- 1Chitkara College of Pharmacy, Chitkara University, Punjab, India
- 2Department of Biosciences, Shifa Tameer-e-milat University, Islamabad, Pakistan
- 3Yunnan Herbal Laboratory, College of Ecology and Environmental Sciences, Yunnan University, Kunming, China
- 4Maharishi Markandeshwar (MM) School of Pharmacy, Maharishi Markandeshwar University, Sadopur-Ambala, India
- 5Maharishi Markandeshwar (MM) College of Pharmacy, Maharishi Markandeshwar (Deemed to be University), Mullana-Ambala, India
- 6Department of Biotechnology, Parul Institute of Applied Sciences and Animal Cell Culture and Immunobiochemistry Lab, Centre of Research for Development, Parul University, Vadodara, India
- 7Department of Pharmacy, Hazara University, Mansehra, Pakistan
- 8Faculty of Pharmaceutical Sciences, Government College University, Faisalabad, Pakistan
- 9Department of Pharmaceutics, NITTE Deemed-to-be University, NGSM Institute of Pharmaceutical Sciences, Mangalore, India
- 10Department of Pathology, College of Korean Medicine, Kyung Hee University, Seoul, South Korea
- 11Department of Pharmaceutical Sciences, Pharmacy Program, Batterjee Medical College, Jeddah, Saudi Arabia
- 12Pharmacology Department, Faculty of Veterinary Medicine, Suez Canal University, Ismailia, Egypt
- 13Department of Medical Laboratories, College of Applied Medical Sciences, Qassim University, Buraydah, Saudi Arabia
- 14Department of Pharmacy, BGC Trust University Bangladesh, Chittagong, Bangladesh
- 15Department of Pharmacy, Faculty of Allied Health Sciences, Daffodil International University, Dhaka, Bangladesh
There are more than two hundred fifty different types of cancers, that are diagnosed around the world. Prostate cancer is one of the suspicious type of cancer spreading very fast around the world, it is reported that in 2018, 29430 patients died of prostate cancer in the United State of America (USA), and hence it is expected that one out of nine men diagnosed with this severe disease during their lives. Medical science has identified cancer at several stages and indicated genes mutations involved in the cancer cell progressions. Genetic implications have been studied extensively in cancer cell growth. So most efficacious drug for prostate cancer is highly required just like other severe diseases for men. So nutraceutical companies are playing major role to manage cancer disease by the recommendation of best natural products around the world, most of these natural products are isolated from plant and mushrooms because they contain several chemoprotective agents, which could reduce the chances of development of cancer and protect the cells for further progression. Some nutraceutical supplements might activate the cytotoxic chemotherapeutic effects by the mechanism of cell cycle arrest, cell differentiation procedures and changes in the redox states, but in other, it also elevate the levels of effectiveness of chemotherapeutic mechanism and in results, cancer cell becomes less reactive to chemotherapy. In this review, we have highlighted the prostate cancer and importance of nutraceuticals for the control and management of prostate cancer, and the significance of nutraceuticals to cancer patients during chemotherapy.
1 Introduction
Natural derivatives are excellent sources of bioactive composites and are widely distributed as the most efficacious modern medicines (1–3). In recent years, several researchers attained a lot of interest in the natural dietary agents, due to their therapeutic potential in cancer suppression and lowering the threat of cancer cell development (4). Nutraceuticals are characterized as an emerging food category that includes dietary components, and delivered the benefits to keep balance in health by improving nutritious standards. Nutraceuticals are predicted to have relatively lower toxicity and are associated with adverse effects as compared to traditional synthetic medications, which are used to cure identical symptoms since they are derived from natural nutritional resources; but have presented dose-dependent effects (5–11). Nutraceuticals act as an interface between, nutrition and pharmaceuticals (12). It may be challenging to consume the entire nutrients required for the maintenance of normal physiological and physical health. The amalgamation of novel nutraceutical derivatives with foodstuff are easy to consume and becomes functional foods for body (13).
In recent decades, the combination between nutraceuticals and nanotechnology has received a lot of attention from several research organizations. Unfortunately, several nutraceutical products are of major concerned, because it has less benefits to health, due to their weak physicochemical characteristics such as poor absorption, less stability, lower water solubility, and probable chemical alterations after their administration. Nanotechnology could be considered as a breakthrough in activating the therapeutic characteristics of nutraceutical products for the human well-being as immunity booster and protect the body from forigen harmful entities. Variety of ailments based on their nutraceuticals potential efficacy and limiting bioavailibilty aspects. As a result, nanotechnology could be a new frontier in the development of novel supplementary nutritional products with less adverse effects and more health benefits (14). Several nutraceuticals such as quercetin, curcumin, coenzyme Q, thymoquinone, and green tea polyphenols have been delivered into nanoparticles and are effective in ‘‘nano chemotherapy” and ‘‘nano chemoprevention’’ (4). The various role of nanotechnology in the delivery of nutraceuticals is illustrated in Figure 1.
2 Cancer and Challenges Faced for Treatment Using Traditional Approaches
Cancer is a hazardous, life-threatening ailment having the utmost challenging afflictions worldwide (15). It portrays enormously alarming circumstances that are illustrated by unregulated cell growth, resulting in the incursion of nearby tissues and oftenly extending to other sections of body (16).
Regardless, the usual methodology of cancer treatment has been notclear; it relies on surgery, chemotherapy, and radiotherapy. Usually, radiotherapy and surgical resection are considered asoften successful techniques in the abolition of the primary tumor, even though disease relapse due to metastasis or residual cancerous cells is a ubiquitous problematic issue. As a result, chemotherapy is frequently used to address these issues (17).
There are a number of therapy options available to men diagnosed with localized prostate cancer—that is, illness that hasn’t progressed beyond the prostate region—based on the stage and grade of the disease (potential aggressiveness of the tumor). Some patients may have surgery on their own. Radiation treatment may be the sole option for some.
Some people may have both of these conditions at the same time. When there’s a fear that the operation didn’t remove all of the tumor tissue, this is a common reaction. Radiation treatment may also be advised if a patient’s PSA levels begin to increase months or even years after surgery, even if imaging has not been able to detect tumor development. A shorter and more intense course of radiation treatment after surgery is safe for many patients with prostate cancer. Aside from destroying or slowing the development of cancer cells, radiation may harm healthy cells in the area as well. Side effects are possible if healthy cells are damaged.
Radiation treatment often leaves its victims exhausted. To be fatigued, one must feel drained and worn out on a regular basis. When it happens, it might either come on quickly or gradually. If you’re having the same quantity of radiation treatment to the same place of your body as someone else, you may experience weariness in different ways.
The use of hypofractionated radiation therapy to treat prostate cancer has already been acknowledged by certain patients who are receiving radiation therapy alone for the disease. However, it’s not known whether this form of radiation treatment should be used after surgery.
There are several sensitive sites in your bladder and rectum that might be targeted by radiation following surgery. Radiation-damaged healthy cells often recover within a few months of therapy ending. It’s possible, though, that some individuals may have adverse effects that don’t go away. In other cases, symptoms may not appear for many months or even years after the completion of radiation treatment.
According to previous research it has been observed that several plant-based medicines play a vital role in molecular and cellular processes that underlie tumor development. Numerous chemotherapeutic agents for cancer treatment are produced from plants such as vinca alkaloids (e.g.,vincristine and vinblastine) and Taxol (Taxus brevifolia). Nutritional dietary agents could be advantageous in cancer treatment. Several evidences revealed that meals that are relatively lower in carbohydrates and higher in high-quality proteins, fats, and fibers are considered to be beneficial for cancer patients. In addition, nutraceutical products may also be advantageous in the reduction of toxicity and adverse effects allied with radiation therapy and chemotherapy, and provide improved living circumstances by plummeting tumor cachexia (18). This prevalent usage of nutraceuticals has receiveda lot of consideration for the importance of dietary nutrients in cancer pathogenesis (19).
3 Why Nutraceuticals Required for Cancer
Although numerous anticancer medications are available commercially, but the advent of acquired drug resistance, as well as the extreme side effects of these widely used treatments are main problems in efficacious chemotherapy. As a result, it is suggested that newer and innovative drugs have to be designed rationally with fewer adverse effects (20).
Nutritional dietary components and phytochemicals have a long and illustrious history, as well as substantial applications in the field of modern medicines. Nutraceuticals can influence DNA transcription and regulate the factors responsible for DNA damage in tumor cells (21). They have shown to re-sensitize drug-resistant tumors due to their pleiotropic property and capability to affect various signaling pathways (AMPK signalling pathway, EGF-mediated signalling pathways, NF-kB signalling pathway etc), which is a positive attribute of natural components. Nutraceuticals target the cancerous cells at multiple levels by acting on their molecular targets and cause cell cycle arrest or apoptosis by inhibiting the proliferation of cancer cells, and suppression of metastasis, invasion, or angiogenesis (22). They stop cancer from spreading by inhibiting the signaling pathways that are essential for cancer progression (23). For instance, Oleuropein reduces cell proliferation primarily through two mechanisms: on the one hand, it acts by inhibiting the cell cycle via upregulation of cyclin-dependent kinase (CDK) inhibitors, and on the other hand, it modulates the genic expression responsible for the induction of intrinsic and extrinsic pathways of apoptosis via the upregulation of p53 and p21. In addition, oleuropein may change the activity of critical molecules implicated in the initiation and progression of cancer, including MAPKs, the c-Met proto-oncogene, and the fatty acid synthase (FASN) enzyme (24). Demidenko et al. found that luteolin inhibited cancer cell proliferation throughout the G1/S and G2/M stages by inhibiting the HT-29 cell cycle (25). In addition, luteolin inhibits the overexpression of certain antiapoptotic proteins in afflicted cells and regulates the expression and activity of CDC2 (CDK1) kinase and cyclin B1 proteins, which trigger the G2/M transition phases in luteolin-treated colon cancer cell lines.
Many nutraceutical products such as soy isoflavones, curcumin, resveratrol, indole-3-carbinol, lycopene, green tea polyphenols, epigallocatechin-3-gallate, and 3,3’-diindolylmethane (DIM) cause downregulation of signal transductions such as Akt, PI3K, NFkB, mTOR and other pathways that are required for cancer progression (26). Some of them has been tabulated as Table 1.
Nutraceuticals have a great potential to modulate various molecular targets, such asgrowth factors [e.g., epidermal growth factor receptor (EGFR), insulin-like growth factor-1 receptor (IGF-1R), HER2, and VEGFR], transcription factors [e.g., STAT3, NF-κB, NRF-2, activator protein (AP-1), HIF-1α and peroxisome proliferator-activated receptor (PPARγ)], protein kinases [e.g., Bcr-abl, phosphoinositide 3-kinase (PI3K), Raf/Ras and AMPK], inflammatory mediators [e.g., TNF-α, 5-LOX, COX-2, CRP, IL-6, IL-8, and iNOS], and other targets that are involved in cancer progression (116). These therapeutic characteristics make nutraceuticals good candidates for suppressing carcinogenesis and improving treatment results in cancer patients (22). The molecular targets and mechanism of action of nutraceutical products in prostate cancer therapy are depicted in Figure 2.
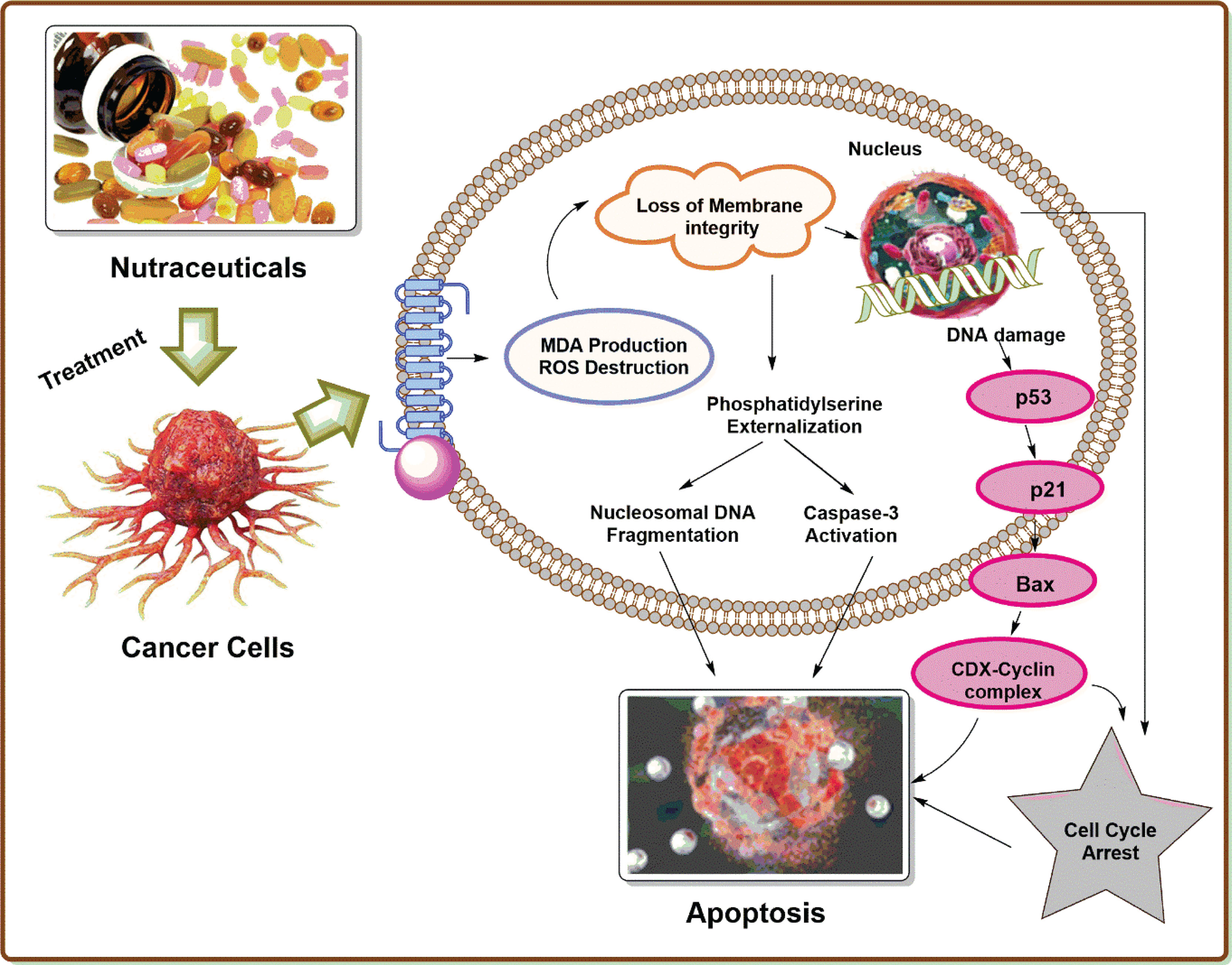
Figure 2 Molecular targets and mechanism of action of nutraceutical products in prostate cancer therapy.
4 Prostate Cancer
Cancer is the leading cause of death worldwide, where the mortalilty rate of cancer is increasing dramatically. Majority of the cancer cases in the world is represented by prostate cancer (PC) with an estimated value of 13.5% (117). PC is well known as a non-skin cancer and is considered as the most common form of male cancer in the world (118). The abnormal growth of cells from the prostate gland leads to the development of PC. It is slowly growing cancer that spreads tumor cells to the other parts of the body, especially to the bones and upper lymph nodes. The major risk factor for the development PC in men is their age, race, and family history of disease. In the first stage, PC is less pronounced. In the later stages Lower Urinary Tract Symptoms (LUTS) including pain and urinary incontinence, presence of blood in the urine, back pain, and pain in the pelvis region have been reported (119). The risk of death from the PC is less malignant as the slow growth of tumor cells is not fatal to the patient, and hence patient can survive with proper and effective treatment strategy. Deaths from PC occur in the metastasis stage, which is the worst part of cancer, where it spread to almost every organ of the body, including the spine, rectum, brain, bone, and lymph nodes (120). In general, PC is rare for the people below 50 years of age. The average age of the patient with PC is between 72-74 years, and about 85% of patients are diagnosed with it above the age of 65. Due to the genetic predisposition, the incidence rate of PC is high in families when compared with other forms of cancer. About 10-15% of patients diagnosed with PC will have at least one relative with the disease, and the first relatives of patients with PC are two to three times more likely to be affected with it. According to GLOBCAN 2020, PC is another common cancer after lung cancer affects men worldwide, including an estimate of 1,414,259 young people and 375,304 deaths by 2020. The incidence and mortalilty rate of PC in the world is associated with increase in the age on diagnosis (121). The incidence rate of PC increases with age, where among 350 men one under the age of 50 are diagnosed with PC. In every 52 males 1 between the age of 50-59 increases the incidence of PC, even 60% men above the age limit of 65 also increases the incidence rate of PC (122). The mortality rate of PC increases with the age and approximately 55% of deaths occur above the age of 65.
PC is mainly associated with the prostate gland. The prostate gland is a part of male reproductive system that produces alkaline prostatic fluid to maintains the health and function of the sperm (123). The prostate grows and matures quickly under normal circumstances as circulating androgen levels rises during adolescence (124). The prostate can be prone to inflammation, hyperplasia, and cancer, that can alter testosterone-regulated growth and function (125). The entire structure of prostate has been altered by cancerous growth due to the effects of increasing levels of androgens (126).
PC is caused by environmental factors, diet, hormones, lifestyle factors, and a person’s genetic history (127). PC is mainly related to the western lifestyle, especially foods high in fat, meat, and dairy products. PC occurs due to the abnormal growth in cells of prostate gland. This tumor growth is followed by initial mutations and genetic mutations including the p53 gene and retinoblastoma, ultimately leading to tumor progression and metastasis. As PC invades the area, abscesses in the temporal area spread to the neck of the bladder, and the peripheral-zone abscesses extend into the ducts and seminal vesicles. About 90% of PCs are adenocarcinomas. Squamous cell carcinomas make up less than 1% of all prostate carcinomas. In PC, 70% come from the surrounding region, 15-20% from the central region, and 10-15% from the temporal area. Most importantly, PC has multifocal and coordinated involvement of many prostate sites due to clonal and nonclonal cancer cells.
The treatment of PC and its recommendation rely on most of the factors which includes, possible side effect, type, stage of cancer, patient’s preferences, and overall health condition. Along with all treatments, patients should be monitored closely to demonstrate clinical, biomedical, and radiological progress. Repeated photography and baseline scanning throughout 3–6 months is highly recommended as a major challenge in determining appropriate treatment (128). The treatment policy of PC covers three approaches- radiation, surgery, and chemotherapy. The pharmacological agents available for the treatment of PC include antineoplastics, systemic antifungals, chemotherapy modulating agents, endocrine monoclonal antibodies, corticosteroids, bisphosphonate derivatives and radiopharmaceuticals (129). Docetaxel chemotherapy was the first treatment that showed improvement in PC. Survival benefits have been seen in all age groups of patients affected with PC, followed by the established form of docetaxel injected three times a week in 10 cycles as first-line chemotherapy. The side effects of docetaxel are similar to those seen with other medications such as nausea and vomiting. The main drawback of this drug is that it is associated with motor and sensory peripheral neuropathy. Moreover, testing for men with recurrent disease is higher, especially after more than six months of relief from docetaxel exposure (130). Cabaxitaxel is another PC chemotherapy medication, approved by FDA. The main drawback of this drug in patients receiving toxic substances such as neutropenic sepsis cannot tolerate the side effects produced by this drug. Concomitant steroids and antiemetics are offered to reduce the side effects produced by Cabaxitaxel (131). Abiraterone which falls under hormone therapy, is a selective inhibitor of cytochrome p450 17A1 (CYP17). The drug Abiraterone is available in the form of oral dosage form and is given in combination with prednisolone in low doses. The common side effects of this drug include an increase in mineral corticoid levels, leading to hypertension, hypokalaemia, and fluid retention (132). A major risk factor for this drug is high levels of transaminase, which may interfere with liver function over time. Enzalutamide is a novel antiandrogen that has shown significant antitumor activity before and after PC chemotherapy. The limitation of enzalutamide is the occurrence of seizures reported in less than 1% of patient treated with it (133). Radium-223 is a radiopharmaceutical compound known as alpharadin to treat PC (134). The drug attracts double-stranded DNA, breaks down nearby cancer cells simultaneously, and saves normal tissue without significant visual effects. One of this drug’s most common side effects is bone pain because it is not recommended for patients with arthritis. Sipuleucel-T is the only immunomodulatory agent approved to treat PC and the first FDA-approved medical vaccine. The main limitation of this drug is that it affects sexual and reproductive problems in patients (135).
4.1 Pathophysiology for PCa
Androgen receptor (AR) signalling is crucial for prostate differentiation and function, as well as PC development and progression. A single copy gene on the X-chromosome encodes the human AR protein (Xq11.2-q12). It is a 919-amino-acid protein that may vary in length due to poly-glutamine, poly-glycine, and poly-proline repeats of varying lengths. The length of poly-glutamine repeats has been linked to receptor activity levels. The length of the repetitions varies from 9 to 36 residues, with an average of 18 to 22 repeats. Spinal and bulbar muscle atrophy are linked to very lengthy repetitions (136, 137). Although there is some indication that the length of the poly-glutamine repeat is linked to the risk of PC, epidemiological studies have revealed no substantial link (138).
AR, like other nuclear receptor family ligand-activated transcription factors, has three main domains: an amino-terminal transcriptional activation domain (NTD), a DNA-binding domain (DBD) with two zinc finger motifs that determine the DNA sequences recognised by the receptors, and a carboxyl terminal ligand-binding domain (LBD) that provides the regulatory switch by which androgens control the receptor’s transcriptional activity. A nuclear localization signal is seen in the hinge region (H), which joins the DBD with the LBD. High-affinity DNA binding is also facilitated by a portion of the hinge region (138).
Androgen-receptor (AR) protein is stabilised and protected from degradation by heat shock proteins when androgens aren’t present (Figure 3). The two primary ligands of the AR, testosterone and dihydrotestosterone, control its activity (DHT). Prostate 5-reductase converts testosterone into the more powerful metabolite DHT, which is generated by testicular Leydig cells. In terms of AR binding affinity, DHT has a nearly 10-fold advantage over testosterone. The phosphorylation of numerous serine residues occurs as a consequence of DHT binding to the AR. Protection against proteolytic degradation, stability, and transcriptional activation are all possible outcomes of AR phosphorylation (139). AR transactivation is regulated by a number of coregulatory proteins that are able to react to changes in the microenvironment to control particular gene targets that are critical for cell growth and survival (140). There is a natural balance between cell proliferation and cell death in the normal prostate epithelium, but this equilibrium is broken in PC, resulting in tumor development (141).
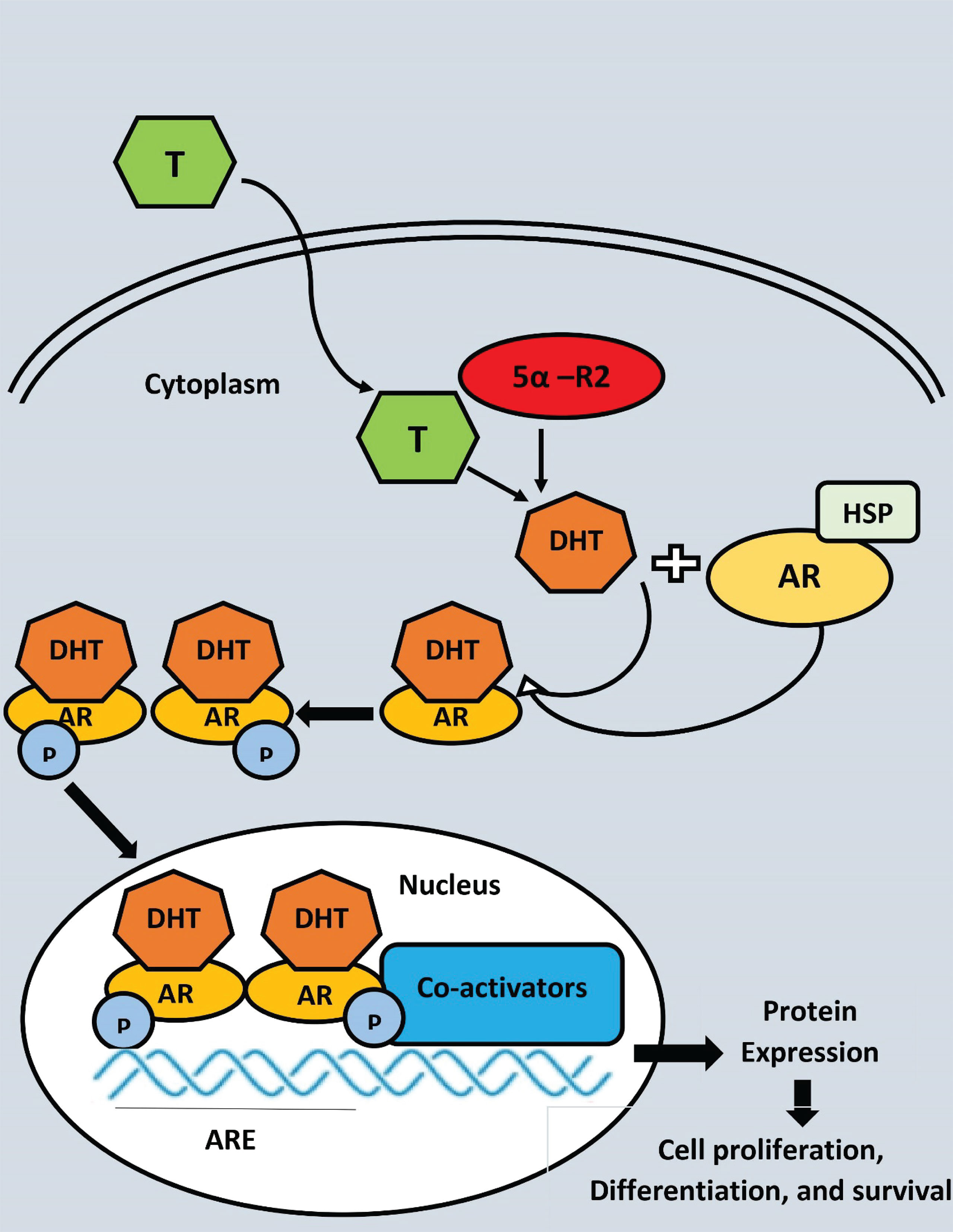
Figure 3 Androgen receptor (AR) ligand-dependent gene transactivation mechanism 5-reductase converts testosterone (T) to dihydrostosterone (DHT) in theprostate epithelical cell. DHT binding to AR causes dissociation of the AR-HSP (Heat shock protein) complex, dimerization, and nuclear translocation. AR binds to androgen response elements (ARE) in DNA and recruits co-activators to enhance transcription, where P, Phosphate group.
4.2 Nanonutraceuticals Based Approaches for Prostate Cancer
Nanotechnology deals with wide range of technologies, materials, and production processes for the development of many medical products. The origins of nanotechnology create a variety of opportunities in various fields, maximum benefits are especially observed in the field of nanomedicine. Over the past two decades, the successive generation of nanoscale science and nanotechnology has been responsible for significant growth in the field of nanomedicine. At present, nanotechnology offers various benefits in the development of novel anticancer drugs that help to increase the immune system strength as compare to traditional medicine. Drug treatment associated with PC depends on the severity of the disease, especially the two types of methods have been considered for PC treatment. In the first case, early detection and pharmacotherapy are recommended and other forms of pharmacotherapy after surgical removal or radiation treatment has been recommended (142). Conventional treatment with the use of drug cells and nanotherapy may not work as the body tissue of the tumor patients and drug resistance varies greatly while simultaneously increasing the dose leads to systemic drug accumulation and toxicity. Therefore, it is an urgent requirement of improved drug treatment by increasing specificity by reducing systemic toxicity. The development of controlled nanocarriers with improved safety and efficacy, which could meet the clinical requirements for disease intensification and the production of a suitable clinical protocol has been widely accepted. With the discovery of newly identified approaches to relevant clinical challenges, nanotechnology plays a vital role in the treatment of PC (143).
The benefits listed above can be listed as targeted drug delivery for tumors, the onset of apoptosis, and drug accumulation in targeted tissues to increase the exposure of cancer cells (144). Despite the complex nature of the need for a natural origin for drug possibilities, studies began to focus on the possible additional use of dietary products that could be used to prevent, treat (or) delay the onset of certain health problems (145). Nutraceuticals, a compound name derived from ‘nutrients’ and’ medicines’, are defined as’ Phyto complex if their origin comes from the diet of vegetables and secondary metabolites when found in animal foods, are concentrated and mistreated (146). The most effective and promising way to support nutraceuticals’ health benefits is by the development of nutraceuticals at the nano level. Nanonutraceuticals provide greater safety and efficacy when used to treat health conditions, especially in those patients who cannot afford standard medical treatments. In addition to conventional therapy, a diet high in vegetables and fruits was associated with PC, suggesting that the disease could be prevented to some degree by changing lifestyle (or) eating habits (147). Lycopene, the primary carotenoid in tomato, has been linked to a reduced risk of prostate cancer, and preclinical studies have showed encouraging findings in normal prostate tissue showing tomato and lycopene may suppress androgen signaling. Live-cell Raman microscopy was used by Scarpitti et al. to study the transport of lycopene into PC-3 prostate cancer cells (148). In order to overcome lycopene’s low aqueous solubility and the difficulty of replicating physiological uptake by cells, the tween 80 micelle mimics natural lipoprotein complexes that deliver lycopene in vivo. It also provides a stable signal for assessing cellular uptake of the nutraceutical formulation. The Raman pictures show the lycopene’s subcellular distribution in the cells. At 532 nm, the Raman signal for lycopene is resonantly amplified, enabling a simple, sensitive, and label-free method to detect and quantify lycopene absorption in live cells. A reduction in local androgen regulatory signals and the production of insulin growth factor type-1 (IGF-1) and interleukin 6 were shown to enhance the rate of necrosis in mice prostate cells when lycopene was applied (149). For example, antioxidative, cell progression, apoptotic and insulin growth factor type 1 inhibitors of lycopene have been identified (150–152). In the past, researchers have shown a link between rising IGF-I blood levels and an increased risk of cancer, particularly prostate cancer. In transgenic rats, a higher frequency of prostatic intraepithelial neoplasia (PIN) was associated with higher levels of IGF-1 expression on the prostatic epithelium. As a result, IGF-1 was not only linked to an increased risk of prostate cancer, but it was also playing a role in carcinogenesis by promoting cell proliferation and interfering with the process of cell death (153, 154). Lycopene’s ability to suppress IGF-1 might be a major factor in preventing prostate cancer.In the field of targeted medication delivery, liposomes are the most widely used and thoroughly studied nanocarriers. Stabilizing therapeutic chemicals, overcoming barriers to cellular and tissue absorption, and enhancing biodistribution of compounds to target areas in vivo have all contributed to better therapeutics for a variety of biomedical applications (155–158). There are liposomes that have distinct aqueous regions that are made up of one or more concentric bilayers of lipid. Liposomal vesicles are able to encapsulate a wide spectrum of medications because of their unique capacity to encapsulate both lipophilic and hydrophilic molecules. There are hydrophilic and hydrophobic molecules in the aqueous core of the bilayer membrane A variety of macromolecules, including as DNA, proteins, and imaging agents, may be delivered through the vast aqueous core and biocompatible lipid shell (159–169). Drug delivery systems like liposomes have a broad variety of biophysical and physicochemical features that may be manipulated to alter their biological qualities. Particle size, charge, the number of lamellae, the lipid content, and surface modification with polymers and ligands all influence the stability of liposomal formulations in both in vitro and in vivo (170–177). Due to their natural phospholipid composition, liposomes are commonly thought to be pharmacologically non-toxic with minimal side effects. However, a growing number of studies have shown that liposomes may not be as immune-inert as once thought (178–183).
The use of liposomes in medicine opens up a world of therapeutic possibilities for a broad variety of diseases. Research into lipid-based medication delivery has grown significantly in the experimental in vitro and in vivo phases in the last 50 years since liposomes were first discovered. The use of liposomes in the administration of a broad variety of therapeutic and diagnostic substances and agents, such as drug molecules, gene therapy, and bioactive agents, is well-established in the field of liposome technology. There are several ways to increase the effectiveness of these formulations, including as altering the lipid composition, the charge of the lipid, and the inclusion of surface coatings or ligands (184–194).
Curcuma longa (curcumin) is a well-studied nutraceutical derived from the turmeric plant in its purest crystalline form (195) and has been carefully described in several studies. Various signalling pathways, including mitogen-activated protein kinase (MAPK), epidermal growth factor receptor (EGFR), and nuclear factor B (NFB), have been found to suppress PC cell proliferation and invasion and cause apoptosis in vitro and in vivo (196–198). Genes involved in inflammation, cell proliferation, and cell survival are heavily regulated by the NF-kB transcription factor. Many NF-kB-regulated genes, including as COX-2 (Cyclooxygenase-2), 5-LOX (5-lipoxygenase), TNF (Tumor necrosis factor), IL-6 (Interleukin 6), and EGFR tyrosine kinase activity, have been shown to be inhibited by curcumin (199–202). As part of the steroid receptor family, androgens play a critical role in the development and progression of PC (203, 204). To promote PC aggressive growth, aberrant activation of androgen signalling is caused by AR mutation and amplification (205). ARs and AR-associated cofactors have been shown to be suppressed by curcumin (206, 207). NKX3.1 (NK3 Homeobox 1), KLK3/(PSA) (Kallikrein related peptidase 3/Prostate-specific antigen), TMPRSS2 (Transmembrane serine protease 2), and TMPRSS2 (Transmembrane serine protease 2) were all downregulated by curcumin in both androgen dependent LNCaP and androgen-independent C4-2B cells. Nearly 90% of cancer-related fatalities are the result of metastasis (206, 207), which is a condition characterised by rapid cell proliferation. Understanding that several cell signalling pathways are disrupted in PC growth and bone metastases, the majority of PC medicines target particular targets. Thangapazham et al., formulated a liposomal formulation of curcumin to enhance curcumin’s anticancer activity against PC (208). The liposome of curcumin composed of dimyristoyl phosphatidyl choline (DMPC) and cholesterol as a primary ingredient for its preparation. The average particle size of liposomes was found to be 100-150 nm. When cells were exposed to DMPC liposomal curcumin (5-10 M) for 24-48 hours at 37 C, cell growth was 70-80% inhibited. Free curcumin, on the other hand, only showed comparable inhibition at levels ten times greater (>50 M). LNCaP cells were likewise shown to be more responsive to liposomal curcumin-mediated inhibition of cell growth than C4-2B cells. Curcumin liposomes and free curcumin both have a positive effect on LNCaP and C4-2B cells, with 31 and 70 percent of LNCaP cells surviving 10 M liposomal and free curcumin therapy, respectively. PC cell growth was inhibited more effectively by DPPC and DMPC liposomal curcumin than free curcumin. However, DMPC liposomal curcumin was shown to be the most effective of the liposomes examined.
Phan et al., prepared genistein loaded liposomes and stealth liposomes (GenLip) as a novel nanocarrier to enhance the solubility, bioavailability, pharmacokinetic properties, and cytotoxicity of genistein for specific induction of apoptosis in breast, ovarian and PC (209). The conventional and stealth liposomes containing phospholipid and cholesterol boosted genistein’s solubility, stability, and extended-release profile. The antioxidant activity showed peroxide neutralization in fluorescent probe oxidation assay quantitatively and microscopically for GenLip. The anticancer activity of GenLip was performed in a murine and human cancer cell line in a concentration and time-dependent manner. They performed the pro-apoptopic activity whereGenLip has maximum P53-independent apoptotic pathway markers compared with all treatments (209).
Silibinin and cabazitaxel based liposomes were prepared by Mahira et al, using ethanol injection based approaches (210). Lipids along with silibinin and cabazitaxel were dissolved in ethanol solvent, and TPGS was added with constant stirring to form liposomes during evaporation of solvents. The liposomes thus prepared has particle size of 100nm and showed enhanced antitumor activity on PC cell lines, indicating the potential for co-loading the molecules. The Hyaluronic acid based liposomes interfered in the G2/M cell cycle arrest causing apoptosis. The presence of HA caused increased in delivery of entrapped molecules into the CD44 expressing cells, and suppressing them (210).
The EMT, STST3, and AKT pathways, all of which are necessary for the evolution of PC, were inhibited by plumbagin therapy in the PTEN deletion PC mouse model, as described by Hafeez et al. (211). Oncogenic cells need high glucose absorption in order to fulfil their energy and anabolic demands in order to maintain fast proliferation and angiogenesis; as a result, cancer cells overexpress the GLUT transporter family, which consists of 14 members (212). Glucose transport in cells is increasingly being implicated in oncogenesis and tumor suppression, according to mounting evidence (213). This suggests that GLUT receptor expression might be suppressed in order to better our knowledge of the illness as well as to decrease tumor development. Genistein, a naturally occurring isoflavone, has been shown to have several health advantages, including anticancer properties (214). Genistein has been shown to increase apoptosis in hepatocellular carcinoma (HCC) via inactivating GLUT1 and thereby reducing aerobic glycolysis. PC cell lines were investigated by Chandler et al. for the presence of GLUT1 and GLUT12 mRNA and protein (215). The GLUT1 and GLUT12 proteins were found in the plasma membrane and cytoplasm by immunofluorescence. Tumors in the prostate, both benign and malignant, have various GLUT proteins (216). Experimentation with genistein on PC cells has demonstrated that Bax expression is increased, apoptotic signals are stimulated, and the anticancer effect of Cabazitaxel is enhanced to inhibit castration-resistant PC growth, as previously reported (mCRPC). The combination of genistein and cabazitaxel in the treatment of mCRPC xenograft tumors was shown to have a substantial effect on the growth of the tumors (217). Genistein’s action on cancer cells is restricted to the blockage of GLUT receptors, preventing glucose absorption, according to previous studies. As a result, administering it alone may not be sufficient to stop the spread of PC cells. It is thus possible to use genistein in conjunction with well-known anticancer medicines to better target cancer cells and achieve a higher therapeutic index. Tian et al., have developed liposomal preparation containing genistein and plumbagin for targeting delivery to PC specific membrane antigen (218). The Genistein Plumbagin liposomes (GPL) with size 80-100nm size, were conjugated with PSMA specific antibodies. The Plumbagin released rapidly from liposomes in comparison to Genistein. Plumbagin showed sensitizer effect on genistein, thus improving the anticancer activity and inhibiting the pristatae cancer. The GPL showed better effect on the LNCaP cell lines in comparison to PC-3 cells, which can be due to high expression of PSMA on LNCaP cell lines. The GPL increased the presence of free radicals and decreased expression of GLUT-1 receptors and Akt3 proteins. These events led to inhibition of proliferation of PC cells.
Unprocessed olive fruit and leaves are high in the natural component oleuropein (OL), which is a significant member of the secoiridoid family. Chemically, it is an elenolic acid/dihydroxyphenylethanolheterosidic ester that hydrolyzes to eleonolic acid and hydroxytyrosol (219), among other beneficial compounds. A variety of pharmacological actions have been found to include cardioprotection (antiarrhythmic), hypotensive (spasmolytic), and anti-inflammatory characteristics in OL. In addition, a human pharmacokinetic investigation found that oral administration of olive phenolic compounds resulted in fast absorption, metabolism, and renal clearance. Oleuropein’s bioavailability and metabolism were also shown to be very variable and depending on formulation factors as well as gender (220). As a result, intravenous injection is often recommended to address the drawbacks of oral delivery. However, no research has been done in animals to illustrate the pharmacokinetic characteristics of OL following intravenous administration. The drug is likely to have little impact on prostate tissue owing to its quick metabolism and elimination. A long-circulating intravenous approach is needed to circumvent the problems encountered by the oral route and to properly target PC cells with an effective dose of OL in PC care. By using a conventional film-hydration approach, the liposomal formulation was produced and extruded to produce nanosized vesicles with a limited range of sizes (221). In the passive targeting of cancer cells, increased permeability and retention (EPR) effect plays a significant role. The fenestrations in cancer cell membranes are 200 nm greater than in normal cell membranes, which typically have fenestrations of 50 nm. It is conceivable that nanocarriers that are between the lengths of 50 and 200 nm will find their way into cancerous tissues. At addition, the weak lymphatic system in the tumor site results in extended nanocarrier retention (222). It has been shown that cancer cells have a negatively charged surface owing to the release of lactic acid by cells with low oxygen levels (223). This liposome exhibits a positive zeta potential because cholesterol was incorporated into the lipid bilayer, which contains a positive charge head group. It was hoped that the liposomes’ positive charge would aid in tumor retention. This low positive potential may, however, lead to less stability due to decreased repulsion between charged particles. A substantial inter-bilayer repulsion was given by the presence of PEG on the liposome surface, which would have prevented aggregation (224). Comparative DSC thermograms of OL and OL-FML revealed a reduced peak of OL in the liposomes, which indicated that OL molecules were mostly contained inside the vesicular core. Liposomes’ polar surface and the presence of hydrophilic PEG strands make it possible for certain OL molecules to be adsorbed on their surface. Within the first hour of the investigation, 27.54 ± 2.995 percent of OL was released from the OL-FML, a burst release effect was detected. After then, there was a steady discharge over the next 24 hours. An aqueous soluble drug’s release pattern was seen in the OL solution. During the first hour of the experiment, the majority of the OL was excreted. The anticancer effectiveness of compounds and drug delivery systems is first determined via basic studies on cell viability. Researchers found that OL-FML has significantly inhibited cell viability in comparison to OL at all of the concentrations tested. The IC50 of OL-FML was much lower than that of OL solution. Cellular surface adsorption of liposomes and subsequent endocytosis are facilitated by the attraction of positively charged liposomes to negatively charged cell surfaces. Apoptosis and growth-promoting signals may be activated in cancer cells by persistent oxidative stress (225). In addition, OL inhibits Akt signalling by downregulating pAkt (226), which in turn leads to the activation of apoptosis in cancer cells. In the SH-SY5Y cell line, OL was tested for in situ TUNEL of nicked DNA and shown to induce apoptosis (227). TUNEL test has demonstrated that both OL and OL-FML induce apoptosis in 22Rv1 cells.
Zhou et al, developed curcumin-metal ion based liposomal formulation (228). The flower shaped confirmation of liposome was reported first time and effect of various metal ions were evaluated on the cancer cell lines. Metal and ligand selection was critical to the success of the cancer treatment medication complexes (229). Endogenous metal ions, which included a variety of trace metals, were not harmful to normal cells and were engaged in several metabolic activities. This study looked at the effects of various metal ions on the activities of drug metal complexes using the cations copper (Cu2+) and zinc (Zn2+). Because of verstality of curcumin, it was selected as a ligand in cancer treatment. Despite the Curcumin potential as an antitumor drug, its low bioavailability makes it less effective (230). Curcumin chemical stability in extreme physical conditions was greatly enhanced by complexation with metal ions (231). In spite of this, the Curcumin metal ions complex’s limited water solubility was a hurdle to its implementation. It was initially hoped that liposomes pre-loaded with metal ions solutions would be used to generate the Curcumin metal ion complexes. Intravenous injections might be used to administer the complexes produced in the liposomes. Curcumin metal ions complex liposomes’ characteristics will be affected by the kind of salt solution used to dissolve the metal ions (Cur-M liposomes). The liposomes seemed to change colour after the liposomes have become Cur-M. For example, liposomes containing copper or zinc exhibited a greater electrical conductivity (EE) than those that did not. Using Ca(Ac)2 liposomes, the Curcumin precipitated and retained its original colour when the trapping agents were Ca(Ac)2. Lipid liposomal formulation increased EE by increasing Chol content, while decreasing size. According to earlier studies, Chol content have presented opposite impact on hydrophobic medicines (232, 233). It was hypothesised that Chol’s interaction with hydrophobic medicines would reduce hydrophobic medications’ retention. As long as liposomes retain their rigid structure, the reactions between Cur and metal ions are made easier by adding high ratio Chol to them. It was noted that the EE of liposomes reduced when the drug to lipid ratio was more than 1:5. PBS (pH 7.4) with or without 10 mM EDTA was used for Cur-M liposomes release profiles. Cur-M liposomes, on the other hand, it has a more gradual release than Cur solution. There was a noticeable difference between Cur-Cu liposomes and Cur-Zn liposomes in terms of their structure. EDTA has no effect on liposomal release of Cur from Cur-Zn complexes. Cu-Cu liposomes’ Cur release may be substantially increased by mixing with EDTA. Cur-Cu complexes were shown to be more durable than Cur-Zn complexes to remain in solution over time. While Zn2+ ions remained in the liposome, Cur dissociated from Cur-Zn complexes. Liposomes containing Cur-Cu complexes progressively released the compounds. It was difficult to estimate the amount of released insoluble Cur-Cu complexes. Because of the trans-chelation reaction of the EDTA, the Cur was released from the Cur-Cu complexes. More than two times as long as the Cur-Zn liposomes, the t1/2 of Cur-Cu liposomes was 11.67 ± 4.45 h. With the Cur-M liposomes and FBS, Cur’s retention was also evidently different. Less Cur-Cu liposomal leakage in the early phase (0–12 h) was associated with a smaller change in Cur-Cu liposomal size. It was used as a trans-chelator in media and biological contexts. Because of its increased stability, Cur-Cu liposomes may function better than Cur-Zn liposomes in the bodily circulation when targeting tumors. Cur-M liposomal carriers combine the advantages of both coordination and encapsulation, and as predicted, they preserve Cur against degradation more effectively than earlier techniques. Due to the presence of serum proteins, liposomal formulations generated by the passive loading method during blood circulation are not able to protect Cur against degradation during blood circulation. As different formulations were taken up, so did the ROS level. This study found that after 2 h, the ROS level was greater in Cur solutions, and at 8 h it was higher in Cur–M liposomes treated groups. ROS production was mildly induced by both Zn2+ and Cu2+ liposomes. Cur-Cu liposomes were a little more effective in generating ROS than Cur-Zn liposomes. Metal ions have been found to interact with GSH, consuming GSH and influencing the ROS/GSH equilibrium, resulting in ROS production (234). In cancer cells with high GSH expression, the release of Cur from Cur-M liposomes would be accelerated, resulting in an increase in ROS production. GSH and other thiols, such as Cur, have been shown to bind covalently to ROS (235). Products of GSH-Cur conjugates that cannot be reversed, and may lead to oxidative stress. Cur’s cytotoxicity against cancer cells was enhanced by the cooperation of Cur and metal ions, which generated ROS. Cur-M liposomes were compared to Cur solutions in a subcutaneous tumor model and a lung metastasis model. For intravenous administration, 20 mg/kg of Cur was dissolved in ethanol and Tween 80. The Cur-Cu liposomes, on the other hand, outperformed other groups in terms of tumor inhibition. Cur-Cu liposomes may have a long-term anticancer impact because of the increased concentration of Cur in the tumor and the prolonged release of Cur to achieve this effect. The liposomes’ toxification-reactive complexes were potent in their ability to inhibit tumor development. Cur-Zn liposomes outperformed Cur solutions in terms of anticancer efficacy and circulating stability, as well as tumor tissue accumulation. Even though the Doxil-treated group have presented higher therapeutic outcomes, the lowest safety of Doxil revealed the downsides of chemotherapy. Though Cu2+ liposomes showed no toxicity to 4T1 cells when tested, they were shown to have a mild inhibitory impact on tumor development, equivalent to that of Cur solutions. As a result of this discrepancy, it was hypothesised that Cu may make a metalloenzyme inactive in metalloproteins, which were thought to be crucial to cancer cell metabolism through Zn replacement (229, 234, 236). In the meanwhile, Cur-Cu liposomes may have a greater impact on cancer treatment than Cur-Zn liposomes because of this.
Shikonin (SHK)was encapsulated as liposome moiety, to induce immunogenic cell death, at high dose (237). But loading resulted in hepatotoxic effect, so inorder to circumvent this issue, anthracycline mitoxantrone and doxorubicin were co-loaded to liposomes, for inducing the synegestic effect on tumor cells. A metal ion gradient was selected as the inner phase to stabilise SHK because it possesses the functional group necessary to form complexes with divalent metal ions (as shown in Figure 4). Cu2+ and Zn+ were found to be the only metal ions that could be successfully encapsulated in the first step of the experiment. Liposomes containing SHK-Zn were shown to be unstable because, after a few hours, a purple sediment of SHK-Zn developed. High-transition-temperature (HTT)-satiated phospholipid HSPC was chosen to increase the stiffness and stability of the lipid bilayer (237). Chol increases liposome size and decreases loading efficiency because hydrophobic drugs interact with Chol and get caught in the lipid membrane readily. According to earlier studies, reducing Chol concentration improves the retention of hydrophobic drugs (232). SHK was leaked when 10% Chol was being used. In order to avoid the other formulations’ instability, neutral Cu-gluconate was chosen to operate as the inner phase because SHK was successfully protonated and interacted with copper more closely in the neutral state than it did in the acidic condition (232). It was also shown that the greater the copper ion concentration, the more medication was loaded into the liposome and the more stable it was. To avoid copper toxicity, a threshold value of 200 mM was established for future study. Low levels of DSPE-PEG2000 (0.5 percent, molar ratio) were utilised to avoid ABC (accelerated blood clearance) and increase stability (238). It is necessary to utilise an organic solvent in order to enable SHK penetrate the bilayer; DMSO was employed at 5% and the drug/lipid ratio was 0.125. Its structural isomer alkannin was also employed to assess its encapsulation into liposomes throughout the formulation’s optimization process, yielding an unexpected result given that alkannin could not be loaded into liposomes despite the tiny structural difference.
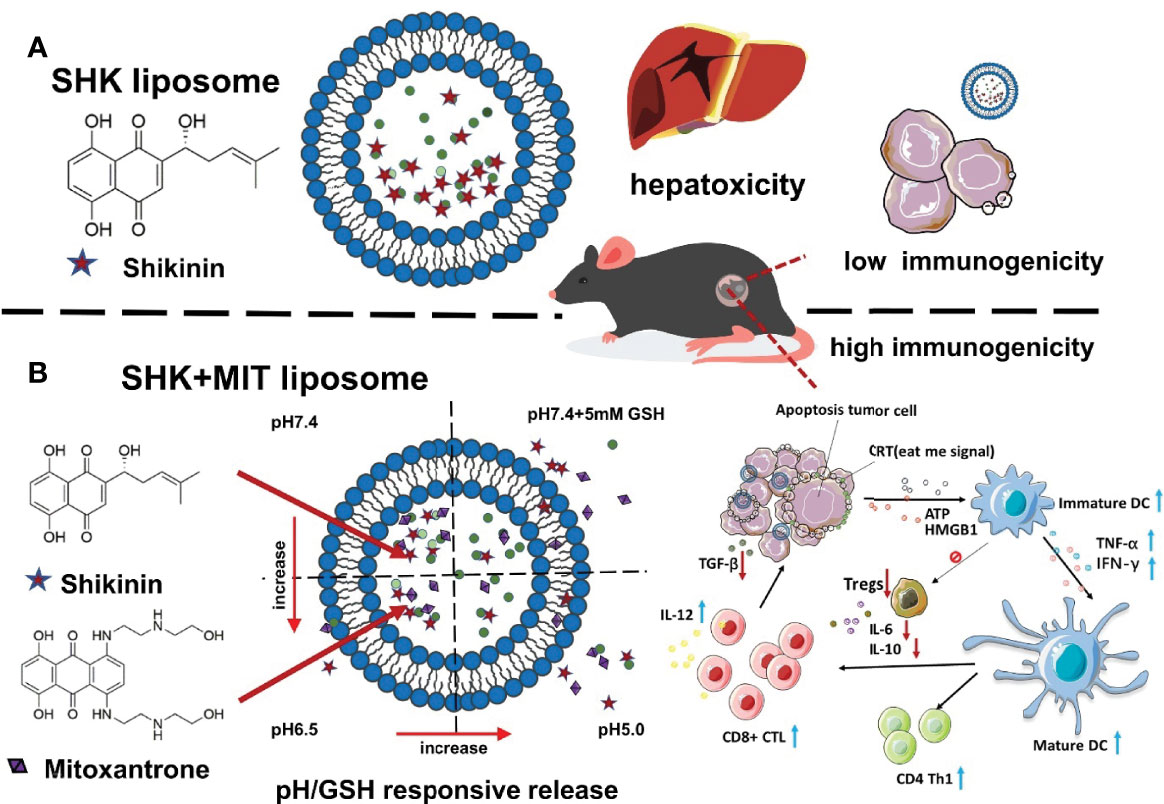
Figure 4 SHK and MIT co-loaded liposomes with dual-responsive release for synergistic chemo-immunotherapy through ICD. (A) Result of limited immunogenicity, SHK liposomes may cause hepatotoxicity. (B) Inducing strong apoptosis in cancer cells and stimulating an ICD effect and adaptive immune response using SHK and MIT co-loaded liposomes might shrink tumor volume and produce strong apoptosis in cancer cells.
4.2.2 Nanoparticles
The increased permeability and retention (EPR) effect allows nanoparticles to deliver medications to tumors more effectively (239). A polymer core and a lipid shell form the core of lipid-polymer hybrid nanoparticles (LPNs) (240). Hydrophilic and hydrophobic pharmaceuticals may be encapsulated by the polymer core, which is covered by a lipid shell that acts as a barrier to prevent fast drug leakage and extend the release time (241). A recent study suggests that LPNs are an important component of combined PC treatment (242). Aptamer-functionalized LPNs have received the most attention from researchers in the development of ligands-functionalized LPNs for the treatment of cancer (243, 244). CUR and cabazitaxel (CTX) were conjugated ligands that were used to construct a curcumin and aptamer-functionalized hybrid lipid polymer nanoparticle (APT-CUR/CTX-LPN) (29). Each of the APT-CUR/CTX-LPNs measured on average at 121.3 nm in diameter and have noticed an electrically positive surface charge of 23.5%. PEG-PLGA or APT-PEG-PLGA nanoparticles, did not slow down drug release as much as LPNs, perhaps because of the presence of lipids. For sustained circulation, PEG is by far the most essential moiety because of its low immunogenicity and toxicity, as well as its great flexibility and little impact on the biological characteristics of medications that have been decorated (245). Because the pharmaceuticals released from APT-CUR/CTX-LPNs were slower than those released from CUR/CTX-LPNs, the aptamer on the surface may serve as a molecular barrier to keep the medications inside NPs (246). The cytotoxicity of LPNs was investigated in PSMA-positive LNCaP cells and PSMA-negative PC3 cells, both of which expressed PSMA. This may be due to the low cytotoxicity of blank APT-LPNs, which were composed mostly of biocompatible aptamer, SPC, PEG, and PLGA components (247). As a result, the cytotoxicity of the systems is caused by the drugs loaded into the LPNs. Evidence that LPN delivery methods may improve drug toxicity was found by comparing the cytotoxicity of medicines put into LPNs with that of drug solutions (248). PSMA positive LNCaP cells were more sensitive to APT-CUR/CTX-LPNs than PSMA negative PC3 cells, which suggests that APT-CUR/CTX-LPNs have the capacity to target PSMA positive cancer cells (249). In this work, the Chou-Talalay approach was used to evaluate the synergistic effects of the combination medication delivery system. CICUR+CTX values were lowest when the CUR/CTX ratio was 2:5, supporting the synergistic effect and pointing to the ratio in the APT-CUR/CTX-LPNs formulation. CUR has been shown to interact with a number of proteins that are involved in angiogenesis, metastasis, and cell survival, as well as disrupting dysregulated signaling pathways in cancer cells, such as PI3K/Akt and NF-kB. To increase CTX effectiveness in PC-3 cells, CUR increases the activity of many key enzymes including COX-2, NF-B, phospho-Akt, PI3K, and RTK (250, 251). More APT-CUR/CTX LPN dispersion was seen in tumor tissue than in CUR/CTX LPN or medication solutions. Gu et al. observed that aptamer-conjugated nanoparticles accumulated more in the tumor than unconjugated nanoparticles. Modifications to aptamers may explain this phenomena by delivering medicines to PC cells and causing tumor inhibition effects (252). CUR/CTX-LPNs have a significant antitumor activity compared to medicines. That the lipid outer layer has a strong affinity for the cell membrane and has merged with the cells and allowed medications to enter the cells (253) might be evidence of this. Experiments using APT-LPNs and 0.9 percent water demonstrated anorexia and anxiety in the mice, which might be the cause of their weight loss. Drug-loaded LPNs showed no noticeable changes in body weight, ALT, LDH, or BUN, indicating that severe adverse effects of anticancer treatment, have been adequately relieved while considerable co-therapeutic benefits had been preserved (254). Thipe et al., designed resveratrol-conjugated gold nanoparticles (Res-AuNPs) as an innovative green nanotechnological approach to enhance the efficiency of bioactive phytochemical substance in cancer treatment (255). The study was planned to utilize the pro-apoptotic properties of gold nanoparticles (AuNPs) with synergistic antitumor properties of resveratrol as a green nanotechnological approach in cancer therapy. Res-AuNPs was coated with gum Arabic for stability of AuNPs. The particle size of Res-AuNPs and Res-GA-AuNPs was found to be 16.1 ± 5.0 and 14.9 ± 4.4 nm with negative zeta potential of -25 and -22 mV. The in vitro anticancer effect of resveratrol conjugated gold nanoparticle was conducted on human breast, pancreatic, and PC cell lines (MDAMB-231, PANC-1, PC-3). The results of their study on cancer cell lines provide evidence, which signify that increased corona of resveratrol on AuNPs improved the bioavailability of therapeutic active moiety in cancer cells.
Co-delivery systems for curcumin (CUR) and bioenhancement (B), “trikatu,” have been developed by Sharma et al. to treat hormone resistant PC (255). Spices such as black pepper (Piper nigrum Linn.) and long pepper (Piper longum Lind.) containing the active ingredient piperine (Zingiber officinale Rosc.) are known as “trikatu” in Japanese. Natural bio-enhancers combined with established anticancer medications in a delivery system may lead to higher bioefficacy and bioavailability, as well as expanded surface area, rescue of bioenhancers from degradation, and specificity in the treatment of tumors. Peeking into the drug transport pathway in PC3 cells using the neutral red test reveals an 18.4 percent increase in CUR influx from the inside of the cells. Bioenhancers like piperine, which inhibits the PGP, and gingerol, which affects cell membrane permeability and changes membrane dynamics are notable for this property (256, 257). To validate drug distribution, FITC-loaded nanoformulations were stained with DAPI for nucleus staining, and the remarkable impact was attributable to CH as a nanocarrier with pluronic F68, however CH conjugation with FA made the effect much more evident. Curcumin’s cellular internalisation is inhibited when given in conjunction with piperine in a nano-delivery method, providing further evidence that polyphenolic components like piperine and gingerol-6 modulate the transport mechanism. Additionally, it increases the CUR oral bioavailability (257, 258). Folate-conjugated nano-delivery systems may be increased since folic acid receptors are expressed on intestinal epithelial cells. FA enters cells by caveolae-mediated endocytosis and decreased folate carrier separate routes via facilitated transport, although the two pathways are not mutually exclusive (259). The synergistic impact of the polyphenolic bioenhancer in the formulation significantly reduced the IC50 of the CUR-B-CH-NPs in a cytotoxicity investigation using the PC cell line PC3. Unlike other cancer cell lines, the AR-negative PC3 cell line possesses very aggressive cells (259). To further understand the role of CUR-B-CH-NPs in hormone-independent PC, researchers extended studies on PC3 cells. Although CUR has been shown to be effective against AR arbitrated malignancies, it has some issues with cell absorption and bioavailability even in formulations designed for oral delivery (260). Apoptosis studies on PC3 cells showed that CUR-B-CH-NPs increased the percentage of apoptotic cells by 2.3 times higher as compared to CUR alone. It was discovered that the polyphenols in CUR-B-CH-NPs have an important role in apoptosis, since a complex mix of bioenhancers may activate distinct apoptosis-related pathways. The JC-1 dye based assay revealed a change in mitochondrial membrane potential, which is a key component of apoptosis. MMP levels were lowered by 2.7-fold, suggesting that MMP disruption is an inherent apoptotic mechanism. Polyphenols, which have a function in promoting oxidative stress in cancer cells, were shown to disrupt MMPs. As compared to the positive control H2O2, the DCFDA staining of cells showed almost 1.68 fold increased ROS levels in PC3 cells in the presence of CUR-B-CH-NPs. Bioenhancers P, N, and Z, on the other hand, has a negligible cytotoxic impact on PC-3 cells. Weak phytochemicals in combination have been shown in previous investigations to produce an outstanding synergistic cocktail (260). According to in-vivo results, CUR-B-CH-NPs displayed a markedly enhanced bioavailability compared to CUR-bioenhancement combo CZP/CUR solution, which is well expected. Relative to CZP and CUR solution, CUR-B-CH-NPs have significantly higher AUC by 4 and 6 times, as well as Cmax by 1.9 and 7.7 times, respectively. This may be due to the chitosan protecting CZP, which protects the drug from acidic degradation and also facilitates controlled release with the help of surfactant pluronic F68, according to the detailed statistical data. By enhancing intestinal membrane permeability and CZP cellular internalisation in CH-NPs1, Pluronic F68 improves CUR solubility.
Yallupu et al., developed curcumin-PLGA based nanoparticles for PCa (261). The internalisation of PLGA-CUR NPs was time-dependent. There was a significant amount of NPs in membrane vesicles during the first hour, and by the end of the second hour, NPs have completely entered the cells. NPs were found around the nucleus at the 18-hour mark. Based on the substantial internalisation and distribution pattern of NPs, the endocytosis/phagocytosis mechanism is likely clathrin-mediated. After being endocytosed, nanoformulations may be released from the endosome and end up in the nucleus or cytoplasm. Flow cytometry demonstrated the absorption of PLGA-CUR NPs at the 18-hour time point, with obvious cellular localisation. Variable PC cells have different levels of PLGA-CUR NP endocytosis. In the three studied PC cell lines, there may be variances in the method of internalisation of PLGA-CUR NPs because of the existence of different lipid membranes on their surfaces.Long-term accumulation and retention, in addition to internalisation, are critical for improving the therapeutic effectiveness of cancer medicines. Rapid breakdown or cellular export of many cancer medicines prevents therapeutic levels from reaching cells. PLGA-CUR NPs showed increased accumulation and retention compared to free CUR at each time point. All three cell lines examined retained a different amount of DNA, which was in keeping with the results from the TEM. The concentration of DU-145 cells peaked on day 2 and decreased dramatically on day 4. Day 1 to 2 saw an increase in C4-2 cells, which remained essentially constant at day 4, but day 3 to 4 saw a decrease. Free curcumin and PLGA-CUR NPs were substantially less stable in PC-3 than in PC-1, with a peak accumulation on day 2. Varied cancer cell types may respond to PLGA-CUR NPs differently because of their different cellular levels. Both C4-2 and DU-145 cancer cells produced a significant number of vacuoles under TEM. Lysosomal activity was also found to be abnormally high in this group of cells, which may have resulted from membrane instability that causes cell death/apoptosis signals. All of the vacuoles were shown to leak bioactive CUR from the nucleus’s periphery when NPs are internalised (262). By causing vacuoles in the cell body, the presence of PLGA-CUR NPs in PC cells led in cell membrane protrusion and disruption of the cytoskeleton (263). PC cells were not affected by the vacuolization induced by free CUR (264). Smaller and fewer cysts in PC-3 cells imply less absorption of nanoparticles than larger and larger vacuoles.
Cell death in PC cells was enhanced by PLGA-CUR nanoparticles, which promoted PARP cleavage and reduced the expression of anti-apoptotic proteins such Bcl-xL and Mcl-1 (265). Cleaved PARP plays a vital role in the activation of Caspase-3/7, which leads to apoptosis (265). When Mcl-1 and Bcl-xL are downregulated, platelet-derived growth factors (PDGF) and β-catenin transcription factors (TCF) are suppressed. On the other hand, the expression of AR and beta-catenin in cells was shown to be inhibited by the PLGA-CUR NPs. β-catenin is a multifunctional protein that plays a critical role in both ontogenesis and oncogenesis. Increased AR activation has been linked to β-catenin dysregulation (266) and the development of various malignancies, including PC. The upregulation of PKD1 by PLGA-CUR which has been shown to suppress the production of nuclear β-catenin and AR (267, 268).
In 2017, Azandeh et al. published a complementary research on PC-3 PC cells treated with Cur-PLGA NPs (269). When curcumin was applied to PC-3 cells, cell viability and proliferation decreased, with a higher loss in cell viability for Cur-PLGA NP-treated cells than for Cur-treated cells. PC-3 cell growth was dramatically decreased by curcumin-PLGA NPs, but PNT2 (healthy) cells were unaffected by treatment with curcumin. It was determined that Cur-PLGANPs have affected the chromatin structure of PC-3 cells, but PNT2 cells did not respond to treatment with the Cur-PLGA NPs. Annexin V/PI staining also showed that cells treated with Cur-PLGA NP having greater apoptosis and necrosis index. This therapy is promising for future investigations against PC since it induced cell death via both types I (apoptosis) and type II (autophagy/necrosis) of programmed cell death (269).
Anitha et al., formulated water soluble O-carboxymethyl chitosan based curcumin nanoparticles(O-CMC Nps) (270). Curcumin encapsulation and loading efficiency in O-CMC Nps were determined to be 87% and 48%, respectively. Results showed that the drug loading was greatly affected by the concentrations of O-CMC and curcumin. The higher the O-CMC concentration, the larger the particles were. Where the drug tends to precipitate, trapping efficiency decreased with greater drug concentrations. Curcumin and curcumin-O-CMC Nps in the dose range of 1–5 mg/ml decreased the viability of L929 but not curcumin alone when exposed to O-CMC Nps. Curcumin, O-CMC Nps, and curcumin-O-CMC Nps were not hazardous to normal cells, as shown by the fact that 80% of the cells were alive. MCF-7 exhibited no toxicity for O-CMC Nps whereas curcumin and curcumin-O CMC Nps showed significant toxicity. A similar toxicity was seen in PC-3 wherein cell viability was decreased to 30% at 5 mg/ml, confirming its anticancer properties. Curcumin-O-CMC Nps and curcumin has a similar impact on cancer cells, indicating that curcumin maintains its anticancer action even after being placed into a polymer matrix. Increases in curcumin-O-CMC Nps concentrations boost the absorption in both cell lines, according to the uptake profile.The negative charge of curcumin-O-CMC Nps may explain the lack of variation in particle absorption between normal and malignant cell lines. Cellular absorption is non-specific and concentration-dependent, according to this study’s findings. O-CMC Nps did not cause apoptosis in both cancerous and non-cancerous cells exposed to it. Compared to L929, MCF-7 has a higher proportion of apoptotic cells, which is obvious. The greater dose of curcumin-O-CMC Nps (5 mg/ml) resulted in a larger percentage of cells showing apoptosis than the lower quantity (1 mg/ml). Curcumin-O-CMC Nps may have been more hazardous to cancer cells at greater concentrations because of the increased absorption of curcumin-O-CMC Nps. In spite of the fact that normal and cancer cells have a similar absorption rate of particles, the greater apoptosis in cancer cells suggests the release of curcumin inside cancer cells and demonstrates the drug’s particular anticancer effect.” One explanation for this is curcumin’s ability to target signalling molecules found in high concentrations in cancer cells. Curcumin stops cell division in G0 phase without causing apoptosis in normal cells since these mechanisms are controlled.
Vodnik et al., developed Genistein (Gen) stabilized gold nanoparticles for targeting PCa (271). TEM was able to characterise the shape, size, and distribution of well-dispersed AuNPs when Gen was used as a reducing and capping agent in the same molecule. Capped Gen-coated AuNPs are round, and their size distribution is restricted. For Gen@AuNPs1 and Gen@AuNPs2, the average particle diameter (dav) was determined to be 10 nm and 23 nm, respectively. As a consequence of the greater Gen and Au3+ concentrations added, the second conjugate grew in diameter. In cell culture, treatment with Gen resulted in a dose-dependent reduction in cell numbers, as measured by mitochondrial respiration. It was found that the half-life values determined for PC3 cells and DU 145 cells were quite close (21.0 +/-0.6 and 22.3 +/-1.3), but LNCaP cells were more susceptible (13.9 +/-0.8). These two cell lines, DU 145 and PC3, are more aggressive than LNCaP, which explains their lower response. Considering that less than 50% of Gen was loaded onto each AuNP, it is clear that binding to AuNPs increased Gen’s cytotoxicity. Gene concentrations at 9 and 14 g/mL were measured in LNCaP cells using Gen@AuNPs1 (46 percent Gen loaded onto AuNPs) and Gen@AuNPs2 (48 percent loaded Gen), respectively, according to the proportion of loaded Gen. Even while Gen’s anticancer potential isn’t much boosted, the improved stability and distribution of Gen in this formulation may be critical for its continuing use in vivo. Both early and late apoptosis were not seen when Gen was incubated with free Gen or Gen@AuNPs1 formulations. Autophagy was likewise unaffected by both treatments, demonstrating that experimental therapies do not rely on programmed cell death type II as a cytotoxic mechanism (272). There was a considerable reduction in viability, however, since cell multiplication was considerably suppressed. By affecting the production of the human telomerase reverse transcriptase and different microRNAs, genistein has been shown to decrease PC cell growth (273, 274).
As a result, these anticancer nano-formulations are capable of increasing medication release and activation inside tumors, enhancing the therapeutic efficiency of the treatments. Tumor microenvironment features including hypoxia, acidity, the EPR effect, the presence of proteolytic enzymes, and the overexpression of certain cell membrane antigens or proteolytic factors may all cause disease-specific activation in solid tumors. As a result of the presence of these tumor-specific endogenous properties, it is possible to create formulations that are more active in the tumor microenvironment. When it comes to medication delivery strategies for cancer, polyglutamate (PGA) is one of the biodegradable polymers that have proved effective (275). However, PGA may be digested by cathepsin-B, which is released into the tumor microenvironment of most solid tumors and is lysosomal protease cathepsin-B. Results show that cathepsin B-induced digestion of nanoparticles into smaller particles might result in better dispersion of the sensitizer in dense tumor formations, as shown by this study (276). Because of the high interstitial fluid pressure and the thick network of collagen fibres, nanoparticle transport is impeded in dense tumor masses (277). Nanoparticles may be digested into smaller particles by the enhanced pericellular cathepsin B released by malignant tumors, according to the findings of this study. To reduce perivascular sequestration and trapping, this may help increase particle diffusion across the dense tumor mass during extravasation. lysosomes are predicted to be the site where cathepsin B digests nanoparticles completely and releases hematoporphyrin. The sonochemical effects of ultrasonic irradiation may stimulate the inclusion of free hematoporphyrin or amphiphilic hematoporphyrin complexes in the lysosomal membrane, the sensitization of the latter and the subsequent lysosome collapse (278). Previously, it has been demonstrated that lysosomal collapse may cause to apoptosis via lowering cytoplasmic pH. As a result, the treatment modality presented in this work is speculated to have a plausible mechanism of action, and the effect is supported by the nanoparticulate formulation’s responsiveness to cathepsin B. Although hydrogen peroxide is not a typical harmful ROS produced during sonodynamic activation utilising the nanoparticulate formulation, the ROS-induced DPBF breakdown and subsequent drop in absorbance were both shown to occur over the course of five minutes. Additionally, ROS generation was compared when hematoporphyrin was free and when no sensitising agent was present, namely just when ultrasonic irradiation was used. Nanoformulations show equivalent efficacy to free hematoporphyrin (p > 0.05) in terms of ROS generation, which is suggestive of an effective SDT-induced antitumor impact. As a result of this research, a nanoparticulate formulation has been created that specifically targets the acidic tumor interstium and cathepsin B. Cathepsin B is a proteolytic enzyme common in malignant tumor microenvironments, and cancer cells modulate its production and release extensively based on interstitial pH. This enzyme’s intracellular and secreted levels in LNCaP cells were examined as a critical first step in our attempt to use cathepsin B to enhance our therapeutic platform’s performance in SDT. The incubations were carried out under hypoxic circumstances, at 2 mmHg O2, since hypoxia is known to activate cathepsin B production in tumors (279). As compared to pH 7.4, levels of cathepsin B in LNCaP cells at pH 6.4 were 35 percent higher and 61 percent higher than those at pH 7.4. Finally, cellular absorption of 5-g/mL nanoparticles was measured for both pH conditions at final concentrations of 5 g/mL. For pH 6.4, HPNP absorption by LNCaP cells was enhanced by 75%, and this corresponds to an increase in cathepsin B. Although there isn’t conclusive proof of a link between cathepsin B levels and cellular absorption of PGATyr-based nanoparticles, it does show that the proteolytic enzyme’s levels influence cellular uptake. These findings may in part be owing to an enhanced protonation under acidic conditions of the glutamate residue side chains, which would contribute to an improvement in cell internalisation. HP and PGATyr co-polymer have been used to generate a nanoparticle formulation. To find out how well the nanoparticles performed when exposed to pH and cathepsin B, researchers examined the nanoparticles’ reactivity to each. According to the research findings, cathepsin B digestion reduced the size and overall negative charge of the formulation’s nanoparticles, perhaps allowing for better nanoparticle diffusion into impenetrable tumor tissues after extrusion. For LNCaP and PC3, the “silent” toxicity profiles were different, although the acidic pH increased the nanoparticle toxicity for both cell lines. Cellular absorption seemed to be inversely related to the production and secretion of cathepsin B. For PC cells treated with HPNP and treated in vitro with sonodynamic therapy, the stimulus (ultrasound) and formulation has little or no impact when not combined. Compared to the free sensitizer, the nanoparticulate formulation considerably increased HP’s sonodynamic activity in cell-based systems, principally due to better cellular absorption of the HP nanoparticles. SDT therapy in immunodeficient mice resulted in a 36% decrease in LNCaP tumor sizes after 24 hours of administration of a single dose of nanoparticles. There were no detrimental effects on the nanoparticle-treated animals, and their weight remained steady.
4.3 Micellaenous
Since quercetin has a low bioavailability in castration-resistant PC, Zhao et al. used nano micelles to encapsulate it for in vitro and in vivo research (280). Quercetin’s water solubility was increased 450-fold by encapsulating at 1 mg/mL. According to the results of the in vitro investigations, micellar quercetin formulation has a half maximal inhibitory concentration of 20 M, whereas free quercetin has noticed a concentration of 200 M. As a result, the nano based formulation effectively triggered apoptosis and suppressed cell growth in human androgen prostate cancer cell lines. In addition, quercetin-loaded micelles in vivo showed greater anticancer activity, and the proliferation rate dropped by 52% compared to the control group in the PC-3 xenograft mouse model, possibly owing to higher permeability and retention of micellar quercetin at the tumor site.
Cancer relapse, chemoresistance, and recurrence are all made more likely by cellular senescence, a persistent problem in cancer treatment. Drug-induced senescence is a common side effect of long-term chemotherapy treatment. It is well-known that Docetaxel, a prostate cancer medication authorised by the FDA, may cause cellular senescence, reducing the overall survival time of patients. Anti-aging strategies for cells and drugs are still unmet therapeutic needs. With the goal of developing an innovative therapy that targets and destroys senescent cells, researchers created a nanoformulation of tannic acid–docetaxel self-assemblies in an attempt to achieve this goal (DSAs) (281). Particle size, spectroscopic, thermal, and biocompatibility investigations verified the creation of DSAs. Docetaxel was shown to be more effective in this formulation when compared to docetaxel alone in a variety of biological functional tests. Senescence-associated TGFR1/FOXO1/p21 signalling was altered by DSAs exposure, according to microarray and immunoblot research findings. After DSAs exposure, a decrease in -galactosidase staining indicated a reversal of drug-induced senescence. In addition, DSAs triggered apoptosis by circumventing senescence, resulting in a dramatic increase in cell death. In addition, imaging studies in mice with PC-3 xenograft tumors showed that DSAs target tumors both in vivo and ex vivo. Using the PC-3 xenograft mouse model, the antisenescence and anticancer effect of DSAs was shown by suppressing TGFR1 proteins and regressing tumor development via apoptosis. These enhanced properties of DSAs were all attributed to the use a natural substance as the matrix/binder for docetaxel in the formulation. Docetaxel effectiveness was enhanced by DSAs’ greater tumor targeting and increased cell internalisation. Prostate cancer treatment may benefit greatly from these discoveries.
Radhakrishnan et al. developed epigallocatechin-3-gallate (EGCG) loaded solid lipid nanoparticles (SLN) by double emulsification method to enhance the stability and anticancer efficacy of loaded phytoconstituents (282). The in vitro cytotoxicity was performed by MTT assay using breast cancer, and prostate cancer cell line (MDA MB-231, and DU-145 respectively), where EGCG-SLN showed increase in cytotoxicity against (8.1 fold) MDA MB-231 and (3.8-fold) DU-145 cell lines. In a colloidal stability study, stability with high resistance to electrolyte synthesis was observed in both serum and P135. They concluded that EGCG-SLN acts as a promising nanocarrier for delivering epigallocatechin-3-gallate as a powerful anticancer agent.
Khan et al. suggested a different approach involving the nanoencapsulation of epigallocatechin-3-gallate (EGCG) by oral administration to treat prostate cancer (283). Chit-nano EGCG performed kinetic experiments, in which the release of EGCG has minimal effects of mimicking gut juice simultaneously while EGCG was able to be released rapidly. The effectiveness of the antitumor Chit-nano EGCG was tested by implanting 22Rv1 xenografts under the skin in naked Athymic mice. By comparing the tumor tissue of mice treated with chit-nano EGCG to the EGCG control groups, significant effects were observed as cracking of ADP-ribose polymerase induction, increased Bax protein exposure and a corresponding decrease in Bcl-2 expression. Activation of caspases, reduction of Ki-67 and proliferation of cell nuclear antigen. Through this study, they came to the conclusion that EGCG acts as a preventive and curative agent for prostate cancer.
Blanco et al., prepared β-Lapachone (β-lap) loaded PEG-PLA polymeric micelles to treat quinone oxidoreductase 1 (NQO1) overexpressing tumors (284). β-Lapachone is a chemotherapeutic agent, biologically activated by NADP(H): quinone oxidoreductase 1 coenzyme which is overexpressed in most of the tumor cells. To increase the loading efficiency of micelles dialysis, solvent evaporation and sonication method are used in which film sonication method yielded maximum loading density (4.7 ± 1.0% to 6.5 ± 1.0) with optimum size of 29.6 ± 1.5 nm. They conducted a drug release kinetic study of polymeric micelles, which shows 50% drug release within 18 h of time period. The in vitro cytotoxicity study was conducted on lung, prostate, and breast cancer cell lines (NQO1-overexpressing (NQO1 +) and NQO1-null H596, DU-145, and MDA-MB-231 cell lines). The results of cytotoxicity showed increase in toxicity on NQO1+ cells over NQO1- cells anticipating β-Lapachone micelles as a promising nanocarrier against NQO1-overexpressing tumor cells.
Mukerjee et al., prepared PLGA nanosphere by solvent evaporation technique to check the potent anticancer effect of curcumin (262). The study’s primary objective was to mask the demerits of curcumin associated with low oral bioavailability and poor aqueous solubility. The particle size of the nanosphere was within the range of 35 to 100 nm, with the mean size of 45 nm. PLGA nanosphere showed encapsulation efficiency of 90.88 ± 0.14%. The cellular viability of curcumin PLGA nanosphere was evaluated on prostate cancer cell line where it showed more pronounced effect when compared with free curcumin. These results of the PLGA nanosphere were more promising, and as adjuvant therapy, it can be used to treat prostate cancer.
5 Nanotoxicity of Nanomaterials
Currently, nanomaterials are pervasive in everyday life (285). This toxicity and the destiny of nanomaterials depends on their physical and chemical characteristics, which are determined by their usage in everyday life. The physiochemical features of nanomaterials, such as charge, surface area, shape, size, and aggregation, are unique to this kind of material. Nanomaterials are more reactive than bulk materials because of their tiny size, and their ability to enter cells and cause toxicity is also higher. Smaller nanomaterials are more harmful than larger ones because they are easier to get into the body’s organs. Reactive oxygen species (ROS) are formed when nanomaterials are retained in organs (286).
Toxicities associated with nanomaterials may be classified according to their described morphologies, which include rods, cubes, ellipsoids, spheres, and cylinders (287). Surface chemistry characteristics as roughness, charge, and hydrophobicity may have a substantial impact on the toxicological consequences of nanomaterials. It is possible that nanoparticles’ surface features impact the blood-brain barrier and the immune system, as well as the phagocytosis, colloidal behavior, and cellular absorption of nanomaterials. Compared to negatively charged nanoparticles, positively charged nanomaterials have a far greater absorption rate Once nanomaterials permeate membranes and bind tightly to DNA because it is negatively charged, the G0/G1 stages of cell life cycles are prolonged. The positively charged nanomaterials have a stronger affinity for proteins and may modify protein structure, which may lead to the suppression of enzyme activities and the subsequent disruption of biological processes (288).
Materials having cationic surface charges are more likely than neutral or anionic nanoparticles to interact with genetic materials and biological membranes, resulting in greater toxicity (289). Agglomeration and aggregation of nanomaterials, as well as surface charge and size, may change the blood-brain barrier’s integrity (290). Nanomaterials may be made of inorganic or organic components, and a large number of reagents are needed to make them. As a consequence, unanticipated toxicity and side effects may occur owing to the presence of contaminants or other undesired components. The body’s nanomaterial composition may alter due to internal pH and oxidation reduction reaction variations (291).
For nanotoxicity, the solubility of nanomaterials is a key issue. The dissolution of nanomaterials is influenced significantly by temperature and pH fluctuations. Unlike insoluble nanoparticles, soluble nanomaterials may be very hazardous. Even though nanoparticles have diverse physiochemical characteristics, agglomeration may produce considerable toxicity and increasing exposure levels to nanomaterials might induce chronic illnesses including cancer and fibrosis. Nanotoxicology relies heavily on nanoparticles’ morphology. According to Firme III and Bandaru (292),, long-term inhalation of nanofibers or nanomaterials may lead to lung inflammation and cancer. It’s been shown via several research that carbon nanotubes are much more hazardous to health than either silica dust or ultrafine carbon black (293).
When nanomaterials are dispersed and agglomerated, their toxicity is directly affected by the circumstances in which they are dispersed and agglomerated. Additionally, the hazardous effects of nano-materials are influenced by the media in which they are dispersed. Using the same nanomaterials in a variety of different environments results in various harmful effects (294). Some of the dispersion agents may increase the physical and chemical characteristics of nanomaterials in the medium, while others can have detrimental impacts on the environment, resulting in hazardous consequences.
In terms of toxicity, nanoparticles are mostly determined by their surface characteristics. The physicochemical features of nanomaterials, such as their chemical reactivity and their optical, magnetic, and electrical properties, may be modified by surface coating (295).
For nanoparticles, researchers have developed a risk assessment framework that incorporates alternative testing methods for individual nanomaterials. However, animal model testing is significantly reliant on most testing methods for assessing the toxicity of nanomaterials (296). Due to the fact that nanomaterials interact with the human body in a unique way, further study is needed to establish a long-term and effective solution.
6 Conclusion and Future Directions
Toxicities, poor bioavailability, limited selectivity, and multidrug resistance have all been addressed using nanotechnology in cancer therapy. Chemotherapy’s non-specificity has long destroyed normal growing tissues in patients, causing immunodeficiency and long-term adverse effects. Nanotechnology has offered powerful treatment techniques due to its selectivity to target malignant cells. Patients treated with nanoparticles had improved therapy response and long-term survival. Nanomedicine in cancer treatment may therefore easily solve the obstacles hampering traditional therapy regimens. Developing patient-specific medication delivery systems would assist personalise and manage therapy depending on the patient’s clinical profile. Nanomedicine promises to be the next worldwide transformation in cancer therapies, allowing for early tumor detection and patient management.
To prevent tumor formation or reduce cancer incidence, chemopreventive medicines are much sought after. Because the current therapeutic options include chemotherapy, radiation, and surgery, which all have considerable adverse effects, alternative or adjuvant treatments are urgently needed. Phytochemicals are harmless and plentiful in foods. So, alternative medicine attempts to use these non-essential nutrients to prevent and cure cancer. Many studies support the use of biomolecules in cancer therapy, however most are in vitro. Despite few in vivo and clinical trials, phytochemicals offer considerable potential in cancer therapy. Efficacy of these compounds in clinical studies must be approached with caution as numerous variables influence their biological effects. This is particularly true when low nontoxic dosages are necessary for lengthy durations to achieve significant chemotherapeutic results with minimum adverse effects. Dosage and delivery are now important issues. To maintain a consistent physiological serum dosage availability, the agent must be concentrated and stable in the target tissue. Combination technology may solve this issue. Nanotechnology is rapidly becoming the next level of scientific technology. In vitro research have showed that encapsulating dietary supplements in nanoparticles increases their delivery, stability, and availability. Maybe studies should look at employing combo therapies. Given the findings in this analysis, it will be fascinating to gather further pre-clinical evidence on these compounds’ anticancer properties. Although little is known regarding natural chemical bioavailability in vivo. However, further high-quality research are required to conclusively confirm plant extracts’ therapeutic usefulness, solely and as synergisticaly.
Author Contributions
Conceptualization, HC and TE; data curation, HC, SB, and RaG; writing —original draft preparation, HC, RaG, RuG, RT, TU, MM, MS, and MH; validation, KK, GG, IS, JK, JJ, MA-D, FA, TE, and BK; formal analysis, KK, GG, IS, JK, JJ, MA-D, FA, TE, and BK; investigation, HC, RaG, RuG, RT, TU, MM, MS, and MH; resources, HC and TE; writing—reviewing and editing, KK, GG, IS, JK, JJ, TE, and BK;; visualization, KK, GG, IS, JK, JJ, MA-D, FA, TE, and BK; supervision, SB, JJ, TE, and BK; project administration, SB, JJ, TE, and BK; funding acquisition, TE and BK;. All authors have read and agreed to the published version of the manuscript.
Funding
This research was supported by Basic Science Research Program through the National Research Foundation of Korea (NRF) funded by the Ministry of Education (NRF-2020R1I1A2066868), the National Research Foundation of Korea (NRF) grant funded by the Korea government (MSIT) (No. 2020R1A5A2019413), a grant of the Korea Health Technology R&D Project through the Korea Health Industry Development Institute (KHIDI), funded by the Ministry of Health & Welfare, Republic of Korea (grant number: HF20C0116), and a grant of the Korea Health Technology R&D Project through the Korea Health Industry Development Institute (KHIDI), funded by the Ministry of Health & Welfare, Republic of Korea (grant number: HF20C0038).
Conflict of Interest
The authors declare that the research was conducted in the absence of any commercial or financial relationships that could be construed as a potential conflict of interest.
Publisher’s Note
All claims expressed in this article are solely those of the authors and do not necessarily represent those of their affiliated organizations, or those of the publisher, the editors and the reviewers. Any product that may be evaluated in this article, or claim that may be made by its manufacturer, is not guaranteed or endorsed by the publisher.
References
1. Chopra H, Mishra AK, Baig AA, Mohanta TK, Mohanta YK, Baek K-H. Narrative Review: Bioactive Potential of Various Mushrooms as the Treasure of Versatile Therapeutic Natural Product. J Fungi (2021) 7:728. doi: 10.3390/JOF7090728
2. Kour J, Chopra H, Bukhari S, Sharma R, Bansal R, Hans M, et al. Nutraceutical-A Deep and Profound Concept. Nutraceuticals Heal Care (2022) 1:1–28. doi: 10.1016/B978-0-323-89779-2.00021-1
3. Bhattacharya T, Soares GAB, Chopra E, Rahman H., Hasan M.M., Swain Z.S., Cavalu S.S. Applications of Phyto-Nanotechnology for the Treatment of Neurodegenerative Disorders. Mater 2022 (2022) 15:804. doi: 10.3390/MA15030804
4. Nair HB, Sung B, Yadav VR, Kannappan R, Chaturvedi MM, Aggarwal BB. Delivery of Antiinflammatory Nutraceuticals by Nanoparticles for the Prevention and Treatment of Cancer. Biochem Pharmacol (2010) 80:1833–43. doi: 10.1016/j.bcp.2010.07.021
5. Holcombe RF, Martinez M, Planutis K, Planutiene M. Effects of a Grape-Supplemented Diet on Proliferation and Wnt Signaling in the Colonic Mucosa Are Greatest for Those Over Age 50 and With High Arginine Consumption. Nutr J (2015) 14:1–8. doi: 10.1186/s12937-015-0050-z
6. Linnewiel-Hermoni K, Khanin M, Danilenko M, Zango G, Amosi Y, Levy J, et al. The Anti-Cancer Effects of Carotenoids and Other Phytonutrients Resides in Their Combined Activity. Arch Biochem Biophys (2015) 572:28–35. doi: 10.1016/j.abb.2015.02.018
7. Piao L, Mukherjee S, Chang Q, Xie X, Li H, Castellanos MR, et al. TriCurin, a Novel Formulation of Curcumin, Epicatechin Gallate, and Resveratrol, Inhibits the Tumorigenicity of Human Papillomaviruspositive Head and Neck Squamous Cell Carcinoma. Oncotarget (2017) 8:60025–35. doi: 10.18632/oncotarget.10620
8. Cen L, Hutzen B, Ball S, DeAngelis S, Chen CL, Fuchs JR, et al. New Structural Analogues of Curcumin Exhibit Potent Growth Suppressive Activity in Human Colorectal Carcinoma Cells. BMC Cancer (2009) 9:1–8. doi: 10.1186/1471-2407-9-99
9. Lassed S, Deus CM, Djebbari R, Zama D, Oliveira PJ, Rizvanov AA, et al. Protective Effect of Green Tea (Camellia Sinensis (L.) Kuntze) Against Prostate Cancer: From In Vitro Data to Algerian Patients. Evidence-Based Complement. Altern Med (2017) 2017:1–12. doi: 10.1155/2017/1691568
10. Diaz-Gerevini GT, Repossi G, Dain A, Tarres MC, Das UN, Eynard AR. Beneficial Action of Resveratrol: How and Why? Nutrition (2016) 32:174–8. doi: 10.1016/j.nut.2015.08.017
11. McCubrey JA, Lertpiriyapong K, Steelman LS, Abrams SL, Yang LV, Murata RM, et al. Effects of Resveratrol, Curcumin, Berberine and Other Nutraceuticals on Aging, Cancer Development, Cancer Stem Cells and microRNAs. Aging (Albany NY) (2017) 9:1477–536. doi: 10.18632/aging.101250
12. Faridi Esfanjani A, Assadpour E, Jafari SM. Improving the Bioavailability of Phenolic Compounds by Loading Them Within Lipid-Based Nanocarriers. Trends Food Sci Technol (2018) 76:56–66. doi: 10.1016/j.tifs.2018.04.002
13. Gonçalves RFS, Martins JT, Duarte CMM, Vicente AA, Pinheiro AC. Advances in Nutraceutical Delivery Systems: From Formulation Design for Bioavailability Enhancement to Efficacy and Safety Evaluation. Trends Food Sci Technol (2018) 78:270–91. doi: 10.1016/J.TIFS.2018.06.011
14. Paolino D, Mancuso A, Cristiano MC, Froiio F, Lammari N, Celia C, et al. Nanonutraceuticals: The New Frontier of Supplementary Food. Nanomaterials (2021) 11:1–19. doi: 10.3390/nano11030792
15. Kumar AR, Lingaiah BPV, Rao PS, Narsaiah B, Sriram D, Sowjanya P. Synthesis and Biological Evaluation of Novel N1-Decyl and C7- Sec Amine Substituted Fluoroquinolones as Antitubercular and Anticancer Agents. Indian J Chem - Sect. B Org Med Chem (2015) 54B:1495–501.
16. Sharma CP, Kaur G., Pahwa R., Sharma A., Rajak H. Quinazolinone Analogs as Potential Therapeutic Agents. Curr Med Chem (2011) 18:4786 – 4812. doi: 10.2174/092986711797535326
17. de Santana TI, Barbosa MdeO, Gomes PATdeM, da Cruz ACN, da Silva TG, Leite ACL. Synthesis, Anticancer Activity and Mechanism of Action of New Thiazole Derivatives. Eur J Med Chem (2018) 144:874–86. doi: 10.1016/j.ejmech.2017.12.040
18. Ranzato E, Martinotti S, Calabrese CM, Calabrese G. Role of Nutraceuticals in Cancer Therapy. J Food Res (2014) 3:18–25. doi: 10.5539/jfr.v3n4p18
19. Sreedhar A, Li J, Zhao Y. Next-Gen Therapeutics for Skin Cancer: Nutraceuticals. Nutr Cancer (2018) 70:697–709. doi: 10.1080/01635581.2018.1470651
20. Sharma PC, Goyal R, Sharma A, Sharma D, Saini N, Rajak H, et al. Insights on Fluoroquinolones in Cancer Therapy: Chemistry and Recent Developments. Mater Today Chem (2020) 17:1–11. doi: 10.1016/j.mtchem.2020.100296
21. Kuppusamy P, Yusoff MM, Maniam GP, Ichwan SJA, Soundharrajan I, Govindan N. Nutraceuticals as Potential Therapeutic Agents for Colon Cancer: A Review. Acta Pharm Sin B (2014) 4:173–81. doi: 10.1016/j.apsb.2014.04.002
22. Ahmad A, Ginnebaugh KR, Li Y, Padhye SB, Sarkar FH. Molecular Targets of Naturopathy in Cancer Research: Bridge to Modern Medicine. Nutrients (2015) 7:321–34. doi: 10.3390/nu7010321
23. Arora D, Jaglan S. Nanocarriers Based Delivery of Nutraceuticals for Cancer Prevention and Treatment: A Review of Recent Research Developments. Trends Food Sci Technol (2016) 54:114–26. doi: 10.1016/j.tifs.2016.06.003
24. Gallazzi M, Festa M, Corradino P, Sansone C, Albini A, Noonan DM. An Extract of Olive Mill Wastewater Downregulates Growth, Adhesion and Invasion Pathways in Lung Cancer Cells: Involvement of CXCR4. Nutrients (2020) 12:1–16. doi: 10.3390/nu12040903
25. Demidenko ZN, Blagosklonny MV. Flavopiridol Induces P53 via Initial Inhibition of Mdm2 and P21 and, Independently of P53, Sensitizes Apoptosis-Reluctant Cells to Tumor Necrosis Factor. Cancer Res (2004) 64:3653–60. doi: 10.1158/0008-5472.CAN-04-0204
26. Sarkar FH, Li Y, Wang Z, Kong D. The Role of Nutraceuticals in the Regulation of Wnt and Hedgehog Signaling in Cancer. Cancer Metastasis Rev (2010) 29:383–94. doi: 10.1007/s10555-010-9233-4
27. Romanucci V, Giordano M, Pagano R, Agarwal C, Agarwal R, Zarrelli A, et al. Solid-Phase Synthesis of Curcumin Mimics and Their Anticancer Activity Against Human Pancreatic, Prostate, and Colorectal Cancer Cell Lines. Bioorg. Med Chem (2021) 42:116249. doi: 10.1016/J.BMC.2021.116249
28. Mirzaei A, Jahanshahi F, Khatami F, Reis LO, Aghamir SMK. Human Prostate Cancer Cell Epithelial-to-Mesenchymal Transition as a Novel Target of Arsenic Trioxide and Curcumin Therapeutic Approach. Tissue Cell (2022) 76:101805. doi: 10.1016/J.TICE.2022.101805
29. Chen Y, Deng Y, Zhu C, Xiang C. Anti Prostate Cancer Therapy: Aptamer-Functionalized, Curcumin and Cabazitaxel Co-Delivered, Tumor Targeted Lipid-Polymer Hybrid Nanoparticles. Biomed Pharmacother (2020) 127:110181. doi: 10.1016/J.BIOPHA.2020.110181
30. Sha J, Li J, Wang W, Pan L, Cheng J, Li L, et al. Curcumin Induces G0/G1 Arrest and Apoptosis in Hormone Independent Prostate Cancer DU-145 Cells by Down Regulating Notch Signaling. Biomed Pharmacother (2016) 84:177–84. doi: 10.1016/J.BIOPHA.2016.09.037
31. Liu T, Chi H, Chen J, Chen C, Huang Y, Xi H, et al. Curcumin Suppresses Proliferation and In Vitro Invasion of Human Prostate Cancer Stem Cells by ceRNA Effect of miR-145 and lncRNA-ROR. Gene (2017) 631:29–38. doi: 10.1016/J.GENE.2017.08.008
32. Yallapu MM, Jaggi M, Chauhan SC. β-Cyclodextrin-Curcumin Self-Assembly Enhances Curcumin Delivery in Prostate Cancer Cells. Colloids Surfaces B Biointerfaces (2010) 79:113–25. doi: 10.1016/J.COLSURFB.2010.03.039
33. Chiyomaru T, Yamamura S, Fukuhara S, Yoshino H, Kinoshita T, Majid S, et al. Genistein Inhibits Prostate Cancer Cell Growth by Targeting miR-34a and Oncogenic HOTAIR. PLoS One (2013) 8:1–10. doi: 10.1371/journal.pone.0070372
34. Wu Y, Zhang L, Na R, Xu J, Xiong Z, Zhang N, et al. Plasma Genistein and Risk of Prostate Cancer in Chinese Population. Int Urol Nephrol (2015) 47:965–70. doi: 10.1007/s11255-015-0981-5
35. Hörmann V, Kumi-Diaka J, Durity M, Rathinavelu A. Anticancer Activities of Genistein-Topotecan Combination in Prostate Cancer Cells. J Cell Mol Med (2012) 16:2631–6. doi: 10.1111/j.1582-4934.2012.01576.x
36. Travis RC, Allen NE, Appleby PN, Price A, Kaaks R, Chang-Claude J, et al. Prediagnostic Concentrations of Plasma Genistein and Prostate Cancer Risk in 1,605 Men With Prostate Cancer and 1,697 Matched Control Participants in EPIC. Cancer Causes Control (2012) 23:1163–71. doi: 10.1007/s10552-012-9985-y
37. Bilir B, Sharma NV, Lee J, Hammarstrom B, Svindland A, Kucuk O, et al. Effects of Genistein Supplementation on Genome-Wide DNA Methylation and Gene Expression in Patients With Localized Prostate Cancer. Int J Oncol (2017) 51:223–34. doi: 10.3892/ijo.2017.4017
38. Xu L, Ding Y, Catalona WJ, Yang XJ, Anderson WF, Jovanovic B, et al. MEK4 Function, Genistein Treatment, and Invasion of Human Prostate Cancer Cells. J Natl Cancer Inst (2009) 101:1141–55. doi: 10.1093/jnci/djp227
39. Wang J, Eltoum IE, Lamartiniere CA. Genistein Chemoprevention of Prostate Cancer in TRAMP Mice. J Carcinog (2007) 6:1–10. doi: 10.1186/1477-3163-6-3
40. Hirata H, Hinoda Y, Shahryari V, Deng G, Tanaka Y, Tabatabai ZL, et al. Genistein Downregulates onco-miR-1260b and Upregulates Sfrp1 and Smad4 via Demethylation and Histone Modification in Prostate Cancer Cells. Br J Cancer (2014) 110:1645–54. doi: 10.1038/bjc.2014.48
41. Suzuki K, Koike H, Matsui H, Ono Y, Hasumi M, Nakazato H, et al. Genistein, a Soy Isoflavone, Induces Glutathione Peroxidase in the Human Prostate Cancer Cell Lines LNCaP and PC-3. Int J Cancer (2002) 99:846–52. doi: 10.1002/ijc.10428
42. Vanella L, Di Giacomo C, Acquaviva R, Barbagallo I, Li Volti G, Cardile V, et al. Effects of Ellagic Acid on Angiogenic Factors in Prostate Cancer Cells. Cancers (Basel) (2013) 5:726–73. doi: 10.3390/cancers5020726
43. Vanella L, Di Giacomo C, Acquaviva R, Barbagallo I, Cardile V, Kim DH, et al. Apoptotic Markers in a Prostate Cancer Cell Line: Effect of Ellagic Acid. Oncol Rep (2013) 30:2804–10. doi: 10.3892/or.2013.2757
44. Eskra JN, Schlicht MJ, Bosland MC. Effects of Black Raspberries and Their Ellagic Acid and Anthocyanin Constituents on Taxane Chemotherapy of Castration-Resistant Prostate Cancer Cells. Sci Rep (2019) 9:1–12. doi: 10.1038/s41598-019-39589-1
45. Pitchakarn P, Chewonarin T, Ogawa K, Suzuki S, Asamoto M, Takahashi S, et al. Ellagic Acid Inhibits Migration and Invasion by Prostate Cancer Cell Lines. Asian Pacific J Cancer Prev (2013) 14:2859–63. doi: 10.7314/APJCP.2013.14.5.2859
46. Wang L, Li W, Lin M, Garcia M, Mulholland D, Lilly M, et al. Luteolin, Ellagic Acid and Punicic Acid Are Natural Products That Inhibit Prostate Cancer Metastasis. Carcinogenesis (2014) 35:2859–63. doi: 10.1093/carcin/bgu145
47. Saleem YIM, Selim MI. MDM2 as a Target for Ellagic Acid.Mediated Suppression of Prostate Cancer Cells. vitro Oncol Rep (2020) 44:1255–65. doi: 10.3892/or.2020.7664
48. Eskra JN, Dodge A, Schlicht MJ, Bosland MC. Effects of Black Raspberries and Their Constituents on Rat Prostate Carcinogenesis and Human Prostate Cancer Cell Growth. In Vitro Nutr Cancer (2020) 72:672–85. doi: 10.1080/01635581.2019.1650943
49. Falsaperla M, Morgia G, Tartarone A, Ardito R, Romano G. Support Ellagic Acid Therapy in Patients With Hormone Refractory Prostate Cancer (HRPC) on Standard Chemotherapy Using Vinorelbine and Estramustine Phosphate. Eur Urol (2005) 47:449–55. doi: 10.1016/j.eururo.2004.12.001
50. Li X, Zhang A, Sun H, Liu Z, Zhang T, Qiu S, et al. Metabolic Characterization and Pathway Analysis of Berberine Protects Against Prostate Cancer. Oncotarget (2017) 8:65022–41. doi: 10.18632/oncotarget.17531
51. Huang ZH, Zheng HF, Wang WL, Wang Y, Zhong LF, Wu JL, et al. Berberine Targets Epidermal Growth Factor Receptor Signaling to Suppress Prostate Cancer Proliferation. vitro Mol Med Rep (2015) 11:2125–8. doi: 10.3892/mmr.2014.2929
52. Liu CH, Tang WC, Sia P, Huang CC, Yang PM, Wu MH, et al. Berberine Inhibits the Metastatic Ability of Prostate Cancer Cells by Suppressing Epithelial-to-Mesenchymal Transition (EMT)-Associated Genes With Predictive and Prognostic Relevance. Int J Med Sci (2015) 12:63–71. doi: 10.7150/ijms.9982
53. Lu W, Du S, Wang J. Berberine Inhibits the Proliferation of Prostate Cancer Cells and Induces G0/G1 or G2/M Phase Arrest at Different Concentrations. Mol Med Rep (2015) 11:3920–4. doi: 10.3892/mmr.2014.3139
54. Zhang LY, Wu YL, Gao XH, Guo F. Mitochondrial Protein Cyclophilin-D-Mediated Programmed Necrosis Attributes to Berberine-Induced Cytotoxicity in Cultured Prostate Cancer Cells. Biochem Biophys Res Commun (2014) 450:697–703. doi: 10.1016/j.bbrc.2014.06.039
55. Choi MS, Oh JH, Kim SM, Jung HY, Yoo HS, Lee YM, et al. Berberine Inhibits P53-Dependent Cell Growth Through Induction of Apoptosis of Prostate Cancer Cells. Int J Oncol (2009) 34:1221–30. doi: 10.3892/ijo_00000250
56. Zhang Q, Zhang C, Yang X, Yang B, Wang J, Kang Y, et al. Berberine Inhibits the Expression of Hypoxia Induction Factor-1alpha and Increases the Radiosensitivity of Prostate Cancer. Diagn Pathol (2014) 9:1–7. doi: 10.1186/1746-1596-9-98
57. Meeran SM, Katiyar S, Katiyar SK. Berberine-Induced Apoptosis in Human Prostate Cancer Cells Is Initiated by Reactive Oxygen Species Generation. Toxicol Appl Pharmacol (2008) 229:33–43. doi: 10.1016/j.taap.2007.12.027
58. Mathangi, Nalini D, Ponnulakshmi R, Prasad M, Priya L, Selvaraj J, et al. Chemo-Preventive Effect of 1-Piperoyl Piperidine (Piperine) Is Mediated Through Intrinsic Signaling Mechanisms in Human Prostate Cancer Cells (Pc-3) In Vitro. J Angiother (2021) 5:1–7. doi: 10.25163/ANGIOTHERAPY.52214112308081221
59. Ouyang Dy, Zeng Lh, Pan H, Xu Lh, Wang Y, Liu Kp, et al. Piperine Inhibits the Proliferation of Human Prostate Cancer Cells via Induction of Cell Cycle Arrest and Autophagy. Food Chem Toxicol (2013) 60:424–30. doi: 10.1016/j.fct.2013.08.007
60. George K, Thomas NS, Malathi R. Piperine Blocks Voltage Gated K + Current and Inhibits Proliferation in Androgen Sensitive and Insensitive Human Prostate Cancer Cell Lines. Arch Biochem Biophys (2019) 667:36–48. doi: 10.1016/j.abb.2019.04.007
61. Ba Y, Malhotra A. Potential of Piperine in Modulation of Voltagegated K+ Current and its Influences on Cell Cycle Arrest and Apoptosis in Human Prostate Cancer Cells. Eur Rev Med Pharmacol Sci (2018) 22:8999–9011. doi: 10.26355/eurrev_201812_16671
62. Jonnalagadda B, Arockiasamy S, Vetrivel U. P. A, A. In Silico Docking of Phytocompounds to Identify Potent Inhibitors of Signaling Pathways Involved in Prostate Cancer. J Biomol Struct Dyn (2020) 39(14):5182–208. doi: 10.1080/07391102.2020.1785944
63. Crocetto F, Di Zazzo E, Buonerba C, Aveta A, Pandolfo SD, Barone B, et al. Kaempferol, Myricetin and Fisetin in Prostate and Bladder Cancer: A Systematic Review of the Literature. Nutrients (2021) 13:1–14. doi: 10.3390/nu13113750
64. Szliszka E, Helewski KJ, Mizgala E, Krol W. The Dietary Flavonol Fisetin Enhances the Apoptosis-Inducing Potential of TRAIL in Prostate Cancer Cells. Int J Oncol (2011) 39:771–779. doi: 10.3892/ijo.2011.1116
65. Adhami VM, Syed DN, Khan N, Mukhtar H. Dietary Flavonoid Fisetin: A Novel Dual Inhibitor of PI3K/Akt and mTOR for Prostate Cancer Management. Biochem Pharmacol (2012) 84:1277–81. doi: 10.1016/j.bcp.2012.07.012
66. Haddad AQ, Fleshner N, Nelson C, Saour B, Musquera M, Venkateswaran V, et al. Antiproliferative Mechanisms of the Flavonoids 2,2’-Dihydroxychalcone and Fisetin in Human Prostate Cancer Cells. Nutr Cancer (2010) 62:668–81. doi: 10.1080/01635581003605524
67. Khan MI, Adhami VM, Lall RK, Sechi M, Joshi DC, Haidar OM, et al. YB-1 Expression Promotes Epithelial-to-Mesenchymal Transition in Prostate Cancer That Is Inhibited by a Small Molecule Fisetin. Oncotarget (2014) 5:2462–74. doi: 10.18632/oncotarget.1790
68. Kashyap D, Garg VK, Tuli HS, Yerer MB, Sak K, Sharma AK, et al. Fisetin and Quercetin: Promising Flavonoids With Chemopreventive Potential. Biomolecules (2019) 9:1–22. doi: 10.3390/biom9050174
69. Lorenzo G, Scafuri L, Costabile F, Pepe L, Scognamiglio A, Crocetto F, et al. Fisetin as an Adjuvant Treatment in Prostate Cancer Patients Receiving Androgen-Deprivation Therapy. Futur Sci OA (2022) 8:1–3. doi: 10.2144/fsoa-2022-0002
70. Khan N, Afaq F, Syed DN, Mukhtar H. Fisetin, a Novel Dietary Flavonoid, Causes Apoptosis and Cell Cycle Arrest in Human Prostate Cancer LNCaP Cells. Carcinogenesis (2008) 29:1049–56. doi: 10.1093/carcin/bgn078
71. Paludo MC, de Oliveira SBP, de Oliveira LF, Colombo RC, Gómez-Alonso S, Hermosín-Gutiérrez I, et al. Phenolic Composition of Peels From Different Jaboticaba Species Determined by HPLC-DAD-ESI/MSn and Antiproliferative Activity in Tumor Cell Lines. Curr Plant Biol (2022) 29:1–12. doi: 10.1016/j.cpb.2021.100233
72. Ranjha MMAN, Shafique B, Wang L, Irfan S, Safdar MN, Murtaza MA, et al. A Comprehensive Review on Phytochemistry, Bioactivity and Medicinal Value of Bioactive Compounds of Pomegranate (Punica Granatum). Adv Tradit. Med (2021) 1–21. doi: 10.1007/s13596-021-00566-7
73. Leite-Legatti AV, Batista AG, Dragano NRV, Marques AC, Malta LG, Riccio MF, et al. Jaboticaba Peel: Antioxidant Compounds, Antiproliferative and Antimutagenic Activities. Food Res Int (2012) 49:596–603. doi: 10.1016/j.foodres.2012.07.044
74. Kim MH, Kim MH, Park YJ, Chang YC, Park YY, Song HO. Delphinidin Suppresses Angiogenesis via the Inhibition of HIF-1α and STAT3 Expressions in PC3M Cells. Korean J Food Sci Technol (2016) 48:66–71. doi: 10.9721/KJFST.2016.48.1.66
75. Hafeez B. Bin; Asim, M.; Siddiqui, I.A.; Adhami, V.M.; Murtaza, I.; Mukhtar, H. Delphinidin, a Dietary Anthocyanidin in Pigmented Fruits and Vegetables: A New Weapon to Blunt Prostate Cancer Growth. Cell Cycle (2008) 7:3320–6. doi: 10.4161/cc.7.21.6969
76. Mottaghipisheh J, Doustimotlagh AH, Irajie C, Tanideh N, Barzegar A, Iraji A. The Promising Therapeutic and Preventive Properties of Anthocyanidins/Anthocyanins on Prostate Cancer. Cells (2022) 11:1–27. doi: 10.3390/cells11071070
77. Lu Z, Zhou R, Kong Y, Wang J, Xia W, Guo J, et al. S-Equol, a Secondary Metabolite of Natural Anticancer Isoflavone Daidzein, Inhibits Prostate Cancer Growth. In Vitro and In Vivo Though Activating Akt/FOXO3a Pathway Curr Cancer Drug Targets (2016) 16:455–65. doi: 10.2174/1568009616666151207105720
78. Sivoňová MK, Kaplán P, Tatarková Z, Lichardusová L, Dušenka R, Jurečeková J. Androgen Receptor and Soy Isoflavones in Prostate Cancer (Review). Mol Clin Oncol (2019) 10:191–204. doi: 10.3892/mco.2018.1792
79. Adjakly M, Ngollo M, Boiteux JP, Bignon YJ, Guy L, Bernard-Gallon D. Genistein and Daidzein: Different Molecular Effects on Prostate Cancer. Anticancer Res (2013) 33:39–44.
80. Akaza H, Miyanaga N, Takashima N, Naito S, Hirao Y, Tsukamoto T, et al. Is Daidzein non-Metabolizer a High Risk for Prostate Cancer? A Case-Controlled Study of Serum Soybean Isoflavone Concentration. Jpn J Clin Oncol (2002) 32:296–300. doi: 10.1093/jjco/hyf064
81. Liu Y, Wu X, Jiang H. High Dietary Fat Intake Lowers Serum Equol Concentration and Promotes Prostate Carcinogenesis in a Transgenic Mouse Prostate Model. Nutr Metab (2019) 16:1–7. doi: 10.1186/s12986-019-0351-x
82. Dong X, Xu W, Sikes RA, Wu C. Combination of Low Dose of Genistein and Daidzein has Synergistic Preventive Effects on Isogenic Human Prostate Cancer Cells When Compared With Individual Soy Isoflavone. Food Chem (2013) 141:1923–33. doi: 10.1016/j.foodchem.2013.04.109
83. Singh-Gupta V, Zhang H, Yunker CK, Ahmad Z, Zwier D, Sarkar FH, et al. Daidzein Effect on Hormone Refractory Prostate Cancer In Vitro and In Vivo Compared to Genistein and Soy Extract: Potentiation of Radiotherapy. Pharm Res (2010) 27:1115–27. doi: 10.1007/s11095-010-0107-9
84. Ajdžanović V, Mojić M, Maksimović-Ivanić D, Bulatović M, Mijatović S, Milošević V, et al. Membrane Fluidity, Invasiveness and Dynamic Phenotype of Metastatic Prostate Cancer Cells After Treatment With Soy Isoflavones. J Membr Biol (2013) 246:307–14. doi: 10.1007/s00232-013-9531-1
85. Pan H, Jansson KH, Beshiri ML, Yin JJ, Fang L, Agarwal S, et al. Gambogic Acid Inhibits Thioredoxin Activity and Induces ROSmediated Cell Death in Castration-Resistant Prostate Cancer. Oncotarget (2017) 8:77181–94. doi: 10.18632/oncotarget.20424
86. Pan H, Lu Ly, Wang Xq, Li Bx, Kelly K, Lin Hs. Gambogic Acid Induces Cell Apoptosis and Inhibits MAPK Pathway in PTEN–/–/p53–/– Prostate Cancer Cells. In Vitro and Ex Vivo Chin J Integr Med (2018) 24:109–16. doi: 10.1007/s11655-017-2410-3
87. Lü L, Tang D, Wang L, Huang LQ, Jiang GS, Xiao XY, et al. Gambogic Acid Inhibits TNF-α-Induced Invasion of Human Prostate Cancer PC3 Cells In Vitro Through PI3K/Akt and NF-κb Signaling Pathways. Acta Pharmacol Sin (2012) 33:531–41. doi: 10.1038/aps.2011.180
88. Saeed LM, Mahmood M, Xu Y, Nima ZA, Kannarpady GK, Bratton SM, et al. Nanodelivery of Gambogic Acid by Functionalized Graphene Enhances Inhibition of Cell Proliferation and Induces G0/G1 Cell Cycle Arrest in Cervical, Ovarian, and Prostate Cancer Cells. RSC Adv (2015) 5:44022–30. doi: 10.1039/c5ra00833f
89. Mirahmadi M, Azimi-Hashemi S, Saburi E, Kamali H, Pishbin M, Hadizadeh F. Potential Inhibitory Effect of Lycopene on Prostate Cancer. Biomed Pharmacother (2020) 129:1–8. doi: 10.1016/j.biopha.2020.110459
90. Jiang LN, Liu Y. Bin; Li, B.H. Lycopene Exerts Anti-Inflammatory Effect to Inhibit Prostate Cancer Progression. Asian J Androl (2019) 21:80–5. doi: 10.4103/aja.aja_70_18
91. Zu K, Mucci L, Rosner BA, Clinton SK, Loda M, Stampfer MJ, et al. Dietary Lycopene, Angiogenesis, and Prostate Cancer: A Prospective Study in the Prostate-Specific Antigen Era. J Natl Cancer Inst (2014) 106:1–10. doi: 10.1093/jnci/djt430
92. Holzapfel NP, Holzapfel BM, Champ S, Feldthusen J, Clements J, Hutmacher DW. The Potential Role of Lycopene for the Prevention and Therapy of Prostate Cancer: From Molecular Mechanisms to Clinical Evidence. Int J Mol Sci (2013) 14:14620–46. doi: 10.3390/ijms140714620
93. Wei MY, Giovannucci EL. Lycopene, Tomato Products, and Prostate Cancer Incidence: A Review and Reassessment in the Psa Screening Era. J Oncol (2012) 2012:1–7. doi: 10.1155/2012/271063
94. Haseen F, Cantwell MM, O’Sullivan JM, Murray LJ. Is There a Benefit From Lycopene Supplementation in Men With Prostate Cancer? A Systematic Review. Prostate Cancer Prostatic Dis (2009) 12(4)1–11. doi: 10.1017/S0029665109990802
95. Elgass S, Cooper A, Chopra M. Lycopene Treatment of Prostate Cancer Cell Lines Inhibits Adhesion and Migration Properties of the Cells. Int J Med Sci (2014) 11:948–54. doi: 10.7150/ijms.9137
96. Rafi MM, Kanakasabai S, Reyes MD, Bright JJ. Lycopene Modulates Growth and Survival Associated Genes in Prostate Cancer. J Nutr Biochem (2013) 24:1724–34. doi: 10.1016/j.jnutbio.2013.03.001
97. Kristal AR, Till C, Platz EA, Song X, King IB, Neuhouser ML, et al. Serum Lycopene Concentration and Prostate Cancer Risk: Results From the Prostate Cancer Prevention Trial. Cancer Epidemiol. Biomarkers Prev (2011) 20:638–46. doi: 10.1158/1055-9965.EPI-10-1221
98. Fraser ML, Lee AH, Binns CW. Lycopene and Prostate Cancer: Emerging Evidence. Expert Rev Anticancer Ther (2005) 5(5):847–54. doi: 10.1586/14737140.5.5.847
99. Seo Y, Ryu K, Park J, Jeon DK, Jo S, Lee HK, et al. Inhibition of ANO1 by Luteolin and Its Cytotoxicity in Human Prostate Cancer PC-3 Cells. PLoS One (2017) 12:1–13. doi: 10.1371/journal.pone.0174935
100. Han K, Meng W, Zhang JJ, Zhou Y, Wang YL, Su Y, et al. Luteolin Inhibited Proliferation and Induced Apoptosis of Prostate Cancer Cells Through miR-301. Onco Targets Ther (2016) 9:3085–94. doi: 10.2147/OTT.S102862
101. Ye Y, Huang Z, Chen M, Mo Y, Mo Z. Luteolin Potentially Treating Prostate Cancer and COVID-19 Analyzed by the Bioinformatics Approach: Clinical Findings and Drug Targets. Front Endocrinol (Lausanne) (2022) 12:1–9. doi: 10.3389/fendo.2021.802447
102. Naiki-Ito A, Naiki T, Kato H, Iida K, Etani T, Nagayasu Y, et al. Recruitment of miR-8080 by Luteolin Inhibits Androgen Receptor Splice Variant 7 Expression in Castration-Resistant Prostate Cancer. Carcinogenesis (2020) 41:1145–57. doi: 10.1093/carcin/bgz193
103. Han K, Lang T, Zhang Z, Zhang Y, Sun Y, Shen Z, et al. Luteolin Attenuates Wnt Signaling via Upregulation of FZD6 to Suppress Prostate Cancer Stemness Revealed by Comparative Proteomics. Sci Rep (2018) 8:1–13. doi: 10.1038/s41598-018-26761-2
104. Zhou Q, Yan B, Hu X, Li XB, Zhang J, Fang J. Luteolin Inhibits Invasion of Prostate Cancer PC3 Cells Through E-Cadherin. Mol Cancer Ther (2009) 8:1684–91. doi: 10.1158/1535-7163.MCT-09-0191
105. Imran M, Rauf A, Abu-Izneid T, Nadeem M, Shariati MA, Khan IA, et al. Luteolin, a Flavonoid, as an Anticancer Agent: A Review. Biomed Pharmacother (2019) 112:1–10. doi: 10.1016/j.biopha.2019.108612
106. Fang J, Zhou Q, Shi XL, Jiang BH. Luteolin Inhibits Insulin-Like Growth Factor 1 Receptor Signaling in Prostate Cancer Cells. Carcinogenesis (2007) 28:713–23. doi: 10.1093/carcin/bgl189
107. Chiu FL, Lin JK. Downregulation of Androgen Receptor Expression by Luteolin Causes Inhibition of Cell Proliferation and Induction of Apoptosis in Human Prostate Cancer Cells and Xenografts. Prostate (2008) 68:61–71. doi: 10.1002/pros.20690
108. Ganai SA, Sheikh FA, Baba ZA, Mir MA, Mantoo MA, Yatoo MA. Anticancer Activity of the Plant Flavonoid Luteolin Against Preclinical Models of Various Cancers and Insights on Different Signalling Mechanisms Modulated. Phyther Res (2021) 35:3509–32. doi: 10.1002/ptr.7044
109. Singh KB, Hahm ER, Rigatti LH, Normolle DP, Yuan JM, Singh SV. Inhibition of Glycolysis in Prostate Cancer Chemoprevention by Phenethyl Isothiocyanate. Cancer Prev Res (2018) 11:337–46. doi: 10.1158/1940-6207.CAPR-17-0389
110. Zhang C, Shu L, Kim H, Khor TO, Wu R, Li W, et al. Phenethyl Isothiocyanate (PEITC) Suppresses Prostate Cancer Cell Invasion Epigenetically Through Regulating microRNA-194. Mol Nutr Food Res (2016) 60:1427–36. doi: 10.1002/mnfr.201500918
111. Wang LG, Chiao JW. Prostate Cancer Chemopreventive Activity of Phenethyl Isothiocyanate Through Epigenetic Regulation (Review). Int J Oncol (2010) 37:533–9. doi: 10.3892/ijo_00000702
112. Crowley E, Rowan NJ, Faller D, Friel AM. Natural and Synthetic Isothiocyanates Possess Anticancer Potential Against Liver and Prostate Cancer. In vitro. Anticancer Res (2019) 39:3469–85. doi: 10.21873/anticanres.13493
113. Sakao K, Vyas AR, Chinni SR, Amjad AI, Parikh R, Singh SV. CXCR4 is a Novel Target of Cancer Chemopreventative Isothiocyanates in Prostate Cancer Cells. Cancer Prev Res (2015) 8:365–74. doi: 10.1158/1940-6207.CAPR-14-0386
114. Wang C, Sargsyan D, Zhang C, Wu R, Yang Y, Kong AN. Transcriptomic Analysis of Histone Methyltransferase Setd7 Knockdown and Phenethyl Isothiocyanate in Human Prostate Cancer Cells. Anticancer Res (2018) 38:6069–83. doi: 10.21873/anticanres.12957
115. Novío S, Cartea ME, Soengas P, Freire-Garabal M, Núñez-Iglesias MJ. Effects of Brassicaceae Isothiocyanates on Prostate Cancer. Molecules (2016) 21:1–28. doi: 10.3390/molecules21050626
116. Sung B, Prasad S, Yadav VR, Aggarwal BB. Cancer Cell Signaling Pathways Targeted by Spice-Derived Nutraceuticals. Nutr Cancer (2012) 64:173–97. doi: 10.1080/01635581.2012.630551
117. Bray F, Ferlay J, Soerjomataram I, Siegel RL, Torre LA, Jemal A. Global Cancer Statistics 2018: GLOBOCAN Estimates of Incidence and Mortality Worldwide for 36 Cancers in 185 Countries. CA Cancer J Clin (2018) 68:394–424. doi: 10.3322/caac.21492
118. Carlsson SV, Vickers AJ. Screening for Prostate Cancer. Med Clin North Am (2020) 104:1051–62. doi: 10.1016/j.mcna.2020.08.007
119. Merriel SWD, Funston G, Hamilton W. Prostate Cancer in Primary Care. Adv Ther (2018) 35:1285–94. doi: 10.1007/s12325-018-0766-1
120. Schatten H. Brief Overview of Prostate Cancer Statistics, Grading, Diagnosis and Treatment Strategies. In: Schatten H. (eds) Cell & Molecular Biology of Prostate Cancer. Advances in Experimental Medicine and Biology, Vol. 1095. (2018). Cham:Springer, Cham. doi: 10.1007/978-3-319-95693-0_1.
121. Sung H, Ferlay J, Siegel RL, Laversanne M, Soerjomataram I, Jemal A, et al. Global Cancer Statistics 2020: GLOBOCAN Estimates of Incidence and Mortality Worldwide for 36 Cancers in 185 Countries. CA Cancer J Clin (2021) 71:209–49. doi: 10.3322/CAAC.21660
122. Etzioni R, Tsodikov A, Mariotto A, Szabo A, Falcon S, Wegelin J, et al. Quantifying the Role of PSA Screening in the US Prostate Cancer Mortality Decline. Cancer Causes Control (2008) 19:175–81. doi: 10.1007/s10552-007-9083-8
123. Marker PC, Donjacour AA, Dahiya R, Cunha GR. Hormonal, Cellular, and Molecular Control of Prostatic Development. Dev Biol (2003) 253:165–74. doi: 10.1016/S0012-1606(02)00031-3
124. McNeal JE. Regional Morphology and Pathology of the Prostate. Am J Clin Pathol (1968) 49:347–57. doi: 10.1093/ajcp/49.3.347
125. Singla RK, Sai CS, Chopra H, Behzad S, Bansal H, Goyal R, et al. Natural Products for the Management of Castration-Resistant Prostate Cancer: Special Focus on Nanoparticles Based Studies. Front Cell Dev Biol (2021) 9:1–15. doi: 10.3389/FCELL.2021.745177
126. Daniel M, Dehm SM. Lessons From Tissue Compartment-Specific Analysis of Androgen Receptor Alterations in Prostate Cancer. J Steroid Biochem Mol Biol (2017) 166:28–37. doi: 10.1016/j.jsbmb.2016.04.016
127. Wang Z, Fan J, Liu M, Yeung S, Chang A, Chow MS, et al. Nutraceuticals for Prostate Cancer Chemoprevention: From Molecular Mechanisms to Clinical Application. Expert Opin Investig Drugs (2013) 22:1613–26. doi: 10.1517/13543784.2013.833183
128. Sumanasuriya S, De Bono J. Treatment of Advanced Prostate Cancer—A Review of Current Therapies and Future Promise. Cold Spring Harb Perspect Med (2018) 8:1–13. doi: 10.1101/cshperspect.a030635
129. Board, P.D.Q.A.T.E. Prostate Cancer Treatment (PDQ(R)): Health Professional Version. PDQ Cancer Inf Summ (2002) accessed on: https://www.cancer.gov/types/prostate/hp/prostate-treatment-pdq.
130. Seruga B, Tannock IF. Chemotherapy-Based Treatment for Castration-Resistant Prostate Cancer. J Clin Oncol (2011) 29:3686–94. doi: 10.1200/JCO.2010.34.3996
131. Wissing MD, Van Oort IM, Gerritsen WR, Van Den Eertwegh AJM, Coenen JLLM, Bergman AM, et al. Cabazitaxel in Patients With Metastatic Castration-Resistant Prostate Cancer: Results of a Compassionate Use Program in the Netherlands. Clin Genitourin Cancer (2013) 11:P238–50. doi: 10.1016/j.clgc.2013.04.004
132. Fizazi K, Scher HI, Molina A, Logothetis CJ, Chi KN, Jones RJ, et al. Abiraterone Acetate for Treatment of Metastatic Castration-Resistant Prostate Cancer: Final Overall Survival Analysis of the COU-AA-301 Randomised, Double-Blind, Placebo-Controlled Phase 3 Study. Lancet Oncol (2012) 13:P983–92-. doi: 10.1016/S1470-2045(12)70379-0
133. Hoffman-Censits J, Kelly WK. Enzalutamide: A Novel Antiandrogen for Patients With Castrate-Resistant Prostate Cancer. Clin Cancer Res (2013) 19:1335–9. doi: 10.1158/1078-0432.CCR-12-2910
134. Parker C, Nilsson S, Heinrich D, Helle SI, O’Sullivan JM, Fosså SD, et al. Alpha Emitter Radium-223 and Survival in Metastatic Prostate Cancer. N Engl J Med (2013) 369:213–23. doi: 10.1056/nejmoa1213755
135. Di Lorenzo G, Ferro M, Buonerba C. Sipuleucel-T (Provenge®) for Castration-Resistant Prostate Cancer. BJU Int (2012) 110:E99–E104. doi: 10.5114/aoms.2012.31610
136. Merry DE, Walcott JL. Trinucleotide Repeat Disease: The Androgen Receptor in Spinal and Bulbar Muscular Atrophy. Vitam Horm (2002) 65:149–57. doi: 10.1016/s0083-6729(02)65062-9
137. Casella R, Maduro MR, Lipshultz LI, Lamb DJ. Significance of the Polyglutamine Tract Polymorphism in the Androgen Receptor. Urology (2001) 58:P651–6. doi: 10.1016/S0090-4295(01)01401-7
138. Agoulnik IU, Weigel NL. Androgen Receptor Action in Hormone-Dependent and Recurrent Prostate Cancer. J Cell Biochem (2006) 99:362–72. doi: 10.1002/jcb.20811
139. Edwards J, Bartlett JMS. The Androgen Receptor and Signal-Transduction Pathways in Hormone-Refractory Prostate Cancer. Part 1: Modifications to the Androgen Receptor. BJU Int (2005) 95:1320–6. doi: 10.1111/j.1464-410X.2005.05526.x
140. Chmelar R, Buchanan G, Need EF, Tilley W, Greenberg NM. Androgen Receptor Coregulators and Their Involvement in the Development and Progression of Prostate Cancer. Int J Cancer (2007) 120:719–33. doi: 10.1002/ijc.22365
141. Denmeade SR, Lin XS, Isaacs JT. Role of Programmed (Apoptotic) Cell Death During the Progression and Therapy for Prostate Cancer. Prostate (1996) 28:251–65. doi: 10.1002/(SICI)1097-0045(199604)28:4<251::AID-PROS6>3.0.CO;2-G
142. Kumar N, Jose J. Current Developments in the Nanomediated Delivery of Photoprotective Phytochemicals. Environ Sci pollut Res (2020) 27:38446–71. doi: 10.1007/s11356-020-10100-y
143. Sasikumar A, Kamalasanan K. Nanomedicine for Prostate Cancer Using Nanoemulsion: A Review. J Control Release (2017) 260:111–23. doi: 10.1016/j.jconrel.2017.06.001
144. Salama L, Pastor ER, Stone T, Mousa SA. Emerging Nanopharmaceuticals and Nanonutraceuticals in Cancer Management. Biomedicines (2020) 8:1–22. doi: 10.3390/biomedicines8090347
145. Durazzo A, Nazhand A, Lucarini M, Atanasov AG, Souto EB, Novellino E, et al. An Updated Overview on Nanonutraceuticals: Focus on Nanoprebiotics and Nanoprobiotics. Int J Mol Sci (2020) 21:1–24. doi: 10.3390/ijms21072285
146. Daliu P, Santini A, Novellino EA. Decade of Nutraceutical Patents: Where are We Now in 2018? Expert Opin Ther Pat (2018) 28:875–82. doi: 10.1080/13543776.2018.1552260
147. Mills PK, Beeson WL, Phillips RL, Fraser GE. Cohort Study of Diet, Lifestyle, and Prostate Cancer in Adventist Men. Cancer (1989) 64:598–604. doi: 10.1002/1097-0142(19890801)64:3<598::AID-CNCR2820640306>3.0.CO;2-6
148. Scarpitti BT, Chitchumroonchokchai C, Clinton SK, Schultz ZD. In Vitro Imaging of Lycopene Delivery to Prostate Cancer Cells. Anal Chem (2022) 94:5106–12. doi: 10.1021/ACS.ANALCHEM.1C05442
149. Siler U, Herzog A, Spitzer V, Seifert N, Denelavas A, Hunziker PB, et al. Lycopene Effects on Rat Normal Prostate and Prostate Tumor Tissue. J Nutr (2005) 135:2050S–2S
150. Ansari MS, Gupta NP. Lycopene: A Novel Drug Therapy in Hormone Refractory Metastatic Prostate Cancer. Urol Oncol Semin Orig Investig (2004) 22:415–20. doi: 10.1016/S1078-1439(04)00122-X
151. Giovannucci E. A Review of Epidemiologic Studies of Tomatoes, Lycopene, and Prostate Cancer. Proc Exp Biol Med (2002) 227:852–9
152. Basu A, Imrhan V. Tomatoes Versus Lycopene in Oxidative Stress and Carcinogenesis: Conclusions From Clinical Trials. Eur J Clin Nutr (2007) 61:295–303. doi: 10.1038/sj.ejcn.1602510
153. Liu X, Allen JD, Arnold JT, Blackman MR. Lycopene Inhibits IGF-I Signal Transduction and Growth in Normal Prostate Epithelial Cells by Decreasing DHT-Modulated IGF-I Production in Co-Cultured Reactive Stromal Cells. Carcinogenesis (2008) 29:816–23. doi: 10.1093/carcin/bgn011
154. Grzmil M, Hemmerlein B, Thelen P, Schweyer S, Burfiend P. Blockade of the Type I IGF Receptor Expression in Human Prostate Cancer Cells Inhibits Proliferation and Invasion, Up-Regulates IGF Binding Protein-3 and Suppresses MMP-2 Expression. J Pathol (2004) 202:50–9. doi: 10.1002/path.1492
155. Alavi M, Karimi N, Safaei M. Application of Various Types of Liposomes in Drug Delivery Systems. Adv Pharm Bull (2017) 7:3–9. doi: 10.15171/apb.2017.002
156. He H, Lu Y, Qi J, Zhu Q, Chen Z, Wu W. Adapting Liposomes for Oral Drug Delivery. Acta Pharm Sin B (2019) 9:36–48. doi: 10.1016/j.apsb.2018.06.005
157. Guimarães D, Cavaco-Paulo A, Nogueira E. Design of Liposomes as Drug Delivery System for Therapeutic Applications. Int J Pharm (2021) 601:1–15. doi: 10.1016/j.ijpharm.2021.120571
158. Koning GA, Storm G. Targeted Drug Delivery Systems for the Intracellular Delivery of Macromolecular Drugs. Drug Discov Today (2003) 8:482–3. doi: 10.1016/s1359-6446(03)02699-0
159. Xia Y, Xu C, Zhang X, Ning P, Wang Z, Tian J, et al. Liposome-Based Probes for Molecular Imaging: From Basic Research to the Bedside. Nanoscale (2019) 11:5822–38. doi: 10.1039/C9NR00207C
160. Kamaly N, Miller AD. Paramagnetic Liposome Nanoparticles for Cellular and Tumour Imaging. Int J Mol Sci (2010) 11:1759–76. doi: 10.3390/ijms11041759
161. Onishchenko N, Tretiakova D, Vodovozova E. Spotlight on the Protein Corona of Liposomes. Acta Biomater (2021) 134:57–78. doi: 10.1016/j.actbio.2021.07.074
162. Syed AM, MacMillan P, Ngai J, Wilhelm S, Sindhwani S, Kingston BR, et al. Liposome Imaging in Optically Cleared Tissues. Nano Lett (2020) 20:1362–9. doi: 10.1021/acs.nanolett.9b04853
163. Petersen AL, Hansen AE, Gabizon A, Andresen TL. Liposome Imaging Agents in Personalized Medicine. Adv Drug Deliv Rev (2012) 64:1417–35. doi: 10.1016/j.addr.2012.09.003
164. Gómez-Varela AI, Gaspar R, Miranda A, Assis JL, Valverde RHF, Einicker-Lamas M, et al. Fluorescence Cross-Correlation Spectroscopy as a Valuable Tool to Characterize Cationic Liposome-DNA Nanoparticle Assembly. J Biophotonics (2021) 14. doi: 10.1002/jbio.202000200
165. Son KK, Patel DH, Tkach D, Park A. Cationic Liposome and Plasmid DNA Complexes Formed in Serum-Free Medium Under Optimum Transfection Condition are Negatively Charged. Biochim Biophys Acta - Biomembr (2000) 1466:11–5. doi: 10.1016/S0005-2736(00)00176-0
166. Cong X, Tian H, Liu S, Mao K, Chen H, Xin Y, et al. Cationic Liposome/DNA Complexes Mediate Antitumor Immunotherapy by Promoting Immunogenic Tumor Cell Death and Dendritic Cell Activation. ACS Appl Mater Interfaces (2020) 12:28047–56. doi: 10.1021/acsami.0c08112
167. Capriotti AL, Cavaliere C, Piovesana S. Liposome Protein Corona Characterization as a New Approach in Nanomedicine. Anal Bioanal Chem (2019) 411:4313–26. doi: 10.1007/s00216-019-01656-x
168. Palchetti S, Colapicchioni V, Digiacomo L, Caracciolo G, Pozzi D, Capriotti AL, et al. The Protein Corona of Circulating PEGylated Liposomes. Biochim Biophys Acta - Biomembr (2016) 1858:189–96. doi: 10.1016/j.bbamem.2015.11.012
169. Caracciolo G. Liposome-Protein Corona in a Physiological Environment: Challenges and Opportunities for Targeted Delivery of Nanomedicines. Nanomedicine Nanotechnology Biol Med (2015) 11:543–57. doi: 10.1016/j.nano.2014.11.003
170. Gabrijelčič V. Šentjurc, M. Influence of Hydrogels on Liposome Stability and on the Transport of Liposome Entrapped Substances Into the Skin. Int J Pharm (1995) 118:207–12. doi: 10.1016/0378-5173(94)00362-9
171. Briuglia ML, Rotella C, McFarlane A, Lamprou DA. Influence of Cholesterol on Liposome Stability and on. in Vitro Drug Release Drug Deliv Transl Res (2015) 5:231–42. doi: 10.1007/s13346-015-0220-8
172. Feng S, Sun Y, Wang P, Sun P, Ritzoulis C, Shao P. Co-Encapsulation of Resveratrol and Epigallocatechin Gallate in Low Methoxyl Pectin-Coated Liposomes With Great Stability in Orange Juice. Int J Food Sci Technol (2020) 55:1872–80. doi: 10.1111/ijfs.14323
173. Popova AV, Hincha DK. Effects of Flavonol Glycosides on Liposome Stability During Freezing and Drying. Biochim Biophys Acta - Biomembr (2016) 1858:3050–60. doi: 10.1016/j.bbamem.2016.09.020
174. Chen L, Wu Z, Wu X, Liao Y, Dai X, Shi X. The Application of Coarse-Grained Molecular Dynamics to the Evaluation of Liposome Physical Stability. AAPS PharmSciTech (2020) 21:1–8. doi: 10.1208/s12249-020-01680-6
175. Doskocz J, Dałek P, Foryś A, Trzebicka B, Przybyło M, Mesarec L, et al. The Effect of Lipid Phase on Liposome Stability Upon Exposure to the Mechanical Stress. Biochim Biophys Acta - Biomembr (2020) 1862:1–9. doi: 10.1016/j.bbamem.2020.183361
176. Nakhaei P, Margiana R, Bokov DO, Abdelbasset WK, Jadidi Kouhbanani MA, Varma RS, et al. Liposomes: Structure, Biomedical Applications, and Stability Parameters With Emphasis on Cholesterol. Front Bioeng Biotechnol (2021) 9. doi: 10.3389/fbioe.2021.705886
177. Sopyan I, Insan Sunan K, Gozali DA. Review: A Novel of Efforts to Enhance Liposome Stability as Drug Delivery Approach. Syst Rev Pharm (2020) 11:555–62. doi: 10.31838/srp.2020.6.85
178. Yoshizaki Y, Yuba E, Sakaguchi N, Koiwai K, Harada A, Kono K. pH-Sensitive Polymer-Modified Liposome-Based Immunity-Inducing System: Effects of Inclusion of Cationic Lipid and CpG-DNA. Biomaterials (2017) 141:272–83. doi: 10.1016/j.biomaterials.2017.07.001
179. Wang N, Chen M, Wang T. Liposomes Used as a Vaccine Adjuvant-Delivery System: From Basics to Clinical Immunization. J Control Release (2019) 303:130–50. doi: 10.1016/j.jconrel.2019.04.025
180. Bayyurt B, Tincer G, Almacioglu K, Alpdundar E, Gursel M, Gursel I. Encapsulation of Two Different TLR Ligands Into Liposomes Confer Protective Immunity and Prevent Tumor Development. J Control Release (2017) 247:134–44. doi: 10.1016/j.jconrel.2017.01.004
181. Altin JG, Van Broekhoven CL, Parish CR. Targeting Dendritic Cells With Antigen-Containing Liposomes: Antitumour Immunity. Expert Opin Biol Ther (2004) 4:1735–47. doi: 10.1517/14712598.4.11.1735
182. Ssemaganda A, Giddam AK, Zaman M, Skwarczynski M, Toth I, Stanisic DI, et al. Induction of Plasmodium-Specific Immune Responses Using Liposome-Based Vaccines. Front Immunol (2019) 10. doi: 10.3389/fimmu.2019.00135
183. Yuba E. Liposome-Based Immunity-Inducing Systems for Cancer Immunotherapy. Mol Immunol (2018) 98:8–12. doi: 10.1016/j.molimm.2017.11.001
184. Mady MM, Darwish MM. Effect of Chitosan Coating on the Characteristics of DPPC Liposomes. J Adv Res (2010) 1:187–91. doi: 10.1016/j.jare.2010.05.008
185. Lopez-Polo J, Silva-Weiss A, Zamorano M, Osorio FA. Humectability and Physical Properties of Hydroxypropyl Methylcellulose Coatings With Liposome-Cellulose Nanofibers: Food Application. Carbohydr Polym (2020) 231:1–10. doi: 10.1016/j.carbpol.2019.115702
186. Eroğlu İ., İbrahim M. Liposome–ligand Conjugates: A Review on the Current State of Art. J Drug Targeting (2020) 28:225–44. doi: 10.1080/1061186X.2019.1648479
187. Valh JV, Peršin Z, Vončina B, Vrezner K, Tušek L, Zemljič LF. Microencapsulation of Cannabidiol in Liposomes as Coating for Cellulose for Potential Advanced Sanitary Material. Coatings (2021) 11:1–18. doi: 10.3390/coatings11010003
188. Sebaaly C, Trifan A, Sieniawska E, Greige-Gerges H. Chitosan-Coating Effect on the Characteristics of Liposomes: A Focus on Bioactive Compounds and Essential Oils: A Review. Processes (2021) 9:1–47. doi: 10.3390/pr9030445
189. Joshi P, Becerra-Mora N, Vargas-Lizarazo AY, Kohli P, Fisher DJ, Choudhary R. Use of Edible Alginate and Limonene-Liposome Coatings for Shelf-Life Improvement of Blackberries. Futur Foods (2021) 4:1–9. doi: 10.1016/j.fufo.2021.100091
190. Bonechi C, Tamasi G, Donati A, Leone G, Consumi M, Cangeloni L, et al. Physicochemical Characterization of Hyaluronic Acid and Chitosan Liposome Coatings. Appl Sci (2021) 11:1–12. doi: 10.3390/app112412071
191. Lila ASA, Ishida T. Liposomal Delivery Systems: Design Optimization and Current Applications. Biol Pharm Bull (2017) 40:1–10. doi: 10.1248/bpb.b16-00624
192. Sharma G, Modgil A, Layek B, Arora K, Sun C, Law B, et al. Cell Penetrating Peptide Tethered Bi-Ligand Liposomes for Delivery to Brain In Vivo: Biodistribution and Transfection. J Control Release (2013) 167:1–10. doi: 10.1016/j.jconrel.2013.01.016
193. Ran R, Wang H, Liu Y, Hui Y, Sun Q, Seth A, et al. Microfluidic Self-Assembly of a Combinatorial Library of Single- and Dual-Ligand Liposomes for In Vitro and In Vivo Tumor Targeting. Eur J Pharm Biopharm (2018) 130:1–10. doi: 10.1016/j.ejpb.2018.06.017
194. Belfiore L, Saunders DN, Ranson M, Thurecht KJ, Storm G, Vine KL. Towards Clinical Translation of Ligand-Functionalized Liposomes in Targeted Cancer Therapy: Challenges and Opportunities. J Control Release (2018) 277:1–13. doi: 10.1016/j.jconrel.2018.02.040
195. Chopra H, Dey PS, Das D, Bhattacharya T, Shah M, Mubin S, et al. Curcumin Nanoparticles as Promising Therapeutic Agents for Drug Targets. Molecules (2021) 26(16):4998. doi: 10.3390/molecules26164998
196. Pugazhenthit S, Nesterova A, Sable C, Heidenreich KA, Boxer LM, Heasley LE, et al. Akt/protein Kinase B Up-Regulates Bcl-2 Expression Through cAMP-Response Element-Binding Protein. J Biol Chem (2000) 275:P1076–10766. doi: 10.1074/jbc.275.15.10761
197. Maehama T, Dixon JE. The Tumor Suppressor, PTEN/MMAC1, Dephosphorylates the Lipid Second Messenger, Phosphatidylinositol 3,4,5-Trisphosphate. J Biol Chem (1998) 273:13375–8. doi: 10.1074/jbc.273.22.13375
198. Yu S, Shen G, Tin OK, Kim JH, Kong ANT. Curcumin Inhibits Akt/mammalian Target of Rapamycin Signaling Through Protein Phosphatase-Dependent Mechanism. Mol Cancer Ther (2008) 7:2609–20. doi: 10.1158/1535-7163.MCT-07-2400
199. Dehm SM, Tindall DJ. Molecular Regulation of Androgen Action in Prostate Cancer. J Cell Biochem (2006) 99:333–44. doi: 10.1002/jcb.20794
200. Khor TO, Keum YS, Lin W, Kim JH, Hu R, Shen G, et al. Combined Inhibitory Effects of Curcumin and Phenethyl Isothiocyanate on the Growth of Human PC-3 Prostate Xenografts in Immunodeficient Mice. Cancer Res (2006) 66:613–21. doi: 10.1158/0008-5472.CAN-05-2708
201. Non L, Duong D, Peehl DM. Chemopreventive Anti-Inflammatory Activities of Curcumin and Other Phytochemicals Mediated by MAP Kinase Phosphatase-5 in Prostate Cells. Carcinogenesis (2007) 28:1188–96. doi: 10.1093/carcin/bgl241
202. Hong JH, Ahn KS, Bae E, Jeon SS, Choi HY. The Effects of Curcumin on the Invasiveness of Prostate Cancer In Vitro and In Vivo. Prostate Cancer Prostatic Dis (2006) 9:147–52. doi: 10.1038/sj.pcan.4500856
203. Fujita K, Nonomura N. Role of Androgen Receptor in Prostate Cancer: A Review. World J Men?s Heal (2019) 37:288–95. doi: 10.5534/wjmh.180040
204. Nakamura K, Yasunaga Y, Segawa T, Ko D, Moul JW, Srivastava S, et al. Curcumin Down-Regulates AR Gene Expression and Activation in Prostate Cancer Cell Lines. Int J Oncol (2002) 21:825–30. doi: 10.3892/ijo.21.4.825
205. Reuhl KR, Lou YR, Ma W, Newmark HL, Conney AH. Inhibitory Effects of Dietary Curcumin on Forestomach, Duodenal, and Colon Carcinogenesis in Mice. Cancer Res (1994) 54:5841–7.
206. McNally SJ, Harrison EM, Ross JA, Garden OJ, Wigmore SJ. Curcumin Induces Heme Oxygenase 1 Through Generation of Reactive Oxygen Species, P38 Activation and Phosphatase Inhibition. Int J Mol Med (2007) 19:165–72. doi: 10.3892/ijmm.19.1.165
207. Yan C, Jamaluddin MS, Aggarwal B, Myers J, Boyd DD. Gene Expression Profiling Identifies Activating Transcription Factor 3 as a Novel Contributor to the Proapoptotic Effect of Curcumin. Mol Cancer Ther (2005) 4:233–41.
208. Hasima N, Aggarwal BB. Cancer-Linked Targets Modulated by Curcumin. Int J Biochem Mol Biol (2012) 3:328–51.
209. Phan V, Walters J, Brownlow B, Elbayoumi T. Enhanced Cytotoxicity of Optimized Liposomal Genistein via Specific Induction of Apoptosis in Breast, Ovarian and Prostate Carcinomas. J Drug Targeting (2013) 21:1001–11. doi: 10.3109/1061186X.2013.847099
210. Biswas P, Hasan MM, Dey D, dos Santos Costa AC, Polash SA, Bibi S, et al. Candidate Antiviral Drugs for COVID-19 and Their Environmental Implications: A Comprehensive Analysis. Environ Sci pollut Res (2021) 1–24:59570–93. doi: 10.1007/s11356-021-16096-3
211. Friesner RA, Banks JL, Murphy RB, Halgren TA, Klicic JJ, Mainz DT, et al. Glide: A New Approach for Rapid, Accurate Docking and Scoring. 1. Method and Assessment of Docking Accuracy. J Med Chem (2004) 47:1739–49. doi: 10.1021/jm0306430
212. Barron CC, Bilan PJ, Tsakiridis T, Tsiani E. Facilitative Glucose Transporters: Implications for Cancer Detection, Prognosis and Treatment. Metabolism (2016) 65:124–39. doi: 10.1016/j.metabol.2015.10.007
213. Cairns RA, Harris IS, Mak TW. Regulation of Cancer Cell Metabolism. Nat Rev Cancer (2011) 11:85–95. doi: 10.1038/nrc2981
214. Li S, Li J, Dai W, Zhang Q, Feng J, Wu L, et al. Genistein Suppresses Aerobic Glycolysis and Induces Hepatocellular Carcinoma Cell Death. Br J Cancer (2017) 117:1518–23. doi: 10.1038/bjc.2017.323
215. Chandler JD, Williams ED, Slavin JL, Best JD, Rogers S. Expression and Localization of GLUT1 and GLUT12 in Prostate Carcinoma. Cancer (2003) 97:2035–42. doi: 10.1002/cncr.11293
216. Reinicke K, Sotomayor P, Cisterna P, Delgado C, Nualart F, Godoy A. Cellular Distribution of Glut-1 and Glut-5 in Benign and Malignant Human Prostate Tissue. J Cell Biochem (2012) 113:553–62. doi: 10.1002/jcb.23379
217. Zhang S, Wang Y, Chen Z, Kim S, Iqbal S, Chi A, et al. Genistein Enhances the Efficacy of Cabazitaxel Chemotherapy in Metastatic Castration-Resistant Prostate Cancer Cells. Prostate (2013) 73:1681–9. doi: 10.1002/pros.22705
218. Tian Jy, Chi Cl, Bian G, Xing D, Guo Fj, Wang Xq. PSMA Conjugated Combinatorial Liposomal Formulation Encapsulating Genistein and Plumbagin to Induce Apoptosis in Prostate Cancer Cells. Colloids Surf B Biointerfaces (2021) 203:111723. doi: 10.1016/J.COLSURFB.2021.111723
219. Benavente-García O, Castillo J, Lorente J, Ortuño A, Del Rio JA. Antioxidant Activity of Phenolics Extracted From Olea Europaea L. Leaves. Food Chem (2000) 68:457–62. doi: 10.1016/S0308-8146(99)00221-6
220. de Bock M, Thorstensen EB, Derraik JGB, Henderson HV, Hofman PL, Cutfield WS. Human Absorption and Metabolism of Oleuropein and Hydroxytyrosol Ingested as Olive (Olea Europaea L.) Leaf Extract. Mol Nutr Food Res (2013) 57:2079–85. doi: 10.1002/mnfr.201200795
221. Nassir AM, Ibrahim IAA, Md S, Waris M. Surface Functionalized Folate Targeted Oleuropein Nano-Liposomes for Prostate Tumor Targeting: In Vitro and In Vivo Activity. Life Sci (2019) 220:136–46. doi: 10.1016/J.LFS.2019.01.053
222. Rodzinski A, Guduru R, Liang P, Hadjikhani A, Stewart T, Stimphil E, et al. Targeted and Controlled Anticancer Drug Delivery and Release With Magnetoelectric Nanoparticles. Sci Rep (2016) 6:20867. doi: 10.1038/srep20867
223. Chen B, Le W, Wang Y, Li Z, Wang D, Ren L, et al. Targeting Negative Surface Charges of Cancer Cells by Multifunctional Nanoprobes. Theranostics (2016) 6:1887–1898. doi: 10.7150/thno.16358
224. Needham D, McIntosh TJ, Lasic DD. Repulsive Interactions and Mechanical Stability of Polymer-Grafted Lipid Membranes. BBA - Biomembr (1992) 1108:40–8. doi: 10.1016/0005-2736(92)90112-Y
225. Brown NS, Bicknell R. Hypoxia and Oxidative Stress in Breast Cancer: Oxidative Stress: Its Effects on the Growth, Metastatic Potential and Response to Therapy of Breast Cancer. Breast Cancer Res (2001) 3:323–327. doi: 10.1186/BCR315
226. Tang FY, Shih CJ, Cheng LH, Ho HJ, Chen HJ. Lycopene Inhibits Growth of Human Colon Cancer Cells via Suppression of the Akt Signaling Pathway. Mol Nutr Food Res (2008) 52:646–54. doi: 10.1002/mnfr.200700272
227. Seçme M, Eroğlu C, Dodurga Y, Bağci G. Investigation of Anticancer Mechanism of Oleuropein via Cell Cycle and Apoptotic Pathways in SH-SY5Y Neuroblastoma Cells. Gene (2016) 585:93–9. doi: 10.1016/j.gene.2016.03.038
228. Zhou S, Li J, Yu J, Wang Y, Liu H, Lin G, et al. Unique Flower-Like Cur-Metal Complexes Loaded Liposomes for Primary and Metastatic Breast Cancer Therapy. Mater Sci Eng. C (2021) 121:111835. doi: 10.1016/j.msec.2020.111835
229. Englinger B, Pirker C, Heffeter P, Terenzi A, Kowol CR, Keppler BK, et al. Metal Drugs and the Anticancer Immune Response. Chem Rev (2018) 119:1519–624. doi: 10.1021/ACS.CHEMREV.8B00396
230. Mahmood K, Zia KM, Zuber M, Salman M, Anjum MN. Recent Developments in Curcumin and Curcumin Based Polymeric Materials for Biomedical Applications: A Review. Int J Biol Macromol (2015) 81:877–90. doi: 10.1016/J.IJBIOMAC.2015.09.026
231. Wu R, Mei X, Ye Y, Xue T, Wang J, Sun W, et al. Zn(II)-Curcumin Solid Dispersion Impairs Hepatocellular Carcinoma Growth and Enhances Chemotherapy by Modulating Gut Microbiota-Mediated Zinc Homeostasis. Pharmacol Res (2019) 150:104454. doi: 10.1016/J.PHRS.2019.104454
232. Tang WL, Tang WH, Szeitz A, Kulkarni J, Cullis P, Li SD. Systemic Study of Solvent-Assisted Active Loading of Gambogic Acid Into Liposomes and its Formulation Optimization for Improved Delivery. Biomaterials (2018) 166:13–26. doi: 10.1016/J.BIOMATERIALS.2018.03.004
233. Dos Santos N, Cox KA, McKenzie CA, Van Baarda F, Gallagher RC, Karlsson G, et al. pH Gradient Loading of Anthracyclines Into Cholesterol-Free Liposomes: Enhancing Drug Loading Rates Through Use of Ethanol. Biochim Biophys Acta - Biomembr (2004) 1661:47–60. doi: 10.1016/J.BBAMEM.2003.11.016
234. Marzano C, Pellei M, Tisato F, Santini C. Copper Complexes as Anticancer Agents. Anticancer Agents Med Chem (2012) 9:185–211. doi: 10.2174/187152009787313837
236. Santini C, Pellei M, Gandin V, Porchia M, Tisato F, Marzano C. Advances in Copper Complexes as Anticancer Agents. Chem Rev (2013) 114:815–62. doi: 10.1021/CR400135X
237. Li J, Zhou S, Yu J, Cai W, Yang Y, Kuang X, et al. Low Dose Shikonin and Anthracyclines Coloaded Liposomes Induce Robust Immunogenetic Cell Death for Synergistic Chemo-Immunotherapy. J Control Release (2021) 335:306–19. doi: 10.1016/J.JCONREL.2021.05.040
238. Yang W, Yang Z, Fu J, Guo M, Sun B, Wei W, et al. The Influence of Trapping Agents on the Antitumor Efficacy of Irinotecan Liposomes: Head-To-Head Comparison of Ammonium Sulfate, Sulfobutylether-β-Cyclodextrin and Sucrose Octasulfate. Biomater Sci (2019) 7(1):419–28. doi: 10.1039/c8bm01175c
239. Iyer R, Croucher JL, Chorny M, Mangino JL, Alferiev IS, Levy RJ, et al. Nanoparticle Delivery of an SN38 Conjugate is More Effective Than Irinotecan in a Mouse Model of Neuroblastoma. Cancer Lett (2015) 360:205–12. doi: 10.1016/j.canlet.2015.02.011
240. Hadinoto K, Sundaresan A, Cheow WS. Lipid-Polymer Hybrid Nanoparticles as a New Generation Therapeutic Delivery Platform: A Review. Eur J Pharm Biopharm (2013) 85:427–43. doi: 10.1016/j.ejpb.2013.07.002
241. Ma P, Li T, Xing H, Wang S, Sun Y, Sheng X, et al. Local Anesthetic Effects of Bupivacaine Loaded Lipid-Polymer Hybrid Nanoparticles. In Vitro Vivo evaluation Biomed Pharmacother (2017) 89:689–95. doi: 10.1016/j.biopha.2017.01.175
242. Wang Q, Alshaker H, Böhler T, Srivats S, Chao Y, Cooper C, et al. Core Shell Lipid-Polymer Hybrid Nanoparticles With Combined Docetaxel and Molecular Targeted Therapy for the Treatment of Metastatic Prostate Cancer. Sci Rep (2017) 7:5901. doi: 10.1038/s41598-017-06142-x
243. Powell D, Chandra S, Dodson K, Shaheen F, Wiltz K, Ireland S, et al. Aptamer-Functionalized Hybrid Nanoparticle for the Treatment of Breast Cancer. Eur J Pharm Biopharm (2017) 114:108–18. doi: 10.1016/j.ejpb.2017.01.011
244. Perepelyuk M, Maher C, Lakshmikuttyamma A, Shoyele SA. Aptamer-Hybrid Nanoparticle Bioconjugate Efficiently Delivers miRNA-29b to non-Small-Cell Lung Cancer Cells and Inhibits Growth by Downregulating Essential Oncoproteins. Int J Nanomedicine (2016) 11:3533–44. doi: 10.2147/IJN.S110488
245. Xu G, Chen Y, Shan R, Wu X, Chen L. Transferrin and Tocopheryl-Polyethylene Glycol-Succinate Dual Ligands Decorated, Cisplatin Loaded Nano-Sized System for the Treatment of Lung Cancer. Biomed Pharmacother (2018) 99:354–62. doi: 10.1016/j.biopha.2018.01.062
246. Zhang R, Ru Y, Gao Y, Li J, Mao S. Layer-By-Layer Nanoparticles Co-Loading Gemcitabine and Platinum (IV) Prodrugs for Synergistic Combination Therapy of Lung Cancer. Drug Des Devel Ther (2017) 11:2631–42. doi: 10.2147/DDDT.S143047
247. Zheng G, Zheng M, Yang B, Fu H, Li Y. Improving Breast Cancer Therapy Using Doxorubicin Loaded Solid Lipid Nanoparticles: Synthesis of a Novel Arginine-Glycine-Aspartic Tripeptide Conjugated, pH Sensitive Lipid and Evaluation of the Nanomedicine. Vitro vivo Biomed Pharmacother (2019) 116:109006. doi: 10.1016/j.biopha.2019.109006
248. Cui T, Sihao Z, Sun H. Co-Delivery of Doxorubicin and pH-Sensitive Curcumin Prodrug by Transferrin-Targeted Nanoparticles for Breast Cancer Treatment. Oncol Rep (2017) 37:1253–60. doi: 10.3892/or.2017.5345
249. Xu L, He XY, Liu BY, Xu C, Ai SL, Zhuo RX, et al. Aptamer-Functionalized Albumin-Based Nanoparticles for Targeted Drug Delivery. Colloids Surfaces B Biointerfaces (2018) 171:24–30. doi: 10.1016/j.colsurfb.2018.07.008
250. Tan BL, Norhaizan ME. Curcumin Combination Chemotherapy: The Implication and Efficacy in Cancer. Molecules (2019) 24:2527. doi: 10.3390/molecules24142527
251. Mellado B, Jimenez N, Marin-Aguilera M, Reig O. Diving Into Cabazitaxel’s Mode of Action: More Than a Taxane for the Treatment of Castration-Resistant Prostate Cancer Patients. Clin Genitourin Cancer (2016) 14:265–70. doi: 10.1016/j.clgc.2015.12.030
252. Duan T, Xu Z, Sun F, Wang Y, Zhang J, Luo C, et al. HPA Aptamer Functionalized Paclitaxel-Loaded PLGA Nanoparticles for Enhanced Anticancer Therapy Through Targeted Effects and Microenvironment Modulation. Biomed Pharmacother (2019) 117:109121. doi: 10.1016/j.biopha.2019.109121
253. Zhang T, Ma J, Li C, Lin K, Lou F, Jiang H, et al. Core-Shell Lipid Polymer Nanoparticles for Combined Chemo and Gene Therapy of Childhood Head and Neck Cancers. Oncol Rep (2017) 37:1653–61. doi: 10.3892/or.2017.5365
254. Xiong Y, Zhao Y, Miao L, Lin CM, Huang L. Co-Delivery of Polymeric Metformin and Cisplatin by Self-Assembled Core-Membrane Nanoparticles to Treat non-Small Cell Lung Cancer. J Control Release (2016) 244:63–73. doi: 10.1016/j.jconrel.2016.11.005
255. Thipe VC, Amiri KP, Bloebaum P, Karikachery AR, Khoobchandani M, Katti KK, et al. Development of Resveratrol-Conjugated Gold Nanoparticles: Interrelationship of Increased Resveratrol Corona on Anti-Tumor Efficacy Against Breast, Pancreatic and Prostate Cancers. Int J Nanomedicine (2019) 14:4413–4428. doi: 10.2147/IJN.S204443
256. Borowski E, Bontemps-Gracz MM, Piwkowska A. Strategies for Overcoming ABC-Transporters-Mediated Multidrug Resistance (MDR) of Tumor Cells Acta Biochim Pol (2005) 52(3):609–27.
257. Bhardwaj RK, Glaeser H, Becquemont L, Klotz U, Gupta SK, Fromm MF. Piperine, a Major Constituent of Black Pepper, Inhibits Human P-Glycoprotein and CYP3A4. J Pharmacol Exp Ther (2002) 302:645–50. doi: 10.1124/jpet.102.034728
258. Nabekura T, Kamiyama S, Kitagawa S. Effects of Dietary Chemopreventive Phytochemicals on P-Glycoprotein Function. Biochem Biophys Res Commun (2005) 327:866–70. doi: 10.1016/J.BBRC.2004.12.081
259. Roger E, Kalscheuer S, Kirtane A, Guru BR, Grill AE, Whittum-Hudson J, et al. Folic Acid Functionalized Nanoparticles for Enhanced Oral Drug Delivery. Mol Pharm (2012) 9:2103–10. doi: 10.1021/MP2005388
260. Dorai T, Cao YC, Dorai B, Buttyan R, Katz AE. Therapeutic Potential of Curcumin in Human Prostate Cancer. III. Curcumin Inhibits Proliferation, Induces Apoptosis, and Inhibits Angiogenesis of LNCaP Prostate Cancer Cells In Vivo. Prostate (2001) 47:293–303. doi: 10.1002/PROS.1074
261. Yallapu MM, Khan S, Maher DM, Ebeling MC, Sundram V, Chauhan N, et al. Anti-Cancer Activity of Curcumin Loaded Nanoparticles in Prostate Cancer. Biomaterials (2014) 35:8635–48. doi: 10.1016/j.biomaterials.2014.06.040
262. Mukerjee A, Vishwanatha JK. Formulation, Characterization and Evaluation of Curcumin-Loaded PLGA Nanospheres for Cancer Therapy. Anticancer Res (2009) 29:3867–75.
263. Aoki H, Takada Y, Kondo S, Sawaya R, Aggarwal BB, Kondo Y. Evidence That Curcumin Suppresses the Growth of Malignant Gliomas In Vitro and In Vivo Through Induction of Autophagy: Role of Akt and Extracellular Signal-Regulated Kinase Signaling Pathways. Mol Pharmacol (2007) 72:29–39. doi: 10.1124/mol.106.033167
264. Mohan Yallapu M, Ray Dobberpuhl M, Michele Maher D, Jaggi M, Chand Chauhan S. Design of Curcumin Loaded Cellulose Nanoparticles for Prostate Cancer. Curr Drug Metab (2011) 13:120–8. doi: 10.2174/138920012798356952
265. Algeciras-Schimnich A, Barnhart BC, Peter ME. Apoptosis Dependent and Independent Functions of Caspases. In: Madame Curie Bioscience Database [Internet]. Austin (TX): Landes Bioscience; (2013)2000–13. Available at: https://www.ncbi.nlm.nih.gov/books/NBK6198
266. Wang G, Wang J, Sadar MD. Crosstalk Between the Androgen Receptor and β-Catenin in Castrate Resistant Prostate Cancer. Cancer Res (2008) 68:9918–9927. doi: 10.1158/0008-5472.CAN-08-1718
267. Mak P, Jaggi M, Syed V, Chauhan SC, Hassan S, Biswas H, et al. Protein Kinase D1 (PKD1) Influences Androgen Receptor (AR) Function in Prostate Cancer Cells. Biochem Biophys Res Commun (2008) 373(4):618–23. doi: 10.1016/j.bbrc.2008.06.097
268. Jaggi M, Chauhan SC, Du C, Balaji KC. Bryostatin 1 Modulates Beta-Catenin Subcellular Localization and Transcription Activity Through Protein Kinase D1 Activation. Mol Cancer Ther (2008) 7:2703–12. doi: 10.1158/1535-7163.MCT-08-0119
269. Azandeh SS, Abbaspour M, Khodadadi A, Khorsandi L, Orazizadeh M, Heidari-Moghadam A. Anticancer Activity of Curcumin-Loaded PLGA Nanoparticles on PC3 Prostate Cancer Cells. Iran J Pharm Res (2017) 16:868–79. doi: 10.22037/ijpr.2017.2097
270. Anitha A, Maya S, Deepa N, Chennazhi KP, Nair SV, Tamura H, et al. Efficient Water Soluble O-Carboxymethyl Chitosan Nanocarrier for the Delivery of Curcumin to Cancer Cells. Carbohydr Polym (2011) 83:452–61. doi: 10.1016/J.CARBPOL.2010.08.008
271. Vodnik VV, Mojić M, Stamenović U, Otoničar M, Ajdžanović V, Maksimović-Ivanić D, et al. Development of Genistein-Loaded Gold Nanoparticles and Their Antitumor Potential Against Prostate Cancer Cell Lines. Mater Sci Eng C (2021) 124:112078. doi: 10.1016/J.MSEC.2021.112078
272. Li YJ, Lei YH, Yao N, Wang CR, Hu N, Ye WC, et al. Autophagy and Multidrug Resistance in Cancer. Chin J Cancer (2017) 36(52)1–10. doi: 10.1186/s40880-017-0219-2
273. Tuli HS, Tuorkey MJ, Thakral F, Sak K, Kumar M, Sharma AK, et al. Molecular Mechanisms of Action of Genistein in Cancer: Recent Advances. Front Pharmacol (2019) 10:1336. doi: 10.3389/fphar.2019.01336
274. Ouchi H, Ishiguro H, Ikeda N, Hori M, Kubota Y, Uemura H. Genistein Induces Cell Growth Inhibition in Prostate Cancer Through the Suppression of Telomerase Activity. Int J Urol (2005) 12:73–80. doi: 10.1111/j.1442-2042.2004.00973.x
275. Li C. Poly(L-Glutamic Acid)-Anticancer Drug Conjugates. Adv Drug Deliv Rev (2002) 54:963–86. doi: 10.1016/S0169-409X(02)00045-5
276. Hadi MM, Nesbitt H, Masood H, Sciscione F, Patel S, Ramesh BS, et al. Investigating the Performance of a Novel pH and Cathepsin B Sensitive, Stimulus-Responsive Nanoparticle for Optimised Sonodynamic Therapy in Prostate Cancer. J Control Release (2021) 329:76–86. doi: 10.1016/J.JCONREL.2020.11.040
277. Heldin CH, Rubin K, Pietras K, Östman A. High Interstitial Fluid Pressure - An Obstacle in Cancer Therapy. Nat Rev Cancer (2004) 4:806–13. doi: 10.1038/nrc1456
278. Hirschberg H, Madsen SJ. Synergistic Efficacy of Ultrasound, Sonosensitizers and Chemotherapy: A Review. Ther Deliv (2017) 8:331–42. doi: 10.4155/tde-2016-0080
279. Xiaofei C, Yanqing L, Dongkai Z, Dong C, Feng Z, Weilin W. Identification of Cathepsin B as a Novel Target of Hypoxia-Inducible Factor-1-Alpha in HepG2 Cells. Biochem Biophys Res Commun (2018) 503:1057–62. doi: 10.1016/j.bbrc.2018.06.116
280. Zhao J, Liu J, Wei T, Ma X, Cheng Q, Huo S, et al. Quercetin-Loaded Nanomicelles to Circumvent Human Castration-Resistant Prostate Cancer. Vitro vivo Nanoscale (2016) 8:5126–38. doi: 10.1039/c5nr08966b
281. Nagesh PKB, Chowdhury P, Hatami E, Kumari S, Kashyap VK, Tripathi MK, et al. Cross-Linked Polyphenol-Based Drug Nano-Self-Assemblies Engineered to Blockade Prostate Cancer Senescence. ACS Appl Mater Interfaces (2019) 11:38537–54. doi: 10.1021/ACSAMI.9B14738/SUPPL_FILE/AM9B14738_SI_001.PDF
282. Radhakrishnan R, Kulhari H, Pooja D, Gudem S, Bhargava S, Shukla R, et al. Encapsulation of Biophenolic Phytochemical EGCG Within Lipid Nanoparticles Enhances its Stability and Cytotoxicity Against Cancer. Chem Phys Lipids (2016) 198:51–60. doi: 10.1016/J.CHEMPHYSLIP.2016.05.006
283. Khan N, Bharali DJ, Adhami VM, Siddiqui IA, Cui H, Shabana SM, et al. Oral Administration of Naturally Occurring Chitosan-Based Nanoformulated Green Tea Polyphenol EGCG Effectively Inhibits Prostate Cancer Cell Growth in a Xenograft Model. Carcinogenesis (2014) 35:415–23. doi: 10.1093/CARCIN/BGT321
284. Blanco E, Bey EA, Dong Y, Weinberg BD, Sutton DM, Boothman DA, et al. β-Lapachone-Containing PEG-PLA Polymer Micelles as Novel Nanotherapeutics Against NQO1-Overexpressing Tumor Cells. J Control Release (2007) 122:365–374. doi: 10.1016/J.JCONREL.2007.04.014
285. Donia DT, Carbone M. Fate of the Nanoparticles in Environmental Cycles. Int J Environ Sci Technol (2019) 16:583–600. doi: 10.1007/s13762-018-1960-z
286. Gnach A, Lipinski T, Bednarkiewicz A, Rybka J, Capobianco JA. Upconverting Nanoparticles: Assessing the Toxicity. Chem Soc Rev (2015) 44:1561–84. doi: 10.1039/C4CS00177J
287. Sukhanova A, Bozrova S, Sokolov P, Berestovoy M, Karaulov A, Nabiev I. Dependence of Nanoparticle Toxicity on Their Physical and Chemical Properties. Nanoscale Res Lett (2018) 13:1–21. doi: 10.1186/s11671-018-2457-x
288. Guerrini L, Alvarez-Puebla RA, Pazos-Perez N. Surface Modifications of Nanoparticles for Stability in Biological Fluids. Materials (Basel) (2018) 11:1154. doi: 10.3390/ma11071154
289. Navya PN, Daima HK. Rational Engineering of Physicochemical Properties of Nanomaterials for Biomedical Applications With Nanotoxicological Perspectives. Nano Converg (2016) 3:1–14. doi: 10.1186/s40580-016-0064-z
290. Gatoo MA, Naseem S, Arfat MY, Mahmood Dar A, Qasim K, Zubair S. Physicochemical Properties of Nanomaterials: Implication in Associated Toxic Manifestations. BioMed Res Int (2014) 2014:498420. doi: 10.1155/2014/498420
291. Ali A, Zafar H, Zia M, ul Haq I, Phull AR, Ali JS, et al. Synthesis, Characterization, Applications, and Challenges of Iron Oxide Nanoparticles. Nanotechnol Sci Appl (2016) 9:49–67. doi: 10.2147/NSA.S99986
292. Firme CP, Bandaru PR. Toxicity Issues in the Application of Carbon Nanotubes to Biological Systems. Nanomedicine Nanotechnology Biol Med (2010) 6:245–56. doi: 10.1016/j.nano.2009.07.003
293. Chalupa DC, Morrow PE, Oberdörster G, Utell MJ, Frampton MW. Ultrafine Particle Deposition in Subjects With Asthma. Environ Health Perspect (2004) 112:879–82. doi: 10.1289/ehp.6851
294. Hou WC, Westerhoff P, Posner JD. Biological Accumulation of Engineered Nanomaterials: A Review of Current Knowledge. Environ Sci Process Impacts (2013) 15:103–22. doi: 10.1039/C2EM30686G
295. Yin H, Too HP, Chow GM. The Effects of Particle Size and Surface Coating on the Cytotoxicity of Nickel Ferrite. Biomaterials (2005) 26:5818–26. doi: 10.1016/j.biomaterials.2005.02.036
296. Oomen AG, Steinhäuser KG, Bleeker EAJ, van Broekhuizen F, Sips A, Dekkers S, et al. Risk Assessment Frameworks for Nanomaterials: Scope, Link to Regulations, Applicability, and Outline for Future Directions in View of Needed Increase in Efficiency. NanoImpact (2018) 9:1–13. doi: 10.1016/j.impact.2017.09.001
Keywords: cancer, chemotherapy, prostate cancer, nutraceutical, supplements, nanotechnology, nutrition
Citation: Chopra H, Bibi S, Goyal R, Gautam RK, Trivedi R, Upadhyay TK, Mujahid MH, Shah MA, Haris M, Khot KB, Gopan G, Singh I, Kim JK, Jose J, Abdel-Daim MM, Alhumaydhi FA, Emran TB and Kim B (2022) Chemopreventive Potential of Dietary Nanonutraceuticals for Prostate Cancer: An Extensive Review. Front. Oncol. 12:925379. doi: 10.3389/fonc.2022.925379
Received: 21 April 2022; Accepted: 25 May 2022;
Published: 12 July 2022.
Edited by:
Saroj Arora, Guru Nanak Dev University, IndiaReviewed by:
Bharat B. Aggarwal, University of Texas MD Anderson Cancer Center, United StatesMonia Lenzi, University of Bologna, Italy
Copyright © 2022 Chopra, Bibi, Goyal, Gautam, Trivedi, Upadhyay, Mujahid, Shah, Haris, Khot, Gopan, Singh, Kim, Jose, Abdel-Daim, Alhumaydhi, Emran and Kim. This is an open-access article distributed under the terms of the Creative Commons Attribution License (CC BY). The use, distribution or reproduction in other forums is permitted, provided the original author(s) and the copyright owner(s) are credited and that the original publication in this journal is cited, in accordance with accepted academic practice. No use, distribution or reproduction is permitted which does not comply with these terms.
*Correspondence: Bonglee Kim, Ym9uZ2xlZWtpbUBraHUuYWMua3I=; Jobin Jose, amptYXR0YW0wN0BnbWFpbC5jb20=; Talha Bin Emran, dGFsaGFibWJAYmdjdHViLmFjLmJk; Shabana Bibi, ZGF6emxpbmcuZ3JpbkBnbWFpbC5jb20=