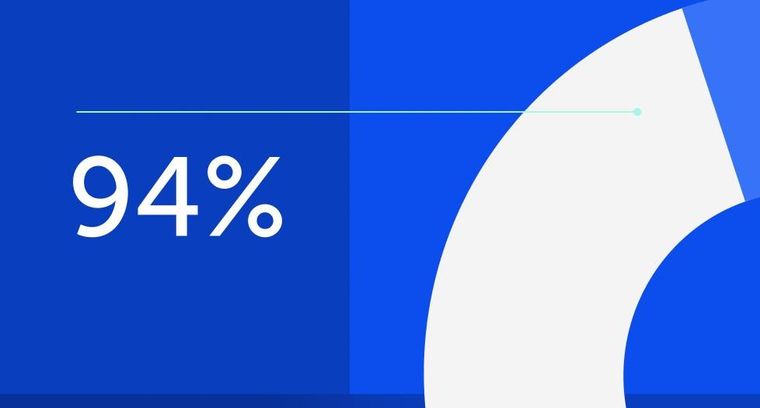
94% of researchers rate our articles as excellent or good
Learn more about the work of our research integrity team to safeguard the quality of each article we publish.
Find out more
ORIGINAL RESEARCH article
Front. Oncol., 29 July 2022
Sec. Molecular and Cellular Oncology
Volume 12 - 2022 | https://doi.org/10.3389/fonc.2022.910505
This article is part of the Research TopicUnravelling the Sarcoma Microenvironment: Impact of the Genomic Landscape on Molecular Signaling, Immunosuppression, and Treatment ResistanceView all 6 articles
Malignant peripheral nerve sheath tumors (MPNST) are aggressive soft-tissue sarcomas which lack effective drugs. Loss of the RAS GTPase-activating protein NF1 and subsequent overactivation of mitogen-activated protein kinase kinase (MAPK) signaling exist nearly uniformly in MPNST, making MAPK inhibition a promising therapeutic intervention. However, the efficacy of MEK inhibitor (MEKi) monotherapy was limited in MPNST and the relative mechanisms remained largely unexplored. In this study, we generated three MEKi-resistant cell models and investigated the mechanisms of MEKi resistance using high-throughput transcriptomic sequencing. We discovered that cell apoptosis and cell cycle arrest induced by MEKi were rescued in MEKi-resistant cells and the upregulation of LAMA4/ITGB1/FAK/SRC signaling conferred resistance to MEKi. In addition, concurrent inhibition of MAPK signaling and FAK/SRC cascade could sensitize MPNST cells to MEKi. Our findings provide potential solutions to overcome MEKi resistance and effective combination therapeutic strategies for treating MPNSTs.
Malignant peripheral nerve sheath tumors (MPNST) are rare soft tissue sarcomas that affect approximately 10% of patients with neurofibromatosis type 1 (NF1), causing severe organ damage and high morbidity (1–4). The only effective therapeutic approach is extended resection with a sufficiently wide margin (3). Unfortunately, many patients lose the chance for surgical resection at the time of diagnosis due to the location or early metastasis of the tumor, and there is no targeted therapy available in the clinic (5). A lack of effective curation makes MPNST the leading cause of early death in adults with NF1 (6). Therefore, it is of great importance to accelerate the development of targeted therapies to improve the prognosis of MPNST patients.
The genomic characterization of MPNST cohorts revealed genes that are dysregulated in MPNSTs, including NF1, CDKN2A, TP53, EED and SUZ12 (7–10). As the most frequent genetic alteration in MPNSTs, inactivation of NF1 leads to the aberrant amplification of Ras and downstream mitogen-activated protein kinase (MAPK) oncogenic signaling, suggesting that this pathway is a potential therapeutic target for MPNSTs. MAPK inhibition using MEK inhibitor (MEKi) has been proven to be a promising strategy for RAS-driven tumors such as melanoma and non–small cell lung cancer (11–13). MEKi selumetinib has been also approved for use in plexiform neurofibroma (pNF), the precursor lesion of MPNSTs (14, 15). However, the efficacy of MEKi monotherapy was relatively limited in MPNSTs and the underlying mechanisms remain largely unexplored, which hinders the implications of MEK inhibitors (MEKis) in MPNST therapy.
Despite the limited effectiveness of MEKis, exploring key mechanisms of MEKi resistance could suggest targets for combinational strategies that hold promise to improve their therapeutic effects. Various mechanisms of MEKi resistance have been elucidated in previous studies (16–21), including kinome reprogramming, tumor microenvironment alterations, and activation of resistance pathways including PI3K/AKT, MAPK, and STAT3 (22–24). In MPNSTs, the research on the mechanisms underlying MEKi resistance was limited and mainly focused on the reactivation of receptor tyrosine kinases (RTK). Although various RTKs that contributed to MEKi resistance have been identified, including MET, PDGFR, and ALK, the development of combination therapy still faces great challenges due to the absence of a commonly dysregulated RTK (25, 26). These findings highlighted the complexity of drug-resistance mechanisms in MEK-targeted therapy, which motivated us to systematically explore the potential mechanisms of MEKi resistance in MPNSTs.
In this study, we established MEKi-resistant MPNST cell models and investigated the mechanism of MEKi resistance using high-throughput transcriptomic sequencing, aiming to improve the efficacy of MEKis in the treatment of MPNSTs. We discovered that the upregulation of LAMA4/ITGB1/FAK/SRC axis led to the reactivation of MAPK pathway, which played a crucial role in MEK inhibitor resistance. We also confirmed that targeting the FAK/SRC cascade could enhance the response of resistant and sensitive MPNST cells to MEKis, providing a potential therapeutic strategy for MPNST therapy.
MPNST cell lines S462, S462TY, and ST8814 were kindly granted by Prof. Vincent Keng and Prof. Jilong Yang. MPNST cell lines were maintained in high-glucose DMEM supplemented with 10% fetal bovine serum (FBS) and 1% penicillin/streptomycin in a 37°C, 5% CO2 incubator. All the cell lines were tested mycoplasma negative every 3 months. Verification of cell lines was confirmed by Short Tandem Repeat DNA profiling (Applied Biological Materials Inc., Canada).
Reagents and antibodies used in this article are described in Supplementary Table S1.
To generate MEKi-resistant cell lines, the parental NF1-MPNST cell line S462 was induced by conventional continuous exposure to trametinib, TAK-733, and selumetinib in a dose-stepwise increment for five months (with a change in medium three times per week).
A Cell Counting Kit-8 (CCK-8) assay was implemented to assess cell proliferation and cytotoxicity. a total of 3*103 cells per well were seeded and treated with 0.1% DMSO or the indicated drugs. After 72 h, 10 μL CCK-8 solution (Dojindo, Japan)dispersed in 90 μL DMEM was added per well to measure the450 nm OD value after a 2 h incubation. Percentage cell viabilitywas calculated as 100% × (OD of drug-treated cells - OD ofbackground control)/(OD of untreated cells - OD of backgroundcontrol). The IC50 of indicated drugs was calculated by Prism8.4.0 using [inhibitor] vs. normalized response – Variable slopeanalysis. Potential synergistic or additive effects were quantifiedusing the software CompuSyn (Cambridge, UK) as previouslydescribed (27, 28). Combination index (CI) values calculated,where CI < 0.9, 0.9–1.1, and > 1.1 indicate synergism, additiveeffect, and antagonism, respectively.
Annexin V–FITC and propidium iodide (PI) assays were implemented to detect cell apoptosis. MPNST cells were seeded into 6-well culture plates and treated with DMSO or the indicated inhibitors for 24 h. Afterwards, MPNST cells were stained with annexin V–FITC and PI (BD-Pharmingen, United States) at room temperature in the dark for 15 minutes, followed by analysis using a flow cytometer (Beckman Coulter, Shanghai) equipped with CytExpert software (Beckman Coulter, Shanghai).
Cell cycle assays were implemented to monitor cell cycle. MPNST cells were seeded into 6-well culture plates and treated with DMSO or the indicated inhibitors for 24 h. Afterwards, A total of 106 cells were collected and fixed overnight in 70% ethanol. The fixed cells were washed with PBS 3 times, stained with PI (BD-Pharmingen, United States) for 15 min, and analyzed using a flow cytometer (Beckman Coulter, Shanghai). The analysis of cell cycle was performed using ModFit LT 5.0 software.
Cells were lysed in RIPA buffer (Beyotime, China) with protease and phosphatase inhibitor cocktails (Beyotime, China). Proteins separated by SDS-PAGE were transferred onto PVDF membranes and blocked in 3% bovine serum. Afterwards, membranes were incubated overnight in primary antibody solution at 4°C, followed by incubation with an HRP-conjugated secondary antibody for 1 h at room temperature. Band signals were detected using an Amersham Imager 600 (General Electric Company, Boston, MA, United States), and quantification was performed using ImageJ software.
Total RNA was extracted using TRIzol reagent (Invitrogen) according to the manufacturer’s instructions. RNA-seq data from cell lines were generated using 2*100 bp paired-end sequencing using the Illumina HiSeq 2000 platform. Paired-end reads were mapped to the University of California at Santa Cruz (UCSC) hg19 reference genome using TopHat2. The normalized expression level of each gene is expressed as the fragments per kilobase of transcript per million mapped reads (FPKM) value. Differential expression analysis of two cell lines (3 biological replicates) was performed using the DESeq2 R package (1.16.1). The resulting P-values were adjusted using Benjamini and Hochberg’s approach for controlling the false discovery rate. Genes with an adjusted P-value < 0.05 found by DESeq2 were assigned as differentially expressed.
Total RNA was extracted according to the procedure of the RNeasy kit (Qiagen, Canada). cDNA from each sample was reverse transcribed using the PrimeScript RT Master Mix Kit (Takara, Japan). Quantitative PCR was performed on cDNA using the SYBR Green System (Applied Biosystems). GAPDH was used as an endogenous control. The relative quantification of RT-qPCR data was calculated using the 2−ΔΔCT method as described previously.
Data are presented as the mean ± standard error of the mean (SEM) or standard deviation (SD). Statistical analysis was conducted using Prism 8.0 (GraphPad Software, San Diego, CA). Statistical analyses were performed with the chi-square test, Student’s t-test, and one-way analysis of variance (ANOVA), as appropriate. P-values < 0.05 were considered to indicate statistical significance, and asterisks (*) are used to indicate significant differences between two specified groups. ** indicates a P-value < 0.01, while *** indicates a P-value < 0.001. P-values > 0.05 qualified as not statistically significant.
To investigate mechanisms relevant to MEKi resistance and to explore effective targets for combination therapy, we generated 3 S462 cell lines resistant to trametinib (S462 R1), TAK-733 (S462 R2), and selumetinib (S462 R3) via continuous exposure of the MEKi-sensitive cell line (S462) to either vehicle or different MEKis (Figure 1A). The drug resistance of MEKi-resistant cell lines was verified by CCK-8 assays after withdrawal of MEKis for over 2 months (Figures 1B–D, Figure S1). The results showed that the cell viability, as well as the IC50 value, significantly increased following the acquisition of drug resistance in S462 cells.
Figure 1 Establishment and verification of MEKi-resistant MPNST cell lines. (A) Schematic representation of developing MEKi-resistant MPNST cell models. (B–D) Cell viability assays of three MEKi-resistant cell lines and parental cells treated with trametinib (B), TAK-733 (C), and selumetinib (D). All experiments were performed in triplicate, and each point represents Mean ± SEM. S462 P: S462 parental cells, S462 R1: S462 cells resistant to trametinib, S462 R2: S462 cells resistant to TAK-733, S462 R3: S462 cells resistant to selumetinib. .
It was demonstrated in previous studies that MEKis exerted anti-proliferative effects via induction of cell apoptosis and cell cycle arrest. Therefore, following the validation of the resistant cell population, we set out to characterize the differences in cell cycle and cell apoptosis in MEKi-resistant and parental cells exposed to MEKis. As demonstrated in the flow cytometry analysis, the G0/G1 phase blockade induced by trametinib was rescued in S462 R1 cells (Figure 2A). Similarly, the ratios of apoptotic cells significantly decreased in S462 R1 cells compared to S462 parental cells (Figure 2B). In addition, the upregulation of cleaved PARP expression after trametinib treatment was less obvious in S462 R1 cells compared to S462 parental cells (Figure S2), indicating that the survival and proliferation capacity of MPNST cells significantly improved after the acquisition of resistance to MEKis. Since the previous studies showed that the reactivation of MAPK pathway or the compensatory activation of parallel pathway was the mechanisms mainly contributing to MEKi resistance (20, 29), we next evaluated the MEK/ERK and AKT activities in MEKi-resistant cell lines (S462 R1, S462 R2, S462 R3). The expression of p-MEK and p-ERK was upregulated in 3 MEKi-resistant cell lines compared with S462 P, while the expression of P-AKT/AKT did not increase (Figure 2C).
Figure 2 MEKi-induced cell-cycle arrest and apoptosis were rescued in MEKi-resistant MPNST cell lines. (A) cell cycle assays and (B) Annexin V–FITC and propidium iodide (PI) assays of S462 R1 and S462 P cells upon trametinib or vehicle treatment for 24 h. All experiments were performed in triplicate, and each column represents Mean ± SEM. (C) Expression of p-MEK, MEK, p-ERK, and ERK in S462 P, S462 R1, S462 R2, and S462 R3 cells evaluated by western blot. All experiments were performed in triplicate, and each column represents Mean ± SEM. ***p < 0.001. S462 P: S462 parental cells, S462 R1: S462 cells resistant to trametinib, S462 R2: S462 cells resistant to TAK-733, S462 R3: S462 cells resistant to selumetinib.
To investigate mechanisms contributing to MEKi resistance in MPNSTs, high-throughput transcriptomic sequencing of 3 MEKi-resistant cell lines (S462 R1, S462 R2, and S462 R3) was conducted, and the S462 P cell line served as a control. The overall differences in gene expression at transcriptional level were visualized with the heatmap (Figure 3A). The common differentially expressed genes (DEGs) in 3 MEKi-resistant cell lines were demonstrated and verified by RT-qPCR in Figure 3B. In addition, gene set enrichment analysis in the Kyoto Encyclopedia of Genes and Genomes (KEGG) and Gene Ontology (GO) identified signaling pathways that significantly dysregulated in MEKi-resistant MPNST cells. Both KEGG and GO analysis identified significant activation of focal adhesion signaling pathway in three MEKi-resistant MPNST cell models (Figures 3C, D, Figure S3).
Figure 3 Focal adhesion signaling pathway is dysregulated in MEKi-resistant MPNST cell lines. (A) RNA-seq heat map showing the differences of gene expression in 3 MEKi-resistant cell lines. The S462 parental cell line served as a control (blue: decreased expression, red: increased expression). (B) The expression of the top 10 upregulated and downregulated genes was confirmed by PCR analysis. All experiments were performed in triplicate, and each column represents Mean ± SEM. (C, D) KEGG (C) and GO enrichment (D) analysis of the common DEGs in MEKi-resistant MPNST cell lines S462R1, S462 R2, and S462 R3. S462 P: S462 parental cells, S462 R1: S462 cells resistant to trametinib, S462 R2: S462 cells resistant to TAK-733, S462 R3: S462 cells resistant to selumetinib.
RT-qPCR analysis was performed to explore the expression of the DEGs related to focal adhesion signaling in 3 MEKi-resistant cell lines and parental cells. The results showed that the top gene significantly upregulated was LAMA4, whose receptors including ITGA1 and ITGB1 were also overexpressed (Figure 4A). Western blot analysis further confirmed that the protein levels of these genes were upregulated in MEKi-resistant cell lines (Figure 4B). Furthermore, inhibiting integrinα1β1 using the highly selective inhibitor obtustatin increased the sensitivity to MEKis according to cell viability assays (Figure 4C), indicating that the upregulation of LAMA4/ITGB1 axis contributed to MEKi resistance. Based on these results, we further evaluated the expression of FAK/SRC, the key regulatory molecules of integrin signaling, and its downstream pathways (Figure 4D). According to western blot analysis, the phosphorylation of FAK and SRC was significantly increased in 3 MEKi-resistant cell lines (S462 R1, S462 R2, S462 R3) compared with S462 parental cells (S462 P). Altogether, these data suggested that the upregulation of the laminin/integrin/FAK/SRC axis could reactivate MAPK signaling, leading to MEKi resistance.
Figure 4 The upregulation of laminin/integrin/FAK/SRC axis mediated MEKi resistance in MPNSTs. (A) RT-qPCR analysis of the DEGs related to focal adhesion pathway in S462 P, S462 R1, S462 R2, and S462 R3 cells. All experiments were performed in triplicate. (B) western blot analysis of f LAMA4, ITGA1, and ITGB1 in S462 P, S462 R1, S462 R2, and S462 R3 cells. All experiments were performed in triplicate, and each column represents Mean ± SEM. *p < 0.05, **p < 0.01, ***p < 0.001. (C) Cell viability of S462 R1 exposed to DMSO, trametinib (Tram), obtustatin (Obtu) or combination therapy, cell viability of S462 R2 exposed to DMSO, TAK-733 (Tak), obtustatin or combination therapy, and cell viability of S462 R3 exposed to DMSO, selumetinib (Selu), obtustatin or combination therapy. All experiments were performed in triplicate, and each column represents Mean ± SEM. *p < 0.05, **p < 0.01, ***p < 0.001. (D) Expression of p-FAK, FAK, p-SRC, and SRC in S462 P, S462 R1, S462 R2, and S462 R3 cells was evaluated by western blot. All experiments were performed in triplicate, and each column represents Mean ± SEM. *p < 0.05, **p < 0.01, ***p < 0.001. S462 P: S462 parental cells, S462 R1: S462 cells resistant to trametinib, S462 R2: S462 cells resistant to TAK-733, S462 R3: S462 cells resistant to selumetinib.
Since our findings were consistent with previous studies showing that FAK/SRC conferred resistance to targeted therapies in a variety of solid tumors (30), we hypothesized that targeting the FAK/SRC signaling is a potential therapeutic strategy to overcome resistance to MEKis. We tested FAK inhibitor GSK2256098 and SRC inhibitor 1, as single agents and in combination with MEKis in S462 R1, and S462 R2 (Figure 5A, Figures S4, 5). According to CCK-8 assays, we found that combinational inhibition of FAK/SRC and MEK had greater effects on cell viability than single agents. In addition, the S-phase arrest and apoptosis induced by SRC inhibitor 1 and trametinib combination therapy were much more significant than single agents in S462 R1 cells (Figures 5B, C). The results above indicated that concurrent inhibition of MAPK signaling and FAK/SRC cascade could restore the sensitivity of MPNST cells to MKEis.
Figure 5 Concurrent inhibition of MAPK signaling and FAK/SRC cascade partially overcame MEKi resistance in MPNST cell lines. (A) Cell viability of S462 R1 exposed to DMSO, trametinib (Tram), GSK2256098 (GSK), SRC inhibitor 1 (SRCi), or combination therapy and cell viability of S462 R2 exposed to DMSO, TAK-733 (TAK), GSK2256098 (GSK), SRC inhibitor 1 (SRCi) or combination therapy. All experiments were performed in triplicate, and each column represents Mean ± SEM. ***p < 0.001. (B) Cell cycle and (C) apoptosis ratios of S462 R1 treated with DMSO, trametinib (Tram), SRC inhibitor 1 (SRCi), or combination therapy. All experiments were performed in triplicate, and each column represents Mean ± SEM. ***p < 0.001. S462 P: S462 parental cells, S462 R1: S462 cells resistant to trametinib, S462 R2: S462 cells resistant to TAK-733.
Having shown that FAK/SRC inhibitors sensitized resistant cell lines to MEKis, we next investigated whether combined MEK/SRC inhibition could reduce cell growth in MEKi-sensitive MPNST cells. The CCK-8 assays showed that trametinib and SRC inhibitor 1 had only modest activity as single agents in MPNST cell lines ST8814, S462, and S462TY, but the combination therapy had a more profound effect on cell viability compared to either drug alone (Figure 6A, Figure S6). To quantify drug synergy between trametinib and SRC inhibitor 1, we calculated combination index (CI) values using Compusyn software. The CI values for S462, ST8814, and S462TY cells are 0.52, 0.44, and 0.62, respectively, indicating the synergistic effects between trametinib and SRC inhibitor 1 in MPNST cells.
Figure 6 The efficacy of combined MEKi and SRCi therapy in MPNSTs. (A) Cell viability of S462, ST8814, and S462TY exposed to DMSO, trametinib (Tram), SRC inhibitor 1 (SRCi), or combination therapy. All experiments were performed in triplicate, and each column represents Mean ± SEM. *p < 0.05, **p < 0.01, ***p < 0.001. (B) Cell cycle and (C) apoptosis ratios of S462 treated with DMSO, trametinib (Tram), SRC inhibitor 1 (SRCi), or combination therapy. All experiments were performed in triplicate, and each column represents Mean ± SEM. *p < 0.05, **p < 0.01, ***p < 0.001.
To explore the mechanism underlying the synthetic lethality, we conducted cell cycle and apoptosis assays. The results revealed that the combination therapy induced cell apoptosis much more robust than single agents in S462 cells. According to western blot assays, SRC inhibitor 1 could not upregulate cleaved PARP expression in S462 cells but can significantly increase the activation of cleaved PARP induced by MEKi trametinib. However, the cell cycle arrest was not increased in S462 cells treated with combination therapy (Figures 6B, C, Figure S7). These results indicated that the combination effect of MEK and SRC inhibitors was caused by the induction of apoptosis in MPNSTs.
In this study, we investigated mechanisms of MEKi resistance in MPNSTs using MEKi-resistant cell models and high-throughput transcriptomic sequencing. Our study identified the upregulation of LAMA4/ITGB1/FAK/SRC axis and subsequent reactivation of MAPK pathway as causative factors that mediated resistance to MEKis. In addition, we demonstrated the effectiveness of combined FAK/SRC and MEK inhibition in enhancing the response of both resistant and sensitive MPNST cells to MEKis (Figure 7).
Figure 7 Schematic of the study. LAMA4 induced MEKi resistance in MPNSTs by upregulating integrin b1/FAK/SRC pathway and subsequently reactivating MAPK signaling.
Hyperactivation of RAS is a key oncogenic event contributing to many cancers including MPNST. Although targeting its downstream MAPK signaling pathway was considered a promising strategy, the efficacy of MEKi monotherapy was insufficient for the treatment of MPNSTs (31, 32). To improve the clinical effect of MEKis and to develop effective targeted therapeutic strategies for MPNSTs, mechanisms of MEKi resistance need to be clarified. As shown in previous investigations, tumors evade targeted cancer therapies via an extensive repertoire of resistance mechanisms. The mechanisms of MEKi resistance that have been identified include, but are not limited to, reactivation of the MAPK pathway, activation of parallel signaling pathways, dysregulation of transcription factors, and transformation in cellular phenotype (29, 33–35). Specifically, Wang et al. discovered that HGF overexpression conferred MEKi resistance by re-activating both PI3K/AKT and MAPK signaling in MPNSTs (25). Our team identified the activation of cyclin-dependent kinase signaling as the vulnerability to overcome MEKi resistance in pNF (36). In this study, we verified the reactivation of MAPK signaling in various MEKi-resistant cell models, accompanied by enhanced abilities to survive and proliferate against MEKis. Previous studies also verified the importance of MAPK reactivation in MEKi resistance and revealed various mechanisms that led to ERK activation, including alterations to molecules upstream of ERK such as RAF, mutation of MEK, and reactivation of multiple RTKs upstream of the MAPK pathway (37–41). These findings suggested that targeting the mechanisms of MAPK reactivation could be an effective strategy to overcome MEKi resistance in MPNSTs.
In this study, we identified that the upregulation of LAMA4/ITGB1/FAK/SRC signaling conferred reactivation of MAPK signaling and resistance to MEKis using high-throughput transcriptomic sequencing. Laminin α4 and its receptor β1 integrin play important roles in mediating cell adhesion and mechanochemical signaling (42, 43). Recent studies revealed that aberrant expression of laminin/integrin signaling was involved in mediating tumor resistance to chemotherapy and targeted therapies (44–46), termed cell adhesion-mediated drug resistance (CAMDR). For example, it was demonstrated in ErbB2-positive breast cancer that laminin and integrins a6b4, a3b1 could lead to acquired resistance to lapatinib and trastuzumab through mitogenic and pro-survival signaling (47). In addition, a previous study also verified that laminin/integrin signaling could promote the survival and proliferation of MPNST tumorigenic cells (48). These findings highlight the important role of laminin/integrin signaling in MEKi resistance.
As the key downstream mediator of the integrin signaling pathway, focal adhesion kinase (FAK), also has an important role in mediating drug resistance (49–52). Phosphorylated FAK forms a complex with SRC, which activates downstream signaling pathways such as PI3K/Akt, MAPK, and Rho, promoting the proliferation, survival, and protein synthesis of tumor cells (53, 54). Our study demonstrated that the phosphorylation of FAK/SRC also significantly increased in MEKi-resistant MPNST cells. In addition, concurrent inhibition of FAK/SRC and MAPK signaling reversed MEKi resistance by inducing apoptosis and cell cycle arrest. These observations were consistent with previous studies which also verified that targeting FAK/SRC sensitized drug-resistant tumor cells to targeted therapies (55). In breast cancer, inhibition of FAK expression with small-molecule inhibitors significantly increased the sensitivity of breast cancer cells to the ErbB2 antibody trastuzumab (56). Meanwhile, in a mouse model of orthotopic glioblastoma, blocking FAK autophosphorylation promoted temozolomide-induced cell death (57). It was also demonstrated that SRC small molecule inhibitor overcame the MAPKi and PI3K/mTORi dual-drug resistance in melanoma (58).
Targeting the key pathways of drug resistance is an effective way to improve the sensitivity of cancers, which is of great significance for improving the survival rate of patients with advanced tumors (18, 59). For example, in BRAF-mutated malignant melanoma, the combination of BRAF inhibitor encorafenib and MEK inhibitor binimetinib was more effective than single agents, showing significant effect in delaying the onset of drug resistance and improving the overall survival rate of patients (60, 61). Therefore, we speculate that the combination therapy targeting FAK/SRC and MEK could be an effective therapeutic strategy for MPNSTs. We investigated the efficacy of combination therapy with SRC and MEK inhibitors in several MPNST cell models and found out that combined MEK/SRC inhibitor treatment exerted synthetic lethality by inducing cell apoptosis in MPNST. At present, there are a few clinical trials evaluating the efficacy of combination therapy with FAK/SRC and MEK inhibitors. A phase I clinical trial also revealed that the combination of FAK inhibitor defactinib and RAF/MEK inhibitor VS-6766 achieved promising results in low-grade serous ovarian cancer (62). Therefore, co-targeting FAK/SRC and MEK might be an effective strategy for the treatment of MPNSTs, which is worth exploring in the clinic.
In conclusion, we discovered that the upregulation of integrin signaling mediated resistance to MEKis. Combinational inhibition of FAK/SRC and MAPK signaling was an effective strategy to overcome MEKi resistance and improve the efficacy of MEKis in MPNSTs. Further clinical investigations that combine MEK and FAK/SRC inhibitors in MPNSTs should be considered in the future.
The datasets presented in this study can be found in online repositories. The names of the repository/repositories and accession number(s) can be found below: https://www.ncbi.nlm.nih.gov/geo/, GSE174100.
YG, CW, MC and HL: Conceptualization, Investigation. ZG, ML, YL and RA: Formal analysis, Visualization. WW and YG: Writing; QL and ZW: Supervision. All authors contributed to the article and approved the submitted version.
This work was supported by grants from National Natural Science Foundation of China (82102344; 82172228); Shanghai Rising Star Program supported by Science and Technology Commission of Shanghai Municipality (20QA1405600); Science and Technology Commission of Shanghai Municipality (19JC1413); Natural Science Foundation of Shanghai (22ZR1422300); “Chenguang Program” supported by Shanghai Education Development Foundation (SHEDF) (19CG18); Shanghai Municipal Key Clinical Specialty (shslczdzk00901); Innovative research team of high-level local universities in Shanghai (SSMU-ZDCX20180700); the Project of Biobank (YBKA201901) from Shanghai Ninth People’s Hospital, Shanghai Jiao Tong University School of Medicine; Shanghai Anticancer Association (SHCY-JC-2021114).
The authors would like to thank Prof. Vincent Keng and Prof. Jilong Yang for providing cell lines. Figures were created using Adobe illustrator 2019 and Biorender.
The authors declare that the research was conducted in the absence of any commercial or financial relationships that could be construed as a potential conflict of interest.
All claims expressed in this article are solely those of the authors and do not necessarily represent those of their affiliated organizations, or those of the publisher, the editors and the reviewers. Any product that may be evaluated in this article, or claim that may be made by its manufacturer, is not guaranteed or endorsed by the publisher.
The Supplementary Material for this article can be found online at: https://www.frontiersin.org/articles/10.3389/fonc.2022.910505/full#supplementary-material
1. Widemann BC. Current status of sporadic and neurofibromatosis type 1-associated malignant peripheral nerve sheath tumors. Curr Oncol Rep (2009) 11(4):322–8. doi: 10.1007/s11912-009-0045-z
2. Mowery A, Clayburgh D. Malignant peripheral nerve sheath tumors: Analysis of the national cancer database. Oral Oncol (2019) 98:13–9. doi: 10.1016/j.oraloncology.2019.09.010
3. Kolberg M, Holand M, Agesen TH, Brekke HR, Liestol K, Hall KS, et al. Survival meta-analyses for >1800 malignant peripheral nerve sheath tumor patients with and without neurofibromatosis type 1. Neuro Oncol (2013) 15(2):135–47. doi: 10.1093/neuonc/nos287
4. Miller SJ, Rangwala F, Williams J, Ackerman P, Kong S, Jegga AG, et al. Large-Scale molecular comparison of human schwann cells to malignant peripheral nerve sheath tumor cell lines and tissues. Cancer Res (2006) 66(5):2584–91. doi: 10.1158/0008-5472.CAN-05-3330
5. van Noesel MM, Orbach D, Brennan B, Kelsey A, Zanetti I, de Salvo GL, et al. Outcome and prognostic factors in pediatric malignant peripheral nerve sheath tumors: An analysis of the european pediatric soft tissue sarcoma group (EpSSG) NRSTS-2005 prospective study. Pediatr Blood Cancer (2019) 66(10):e27833. doi: 10.1002/pbc.27833
6. Katz D, Lazar A, Lev D. Malignant peripheral nerve sheath tumour (MPNST): The clinical implications of cellular signalling pathways. Expert Rev Mol Med (2009) 11:e30. doi: 10.1017/S1462399409001227
7. Mantripragada KK, Diaz de Stahl T, Patridge C, Menzel U, Andersson R, Chuzhanova N, et al. Genome-wide high-resolution analysis of DNA copy number alterations in NF1-associated malignant peripheral nerve sheath tumors using 32K BAC array. Genes Chromosomes Cancer (2009) 48(10):897–907. doi: 10.1002/gcc.20695
8. Rasmussen SA, Overman J, Thomson SA, Colman SD, Abernathy CR, Trimpert RE, et al. Chromosome 17 loss-of-heterozygosity studies in benign and malignant tumors in neurofibromatosis type 1. Genes Chromosomes Cancer (2000) 28(4):425–31. doi: 10.1002/1098-2264(200008)28:4<425::AID-GCC8>3.0.CO;2-E
9. Lee W, Teckie S, Wiesner T, Ran L, Prieto Granada CN, Lin M, et al. PRC2 is recurrently inactivated through EED or SUZ12 loss in malignant peripheral nerve sheath tumors. Nat Genet (2014) 46(11):1227–32. doi: 10.1038/ng.3095
10. Sohier P, Luscan A, Lloyd A, Ashelford K, Laurendeau I, Briand-Suleau A, et al. Confirmation of mutation landscape of NF1-associated malignant peripheral nerve sheath tumors. Genes Chromosomes Cancer (2017) 56(5):421–6. doi: 10.1002/gcc.22446
11. Barbosa R, Acevedo LA, Marmorstein R. The MEK/ERK network as a therapeutic target in human cancer. Mol Cancer Res (2021) 19(3):361–74. doi: 10.1158/1541-7786.MCR-20-0687
12. Han J, Liu Y, Yang S, Wu X, Li H, Wang Q. MEK inhibitors for the treatment of non-small cell lung cancer. J Hematol Oncol (2021) 14(1):1. doi: 10.1186/s13045-020-01025-7
13. Fuse MA, Dinh CT, Vitte J, Kirkpatrick J, Mindos T, Plati SK, et al. Preclinical assessment of MEK1/2 inhibitors for neurofibromatosis type 2-associated schwannomas reveals differences in efficacy and drug resistance development. Neuro Oncol (2019) 21(4):486–97. doi: 10.1093/neuonc/noz002
14. Gross AM, Wolters PL, Dombi E, Baldwin A, Whitcomb P, Fisher MJ, et al. Selumetinib in children with inoperable plexiform neurofibromas. N Engl J Med (2020) 382(15):1430–42. doi: 10.1056/NEJMoa1912735
15. Dombi E, Baldwin A, Marcus LJ, Fisher MJ, Weiss B, Kim A, et al. Activity of selumetinib in neurofibromatosis type 1-related plexiform neurofibromas. N Engl J Med (2016) 375(26):2550–60. doi: 10.1056/NEJMoa1605943
16. Sun C, Hobor S, Bertotti A, Zecchin D, Huang S, Galimi F, et al. Intrinsic resistance to MEK inhibition in KRAS mutant lung and colon cancer through transcriptional induction of ERBB3. Cell Rep (2014) 7(1):86–93. doi: 10.1016/j.celrep.2014.02.045
17. Manchado E, Weissmueller S, JPt M, CC C, Wullenkord R, Lujambio A, et al. A combinatorial strategy for treating KRAS-mutant lung cancer. Nature (2016) 534(7609):647–51. doi: 10.1038/nature18600
18. Fedele C, Ran H, Diskin B, Wei W, Jen J, Geer MJ, et al. SHP2 inhibition prevents adaptive resistance to MEK inhibitors in multiple cancer models. Cancer Discov (2018) 8(10):1237–49. doi: 10.1158/2159-8290.CD-18-0444
19. Smith AM, Zhang CRC, Cristino AS, Grady JP, Fink JL, Moore AS. PTEN deletion drives acute myeloid leukemia resistance to MEK inhibitors. Oncotarget (2019) 10(56):5755–67. doi: 10.18632/oncotarget.27206
20. Tsubaki M, Takeda T, Noguchi M, Jinushi M, Seki S, Morii Y, et al. Overactivation of akt contributes to MEK inhibitor primary and acquired resistance in colorectal cancer cells. Cancers (Basel) (2019) 11(12):1866. doi: 10.3390/cancers11121866
21. Liu S, Zou Q, Chen JP, Yao X, Guan P, Liang W, et al. Targeting enhancer reprogramming to mitigate MEK inhibitor resistance in preclinical models of advanced ovarian cancer. J Clin Invest (2021) 131(20):e145035. doi: 10.1172/JCI145035
22. Duncan JS, Whittle MC, Nakamura K, Abell AN, Midland AA, Zawistowski JS, et al. Dynamic reprogramming of the kinome in response to targeted MEK inhibition in triple-negative breast cancer. Cell (2012) 149(2):307–21. doi: 10.1016/j.cell.2012.02.053
23. Johnson GL, Stuhlmiller TJ, Angus SP, Zawistowski JS, Graves LM. Molecular pathways: adaptive kinome reprogramming in response to targeted inhibition of the BRAF-MEK-ERK pathway in cancer. Clin Cancer Res (2014) 20(10):2516–22. doi: 10.1158/1078-0432.CCR-13-1081
24. Claas AM, Atta L, Gordonov S, Meyer AS, Lauffenburger DA. Systems modeling identifies divergent receptor tyrosine kinase reprogramming to MAPK pathway inhibition. Cell Mol Bioeng (2018) 11(6):451–69. doi: 10.1007/s12195-018-0542-y
25. Wang J, Pollard K, Calizo A, Pratilas CA. Activation of receptor tyrosine kinases mediates acquired resistance to MEK inhibition in malignant peripheral nerve sheath tumors. Cancer Res (2021) 81(3):747–62. doi: 10.1158/0008-5472.CAN-20-1992
26. Wang J, Pollard K, Allen AN, Tomar T, Pijnenburg D, Yao Z, et al. Combined inhibition of SHP2 and MEK is effective in models of NF1-deficient malignant peripheral nerve sheath tumors. Cancer Res (2020) 80(23):5367–79. doi: 10.1158/0008-5472.CAN-20-1365
27. Buettner R, Nguyen LXT, Morales C, Chen MH, Wu X, Chen LS, et al. Targeting the metabolic vulnerability of acute myeloid leukemia blasts with a combination of venetoclax and 8-chloro-adenosine. J Hematol Oncol (2021) 14(1):70. doi: 10.1186/s13045-021-01076-4
28. Chou TC. Drug combination studies and their synergy quantification using the chou-talalay method. Cancer Res (2010) 70(2):440–6. doi: 10.1158/0008-5472.CAN-09-1947
29. Kun E, Tsang YTM, Ng CW, Gershenson DM, Wong KK. MEK inhibitor resistance mechanisms and recent developments in combination trials. Cancer Treat Rev (2021) 92:102137. doi: 10.1016/j.ctrv.2020.102137
30. Quispe PA, Lavecchia MJ, Leon IE. Focal adhesion kinase inhibitors in the treatment of solid tumors: Preclinical and clinical evidence. Drug Discov Today (2022) 27(2):664–74. doi: 10.1016/j.drudis.2021.11.025
31. Jessen WJ, Miller SJ, Jousma E, Wu J, Rizvi TA, Brundage ME, et al. MEK inhibition exhibits efficacy in human and mouse neurofibromatosis tumors. J Clin Invest (2013) 123(1):340–7. doi: 10.1172/JCI60578
32. Butler E, Schwettmann B, Geboers S, Hao G, Kim J, Nham K, et al. Functional imaging of RAS pathway targeting in malignant peripheral nerve sheath tumor cells and xenografts. Pediatr Blood Cancer (2020) 67(12):e28639. doi: 10.1002/pbc.28639
33. Irvine M, Stewart A, Pedersen B, Boyd S, Kefford R, Rizos H. Oncogenic PI3K/AKT promotes the step-wise evolution of combination BRAF/MEK inhibitor resistance in melanoma. Oncogenesis (2018) 7(9):72. doi: 10.1038/s41389-018-0081-3
34. Padhye A, Konen JM, Rodriguez BL, Fradette JJ, Ochieng JK, Diao L, et al. Targeting CDK4 overcomes EMT-mediated tumor heterogeneity and therapeutic resistance in KRAS-mutant lung cancer. JCI Insight (2021) 6(17):e148392. doi: 10.1172/jci.insight.148392
35. Boussemart L, Malka-Mahieu H, Girault I, Allard D, Hemmingsson O, Tomasic G, et al. eIF4F is a nexus of resistance to anti-BRAF and anti-MEK cancer therapies. Nature (2014) 513(7516):105–9. doi: 10.1038/nature13572
36. Wang W, Cui XW, Gu YH, Wei CJ, Li YH, Ren JY, et al. Combined cyclin-dependent kinase inhibition overcomes MAPK/Extracellular signal-regulated kinase kinase inhibitor resistance in plexiform neurofibroma of neurofibromatosis type I. J Invest Dermatol (2022) 142(3 Pt A):613–23 e7. doi: 10.1016/j.jid.2021.07.164
37. Caunt CJ, Sale MJ, Smith PD, Cook SJ. MEK1 and MEK2 inhibitors and cancer therapy: the long and winding road. Nat Rev Cancer (2015) 15(10):577–92. doi: 10.1038/nrc4000
38. Kozar I, Margue C, Rothengatter S, Haan C, Kreis S. Many ways to resistance: How melanoma cells evade targeted therapies. Biochim Biophys Acta Rev Cancer (2019) 1871(2):313–22. doi: 10.1016/j.bbcan.2019.02.002
39. Wagle N, Van Allen EM, Treacy DJ, Frederick DT, Cooper ZA, Taylor-Weiner A, et al. MAP kinase pathway alterations in BRAF-mutant melanoma patients with acquired resistance to combined RAF/MEK inhibition. Cancer Discov (2014) 4(1):61–8. doi: 10.1158/2159-8290.CD-13-0631
40. Kauko O, O’Connor CM, Kulesskiy E, Sangodkar J, Aakula A, Izadmehr S, et al. PP2A inhibition is a druggable MEK inhibitor resistance mechanism in KRAS-mutant lung cancer cells. Sci Transl Med (2018) 10(450):eaaq1093. doi: 10.1126/scitranslmed.aaq1093
41. Colombo I, Garg S, Danesh A, Bruce J, Shaw P, Tan Q, et al. Heterogeneous alteration of the ERBB3-MYC axis associated with MEK inhibitor resistance in a KRAS-mutated low-grade serous ovarian cancer patient. Cold Spring Harb Mol Case Stud (2019) 5(6):a004341. doi: 10.1101/mcs.a004341
42. Kechagia JZ, Ivaska J, Roca-Cusachs P. Integrins as biomechanical sensors of the microenvironment. Nat Rev Mol Cell Biol (2019) 20(8):457–73. doi: 10.1038/s41580-019-0134-2
43. Karamanos NK, Theocharis AD, Piperigkou Z, Manou D, Passi A, Skandalis SS, et al. A guide to the composition and functions of the extracellular matrix. FEBS J (2021) 288(24):6850–912. doi: 10.1111/febs.15776
44. Cooper J, Giancotti FG. Integrin signaling in cancer: Mechanotransduction, stemness, epithelial plasticity, and therapeutic resistance. Cancer Cell (2019) 35(3):347–67. doi: 10.1016/j.ccell.2019.01.007
45. Wantoch von Rekowski K, Konig P, Henze S, Schlesinger M, Zawierucha P, Januchowski R, et al. The impact of integrin-mediated matrix adhesion on cisplatin resistance of W1 ovarian cancer cells. Biomolecules (2019) 9(12):788. doi: 10.3390/biom9120788
46. Yu Q, Xiao W, Sun S, Sohrabi A, Liang J, Seidlits SK. Extracellular matrix proteins confer cell adhesion-mediated drug resistance through integrin alpha v in glioblastoma cells. Front Cell Dev Biol (2021) 9:616580. doi: 10.3389/fcell.2021.616580
47. Yang XH, Flores LM, Li Q, Zhou P, Xu F, Krop IE, et al. Disruption of laminin-integrin-CD151-focal adhesion kinase axis sensitizes breast cancer cells to ErbB2 antagonists. Cancer Res (2010) 70(6):2256–63. doi: 10.1158/0008-5472.CAN-09-4032
48. Buchstaller J, McKeever PE, Morrison SJ. Tumorigenic cells are common in mouse MPNSTs but their frequency depends upon tumor genotype and assay conditions. Cancer Cell (2012) 21(2):240–52. doi: 10.1016/j.ccr.2011.12.027
49. Sawai H, Okada Y, Funahashi H, Matsuo Y, Takahashi H, Takeyama H, et al. Activation of focal adhesion kinase enhances the adhesion and invasion of pancreatic cancer cells via extracellular signal-regulated kinase-1/2 signaling pathway activation. Mol Cancer (2005) 4:37. doi: 10.1186/1476-4598-4-37
50. Mitra SK, Schlaepfer DD. Integrin-regulated FAK-src signaling in normal and cancer cells. Curr Opin Cell Biol (2006) 18(5):516–23. doi: 10.1016/j.ceb.2006.08.011
51. Eke I, Cordes N. Focal adhesion signaling and therapy resistance in cancer. Semin Cancer Biol (2015) 31:65–75. doi: 10.1016/j.semcancer.2014.07.009
52. Tian T, Li CL, Fu X, Wang SH, Lu J, Guo H, et al. beta1 integrin-mediated multicellular resistance in hepatocellular carcinoma through activation of the FAK/Akt pathway. J Int Med Res (2018) 46(4):1311–25. doi: 10.1177/0300060517740807
53. Zhang Y, Sun X. Role of focal adhesion kinase in head and neck squamous cell carcinoma and its therapeutic prospect. Onco Targets Ther (2020) 13:10207–20. doi: 10.2147/OTT.S270342
54. Zhou J, Yi Q, Tang L. The roles of nuclear focal adhesion kinase (FAK) on cancer: a focused review. J Exp Clin Cancer Res (2019) 38(1):250. doi: 10.1186/s13046-019-1265-1
55. Dawson JC, Serrels A, Stupack DG, Schlaepfer DD, Frame MC. Targeting FAK in anticancer combination therapies. Nat Rev Cancer (2021) 21(5):313–24. doi: 10.1038/s41568-021-00340-6
56. Lazaro G, Smith C, Goddard L, Jordan N, McClelland R, Barrett-Lee P, et al. Targeting focal adhesion kinase in ER+/HER2+ breast cancer improves trastuzumab response. Endocr Relat Cancer (2013) 20(5):691–704. doi: 10.1530/ERC-13-0019
57. Golubovskaya VM, Huang G, Ho B, Yemma M, Morrison CD, Lee J, et al. Pharmacologic blockade of FAK autophosphorylation decreases human glioblastoma tumor growth and synergizes with temozolomide. Mol Cancer Ther (2013) 12(2):162–72. doi: 10.1158/1535-7163.MCT-12-0701
58. Yu C, Zhang M, Song J, Zheng X, Xu G, Bao Y, et al. Integrin-Src-YAP1 signaling mediates the melanoma acquired resistance to MAPK and PI3K/mTOR dual targeted therapy. Mol Biomed (2020) 1(1):12. doi: 10.1186/s43556-020-00013-0
59. Janne PA, Baik C, Su WC, Johnson ML, Hayashi H, Nishio M, et al. Efficacy and safety of patritumab deruxtecan (HER3-DXd) in EGFR inhibitor-resistant, EGFR-mutated non-small cell lung cancer. Cancer Discov (2022) 12(1):74–89. doi: 10.1158/2159-8290.CD-21-0715
60. Trojaniello C, Festino L, Vanella V, Ascierto PA. Encorafenib in combination with binimetinib for unresectable or metastatic melanoma with BRAF mutations. Expert Rev Clin Pharmacol (2019) 12(3):259–66. doi: 10.1080/17512433.2019.1570847
61. Indini A, Mandala M. Safety and efficacy evaluation of encorafenib plus binimetinib for the treatment of advanced BRAF-mutant melanoma patients. Expert Opin Drug Saf (2020) 19(10):1229–36. doi: 10.1080/14740338.2020.1817376
62. Shinde R, Terbuch A, Little M, Caldwell R, Kurup R, Riisnaes R, et al. Abstract CT143: Phase I study of the combination of a RAF-MEK inhibitor CH5126766 and FAK inhibitor defactinib in an intermittent dosing schedule with expansions in KRAS mutant cancers. Cancer Res (2020) 80(16_Supplement):CT143–CT. doi: 10.1158/1538-7445.AM2020-CT143
Keywords: neurofibromatosis type I, MPNST, MEK, drug resistance, integrins, FAK (focal adhesion kinase)
Citation: Gu Y, Wei C, Chung M, Li H, Guo Z, Long M, Li Y, Wang W, Aimaier R, Li Q and Wang Z (2022) Concurrent inhibition of FAK/SRC and MEK overcomes MEK inhibitor resistance in Neurofibromatosis Type I related malignant peripheral nerve sheath tumors. Front. Oncol. 12:910505. doi: 10.3389/fonc.2022.910505
Received: 01 April 2022; Accepted: 05 July 2022;
Published: 29 July 2022.
Edited by:
Erin B. Dickerson, University of Minnesota Twin Cities, United StatesReviewed by:
David Largaespada, University of Minnesota Twin Cities, United StatesCopyright © 2022 Gu, Wei, Chung, Li, Guo, Long, Li, Wang, Aimaier, Li and Wang. This is an open-access article distributed under the terms of the Creative Commons Attribution License (CC BY). The use, distribution or reproduction in other forums is permitted, provided the original author(s) and the copyright owner(s) are credited and that the original publication in this journal is cited, in accordance with accepted academic practice. No use, distribution or reproduction is permitted which does not comply with these terms.
*Correspondence: Zhichao Wang, c2htdXd6Y0AxNjMuY29t; ZHJfd2FuZ3poaWNoYW9AMTYzLmNvbQ==; Qingfeng Li, bGlxaW5nZmVuZ0BzaHNtdS5lZHUuY24=; ZHJfbGlxaW5nZmVuZzlAMTYzLmNvbQ==
†These authors have contributed equally to this work
Disclaimer: All claims expressed in this article are solely those of the authors and do not necessarily represent those of their affiliated organizations, or those of the publisher, the editors and the reviewers. Any product that may be evaluated in this article or claim that may be made by its manufacturer is not guaranteed or endorsed by the publisher.
Research integrity at Frontiers
Learn more about the work of our research integrity team to safeguard the quality of each article we publish.