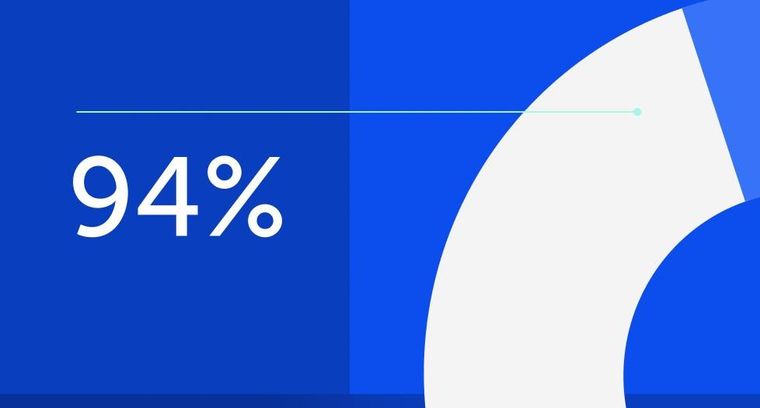
94% of researchers rate our articles as excellent or good
Learn more about the work of our research integrity team to safeguard the quality of each article we publish.
Find out more
REVIEW article
Front. Oncol., 02 September 2022
Sec. Molecular and Cellular Oncology
Volume 12 - 2022 | https://doi.org/10.3389/fonc.2022.924553
This article is part of the Research TopicWomen in Molecular and Cellular Oncology, volume II: 2022View all 15 articles
The extracellular matrix (ECM) is critical for maintaining tissue homeostasis therefore its production, assembly and mechanical stiffness are highly regulated in normal tissues. However, in solid tumors, increased stiffness resulting from abnormal ECM structural changes is associated with disease progression, an increased risk of metastasis and poor survival. As a dynamic and key component of the tumor microenvironment, the ECM is becoming increasingly recognized as an important feature of tumors, as it has been shown to promote several hallmarks of cancer via biochemical and biomechanical signaling. In this regard, melanoma cells are highly sensitive to ECM composition, stiffness and fiber alignment because they interact directly with the ECM in the tumor microenvironment via cell surface receptors, secreted factors or enzymes. Importantly, seeing as the ECM is predominantly deposited and remodeled by myofibroblastic stromal fibroblasts, it is a key avenue facilitating their paracrine interactions with melanoma cells. This review gives an overview of melanoma and further describes the critical roles that ECM properties such as ECM remodeling, ECM-related proteins and stiffness play in cutaneous melanoma progression, tumor cell plasticity and therapeutic resistance. Finally, given the emerging importance of ECM dynamics in melanoma, future perspectives on therapeutic strategies to normalize the ECM in tumors are discussed.
Early-stage cutaneous melanoma (clinical stage I – II) that has not presented with nodal or distant metastasis is effectively treated with surgery (1). However, late-stage cutaneous melanoma (clinical stages III and IV) can present with metastases in the lymph nodes, microsatellite and in-transit metastatic lesions (stage III) or it can disseminate to distant organs (stage IV) (1). It is therefore concerning that approximately 60% of melanoma patients present with metastatic lesions at the lymph nodes and 15% of patients experience metastases at distant organs including the lungs, liver, brain or bone (2, 3). Advanced melanoma is currently treated with chemo- and more recently, with immuno- and targeted therapies, however, it is often recalcitrant to these treatment regimens (2). Prognosis is poor for patients with advanced melanoma, as approximately 50-60% of these patients acquire drug resistance and go into therapeutic failure (3, 4). A possible reason for this, is that melanomas are highly heterogeneous within single tumors but also amongst different tumors at metastatic sites (5). This heterogeneity is in part caused by non-genetic mechanisms, which can drive melanoma cells to readily and reversibly switch between different phenotypic states (6, 7). The tumor microenvironment (TME) critically affects melanoma progression and tumor cell states at both the primary and secondary sites and has been shown to contribute to therapeutic resistance (8, 9).
The TME, which has been recognized as a hallmark of cancer, is a complex and evolving milieu that consists not only of tumor cells but also consists of non-malignant cell types, including fibroblasts, immune cells, nerve cells and endothelial cells, as well as the extracellular matrix (ECM) (10). Thus, the crosstalk between melanoma cells and their cellular and non-cellular surroundings plays a key role in disease progression and impacts the efficacy of anti-cancer therapies (6). The ECM is an important feature of the melanoma TME because ECM-derived biochemical and biomechanical cues have been shown to influence melanoma progression, tumor cell plasticity and therapeutic resistance (11, 12). The ECM is rich in proteins that form a 3D-meshwork of varying alignment and stiffness. Importantly, in cancers including melanoma, the ECM is drastically remodeled resembling fibrosis, especially during invasion and in response to therapies (12–15). This review begins with an overview of melanoma, including disease progression, current therapies and phenotypic plasticity, followed by a description of how ECM architecture is modified in the melanoma TME. Finally, we discuss the role of ECM signaling in melanoma progression, phenotypic plasticity and therapeutic resistance and give future perspectives on potential therapeutic strategies to normalize the melanoma TME.
Cutaneous melanoma arises from neural-crest derived pigment producing melanocytes in the epidermis that have undergone genetic alterations, leading to aberrant growth and clonal expansion (16). While some melanomas arise from pre-existing naevi, the majority of melanomas arise de novo (17). The Clark model of cutaneous melanoma progression is a classical and well accepted model based on histopathological analyses of melanoma tissue samples at various stages of progression (18). This model begins with aggregated melanocytes forming a benign nevus in the epidermis that experience abnormal growth to form a dysplastic nevus, which can progress, upon clonal expansion, to the radial growth phase (RGP), where the melanoma cells are confined to the epidermis (18). Should the melanoma cells proliferate further and breach the basement membrane, they progress to the vertical growth phase (VGP), as the melanoma cells are able to invade the dermis (18). At the molecular level, melanocytic cells in the benign nevus, frequently have oncogenic mutations in the BRAF or NRAS genes. Furthermore, these lesions undergo dysplasia due to the loss of tumor suppressive signaling and the loss of expression of key cell cycle regulators. For example, the progression from a dysplastic nevus to the RGP occurs as a result of unchecked mutations and epigenetic changes in cell cycle and cell survival regulators including PTEN, NF1, CDKN2A, and CCND1 that allow the melanoma cells to bypass senescence (18–20). Additionally, approximately 15% of melanomas occur due to a familial genetic predisposition, resulting in germline mutations in CDKN2A (most common) CDK4, TERT, ACD, TERF2IP, POT1, MITF, MC1R, and BAP1 (21). The transition from the RGP to the VGP is characterized by an upregulation of mesenchymal markers such as N-cadherin, secreted protein acidic and cysteine rich (SPARC) and β-catenin, as well as the downregulation of the cell-cell adhesion molecule E-cadherin (18, 22, 23). Once the melanoma cells have invaded the dermis they can disseminate via lymphatic or blood vessels and metastasize to distant organs, which is considered the metastatic phase (18).
Although the Clark model has often been used as a reference for melanoma progression in studies investigating the molecular mechanisms that are driving melanoma progression, it is not routinely used for clinical melanoma diagnosis and classification. The first melanoma classification used by clinicians is the Breslow index, which measures the thickness of the tumor because a direct correlation exists between the depth of the melanoma in the skin and patient survival (24). Furthermore, the American Joint Committee on Cancer (AJCC) has recommended the use of the TNM (Tumor, Node, Metastasis) classification (stages 0 – IV), which grades the melanoma according to the thickness, the presence of ulceration in biopsies and any evidence of metastatic dissemination or lesions (25). More recently following the analysis of RNA, DNA and protein in 333 primary or metastatic samples from 331 melanoma patients, The Cancer Genome Atlas Network has proposed the classification of melanoma according to genetic mutations into 4 categories: BRAF, NRAS, NF-1 and triple negative (wild type) (20). The data indicated that mutations in BRAF are prevalent in 50% of melanoma patient samples, followed by NRAS (30%) and NF1 (14%) and 15% of melanomas did not harbor somatic mutations in BRAF, NRAS or NF1 (20).
Current FDA approved therapies for advanced melanoma include chemo-, immuno- and targeted therapies (2). The most commonly used chemotherapeutic drug used for melanoma treatment is Dacarbazine, which was initially approved for melanoma in the 1970s (26). However, as a monotherapy it was shown to have minimal efficacy in treating advanced melanoma, with the average response rate in patients being less than 5% and the 5 year survival of patients was suggested to be less than 6% (26–28). Furthermore, the combination of Dacarbazine treatment with earlier immunotherapies such as Interferon -2β and Interleukin-2 showed very little improvement in patient survival (2, 28). Notably, the landscape of advanced melanoma treatment has changed drastically in the last decade, following the FDA-approval of immune checkpoint/blockade inhibitors and targeted therapies (4, 29). It is important to note that the genetic classification of melanomas has become particularly important in determining whether a patient is eligible for immuno- or targeted therapy, as the administration of either immuno- or targeted therapy depends on the BRAF status (2). Should the patient have wild type BRAF, they are treated with immune check-point inhibitors and if a V600E/K mutation is identified in BRAF, they can be treated with BRAF and MEK inhibitors or with immunotherapy (2, 26).
Immunotherapy involves the administration of immune checkpoint inhibitors, which are antibodies against cytotoxic T-lymphocyte-associated antigen 4 (CTLA-4) and programmed cell death protein 1 (PD-1) receptor/PD-1 ligand (PD-L1) (2, 30). Mechanistically, the anti-CTLA-4 antibody binds to the inhibitory checkpoint CTLA-4 receptor that is present on the surface of regulatory T-cells, which prohibits the interaction of T-cells with antigen presenting cells that express the CTLA-4 ligand, B7 co-stimulatory molecule (31). The binding of anti-CTLA-4 antibodies to CTLA-4 promotes the activation of T-cells and the inhibition of the immune checkpoint blockade (30, 31). Activated T-cells are then able to illicit an immune response via cytokine secretion, clonal expansion and infiltration into the tumor leading to tumor regression (30, 31). To date, Ipilimumab is the only FDA approved anti- CTLA-4 antibody for the treatment of malignant melanoma and is used in clinics as a monotherapy or in combination with the anti-PD-1 antibody Nivolumab (2, 30). T-cell activation can also be suppressed via another receptor-ligand interaction, whereby ligands PD-L1 and PD-L2 can bind the PD-1 receptor, resulting in a co-inhibitory effect (2, 30). The anti-PD-L1 antibody prevents the binding of PD-L1 or PD-L2 to the PD-1 receptor, thereby promoting T-cell activation (32). The PD-1 receptor is expressed on the surface of immune cells including T-cells. Melanoma cells express the ligands PD-L1 and PD-L2 and fibroblasts present in the TME were shown to express the ligand PD-L2 (33, 34). In 2014, Nivolumab became the first FDA-approved anti-PD-1 antibody for the treatment of unresectable or metastatic melanoma and subsequently, Pembrolizumab was also approved by the FDA for malignant melanoma treatment in 2015 (2, 28, 30). Currently, Nivolumab and Pembrolizumab are administered as monotherapies or Ipilimumab can be administered in combination Nivolumab with as a combination therapy (2, 28, 35). Although immunotherapies have improved overall and relapse free survival in patients with late stage (stage III and IV) melanoma, immune-related adverse effects are particularly common in these patients including diarrhea, fatigue, skin rash, nausea, headaches and joint pain (2).
BRAF and MEK inhibitors, target the mitogen-activated protein kinase (MAPK)/ERK signaling pathway which is constitutively active in BRAF mutant melanoma (2, 30). BRAF encodes the rapidly accelerated fibrosarcoma (RAF) serine/threonine kinases (isoforms: ARAF, BRAF and CRAF) that constitutively dimerize due to the V600E mutation (36). Seeing as BRAF and its isoforms are upstream of the MAPK signaling cascade, they amplify its constitutive activation (37, 38). The MAPK signalling cascade has been shown to play a role in key processes of melanomagenesis such as differentiation, survival, proliferation, metastasis, invasion and angiogenesis (36). Briefly, this signaling pathway is activated by extracellular signals binding to G-coupled receptors or receptor tyrosine kinase (RTK) at the cell membrane, which then activates intracellular GTPase NRAS (also KRAS and HRAS) to NRAS-GTP that is able to further activate all 3 RAF protein isoforms, including BRAF (37, 39). This results in the phosphorylation of downstream MAP/ERK kinases (MEK) and the initiation of the MAPK signaling cascade by further phosphorylation of ERK1/2, which can induce transcriptional and translational signaling (37, 39). Vemurafenib was the first selective oral BRAF small molecule inhibitor, approved in 2011 by the FDA, for advanced melanoma treatment and in 2013 a second selective BRAF inhibitor, Dabrafenib, was approved by the FDA (2, 39). Furthermore, the MEK inhibitor Trametinib became FDA-approved in 2013. In clinical trials Trametinib was also partially successful in treating melanomas harbouring NRAS mutations (2, 40). Vemurafenib, Dabrafenib and Trametinib can all be administered as monotherapies and Dabrafenib and Trametinib can also be administered as a part of BRAF and MEK inhibitor combination therapy (2, 4). Furthermore, in 2015, the FDA approved the oral administration of the MEK inhibitor Cobimetinib in combination with Vemurafenib, as a combination therapy (2, 38).
Seeing as these immuno- and targeted therapies significantly improved overall response in approximately 40% of melanoma patients, they are fast becoming the standard of care for unresectable or metastatic melanoma in many countries including the United Kingdom, the United States, Australia and in Europe (2, 30). Approximately 50-60% of melanoma patients treated with the current FDA-approved immuno- and targeted therapies respond transiently to treatment but unfortunately often progress to develop therapeutic resistance (4, 29). It is therefore not surprising that the resistant mechanisms employed by melanoma cells to circumvent these treatments constitute a major research area in the field of melanoma (3, 41).
It is fast becoming accepted that non-genetic heterogeneity is a key feature of melanomas that contributes to tumor progression and therapeutic resistance (3, 6, 41). Non-genetic heterogeneity is characterized by melanoma cell plasticity, as it has been shown that within a tumor. In order to adapt to changing pressure and various conditions in the TME, melanoma cells exist in different phenotypic states and can readily switch between distinct transcriptional programs, which are suggested to be distinct for different phenotypic states (3, 6, 41). For example, some melanoma cells might be highly differentiated, more proliferative and melanocytic, whereas some cells might be in a slow-cycling dedifferentiated and more invasive mesenchymal-like state (41). Phenotypic switching of melanoma cells has been shown to occur as a result of reversible epigenetic changes caused by but not limited to chromatin remodeling, microRNAs, long non-coding RNAs and histone modifications (3, 41). These transcriptional and epigenetic changes are thought to occur in melanoma cells as an adaptive response to cues in the TME such as hypoxia, glucose or amino acid deprivation, secreted and inflammatory factors, ECM mechano-signaling and in response to immuno- and targeted therapies (3, 7, 41, 42). In response to these cues, melanoma cells are able to temporarily reprogram their phenotype with regards to their proliferation, cell cycling, metabolic status and motility (7, 41).
To date, melanoma cells have been described to exist in several phenotypic states including the differentiated proliferative and melanocytic, the dedifferentiated invasive and mesenchymal-like, the dedifferentiated neural crest stem cell-like, starved, as well as transitory states (43–47). The melanocytic and invasive mesenchymal-like states were identified in melanoma patient tumors using RNA sequencing (44). Single-cell RNA sequencing further identified the differentiated, starved, neural crest-like states, as well as the melanocytic (more proliferative) and mesenchymal-like (more invasive) states in primary melanoma cells that were isolated from biopsies of melanoma patients, who were treated with BRAF or MEK inhibitors (45, 48). These phenotypic states have been linked to distinct transcriptomic profiles and gene signatures in melanoma cells (43–45, 47, 49, 50). More specifically, changes in the expression of the master regulator microphthalmia-associated transcription factor (MITF) has been associated with melanoma phenotypic switching (41, 49, 51).
MITF is a key transcription factor that regulates melanocyte development, pigmentation and melanoma progression and has been implicated in cellular processes such as differentiation, survival, cell cycle regulation, senescence bypass, autophagy and lysosomal production and regulation (52). Furthermore, MITF plays a role in genetic processes such as DNA damage repair and chromosome stability (52). Interestingly, MITF was recently shown to directly repress genes associated with the expression of ECM and focal adhesion pathways, demonstrating its involvement in regulating cell-ECM interactions (53). With regards to melanoma phenotypic states, MITFhigh expressing melanoma cells have been associated with a more differentiated, melanocytic and proliferative state, whereas MITFlow expressing melanoma cells have been characterized as more aggressive, dedifferentiated and mesenchymal-like and were found to be associated with invasion and therapeutic resistance (44, 49, 51).
A second transcriptomic signature that has been associated with the melanoma cell phenotypic switch that is inversely correlated to MITF expression, is the expression of the receptor tyrosine kinase AXL (50, 54). This receptor is expressed by cancer cells including melanoma cells and immune cells (55). The binding of AXL and its ligand growth arrest-specific protein 6 (GAS6) induces intracellular signaling that can affect cancer progression, metastasis and drug resistance (55). In melanoma, compared to the MITFhighAXLlow signature, which promotes a more proliferative and drug sensitive phenotype, the MITFlowAXLhigh signature is specifically associated with an invasive, mesenchymal-like phenotype (50, 54). Importantly, MITFlowAXLhigh expression was shown to be common in BRAF mutated melanoma and has been identified in patients who relapsed following BRAF and MEK inhibitors treatment, thereby indicating that this signature results in a drug resistant phenotype (50).
Melanoma cells that have the MITFlowAXLhigh signature, which are associated with a more dedifferentiated phenotype can express epithelial to mesenchymal transition (EMT) molecular markers (3, 41, 56). It is important to note that melanoma cells cannot undergo EMT as melanocytes arise from the neuroectoderm but melanoma cells can be reprogrammed to mesenchymal-like cells (56, 57). In this regard, MITF/AXL expression was shown to correlate with SOX10 and SOX9 transcriptional signatures, which are molecular markers of epithelial-like and mesenchymal-like phenotypes, respectively (41). Therefore, MITFlowAXLhigh expressing melanoma cells have been associated with SOX10lowSOX9high expression and the opposite was shown for MITFhighAXLlow expressing cells. Furthermore, lower levels of SOX10 have been shown to contribute to therapeutic resistance, in part, via the transcription factors JUN and/or AP-1 and TEAD (47, 58). The SOX10lowSOX9high signature has also been shown to induce a mesenchymal-like phenotype in melanoma cells with the increased expression of mesenchymal markers such as vimentin, platelet derived growth factor receptor-β (PDGFRβ) and α-smooth muscle actin (αSMA) (46, 59–61). Importantly, this signature has also been associated with drug resistance (12, 14).
Additionally, the HIPPO pathway, in particular the transcriptional co-activator yes-activated protein (YAP)-mediated signaling, has been implicated in the dedifferentiated, invasive mesenchymal-like phenotype (14, 41, 56). Accordingly, YAP and its downstream TEAD signature have been shown to be associated with melanoma metastasis and invasion (62–65). Importantly, there is also some evidence that in melanoma cells, the nuclear accumulation of the two transcriptional co-activator paralogs and mechanotransducers YAP and TAZ promotes resistance to BRAF inhibitors (65). More specifically, in MITFlowAXLhigh melanoma cells that were resistant to the BRAF inhibitor Vemurafenib, increased actin stress-fiber formation and remodeling were observed, which were shown to be dependent on increased levels of nuclear YAP/TAZ (65). Furthermore, the pharmacological inhibition or knockdown of YAP in BRAF inhibitor resistant melanoma cells decreased ERK1/2 signaling, remodeling of the actin cytoskeleton and tumor growth and therefore enhanced BRAF inhibitor efficacy (14, 66). Importantly, this adaptive phenotypic transition of melanoma cells towards a dedifferentiated state, associated with upregulated expression of genes involved in EMT, ECM reprogramming, wound healing, cytoskeletal remodeling and YAP/TAZ/TEAD signatures has also been linked to immune evasion and resistance to PD-1 blockade immunotherapy (67, 68).
Interestingly, melanoma cells in a highly differentiated and melanocytic state, characterized by MITFhigh, expression have also been shown to play a role in resistance to BRAF and MEK targeted therapies, via the rheostatic (on/off) regulation of the transcription factors, PAX3 and BRN2 (51, 69). Furthermore, it has been observed that BRAF inhibitor treatment led to the enrichment of a small population of melanoma cells in a dedifferentiated state, termed BRAF inhibitor persister cells. These persister cells constitutively expressed the Aryl hydrocarbon Receptor (AhR) transcription factor and were shown to promote relapse (21, 70). In addition, another population of persister melanoma cells was recently described to account for minimal residual disease (MRD), as this cell population had acquired tolerance to MAPK inhibitors via non-genetic mechanisms. This distinct phenotype was shown to be a transient neural crest stem cell (NCSC) population, which was shown to be dependent on the glial cell line-derived neurotrophic factor cascade that consequently resulted in AKT driven survival via focal adhesion kinase (FAK) signaling (71). To this end, the evidence for the role of phenotypic switching by melanoma cells, as an adaptive process involved in non-mutational resistance, is convincing but the investigation into other transitory phenotypic states and the factors that influence and regulate this phenotypic switching is ongoing (70). Given that melanoma cells can readily switch between these different phenotypic states in response to external cues from the TME or therapeutic insults, it is critical to improve our understanding of the biochemical and biomechanical signaling between melanoma cells and the tumorigenic ECM.
A major cause of altered ECM organization and dynamics during cancer is dysregulated ECM synthesis and remodeling by fibroblasts that have differentiated into cancer-associated fibroblasts (CAFs). ECM remodeling in the TME and organ fibrosis display striking similarities and are both considered to be dysregulated wound healing processes (12). CAFs are the predominant cells in the stroma that modify ECM architecture using several ECM remodeling mechanisms (72). Importantly, CAFs organize and rigidify the ECM by exuding mechanical and tractional forces, resulting in the deposition of ECM fibers that are radially aligned in a parallel fashion (73, 74). CAFs are activated fibroblasts that can originate from resident fibroblasts but may also arise from mesenchymal stem cells, endothelial cells and epithelial cancer cells that have undergone EMT (72, 75, 76). To date, there is no unique marker by which CAFs can be identified, as CAFs express a combination αSMA, fibroblast activation protein-α (FAPα), β1integrin (CD29), fibroblast specific protein 1 (S100A4), caveolin 1, podoplanin and PDGFRβ (72, 76, 77). Furthermore, CAFs can express various combinations of these markers and at different levels (78). Given the numerous cells of origin of CAFs and their expression of various markers, it is not surprising that the CAF population in the TME is heterogeneous (72). Several studies have identified two key CAF phenotypically distinct clusters, inflammatory CAFs (iCAFs) and myofibroblastic CAFs (myCAFs), in the TME of breast cancer, pancreatic adenocarcinoma and melanoma (78–82). myCAFs exhibit myofibroblastic properties that are associated with increased levels of αSMA expression, ECM remodeling, actin-myosin adhesion, wound healing and transforming growth factor β (TGFβ) and interferon αβ (IFNαβ) signaling pathways (79, 82–84). Furthermore, myCAFs were found to be located in the vicinity of cancer cells suggesting that paracrine signaling between myCAFs and cancer cells is critical for ECM remodeling in the TME (78, 82).
In cutaneous melanoma, melanoma-associated fibroblasts (MAFs) are primarily responsible for depositing and remodeling ECM in the TME (85). Interestingly, in aged skin, ECM architecture was shown to be modified to a more aligned organization by aged fibroblasts that produced less of the hyaluronic and proteoglycan link protein, HAPLN1. This aligned ECM in aged skin was shown to promote metastasis and influence therapeutic response and immune cell motility in the melanoma TME (86). ECM remodeling and fiber alignment by MAFs therefore play an important role in melanoma cell invasion and migration, immune escape, metastasis, intra-tumoral heterogeneity and therapeutic resistance (14, 56, 87–90).
ECM stiffness and fiber alignment in solid tumors, including breast carcinoma, sarcomas and melanoma, contribute to increased interstitial pressure, which results in blood vessel collapse and impaired blood supply, leading to hypoxic conditions (91). Importantly, variations in ECM biophysical properties and mechanical forces have been described to alter cellular metabolism in cancer cells (92, 93). Importantly, immunohistochemical analyses of primary and metastatic melanoma patient samples in a tissue microarray, showed that molecular markers of collagen levels were associated with the transcriptomic signature for melanoma cell phenotypic dedifferentiation via the YAP/PAX3/MITF axis (90). Furthermore, this dedifferentiated phenotype was found to be associated with increased collagen fiber abundance and correlated with poorer survival of melanoma patients (90).
Interestingly, we recently showed that melanoma cells that have the specific MITFlowAXLhigh transcriptomic signature and a dedifferentiated, invasive and mesenchymal-like phenotype, display the ability to autonomously deposit and remodel the ECM, resulting in a dense collagen rich and highly organized (aligned fibers) stiff ECM (14). Furthermore, mass spectrometry was used to compare the biochemical composition of 3D-ECM deposited by dedifferentiated, invasive and mesenchymal-like (MITFlowAXLhigh) or differentiated and melanocytic (MITFhighAXLlow) melanoma cells. Matrices derived from dedifferentiated, melanoma cells were found to be enriched for key ECM structural proteins (e.g., fibronectin, laminin, collagen I, collagen IV, collagen VIII, tenascin) and proteins involved in ECM remodeling processes (e.g., Thrombospondin-1, Lysyl oxidase homolog 2 (LOXL2), ADAMTS-like protein 1 and Plasminogen activator inhibitor 1) (14).
MAFs and dedifferentiated mesenchymal-like melanoma cells can produce and remodel the ECM in response to cues from the TME such as secreted factors (e.g., TGFβ, PDGF, fibroblast growth factor 2), hypoxia, ECM mechanical signals and in response to BRAF inhibitor treatment (85). On the other hand, melanoma cells adhere to the ECM and interact directly with the ECM via cell surface receptors. Melanoma cells are therefore highly sensitive to the presence of specific ECM proteins, the alignment of ECM fibers and the mechanical stiffness of the ECM, which can all induce intracellular signaling and metabolic reprogramming, thereby altering melanoma cell behavior (3, 13, 14, 62). Furthermore, melanoma cells have been shown to reprogram fibroblasts in the lymph node to modify the biochemical properties and architecture of the ECM in the lymph node. This reprogramming of reticular fibroblasts is important for melanoma cell invasion at the metastatic site which is often the lymph node (94, 95). Thus, the role of the ECM in melanoma progression and therapeutic resistance resembles a feedback loop, which involves the interplay between fibroblasts, melanoma cells and ECM biochemical and biomechanical properties.
ECM remodeling leads to alterations in ECM composition, fiber organization and stiffness that can influence melanoma progression, phenotypic switching and therapeutic response (96). The ECM is remodeled via three key mechanisms: i) ECM deposition and post translational modification, ii) degradation and iii) force-mediated ECM modification (97). The protein-rich ECM consists of approximately 300 macromolecules that are termed the core matrisome (98). Key matrisome components include collagens (e.g., collagen I, collagen IV), glycoproteins (e.g., fibronectin, laminin, elastin, tenascin), proteoglycans which are glycoproteins that have attached glycosaminoglycans (e.g., hyaluronan, perlecan). Furthermore, matrisome associated proteins include ECM-bound growth and secreted factors (e.g., vascular endothelial growth factor, hepatocyte growth factor, PDGF) and matricellular proteins (e.g., SPARC) (98, 99). ECM composition is dependent on the deposition and post-translational modification of ECM proteins (97).
During ECM deposition and post translational modification, ECM proteins are synthesized and translocated to the Golgi in the cytoplasm, where further post translational modifications can occur (97). For example, collagen is first synthesized as pre-collagen and is post translationally modified in the Golgi to a procollagen α-chain by glycosylation, pro-peptide alignment, disulphide bond formation and hydroxylation (97). Hydroxylation of the procollagen α-chain by lysyl hydroxylases results in triple helix formation of procollagen α-chains, which is followed by the secretion of procollagen α-helices into the extracellular space (97). In the extracellular space, collagen fibrils are formed by proteolytic cleavage of pro-peptides at the C and N terminals (97). Thereafter, the collagen fibrils are assembled via covalent cross-linking by lysyl oxidase (LOX) family members (97). For example, LOXL2 secreted by CAFs, crosslinks collagen and elastin fibers (100). The cross linking of ECM fibrils, in particular collagen fibers, is important for fiber assembly and also increases the tensile strength and stiffness of the ECM (97). Furthermore, collagen fibers are also cross linked to other ECM fibers such as fibronectin fibers by tissue transglutaminase 2, as the deposition of collagen I and III is dependent on the presence and stability of deposited fibronectin (97).
The second mechanism by which the ECM is modified is degradation, which involves the proteolytic cleavage of ECM components by proteases such as matrix metalloproteases (MMPs) (101). Proteolytic cleavage of ECM components promotes the release of ECM fragments, growth factors and cytokines in the ECM milleu, which promotes migration and infiltration of cells in the TME (102). ECM degradation therefore plays an important role in the crosstalk between the ECM, melanoma cells, MAFs and other cells present in the TME such as immune and endothelial cells (96, 97).
The third mechanism by which the ECM is remodeled, is force-mediated modification (96, 97). This involves the binding of cellular receptors such as integrins to ECM molecules including collagens and fibronectin. This leads to traction and mechanical forces, which are transduced to ECM molecules via integrins (96, 97). This results in conformational changes that expose binding sites on ECM molecules, which can then self-assemble into fibrils and create an ECM with aligned and parallel fibers (97). Seeing as melanoma cells interact directly with the ECM via cell membrane receptors at each stage of melanoma progression, ECM remodeling is tightly regulated during melanomagenesis (13). However, the role of ECM-derived mechanical signaling, which is called mechanotransduction, in melanoma biology is not entirely understood and is currently a dynamic and growing topic in the field.
ECM architecture has been shown to play important roles in melanoma phenotypic switching, metastasis and therapeutic resistance because ECM composition, alignment and stiffness directly affect intracellular signaling (96, 97, 103). These intracellular signaling cascades alter melanoma cell behavior and occur as a result of direct binding with the ECM via transmembrane receptors, including integrins and discoidin domain receptors (DDRs) (102). In this regard, fibrillar collagen I and non-fibrillar collagen IV, as well as fibronectin, play important roles in melanoma cell adhesion and migration, thereby promoting metastasis and invasion (13, 102). Melanoma cells were shown to directly interact with collagen IV in the TME via integrins (α2β1 and α3β1), which promoted melanoma cell spreading and motility, through the activation of MMP2 and MMP9 (104–107). Seeing as collagen IV is a found in basement membranes, integrin-mediated interaction of melanoma cells with collagen IV is necessary for melanoma cells to breach the basement membrane when progressing from the RGP to the VGP, where they invade into the dermis prior to disseminating to other organs via lymphatics and blood vessels (108, 109). Integrins have also been described to play an important role in melanoma cell adhesion to other ECM components including laminin, which is also found in basement membranes and fibronectin (108, 110). For example, integrins (α4β1 and α5β1) were found to be involved in promoting melanoma cell attachment to fibronectin fibers which promoted migration of melanoma cells (108).
The direct interaction of melanoma cells with fibrillar collagen I via DRR1 and DDR2 has also been shown to contribute to melanoma cell migration and invasion (111–113). For example, one study showed that in murine melanoma cells, the interaction of DDR2 with collagen I resulted in increased expression of MMP2 and MMP9 via the intracellular ERK/NF-κB signaling pathway and promoted melanoma cell invasion (112). Furthermore, the interaction of cancer cells with aligned ECM fibers promoted directional migration of cancer cells (102, 114). In this regard, melanoma cells were found to mimic the directional migration of fibroblasts along collagen fibrils in a 3D collagen ECM model (114, 115). Melanoma cells were described to elongate and migrate in parallel to collagen fibrils by extending actin-rich protrusions, called filapodia, which adhere to and detach from collagen fibrils in cycles, as the melanoma cells are migrating (115). Furthermore, fibronectin was found to be an essential ECM adhesive component required for the directional migration of pancreatic, prostate and head and neck squamous carcinoma cells (116). The fibronectin monomer consists of 15 domains and two extra domains and the extra domain A (EDA) has also been implicated in the activation of TGFβ signaling and its presence in tumors has been associated with increased ECM remodeling, MMP expression and actin cytoskeleton re-organization (117).
Interestingly, melanoma invasion is affected by both ECM fiber alignment and ECM stiffness, as the shape of melanoma cells was shown to change in response to stiffer and more fibrous substrates (118). For example, the elongation of melanoma cells along aligned collagenous fibers was observed and melanoma cell polarization was found to be directly proportional to collagen matrix fiber alignment (118). Several other studies support these findings, as aligned matrices were shown to promote carcinoma, pancreatic cancer and ovarian cancer cell elongation and invasion (87, 119, 120). Furthermore, ECM fiber alignment and stiffness have been reported to be critical for breast cancer invasion, which is reviewed in detail by Kai et al, 2019 (102, 121–123). Surprisingly in contrast to these studies, experiments using varying ECM stiffness models, suggested that the correlation between melanoma cell invasion and substrate stiffness might differ to what was observed for other cancer models. Melanoma cell invasion was shown to be restricted by both soft and highly stiff collagen substrates, suggesting a non-linear relationship between substrate stiffness and optimum invasion (14, 124). These findings are further supported by another group which showed that collagen matrices of an intermediate stiffness provided the optimal conditions for facilitating melanoma cell invasion (118). These differences might be related to the tissue origin of the breast cancer and melanoma cells. Breast tissue is softer than the skin because breast tissue differs to the skin in term of its rigidity and elasticity. Thus, during malignancy, breast cancer cells initially progress in a softer matrix associated with the physiological breast tissue stiffness but require a stiffer matrix for invasion, whereas melanoma cells initially progress in a stiffer environment in the skin and require a softer and less dense matrix for invasion (125, 126).
It is important to note that the studies discussed in this section employed in vitro (e.g., hydrogels or decellularized matrices) or animal models to investigate the role of ECM alignment and stiffness in melanoma progression, in particular during melanoma cell migration and invasion. These findings have yet to be confirmed in clinical melanoma samples.
ECM stiffness and alignment, caused by collagen abundance and crosslinking in the TME, have been shown to contribute to melanoma cell phenotypic switching and therapeutic resistance by inducing mechanosignaling, which can alter cellular behavior to promote a mechanosensitive and resistant mesenchymal-like phenotype (14, 90). Collagen abundance and stiffness were shown to promote the nuclear localization of YAP, which regulated melanoma cell pigmentation and differentiation through the expression of MITF (90). Moreover, one study suggested that higher MITF levels in melanoma cells might be associated with the repression of ECM and focal adhesion genes (53). The effect of MITF repression of these genes was found to be reversible when MITF was knocked down in melanoma cells, resulting in increased focal adhesion complexes and resistance to BRAF inhibitor treatment (53). Interestingly, our group demonstrated that stiffer collagen substrates resulted in increased in mechanosensing via YAP and myocardin-related transcription factor (MRTF) in MITFlow, dedifferentiated, invasive mesenchymal-like melanoma cells, in response to BRAF inhibitor treatment (14). Furthermore, this mechanosensitivity promoted melanoma cells to deposit a stiff, organized and drug-protective ECM and these observations were further confirmed in vivo (14).
We also recently demonstrated that stiff and aligned collagen and fibronectin rich matrices deposited by MAFs protected melanoma cells from BRAF/MEK inhibitor treatment, when melanoma cells were cultured on top of these matrices (127). This matrix-mediated drug resistance was demonstrated to be DDR1 and DDR2 dependent and promoted melanoma cell survival via the NIK/IKKα/NF-κB2 signaling pathway (127). Therefore, the alignment of fibrils and ECM composition plays an important role in melanoma cell migration and contributes to melanoma cell escape mechanisms to targeted therapies. These studies therefore suggest that in melanoma cells, ECM topography plays important roles in MITF levels and therefore contribute to phenotypic switching and drug adaptive responses.
The induction of ECM-related signaling pathways is a mechanism that is implored by melanoma cells to evade BRAF inhibition and confers tolerance and resistance to BRAF inhibitor treatment (53, 71, 88, 127, 128). For example, one study described upregulated expression of the collagenase and metalloproteinase MT1-MMP in melanoma cells, which acquired resistance to the BRAF inhibitor Vemurafenib (128). Furthermore, this resistance mechanism involved β1integrin/FAK signaling and was also dependent on the TGFβ cascade (128). Importantly, the inhibition of this ECM signaling cascade, using the MT1-MMP selective inhibitor ND322 restored melanoma cell sensitivity to BRAF inhibitor treatment (128). Interestingly, in PDX models treated with MAPK inhibitors, a subpopulation of drug-tolerant melanoma cells was described to be associated with the emergence of a transient neural crest stem cell (NCSC) state. The NCSC state was a persister state that most closely corresponds to the minimal residual disease (MRD) observed in clinics and displayed increased activation of FAK-dependent AKT survival signaling (71). Delaying the onset of this non-genetic resistance was achieved by FAK inhibition and the consequential targeting of NCSC-like melanoma cells (71).
Interestingly, BRAF inhibitor treatment has also been implicated in activating MAFs to remodel the ECM making it stiffer, which induced drug tolerance in melanoma cells (88). Furthermore, the direct interaction of melanoma cells via β1integrin with the ECM deposited by MAFs, induced intracellular FAK signaling that consequently re-activated the ERK signaling pathway in melanoma cells, following BRAF inhibitor treatment (88). Importantly, a fibroblastic and remodeled ECM was observed in the tumoral stroma of melanoma tissue from Vemurafenib (BRAF inhibitor) treated patients (88). Additionally, myCAF (myofibroblastic MAF) clusters with distinguished ECM and TGFβ signaling signatures have been associated with primary resistance to immunotherapies in samples from non-responder melanoma patients (81).
These studies therefore provide some insight into the contribution of ECM signaling and remodeling in response to targeted- and immuno-therapies, in melanoma. However, these ECM-related resistance mechanisms employed by melanoma cells appear to be multifaceted and warrant further investigation.
Given that MAFs are the primary cells that remodel the ECM in the TME and that ECM composition, alignment and stiffness have been shown to promote metastasis, immunosuppression and therapeutic resistance, an obvious strategy to counteract this effect is to target MAF functions in the melanoma TME. However, it was reported that ablating CAFs in pancreatic ductal adenocarcinoma (PDAC) mouse models resulted in invasive, dedifferentiated and highly hypoxic tumors (129). Furthermore, evidence of EMT and cancer stem cells were observed in tumors, which resulted in poor patient survival and immune suppression. Additionally, the authors also reported that lower myofibroblast number in PDAC patient tumors correlated with reduced patient survival (129). Interestingly, another study showed that targeting iCAFs by JAK inhibition shifted them to a myCAF phenotype in vivo (130). In contrast, another group reported that the blockade of CAF contractility by the JAK1/2 inhibitor, Ruxolitinib, counteracted CAF-dependent carcinoma cell invasion and ECM remodeling (131). Thus, the issue of functional complexity and phenotypic heterogeneity of CAFs must be taken into consideration for the development of effective anti-CAF therapies (78). Therefore, the current opinion in the field is to rather achieve normalization in the TME than completely ablate CAFs (132, 133). This approach focuses on restoring homeostasis in the TME, in particular with regards to the ECM, to resemble a non-tumorigenic normal tissue-like state (132, 133). One possible way to achieve this could be to reprogram MAFs to a normal fibroblast phenotype. However, this requires improved understanding of the mechanisms underpinning the activation and functional diversity of MAFs in the melanoma TME.
In light of the current opinion to rather normalize the fibrotic-like tumorigenic ECM than ablate CAFs in the TME, it might be worthwhile to investigate whether the repurposing of anti-fibrotic drugs that are used to treat idiopathic pulmonary fibrosis, such as the TGFβ regulator Pirfenidone or the triple tyrosine kinase inhibitor Nintedanib, in combination with current therapies, might disrupt MAF signaling and ECM-mediated resistance (62). Another strategy might be to identify novel druggable targets in melanoma cells that promote the ECM remodeling abilities associated with the dedifferentiated, mesenchymal-like and resistant phenotype in melanoma cells. We recently identified a fibrosis-associated miRNA cluster (miR-143/-145) that was shown to play an important role in driving the ECM program in dedifferentiated, invasive and mesenchymal-like melanoma cells (15). Furthermore, we also found that, in an allograft melanoma model, the anti-fibrotic drug Nintedanib was efficient in preventing miR-143/-145 cluster upregulation in melanoma cells and in combination with MAPK targeting therapy, normalized the tumoral ECM niche and delayed relapse (15).
Another approach might be to disrupt ECM fiber linearization in the melanoma TME by targeting the YAP mechanotransduction pathway and DDR1/2-dependent collagen signaling using Verteporfin and Imatinib, respectively (134, 135). This treatment strategy might prevent targeted therapy-induced collagen linearization and ECM remodeling, thereby potentially abrogating the permissive stroma and improve drug response. Interestingly, a recent study found that collagen linearization can be reversed and consequently metastasis can be hampered by genetically or pharmacologically restoring levels of the secreted factor WISP2. WISP2 and WISP1 are matricellular proteins secreted by cancer cells (136). WISP1 binds to linearized collagen type I, whereas WISP2 inhibits the binding of WISP1 to collagen type I and in turn inhibits collagen linearization. The authors found that in cancer patients, WISP2 expression was lower in solid tumors. Therefore, the restoration of a higher WISP2:WISP1 ratio, in an in vivo cancer model, normalized collagen fibers in the TME and drastically inhibited metastasis (136). Therapeutically disrupting ECM fiber linearization might therefore prove to be a valuable strategy in achieving ECM normalization in the melanoma TME.
Approaches to normalize the fibrotic-like ECM in the TME have also been described to improve response to immunotherapy (137). One study showed that, in isogenic murine cancer models, including melanoma, targeting DDR2, using the tyrosine kinase inhibitor Dasatinib, in combination with anti-PD-1, improved therapeutic response to immunotherapy (137). Furthermore, another group showed that targeting collagen stabilization (crosslinking) using a LOX inhibitor in combination with anti-PD-1 in vivo, not only reduced overall tumor stiffness but also allowed for improved T-cell migration in the melanoma TME, thereby improving the overall efficacy of this immunotherapy (138). Furthermore, in a preclinical melanoma study, mouse survival was prolonged when CAFs were targeted with Nintedanib in combination with anti-PD-1 treatment. Tumor growth was described to be repressed due to the disruption the fibrotic-like ECM, which led to improved infiltration of CD8+ T cells and the production of granzyme B, therefore improving anti-tumor immunity and response to immunotherapy in tumor-bearing mice (139). Another potential therapeutic strategy to normalize the TME was suggested by a group that investigated the combination of shPD-L1 loaded nanoparticles and the ECM degradation enzyme hyaluronidase in an in vivo melanoma model (140). The authors found that the hyaluronidase degraded the hyaluronic acid in tumors, thereby increasing the infiltration of the shPD-L1 loaded nanoparticles in the TME and resulted in improved PD-L1 gene silencing (140). Additionally, another group carried out an epigenetic screen to identify novel druggable targets for melanoma treatment and identified TP-472 (targets bromodomain-7/9) as a potential drug, as it effectively inhibited melanoma cell proliferation (141). Interestingly, transcriptome-wide mRNA sequencing revealed that TP-472 treatment downregulated genes encoding various ECM proteins, such as integrins, collagens, and fibronectins in melanoma cells (141). Therefore, these studies suggest that normalizing the fibrotic and dense ECM in the melanoma TME might facilitate improved immune cell infiltration and overall immune anti-tumor response, which has the potential to significantly improve the efficacy of immunotherapies and patient prognosis.
Interestingly, there is some evidence that ECM molecules can serve as biomarkers and can predict the efficacy of immunotherapy treatment in melanoma patients (142, 143). For example, one study described that higher levels of ECM and tissue remodeling proteins such as MMP degraded type III and type IV collagens, quantified in patient serum from metastatic melanoma patients prior to immune checkpoint inhibitor treatment, were predictors of poorer overall survival (143). Furthermore, another group recently demonstrated that levels of the ECM protein elastin microfibril interface-located protein 2 (EMILIN-2) vary amongst melanoma patients and that the absence of EMILIN-2 expression was shown to be associated with higher PD-L1 expression levels, that in turn, improved the efficacy of immunotherapy. A possible reason for this observation was suggested using an in vivo Emilin2-/- mouse model that showed improved vessel normalization and a decrease in hypoxia within tumors (142). Further investigation of ECM markers in melanoma patient serum might therefore be an interesting avenue to further explore, in order to identify novel melanoma prognostic biomarkers.
The normalization of the melanoma TME therefore has the potential to limit immune suppression, increase therapeutic responses and improve advanced melanoma patient prognosis. However, novel therapeutic strategies to prevent or reverse the altered ECM within tumors require further investigation.
The ECM is a dynamic feature of the TME that can dramatically influence cancer biology. Herein, we reviewed how melanoma ECM topology is remodeled by MAFs and dedifferentiated, invasive and mesenchymal-like melanoma cells. These remodeling changes result in ECM architectural modifications including changes in ECM deposition, composition, fiber alignment and mechanical stiffness. Importantly, these biophysical modifications influence melanoma cell, fibroblast and immune cell behaviors, generate intra-tumoral heterogeneity, promote metastasis and compromise anti-melanoma treatments. Furthermore, we give insight into how melanoma cells react to these architectural changes, as they interact directly with the ECM via transmembrane receptors, in a reciprocal manner, which influences melanoma cell migration, invasion and phenotypic tumor states. Notably, we provide evidence on how ECM composition, alignment and stiffness contribute to melanoma cells evading therapies and provide a perspective on current and future strategies to normalize the drug protective ECM in the TME.
AP and ST-D contributed equally to the conceptualization, writing and editing of this review. All authors contributed to the article and approved the submitted version.
AP is a postdoctoral research fellow whose fellowship is funded by the French National Institute for Cancer.
The authors acknowledge grant support from the French League for Cancer (équipe labellisée 2022), the French National Institute for Cancer (INCA N°12673) and the French-South African PHC PROTEA project (N° 46471YC).
The authors declare that the research was conducted in the absence of any commercial or financial relationships that could be construed as a potential conflict of interest.
All claims expressed in this article are solely those of the authors and do not necessarily represent those of their affiliated organizations, or those of the publisher, the editors and the reviewers. Any product that may be evaluated in this article, or claim that may be made by its manufacturer, is not guaranteed or endorsed by the publisher.
1. Ward WH, Lambreton F, Goel N, Yu JQ, Farma JM. Clinical presentation and staging of melanoma. In: Cutaneous melanoma: Etiology and therapy. CA, United States:Codon Publications (2017). p. 79–89.
2. Domingues B, Lopes J, Soares P, Populo H. Melanoma treatment in review. ImmunoTargets Ther (2018) 7:35–49. doi: 10.2147/ITT.S134842
3. Kozar I, Margue C, Rothengatter S, Haan C, Kreis S. Many ways to resistance: How melanoma cells evade targeted therapies. Biochim Biophys Acta - Rev Cancer (2019) 1871:3 13–22. doi: 10.1016/j.bbcan.2019.02.002
4. Robert C, Grob JJ, Stroyakovskiy D, Karaszewska B, Hauschild A, Levchenko E, et al. Five-year outcomes with dabrafenib plus trametinib in metastatic melanoma. N Engl J Med (2019) 381:7. doi: 10.1056/NEJMoa1904059
5. Grzywa TM, Paskal W, Włodarski PK. Intratumor and intertumor heterogeneity in melanoma. Transl Oncol (2017) 10:6. doi: 10.1016/j.tranon.2017.09.007
6. Arozarena I, Wellbrock C. Phenotype plasticity as enabler of melanoma progression and therapy resistance. Nat Rev Cancer (2019) 19:7. doi: 10.1038/s41568-019-0154-4
7. Kemper K, de Goeje PL, Peeper DS, van Amerongen R. Phenotype switching: Tumor cell plasticity as a resistance mechanism and target for therapy. Cancer Res (2014) 74:21. doi: 10.1158/0008-5472.CAN-14-1174
8. Quail DF, Joyce JA. Microenvironmental regulation of tumor progression and metastasis. Nat Med (2013) 19:11. doi: 10.1038/nm.3394
9. Farc O, Cristea V. An overview of the tumor microenvironment, from cells to complex networks. Exp Ther Med (2020) 21:1. doi: 10.3892/etm.2020.9528
10. Hanahan D, Weinberg RA. Hallmarks of cancer: The next generation. Cell (2011) 144:5. doi: 10.1016/j.cell.2011.02.013
11. Villanueva J, Herlyn M. Melanoma and the tumor microenvironment. Curr Oncol Rep (2008) 10:5. doi: 10.1007/s11912-008-0067-y
12. Diazzi S, Tartare-Deckert S, Deckert M. Bad neighborhood: Fibrotic stroma as a new player in melanoma resistance to targeted therapies. Cancers (2020) 12:6. doi: 10.3390/cancers12061364
13. Ju RJ, Stehbens SJ, Haass NK. The role of melanoma cell-stroma interaction in cell motility, invasion, and metastasis. Front Med (2018) 5:307. doi: 10.3389/fmed.2018.00307
14. Girard CA, Lecacheur M, Ben Jouira R, Berestjuk I, Diazzi S, Prod’homme V, et al. A feed-forward mechanosignaling loop confers resistance to therapies targeting the MAPK pathway in BRAF-mutant melanoma. Cancer Res (2020) 80:10. doi: 10.1158/0008-5472.CAN-19-2914
15. Diazzi S, Baeri A, Fassy J, Lecacheur M, Marin-Bejar O, Girard CA, et al. Blockade of the pro-fibrotic reaction mediated by the miR-143/-145 cluster enhances the responses to targeted therapy in melanoma. EMBO Mol Med (2022) 14:3. doi: 10.15252/emmm.202115295
16. Tsao H, Chin L, Garraway LA, Fisher DE. Melanoma: From mutations to medicine. Genes Dev (2012) 26:11. doi: 10.1101/gad.191999.112
17. Busse A, Keilholz U. Role of TGF-β in melanoma. Curr Pharm Biotechnol (2011) 12:12. doi: 10.2174/138920111798808437
18. Miller AJ, Mihm MC. Mechanisms of disease melanoma. N Engl J Med (2006) 1:55–65. doi: 10.1056/NEJMra052166
19. Greene MH, Elder DE, Guerry D, Epstein MN, Greene MH, Van Horn M. A study of tumor progression: The precursor lesions of superficial spreading and nodular melanoma. Hum Pathol (1984) 12:1147–65. doi: 10.1016/s0046-8177(84)80310-x
20. Akbani R, Akdemir KC, Aksoy BA, Albert M, Ally A, Amin SB, et al. Genomic classification of cutaneous melanoma. Cell (2015) 161:7. doi: 10.1016/j.cell.2015.05.044
21. Toussi A, Mans N, Welborn J, Kiuru M. Germline mutations predisposing to melanoma. J Cutan Pathol (2020) 47:7. doi: 10.1111/cup.13689
22. Girotti MR, Fernández M, López JA, Camafeita E, Fernández EA, Albar JP, et al. SPARC Promotes cathepsin b-mediated melanoma invasiveness through a collagen i/α2B1 integrin axis. J Invest Dermatol (2011) 131:12. doi: 10.1038/jid.2011.239
23. Bonitsis N, Batistatou A, Karantima S, Charalabopoulos K. The role of cadherin/catenin complex in malignant melanoma. Exp Oncol (2006) 3:187–93.
24. Breslow A. Thickness, cross-sectional areas and depth of invasion in the prognosis of cutaneous melanoma. Ann Surg (1970) 5:902–8. doi: 10.1097/00000658-197011000-00017
25. Balch CM, Gershenwald JE, Soong SJ, Thompson JF, Atkins MB, Byrd DR, et al. Final version of 2009 AJCC melanoma staging and classification. J Clin Oncol (2009) 27:36. doi: 10.1200/JCO.2009.23.4799
26. Eggermont AMM, Kirkwood JM. Re-evaluating the role of dacarbazine in metastatic melanoma: What have we learned in 30 years? Eur J Cancer (2004) 40:12. doi: 10.1016/j.ejca.2004.04.030
27. Shtivelman E, Davies MA, Hwu P, Yang J, Lotem M, Oren M, et al. Pathways and therapeutic targets in melanoma. Oncotarget (2014) 5:7. doi: 10.18632/oncotarget.1892
28. Fujimura T, Kambayashi Y, Ohuchi K, Muto Y, Aiba S. Treatment of advanced melanoma: Past, present and future. Life (2020) 10:9. doi: 10.3390/life10090208
29. Larkin J, Chiarion-Sileni V, Gonzalez R, Grob J-J, Rutkowski P, Lao CD, et al. Five-year survival with combined nivolumab and ipilimumab in advanced melanoma. N Engl J Med (2019) 381:16. doi: 10.1056/nejmoa1910836
30. Luke JJ, Flaherty KT, Ribas A, Long GV. Targeted agents and immunotherapies: Optimizing outcomes in melanoma. Nat Rev Clin Oncol (2017) 14:8. doi: 10.1038/nrclinonc.2017.43
31. Sullivan RJ, Atkins MB, Kirkwood JM, Agarwala SS, Clark JI, Ernstoff MS, et al. An update on the society for immunotherapy of cancer consensus statement on tumor immunotherapy for the treatment of cutaneous melanoma: Version. J Immunother Cancer (2018) 6:1. doi: 10.1186/s40425-018-0362-6
32. Mahoney KM, Freeman GJ, McDermott DF. The next immune-checkpoint inhibitors: Pd-1/pd-l1 blockade in melanoma. Clin Ther (2015) 37:4. doi: 10.1016/j.clinthera.2015.02.018
33. Yi M, Niu M, Xu L, Luo S, Wu K. Regulation of PD-L1 expression in the tumor microenvironment. J Hematol Oncol (2021) 14:1. doi: 10.1186/s13045-020-01027-5
34. Raskov H, Orhan A, Gaggar S, Gögenur I. Cancer-associated fibroblasts and tumor-associated macrophages in cancer and cancer immunotherapy. Front Oncol (2021) 11:668731. doi: 10.3389/fonc.2021.668731
35. Babacan NA, Eroglu Z. Treatment options for advanced melanoma after anti-PD-1 therapy. Curr Oncol Rep (2020) 22:4. doi: 10.1007/s11912-020-0894-z
36. Alqathama A. BRAF in malignant melanoma progression and metastasis: potentials and challenges. Am J Cancer Res (2020) 4:1103–14.
37. Poulikakos PI, Rosen N. Mutant BRAF melanomas-dependence and resistance. Cancer Cell (2011) 19(1):11–5. doi: 10.1016/j.ccr.2011.01.008
38. Eroglu Z, Ribas A. Combination therapy with BRAF and MEK inhibitors for melanoma: Latest evidence and place in therapy. Ther Adv Med Oncol (2016) 8:1. doi: 10.1177/1758834015616934
39. Kakadia S, Yarlagadda N, Awad R, Kundranda M, Niu J, Naraev B, et al. Mechanisms of resistance to BRAF and MEK inhibitors and clinical update of us food and drug administration-approved targeted therapy in advanced melanoma. Onco Targets Ther (2018) 11:7095–107. doi: 10.2147/OTT.S182721
40. Ascierto PA, Schadendorf D, Berking C, Agarwala SS, van Herpen CML, Queirolo P, et al. MEK162 for patients with advanced melanoma harbouring NRAS or Val600 BRAF mutations: A non-randomised, open-label phase 2 study. Lancet Oncol (2013) 14:3. doi: 10.1016/S1470-2045(13)70024-X
41. Rambow F, Marine JC, Goding CR. Melanoma plasticity and phenotypic diversity: Therapeutic barriers and opportunities. Genes Dev (2019) 33:19. doi: 10.1101/gad.329771.119
42. Friedl P, Alexander S. Review cancer invasion and the Microenvironment: Plasticity and reciprocity. Cell (2012) 147:5. doi: 10.1016/j.cell.2011.11.016
43. Hoek KS, Schlegel NC, Brafford P, Sucker A, Ugurel S, Kumar R, et al. Metastatic potential of melanomas defined by specific gene expression profiles with no BRAF signature. Pigment Cell Res (2006) 19:4. doi: 10.1111/j.1600-0749.2006.00322.x
44. Hoek KS, Eichhoff OM, Schlegel NC, Döbbeling U, Kobert N, Schaerer L, et al. In vivo switching of human melanoma cells between proliferative and invasive states. Cancer Res (2008) 68:3. doi: 10.1158/0008-5472.CAN-07-2491
45. Rambow F, Rogiers A, Marin-Bejar O, Aibar S, Femel J, Dewaele M, et al. Toward minimal residual disease-directed therapy in melanoma. Cell (2018) 174:4. doi: 10.1016/j.cell.2018.06.025
46. Wouters J, Kalender-Atak Z, Minnoye L, Spanier KI, De Waegeneer M, Bravo González-Blas C, et al. Robust gene expression programs underlie recurrent cell states and phenotype switching in melanoma. Nat Cell Biol (2020) 22:8. doi: 10.1038/s41556-020-0547-3
47. Tsoi J, Robert L, Paraiso K, Galvan C, Sheu KM, Lay J, et al. Multi-stage differentiation defines melanoma subtypes with differential vulnerability to drug-induced iron-dependent oxidative stress. Cancer Cell (2018) 33:5. doi: 10.1016/j.ccell.2018.03.017
48. Tirosh I, Izar B, Prakadan SM, Wadsworth MH, Treacy D, Trombetta JJ, et al. Dissecting the multicellular ecosystem of metastatic melanoma by single-cell RNA-seq. Science (2016) 352:6282. doi: 10.1126/science.aad0501
49. Carreira S, Goodall J, Denat L, Rodriguez M, Nuciforo P, Hoek KS, et al. Mitf regulation of Dia1 controls melanoma proliferation and invasiveness. Genes Dev (2006) 20:24. doi: 10.1101/gad.406406
50. Müller J, Krijgsman O, Tsoi J, Robert L, Hugo W, Song C, et al. Low MITF/AXL ratio predicts early resistance to multiple targeted drugs in melanoma. Nat Commun (2014) 5:1. doi: 10.1038/ncomms6712
51. Wellbrock C, Marais R. Elevated expression of MITF counteracts b-RAF-stimulated melanocyte and melanoma cell proliferation. J Cell Biol (2005) 170:5. doi: 10.1083/jcb.200505059
52. Goding CR, Arnheiter H. Mitf–the first 25 years. Genes Dev (2019) 33:15. doi: 10.1101/gad.324657.119
53. Dilshat R, Fock V, Kenny C, Gerritsen I, Lasseur RMJ, Travnickova J, et al. Mitf reprograms the extracellular matrix and focal adhesion in melanoma. elife (2021) 10. doi: 10.7554/eLife.63093
54. Sensi M, Catani M, Castellano G, Nicolini G, Alciato F, Tragni G, et al. Human cutaneous melanomas lacking MITF and melanocyte differentiation antigens express a functional axl receptor kinase. J Invest Dermatol (2011) . 131:12. doi: 10.1038/jid.2011.218
55. Zhu C, Wei Y, Wei X. AXL receptor tyrosine kinase as a promising anti-cancer approach: Functions, molecular mechanisms and clinical applications. Mol Cancer (2019) 18:1. doi: 10.1186/s12943-019-1090-3
56. Pedri D, Karras P, Landeloos E, Marine JC, Rambow F. Epithelial-to-mesenchymal-like transition events in melanoma. FEBS J (2022) 289:5. doi: 10.1111/febs.16021
57. Robert G, Gaggioli C, Bailet O, Chavey C, Abbe P, Aberdam E, et al. SPARC Represses e-cadherin and induces mesenchymal transition during melanoma development. Cancer Res (2006) 66:15. doi: 10.1158/0008-5472.CAN-05-3189
58. Shaffer SM, Dunagin MC, Torborg SR, Torre EA, Emert B, Krepler C, et al. Rare cell variability and drug-induced reprogramming as a mode of cancer drug resistance. Nature (2017) 546:7658. doi: 10.1038/nature22794
59. Li M, Zhang B, Wang X, Ban X, Sun T, Liu Z, et al. A novel function for vimentin: The potential biomarker for predicting melanoma hematogenous metastasis. J Exp Clin Cancer Res (2010) 29:1. doi: 10.1186/1756-9966-29-109
60. Shakhova O, Cheng P, Mishra PJ, Zingg D, Schaefer SM, Debbache J, et al. Antagonistic cross-regulation between Sox9 and Sox10 controls an anti-tumorigenic program in melanoma. PloS Genet (2015) 11:1. doi: 10.1371/journal.pgen.1004877
61. Cheng PF, Shakhova O, Widmer DS, Eichhoff OM, Zingg D, Frommel SC, et al. Methylation-dependent SOX9 expression mediates invasion in human melanoma cells and is a negative prognostic factor in advanced melanoma. Genome Biol (2015) 16:1. doi: 10.1186/s13059-015-0594-4
62. Nazemi M, Rainero E. Cross-talk between the tumor microenvironment, extracellular matrix, and cell metabolism in cancer. Front Oncol (2020) 10:239. doi: 10.3389/fonc.2020.00239
63. Verfaillie A, Imrichova H, Atak ZK, Dewaele M, Rambow F, Hulselmans G, et al. Decoding the regulatory landscape of melanoma reveals TEADS as regulators of the invasive cell state. Nat Commun (2015) 6:1. doi: 10.1038/ncomms7683
64. Zhang X, Yang L, Szeto P, Abali GK, Zhang Y, Kulkarni A, et al. The hippo pathway oncoprotein YAP promotes melanoma cell invasion and spontaneous metastasis. Oncogene (2020) 39:30. doi: 10.1038/S41388-020-1362-9
65. Kim MH, Kim J, Hong H, Lee S, Lee J, Jung E, et al. Actin remodeling confers BRAF inhibitor resistance to melanoma cells through YAP/TAZ activation. EMBO J (2016) 35:5. doi: 10.15252/embj.201592081
66. Wang C, Zhu X, Feng W, Yu Y, Jeong K, Guo W, et al. Verteporfin inhibits YAP function through up-regulating 14-3-3σ sequestering YAP in the cytoplasm. Am J Cancer Res (2016) 1:27–37.
67. Hugo W, Zaretsky JM, Sun L, Song C, Moreno BH, Hu-Lieskovan S, et al. Genomic and transcriptomic features of response to anti-PD-1 therapy in metastatic melanoma. Cell (2017) 168:3. doi: 10.1016/j.cell.2017.01.010
68. Orgaz JL, Crosas-Molist E, Sadok A, Perdrix-Rosell A, Maiques O, Rodriguez-Hernandez I, et al. Myosin II reactivation and cytoskeletal remodeling as a hallmark and a vulnerability in melanoma therapy resistance. Cancer Cell (2020) 37:1. doi: 10.1016/j.ccell.2019.12.003
69. Smith MP, Rana S, Ferguson J, Rowling EJ, Flaherty KT, Wargo JA, et al. A PAX3/BRN2 rheostat controls the dynamics of BRAF mediated MITF regulation in MITFhigh/AXLlow melanoma. Pigment Cell Melanoma Res (2019) 32:2. doi: 10.1111/pcmr.12741
70. Shen S, Faouzi S, Bastide A, Martineau S, Malka-Mahieu H, Fu Y, et al. An epitranscriptomic mechanism underlies selective mRNA translation remodelling in melanoma persister cells. Nat Commun (2019) 10:1. doi: 10.1038/s41467-019-13360-6
71. Marin-Bejar O, Rogiers A, Dewaele M, Femel J, Karras P, Pozniak J, et al. Evolutionary predictability of genetic versus nongenetic resistance to anticancer drugs in melanoma. Cancer Cell (2021) 39:8. doi: 10.1016/j.ccell.2021.05.015
72. Sahai E, Astsaturov I, Cukierman E, DeNardo DG, Egeblad M, Evans RM, et al. A framework for advancing our understanding of cancer-associated fibroblasts. Nat Rev Cancer (2020) 20:3. doi: 10.1038/s41568-019-0238-1
73. Calvo F, Ege N, Grande-Garcia A, Hooper S, Jenkins RP, Chaudhry SI, et al. Mechanotransduction and YAP-dependent matrix remodelling is required for the generation and maintenance of cancer-associated fibroblasts. Nat Cell Biol (2013) 15:6. doi: 10.1038/ncb2756
74. Liu T, Zhou L, Li D, Andl T, Zhang Y. Cancer-associated fibroblasts build and secure the tumor microenvironment. Front Cell Dev Biol (2019) 7:60. doi: 10.3389/fcell.2019.00060
75. Kuzet SE, Gaggioli C. Fibroblast activation in cancer: when seed fertilizes soil. Cell Tissue Res (2016) 365:3. doi: 10.1007/s00441-016-2467-x
76. Ganguly D, Chandra R, Karalis J, Teke M, Aguilera T, Maddipati R, et al. Cancer-associated fibroblasts: Versatile players in the tumor microenvironment. Cancers (2020) 12:9. doi: 10.3390/cancers12092652
78. Mhaidly R, Mechta-Grigoriou F. Fibroblast heterogeneity in tumor micro-environment: Role in immunosuppression and new therapies. Semin Immunol (2020) 48:4. doi: 10.1016/j.smim.2020.101417
79. Costa A, Kieffer Y, Scholer-Dahirel A, Pelon F, Bourachot B, Cardon M, et al. Fibroblast heterogeneity and immunosuppressive environment in human breast cancer. Cancer Cell (2018) 33:3. doi: 10.1016/j.ccell.2018.01.011
80. Givel AM, Kieffer Y, Scholer-Dahirel A, Sirven P, Cardon M, Pelon F, et al. MiR200-regulated CXCL12β promotes fibroblast heterogeneity and immunosuppression in ovarian cancers. Nat Commun (2018) 9:1. doi: 10.1038/s41467-018-03348-z
81. Kieffer Y, Hocine HR, Gentric G, Pelon F, Bernard C, Bourachot B, et al. Single-cell analysis reveals fibroblast clusters linked to immunotherapy resistance in cancer. Cancer Discovery (2020) 10:9. doi: 10.1158/2159-8290.CD-19-1384
82. Öhlund D, Handly-Santana A, Biffi G, Elyada E, Almeida AS, Ponz-Sarvise M, et al. Distinct populations of inflammatory fibroblasts and myofibroblasts in pancreatic cancer. J Exp Med (2017) 214:3. doi: 10.1084/jem.20162024
83. Pelon F, Bourachot B, Kieffer Y, Magagna I, Mermet-Meillon F, Bonnet I, et al. Cancer-associated fibroblast heterogeneity in axillary lymph nodes drives metastases in breast cancer through complementary mechanisms. Nat Commun (2020) 11:1. doi: 10.1038/s41467-019-14134-w
84. Bonneau C, Eliès A, Kieffer Y, Bourachot B, Ladoire S, Pelon F, et al. A subset of activated fibroblasts is associated with distant relapse in early luminal breast cancer. Breast Cancer Res (2020) 22:1. doi: 10.1186/s13058-020-01311-9
85. Romano V, Belviso I, Venuta A, Ruocco MR, Masone S, Aliotta F, et al. Influence of tumor microenvironment and fibroblast population plasticity on melanoma growth, therapy resistance and immunoescape. Int J Mol Sci (2021) 22:10. doi: 10.3390/ijms22105283
86. Kaur A, Ecker BL, Douglass SM, Kugel CH, Webster MR, Almeida FV, et al. Remodeling of the collagen matrix in aging skin promotes melanoma metastasis and affects immune cell motility. Cancer Discovery (2019) 9:1. doi: 10.1158/2159-8290.CD-18-0193
87. Goetz JG, Minguet S, Navarro-Lérida I, Lazcano JJ, Samaniego R, Calvo E, et al. Biomechanical remodeling of the microenvironment by stromal caveolin-1 favors tumor invasion and metastasis. Cell (2011) .146:1. doi: 10.1016/j.cell.2011.05.040
88. Hirata E, Girotti MR, Viros A, Hooper S, Spencer-Dene B, Matsuda M, et al. Intravital imaging reveals how BRAF inhibition generates drug-tolerant microenvironments with high integrin β1/FAK signaling. Cancer Cell (2015) 27:4. doi: 10.1016/j.ccell.2015.03.008
89. Hutchenreuther J, Leask A. Why target the tumor stroma in melanoma? Cell Commun Signal (2018) 12:1. doi: 10.1007/s12079-017-0419-1
90. Miskolczi Z, Smith MP, Rowling EJ, Ferguson J, Barriuso J, Wellbrock C. Collagen abundance controls melanoma phenotypes through lineage-specific microenvironment sensing. Oncogene (2018) 7:23. doi: 10.1038/s41388-018-0209-0
91. Gilkes DM, Semenza GL, Wirtz D. Hypoxia and the extracellular matrix: drivers of tumour metastasis. Nat Rev Cancer (2014) 4:6. doi: 10.1038/nrc3726
92. Bertero T, Gaggioli C. Mechanical forces rewire metabolism in the tumor niche. Mol Cell Oncol (2019) 6:3. doi: 10.1080/23723556.2019.1592945
93. Romani P, Valcarcel-Jimenez L, Frezza C, Dupont S. Crosstalk between mechanotransduction and metabolism. Nat Rev Mol Cell Biol (2020) 22:1. doi: 10.1038/s41580-020-00306-w
94. Riedel A, Shorthouse D, Haas L, Hall BA, Shields J. Tumor induced stromal reprogramming drives lymph node transformation. Nat Immunol (2016) 17:9. doi: 10.1038/ni.3492
95. Rovera C, Berestjuk I, Lecacheur M, Tavernier C, Diazzi S, Pisano S, et al. Secretion of interleukin-1 by dedifferentiated melanoma cells inhibits JAK1-STAT3-driven actomyosin contractility of lymph node fibroblastic reticular cells. Cancer Res (2022) 82:9. doi: 10.1158/0008-5472.CAN-21-0501
96. Pickup MW, Mouw JK, Weaver VM. The extracellular matrix modulates the hallmarks of cancer. EMBO Rep (2014) 15:12. doi: 10.15252/embr.201439246
97. Winkler J, Abisoye-Ogunniyan A, Metcalf KJ, Werb Z. Concepts of extracellular matrix remodelling in tumour progression and metastasis. Nat Commun (2020) 11:1. doi: 10.1038/s41467-020-18794-x
98. Socovich AM, Naba A. The cancer matrisome: From comprehensive characterization to biomarker discovery. Semin Cell Dev Biol (2019) 89:5. doi: 10.1016/j.semcdb.2018.06.005
99. Hynes RO, Naba A. Overview of the matrisome-an inventory of extracellular matrix constituents and functions. Perspect Biol (2012) 4:1. doi: 10.1101/cshperspect.a004903
100. Wu L, Zhu Y. The function and mechanisms of action of LOXL2 in cancer. Int J Mol Med (2015) 36:5. doi: 10.3892/ijmm.2015.2337
101. Frantz C, Stewart KM, Weaver VM. The extracellular matrix at a glance. J Cell Sci (2010) 123:24. doi: 10.1242/jcs.023820
102. Kai FB, Drain AP, Weaver VM. The extracellular matrix modulates the metastatic journey. Dev Cell (2019) 49:3. doi: 10.1016/j.devcel.2019.03.026
103. Leight JL, Drain AP, Weaver VM. Extracellular matrix remodeling and stiffening modulate tumor phenotype and treatment response. Annu Rev Cancer Biol (2017) 1:1. doi: 10.1146/annurev-cancerbio-050216-034431
104. Kramer RH, Marks N. Identification of integrin collagen receptors on human melanoma cells. J Biol Chem (1989) 8:4684–8.
105. Chelberg MK, McCarthy JB, Skubitz APN, Furcht LT, Tsilibary EC. Characterization of a synthetic peptide from type IV collagen that promotes melanoma cell adhesion, spreading, and motility. J Cell Biol (1990) 1:261–70. doi: 10.1083/jcb.111.1.261
106. Pfaff M, Aumailley M, Specks U, Knolle J, Zerwes HG, Timpl R. Integrin and arg-Gly-Asp dependence of cell adhesion to the native and unfolded triple helix of collagen type VI. Exp Cell Res (1993) 1:167–76. doi: 10.1006/excr.1993.1134
107. Pasco S, Brassart B, Ramont L, Maquart FX, Monboisse JC. Control of melanoma cell invasion by type IV collagen. Cancer Detect Prev (2005) 29:3. doi: 10.1016/j.cdp.2004.09.003
108. Etoh T, Beyers HR, Mihm MC. Integrin expression in malignant melanoma and their role in cell attachment and migration on extracellular matrix proteins. J Dermatol (1992) 11:841–6. doi: 10.1111/j.1346-8138.1992.tb03794.x
109. Li C, McCarthy JB, Furcht LT, Fields GB. An all-d amino acid peptide model of α1(IV)531-543 from type IV collagen binds the α3β1 integrin and mediates tumor cell adhesion, spreading, and motility. Biochemistry (1997) 49:15404–10. doi: 10.1021/bi971817g
110. Kramer RH, Vu M, Cheng YF, Ramos DM. Integrin expression in malignant melanoma. Cancer Metastasis Rev (1991) 1:49–59. doi: 10.1007/BF00046843
111. Romayor I, Badiola I, Olaso E. Inhibition of DDR1 reduces invasive features of human A375 melanoma, HT29 colon carcinoma and SK-HEP hepatoma cells. Cell Adh Migr (2020) 14:1. doi: 10.1080/19336918.2020.1733892
112. Poudel B, Lee YM, Kim DK. DDR2 inhibition reduces migration and invasion of murine metastatic melanoma cells by suppressing MMP2/9 expression through ERK/NF-κB pathway. Acta Biochim Biophys Sin (2015) 47:4. doi: 10.1093/abbs/gmv005
113. Reger de Moura C, Battistella M, Sohail A, Caudron A, Feugeas JP, Podgorniak MP, et al. Discoidin domain receptors: A promising target in melanoma. Pigment Cell Melanoma Res (2019) 32:5. doi: 10.1111/pcmr.12809
114. Ray A, Morford RK, Ghaderi N, Odde DJ, Provenzano PP. Dynamics of 3D carcinoma cell invasion into aligned collagen. Integr Biol (2018) 10:2. doi: 10.1039/c7ib00152e
115. Xie R, Li B, Jia L, Li Y. Identification of core genes and pathways in melanoma metastasis via bioinformatics analysis. Int J Mol Sci (2022) 23:2. doi: 10.3390/ijms23020794
116. Erdogan B, Ao M, White LM, Means AL, Brewer BM, Yang L, et al. Cancer-associated fibroblasts promote directional cancer cell migration by aligning fibronectin. J Cell Biol (2017) 216:11. doi: 10.1042/BST20160387
117. Su S, Chen J, Yao H, Liu J, Yu S, Lao L, et al. CD10+GPR77+ cancer-associated fibroblasts promote cancer formation and chemoresistance by sustaining cancer stemness. Cell (2018) 172:4. doi: 10.1016/j.cell.2018.01.009
118. Ahmadzadeh H, Webster MR, Behera R, Valencia AMJ, Wirtz D, Weeraratna AT, et al. Modeling the two-way feedback between contractility and matrix realignment reveals a nonlinear mode of cancer cell invasion. Proc Natl Acad Sci (2017) 114:9. doi: 10.1073/pnas.1617037114
119. Lee HO, Mullins SR, Franco-Barraza J, Valianou M, Cukierman E, Cheng JD. FAP-overexpressing fibroblasts produce an extracellular matrix that enhances invasive velocity and directionality of pancreatic cancer cells. BMC Cancer (2011) 11:1. doi: 10.1186/1471-2407-11-245
120. Kwon Y, Cukierman E, Godwin AK. Differential expressions of adhesive molecules and proteases define mechanisms of ovarian tumor cell matrix Penetration/Invasion. PloS One (2011) 6:4. doi: 10.1371/journal.pone.0018872
121. Acerbi I, Cassereau L, Dean I, Shi Q, Au A, Park C, et al. Human breast cancer invasion and aggression correlates with ECM stiffening and immune cell infiltration. Integr Biol (2015) 7:10. doi: 10.1039/c5ib00040h
122. Berger AJ, Renner CM, Hale I, Yang X, Ponik SM, Weisman PS, et al. Scaffold stiffness influences breast cancer cell invasion via EGFR-linked mena upregulation and matrix remodeling. Matrix Biol (2020) 85:1. doi: 10.1016/j.matbio.2019.07.006
123. Koorman T, Jansen KA, Khalil A, Haughton PD, Visser D, Rätze MAK, et al. Spatial collagen stiffening promotes collective breast cancer cell invasion by reinforcing extracellular matrix alignment. Oncogene (2022) 41(17):2458–69. doi: 10.1038/s41388-022-02258-1
124. Rathore M, Girard C, Ohanna M, Tichet M, Ben Jouira R, Garcia E, et al. Cancer cell-derived long pentraxin 3 (PTX3) promotes melanoma migration through a toll-like receptor 4 (TLR4)/NF-κB signaling pathway. Oncogene (2019) 38:30. doi: 10.1038/s41388-019-0848-9
125. Kaushik S, Pickup MW, Weaver VM. From transformation to metastasis: deconstructing the extracellular matrix in breast cancer. Cancer Metastasis Rev (2016) 35:4. doi: 10.1007/S10555-016-9650-0
126. Pfisterer K, Shaw LE, Symmank D, Weninger W. The extracellular matrix in skin inflammation and infection. Front Cell Dev Biol (2021) 9:682414. doi: 10.3389/fcell.2021.682414
127. Berestjuk I, Lecacheur M, Carminati A, Diazzi S, Rovera C, Prod’homme V, et al. Targeting discoidin domain receptors DDR1 and DDR2 overcomes matrix-mediated tumor cell adaptation and tolerance to BRAF-targeted therapy in melanoma. EMBO Mol Med (2022) 14:2. doi: 10.15252/emmm.201911814
128. Marusak C, Thakur V, Li Y, Freitas JT, Zmina PM, Thakur VS, et al. Targeting extracellular matrix remodeling restores BRAF inhibitor sensitivity in BRAFi resistant melanoma. Clin Cancer Res (2020) 26:22. doi: 10.1158/1078-0432.CCR-19-2773
129. Özdemir BC, Pentcheva-Hoang T, Carstens JL, Zheng X, Wu CC, Simpson TR, et al. Depletion of carcinoma-associated fibroblasts and fibrosis induces immunosuppression and accelerates pancreas cancer with reduced survival. Cancer Cell (2014) 25:6. doi: 10.1016/j.ccr.2014.04.005
130. Biffi G, Oni TE, Spielman B, Hao Y, Elyada E, Park Y, et al. Il1-induced Jak/STAT signaling is antagonized by TGFβ to shape CAF heterogeneity in pancreatic ductal adenocarcinoma. Cancer Discovery (2019) 9:2. doi: 10.1158/2159-8290.CD-18-0710
131. Albrengues J, Bourget I, Pons C, Butet V, Hofman P, Tartare-Deckert S, et al. LIF mediates proinvasive activation of stromal fibroblasts in cancer. Cell Rep (2014) 7:5. doi: 10.1016/j.celrep.2014.04.036
132. Abyaneh HS, Regenold M, McKee TD, Allen C, Gauthier MA. Towards extracellular matrix normalization for improved treatment of solid tumors. Theranostics (2020) 10:4. doi: 10.7150/thno.39995
133. Zheng J, Gao P. Toward normalization of the tumor microenvironment for cancer therapy. Integr Cancer Ther (2019) 18. doi: 10.1177/1534735419862352
134. Dong L, Lin F, Wu W, Liu Y, Huang W. Verteporfin inhibits YAP-induced bladder cancer cell growth and invasion via hippo signaling pathway. Int J Med Sci (2018) 15:6. doi: 10.7150/ijms.23460
135. Olsson PO, Gustafsson R, In’t Zandt R, Friman T, Maccarana M, Tykesson E, et al. The tyrosine kinase inhibitor imatinib augments extracellular fluid exchange and reduces average collagen fibril diameter in experimental carcinoma. Mol Cancer Ther (2016) 15:10. doi: 10.1158/1535-7163.MCT-16-0026
136. Janjanam J, Pano G, Wang R, Minden-Birkenmaier BA, Breeze-Jones H, Baker E, et al. Matricellular protein WISP2 is an endogenous inhibitor of collagen linearization and cancer metastasis. Cancer Res (2021) 81:22. doi: 10.1158/0008-5472.CAN-20-3982
137. Tu MM, Lee FYF, Jones RT, Kimball AK, Saravia E, Graziano RF, et al. Targeting DDR2 enhances tumor response to anti–PD-1 immunotherapy. Sci Adv (2019) 5:2. doi: 10.1126/sciadv.aav2437
138. Nicolas-Boluda A, Vaquero J, Vimeux L, Guilbert T, Barrin S, Kantari-Mimoun C, et al. Tumor stiffening reversion through collagen crosslinking inhibition improves T cell migration and anti-PD-1 treatment. elife (2021) 10. doi: 10.7554/eLife.58688
139. Kato R, Haratani K, Hayashi H, Sakai K, Sakai H, Kawakami H, et al. Nintedanib promotes antitumour immunity and shows antitumour activity in combination with PD-1 blockade in mice: potential role of cancer-associated fibroblasts. Br J Cancer (2021) 124:5. doi: 10.1038/s41416-020-01201-z
140. Guan X, Lin L, Chen J, Hu Y, Sun P, Tian H, et al. Efficient PD-L1 gene silence promoted by hyaluronidase for cancer immunotherapy. J Control Release (2019) 293:104–12. doi: 10.1016/j.jconrel.2018.11.022
141. Mason LD, Chava S, Reddi KK, Gupta R. The BRD9/7 inhibitor TP-472 blocks melanoma tumor growth by suppressing ECM-mediated oncogenic signaling and inducing apoptosis. Cancers (2021) 13:21. doi: 10.3390/cancers13215516
142. Fejza A, Polano M, Camicia L, Poletto E, Carobolante G, Toffoli G, et al. The efficacy of anti-PD-L1 treatment in melanoma is associated with the expression of the ECM molecule EMILIN2. Int J Mol Sci (2021) 22:14. doi: 10.3390/ijms22147511
143. Jensen C, Madsen DH, Hansen M, Schmidt H, Svane IM, Karsdal MA, et al. Non-invasive biomarkers derived from the extracellular matrix associate with response to immune checkpoint blockade (anti-CTLA-4) in metastatic melanoma patients. J Immunother Cancer (2018) 6:1. doi: 10.1186/s40425-018-0474-z
Keywords: tumor stroma cross-talk, ECM remodeling, ECM mediated therapeutic resistance, melanoma associated fibroblasts, melanoma progression and phenotypic switching
Citation: Popovic A and Tartare-Deckert S (2022) Role of extracellular matrix architecture and signaling in melanoma therapeutic resistance. Front. Oncol. 12:924553. doi: 10.3389/fonc.2022.924553
Received: 20 April 2022; Accepted: 15 August 2022;
Published: 02 September 2022.
Edited by:
Shilpa S Dhar, University of Texas MD Anderson Cancer Center, United StatesReviewed by:
Elizabeth Martin, Louisiana State University, United States;Banu Bayyurt –Kocabas, National Heart, Lung, and Blood Institute (NIH), United StatesCopyright © 2022 Popovic and Tartare-Deckert. This is an open-access article distributed under the terms of the Creative Commons Attribution License (CC BY). The use, distribution or reproduction in other forums is permitted, provided the original author(s) and the copyright owner(s) are credited and that the original publication in this journal is cited, in accordance with accepted academic practice. No use, distribution or reproduction is permitted which does not comply with these terms.
*Correspondence: Ana Popovic, QW5hLlBPUE9WSUNAdW5pdi1jb3RlZGF6dXIuZnI=; Sophie Tartare-Deckert, c29waGllLlRBUlRBUkUtREVDS0VSVEB1bml2LWNvdGVkYXp1ci5mcg==
Disclaimer: All claims expressed in this article are solely those of the authors and do not necessarily represent those of their affiliated organizations, or those of the publisher, the editors and the reviewers. Any product that may be evaluated in this article or claim that may be made by its manufacturer is not guaranteed or endorsed by the publisher.
Research integrity at Frontiers
Learn more about the work of our research integrity team to safeguard the quality of each article we publish.