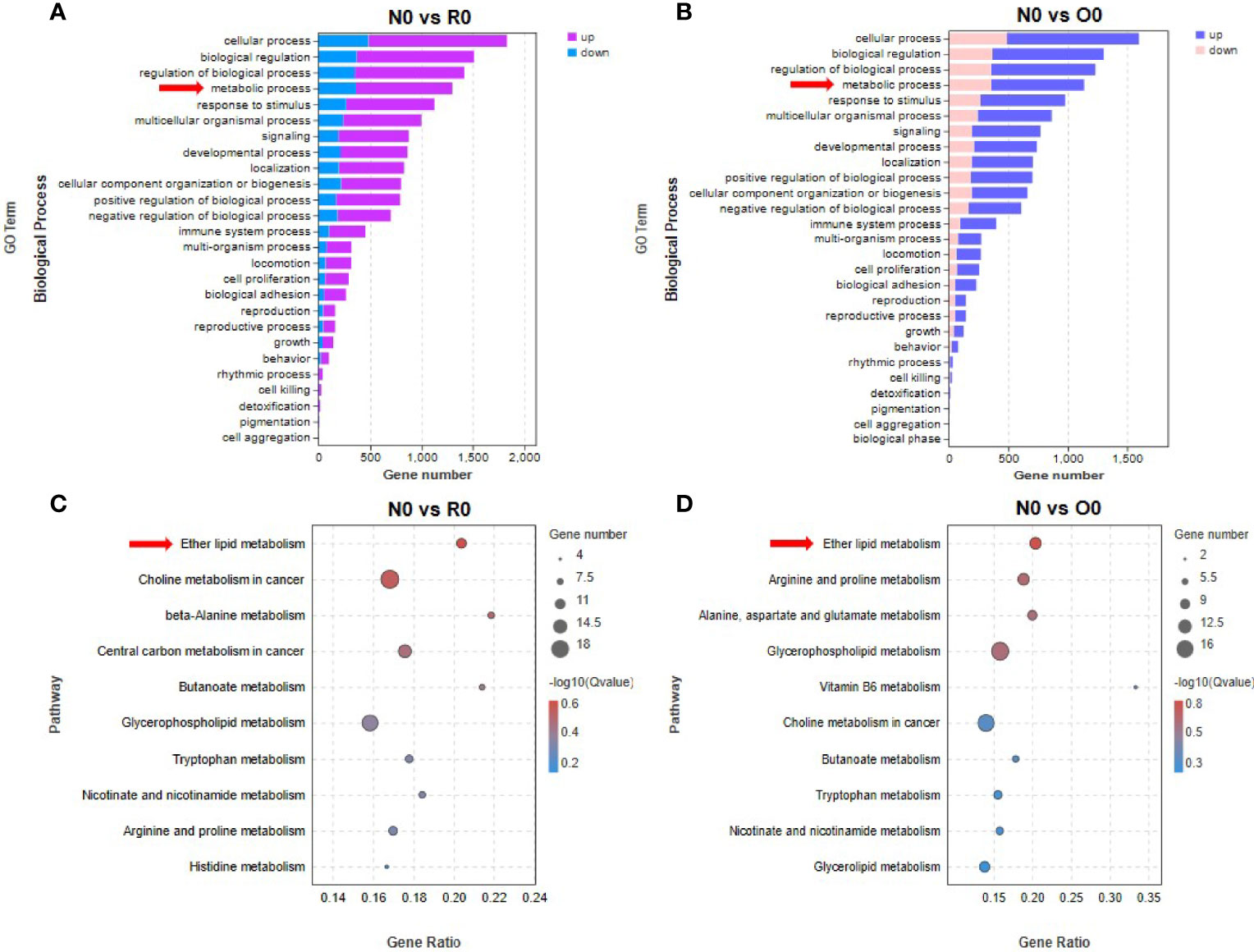
Figure 4 Ether lipid metabolism was associated with the mechanism of HHT resistance. By GO enrichment analysis, several biological processes were found to be significantly affected in long-term HHT-treated cell lines (A, B). Significant effects of HHT on metabolism-related signaling pathways were found by KEGG enrichment analysis (C, D).
Ether Lipid Metabolism Was Associated With the Resistance to HHT in AML
In groups of N0 vs R0 and N0 vs O0, GO enrichment analysis revealed that genes in metabolism-related biological processes were significantly enriched (Figures 4A, B). To clarify specific metabolic pathways, we performed KEGG enrichment analysis and found that the genes in ether lipid metabolism pathway were significantly enriched after long-term HHT intervention (N0 vs R0, p= 0.048) (Figure 4C). In the off-drug group, genes in this pathway were also significantly enriched (N0 vs O0, p = 0.02) (Figure 4D). In contrast, this metabolic pathway was not significantly enriched in HHT short-term intervention groups (N0 vs N6, p = 0.30; O0 vs O6, p = 0.51; R0 vs R6, p = 0.25). Therefore, we conclude that the ether lipid metabolism pathway is involved in promoting the development of resistance to HHT in AML cells. Interestingly, in the N0 vs O0 group, “arginine and proline metabolism” (p = 0.037), “alanine, aspartate and glutamate metabolism” (p = 0.044), and “glycerophospholipid metabolism” (p = 0.045) were also significantly enriched, and these pathways may contribute to the re-sensitization of O0 strain to HHT.
AL162417.1, PLA2G2D, and LPCAT2 Were Key Genes in the Metabolic Signature of HHT-Treated AML Cells
By using KEGG enrichment analysis, we identified the core set of genes associated with the treatment of HHT in AML cells. Drug efficacy gene of HHT was AL162417.1 (Figures 5A, C). The key genes of resistance to HHT were PLA2G2D, LPCAT2, UGT8, CHPT1, and GDPD1 (Figure 5B).
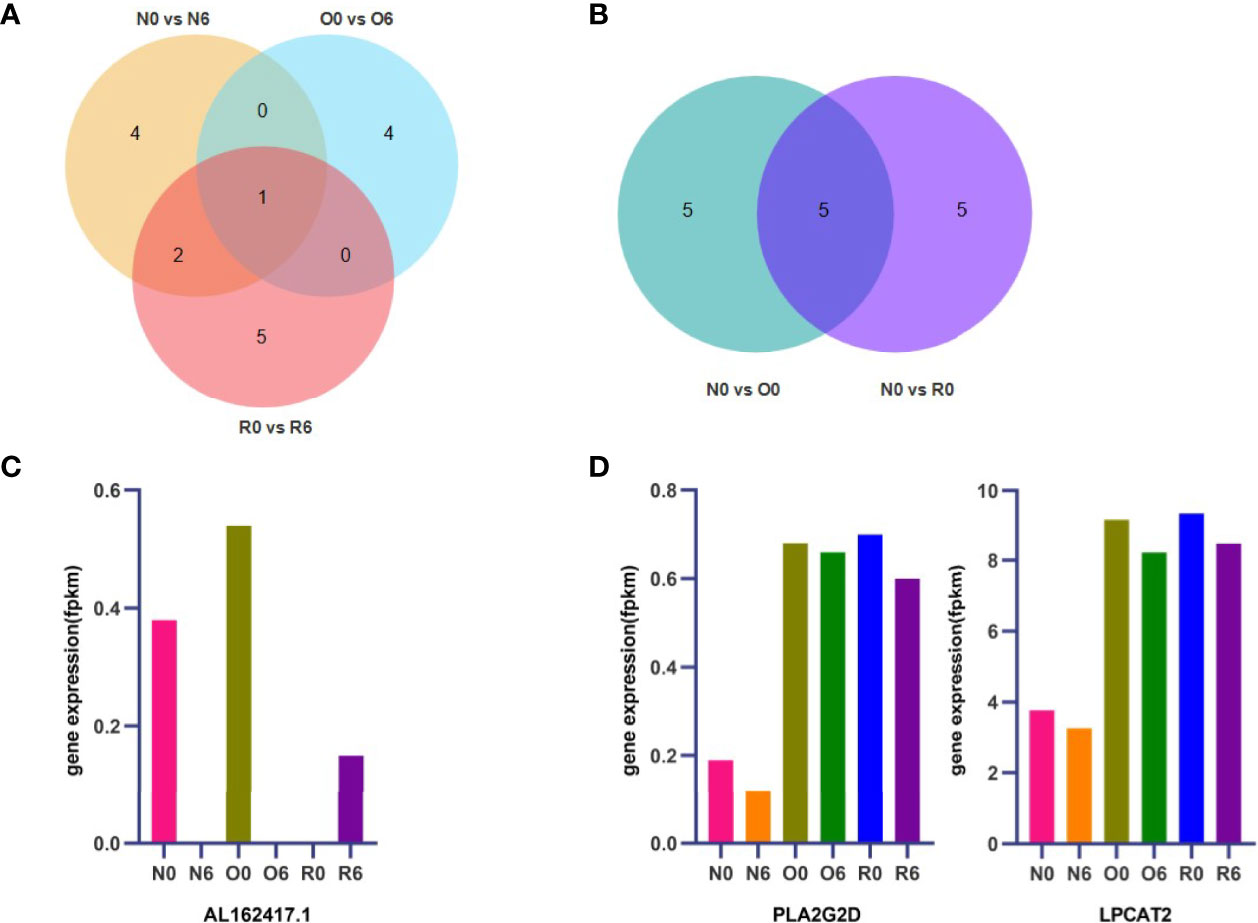
Figure 5 AL162417.1, PLA2G2D, and LPCAT2 are candidate genes for drug efficacy and resistance. The core genes of drug efficacy and resistance related pathways identified by KEGG enrichment analysis and the shared key genes were found by Venn diagram (A, B). Expression of key genes for drug efficacy and resistance were detected by RNA-seq (C, D).
Since the fluctuation of drug resistance genes should not be significant changed after short-term administration or withdrawal of HHT, CHPT1, UGT8, and GDPD1 were excluded (Figure S1A). The key drug resistance-related genes were identified as PLA2G2D and LPCAT2 (Figure 5D). In addition, we found that PLA2G2D and LPCAT2 were significantly enriched in the group of N0 vs O0 by GSEA (Figure S1B), which further validated PLA2G2D and LPCAT2 as key HHT-resistance genes in AML cells.
Discussion
Tumorigenesis and progression require metabolic reprogramming of cancer cells (33, 34), and understanding this process is important for tumor diagnosis, treatment, and prognosis. Abnormalities in choline metabolism have been reported in a variety of tumors. Several studies have reported that C-choline PET/CT can detect biochemical recurrent prostate cancer recurrence and survival (35, 36). In Glioblastomas cell lines U87MG and GBM5, choline increases tumor cell proliferation and anti-apoptosis by activating α7- and α9-containing nicotinic receptors and promoting AKT and ERK phosphorylation (37). The increased choline uptake was reported in endometrial cancer cell lines compared to normal endometrial stromal cells (38). CHPT1 drives cytidine diphosphate-choline (CDP-Cho) to generate phosphatidylcholine (PtdCho). Knockdown of CHPT1 inhibits the growth and proliferation of breast cancer cells, and in vivo experiments have shown that knockdown of CHPT1 inhibits the early metastasis of breast cancer cells (39). Genomic pooling analysis of colorectal cancer gut microbes shows that the choline trimethylamine-lyase gene is overexpressed in colorectal cancer (40). It is reported that in some types of tumors, the upregulation of choline metabolic pathway may lead to proliferation and anti-apoptosis of tumor cells. Wang, Musharraf et al. found significant alterations in choline metabolism in AML patients (41, 42). Besides, increased phosphorylcholine (PCho) was found to be a common feature of all observed AML cell lines (43). However, the specific pathological significance of abnormal choline metabolism in AML has not been revealed. In this study, we found that the drug efficacy mechanism of HHT is closely related to the choline metabolism, and further identified key genes in the pathway. Note that AL162417.1 is a novel protein-coding gene that is a homolog of RALGDS, which activates CHK (44), and promotes the production of PCho. Therefore, AL162417.1 could serve as a potential target of HHT in treating AML cells. The current findings on this gene are limited, which deserves further in-depth studies.
Ether lipids play a role in membrane fusion, participate in cellular differentiation and signaling, and act as endogenous antioxidants (45). Ether lipid levels have been shown to elevate in tumors. The enzyme alkylglycer-onephosphate synthase (AGPS) is upregulated in several types of aggressive human cancer cells and primary tumors, and knockdown of AGPS in cancer cells decreases survival of tumor cells, cancer invasiveness, and tumor growth [24]. In this study, we found that HHT resistance mechanism is closely related to the ether lipid metabolism pathway, and further identified key genes as PLA2G2D and LPCAT2. PLA2G2D encodes a secreted member of the phospholipase A2 family, which produces lysophospholipids and free fatty acid and involves in ether lipid metabolism. Studies show that oxidative stress may induce the expression of PLA2G2D in mouse and human monocyte-derived macrophages through lipid peroxidation. The high expression of PLA2G2D increases viral infection, and mice lacking PLAG2D are protected from COVID-19 invasion (46, 47). Besides, it has been reported that upregulation of PLA2G2D could be a potential biomarker for cancer immunotherapy (48). LPCAT2 is involved in ether lipid metabolism and is a member of the family of enzymes encoding lysophospholipid acyltransferases. The expression level of LPCAT2 is positively correlated with aggressive prostate cancer (49). Importantly, LPCAT2 mediated lipid droplet production has been shown to promote resistance to chemotherapy in colorectal cancer (50). Therefore, LPCAT2 is more promising candidate genes for HHT resistance. Furthermore, by ether lipids metabolism KEGG signaling pathway diagram (Figure S2), we found that PLA2G2D and LPCAT2 are involved in the conversion between lysoPAF and plasmanylcholine, but overall promote lysoPAF to plasmanylcholine production.
In summary, our study found that the mechanism of HHT efficacy in AML is related to choline metabolism, and the key candidate gene was AL162417.1. The resistance mechanism is related to ether lipid metabolism, and the core resistance candidate genes are PLA2G2D and LPCAT2. This study provides a new perspective for the rational clinical use of HHT and the development of new HHT-resistant treatment strategies.
Data Availability Statement
The datasets presented in this study can be found in online repositories. The names of the repository/repositories and accession number(s) can be found in the article/Supplementary Material.
Author Contributions
YP, CC, and YC conceived the project. YLZ, NL, ZC and HW performed the experiments. QZ, DZ, JH, YG and YMZ analyzed data. YLZ, ZC, and HW wrote the manuscript. All authors contributed to the article and approved the submitted version.
Funding
The work was supported by Guangdong Provincial Key Laboratory of Digestive Cancer Research (No. 2021B1212040006). We also thank Guangdong Basic and Applied Basic Research Foundation (2019A1515110121) to support the design of the study, National Natural Science Foundation of China (NSFC, Grant No. 82000150), Shenzhen Healthcare Research Project (Grant No. SZLY2018001), and Shenzhen Science and Technology Innovation Commission (JCYJ20190814164601648 and JCYJ20210324123003009) to support sample collection and analysis, and Sanming Project of Medicine in Shenzhen (No. SZSM202011004) to support the manuscript preparation and publication.
Conflict of Interest
The authors declare that the research was conducted in the absence of any commercial or financial relationships that could be construed as a potential conflict of interest.
Publisher’s Note
All claims expressed in this article are solely those of the authors and do not necessarily represent those of their affiliated organizations, or those of the publisher, the editors and the reviewers. Any product that may be evaluated in this article, or claim that may be made by its manufacturer, is not guaranteed or endorsed by the publisher.
Supplementary Material
The Supplementary Material for this article can be found online at: https://www.frontiersin.org/articles/10.3389/fonc.2022.931527/full#supplementary-material
References
1. Newell LF, Cook RJ. Advances in Acute Myeloid Leukemia. BMJ (2021) 375:n2026. doi: 10.1136/bmj.n2026
2. Burd A, Levine RL, Ruppert AS, Mims AS, Borate U, Stein EM, et al. Precision Medicine Treatment in Acute Myeloid Leukemia Using Prospective Genomic Profiling: Feasibility and Preliminary Efficacy of the Beat Aml Master Trial. Nat Med (2020) 26(12):1852–8. doi: 10.1038/s41591-020-1089-8
3. Carter JL, Hege K, Yang J, Kalpage HA, Su Y, Edwards H, et al. Targeting Multiple Signaling Pathways: The New Approach to Acute Myeloid Leukemia Therapy. Signal Transduct Target Ther (2020) 5(1):288. doi: 10.1038/s41392-020-00361-x
4. DeWolf S, Tallman MS. How I Treat Relapsed or Refractory Aml. Blood (2020) 136(9):1023–32. doi: 10.1182/blood.2019001982
5. Cortes J, Lipton JH, Rea D, Digumarti R, Chuah C, Nanda N, et al. Phase 2 Study of Subcutaneous Omacetaxine Mepesuccinate After Tki Failure in Patients With Chronic-Phase Cml With T315i Mutation. Blood (2012) 120(13):2573–80. doi: 10.1182/blood-2012-03-415307
6. Lu S, Wang J. Homoharringtonine and Omacetaxine for Myeloid Hematological Malignancies. J Hematol Oncol (2014) 7:2. doi: 10.1186/1756-8722-7-2
7. Harringtonine Research Collaborative Group. Preliminary Clinical Evaluation of Harringtonine Alkaloids in the Treatment of Acute Leukemia. Zhejiang Tumor Newslett (1976) (01):56–62.
8. Jin J, Wang JX, Chen FF, Wu DP, Hu J, Zhou JF, et al. Homoharringtonine-Based Induction Regimens for Patients With De-Novo Acute Myeloid Leukaemia: A Multicentre, Open-Label, Randomised, Controlled Phase 3 Trial. Lancet Oncol (2013) 14(7):599–608. doi: 10.1016/S1470-2045(13)70152-9
9. Lam SS, Ho ES, He BL, Wong WW, Cher CY, Ng NK, et al. Homoharringtonine (Omacetaxine Mepesuccinate) as an Adjunct for Flt3-Itd Acute Myeloid Leukemia. Sci Transl Med (2016) 8(359):359ra129. doi: 10.1126/scitranslmed.aaf3735
10. Zhang JJ, Geng HY, Liu L, Zhang H. Synergistic Cytotoxicity of Homoharringtonine and Etoposide in Acute Myeloid Leukemia Cells Involves Disrupted Antioxidant Defense. Cancer Manage Res (2019) 11:1023–32. doi: 10.2147/cmar.S187597
11. Li C, Dong L, Su R, Bi Y, Qing Y, Deng X, et al. Homoharringtonine Exhibits Potent Anti-Tumor Effect and Modulates DNA Epigenome in Acute Myeloid Leukemia by Targeting Sp1/Tet1/5hmc. Haematologica (2020) 105(1):148–60. doi: 10.3324/haematol.2018.208835
12. Cao H, Cheng Y, You L, Qian J, Qian W. Homoharringtonine and Saha Synergistically Enhance Apoptosis in Human Acute Myeloid Leukemia Cells Through Upregulation of Trail and Death Receptors. Mol Med Rep (2013) 7(6):1838–44. doi: 10.3892/mmr.2013.1440
13. Tan M, Zhang Q, Yuan X, Chen Y, Wu Y. Synergistic Killing Effects of Homoharringtonine and Arsenic Trioxide on Acute Myeloid Leukemia Stem Cells and the Underlying Mechanisms. J Exp Clin Cancer Res (2019) 38(1):308. doi: 10.1186/s13046-019-1295-8
14. Zhang T, Shen S, Zhu Z, Lu S, Yin X, Zheng J, et al. Homoharringtonine Binds to and Increases Myosin-9 in Myeloid Leukaemia. Br J Pharmacol (2016) 173(1):212–21. doi: 10.1111/bph.13359
15. Chen XJ, Zhang WN, Chen B, Xi WD, Lu Y, Huang JY, et al. Homoharringtonine Deregulates Myc Transcriptional Expression by Directly Binding Nf-Kappab Repressing Factor. Proc Natl Acad Sci U.S.A. (2019) 116(6):2220–5. doi: 10.1073/pnas.1818539116
16. Wang L, You LS, Ni WM, Ma QL, Tong Y, Mao LP, et al. Beta-Catenin and Akt Are Promising Targets for Combination Therapy in Acute Myeloid Leukemia. Leuk Res (2013) 37(10):1329–40. doi: 10.1016/j.leukres.2013.06.023
17. Zhang W, Lu Y, Zhen T, Chen X, Zhang M, Liu P, et al. Homoharringtonine Synergy With Oridonin in Treatment of T (8, 21) Acute Myeloid Leukemia. Front Med (2019) 13(3):388–97. doi: 10.1007/s11684-018-0624-1
18. Zhou DC, Ramond S, Viguie F, Faussat AM, Zittoun R, Marie JP. Sequential Emergence of Mrp- and Mdr1-Gene Over-Expression as Well as Mdr1-Gene Translocation in Homoharringtonine-Selected K562 Human Leukemia Cell Lines. Int J Cancer (1996) 65(3):365–71. doi: 10.1002/(SICI)1097-0215(19960126)65:3<365::AID-IJC15>3.0.CO;2-9
19. Chen P, Zhan W, Wang B, You P, Jin Q, Hou D, et al. Homoharringtonine Potentiates the Antileukemic Activity of Arsenic Trioxide Against Acute Myeloid Leukemia Cells. Exp Cell Res (2019) 376(2):114–23. doi: 10.1016/j.yexcr.2019.02.008
20. Russo D, Michelutti A, Melli C, Damiani D, Michieli MG, Candoni A, et al. Mdr-Related P170-Glycoprotein Modulates Cytotoxic Activity of Homoharringtonine. Leukemia (1995) 9(3):513–6.
21. Wang F, Huang J, Guo T, Zheng Y, Zhang L, Zhang D, et al. Homoharringtonine Synergizes With Quizartinib in Flt3-Itd Acute Myeloid Leukemia by Targeting Flt3-Akt-C-Myc Pathway. Biochem Pharmacol (2021) 188:114538. doi: 10.1016/j.bcp.2021.114538
22. Zhang C, Lam SSY, Leung GMK, Tsui SP, Yang N, Ng NKL, et al. Sorafenib and Omacetaxine Mepesuccinate as a Safe and Effective Treatment for Acute Myeloid Leukemia Carrying Internal Tandem Duplication of Fms-Like Tyrosine Kinase 3. Cancer (2020) 126(2):344–53. doi: 10.1002/cncr.32534
23. Joshi SK, Nechiporuk T, Bottomly D, Piehowski PD, Reisz JA, Pittsenbarger J, et al. The Aml Microenvironment Catalyzes a Stepwise Evolution to Gilteritinib Resistance. Cancer Cell (2021) 39(7):999–1014.e8. doi: 10.1016/j.ccell.2021.06.003
24. Gregory MA, Nemkov T, Park HJ, Zaberezhnyy V, Gehrke S, Adane B, et al. Targeting Glutamine Metabolism and Redox State for Leukemia Therapy. Clin Cancer Res (2019) 25(13):4079–90. doi: 10.1158/1078-0432.CCR-18-3223
25. Short NJ, Konopleva M, Kadia TM, Borthakur G, Ravandi F, DiNardo CD, et al. Advances in the Treatment of Acute Myeloid Leukemia: New Drugs and New Challenges. Cancer Discovery (2020) 10(4):506–25. doi: 10.1158/2159-8290.CD-19-1011
26. Lagadinou ED, Sach A, Callahan K, Rossi RM, Neering SJ, Minhajuddin M, et al. Bcl-2 Inhibition Targets Oxidative Phosphorylation and Selectively Eradicates Quiescent Human Leukemia Stem Cells. Cell Stem Cell (2013) 12(3):329–41. doi: 10.1016/j.stem.2012.12.013
27. Klanova M, Andera L, Brazina J, Svadlenka J, Benesova S, Soukup J, et al. Targeting of Bcl2 Family Proteins With Abt-199 and Homoharringtonine Reveals Bcl2- and Mcl1-Dependent Subgroups of Diffuse Large B-Cell Lymphoma. Clin Cancer Res (2016) 22(5):1138–49. doi: 10.1158/1078-0432.CCR-15-1191
28. Hanahan D. Hallmarks of Cancer: New Dimensions. Cancer Discovery (2022) 12(1):31–46. doi: 10.1158/2159-8290.CD-21-1059
29. Sun LY, Li XJ, Sun YM, Huang W, Fang K, Han C, et al. Lncrna Anril Regulates Aml Development Through Modulating the Glucose Metabolism Pathway of Adipor1/Ampk/Sirt1. Mol Cancer (2018) 17(1):127. doi: 10.1186/s12943-018-0879-9
30. Chen WL, Wang JH, Zhao AH, Xu X, Wang YH, Chen TL, et al. A Distinct Glucose Metabolism Signature of Acute Myeloid Leukemia With Prognostic Value. Blood (2014) 124(10):1645–54. doi: 10.1182/blood-2014-02-554204
31. Ito H, Nakamae I, Kato J, Yoneda-Kato N. Stabilization of Fatty Acid Synthesis Enzyme Acetyl-Coa Carboxylase 1 Suppresses Acute Myeloid Leukemia Development. J Clin Invest (2021) 131(12):e141529. doi: 10.1172/jci141529
32. Subramanian A, Tamayo P, Mootha VK, Mukherjee S, Ebert BL, Gillette MA, et al. Gene Set Enrichment Analysis: A Knowledge-Based Approach for Interpreting Genome-Wide Expression Profiles. Proc Natl Acad Sci U.S.A. (2005) 102(43):15545–50. doi: 10.1073/pnas.0506580102
33. Martinez-Reyes I, Chandel NS. Cancer Metabolism: Looking Forward. Nat Rev Cancer (2021) 21(10):669–80. doi: 10.1038/s41568-021-00378-6
34. Pedroza-Torres A, Romero-Cordoba SL, Justo-Garrido M, Salido-Guadarrama I, Rodriguez-Bautista R, Montano S, et al. Micrornas in Tumor Cell Metabolism: Roles and Therapeutic Opportunities. Front Oncol (2019) 9:1404. doi: 10.3389/fonc.2019.01404
35. Michaud L, Touijer KA, Mauguen A, Zelefsky MJ, Morris MJ, Lyashschenko SK, et al. C-Choline Pet/Ct in Recurrent Prostate Cancer: Retrospective Analysis in a Large U.S. Patient Series. J Nucl Med (2020) 61(6):827–33. doi: 10.2967/jnumed.119.233098
36. Giovacchini G, Guglielmo P, Mapelli P, Incerti E, Gajate AMS, Giovannini E, et al. C-Choline Pet/Ct Predicts Survival in Prostate Cancer Patients With Psa < 1 Ng/Ml. Eur J Nucl Med Mol Imaging (2019) 46(4):921–9. doi: 10.1007/s00259-018-4253-3
37. Pucci S, Fasoli F, Moretti M, Benfante R, Di Lascio S, Viani P, et al. Choline and Nicotine Increase Glioblastoma Cell Proliferation by Binding and Activating Alpha7- and Alpha9- Containing Nicotinic Receptors. Pharmacol Res (2021) 163:105336. doi: 10.1016/j.phrs.2020.105336
38. Trousil S, Lee P, Pinato DJ, Ellis JK, Dina R, Aboagye EO, et al. Alterations of Choline Phospholipid Metabolism in Endometrial Cancer Are Caused by Choline Kinase Alpha Overexpression and a Hyperactivated Deacylation Pathway. Cancer Res (2014) 74(23):6867–77. doi: 10.1158/0008-5472.CAN-13-2409
39. Jia M, Andreassen T, Jensen L, Bathen TF, Sinha I, Gao H, et al. Estrogen Receptor Alpha Promotes Breast Cancer by Reprogramming Choline Metabolism. Cancer Res (2016) 76(19):5634–46. doi: 10.1158/0008-5472.CAN-15-2910
40. Thomas AM, Manghi P, Asnicar F, Pasolli E, Armanini F, Zolfo M, et al. Metagenomic Analysis of Colorectal Cancer Datasets Identifies Cross-Cohort Microbial Diagnostic Signatures and a Link With Choline Degradation. Nat Med (2019) 25(4):667–78. doi: 10.1038/s41591-019-0405-7
41. Wang Y, Zhang L, Chen WL, Wang JH, Li N, Li JM, et al. Rapid Diagnosis and Prognosis of De Novo Acute Myeloid Leukemia by Serum Metabonomic Analysis. J Proteome Res (2013) 12(10):4393–401. doi: 10.1021/pr400403p
42. Musharraf SG, Siddiqui AJ, Shamsi T, Choudhary MI, Rahman AU. Serum Metabonomics of Acute Leukemia Using Nuclear Magnetic Resonance Spectroscopy. Sci Rep (2016) 6:30693. doi: 10.1038/srep30693
43. Lo Presti C, Fauvelle F, Mondet J, Mossuz P. The Differential Activation of Metabolic Pathways in Leukemic Cells Depending on Their Genotype and Micro-Environmental Stress. Metabolomics (2020) 16(1):13. doi: 10.1007/s11306-020-1633-z
44. Gallego-Ortega D, Ramirez de Molina A, Ramos MA, Valdes-Mora F, Barderas MG, Sarmentero-Estrada J, et al. Differential Role of Human Choline Kinase Alpha and Beta Enzymes in Lipid Metabolism: Implications in Cancer Onset and Treatment. PloS One (2009) 4(11):e7819. doi: 10.1371/journal.pone.0007819
45. Dean JM, Lodhi IJ. Structural and Functional Roles of Ether Lipids. Protein Cell (2018) 9(2):196–206. doi: 10.1007/s13238-017-0423-5
46. Vijay R, Hua X, Meyerholz DK, Miki Y, Yamamoto K, Gelb M, et al. Critical Role of Phospholipase A2 Group Iid in Age-Related Susceptibility to Severe Acute Respiratory Syndrome-Cov Infection. J Exp Med (2015) 212(11):1851–68. doi: 10.1084/jem.20150632
47. Wong LR, Zheng J, Wilhelmsen K, Li K, Ortiz ME, Schnicker NJ, et al. Eicosanoid Signaling Blockade Protects Middle-Aged Mice From Severe Covid-19. Nature (2022) 605(7908):146–51. doi: 10.1038/s41586-022-04630-3
48. Liu H, Xu R, Gao C, Zhu T, Liu L, Yang Y, et al. Metabolic Molecule Pla2g2d Is a Potential Prognostic Biomarker Correlating With Immune Cell Infiltration and the Expression of Immune Checkpoint Genes in Cervical Squamous Cell Carcinoma. Front Oncol (2021) 11:755668. doi: 10.3389/fonc.2021.755668
49. Williams KA, Lee M, Hu Y, Andreas J, Patel SJ, Zhang S, et al. A Systems Genetics Approach Identifies Cxcl14, Itgax, and Lpcat2 as Novel Aggressive Prostate Cancer Susceptibility Genes. PloS Genet (2014) 10(11):e1004809. doi: 10.1371/journal.pgen.1004809
Keywords: homoharringtonine, AML, ether lipid metabolism, RNA-seq, choline metabolism
Citation: Zhang Y, Li N, Chang Z, Wang H, Pei H, Zhang D, Zhang Q, Huang J, Guo Y, Zhao Y, Pan Y, Chen C and Chen Y (2022) The Metabolic Signature of AML Cells Treated With Homoharringtonine. Front. Oncol. 12:931527. doi: 10.3389/fonc.2022.931527
Received: 29 April 2022; Accepted: 18 May 2022;
Published: 14 June 2022.
Edited by:
Jian Yu, Beihang University, ChinaCopyright © 2022 Zhang, Li, Chang, Wang, Pei, Zhang, Zhang, Huang, Guo, Zhao, Pan, Chen and Chen. This is an open-access article distributed under the terms of the Creative Commons Attribution License (CC BY). The use, distribution or reproduction in other forums is permitted, provided the original author(s) and the copyright owner(s) are credited and that the original publication in this journal is cited, in accordance with accepted academic practice. No use, distribution or reproduction is permitted which does not comply with these terms.
*Correspondence: Yun Chen, Y2hlbnk2NTNAbWFpbC5zeXN1LmVkdS5jbg==; Chun Chen, Y2hlbmNodW5AbWFpbC5zeXN1LmVkdS5jbg==; Yihang Pan, cGFueWloQG1haWwuc3lzdS5lZHUuY24=
†These authors have contributed equally to this work