- 1Department of Internal Medicine IV (Gastroenterology, Hepatology, and Infectious Diseases), Jena University Hospital, Jena, Germany
- 2Else Kröner Graduate School for Medical Students “Jena School for Ageing Medicine (JSAM)”, Jena University Hospital, Jena, Germany
- 3Department of Urology, Jena University Hospital, Jena, Germany
IQ motif-containing GTPase-activating proteins (IQGAPs) are a class of scaffolding proteins, including IQGAP1, IQGAP2, and IQGAP3, which govern multiple cellular activities by facilitating cytoskeletal remodeling and cellular signal transduction. The role of IQGAPs in cancer initiation and progression has received increasing attention in recent years, especially in hepatocellular carcinoma (HCC), where the aberrant expression of IQGAPs is closely related to patient prognosis. IQGAP1 and 3 are upregulated and are considered oncogenes in HCC, while IQGAP2 is downregulated and functions as a tumor suppressor. This review details the three IQGAP isoforms and their respective structures. The expression and role of each protein in different liver diseases and mainly in HCC, as well as the underlying mechanisms, are also presented. This review also provides a reference for further studies on IQGAPs in HCC.
1 Introduction
IQ motif-containing GTPase-activating proteins (IQGAPs) are a family of evolutionarily conserved proteins found in eukaryotic cells, ranging from yeast to humans, and consist of three closely related homologs; IQGAP1, IQGAP2, and IQGAP3. These homologs comprise multiple domains involved in regulating a broad spectrum of biological processes such as cytoskeleton remodeling, cytokinesis, protein trafficking, cell adhesion, proliferation, migration, and tumorigenesis (1–6). Aberrant IQGAP expression has been extensively reported in a range of malignancies, including liver, gastric, lung, breast, and colon cancers, and is highly related to poor clinical characteristics and prognosis (7–10).
Globally, liver cancer is a significant public health threat with increasing prevalence. By 2025, liver cancer is anticipated to affect more than one million individuals annually (11). Hepatocellular carcinoma (HCC), the most common subtype of primary liver cancer, which is the sixth most frequently diagnosed type of cancer and the third most common cause of cancer-related mortality worldwide in 2020 (12). Several imperative risk factors such as chronic Hepatitis B and C virus (HBV and HCV) infections, alcoholic and non-alcoholic liver diseases, e.g., non-alcoholic fatty liver disease (NAFLD) and the advanced state of NAFLD termed as non-alcoholic steatohepatitis (NASH), and hereditary hemochromatosis have been described for HCC (13). Apart from these risk factors, any other causes leading to liver cirrhosis, including HBV and HCV, are also identified as crucial risk factors for HCC (11, 14). Over the last decade, the discovery of novel biomarkers and innovation in imaging technologies have led to tremendous progress in the management and therapy of HCC. Hepatectomy and liver transplantation have been the mainstays of treatment for patients with HCC (15). Additionally, alternative adjuvant therapies such as radiofrequency ablation, transarterial chemoembolization, and immunotherapy have dramatically improved patient prognosis (11).
IQGAPs are important molecular factors that play a comprehensive and distinctive role in HCC initiation and progression. IQGAP1 is overexpressed in HCC and contributes to cancer development and advancement (16, 17). IQGAPs have complex interactions with several different factors at the molecular level and thus play a vital role in cancer progression. Likewise, IQGAP1 can bind to the cell cycle regulators and might boost cell division and enhance the invasive and migratory abilities of HCC cells, as shown for the Huh-7 cell line (18). Moreover, IQGAP1 also interacts with certain transcription factors and co-activators and affects the signaling pathways involved in apoptosis and survival processes (19). Additionally, IQGAP1 has been shown to render anoikis resistance and metastasis in HCC (19, 20). Similar to IQGAP1, IQGAP3 is considered an oncogene in HCC, where it supports intrahepatic and distant metastasis and epithelial-to-mesenchymal transition (EMT) (21, 22). In contrast to isoform 1 and 3 of IQGAP, IQGAP2 is considered a tumor suppressor in HCC since its expression coincides with patient prognosis and is decreased in HCC tissues (23). Furthermore, IQGAP2-deficient mice were more prone to spontaneously growing HCC (24). Therefore, all these findings indicate that IQGAPs are potential therapeutic targets in HCC. This review details the three IQGAP isoforms and their distinct structures. In addition, we compare the characteristics of IQGAPs and summarize their expression and functions in different liver diseases and mainly in HCC, as well as their associated mechanisms.
2 The Isoforms of IQGAPS
Although IQGAPs share a similar domain composition, they differ in tissue expression, subcellular localization, and function. IQGAP1, the most extensively investigated isoform among IQGAPs, has gained considerable attention since its discovery in 1994 (25). IQGAP1 is a ubiquitously expressed scaffold protein involved in various vital cellular functions (4, 5). IQGAP1 has been postulated to maintain matrix homeostasis via collagen phagocytosis in physiological tissue remodeling (26). Furthermore, IQGAP1 appears to be an exceptionally attractive therapeutic target because it acts as a hub for signaling pathways involved in cancer progression (20, 27–29).
IQGAP2 was initially identified in 1996 as a large cytoplasmic scaffold protein that is predominantly expressed in the liver but also in the prostate, kidney, stomach, testis, and platelets (30). IQGAP2 is a tumor suppressor, as a decreased expression has been observed in human breast cancer and HCC (23, 31). Moreover, IQGAP2-mutant mice are susceptible to developing HCC. Additionally, IQGAP2 inhibits EMT and angiogenesis and promotes apoptosis in breast cancer. Thereby, low expression levels of IQGAP2 results in a poor prognosis for patients (7, 31).
IQGAP3 has received scant attention despite its discovery in 2007 (32) and its detection in various organs, including the liver, stomach, ovaries, prostate, breast, pancreas, and lung (8, 33–38). IQGAP3 is mainly expressed in brain (39) and is critical for regulation of neurite outgrowth of the neurons (32). In addition to regulating mitosis progression, genome integrity, and stability, IQGAP3 is necessary and sufficient for proper cell proliferation and migration (35, 40). Accordingly, IQGAP3 is classified as an oncogene owing to its intimate association with tumorigenesis and metastasis. Its expression is inversely linked with clinical characteristics and survival in most cancers (8, 38, 41, 42).
3 The Molecular Domains of IQGAPS
IQGAPs have a high degree of amino acid sequence homology and a comparable domain structure (Figure 1), with IQGAP1 having 62% and 59% amino acid sequence identity to IQGAP2 and IQGAP3, respectively (43, 44). IQGAPs contain six distinct functional domains depending on the amino acid sequence. These diverse domains allow them to bind to a variety of partners and modulate the spatiotemporal distribution of various signal transduction complexes (9, 45).
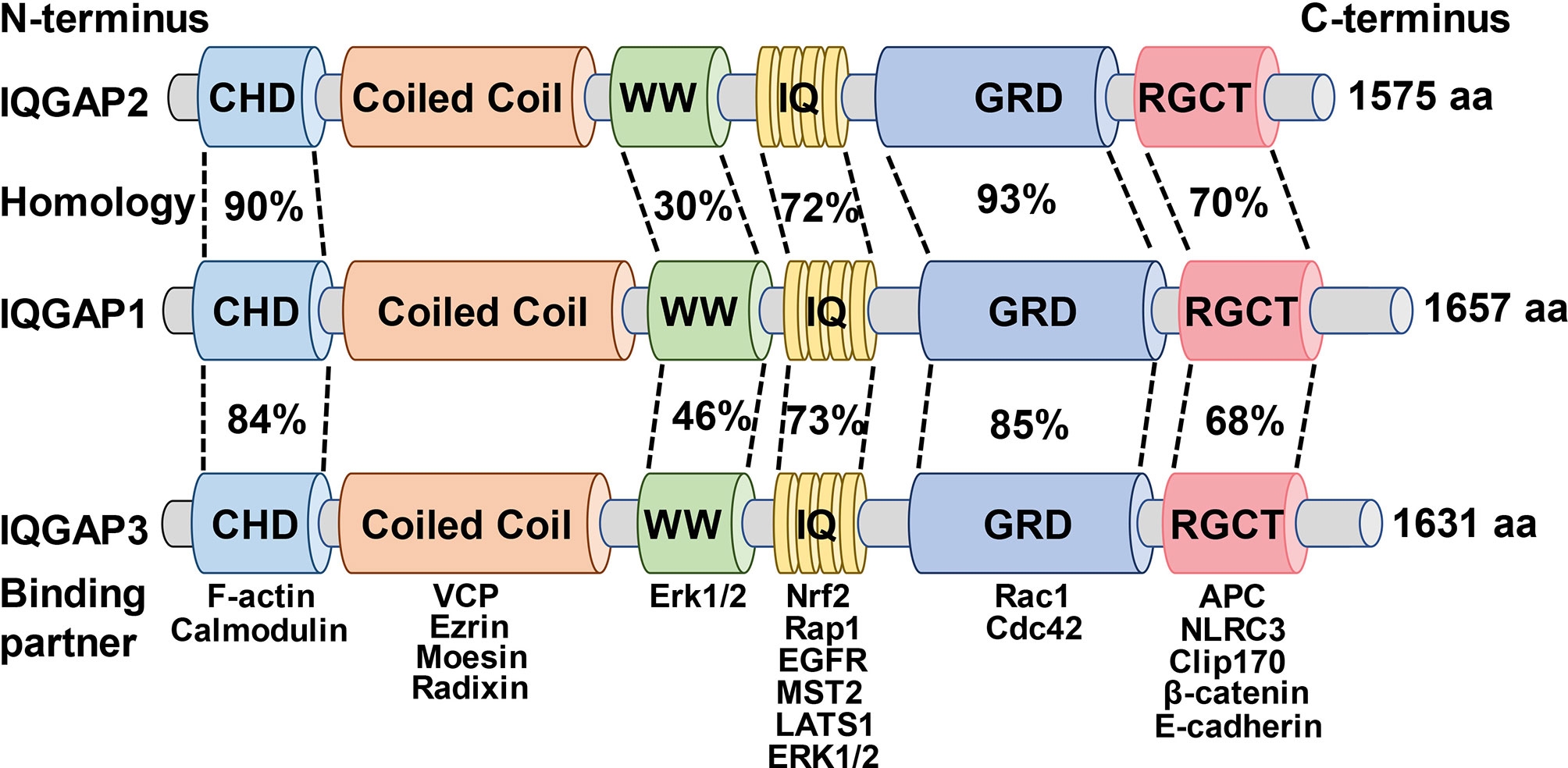
Figure 1 Schematic illustration of the domain organization and amino acid homology of the IQGAPs family and the binding partners of the respective domains.
Starting from the N-terminus, IQGAPs comprise a calmodulin homology domain (CHD) that mediates actin-binding (46). For instance, the interaction between the CHD of IQGAP1 and actin filaments governs the cytoskeleton modulation to facilitate actin binding and polymerization, thus further regulating cell division, cell migration, and the stability of cell-cell contacts (47). Moreover, it has been shown previously that IQGAP1 dynamically contributes to the formation of the spine head in the cultured rat hippocampal neurons through CHD (48).
Following the CHD is the coiled-coil (CC), also known as heptad domains, which are characterized by the presence of repetitive hydrophobic and charged amino acids (49). The CC repeat sequence enables IQGAP1 and IQGAP2 to bind to the ERM protein family (ezrin, radixin, and moesin) that crosslinks actin-based cytoskeletons to plasma membranes and participates in multiple intracellular signaling pathways, reinforcing the role of IQGAPs as cytoskeletal regulators and signal transduction hubs (50, 51). Additionally, the CC repeat region of all IQGAPs isoforms stimulates neural development and spine morphogenesis via interaction with valosin containing protein (VCP) which also suggests a crucial role of IQGAPs in the pathophysiology of neurodegenerative diseases (52).
The polyproline protein-protein (WW) domain contains two functionally conserved tryptophans, W, which interacts with other proteins, including proline-rich regions. WW domain of IQGAP1, through a polyproline motif, binds to classical MAP kinases (MAPK) and activates downstream signaling pathways and advances tumor development, growth, and invasion (53, 54). Likewise, interruption of IQGAP1-kinase- connections by ectopic expression of WW peptide in mice selectively suppressed Ras-MAPK -mediated oncogenesis and tumor invasion. Moreover, this effect also remarkably improves the life span of tumor-bearing mice (54). However, a later study reported contradictory findings that the WW domain of IQGAP1 was neither sufficient nor essential for binding to MAPK, whereas the IQ domain that immediately follows WW domain was both adequate and necessary for high-affinity IQGAP1-kinase interaction (55).
IQ domain comprises of four tandem isoleucine-glutamine (IQ) motifs. This domain interacts with calmodulin, a calcium-sensing protein capable of binding to and regulating a diverse array of target proteins (56). The IQ domain also interacts with protein kinases and cell surface receptors such as epidermal growth factor receptor (EGFR) (55, 57–60). The IQ domain of IQGAP1 activates MAPK signaling cascade by binding to EGFR, which is associated with epidermal cancer progression and invasion (61). Subsequently, IQGAP1-IQ motif decoy peptide supplementation in vivo inhibited the oncogenic signaling pathway and carcinogenesis without compromising normal epidermal proliferation or differentiation (61).
The IQ domain is followed by a GTPase activation-related structural domain (GRD) that is responsible for the nomenclature of IQGAPs (49). This domain is highly homologous to the functional components of Ras GTPase-activating proteins (GAPs) and interacts with small GTPases (3, 9, 62). The crystal structure of GRD showed that threonine replaces the catalytic “arginine finger” required for GTP hydrolysis; thus, it does not have GAP function, failing to hydrolyze GTP but rather stabilizing the GTP-bound protein in its active state (63). Such interaction between GRD domain of IQGAP2 and small GTPases is mechanistically crucial for the polymerization of actin filaments during cancer progression (64, 65).
Finally, IQGAPs contain a RasGAP C-terminal (RGCT) structural domain that significantly contributes to cell-cell adhesion, cell polarization, and directional migration by binding to a variety of proteins, including E-cadherin and β-catenin (66–69). Furthermore, IQGAP1 enhanced extracellular matrix (ECM) degradation by binding to the exocyst subunits via RGCT domain to prime tumor cell metastasis and invasion, while the elimination of the exocyst binding site abrogated the enhancement of IQGAP1-induced ECM degradation (70, 71). This indicates that IQGAP1-regulated cytoskeletal remodeling plays an aggressive role in tumor progression. Likewise, mutations of the RGCT can severely impair the interaction of IQGAP1 with small GTPases; therefore, complying that the domains adjacent to GRD are also crucial for the binding of IQGAPs with small GTPases of Rho family proteins (72–75).
4 The Relationship Between IQGAPS and Liver Diseases
As delineated above, IQGAP scaffold proteins are crucial for a variety of vital cellular processes, and their molecular interactions with other biological molecules are very important for the development and progression of cancer. Likewise, role of IQGAPs has been quite extensively explored in various cancers including HCC. However, the investigations for the implications of IQGAPs in other liver diseases such as fatty liver disease, fibrosis, and cirrhosis are still too scarce and need more scientific research to explore the role and importance of these scaffold proteins in liver diseases (76–79).
4.1 Fatty Liver Disease
Fatty liver disease is a chronic liver disease also worthy of attention, affecting more than 25% of the population globally (80). Moreover, studies have shown that IQGAPs play an essential role in fatty acid and glucose metabolism (76–78). Likewise, a recent study demonstrated that in IQGAP1 knock out mice (Iqgap1–/–), fatty acid oxidation and ketogenesis were significantly reduced compared to the wild type (WT) counterparts. Moreover, livers from Iqgap1–/– mice showed pale appearance and exhibited microsteatosis under a high-fat diet, concomitant with elevated levels of hepatic triglyceride than WT mice implying the compromised roles of peroxisome proliferator-activated receptor alpha (PPAR-α) and mammalian target of rapamycin (mTOR), essential regulators of fatty acid β-oxidation in livers of Iqgap1–/– mice (76). In addition, a previous study by Chawla and colleagues showed that IQGAP1 null mice exhibited impaired glucose tolerance and were unable to clear glucose as efficiently as WT mice. The defective glucose homeostasis might be due to impaired insulin signaling in the absence of IQGAP1 (77). These data imply that IQGAP1 plays a significant role in the complex metabolic regulatory pathways, especially that of fatty acid, glycogen, and insulin signaling in the liver (76, 77). Likewise, the role of IQGAP2 in metabolic homeostasis was demonstrated by Vaitheesvaran and colleagues. IQGAP2 null mice fed with a standard laboratory diet displayed impaired glucose and fatty acid metabolism, suggesting that IQGAP2 deletion results in a pre-diabetic hepatic environment paving the way for the NAFLD manifestation. Moreover, Iqgap2-/- mice had high blood glucose levels and were overweight compared to the WT mice (81). However, another previous study showed that abrogation of IQGAP2 protects against diet-induced hepatic steatosis due to compromised uptake of fatty acid. Hepatocytes from Iqgap2-/- mice fed with high-fat diet displayed selective loss of the facilitated phase of long-chain fatty acids (LCFA) uptake and retention of the intact passive phase of LCFA uptake. Additionally, Iqgap2-/- livers expressed remarkably lower de novo synthesis of lipids in the hepatocytes than the WT counterparts. Similarly, livers from IQGAP2 null mice depicted improved sensitivity to insulin. These findings suggest the fundamental role of IQGAP2 in the coordination of metabolic physiological processes involved in the cellular uptake of functional fatty acid and lipid, lipid synthesis and processing, and, plausibly, regulating glucose levels (78). Apart from the delineated roles of IQGAP1 and IQGAP2, additional research is required to further explore the role of IQGAPs in the pathophysiology of fatty liver disease.
4.2 Fibrosis
Fibrotic liver occurs in most types of chronic liver ailments, e.g., chronic HBV and HCV infections and alcoholic fatty liver disease, and is characterized by the excessive buildup of ECM proteins including collagen. Fibrosis is an aberrant tissue restoration process in response to long-term and persistent liver injuries that may further develop into liver cirrhosis, failure, or cancer if left untreated. Hitherto, chronic liver diseases coupled with fibrotic liver have resulted in remarkable worldwide morbidity and mortality (82–84). Since IQGAP1 is one of the vital scaffolding proteins that have established pivotal roles in cytoskeleton remodeling and rearrangements of cellular networks and various vital cellular processes. It implies that IQGAP1 might have a potential role in the modulation of liver fibrosis. Likewise, in a recent study, mRNA and protein levels of IQGAP1 were shown to be significantly elevated in CCl4-induced liver fibrosis mice and TNF-α-treated hepatic stellate cell (HSC) line, LX-2 cells, which might be related to the development and advancement of liver fibrosis. Furthermore, the overexpression of IQGAP1 in activated LX-2 cells promoted the secretion of inflammatory cytokines. These findings suggest the putative role of IQGAP1 in liver inflammation and fibrogenesis (85). The increased levels of IQGAP1 in fibrotic liver were also confirmed by Ma and colleagues, who further demonstrated the cell type fraction responsible for such increase (79). IQGAP1 expression was found to be remarkably elevated in non-parenchymal cells and myofibroblasts during murine liver fibrosis (79). Sphingosine 1-phosphate (S1P) mediated bone marrow mesenchymal stromal cells (BMSCs) migration from bone marrow to the injured liver is one of the primary sources of myofibroblasts (86). Likewise, Ma and colleagues showed that IQGAP1 mediated SIP-induced BMSC migration and motility (79, 86). S1P induces membrane translocation of IQGAP1 and promotes the interaction between IQGAP1 and Cdc42/Rac1 to facilitate BMSC migration to the liver. Moreover, the knockdown of IQGAP1 significantly reduced cell viability and migratory capacity in BMSC cells (79).
4.3 Viral Hepatitis
Viral infections of liver, especially HBV and HCV infections, are one of the top infectious diseases and are responsible for the demise of 1.4 million people per annum worldwide (87, 88). IQGAP2, mainly expressed in the liver, is shown to have a novel role in the innate antiviral response. The functional analysis revealed that IQGAP2 regulates many of the interferon (IFN) required anti-hepatitis C viral genes together with RelA subunit of nuclear factor κB (NF-κB). Following IFN treatment, IQGAP2 physically interacts with RelA to mediate the early activation of RelA and the downstream targets of NF-κB, which ultimately protects against HCV infection (89). Several studies have also suggested the involvement of IQGAPs in HBV-induced HCC, as detailed in the section “Action mechanisms of IQGAPs in HBV-caused HCC”.
4.4 Hepatocellular Carcinoma
IQGAPs have attracted increasing interest in cancer research because of their fundamental contribution to cytoskeletal remodeling and signal transduction. Numerous studies have shown an association between IQGAPs and HCC initiation, metastasis, and recurrence, as well as poor prognosis in patients with HCC (10, 16–24, 29, 81, 90–98) (details in Table 1).
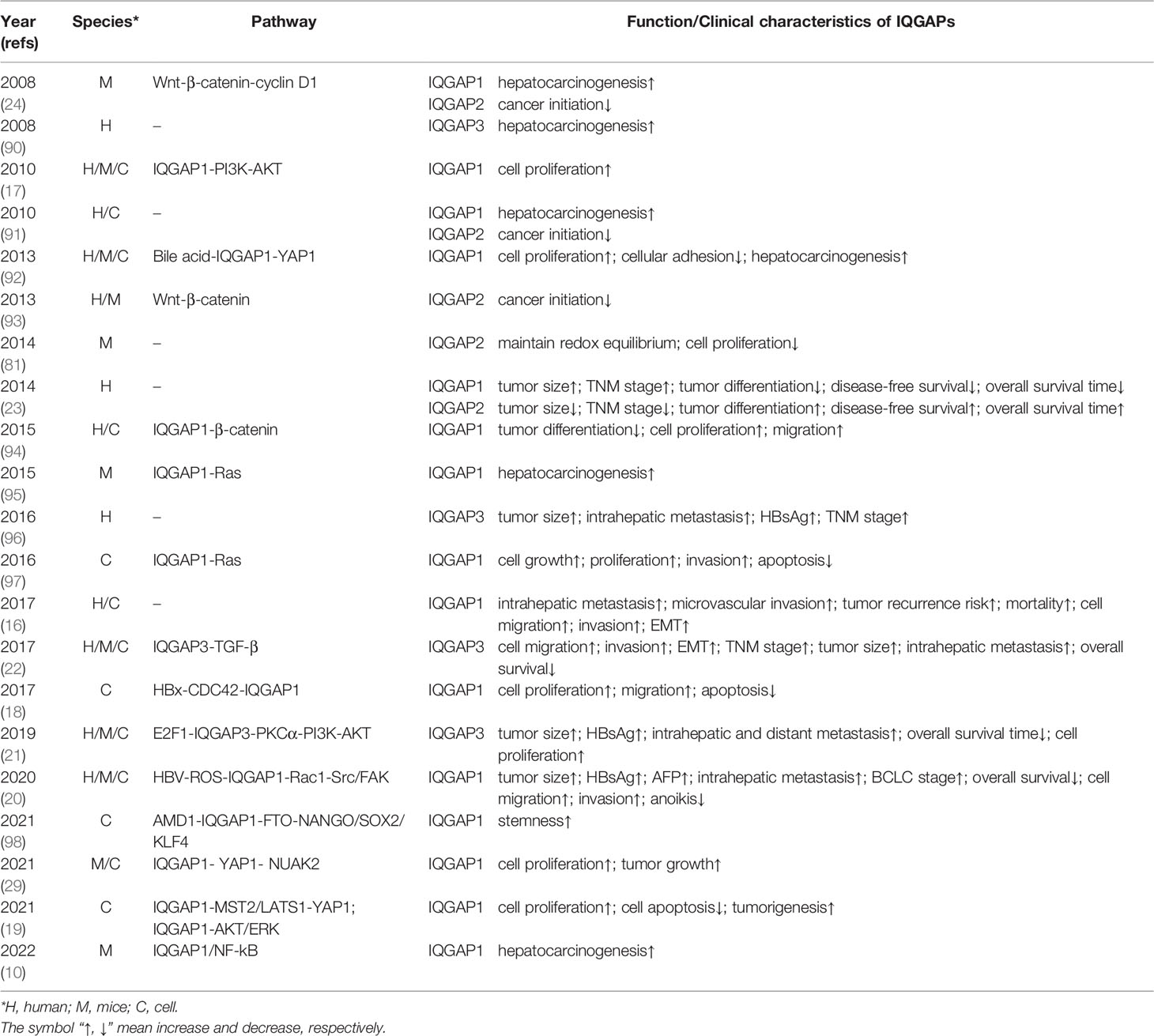
Table 1 Summary of the role of IQGAPs in tumor oncogenesis and related function/clinical characteristics.
IQGAP1 is considered an oncogene in HCC as its mRNA and protein levels are elevated in human HCC tissues compared to para-tumor and normal liver tissues. Moreover, high IQGAP1 expression is associated with poor clinical outcomes (16, 17, 23, 91). In HCC tissues, IQGAP1 expression is positively correlated with tumor size, number, stage, and HBV surface antigen (HBsAg) and alpha-fetoprotein (AFP) expression but inversely correlated with tumor differentiation (16, 23). IQGAP1 promotes microvascular invasion and distant metastasis in HCC, accounting for the higher postoperative recurrence rate and shorter disease-free survival and overall survival of patients with elevated IQGAP1 expression (16). IQGAP1 expression is also upregulated in HBV-induced HCC and is linked to poor prognosis (20).
Consistent with the predominant expression of IQGAP2 in the liver, convincing evidence supports a tumor suppressor role for IQGAP2 in HCC. Decreased IQGAP2 expression is observed in most patients with HCC and is associated with larger tumor size, advanced tumor stage, and poorer tumor differentiation, as well as shorter postoperative tumor-free survival and overall survival after hepatectomy (23, 91). Both low IQGAP2 and high IQGAP1 levels have been reported as independent prognostic risk factors for poor postoperative survival in patients with HCC. Furthermore, patients with IQGAP1+/IQGAP2- tumors have the worst prognosis, whereas those with IQGAP1-/IQGAP2+ tumors have the best prognosis (23). Thus, positive IQGAP1 and negative IQGAP2 are substantially related to HCC progression, validating the distinct functions of IQGAP1 and IQGAP2 in HCC.
IQGAP3, like IQGAP1, is also considered an oncogene in HCC. IQGAP3 is overexpressed in HCC tissues. Its expression is associated with larger tumor size, advanced tumor stage, and poor tumor differentiation (22, 96). IQGAP3 promotes intrahepatic and extrahepatic metastasis in HCC, which dramatically shortens patient survival time (21, 22). Furthermore, IQGAP3 is a novel biomarker for HCC screening and diagnosis and is superior to AFP for detecting small HCCs (96). IQGAP3 can be used as a complementary biomarker of AFP to improve the accuracy of AFP-negative HCC. The combination of AFP, IQGAP3, and chaperonin containing TCP1 complex subunit 3 (CCT3) significantly enhanced the discriminatory ability of HCC compared to AFP alone (96).
5 Action Mechanism of IQGAPS in HCC
Aberrant activation of multiple signaling pathways in HCC, such as the Wnt/β-catenin, Hippo, transforming growth factor β (TGF-β), receptor tyrosine kinase (RTK)-activated phosphatidylinositol 3-kinase/AKT/mTOR (PI3K/AKT/mTOR), and Ras/Raf/MEK/ERK (also known as the MAPK/ERK) pathways, leads to uncontrolled cell division, differentiation, proliferation, motility, and apoptosis (Figure 2). Mounting studies have shown that dysregulated IQGAP expression promotes HCC progression and plays a crucial role in HBV-induced HCC.
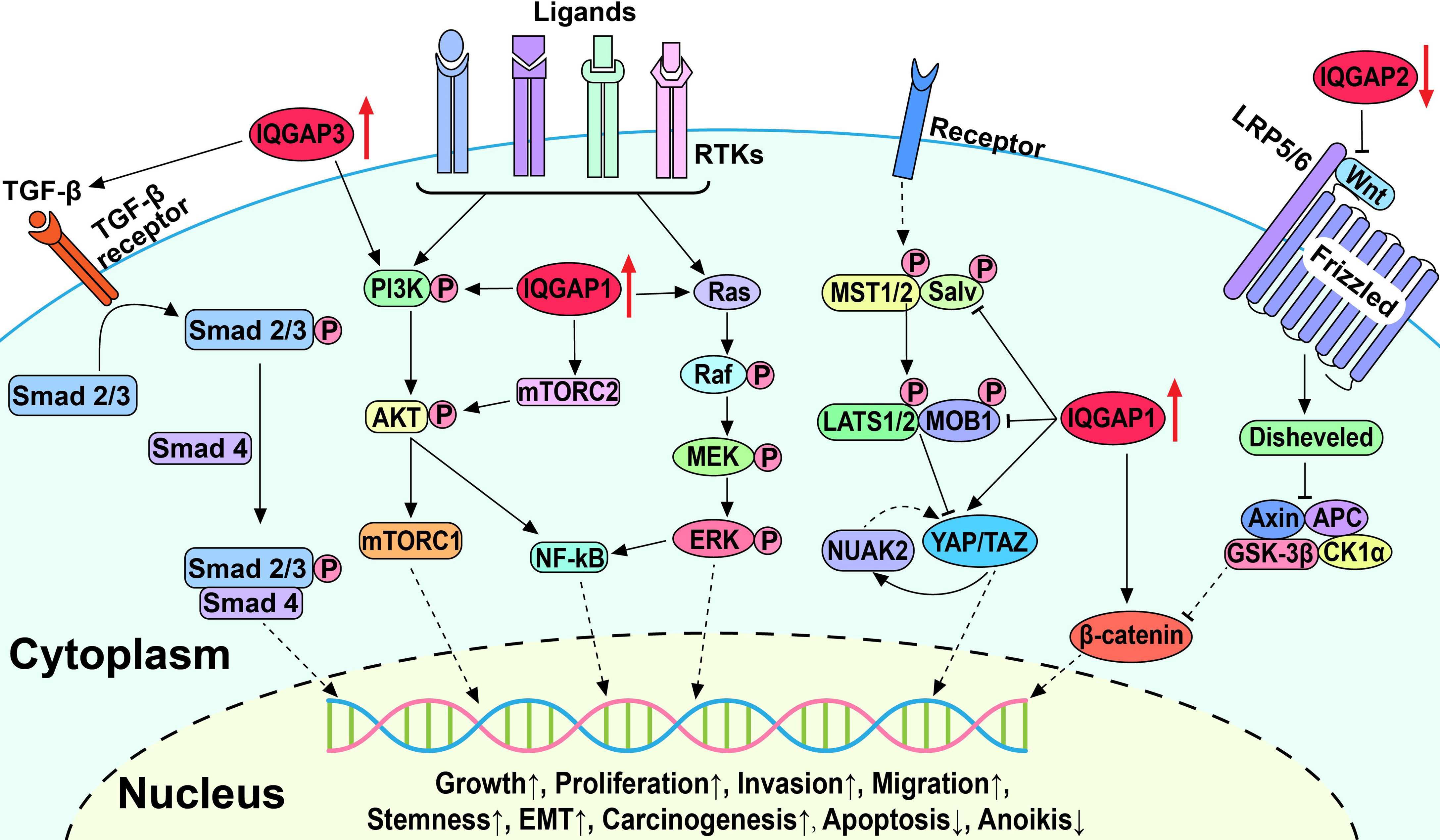
Figure 2 Schematic diagram of IQGAPs-mediated signaling pathways involved in the pathogenesis of hepatocellular carcinoma.
5.1 IQGAPs Influence Multiple Key Oncogenic Pathways in HCC
5.1.1 Wnt/β-Catenin Signaling Pathway
The canonical Wnt/β-catenin signaling pathway is involved in the maintenance of cellular homeostasis and proliferation (99, 100). In the absence of Wnt, β-catenin is trapped in the cytoplasm by a destructive complex resulting in its ubiquitination and eventual degradation. Furthermore, Wnt binds to the transmembrane receptor Frizzled and low-density lipoprotein receptor-related protein 5/6 (LRP5/6) to trigger phosphorylation and activation of Dishevelled family of proteins. This results in the prevention of β-catenin destruction, leading to its release and accumulation, followed by translocation to the nucleus, where it binds to transcription factors and activates the transcription of downstream target genes and ultimately leads to aberrant cell proliferation and neoplasm formation. Scaffold proteins have been shown to upregulate Wnt/β-catenin signaling in human HCC, thereby contributing to the malignant progression of HCC (101). Interestingly, β-catenin expression and signaling activity in HCC tissues is positively correlated with IQGAP1 expression and inversely correlated with IQGAP2 expression (24, 93). Furthermore, these studies showed upregulation of IQGAP1 and downregulation of IQGAP2 exceptionally activate the Wnt/β-catenin signaling pathway. Increased Wnt/β-catenin signaling is strongly implicated in increased HCC cell proliferation, migration, and EMT, which is clinicopathologically correlated with the degree of tumor malignancy (94). However, the elucidation of the precise mechanisms of how IQGAPs activate the Wnt/β-catenin signaling pathway warrants further study.
5.1.2 Hippo Signaling Pathway
The Hippo signaling pathway is evolutionarily conserved and governs organ development and homeostasis by regulating cell proliferation, apoptosis, cell fate, and stem cell self-renewal (102, 103). The core components of Hippo signaling include kinases MST1/2 and LATS1/2, transcriptional cofactors yes-associated-protein-1 (YAP), and transcriptional coactivator with PDZ-binding motif (TAZ). Upon activation of the Hippo kinase cascade, phosphorylated YAP/TAZ is retained in the cytoplasm and then degraded by ubiquitination. Alternatively, the inactivation of upstream kinases results in YAP/TAZ translocation to the nucleus, where they interact with various transcription factors to induce target gene expression (104). Accordingly, the dysregulated Hippo pathway may be involved in cancer initiation and progression by increasing tumor cell proliferation and migration and inhibiting apoptosis (103, 105).
Delgado et al. and Anakk et al. demonstrated that IQGAP1 is an upstream regulator of the Hippo pathway and promotes HCC by activating YAP (29, 92). IQGAP1 can bind to LATS1 and MST2 through the IQ domain and scaffold the MST2-LATS1 complex. However, it inhibits the kinase activity of MST2 and LATS1 and negatively regulates the MST2-LATS1 pro-apoptotic signal (19). Furthermore, bile acids act as promoters of hepatic tumorigenesis and upregulate the Hippo pathway in an IQGAP1-dependent manner (19, 92). In the absence of IQGAP1, bile acids fail to promote cell proliferation because of the reduced levels of IQGAP1-activated YAP (19, 92). Delgado et al. also reported that IQGAP1 activated the YAP-NUAK family kinase 2 (NUAK2) positive feedback loop to promote HCC cell proliferation and growth and accelerate HCC tumorigenesis and growth in mice (29). The inhibition of NUAK2 was shown to attenuate YAP-dependent cancer cell proliferation and liver tumor growth (106). These results suggest that activation of the Hippo signaling pathway by IQGAP1 is essential for hepatocarcinogenesis and progression and may be a promising therapeutic target for liver cancer.
5.1.3 TGF-β Signaling Pathway
The TGF-β signaling pathway affects cellular homeostasis by influencing cell replication, growth, differentiation, and migration (107, 108). Activated TGF-β binds to TGF-β receptor II (TβRII) and recruits the TβRI receptor to form a receptor complex, followed by phosphorylation and activation of a group of related intracellular proteins Smad2/3. Activated Smad2/3 interacts with Smad4 and forms the Smad2/3/4 complex, which translocates to the nucleus and binds to DNA modulating gene expression. During the initial stages of tumor development, TGF-β can induce cancer cell cycle arrest and apoptosis to exert tumor suppressor effects. As tumors progress and cancer cells become resistant to TGF-β-induced apoptosis, TGF-β converts to a tumor-promoting role to enhance cancer cell proliferation, migration, and EMT. TGF-β is highly expressed in HCC, in which cancer-associated fibroblasts (CAFs) derived from stromal cells or hepatocytes are the principal source of TGF-β (109). TGF-β upregulates the expression of transcription repressor Snail and downregulates E-cadherin in HCC to promote EMT, as well as tumor invasion and metastasis, supporting the role of TGF-β in tumor progression and poor patient prognosis (110).
Shi et al. reported that IQGAP3 overexpression amplified TGF-β activity and promoted its translocation into the nucleus, while the expression levels of phosphorylated Smad2, Smad3, and downstream proteins of the TGF-β signaling pathway were also elevated (22). IQGAP3 was also shown to enhance the invasion and migration of HepG2 and HCCLM3 cells. However, these effects were abolished by silencing or knockdown of IQGAP3, silencing Smad3, or treatment with the TGF-β inhibitor SB431542 indicating the important role of the TGF-β signaling pathway in the promotion of IQGAP3-induced invasion and metastasis in HCC (22).
Although IQGAP1- and IQGAP3-activated TGF-β pathways have been shown to promote HCC onset and progression, in another study IQGAP1-induced activation of the TGF-β pathway plays the opposite role in hepatic metastatic carcinoma whereby myofibroblastic differentiation is inhibited (111). The study showed that in colon and lung cancer liver metastases, IQGAP1 binds to TβRII and suppresses TβRII-mediated signaling to prevent HSC differentiation in the tumor microenvironment and constrains metastatic tumor growth. Moreover, in an experimental liver metastasis model, Iqgap1-/- mice showed higher levels of TGF-β receptor and HSC activation, which promoted metastatic tumor growth (111). Further investigation into signaling interactions between the TGF-β pathway and IQGAPs will provide further insight into how the TGF-β pathway and IQGAPs contribute to primary HCC and hepatic metastatic carcinoma.
5.1.4 PI3K/AKT/mTOR Signaling Pathway
The PI3K/AKT/mTOR signaling pathway plays an important role in cell cycle regulation and is involved in cell metabolism, proliferation, apoptosis, and carcinogenesis (112, 113). Numerous cell surface RTKs are involved in the pathways, including insulin receptor (InsR), EGFR, platelet-derived growth factor receptor (PDGFR), hepatocyte growth factor receptor (HGFR), discoidin domain receptor (DDR), leukocyte tyrosine kinase (LTK), and others, which have high affinity for numerous polypeptide growth factors, cytokines, and hormones and are activated by them (114). Activated RTKs phosphorylate and activate downstream kinases leading to the activation of AKT. Subsequently, AKT activates mTOR, causing the increased translation of genes involved in angiogenesis and cell cycle progression, thereby inhibiting apoptosis and promoting cell growth and migration. Additionally, activated mTOR complex 2 (mTORC2) can phosphorylate AKT, causing excessive activation of AKT. Dysregulated RTKs activate the PI3K/AKT/mTOR signaling pathway in HCC and amplify tumor progression, metastasis, and invasion (115, 116).
IQGAP1 was shown to promote HCC cell growth and proliferation through the PI3K/AKT signaling pathway and facilitate the binding of mTORC2 and AKT to accelerate AKT phosphorylation on Ser-473 (17, 19). Ablation of IQGAP1 either by knockdown or mutation of IQGAP1, or treatment with the PI3K inhibitor LY294002 in cells overexpressing IQGAP1 slowed HCC cell proliferation (17). mTOR was also recently shown to activate S-adenosylmethionine decarboxylase proenzyme (AMD1), which phosphorylates obesity-associated protein (FTO) and subsequently increases the expression of a number of transcription factors that participate in cancer genesis, progression, and stemness (98, 117). In HCC, the interaction of IQGAP1 with FTO increases the phosphorylation and expression of FTO and enhances the stem cell-like properties of HCC cells (98, 117, 118). In addition, IQGAP3 interacts with protein kinase C δ (PKCδ) to competitively inhibit the interaction between PKCδ and PKCα, freeing and activating PKCα, and triggering PI3K/AKT signaling pathways to enhance HCC cell proliferation (21). Similarly, PI3K/AKT activity is increased by binding of IQGAP1 to Rac1, which activates the Src/FAK pathway and can promote HCC cell migration, invasion, and anoikis resistance (20, 119). These results confirm the important role of the PI3K/AKT signaling pathway in the IQGAP-induced HCC progression.
5.1.5 MAPK/ERK Signaling Pathway
The MAPK/ERK signaling pathway plays an important role in cell growth, proliferation, differentiation, and apoptosis (120). Activated RTKs subsequently activate both the PI3K/AKT/mTOR and MAPK/ERK pathways. RTK converts Ras-GDP to Ras-GTP, leading to Ras activation and subsequent activation of Raf, possibly via the Src-family tyrosine kinase. Overall, Raf activation promotes phosphorylation and activation of ERK1/2 and triggers downstream signaling pathways in the cytosol, as well as transcription factors in the nucleus to drive cell proliferation and growth. Aberrant activation of the MAPK/ERK signaling pathway was recently shown to promote tumor growth, migration, invasion, and metastasis while also causing resistance to targeted therapies (121, 122).
IQGAP1 and the Ras gene family have been implicated in HCC induction. In the diethylnitrosamine (DEN)-induced mouse liver cancer model, mRNA expressions of IQGAP1 and the Ras gene family were highly elevated in HCC cells compared to normal hepatocytes, and their expression increased in response to the dosage of DEN (95). However, the mRNA expressions of members of Ras gene family significantly decreased after IQGAP1 silencing, which was accompanied by reduced proliferation and invasion ability of HCC cells (97). In addition, ERK and AKT may be involved in IQGAP1-induced elevation of NF-κB, promoting tumorigenesis, metastasis, and resistance to apoptosis (10, 123, 124). These results suggest that IQGAP1 may activate the MAPK/ERK signaling pathway by upregulating Ras gene family expression to promote hepatocarcinogenesis and progression.
5.2 Action Mechanisms of IQGAPs in HBV-Mediated HCC
Chronic HBV infection is etiologically responsible for more than half of all HCC cases, particularly in HBV epidemic regions where the rate can reach up to 80% (13). Compared to the non-infected population, chronic HBV carriers have a 10- to 25-fold increased risk of HCC throughout their lifetime (125). Hepatitis B virus X protein (HBx) has been implicated in HBV-related hepatocarcinogenesis and is considered to be a key oncogenic factor (126–128). HBx transgenic mice developed HCC demonstrating the potential independent carcinogenic effects of HBx (129). Therefore, the specific role of HBx in the development of HCC has attracted widespread interest. Xu et al. reported that HBx upregulates Cdc42 expression and activity. The accumulating active Cdc42 interacts with the GRD domain of IQGAP1 and stimulates the proliferation and inhibition of apoptosis of HCC cells (18). In addition, HBV augmented the association between IQGAP1 and Rac1, leading to increased intracellular levels of reactive oxygen species that subsequently accelerated Src kinase phosphorylation which ultimately activates FAK signaling and promotes anoikis resistance, migration, and invasion of HCC cells (20).
5.3 Putative Explanation for Opposite Functions of IQGAP1/3 and 2 in HCC
Given that IQGAPs share a similar domain structure and sequence homology, the paradoxical phenomenon that IQGAP1/3 and 2 play contrasting roles in HCC may be due to their different protein binding partners, regulated signaling pathways, subcellular localization, and diverse tissue expression. The Rho family of small GTPases mainly includes Cdc42, Rac, and Rho, which cycle between GTP-bound active and GDP-bound inactive states and regulate multiple cellular processes (130). For example, active Cdc42 stimulates cell proliferation, adhesion, migration, polarity, and dynamic changes in the cytoskeleton (131). Besides, RhoA and RhoC are oncogenic and associated with cancer cell proliferation, invasion, and metastasis, while RhoB is tumor suppressive and promotes apoptosis (132). IQGAP1/3 appear to bind selectively to active GTP-Cdc42 and GTP-Rac1 through the GRD domain, while IQGAP2 binds indiscriminately to both GTP- and GDP-bound forms (40, 43, 133). Casteel et al. found that IQGAP1 interacts directly with active RhoA and RhoC via the GRD domain, but not with RhoB (134). Therefore, the differential predisposed binding of IQGAPs to GTPases may lead to their opposite effects in HCC.
In addition to protein binding partners, IQGAPs also regulate different signaling pathways and have opposite effects on the same signaling pathway (Figure 2). IQGAP1 exerts oncogenic effects by promoting PI3K/AKT/mTOR, MAPK/ERK, and Wnt/β-catenin signaling pathways while inhibiting the Hippo signaling pathway (17, 19, 94). IQGAP3 also acts as an oncogenic agent by promoting TGF-β and PI3K/AKT/mTOR signaling pathways, while IQGAP2 suppresses carcinogenesis by inhibiting the Wnt/β-catenin signaling pathway (21, 22, 93). Furthermore, IQGAPs are involved in carcinogenesis by regulating the mitosis of mammalian cancer cells (135). Adachi and coworkers revealed that IQGAP1 was distributed uniformly throughout the cell cortex, and IQGAP3 was specifically localized in the equatorial cortex at anaphase in Hela cells, whereas IQGAP2 was not detected throughout the cell (135). Moreover, the role of IQGAP1/3 in mitosis was further confirmed by suppression of IQGAP1 and IQGAP3 which impaired the localization of anillin and RhoA to the contractile ring and inhibited cytokinesis (135). The foregoing interpretations might lead to distinct roles of IQGAPs in HCC, but the concrete mechanisms still warrant further investigation. More in-depth studies on the molecular structures and mechanisms would contribute to providing precise remedy with IQGAPs as targets for cancer therapy.
6 Conclusion
This review described the structures of the three members of the IQGAP family and their expression and diverse roles in different liver diseases and mainly in HCC. The signaling pathways associated with IQGAPs and the functional mechanisms in HCC were also presented. Although IQGAPs are promising therapeutic targets for HCC, some issues need to be addressed. For example, IQGAP1 plays distinct roles in primary HCC and hepatic metastatic carcinoma, as well as the concrete mechanism of inter-regulation between IQGAP families. Furthermore, the oncogenes IQGAP1 and IQGAP3 play critical roles in cytoskeletal remodeling of the liver and bile ducts, and in liver regeneration after liver injury, respectively (136–138). Thus, the inhibition of IQGAP1 and IQGAP3 may disrupt the integrity of the liver and bile ducts and affect liver regeneration after liver injury. Future detailed studies on the mechanistic roles of IQGAPs in the cytoskeleton and HCC could promote more precise targeted therapy for HCC while avoiding disruption of the normal physiological functions of IQGAPs. Likewise, it is equally important to explore the role of these fundamental scaffolding proteins in other liver diseases such as fatty liver disease, fibrosis, and cirrhosis to unravel the functional relationship of these proteins with chronic liver diseases which if left untreated ultimately lead to HCC.
Author Contributions
QD and QA summarized and wrote the article. MR, FS, and AZ commented and revised the manuscript. All authors contributed to the article and approved the submitted version.
Funding
This study was supported by funding from the Friedrich-Schiller-Universität Jena and Foundation “Else Kröner-Fresenius-Stiftung” within the Else Kröner Graduate School for Medical Students “Jena School for Ageing Medicine (JSAM)”.
Conflict of Interest
The authors declare that the research was conducted in the absence of any commercial or financial relationships that could be construed as a potential conflict of interest.
Publisher’s Note
All claims expressed in this article are solely those of the authors and do not necessarily represent those of their affiliated organizations, or those of the publisher, the editors and the reviewers. Any product that may be evaluated in this article, or claim that may be made by its manufacturer, is not guaranteed or endorsed by the publisher.
References
1. Frechette L, Degrandmaison J, Binda C, Boisvert M, Cote L, Michaud T, et al. Identification of the Interactome of the DP1 Receptor for Prostaglandin D2: Regulation of DP1 Receptor Signaling and Trafficking by IQGAP1. Biochim Biophys Acta Gen Subj (2021) 1865(11):129969. doi: 10.1016/j.bbagen.2021.129969
2. Wei T, Lambert PF. Role of IQGAP1 in Carcinogenesis. Cancers (Basel) (2021) 13(16):3940. doi: 10.3390/cancers13163940
3. Mosaddeghzadeh N, Nouri K, Krumbach OHF, Amin E, Dvorsky R, Ahmadian MR. Selectivity Determinants of RHO GTPase Binding to IQGAPs. Int J Mol Sci (2021) 22(22):12596. doi: 10.3390/ijms222212596
4. Hoeprich GJ, Sinclair AN, Shekhar S, Goode BL. Single-Molecule Imaging of IQGAP1 Regulating Actin Filament Dynamics. Mol Biol Cell (2022) 33(1):ar2. doi: 10.1091/mbc.E21-04-0211
5. Morita R, Numata O, Nakano K, Takaine M. Cell Cycle-Dependent Phosphorylation of IQGAP is Involved in Assembly and Stability of the Contractile Ring in Fission Yeast. Biochem Biophys Res Commun (2021) 534:1026–32. doi: 10.1016/j.bbrc.2020.10.043
6. Carmon KS, Gong X, Yi J, Wu L, Thomas A, Moore CM, et al. LGR5 Receptor Promotes Cell-Cell Adhesion in Stem Cells and Colon Cancer Cells via the IQGAP1-Rac1 Pathway. J Biol Chem (2017) 292(36):14989–5001. doi: 10.1074/jbc.M117.786798
7. Kumar D, Patel SA, Khan R, Chawla S, Mohapatra N, Dixit M. IQ Motif-Containing GTPase-Activating Protein 2 Inhibits Breast Cancer Angiogenesis by Suppressing VEGFR2-AKT Signaling. Mol Cancer Res (2022) 20(1):77–91. doi: 10.1158/1541-7786.MCR-20-1044
8. Hua X, Long ZQ, Guo L, Wen W, Huang X, Zhang WW. IQGAP3 Overexpression Correlates With Poor Prognosis and Radiation Therapy Resistance in Breast Cancer. Front Pharmacol (2020) 11:584450. doi: 10.3389/fphar.2020.584450
9. Peng X, Wang T, Gao H, Yue X, Bian W, Mei J, et al. The Interplay Between IQGAP1 and Small GTPases in Cancer Metastasis. BioMed Pharmacother (2021) 135:111243. doi: 10.1016/j.biopha.2021.111243
10. Zoheir KMA, Abdelhafez MA, Darwish AM, Mahrous KF. New Approach About the Signaling Crosstalk Between IQGAPs/ NF- Kappab/IL-8 and PDCD5/p53/TRAIL Pathways That Modulate Malignant Transformation in Hepatocellular Carcinoma. Asian Pac J Cancer Prev (2022) 23(1):271–9. doi: 10.31557/APJCP.2022.23.1.271
11. Llovet JM, Kelley RK, Villanueva A, Singal AG, Pikarsky E, Roayaie S, et al. Hepatocellular Carcinoma. Nat Rev Dis Primers (2021) 7(1):6. doi: 10.1038/s41572-020-00240-3
12. Sung H, Ferlay J, Siegel RL, Laversanne M, Soerjomataram I, Jemal A, et al. Global Cancer Statistics 2020: GLOBOCAN Estimates of Incidence and Mortality Worldwide for 36 Cancers in 185 Countries. CA Cancer J Clin (2021) 71(3):209–49. doi: 10.3322/caac.21660
13. Mak LY, Cruz-Ramón V, Chinchilla-López P, Torres HA, LoConte NK, Rice JP, et al. Global Epidemiology, Prevention, and Management of Hepatocellular Carcinoma. Am Soc Clin Oncol Educ Book (2018) 38:262–79. doi: 10.1200/EDBK_200939
14. Yang JD, Kim WR, Coelho R, Mettler TA, Benson JT, Sanderson SO, et al. Cirrhosis is Present in Most Patients With Hepatitis B and Hepatocellular Carcinoma. Clin Gastroenterol Hepatol (2011) 9(1):64–70. doi: 10.1016/j.cgh.2010.08.019
15. Kow AWC. Transplantation Versus Liver Resection in Patients With Hepatocellular Carcinoma. Transl Gastroenterol Hepatol (2019) 4:33. doi: 10.21037/tgh.2019.05.06
16. Li C, Wen T-F, Zhang X-Y, Chen X, Shen J-Y. IQGAP1 Expression in Hepatocellular Carcinoma Predicts Poor Prognosis by Inducing Epithelial-Mesenchymal Transition. Trans Cancer Res (2017) 6(3):530–40. doi: 10.21037/tcr.2017.05.40
17. Chen F, Zhu HH, Zhou LF, Wu SS, Wang J, Chen Z. IQGAP1 is Overexpressed in Hepatocellular Carcinoma and Promotes Cell Proliferation by Akt Activation. Exp Mol Med (2010) 42(7):477–83. doi: 10.3858/emm.2010.42.7.049
18. Xu Y, Qi Y, Luo J, Yang J, Xie Q, Deng C, et al. Hepatitis B Virus X Protein Stimulates Proliferation, Wound Closure and Inhibits Apoptosis of HuH-7 Cells via CDC42. Int J Mol Sci (2017) 18(3):586. doi: 10.3390/ijms18030586
19. Quinn NP, Garcia-Gutierrez L, Doherty C, von Kriegsheim A, Fallahi E, Sacks DB, et al. IQGAP1 Is a Scaffold of the Core Proteins of the Hippo Pathway and Negatively Regulates the Pro-Apoptotic Signal Mediated by This Pathway. Cells (2021) 10(2):478. doi: 10.3390/cells10020478
20. Mo CF, Li J, Yang SX, Guo HJ, Liu Y, Luo XY, et al. IQGAP1 Promotes Anoikis Resistance and Metastasis Through Rac1-Dependent ROS Accumulation and Activation of Src/FAK Signalling in Hepatocellular Carcinoma. Br J Cancer (2020) 123(7):1154–63. doi: 10.1038/s41416-020-0970-z
21. Lin M, Liu YM, Ding XK, Ke QJ, Shi JY, Ma ZW, et al. E2F1 Transactivates IQGAP3 and Promotes Proliferation of Hepatocellular Carcinoma Cells Through IQGAP3-Mediated PKC-Alpha Activation. Am J Cancer Res (2019) 9(2):285–99.
22. Shi Y, Qin N, Zhou Q, Chen Y, Huang S, Chen B, et al. Role of IQGAP3 in Metastasis and Epithelial-Mesenchymal Transition in Human Hepatocellular Carcinoma. J Transl Med (2017) 15(1):176. doi: 10.1186/s12967-017-1275-8
23. Xia FD, Wang ZL, Chen HX, Huang Y, Li JD, Wang ZM, et al. Differential Expression of IQGAP1/2 in Hepatocellular Carcinoma and its Relationship With Clinical Outcomes. Asian Pac J Cancer Prev (2014) 15(12):4951–6. doi: 10.7314/APJCP.2014.15.12.4951
24. Schmidt VA, Chiariello CS, Capilla E, Miller F, Bahou WF. Development of Hepatocellular Carcinoma in Iqgap2-Deficient Mice is IQGAP1 Dependent. Mol Cell Biol (2008) 28(5):1489–502. doi: 10.1128/MCB.01090-07
25. Weissbach L, Settleman J, Kalady MF, Snijders AJ, Murthy AE, Yan YX, et al. Identification of a Human rasGAP-Related Protein Containing Calmodulin-Binding Motifs. J Biol Chem (1994) 269(32):20517–21. doi: 10.1016/S0021-9258(17)32023-9
26. Nakajima K, Arora PD, Plaha A, McCulloch CA. Role of the Small GTPase Activating Protein IQGAP1 in Collagen Phagocytosis. J Cell Physiol (2021) 236(2):1270–80. doi: 10.1002/jcp.29933
27. Wei T, Choi S, Buehler D, Lee D, Ward-Shaw E, Anderson RA, et al. Role of IQGAP1 in Papillomavirus-Associated Head and Neck Tumorigenesis. Cancers (Basel) (2021) 13(9):2276. doi: 10.3390/cancers13092276
28. Zhang B, Cheng X, Zhan S, Jin X, Liu T. MIB1 Upregulates IQGAP1 and Promotes Pancreatic Cancer Progression by Inducing ST7 Degradation. Mol Oncol (2021) 15(11):3062–75. doi: 10.1002/1878-0261.12955
29. Delgado ER, Erickson HL, Tao J, Monga SP, Duncan AW, Anakk S. Scaffolding Protein IQGAP1 Is Dispensable, But Its Overexpression Promotes Hepatocellular Carcinoma via YAP1 Signaling. Mol Cell Biol (2021) 41(4):e00596–20. doi: 10.1128/MCB.00596-20
30. White CD, Brown MD, Sacks DB. IQGAPs in Cancer: A Family of Scaffold Proteins Underlying Tumorigenesis. FEBS Lett (2009) 583(12):1817–24. doi: 10.1016/j.febslet.2009.05.007
31. Kumar D, Patel SA, Hassan MK, Mohapatra N, Pattanaik N, Dixit M. Reduced IQGAP2 Expression Promotes EMT and Inhibits Apoptosis by Modulating the MEK-ERK and P38 Signaling in Breast Cancer Irrespective of ER Status. Cell Death Dis (2021) 12(4):389. doi: 10.1038/s41419-021-03673-0
32. Wang S, Watanabe T, Noritake J, Fukata M, Yoshimura T, Itoh N, et al. IQGAP3, a Novel Effector of Rac1 and Cdc42, Regulates Neurite Outgrowth. J Cell Sci (2007) 120(Pt 4):567–77. doi: 10.1242/jcs.03356
33. Wu C, Luo Y, Chen Y, Qu H, Zheng L, Yao J. Development of a Prognostic Gene Signature for Hepatocellular Carcinoma. Cancer Treat Res Commun (2022) 31:100511. doi: 10.1016/j.ctarc.2022.100511
34. Matsuo J, Douchi D, Myint K, Mon NN, Yamamura A, Kohu K, et al. Iqgap3-Ras Axis Drives Stem Cell Proliferation in the Stomach Corpus During Homoeostasis and Repair. Gut (2021) 70(10):1833–46. doi: 10.1136/gutjnl-2020-322779
35. Leone M, Cazorla-Vazquez S, Ferrazzi F, Wiederstein JL, Grundl M, Weinstock G, et al. IQGAP3, a YAP Target, Is Required for Proper Cell-Cycle Progression and Genome Stability. Mol Cancer Res (2021) 19(10):1712–26. doi: 10.1158/1541-7786.MCR-20-0639
36. Zheng X, Xu H, Yi X, Zhang T, Wei Q, Li H, et al. Tumor-Antigens and Immune Landscapes Identification for Prostate Adenocarcinoma mRNA Vaccine. Mol Cancer (2021) 20(1):160. doi: 10.1186/s12943-021-01452-1
37. Zeng Y, Jie X, Wu B, Wu G, Liu L, Xu S. IQGAP3 Interacts With Rad17 to Recruit the Mre11-Rad50-Nbs1 Complex and Contributes to Radioresistance in Lung Cancer. Cancer Lett (2020) 493:254–65. doi: 10.1016/j.canlet.2020.08.042
38. Dongol S, Zhang Q, Qiu C, Sun C, Zhang Z, Wu H, et al. IQGAP3 Promotes Cancer Proliferation and Metastasis in High-Grade Serous Ovarian Cancer. Oncol Lett (2020) 20(2):1179–92. doi: 10.3892/ol.2020.11664
39. Hedman AC, Smith JM, Sacks DB. The Biology of IQGAP Proteins: Beyond the Cytoskeleton. EMBO Rep (2015) 16(4):427–46. doi: 10.15252/embr.201439834
40. Nojima H, Adachi M, Matsui T, Okawa K, Tsukita S, Tsukita S. IQGAP3 Regulates Cell Proliferation Through the Ras/ERK Signalling Cascade. Nat Cell Biol (2008) 10(8):971–8. doi: 10.1038/ncb1757
41. Liu Z, Li X, Ma J, Li D, Ju H, Liu Y, et al. Integrative Analysis of the IQ Motif-Containing GTPase-Activating Protein Family Indicates That the IQGAP3-PIK3C2B Axis Promotes Invasion in Colon Cancer. Onco Targets Ther (2020) 13:8299–311. doi: 10.2147/OTT.S257729
42. Wu J, Chen Z, Cao H, Yu Z, Feng J, Wang K, et al. High Expression of IQGAP3 Indicates Poor Prognosis in Colorectal Cancer Patients. Int J Biol Markers (2019) 34(4):348–55. doi: 10.1177/1724600819876951
43. Brill S, Li S, Lyman CW, Church DM, Wasmuth JJ, Weissbach L, et al. The Ras GTPase-Activating-Protein-Related Human Protein IQGAP2 Harbors a Potential Actin Binding Domain and Interacts With Calmodulin and Rho Family GTPases. Mol Cell Biol (1996) 16(9):4869–78. doi: 10.1128/MCB.16.9.4869
44. Xie Y, Kapoor A, Peng H, Cutz JC, Tao L, Tang D. IQGAP2 Displays Tumor Suppression Functions. J Analytical Oncol (2015) 4(2):86–93. doi: 10.6000/1927-7229.2015.04.02.5
45. Watanabe T, Wang S, Kaibuchi K. IQGAPs as Key Regulators of Actin-Cytoskeleton Dynamics. Cell Struct Funct (2015) 40(2):69–77. doi: 10.1247/csf.15003
46. Palani S, Ghosh S, Ivorra-Molla E, Clarke S, Suchenko A, Balasubramanian MK, et al. Calponin-Homology Domain Mediated Bending of Membrane-Associated Actin Filaments. Elife (2021) 10:e61078. doi: 10.7554/eLife.61078
47. Trenton NJ, McLaughlin RT, Bellamkonda SK, Tsao DS, Rodzinski A, Mace EM, et al. Membrane and Actin Tethering Transitions Help IQGAP1 Coordinate GTPase and Lipid Messenger Signaling. Biophys J (2020) 118(3):586–99. doi: 10.1016/j.bpj.2019.12.023
48. Jausoro I, Mestres I, Quassollo G, Masseroni L, Heredia F, Caceres A. Regulation of Spine Density and Morphology by IQGAP1 Protein Domains. PLos One (2013) 8(2):e56574. doi: 10.1371/journal.pone.0056574
49. Abel AM, Schuldt KM, Rajasekaran K, Hwang D, Riese MJ, Rao S, et al. IQGAP1: Insights Into the Function of a Molecular Puppeteer. Mol Immunol (2015) 65(2):336–49. doi: 10.1016/j.molimm.2015.02.012
50. Liu J, Guidry JJ, Worthylake DK. Conserved Sequence Repeats of IQGAP1 Mediate Binding to Ezrin. J Proteome Res (2014) 13(2):1156–66. doi: 10.1021/pr400787p
51. Tsukita S, Oishi K, Sato N, Sagara J, Kawai A, Tsukita S. ERM Family Members as Molecular Linkers Between the Cell Surface Glycoprotein CD44 and Actin-Based Cytoskeletons. J Cell Biol (1994) 126(2):391–401. doi: 10.1083/jcb.126.2.391
52. Itoh N, Nagai T, Watanabe T, Taki K, Nabeshima T, Kaibuchi K, et al. Valosin-Containing Protein (VCP) is a Novel IQ Motif-Containing GTPase Activating Protein 1 (IQGAP1)-Interacting Protein. Biochem Biophys Res Commun (2017) 493(4):1384–9. doi: 10.1016/j.bbrc.2017.09.159
53. Roy M, Li Z, Sacks DB. IQGAP1 Binds ERK2 and Modulates its Activity .J Biol Chem (2004) 279(17):17329–37. doi: 10.1074/jbc.M308405200
54. Jameson KL, Mazur PK, Zehnder AM, Zhang J, Zarnegar B, Sage J, et al. IQGAP1 Scaffold-Kinase Interaction Blockade Selectively Targets RAS-MAP Kinase-Driven Tumors. Nat Med (2013) 19(5):626–30. doi: 10.1038/nm.3165
55. Bardwell AJ, Lagunes L, Zebarjedi R, Bardwell L. The WW Domain of the Scaffolding Protein IQGAP1 is Neither Necessary Nor Sufficient for Binding to the MAPKs ERK1 and ERK2. J Biol Chem (2017) 292(21):8750–61. doi: 10.1074/jbc.M116.767087
56. Zhang M, Li Z, Jang H, Hedman AC, Sacks DB, Nussinov R. Ca(2+)-Dependent Switch of Calmodulin Interaction Mode With Tandem IQ Motifs in the Scaffolding Protein Iqgap1. Biochemistry (2019) 58(49):4903–11. doi: 10.1021/acs.biochem.9b00854
57. Jeong HW, Li Z, Brown MD, Sacks DB. IQGAP1 Binds Rap1 and Modulates its Activity. J Biol Chem (2007) 282(28):20752–62. doi: 10.1074/jbc.M700487200
58. Cheung KL, Lee JH, Shu L, Kim JH, Sacks DB, Kong AN. The Ras GTPase-Activating-Like Protein IQGAP1 Mediates Nrf2 Protein Activation via the Mitogen-Activated Protein Kinase/Extracellular Signal-Regulated Kinase (ERK) Kinase (MEK)-ERK Pathway. J Biol Chem (2013) 288(31):22378–86. doi: 10.1074/jbc.M112.444182
59. McNulty DE, Li Z, White CD, Sacks DB, Annan RS. MAPK Scaffold IQGAP1 Binds the EGF Receptor and Modulates its Activation. J Biol Chem (2011) 286(17):15010–21. doi: 10.1074/jbc.M111.227694
60. White CD, Li Z, Dillon DA, Sacks DB. IQGAP1 Protein Binds Human Epidermal Growth Factor Receptor 2 (HER2) and Modulates Trastuzumab Resistance. J Biol Chem (2011) 286(34):29734–47. doi: 10.1074/jbc.M111.220939
61. Monteleon CL, McNeal A, Duperret EK, Oh SJ, Schapira E, Ridky TW. IQGAP1 and IQGAP3 Serve Individually Essential Roles in Normal Epidermal Homeostasis and Tumor Progression. J Invest Dermatol (2015) 135(9):2258–65. doi: 10.1038/jid.2015.140
62. Gorisse L, Li Z, Wagner CD, Worthylake DK, Zappacosta F, Hedman AC, et al. Ubiquitination of the Scaffold Protein IQGAP1 Diminishes its Interaction With and Activation of the Rho GTPase Cdc42. J Biol Chem (2020) 295(15):4822–35. doi: 10.1074/jbc.RA119.011491
63. Kurella VB, Richard JM, Parke CL, Lecour LF Jr., Bellamy HD, Worthylake DK. Crystal Structure of the GTPase-Activating Protein-Related Domain From IQGAP1. J Biol Chem (2009) 284(22):14857–65. doi: 10.1074/jbc.M808974200
64. LeCour L Jr., Boyapati VK, Liu J, Li Z, DB S, Worthylake DK. The Structural Basis for Cdc42-Induced Dimerization of IQGAPs. Structure (2016) 24(9):1499–508. doi: 10.1016/j.str.2016.06.016
65. Ozdemir ES, Jang H, Gursoy A, Keskin O, Li Z, Sacks DB, et al. Unraveling the Molecular Mechanism of Interactions of the Rho GTPases Cdc42 and Rac1 With the Scaffolding Protein IQGAP2. J Biol Chem (2018) 293(10):3685–99. doi: 10.1074/jbc.RA117.001596
66. Tocker AM, Durocher E, Jacob KD, Trieschman KE, Talento SM, Rechnitzer AA, et al. The Scaffolding Protein IQGAP1 Interacts With NLRC3 and Inhibits Type I IFN Production. J Immunol (2017) 199(8):2896–909. doi: 10.4049/jimmunol.1601370
67. Kuroda S, Fukata M, Nakagawa M, Fujii K, Nakamura T, Ookubo T, et al. Role of IQGAP1, a Target of the Small GTPases Cdc42 and Rac1, in Regulation of E-Cadherin- Mediated Cell-Cell Adhesion. Science (1998) 281(5378):832–5. doi: 10.1126/science.281.5378.832
68. Fukata M, Watanabe T, Noritake J, Nakagawa M, Yamaga M, Kuroda S, et al. Rac1 and Cdc42 Capture Microtubules Through IQGAP1 and CLIP-170. Cell (2002) 109(7):873–85. doi: 10.1016/s0092-8674(02)00800-0
69. Watanabe T, Wang S, Noritake J, Sato K, Fukata M, Takefuji M, et al. Interaction With IQGAP1 Links APC to Rac1, Cdc42, and Actin Filaments During Cell Polarization and Migration. Dev Cell (2004) 7(6):871–83. doi: 10.1016/j.devcel.2004.10.017
70. Sakurai-Yageta M, Recchi C, Le Dez G, Sibarita JB, Daviet L, Camonis J, et al. The Interaction of IQGAP1 With the Exocyst Complex is Required for Tumor Cell Invasion Downstream of Cdc42 and RhoA. J Cell Biol (2008) 181(6):985–98. doi: 10.1083/jcb.200709076
71. Beaty BT, Condeelis J. Digging a Little Deeper: The Stages of Invadopodium Formation and Maturation. Eur J Cell Biol (2014) 93(10-12):438–44. doi: 10.1016/j.ejcb.2014.07.003
72. Li Z, McNulty DE, Marler KJ, Lim L, Hall C, Annan RS, et al. IQGAP1 Promotes Neurite Outgrowth in a Phosphorylation-Dependent Manner. J Biol Chem (2005) 280(14):13871–8. doi: 10.1074/jbc.M413482200
73. Elliott SF, Allen G, Timson DJ. Biochemical Analysis of the Interactions of IQGAP1 C-Terminal Domain With CDC42. World J Biol Chem (2012) 3(3):53–60. doi: 10.4331/wjbc.v3.i3.53
74. Nouri K, Timson DJ, Ahmadian MR. New Model for the Interaction of IQGAP1 With CDC42 and RAC1. Small GTPases (2020) 11(1):16–22. doi: 10.1080/21541248.2017.1321169
75. Nouri K, Fansa EK, Amin E, Dvorsky R, Gremer L, Willbold D, et al. IQGAP1 Interaction With RHO Family Proteins Revisited: KINETIC AND EQUILIBRIUM EVIDENCE FOR MULTIPLE DISTINCT BINDING SITES. J Biol Chem (2016) 291(51):26364–76. doi: 10.1074/jbc.M116.752121
76. Erickson HL, Anakk S. Identification of IQ Motif-Containing GTPase-Activating Protein 1 as a Regulator of Long-Term Ketosis. JCI Insight (2018) 3(21):e99866. doi: 10.1172/jci.insight.99866
77. Chawla B, Hedman AC, Sayedyahossein S, Erdemir HH, Li Z, Sacks DB. Absence of IQGAP1 Protein Leads to Insulin Resistance. J Biol Chem (2017) 292(8):3273–89. doi: 10.1074/jbc.M116.752642
78. Chiariello CS, LaComb JF, Bahou WF, Schmidt VA. Ablation of Iqgap2 Protects From Diet-Induced Hepatic Steatosis Due to Impaired Fatty Acid Uptake. Regul Pept (2012) 173(1-3):36–46. doi: 10.1016/j.regpep.2011.09.003
79. Ma Y, Chang N, Liu Y, Liu F, Dong C, Hou L, et al. Silencing IQGAP1 Alleviates Hepatic Fibrogenesis via Blocking Bone Marrow Mesenchymal Stromal Cell Recruitment to Fibrotic Liver. Mol Ther Nucleic Acids (2022) 27:471–83. doi: 10.1016/j.omtn.2021.12.020
80. Younossi ZM, Koenig AB, Abdelatif D, Fazel Y, Henry L, Wymer M. Global Epidemiology of Nonalcoholic Fatty Liver Disease-Meta-Analytic Assessment of Prevalence, Incidence, and Outcomes. Hepatology (2016) 64(1):73–84. doi: 10.1002/hep.28431
81. Vaitheesvaran B, Hartil K, Navare A, Zheng, OB P, Golden A, et al. Role of the Tumor Suppressor IQGAP2 in Metabolic Homeostasis: Possible Link Between Diabetes and Cancer. Metabolomics (2014) 10(5):920–37. doi: 10.1007/s11306-014-0639-9
82. Tan Z, Sun H, Xue T, Gan C, Liu H, Xie Y, et al. Liver Fibrosis: Therapeutic Targets and Advances in Drug Therapy. Front Cell Dev Biol (2021) 9:730176. doi: 10.3389/fcell.2021.730176
83. Acharya P, Chouhan K, Weiskirchen S, Weiskirchen R. Cellular Mechanisms of Liver Fibrosis. Front Pharmacol (2021) 12:671640. doi: 10.3389/fphar.2021.671640
84. Bataller R, Brenner DA. Liver Fibrosis. J Clin Invest (2005) 115(2):209–18. doi: 10.1172/jci200524282
85. Yang J, Xu C, Wu M, Wu Y, Jia X, Zhou C, et al. MicroRNA-124 Inhibits Hepatic Stellate Cells Inflammatory Cytokines Secretion by Targeting IQGAP1 Through NF-kappaB Pathway. Int Immunopharmacol (2021) 95:107520. doi: 10.1016/j.intimp.2021.107520
86. Li C, Kong Y, Wang H, Wang S, Yu H, Liu X, et al. Homing of Bone Marrow Mesenchymal Stem Cells Mediated by Sphingosine 1-Phosphate Contributes to Liver Fibrosis. J Hepatol (2009) 50(6):1174–83. doi: 10.1016/j.jhep.2009.01.028
87. Dunn R, Wetten A, McPherson S, Donnelly MC. Viral Hepatitis in 2021: The Challenges Remaining and How We Should Tackle Them. World J Gastroenterol (2022) 28(1):76–95. doi: 10.3748/wjg.v28.i1.76
88. Zhang W, Aryan M, Qian S, Cabrera R, Liu X. A Focused Review on Recent Advances in the Diagnosis and Treatment of Viral Hepatitis. Gastroenterol Res (2021) 14(3):139–56. doi: 10.14740/gr1405
89. Brisac C, Salloum S, Yang V, Schaefer EA, Holmes JA, Chevaliez S, et al. IQGAP2 is a Novel Interferon-Alpha Antiviral Effector Gene Acting non-Conventionally Through the NF-kappaB Pathway. J Hepatol (2016) 65(5):972–9. doi: 10.1016/j.jhep.2016.06.028
90. Skawran B, Steinemann D, Weigmann A, Flemming P, Becker T, Flik J, et al. Gene Expression Profiling in Hepatocellular Carcinoma: Upregulation of Genes in Amplified Chromosome Regions. Mod Pathol (2008) 21(5):505–16. doi: 10.1038/modpathol.3800998
91. White CD, Khurana H, Gnatenko DV, Li Z, Odze RD, Sacks DB, et al. IQGAP1 and IQGAP2 are Reciprocally Altered in Hepatocellular Carcinoma. BMC Gastroenterol (2010) 10:125. doi: 10.1186/1471-230X-10-125
92. Anakk S, Bhosale M, Schmidt VA, Johnson RL, Finegold MJ, Moore DD. Bile Acids Activate YAP to Promote Liver Carcinogenesis. Cell Rep (2013) 5(4):1060–9. doi: 10.1016/j.celrep.2013.10.030
93. Gnatenko DV, Xu X, Zhu W, Schmidt VA. Transcript Profiling Identifies Iqgap2(-/-) Mouse as a Model for Advanced Human Hepatocellular Carcinoma. PLos One (2013) 8(8):e71826. doi: 10.1371/journal.pone.0071826
94. Jin X, Liu Y, Liu J, Lu W, Liang Z, Zhang D, et al. The Overexpression of IQGAP1 and Beta-Catenin Is Associated With Tumor Progression in Hepatocellular Carcinoma In Vitro and In Vivo. PLos One (2015) 10(8):e0133770. doi: 10.1371/journal.pone.0133770
95. Zoheir KM, Abd-Rabou AA, Harisa GI, Ashour AE, Ahmad SF, Attia SM, et al. Gene Expression of IQGAPs and Ras Families in an Experimental Mouse Model for Hepatocellular Carcinoma a Mechanistic Study of Cancer Progression. Int J Clin Exp Pathol (2015) 8(8):8821–31.
96. Qian EN, Han SY, Ding SZ, Lv X. Expression and Diagnostic Value of CCT3 and IQGAP3 in Hepatocellular Carcinoma. Cancer Cell Int (2016) 16:55. doi: 10.1186/s12935-016-0332-3
97. Zoheir KM, Abd-Rabou AA, Harisa GI, Kumar A, Ahmad SF, Ansari MA, et al. IQGAP1 Gene Silencing Induces Apoptosis and Decreases the Invasive Capacity of Human Hepatocellular Carcinoma Cells. Tumour Biol (2016) 37(10):13927–39. doi: 10.1007/s13277-016-5283-8
98. Bian X, Shi D, Xing K, Zhou H, Lu L, Yu D, et al. AMD1 Upregulates Hepatocellular Carcinoma Cells Stemness by FTO Mediated mRNA Demethylation. Clin Transl Med (2021) 11(3):e352. doi: 10.1002/ctm2.352
99. Schunk SJ, Floege J, Fliser D, Speer T. WNT-Beta-Catenin Signalling - a Versatile Player in Kidney Injury and Repair. Nat Rev Nephrol (2021) 17(3):172–84. doi: 10.1038/s41581-020-00343-w
100. Perugorria MJ, Olaizola P, Labiano I, Esparza-Baquer A, Marzioni M, Marin JJG, et al. Wnt-Beta-Catenin Signalling in Liver Development, Health and Disease. Nat Rev Gastroenterol Hepatol (2019) 16(2):121–36. doi: 10.1038/s41575-018-0075-9
101. Yuan J, Han B, Hu H, Qian Y, Liu Z, Wei Z, et al. CUL4B Activates Wnt/beta-Catenin Signalling in Hepatocellular Carcinoma by Repressing Wnt Antagonists. J Pathol (2015) 235(5):784–95. doi: 10.1002/path.4492
102. Wu Z, Guan KL. Hippo Signaling in Embryogenesis and Development. Trends Biochem Sci (2021) 46(1):51–63. doi: 10.1016/j.tibs.2020.08.008
103. Ji S, Liu Q, Zhang S, Chen Q, Wang C, Zhang W, et al. FGF15 Activates Hippo Signaling to Suppress Bile Acid Metabolism and Liver Tumorigenesis. Dev Cell (2019) 48(4):460–74. doi: 10.1016/j.devcel.2018.12.021
104. Mohajan S, Jaiswal PK, Vatanmakarian M, Yousefi H, Sankaralingam S, Alahari SK, et al. Hippo Pathway: Regulation, Deregulation and Potential Therapeutic Targets in Cancer. Cancer Lett (2021) 507:112–23. doi: 10.1016/j.canlet.2021.03.006
105. Lee U, Cho EY, Jho EH. Regulation of Hippo Signaling by Metabolic Pathways in Cancer. Biochim Biophys Acta Mol Cell Res (2022) 1869(4):119201. doi: 10.1016/j.bbamcr.2021.119201
106. Yuan WC, Pepe-Mooney B, Galli GG, Dill MT, Huang HT, Hao M, et al. NUAK2 is a Critical YAP Target in Liver Cancer. Nat Commun (2018) 9(1):4834. doi: 10.1038/s41467-018-07394-5
107. Boye A. A Cytokine in Turmoil: Transforming Growth Factor Beta in Cancer. BioMed Pharmacother (2021) 139:111657. doi: 10.1016/j.biopha.2021.111657
108. Kim BG, Malek E, Choi SH, Ignatz-Hoover JJ, Driscoll JJ. Novel Therapies Emerging in Oncology to Target the TGF-Beta Pathway. J Hematol Oncol (2021) 14(1):55. doi: 10.1186/s13045-021-01053-x
109. Song M, He J, Pan QZ, Yang J, Zhao J, Zhang YJ, et al. Cancer-Associated Fibroblast-Mediated Cellular Crosstalk Supports Hepatocellular Carcinoma Progression. Hepatology (2021) 73(5):1717–35. doi: 10.1002/hep.31792
110. Shrestha R, Bridle KR, Crawford DHG, Jayachandran A. Immune Checkpoint Molecules are Regulated by Transforming Growth Factor (TGF)-Beta1-Induced Epithelial-to-Mesenchymal Transition in Hepatocellular Carcinoma. Int J Med Sci (2021) 18(12):2466–79. doi: 10.7150/ijms.54239
111. Liu C, Billadeau DD, Abdelhakim H, Leof E, Kaibuchi K, Bernabeu C, et al. IQGAP1 Suppresses TbetaRII-Mediated Myofibroblastic Activation and Metastatic Growth in Liver. J Clin Invest (2013) 123(3):1138–56. doi: 10.1172/JCI63836
112. Yu L, Wei J, Liu P. Attacking the PI3K/Akt/mTOR Signaling Pathway for Targeted Therapeutic Treatment in Human Cancer. Semin Cancer Biol (2021) 21:00188. doi: 10.1016/j.semcancer.2021.06.019
113. Gao X, Jiang Y, Xu Q, Liu F, Pang X, Wang M, et al. 4-Hydroxyderricin Promotes Apoptosis and Cell Cycle Arrest Through Regulating PI3K/AKT/mTOR Pathway in Hepatocellular Cells. Foods (2021) 10(9):2036. doi: 10.3390/foods10092036
114. Blume-Jensen P, Hunter T. Oncogenic Kinase Signalling. Nature (2001) 411(6835):355–65. doi: 10.1038/35077225
115. Jin H, Shi Y, Lv Y, Yuan S, Ramirez CFA, Lieftink C, et al. EGFR Activation Limits the Response of Liver Cancer to Lenvatinib. Nature (2021) 595(7869):730–4. doi: 10.1038/s41586-021-03741-7
116. Wang LL, Cheng L, Ma L, Farooqi AA, Qiao G, Zhang YX, et al. Alnustone Inhibits the Growth of Hepatocellular Carcinoma via ROS- Mediated PI3K Akt mTOR P70s6k Axis. Phytother Res (2022) 36(1):525–42. doi: 10.1002/ptr.7337
117. Zabala-Letona A, Arruabarrena-Aristorena A, Martin-Martin N, Fernandez-Ruiz S, Sutherland JD, Clasquin M, et al. Mtorc1-Dependent AMD1 Regulation Sustains Polyamine Metabolism in Prostate Cancer. Nature (2017) 547(7661):109–13. doi: 10.1038/nature22964
118. Tabbaa M, Ruz Gomez T, Campelj DG, Gregorevic P, Hayes A, Goodman CA. The Regulation of Polyamine Pathway Proteins in Models of Skeletal Muscle Hypertrophy and Atrophy: A Potential Role for Mtorc1. Am J Physiol Cell Physiol (2021) 320(6):C987–C99. doi: 10.1152/ajpcell.00078.2021
119. Wang T, Jin H, Hu J, Li X, Ruan H, Xu H, et al. COL4A1 Promotes the Growth and Metastasis of Hepatocellular Carcinoma Cells by Activating FAK-Src Signaling. J Exp Clin Cancer Res (2020) 39(1):148. doi: 10.1186/s13046-020-01650-7
120. Wang Z, Wang H, Wu Q, Chen Y, Liu J, Liu Y, et al. GATA2 Promotes Human Vascular Smooth Muscle Cell Proliferation via Mitofusin2-Mediated Ras/Raf/MEK/ERK Signaling Pathway. Int J Cardiol (2022) 346:62–70. doi: 10.1016/j.ijcard.2021.11.012
121. Yang X, Shang P, Yu B, Jin Q, Liao J, Wang L, et al. Combination Therapy With Mir34a and Doxorubicin Synergistically Inhibits Dox-Resistant Breast Cancer Progression via Down-Regulation of Snail Through Suppressing Notch/NF-kappaB and RAS/RAF/MEK/ERK Signaling Pathway. Acta Pharm Sin B (2021) 11(9):2819–34. doi: 10.1016/j.apsb.2021.06.003
122. Nichols RJ, Haderk F, Stahlhut C, Schulze CJ, Hemmati G, Wildes D, et al. RAS Nucleotide Cycling Underlies the SHP2 Phosphatase Dependence of Mutant BRAF-, NF1- and RAS-Driven Cancers. Nat Cell Biol (2018) 20(9):1064–73. doi: 10.1038/s41556-018-0169-1
123. Tsai JJ, Chen JH, Chen CH, Chung JG, Hsu FT. Apoptosis Induction and ERK/NF-kappaB Inactivation are Associated With Magnolol-Inhibited Tumor Progression in Hepatocellular Carcinoma In Vivo. Environ Toxicol (2020) 35(2):167–75. doi: 10.1002/tox.22853
124. Ha SE, Kim SM, Vetrivel P, Kim HH, Bhosale PB, Heo JD, et al. Inhibition of Cell Proliferation and Metastasis by Scutellarein Regulating PI3K/Akt/NF-kappaB Signaling Through PTEN Activation in Hepatocellular Carcinoma. Int J Mol Sci (2021) 22(16):8841. doi: 10.3390/ijms22168841
125. Jia L, Gao Y, He Y, Hooper JD, Yang P. HBV Induced Hepatocellular Carcinoma and Related Potential Immunotherapy. Pharmacol Res (2020) 159:104992. doi: 10.1016/j.phrs.2020.104992
126. Lei Y, Xu X, Liu H, Chen L, Zhou H, Jiang J, et al. HBx Induces Hepatocellular Carcinogenesis Through ARRB1-Mediated Autophagy to Drive the G1/S Cycle. Autophagy (2021) 17(12):4423–41. doi: 10.1080/15548627.2021.1917948
127. Medhat A, Arzumanyan A, Feitelson MA. Hepatitis B X Antigen (HBx) is an Important Therapeutic Target in the Pathogenesis of Hepatocellular Carcinoma. Oncotarget (2021) 12(24):2421–33. doi: 10.18632/oncotarget.28077
128. Son J, Kim MJ, Lee JS, Kim JY, Chun E, Lee KY. Hepatitis B Virus X Protein Promotes Liver Cancer Progression Through Autophagy Induction in Response to TLR4 Stimulation. Immune Netw (2021) 21(5):e37. doi: 10.4110/in.2021.21.e37
129. Kim CM, Koike K, Saito I, Miyamura T, Jay G. HBx Gene of Hepatitis B Virus Induces Liver Cancer in Transgenic Mice. Nature (1991) 351(6324):317–20. doi: 10.1038/351317a0
130. Mosaddeghzadeh N, Ahmadian MR. The RHO Family GTPases: Mechanisms of Regulation and Signaling. Cells (2021) 10(7):1831. doi: 10.3390/cells10071831
131. Chen L M, Hobbie S, Galán J E. Requirement of CDC42 for Salmonella-Induced Cytoskeletal and Nuclear Responses. Science (1996) 274(5295):2115–8. doi: 10.1126/science.274.5295.2115
132. Sahai E, Marshall CJ. RHO-GTPases and Cancer. Nat Rev Cancer (2002) 2(2):133–42. doi: 10.1038/nrc725
133. Swart-Mataraza JM, Li Z, Sacks DB. IQGAP1 is a Component of Cdc42 Signaling to the Cytoskeleton. J Biol Chem (2002) 277(27):24753–63. doi: 10.1074/jbc.M111165200
134. Casteel DE, Turner S, Schwappacher R, Rangaswami H, Su-Yuo J, Zhuang S, et al. Rho Isoform-Specific Interaction With IQGAP1 Promotes Breast Cancer Cell Proliferation and Migration. J Biol Chem (2012) 287(45):38367–78. doi: 10.1074/jbc.M112.377499
135. Adachi M, Kawasaki A, Nojima H, Nishida E, Tsukita S. Involvement of IQGAP Family Proteins in the Regulation of Mammalian Cell Cytokinesis. Genes Cells (2014) 19(11):803–20. doi: 10.1111/gtc.12179
136. Kunimoto K, Nojima H, Yamazaki Y, Yoshikawa T, Okanoue T, Tsukita S. Involvement of IQGAP3, a Regulator of Ras/ERK-Related Cascade, in Hepatocyte Proliferation in Mouse Liver Regeneration and Development. J Cell Physiol (2009) 220(3):621–31. doi: 10.1002/jcp.21798
137. Emadali A, Muscatelli-Groux B, Delom F, Jenna S, Boismenu D, Sacks DB, et al. Proteomic Analysis of Ischemia-Reperfusion Injury Upon Human Liver Transplantation Reveals the Protective Role of IQGAP1. Mol Cell Proteomics (2006) 5(7):1300–13. doi: 10.1074/mcp.M500393-MCP200
138. Kalantari F, Auguste P, Ziafazeli T, Tzimas G, Malmström L, Bioulac-Sage P, et al. Proteomics Analysis of Liver Pathological Calcification Suggests a Role for the IQ Motif Containing GTPase Activating Protein 1 in Myofibroblast Function. Proteomics - Clin Appl (2009) 3(3):307–21. doi: 10.1002/prca.200780133
Keywords: IQGAPs, hepatocellular carcinoma, HBV, cytoskeleton, signal transduction
Citation: Dai Q, Ain Q, Rooney M, Song F and Zipprich A (2022) Role of IQ Motif-Containing GTPase-Activating Proteins in Hepatocellular Carcinoma. Front. Oncol. 12:920652. doi: 10.3389/fonc.2022.920652
Received: 14 April 2022; Accepted: 10 May 2022;
Published: 16 June 2022.
Edited by:
Yu-Yun Shao, National Taiwan University Hospital, TaiwanReviewed by:
Xianjue Ma, Westlake University, ChinaChun-Chun Li, National Cheng Kung University, Taiwan
Copyright © 2022 Dai, Ain, Rooney, Song and Zipprich. This is an open-access article distributed under the terms of the Creative Commons Attribution License (CC BY). The use, distribution or reproduction in other forums is permitted, provided the original author(s) and the copyright owner(s) are credited and that the original publication in this journal is cited, in accordance with accepted academic practice. No use, distribution or reproduction is permitted which does not comply with these terms.
*Correspondence: Alexander Zipprich, QWxleGFuZGVyLlppcHByaWNoQG1lZC51bmktamVuYS5kZQ==
†These authors have contributed equally to this work