- 1Department of Medicine, Stony Brook School of Medicine, Stony Brook, NY, United States
- 2Medical Service, Northport Veterans Affairs (VA) Medical Center, Northport, NY, United States
Megakaryocytes (MKs) are important components of the hematopoietic niche. Compared to the non-hematopoietic niche cells, MKs serving as part of the hematopoietic niche provides a mechanism for feedback regulation of hematopoietic stem cells (HSCs), in which HSC progeny (MKs) can modulate HSC adaptation to hematopoietic demands during both steady-state and stress hematopoiesis. MKs are often located adjacent to marrow sinusoids. Considering that most HSCs reside close to a marrow vascular sinusoid, as do MKs, the interactions between MKs and vascular endothelial cells are positioned to play important roles in modulating HSC function, and by extrapolation, might be dysregulated in various disease states. In this review, we discuss the interactions between MKs and the vascular niche in both normal and neoplastic hematopoiesis.
Introduction
Hematopoietic stem cells (HSCs) reside in a specified microenvironment, or the “stem cell niche”. Technical advancements in imaging HSCs in the marrow cavity, coupled with a series of elegant functional studies in murine models, have identified a number of HSC niche cells (e.g., perivascular stromal cells, endothelial cells, nerve cells) that provide the secreted factors and cell-surface molecules essential for HSC maintenance and function (1–5). It has become increasingly evident that the cellular niche components exist in close proximity to one another, and that the interaction between these cells contributes to the resilience and function of HSCs. Megakaryocytes (MK) are rare polyploid marrow cells that give rise to blood platelets. Recent evidence has also implicated MKs in regulating HSC quiescence and proliferation during both steady-state and stress hematopoiesis, mediated by the many cytokines and extracellular matrix components produced by these cells (6–12). In addition, MKs express many inflammatory and immunologic surface markers and signaling molecules and may participate in pathogen surveillance and immune response (13–18). MKs are often located adjacent to marrow sinusoids, a “geography” required for the cells to issue platelets directly into the sinusoidal vascular lumen (19, 20). Vascular endothelial cells (ECs) are also an essential component of the hematopoietic niche with most HSCs found adjacent to a marrow sinusoid (the “vascular niche”) (3, 21–24). Considering that MKs, sinusoids, and HSCs are closely located to each other, the interactions between MKs and ECs are positioned to play an important role in modulating HSC function. Few studies have examined the role of MKs in the regulation of stem cell vascular niche function, despite MKs representing a major source of both proangiogenic and antiangiogenic factors in the marrow. Here, we review the interactions between MKs and the vascular niche in both normal and neoplastic hematopoiesis.
Megakaryocytes Are an Important Component of the Hematopoietic Niche
Recent evidence has implicated MKs in regulating HSC quiescence and proliferation during both steady-state and stress hematopoiesis (6–12). For example, MKs produce TGFβ1 and CXCL4 to promote HSC quiescence in vivo (8, 9); during stress hematopoiesis (e.g., after chemotherapy), the production of TGFβ1 and CXCL4 by MKs surrounding clusters of myeloid progenitor cells may help re-establish HSC quiescence (25). MKs can also promote HSC niche remodeling (e.g., osteoblast expansion) following radiation injury (26, 27) and activate HSC proliferation under stress by synthesizing FGF1 and IGF-1 (6–8). Spatial positioning of HSCs next to MKs not only regulates HSC activity, but also affects HSC developmental potential — HSCs located adjacent to MKs are more platelet- and myeloid-biased compared to HSCs enriched in other regions of the marrow (e.g., the arteriolar niche), and MK depletion can reprogram these myeloid-biased HSCs to a lineage-balanced HSCs (28). Taken together, these studies indicate that the functional effects of MKs in the HSC niche are context-dependent and can be tailored based on demands. Compared to the non-hematopoietic niche cells (e.g., endothelial cells, mesenchymal stromal cells), the MK niche provides a mechanism for a feedback regulation of HSCs by their own progeny. The adaptation of the MK niche function to various hematopoietic demands suggests that HSC and its niche can affect each other, which is critical for the resilience and function of the hematopoietic system.
The Interactions Between Vascular Endothelial Cells and Megakaryocytes in the Hematopoietic Niche
MKs are the largest cells in the marrow with a mean diameter of ~20-50um, with their cell sizes correlating with their degree of maturation (19, 29). MKs are mostly sessile, exhibiting minimal migration in a highly crowded environment (19, 29). ~70% of marrow MKs are intimately associated with the sinusoids, a “geography” required for the cells to issue platelets by the forces generated by flowing sinusoidal blood (19, 20, 30). Ultrastructural observations (31) and intravital imaging (19, 29) revealed that these MKs extend both perivascular pseudopodia to anchor the cells to endothelium, and long transendothelial cellular processes (or proplatelets) to release platelets into the flowing sinusoidal blood. Under inflammatory conditions or acute platelet needs, platelet release can also occur via MK rupture (32). In both cases (proplatelet formation and MK rupture), MKs must reside next to the sinusoids to release platelets into the bloodstream.
The MK-EC interactions are regulated by chemokines [e.g., CXCL12 (20, 33–36), FGF4 (20, 37, 38)], adhesion molecules [e.g., VE-cadherin (20), VCAM-1 (20, 39), PECAM-1 (40, 41)], and blood lipids (e.g., sphingosine-1-phosphate or S1P) (42), through many MK cell surface receptors (e.g., CXCR4, aIIbb3, VLA-4, S1P receptor) (20, 34, 43). ECs have an important role in the regulation of MK maturation and release of platelets (20, 33, 42). A functional vascular niche is critical for platelet production; disrupting the marrow vascular niche (e.g., by inhibiting VE-cadherin which supports EC integrity) impairs MK maturation and thrombopoiesis, while enhancing MK-EC interaction (e.g., by administering CXCL12 or FGF4) increases platelet production (20). (Table 1)
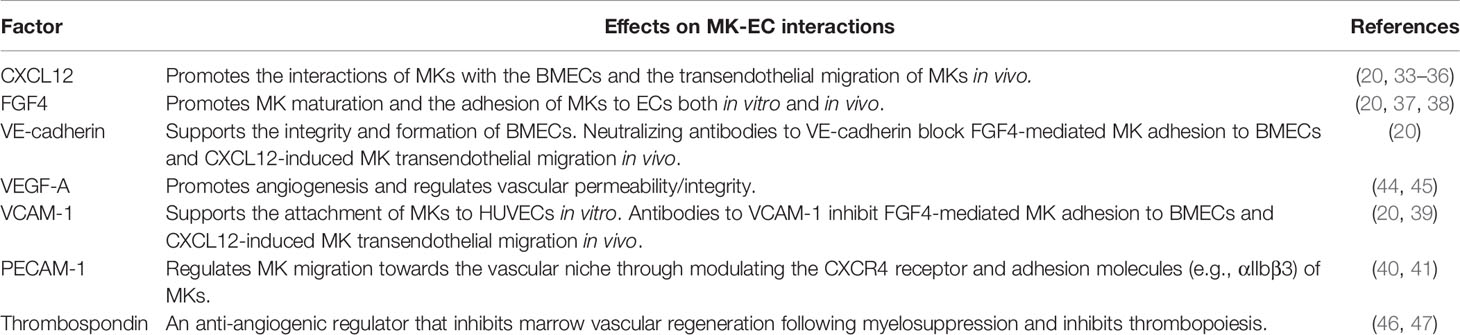
Table 1 Summary of locally acting factors in marrow microenvironment that modulate megakaryocyte-endothelial cell interactions.
MKs also regulate vascular EC function, mediated by the numerous cytokines and growth factors produced by these MK cells. MKs are important sources for both the pro-angiogenic factors such as vascular endothelial growth factor (VEGF) (44, 45) and fibroblast growth factors (FGFs) (6, 48), and the anti-angiogenic factors such as thrombospondin (46) and platelet factor 4 (49). Italiano and colleagues reported that these pro- and anti-angiogenic factors are stored in different alpha-granules and can undergo selective release upon different stimulus (50), providing a mechanism by which MK can differentially stimulate or inhibit the vascular niche under different physiologic and pathologic conditions. These MK vascular regulatory factors may be a key determinant of marrow vascular niche function. However, despite recent progress in understanding the unique process of megakaryocyte lineage development (51–53) and the close interactions between MKs and vascular ECs in the marrow (19, 29) and lung (18, 54), few studies have examined the effects of MKs on vascular niche function and their contributions to normal and neoplastic hematopoiesis.
Altered MK-Vascular Niche Interactions in Myeloproliferative Neoplasms
In addition to normal cell physiologic studies, the mechanisms by which MKs regulate the vascular niche function can be better understood by assessing the dysregulated MK-EC interactions found in neoplastic hematopoiesis. The myeloproliferative neoplasms (MPNs), which include polycythemia vera (PV), essential thrombocythemia (ET) and primary myelofibrosis (PMF), are stem cell disorders characterized by hematopoietic stem/progenitor cell expansion and overproduction of mature blood cells. Patients with MPNs are often characterized by increased marrow angiogenesis (55–57) and MK hyperplasia (58) when compared to normal marrow. In this section, we will discuss the dysregulated MK-EC interactions and their contributions to the neoplastic hematopoiesis in MPNs.
Megakaryocytes Are an Important Component of the Perivascular Stem Cell Niche in MPNs
MK hyperplasia is a hallmark feature of MPNs (58) and many MPN-associated genetic mutations/deregulations are preferentially enriched in MKs (59–61). The acquired kinase mutation JAK2V617F plays a central role in MPNs, having been found in virtually all patients with PV and about half of the patients with ET or PMF. To study the effects of a JAK2V617F-bearing MK niche on MPN disease development in vivo, we crossed mice that bear a Cre-inducible human JAK2V617F transgene (termed Flip-Flop, or FF1) (62) with the Pf4-cre mice [which bear a Cre recombinase driven by the MK-specific platelet factor 4 promoter (63)] to express JAK2V617F specifically in MKs (Pf4+FF1+). We found that the JAK2V617F mutant MKs promote the development of an ET phenotype — at 6-mo of age, JAK2V617F transgenic mice manifest modest thrombocytosis, splenomegaly, 2-3 fold increased numbers of marrow MKs, and 2-3 fold increased numbers of marrow HSCs compared to control mice (64). We observed a strong correlation between marrow MK cell numbers and HSC cell numbers in the Pf4+FF1+ mice, and marrow HSCs in the JAK2V617F-bearing mice are more quiescent and display increased engraftment capacity (65). These results indicate that both HSC number and function are increased in the JAK2V617F-bearing MK niche.
We found that JAK2V617F-driven MK hyperplasia is accompanied by changes in the vascular niche: not only are marrow sinusoids more dilated and MKs are more preferentially located near the marrow sinusoids, but also there is an increased sinusoid vascular density in the Pf4+FF1+ mice compared to age-matched control mice (64). The effect of JAK2V617F MKs on vascular niche function was studied using tube formation assay (as a measure of in vitro angiogenesis) and scratch assay (as a measure of in vitro EC migration) — we found that conditioned medium from the JAK2V617F mutant MK culture significantly stimulated EC tube formation and EC migration compared to conditioned medium from wild-type MKs (64). These findings suggest that JAK2V617F mutant MKs can expand the marrow sinusoidal vascular niche, which in turn could contribute to the thrombocytosis and HSC expansion phenotype.
The JAK2V617F Mutation Alters Megakaryocyte-Endothelial Cell Interactions in the Vascular Niche
In addition to mutant blood cells (including MKs), the JAK2V617F mutation is also present in isolated liver, spleen, and marrow ECs from patients with MPNs (66–68). To study the effects of the JAK2V617F mutation on vascular niche function, we employed a murine model in which mice that bear the Cre-inducible human JAK2V617F transgene (FF1) (62) were crossed with a Tie2-Cre transgenic mouse (69) to express JAK2V617F in all hematopoietic cells (including HSCs and MKs) and ECs (Tie2+FF1+) (70–72). As expected, these mice developed a robust MPN phenotype characterized by neutrophilia, thrombocytosis, splenomegaly, and hematopoietic stem/progenitor cell (HSPC) expansion within 2 months of birth. Histological examination of marrow hematoxylin/eosin sections showed that, compared to control mice, there are markedly increased numbers of MKs in the Tie2+FF1+ mice and many clusters of MKs are preferentially located near marrow sinusoidal vessels (70). Ex vivo co-culture assays revealed that not only do JAK2V617F-bearing ECs directly stimulate JAK2V617F mutant MK expansion, which in turn contributes to HSPC expansion, but also that JAK2V617F mutant MK-conditioned medium significantly stimulates JAK2V617F EC migration compared to that of wild-type MK-conditioned medium. Immunofluorescence staining and deep confocal imaging revealed that marrow MK-EC contact is significantly increased in the Tie2+FF1+ mice compared to WT control mice (Figure 1). These data indicate that the JAK2V617F mutation can alter the MK-EC interactions in the vascular niche to promote the neoplastic hematopoiesis in MPNs.

Figure 1 Immunofluorescence staining and deep confocal imaging of marrow MK-EC interactions. (A) Representative confocal images of wild-type control (left) and Tie2+FF1+ (right) mouse femur marrow stained with antibodies against CD41 (red) and laminin (green). (B) Quantitative analysis revealed increased area of contact between MKs and sinusoid vessels in the marrow of Tie2+FF1+ mice compared to the control mice. Two control mice and two Tie2+FF1+ mice were used and a total of 10 high-quality non-overlapping areas at 20x magnification were selected for analysis with the ImageJ software (National Institute of Health, Bethesda, MD, USA). * P < 0.05.
Megakaryocyte Niche Function Evolves During Hematopoietic Aging in MPNs, and This is Associated With Changes in its Regulation of the Vascular Niche
Aging within the HSC compartment contributes to many age-related diseases including an increased incidence of hematological malignancies in the elderly. HSC aging is characterized by an expansion of phenotypically defined HSCs with impaired function, such as reduced engraftment and self-renewal capacity, a perturbed state of quiescence, and a skewed differentiation towards the myeloid lineage (73, 74). Studies over the past decade suggest that HSC aging is driven by both cell-intrinsic alterations in the stem cells (75–79), and cell-extrinsic mediators from the aged microenvironment in which the stem cells reside (80–83). The relative contribution of intrinsic and extrinsic mechanisms to HSC aging remains debated. One key question is whether microenvironmental alterations initiate HSC aging or whether aged HSCs cause niche remodeling.
In contrast to the non-hematopoietic niche cells, niche MKs provide direct feedback to their HSC precursors, many of which are located directly adjacent to MKs in vivo (8, 9). We investigated the effects of a JAK2V617F-bearing MK niche on HSC aging using the same Pf4+FF1+ murine model in which the JAK2V617F mutation is expressed exclusively in the MK lineage (64, 84). The mice maintain an essential thrombocythemia phenotype during a 2-yr follow-up, with no evidence of transformation to leukemia or myelofibrosis. Compared to age-matched control mice, the Pf4+FF1+ mice demonstrated an acceleration of several hallmarks of HSC aging, including an increase in the absolute numbers of HSCs with myeloid-skewed hematopoiesis (and maintenance of this myeloid skewing during marrow transplantation), a reduced engraftment and self-renewal capacity, and a reduced differentiation capacity (84). We showed that the JAK2V617F mutant MK niche can promote hematopoietic aging mediated by enhanced HSC proliferation/cycling. Both flow cytometry analysis and confocal whole-mount imaging revealed decreased marrow EC numbers (especially CD45-CD31+Sca1- sinusoidal marrow ECs) and decreased marrow vascular areas in 2yr old Pf4+FF1+ compared to age-matched control mice (84). We noticed altered morphology of the marrow sinusoids in the aged Pf4+FF1+ mice, which are narrower in diameter compared to marrow sinusoids in aged control mice (Figure 2) (84). Conditioned medium collected from JAK2V617F mutant MKs of aged Pf4+FF1+ mice significantly inhibited EC tube formation in vitro (84), which differs from what we have observed in the young (6-mo old) Pf4+FF1+ mice (64). While the pro-inflammatory factors [IL-6 (85–87), IL-12 (88), MIP-1a (89)] and anti-angiogenic factors [Fas ligand, IL-10 (90, 91)] are upregulated in old JAK2V617F mutant MKs compared to young mutant MKs, their levels are downregulated in aged control MKs compared to young control MKs. These data suggest that the JAK2V617F-bearing MKs inhibit/disrupt the vascular niche during aging, which in turn can promote HSC aging.
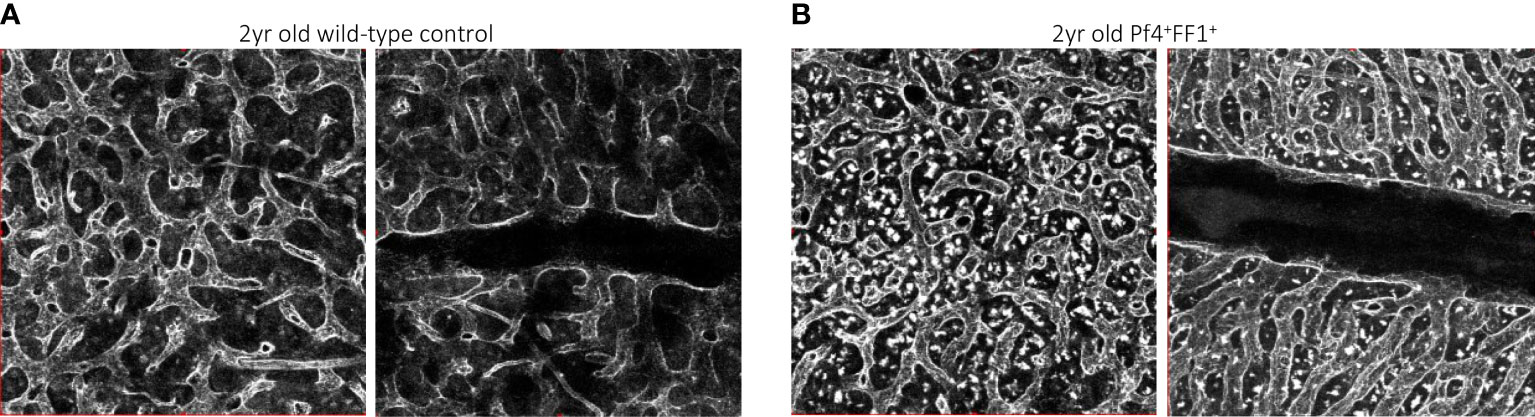
Figure 2 Representative whole-mount confocal images of aged wild-type control (A) and Pf4+FF1+ (B) mouse femur marrow, in which the vasculature was stained intravenously with anti-VE-cadherin antibody before euthanization. Images were acquired with an Olympus IX81 microscope using 20x objective magnification and Olympus Fluoview FV1000 confocal laser scanning system at 512 x 512 pixel resolution.
Despite recent technical breakthroughs in both imaging and functional studies of HSCs in the marrow, we know very little about how the niche changes with age. Our studies showed that the effect of JAK2V617F mutant MKs on HSC function changes during aging in a murine model of MPN: in young mice, mutant MKs expand the marrow sinusoidal vascular niche, induce HSC quiescence with increased repopulating capacity (64, 65); in aged mice, mutant MKs inhibit/disrupt the vascular niche, promote HSC proliferation with a reduced engraftment and self-renewal capacity (84). Despite these changes in MK niche function during aging, how MKs regulate vascular niche function appears to correlate with how MKs regulate HSC function.
Concluding Remarks
Further work is needed to functionally test the importance of MK-EC interaction in niche/HSC function and to understand the molecular mechanisms that mediate these effects. Murine models remain an irreplaceable approach to investigating MK-EC interaction in vivo in both normal and neoplastic hematopoiesis. Patient-derived induced pluripotent stem cells (iPS) provide an important alternative to model human disease in vitro. The capability to differentiate these iPS cell lines towards a specific cell lineage [e.g., MK (92, 93), EC (94)] is a powerful tool to model human diseases with acquired genetic alterations such as the JAK2V617F mutation. It is hoped that such studies will lead to novel therapeutic strategies to target the HSC niche-forming MKs and their interactions with the vascular niche, and to provide better treatments for patients with various aging and neoplastic conditions.
Author Contributions
HZ wrote the manuscript; KK reviewed and revised the manuscript. All authors contributed to the article and approved the submitted version.
Funding
This research was supported by the National Heart, Lung, and Blood Institute grant NIH R01 HL134970 (HZ) and VA Merit Award BX003947 (HZ).
Conflict of Interest
The authors declare that the research was conducted in the absence of any commercial or financial relationships that could be construed as a potential conflict of interest.
Publisher’s Note
All claims expressed in this article are solely those of the authors and do not necessarily represent those of their affiliated organizations, or those of the publisher, the editors and the reviewers. Any product that may be evaluated in this article, or claim that may be made by its manufacturer, is not guaranteed or endorsed by the publisher.
References
1. Schepers K, Campbell TB, Passegue E. Normal and Leukemic Stem Cell Niches: Insights and Therapeutic Opportunities. Cell Stem Cell (2015) 16(3):254–67. doi: 10.1016/j.stem.2015.02.014
2. Yu VW, Scadden DT. Hematopoietic Stem Cell and Its Bone Marrow Niche. Curr Top Dev Biol (2016) 118:21–44. doi: 10.1016/bs.ctdb.2016.01.009
3. Crane GM, Jeffery E, Morrison SJ. Adult Haematopoietic Stem Cell Niches. Nat Rev Immunol (2017). doi: 10.1038/nri.2017.53
4. Pinho S, Frenette PS. Haematopoietic Stem Cell Activity and Interactions With the Niche. Nat Rev Mol Cell Biol (2019) 20(5):303–20. doi: 10.1038/s41580-019-0103-9
5. Mendez-Ferrer S, Bonnet D, Steensma DP, Hasserjian RP, Ghobrial IM, Gribben JG, et al. Bone Marrow Niches in Haematological Malignancies. Nat Rev Cancer (2020) 20(5):285–98. doi: 10.1038/s41568-020-0245-2
6. Zhao M, Ross JT, Itkin T, Perry JM, Venkatraman A, Haug JS, et al. FGF Signaling Facilitates Postinjury Recovery of Mouse Hematopoietic System. Blood (2012) 120(9):1831–42. doi: 10.1182/blood-2011-11-393991
7. Heazlewood SY, Neaves RJ, Williams B, Haylock DN, Adams TE, Nilsson SK. Megakaryocytes Co-Localise With Hemopoietic Stem Cells and Release Cytokines That Up-Regulate Stem Cell Proliferation. Stem Cell Res (2013) 11(2):782–92. doi: 10.1016/j.scr.2013.05.007
8. Zhao M, Perry JM, Marshall H, Venkatraman A, Qian P, He XC, et al. Megakaryocytes Maintain Homeostatic Quiescence and Promote Post-Injury Regeneration of Hematopoietic Stem Cells. Nat Med (2014) 20(11):1321–6. doi: 10.1038/nm.3706
9. Bruns I, Lucas D, Pinho S, Ahmed J, Lambert MP, Kunisaki Y, et al. Megakaryocytes Regulate Hematopoietic Stem Cell Quiescence Through CXCL4 Secretion. Nat Med (2014) 20(11):1315–20. doi: 10.1038/nm.3707
10. Malara A, Currao M, Gruppi C, Celesti G, Viarengo G, Buracchi C, et al. Megakaryocytes Contribute to the Bone Marrow-Matrix Environment by Expressing Fibronectin, Type IV Collagen, and Laminin. Stem Cells (2014) 32(4):926–37. doi: 10.1002/stem.1626
11. Nakamura-Ishizu A, Takubo K, Fujioka M, Suda T. Megakaryocytes are Essential for HSC Quiescence Through the Production of Thrombopoietin. Biochem Biophys Res Commun (2014) 454(2):353–7. doi: 10.1016/j.bbrc.2014.10.095
12. Nakamura-Ishizu A, Takubo K, Kobayashi H, Suzuki-Inoue K, Suda T. CLEC-2 in Megakaryocytes is Critical for Maintenance of Hematopoietic Stem Cells in the Bone Marrow. J Exp Med (2015) 212(12):2133–46. doi: 10.1084/jem.20150057
13. Shiraki R, Inoue N, Kawasaki S, Takei A, Kadotani M, Ohnishi Y, et al. Expression of Toll-Like Receptors on Human Platelets. Thromb Res (2004) 113(6):379–85. doi: 10.1016/j.thromres.2004.03.023
14. Beaulieu LM, Lin E, Morin KM, Tanriverdi K, Freedman JE. Regulatory Effects of TLR2 on Megakaryocytic Cell Function. Blood (2011) 117(22):5963–74. doi: 10.1182/blood-2010-09-304949
15. Crist SA, Elzey BD, Ahmann MT, Ratliff TL. Early Growth Response-1 (EGR-1) and Nuclear Factor of Activated T Cells (NFAT) Cooperate to Mediate CD40L Expression in Megakaryocytes and Platelets. J Biol Chem (2013) 288(47):33985–96. doi: 10.1074/jbc.M113.511881
16. D'Atri LP, Etulain J, Rivadeneyra L, Lapponi MJ, Centurion M, Cheng K, et al. Expression and Functionality of Toll-Like Receptor 3 in the Megakaryocytic Lineage. J Thromb Haemost (2015) 13(5):839–50. doi: 10.1111/jth.12842
17. Sun S, Jin C, Si J, Lei Y, Chen K, Cui Y, et al. Single-Cell Analysis of Ploidy and the Transcriptome Reveals Functional and Spatial Divergency in Murine Megakaryopoiesis. Blood (2021) 138(14):1211–24. doi: 10.1182/blood.2021010697
18. Pariser DN, Hilt ZT, Ture SK, Blick-Nitko SK, Looney MR, Cleary SJ, et al. Lung Megakaryocytes are Immune Modulatory Cells. J Clin Invest (2021) 131(1):15. doi: 10.1172/JCI137377
19. Junt T, Schulze H, Chen Z, Massberg S, Goerge T, Krueger A, et al. Dynamic Visualization of Thrombopoiesis Within Bone Marrow. Science (2007) 317(5845):1767–70. doi: 10.1126/science.1146304
20. Avecilla ST, Hattori K, Heissig B, Tejada R, Liao F, Shido K, et al. Chemokine-Mediated Interaction of Hematopoietic Progenitors With the Bone Marrow Vascular Niche is Required for Thrombopoiesis. Nat Med (2004) 10(1):64–71. doi: 10.1038/nm973
21. Kiel MJ, Yilmaz OH, Iwashita T, Yilmaz OH, Terhorst C, Morrison SJ. SLAM Family Receptors Distinguish Hematopoietic Stem and Progenitor Cells and Reveal Endothelial Niches for Stem Cells. Cell (2005) 121(7):1109–21. doi: 10.1016/j.cell.2005.05.026
22. Sipkins DA, Wei X, Wu JW, Runnels JM, Cote D, Means TK, et al. In Vivo Imaging of Specialized Bone Marrow Endothelial Microdomains for Tumour Engraftment. Nature (2005) 435(7044):969–73. doi: 10.1038/nature03703
23. Acar M, Kocherlakota KS, Murphy MM, Peyer JG, Oguro H, Inra CN, et al. Deep Imaging of Bone Marrow Shows non-Dividing Stem Cells are Mainly Perisinusoidal. Nature (2015) 526(7571):126–30. doi: 10.1038/nature15250
24. Inra CN, Zhou BO, Acar M, Murphy MM, Richardson J, Zhao Z, et al. A Perisinusoidal Niche for Extramedullary Haematopoiesis in the Spleen. Nature (2015) 527(7579):466–71. doi: 10.1038/nature15530
25. Herault A, Binnewies M, Leong S, Calero-Nieto FJ, Zhang SY, Kang YA, et al. Myeloid Progenitor Cluster Formation Drives Emergency and Leukaemic Myelopoiesis. Nature (2017) 544(7648):53–8. doi: 10.1038/nature21693
26. Dominici M, Rasini V, Bussolari R, Chen X, Hofmann TJ, Spano C, et al. Restoration and Reversible Expansion of the Osteoblastic Hematopoietic Stem Cell Niche After Marrow Radioablation. Blood (2009) 114(11):2333–43. doi: 10.1182/blood-2008-10-183459
27. Olson TS, Caselli A, Otsuru S, Hofmann TJ, Williams R, Paolucci P, et al. Megakaryocytes Promote Murine Osteoblastic HSC Niche Expansion and Stem Cell Engraftment After Radioablative Conditioning. Blood (2013) 121(26):5238–49. doi: 10.1182/blood-2012-10-463414
28. Pinho S, Marchand T, Yang E, Wei Q, Nerlov C, Frenette PS. Lineage-Biased Hematopoietic Stem Cells Are Regulated by Distinct Niches. Dev Cell (2018) 44(5):634–41.e4. doi: 10.1016/j.devcel.2018.01.016
29. Stegner D, vanEeuwijk JMM, Angay O, Gorelashvili MG, Semeniak D, Pinnecker J, et al. Thrombopoiesis is Spatially Regulated by the Bone Marrow Vasculature. Nat Commun (2017) 8(1):127. doi: 10.1038/s41467-017-00201-7
30. Tavassoli M, Aoki M. Localization of Megakaryocytes in the Bone Marrow. Blood Cells (1989) 15(1):3–14.
31. Becker RP, De Bruyn PP. The Transmural Passage of Blood Cells Into Myeloid Sinusoids and the Entry of Platelets Into the Sinusoidal Circulation; a Scanning Electron Microscopic Investigation. Am J Anat (1976) 145(2):183–205. doi: 10.1002/aja.1001450204
32. Nishimura S, Nagasaki M, Kunishima S, Sawaguchi A, Sakata A, Sakaguchi H, et al. IL-1alpha Induces Thrombopoiesis Through Megakaryocyte Rupture in Response to Acute Platelet Needs. J Cell Biol (2015) 209(3):453–66. doi: 10.1083/jcb.201410052
33. Hamada T, Mohle R, Hesselgesser J, Hoxie J, Nachman RL, Moore MA, et al. Transendothelial Migration of Megakaryocytes in Response to Stromal Cell-Derived Factor 1 (SDF-1) Enhances Platelet Formation. J Exp Med (1998) 188(3):539–48. doi: 10.1084/jem.188.3.539
34. Wang JF, Liu ZY, Groopman JE. The Alpha-Chemokine Receptor CXCR4 is Expressed on the Megakaryocytic Lineage From Progenitor to Platelets and Modulates Migration and Adhesion. Blood (1998) 92(3):756–64. doi: 10.1182/blood.V92.3.756
35. Lane WJ, Dias S, Hattori K, Heissig B, Choy M, Rabbany SY, et al. Stromal-Derived Factor 1-Induced Megakaryocyte Migration and Platelet Production is Dependent on Matrix Metalloproteinases. Blood (2000) 96(13):4152–9. doi: 10.1182/blood.V96.13.4152
36. Niswander LM, Fegan KH, Kingsley PD, McGrath KE, Palis J. SDF-1 Dynamically Mediates Megakaryocyte Niche Occupancy and Thrombopoiesis at Steady State and Following Radiation Injury. Blood (2014) 124(2):277–86. doi: 10.1182/blood-2014-01-547638
37. Konishi H, Ochiya T, Yasuda Y, Sakamoto H, Muto T, Sugimura T, et al. HST-1/FGF-4 Stimulates Proliferation of Megakaryocyte Progenitors Synergistically and Promotes Megakaryocyte Maturation. Oncogene (1996) 13(1):9–19.
38. Konishi H, Ochiya T, Sakamoto H, Tsukamoto M, Yasuda Y, Muto T, et al. Effective Prevention of Thrombocytopenia Using Adenovirus-Mediated Transfer of HST-11FGF-4 Gene: In Vivo and In Vitro Studies. Leukemia (1997) 11 (Suppl 3):530–2.
39. Avraham H, Cowley S, Chi SY, Jiang S, Groopman JE. Characterization of Adhesive Interactions Between Human Endothelial Cells and Megakaryocytes. J Clin Invest (1993) 91(6):2378–84. doi: 10.1172/JCI116470
40. Dhanjal TS, Pendaries C, Ross EA, Larson MK, Protty MB, Buckley CD, et al. A Novel Role for PECAM-1 in Megakaryocytokinesis and Recovery of Platelet Counts in Thrombocytopenic Mice. Blood (2007) 109(10):4237–44. doi: 10.1182/blood-2006-10-050740
41. Wu Y, Welte T, Michaud M, Madri JA. PECAM-1: A Multifaceted Regulator of Megakaryocytopoiesis. Blood (2007) 110(3):851–9. doi: 10.1182/blood-2006-05-022087
42. Rafii S, Shapiro F, Pettengell R, Ferris B, Nachman RL, Moore MA, et al. Human Bone Marrow Microvascular Endothelial Cells Support Long-Term Proliferation and Differentiation of Myeloid and Megakaryocytic Progenitors. Blood (1995) 86(9):3353–63. doi: 10.1182/blood.V86.9.3353.bloodjournal8693353
43. Larson MK, Watson SP. Regulation of Proplatelet Formation and Platelet Release by Integrin Alpha IIb Beta3. Blood (2006) 108(5):1509–14. doi: 10.1182/blood-2005-11-011957
44. Mohle R, Green D, Moore MA, Nachman RL, Rafii S. Constitutive Production and Thrombin-Induced Release of Vascular Endothelial Growth Factor by Human Megakaryocytes and Platelets. Proc Natl Acad Sci U.S.A. (1997) 94(2):663–8. doi: 10.1073/pnas.94.2.663
45. Bobik R, Hong Y, Breier G, Martin JF, Erusalimsky JD. Thrombopoietin Stimulates VEGF Release From C-Mpl-Expressing Cell Lines and Haematopoietic Progenitors. FEBS Lett (1998) 423(1):10–4. doi: 10.1016/s0014-5793(98)00056-8
46. Kopp HG, Hooper AT, Broekman MJ, Avecilla ST, Petit I, Luo M, et al. Thrombospondins Deployed by Thrombopoietic Cells Determine Angiogenic Switch and Extent of Revascularization. J Clin Invest (2006) 116(12):3277–91. doi: 10.1172/JCI29314
47. Zaslavsky A, Baek KH, Lynch RC, Short S, Grillo J, Folkman J, et al. Platelet-Derived Thrombospondin-1 is a Critical Negative Regulator and Potential Biomarker of Angiogenesis. Blood (2010) 115(22):4605–13. doi: 10.1182/blood-2009-09-242065
48. Pintucci G, Froum S, Pinnell J, Mignatti P, Rafii S, Green D. Trophic Effects of Platelets on Cultured Endothelial Cells are Mediated by Platelet-Associated Fibroblast Growth Factor-2 (FGF-2) and Vascular Endothelial Growth Factor (VEGF). Thromb Haemost (2002) 88(5):834–42. doi: 10.1055/s-0037-1613311
49. Maione TE, Gray GS, Petro J, Hunt AJ, Donner AL, Bauer SI, et al. Inhibition of Angiogenesis by Recombinant Human Platelet Factor-4 and Related Peptides. Science (1990) 247(4938):77–9. doi: 10.1126/science.1688470
50. Italiano JE Jr., Richardson JL, Patel-Hett S, Battinelli E, Zaslavsky A, Short S, et al. Angiogenesis is Regulated by a Novel Mechanism: Pro- and Antiangiogenic Proteins are Organized Into Separate Platelet Alpha Granules and Differentially Released. Blood (2008) 111(3):1227–33. doi: 10.1182/blood-2007-09-113837
51. Sanjuan-Pla A, Macaulay IC, Jensen CT, Woll PS, Luis TC, Mead A, et al. Platelet-Biased Stem Cells Reside at the Apex of the Haematopoietic Stem-Cell Hierarchy. Nature (2013) 502(7470):232–6. doi: 10.1038/nature12495
52. Yamamoto R, Morita Y, Ooehara J, Hamanaka S, Onodera M, Rudolph KL, et al. Clonal Analysis Unveils Self-Renewing Lineage-Restricted Progenitors Generated Directly From Hematopoietic Stem Cells. Cell (2013) 154(5):1112–26. doi: 10.1016/j.cell.2013.08.007
53. Rodriguez-Fraticelli AE, Wolock SL, Weinreb CS, Panero R, Patel SH, Jankovic M, et al. Clonal Analysis of Lineage Fate in Native Haematopoiesis. Nature (2018) 553(7687):212–6. doi: 10.1038/nature25168
54. Lefrancais E, Ortiz-Munoz G, Caudrillier A, Mallavia B, Liu F, Sayah DM, et al. The Lung is a Site of Platelet Biogenesis and a Reservoir for Haematopoietic Progenitors. Nature (2017) 544(7648):105–9. doi: 10.1038/nature21706
55. Medinger M, Skoda R, Gratwohl A, Theocharides A, Buser A, Heim D, et al. Angiogenesis and Vascular Endothelial Growth Factor-/Receptor Expression in Myeloproliferative Neoplasms: Correlation With Clinical Parameters and JAK2-V617F Mutational Status. Br J Haematol (2009) 146(2):150–7. doi: 10.1111/j.1365-2141.2009.07726.x
56. Boveri E, Passamonti F, Rumi E, Pietra D, Elena C, Arcaini L, et al. Bone Marrow Microvessel Density in Chronic Myeloproliferative Disorders: A Study of 115 Patients With Clinicopathological and Molecular Correlations. Br J Haematol (2008) 140(2):162–8. doi: 10.1111/j.1365-2141.2007.06885.x
57. Gianelli U, Vener C, Raviele PR, Savi F, Somalvico F, Calori R, et al. VEGF Expression Correlates With Microvessel Density in Philadelphia Chromosome-Negative Chronic Myeloproliferative Disorders. Am J Clin Pathol (2007) 128(6):966–73. doi: 10.1309/FP0N3LC8MBJUFFA6
58. Ciurea SO, Merchant D, Mahmud N, Ishii T, Zhao Y, Hu W, et al. Pivotal Contributions of Megakaryocytes to the Biology of Idiopathic Myelofibrosis. Blood (2007) 110(3):986–93. doi: 10.1182/blood-2006-12-064626
59. Vannucchi AM, Rotunno G, Bartalucci N, Raugei G, Carrai V, Balliu M, et al. Calreticulin Mutation-Specific Immunostaining in Myeloproliferative Neoplasms: Pathogenetic Insight and Diagnostic Value. Leukemia (2014) 28(9):1811–8. doi: 10.1038/leu.2014.100
60. Kollmann K, Warsch W, Gonzalez-Arias C, Nice FL, Avezov E, Milburn J, et al. A Novel Signalling Screen Demonstrates That CALR Mutations Activate Essential MAPK Signalling and Facilitate Megakaryocyte Differentiation. Leukemia (2017) 31(4):934–44:12. doi: 10.1038/leu.2016.280
61. Prestipino A, Emhardt AJ, Aumann K, O'Sullivan D, Gorantla SP, Duquesne S, et al. Oncogenic JAK2(V617F) Causes PD-L1 Expression, Mediating Immune Escape in Myeloproliferative Neoplasms. Sci Transl Med (2018) 10(429):12. doi: 10.1126/scitranslmed.aam7729
62. Tiedt R, Hao-Shen H, Sobas MA, Looser R, Dirnhofer S, Schwaller J, et al. Ratio of Mutant JAK2-V617F to Wild-Type Jak2 Determines the MPD Phenotypes in Transgenic Mice. Blood (2008) 111(8):3931–40. doi: 10.1182/blood-2007-08-107748
63. Tiedt R, Schomber T, Hao-Shen H, Skoda RC. Pf4-Cre Transgenic Mice Allow the Generation of Lineage-Restricted Gene Knockouts for Studying Megakaryocyte and Platelet Function In Vivo. Blood (2007) 109(4):1503–6. doi: 10.1182/blood-2006-04-020362
64. Zhan H, Ma Y, Lin CH, Kaushansky K. JAK2(V617F)-Mutant Megakaryocytes Contribute to Hematopoietic Stem/Progenitor Cell Expansion in a Model of Murine Myeloproliferation. Leukemia (2016) 30(12):2332–41. doi: 10.1038/leu.2016.114
65. Zhang Y, Lin CHS, Kaushansky K, Zhan H. JAK2V617F Megakaryocytes Promote Hematopoietic Stem/Progenitor Cell Expansion in Mice Through Thrombopoietin/MPL Signaling. Stem Cells (2018) 36(11):1676–84. doi: 10.1002/stem.2888
66. Sozer S, Fiel MI, Schiano T, Xu M, Mascarenhas J, Hoffman R. The Presence of JAK2V617F Mutation in the Liver Endothelial Cells of Patients With Budd-Chiari Syndrome. Blood (2009) 113(21):5246–9. doi: 10.1182/blood-2008-11-191544
67. Rosti V, Villani L, Riboni R, Poletto V, Bonetti E, Tozzi L, et al. Spleen Endothelial Cells From Patients With Myelofibrosis Harbor the JAK2V617F Mutation. Blood (2013) 121(2):360–8. doi: 10.1182/blood-2012-01-404889
68. Helman R, Pereira WO, Marti LC, Campregher PV, Puga RD, Hamerschlak N, et al. Granulocyte Whole Exome Sequencing and Endothelial JAK2V617F in Patients With JAK2V617F Positive Budd-Chiari Syndrome Without Myeloproliferative Neoplasm. Br J Haematol (2018) 180(3):443–5. doi: 10.1111/bjh.14327
69. Constien R, Forde A, Liliensiek B, Grone HJ, Nawroth P, Hammerling G, et al. Characterization of a Novel EGFP Reporter Mouse to Monitor Cre Recombination as Demonstrated by a Tie2 Cre Mouse Line. Genesis (2001) 30(1):36–44. doi: 10.1002/gene.1030
70. Zhan H, Lin CHS, Segal Y, Kaushansky K. The JAK2V617F-Bearing Vascular Niche Promotes Clonal Expansion in Myeloproliferative Neoplasms. Leukemia (2018) 32(2):462–9. doi: 10.1038/leu.2017.233
71. Lin CHS, Zhang Y, Kaushansky K, Zhan H. JAK2V617F-Bearing Vascular Niche Enhances Malignant Hematopoietic Regeneration Following Radiation Injury. Haematologica (2018) 103(7):1160–8. doi: 10.3324/haematol.2017.185736
72. Zhang H, Yeware A, Lee S, Zhan H. A Murine Model With JAK2V617F Expression in Both Hematopoietic Cells and Vascular Endothelial Cells Recapitulates the Key Features of Human Myeloproliferative Neoplasm. Front Oncol (2021). doi: 10.1101/2021.08.24.457585
73. de Haan G, Lazare SS. Aging of Hematopoietic Stem Cells. Blood (2018) 131(5):479–87. doi: 10.1182/blood-2017-06-746412
74. Verovskaya EV, Dellorusso PV, Passegue E. Losing Sense of Self and Surroundings: Hematopoietic Stem Cell Aging and Leukemic Transformation. Trends Mol Med (2019) 25(6):494–515. doi: 10.1016/j.molmed.2019.04.006
75. Rossi DJ, Bryder D, Zahn JM, Ahlenius H, Sonu R, Wagers AJ, et al. Cell Intrinsic Alterations Underlie Hematopoietic1 Stem Cell Aging. Proc Natl Acad Sci U.S.A. (2005) 102(26):9194–9. doi: 10.1073/pnas.0503280102
76. Chambers SM, Shaw CA, Gatza C, Fisk CJ, Donehower LA, Goodell MA. Aging Hematopoietic Stem Cells Decline in Function and Exhibit Epigenetic Dysregulation. PLoS Biol (2007) 5(8):e201. doi: 10.1371/journal.pbio.0050201
77. Flach J, Bakker ST, Mohrin M, Conroy PC, Pietras EM, Reynaud D, et al. Replication Stress is a Potent Driver of Functional Decline in Ageing Haematopoietic Stem Cells. Nature (2014) 512(7513):198–202. doi: 10.1038/nature13619
78. Ho TT, Warr MR, Adelman ER, Lansinger OM, Flach J, Verovskaya EV, et al. Autophagy Maintains the Metabolism and Function of Young and Old Stem Cells. Nature (2017) 543(7644):205–10. doi: 10.1038/nature21388
79. Dykstra B, Olthof S, Schreuder J, Ritsema M, de Haan G. Clonal Analysis Reveals Multiple Functional Defects of Aged Murine Hematopoietic Stem Cells. J Exp Med (2011) 208(13):2691–703. doi: 10.1084/jem.20111490
80. Ergen AV, Boles NC, Goodell MA. Rantes/Ccl5 Influences Hematopoietic Stem Cell Subtypes and Causes Myeloid Skewing. Blood (2012) 119(11):2500–9. doi: 10.1182/blood-2011-11-391730
81. Maryanovich M, Zahalka AH, Pierce H, Pinho S, Nakahara F, Asada N, et al. Adrenergic Nerve Degeneration in Bone Marrow Drives Aging of the Hematopoietic Stem Cell Niche. Nat Med (2018) 24(6):782–91. doi: 10.1038/s41591-018-0030-x
82. Poulos MG, Ramalingam P, Gutkin MC, Llanos P, Gilleran K, Rabbany SY, et al. Endothelial Transplantation Rejuvenates Aged Hematopoietic Stem Cell Function. J Clin Invest (2017) 127(11):4163–78. doi: 10.1172/JCI93940
83. Ho YH, Del Toro R, Rivera-Torres J, Rak J, Korn C, Garcia-Garcia A, et al. Remodeling of Bone Marrow Hematopoietic Stem Cell Niches Promotes Myeloid Cell Expansion During Premature or Physiological Aging. Cell Stem Cell (2019) 25(3):407–18.e6. doi: 10.1016/j.stem.2019.06.007
84. Lee S, Wong H, Castiglione M, Murphy M, Kaushansky K, Zhan H. JAK2V617F Mutant Megakaryocytes Contribute to Hematopoietic Aging in a Murine Model of Myeloproliferative Neoplasm. Stem Cells (2022). doi: 10.1093/stmcls/sxac005
85. Tanaka T, Narazaki M, Kishimoto T. IL-6 in Inflammation, Immunity, and Disease. Cold Spring Harb Perspect Biol (2014) 6(10):a016295. doi: 10.1101/cshperspect.a016295
86. Valletta S, Thomas A, Meng Y, Ren X, Drissen R, Sengul H, et al. Micro-Environmental Sensing by Bone Marrow Stroma Identifies IL-6 and TGFbeta1 as Regulators of Hematopoietic Ageing. Nat Commun (2020) 11(1):4075. doi: 10.1038/s41467-020-17942-7
87. O'Hagan-Wong K, Nadeau S, Carrier-Leclerc A, Apablaza F, Hamdy R, Shum-Tim D, et al. Increased IL-6 Secretion by Aged Human Mesenchymal Stromal Cells Disrupts Hematopoietic Stem and Progenitor Cells' Homeostasis. Oncotarget (2016) 7(12):13285–96. doi: 10.18632/oncotarget.7690
88. Trinchieri G. Interleukin-12: A Proinflammatory Cytokine With Immunoregulatory Functions That Bridge Innate Resistance and Antigen-Specific Adaptive Immunity. Annu Rev Immunol (1995) 13:251–76. doi: 10.1146/annurev.iy.13.040195.001343
89. Cook DN, Beck MA, Coffman TM, Kirby SL, Sheridan JF, Pragnell IB, et al. Requirement of MIP-1 Alpha for an Inflammatory Response to Viral Infection. Science (1995) 269(5230):1583–5. doi: 10.1126/science.7667639
90. Silvestre JS, Mallat Z, Duriez M, Tamarat R, Bureau MF, Scherman D, et al. Antiangiogenic Effect of Interleukin-10 in Ischemia-Induced Angiogenesis in Mice Hindlimb. Circ Res (2000) 87(6):448–52. doi: 10.1161/01.res.87.6.448
91. Kohno T, Mizukami H, Suzuki M, Saga Y, Takei Y, Shimpo M, et al. Interleukin-10-Mediated Inhibition of Angiogenesis and Tumor Growth in Mice Bearing VEGF-Producing Ovarian Cancer. Cancer Res (2003) 63(16):5091–4.
92. Liu Y, Wang Y, Gao Y, Forbes JA, Qayyum R, Becker L, et al. Efficient Generation of Megakaryocytes From Human Induced Pluripotent Stem Cells Using Food and Drug Administration-Approved Pharmacological Reagents. Stem Cells Transl Med (2015) 4(4):309–19. doi: 10.5966/sctm.2014-0183
93. Li Y, Jin C, Bai H, Gao Y, Sun S, Chen L, et al. Human NOTCH4 is a Key Target of RUNX1 in Megakaryocytic Differentiation. Blood (2018) 131(2):191–201. doi: 10.1182/blood-2017-04-780379
Keywords: megakaryocyte (MK), vascular niche, hematopoietic stem cell (HSC), myeloproliferative neoplasms, hematopoietic microenvironment
Citation: Zhan H and Kaushansky K (2022) Megakaryocytes as the Regulator of the Hematopoietic Vascular Niche. Front. Oncol. 12:912060. doi: 10.3389/fonc.2022.912060
Received: 03 April 2022; Accepted: 09 May 2022;
Published: 22 June 2022.
Edited by:
Fabrizio Martelli, National Institute of Health (ISS), ItalyReviewed by:
Hitoshi Takizawa, Kumamoto University, JapanAlessandro Malara, University of Pavia, Italy
Toshio Suda, National University of Singapore, Singapore
James Palis, University of Rochester, United States
Copyright © 2022 Zhan and Kaushansky. This is an open-access article distributed under the terms of the Creative Commons Attribution License (CC BY). The use, distribution or reproduction in other forums is permitted, provided the original author(s) and the copyright owner(s) are credited and that the original publication in this journal is cited, in accordance with accepted academic practice. No use, distribution or reproduction is permitted which does not comply with these terms.
*Correspondence: Huichun Zhan, SHVpY2h1bi5aaGFuQHN0b255YnJvb2ttZWRpY2luZS5lZHU=