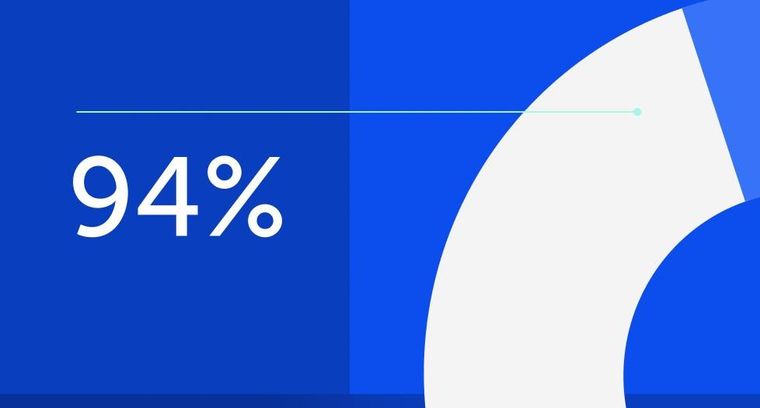
94% of researchers rate our articles as excellent or good
Learn more about the work of our research integrity team to safeguard the quality of each article we publish.
Find out more
REVIEW article
Front. Oncol., 26 May 2022
Sec. Pharmacology of Anti-Cancer Drugs
Volume 12 - 2022 | https://doi.org/10.3389/fonc.2022.907684
This article is part of the Research TopicNanomaterials and Multimodal Tumor TherapyView all 45 articles
Breast cancer (BC) is the most common malignant tumor in women. There are different risk characteristics and treatment strategies for different subtypes of BC. The tumor microenvironment (TME) is of great significance for understanding the occurrence, development, and metastasis of tumors. The TME plays an important role in all stages of BC metastasis, immune monitoring, immune response avoidance, and drug resistance, and also plays an important role in the diagnosis, prevention, and prognosis of BC. Smart nanosystems have broad development prospect in the regulation of the BC drug delivery based on the response of the TME. In particular, TME-responsive nanoparticles cleverly utilize the abnormal features of BC tissues and cells to achieve targeted transport, stable release, and improved efficacy. We here present a review of the mechanisms underlying the response of the TME to BC to provide potential nanostrategies for future BC treatment.
Breast cancer (BC) is a common cancer that affects women’s health worldwide, and is also the most common malignant tumor among women (1, 2). According to the Report of the American Cancer Society, BC is second only to lung cancer in the number of cancer-related deaths among women. In recent years, the incidence of BC has been increasing at an annual rate of approximately 0.3%, with 2.3 million new cases worldwide in 2020 and a significant increase in mortality from 1990 to 2015 (3, 4). According to Surveillance, Epidemiology and End Results (SEER) data, the 5-year survival rate of patients with stage IIIA and stage IIIB BC was 52% and 48%, respectively, and the median survival of patients with stage III BC was 4.9 years (2, 5–8).
The histological types of BC are (1) non-invasive carcinoma, including ductal carcinoma in situ and lobular carcinoma in situ; and (2) invasive cancer, including no special type of invasive cancer, invasive lobular carcinoma, tubular carcinoma, and mucinous carcinoma (9–12). Based on molecular evidence, BC can be categorized into three groups: hormone receptor [estrogen receptor (ER) or progesterone receptor (PR)]-positive, human epidermal receptor 2 (HER2) or ERBB2 receptor-positive, and triple-negative BC (TNBC; ER−, PR−, HER2−). Among them, the luminal subtype can be further divided into luminal A and luminal B tumors, and TNBC can be further divided into six subtypes: basal-like1/basal-like2, mesenchymal, mesenchymal stem-like, immunomodulatory, luminal androgen receptor, and an unspecified group (11, 13–17). The luminal A subtype has the best clinical prognosis, accounting for 40% of all subtypes, and is characterized by high levels of ER expression; therefore, patients with luminal A BC are more likely to benefit from hormone therapy alone. Another less common subtype, luminal B BC (accounting for approximately 20% of all subtypes), is characterized by low levels of ER expression but high expression levels of proliferation-related genes and may require chemotherapy. HER2-enriched tumors (accounting for 15–20% of subtypes) are also characterized by overexpression of proliferation-related genes. The basal-like subtype is characterized by up-regulated expression of genes expressed in basal/myoepithelial cells. The specific molecular pathophysiological mechanism of TNBC tumors is still unclear, accounting for about 15% of all subtypes, and 71% of TNBC tumors are in the basal-like group (18–20).
The three main tumor subtypes of BC, characterized by ER or PR expression and amplification of the ERBB2 gene, have different risk characteristics and treatment strategies, with the optimal treatment for each patient depending on the tumor subtype, anatomic tumor stage, and patient preference (11, 21–25). In addition, the role of genomic analyses such as detection of BRCA1 or BRCA2 germline mutations in treatment decisions has been shown to play an important role in clinical outcomes in BC (26–29).
For patients without metastatic disease, treatment aims to eradicate the tumor from the breast and regional lymph nodes and to prevent recurrence. According to the clinical setting, systemic therapy for non-metastatic BC is mainly based on the molecular characteristics (30–33): patients with hormone receptor-positive tumors receive endocrine therapy and a few patients also receive chemotherapy. Patients with ERBB2-positive tumors are typically treated with ERBB2-targeted antibodies or small-molecule inhibitors (trastuzumab-based) in combination with chemotherapy. Patients with TNBC tumors receive only chemotherapy (6, 14, 18). In addition, local treatment in patients with non-metastatic BC includes surgical excision (including axillary lymph node sampling or excision), and postoperative radiotherapy should be considered if lumpectomy is performed. However, the local treatment of patients with non-metastatic BC is increasingly being performed with some systemic therapy prior to surgery, such as neoadjuvant chemotherapy, followed by postoperative treatment tailored to the preoperative response (18, 34). In contrast, treatment for metastatic BC is also based on molecular subtypes, with the goal of prolonging life and reducing symptoms, which is more complex and personalized (7, 17). Metastatic BC also has a poor prognosis, with a median overall survival of approximately 1 year for metastatic TNBC, compared with approximately 5 years for the other two subtypes (8).
The proliferation of cancer cells and their spread to other parts of the body depend on the microenvironment of the organ, which is also known as the “seed and soil” hypothesis. In brief, cancer-associated fibroblasts (CAFs) in the tumor microenvironment (TME) promote metastasis and secrete large amounts of pro-inflammatory cytokines and chemokines, growth factors, and extracellular matrix (ECM) components. In addition to binding to adjacent tumor cells, CAFs interact with other stromal components in the microenvironment through complex crosstalk. These cells are involved in various activities such as angiogenesis and ECM remodeling, which contribute to tumor growth and metastasis. Thus, the TME is, to some extent, the protagonist of cancer progression, and is more genetically stable than the cancer itself. Therefore, increasing studies aiming toward anticancer drug development are currently focused on strategies targeting the TME (35–37). For example, owing to the genetic and phenotypic heterogeneity of BC cells, the cells or pathways vary among patients or at different periods in the disease course in the same patient, which poses a challenge in conducting targeted research for treatment (38). TME-targeted therapies that use tumor cells, key TME components, or secreted bioactive molecules alone or in combination as therapeutic targets are gaining increasing attention.
Here, we focus on research progress in the development of innovative nanomaterials delivery systems as the key to providing intelligent delivery systems based on active targeting and internalization in the TME and cancer cells (39, 40). In particular, the size and versatility of nano-loaded particles allow for chemical or genetic modification of their surfaces with various targeting groups or active ligands that trigger the specific orientation and recognition of biological targets, enabling them to deliver a steady amount of drugs at the tumor site and control their ability to deliver the drugs effectively (41–43). This review therefore provides an overview of the mechanisms of the TME response to BC, along with examples of the diversity of nanoparticle (NP)-based drug delivery systems developed to date or with potential to target the TME, which can offer an effective dual targeting strategy for BC cells and the TME as the potential key to realizing the successful treatment of BC (44, 45).
The TME is the internal environment in which tumor cells generate and live, which includes not only the tumor cells themselves but also fibroblasts, immune and inflammatory cells, glial cells, and other cells closely related to and interacting with tumor cells. The TME also includes interstitial cells, microvessels, and biomolecules such as secretory factors, proteins, and RNA in the surrounding area (46–49). The TME has long been a key focus of research and the core direction toward gaining a comprehensive understanding of the occurrence and development of tumors. In particular, the TME plays an important role in the transfer process, immune escape, and drug resistance in tumor metastasis, requiring immune monitoring of the TME at various stages (50) for the diagnosis, prevention, and prognosis of tumors (51–54).
Moreover, the TME plays a key role in several malignant phenotypes associated with tumor progression, including the acquisition of aggressive phenotypes, cell migration ability, chemotherapy resistance, protection from anti-tumor immune responses, and neovasculogenesis (46, 47, 55). The TME can also recombine with the ECM to enhance the permeability of chemotherapeutic drugs, as well as with NPs that enable the intelligent coupling of chemotherapy and immunotherapy by increasing immunogenicity and stimulating anti-tumor immunity (35).
Nanotechnology has developed rapidly in recent years, providing a good means to overcome the bottleneck problem of non-specific and non-selective damage to body tissues caused by traditional therapies (e.g., chemotherapy, radiotherapy and immunotherapy), and exhibiting unique advantages in improving the effects of drugs and radiation therapy while reducing adverse reactions (44, 56–58). On the one hand, multifunctional nanocarriers can selectively transport therapeutic drugs by utilizing the difference between the tumor tissue and normal tissue to enhance drug permeability and retention. On the other hand, a series of unique physical and chemical properties produced by the TME, such as weak acidity, a reducing environment, abnormal temperature gradient, overexpressed proteins and enzymes, and hypoxia, can be used to regulate the release of loaded drugs by nanocarriers (44, 59).
Dr. Otto Heinrich Warburg’s work on sea urchin embryology led to pioneering research on the physiological effects of ROS. His discoveries in the field of oxidative respiration earned him a Nobel Prize in 1931, and in 1926, Warburg also pioneered a model of tumor maintenance of oxidation and energy, hoping to exert a profound influence on cell physiology and metabolism by deeply exploring the basic properties of ROS (33).
ROS represent the products of single electron reduction of oxygen in the body, as a form of electron leakage before passing to the terminal oxidase respiratory chain, consuming approximately 2% of the oxygen generated, including the single-electron reduction product of oxygen superoxide anion (O2·–), two-electron reduction product hydrogen peroxide (H2O2), three-electron product hydroxyl free radical (·OH), and nitric oxide, among other highly reactive agents (60). Intracellular ROS are by-products of mitochondrial processes, metabolism, and enzyme activity. Under normal physiological conditions, a large number of electrons are released and reduced by oxygen molecules in the high-oxygen environment and the highly reduced respiratory chain of mitochondria during the transition from state III to state IV (31).
Under normal physiological conditions, ROS can be effectively neutralized by the enzymes superoxide dismutase, catalase, glutathione, and thioredoxin to control the equilibrium oxidation-reduction (redox) state. However, when redox homeostasis is destroyed or relieved, changes in ROS levels will lead to physiological and pathological changes (61, 62). In addition, ROS play a positive role in regulating intracellular signal transduction pathways, with numerous biological conduits being constrained by a variety of ROS species, which are the key to maintaining cellular homeostasis. Any skew in this delicate balance is accompanied by a wave of events that can have either negative or positive impacts for the cell.
From the perspective of cancer, the conventional view that ROS cause widespread damage and chaos within cells has long been debated in the scientific community (63–65). Recently, great progress has been made in the understanding of ROS biology, and there is increasing evidence that the effects of ROS are gradient-dependent, with a threshold of habituation, which affects their signal transduction and toxicity induction function, thus resulting in either “good” or “bad” ROS (33). For example, ROS play a key role in the activation of tumor-promoting signaling pathways (64, 66, 67). Moreover, abnormal production of ROS and ineffective neutralization of excessive ROS levels can lead to tumor growth and progression through different signaling pathways, including phosphatidylinositol 3-kinase/protein kinase inhibitor/mammalian target of rapamycin (PI3/Akt/mTOR), vascular endothelial growth factor (VEGF)/VEGF receptor, phosphatase and tensin congeners (PTEN), and matrix metalloproteinase (MMP) pathways (68). Therefore, an intelligent collaborative treatment system can be developed to ensure the release of chemotherapeutic drugs on demand and reduce ROS production.
The acidic microenvironment caused by massive anaerobic glycolysis is one of the important characteristics of malignant tumors, and is also an important factor inducing the occurrence, metastasis, and drug resistance of BC. In recent years, with increasing understanding of the acidic TME, it has been considered a new target for tumor diagnosis and treatment, which is of great significance for the design of pH-responsive nanomedicine and nano-diagnosis (69).
Gong et al. (70) developed a pH-responsive co-delivery platform for metformin and small interfering RNA (siRNA) targeting the immune checkpoint inhibitor FGL1 (siFGL1) based on hybrid biomimetic membrane-camouflaged poly (lactic-co-glycolic acid) NPs. This pH-triggered CO2 gas generation nanoplatform was developed using the metformin guanidine group, which can react reversibly with CO2 to produce a metformin-CO2 complex. Moreover, the capture/release of CO2 depends on the pH value, thus improving the in vivo lysotic escape of the siRNA to achieve the cytoplasmic transfer of siRNA. Metformin promotes programmed death ligand 1 (PD-L1) by activating adenosine monophosphate-induced protein kinase degradation, and then blocks the inhibitory signal of PD-L1, while siFGL1 can silence the FGL1 gene, promote the T cell-mediated immune response, and enhance anti-tumor immunity. The combination of these two drugs exhibited a high synergistic therapeutic effect on BC in vivo and in vitro (70).
In addition, Shen et al. (71) developed a robust nanoplatform fabricated from a TME pH-responsive polymer (MEO-PEG-B-PPMEMa) and a cationic lipid compound (G0-C14) for the in vivo delivery of cytotoxic saponins in BC treatment. The nanoplatform responds to the TME-specific pH with the rapid release of the saporin/G0-C14 complex, which significantly enhanced the cytoplasmic uptake of saporin and subsequent intracellular escape by tumor cells, thereby effectively inhibiting tumor growth (71).
Enzymes play an important role in metabolism. Thus, abnormal metabolism in the TME will result in the overexpression of certain enzymes. Typical examples are MMPs, which are calcium- and zinc-dependent endopeptidases secreted by cancer cells, tumor blood vessels, and CAFs, and play a key role in cancer cell ECM remodeling (72). Other overexpressed enzymes in the TME include hyaluronidase and lysyl oxidase, along with other enzymes that are overexpressed in specific tumors (73).
Kashyap et al. (74) synthesized a hydrophobic acrylate monomer from the natural resource 3-pentachylphenol by enzyme and thermal reactions, which was co-polymerized with the hydrophilic monomer polyethylene glycol acrylate. This copolymer core-shell NP system was then used for the extracellular and intracellular delivery of doxorubicin (DOX)-responsive polymer nanoscaffolds to improve cancer treatment. In the presence of esterase (pH = 7.4, 37°C), the amphiphilic copolymer broke down in a slow and controlled manner within 12 h, releasing >95% of the drugs and achieving controlled release of the drugs in cells (74). For breast cancer, MNPs were successfully conjugated with MTX by Nosrati et al. and this drug delivery system is dependent on the release of the MTX within the lysosomal compartment for BC cells treatment (75).
The partial pressure of oxygen (PO2) in various tumors is generally below 60 mmHg and gradually decreases with tumor progression. This reduction mainly occurs because the structure and function of tumor microvessels are abnormal, and the diffusion distance between blood vessels and the tumor cells increases, thereby preventing the effective transport of O2 to specific sites. Moreover, the reduced blood oxygenation capacity due to tumor growth or therapeutic anemia also contributes to the local PO2 reduction (72). The resulting hypoxic environment not only promotes tumor growth, progression, metastasis and damage normal tissues but also reduces the efficacy of radiotherapy and chemotherapy (76).
Mpekris et al. (77) found that the blood vessels of lung metastases of BC were compressed, leading to hypoxia. Based on this principle, mechanical tranilast therapy was used to decompress the blood vessels of lung metastasis in mice so as to restore perfusion and relieve hypoxia. This strategy enabled the immune checkpoint blocker atezolizumab and the nanodrug doxil to more effectively exert cytotoxic effects on metastases and stimulate an antitumor immune response (77).
Compared with normal tissue, the tumor tissue has a higher temperature, which makes it possible to control and release drugs by external heating at the tumor site, and this property has been exploited in the development of smart drug delivery systems (SDDSs) targeting tumors (74, 78–82). Ding et al. (79) designed a multifunctional SDDS with a double-layer structure: a thermo-sensitive copolymer as the building block of the core-shell structure, two chemotherapeutic drugs [paclitaxel (PTX) and DOX; NP-PD) were encapsulated to form copolymers, and the siRNA (NP-PD-S) affecting survival was absorbed on the surface to form nanostructures, which were finally wrapped by self-polymerizing dopamine (PDA) membranes (NP-PD-S-PDA). This PDA film not only exhibited a photothermal therapeutic effect but also had a protective effect against the uncontrolled release of the drugs. In vitro experiments with the MDA-MB-231 BC cell line showed that the survival rate of NP-PD-S-PDA was significantly decreased after 5 min of laser irradiation, indicating that this is an effective combination therapy. In vivo experiments with female BALB/C nude mice also showed that in tumors irradiated by NP-PD-S-PDA plus near infrared light, PDA generated sufficient heat, and thermal ablation led to collapse of the NPs, thereby triggering the release of PTX and DOX within the tumor to ultimately achieve antitumor effects (79).
In addition, Hu et al. (80) developed a system for delivery of the hydrophobic chemotherapy drug PTX and the biological macromolecule interleukin (IL)-12 through the low-temperature expansion effect of the acid-sensitive material MPEG-Dlinkm-PDLla and pluronic F127 co-delivered NPs. PTX and IL-12 jointly activated T lymphocytes and natural killer cells to release interferon-gamma, selectively inhibited regulatory T cells, induced the M1-type differentiation of tumor-associated macrophages, improved the tumor immunosuppressive microenvironment, significantly inhibited the growth and metastasis of 4T1 BC cells, and prolonged the overall survival of tumor-bearing mice (80).
The tumor microenvironment is the ecosystem that surrounds a tumor inside the body. It includes immune cells, the extracellular matrix, blood vessels and other cells, like fibroblasts. CAFs are the most abundant cells in the TME matrix, secreting a large number of growth factors (e.g., transforming growth factor-β, VEGF, platelet-derived growth factor), cytokines, interleukins, chemokines, and ECM proteins (e.g., MMPs), which all have an impact on tumor development. CAFs actively reconstruct the cellular and stromal components of the TME. M2-polarized macrophages promote metastasis and contribute to the development of an immunosuppressive environment. Endothelial cells and pericytes contribute to tumor angiogenesis and handle the oxygen supply. The interstitial ECM supports the tumor structure and regulates drug penetration. The external matrix basement membrane acts as a barrier around the tumor. Both the external matrix and blood vessels contribute to increased interstitial fluid pressure and tumor hypoxia, which is a major barrier to tumor treatment. MMP also supports tumor growth, creates physical barriers to drugs and immune invasion, and promotes cancer invasion (83, 84). In short, normal fibroblasts inhibit tumor formation, whereas CAFs promote BC cell proliferation, angiogenesis, and the inflammatory response, and remodeling the ECM promotes the malignant phenotype of BC cells, resulting in a poor prognosis (85, 86).
Some biological markers of CAFs can be used to identify and target CAFs in the BC TME, which creates opportunities for nanodelivery systems to specifically target the BC metastatic TME. These proteins include fibroblast activating protein (FAP), alpha-smooth muscle actin, fibroblast-specific protein, vimentin, and proline 4-hydroxylase (87, 88). As the most common tumor-targeting CAF antigen, FAP is overexpressed on the surface of CAFs, and nanoparticles can specifically target FAP to promote the infiltration and accumulation of nano-loaded drugs in tumor tissues, thus playing an anti-tumor role. Zhen et al. (89) developed a photoimmunotherapy nanostrategy using ferritin as a photosensitizer carrier and a single-chain variable fragment of anti-FAP antibody conjugated with ferritin.
There has been great progress in the development of intelligent NPs for the effective loading of anti-BC drugs, specific enrichment of tumor cells and tissues, and the controlled release and efficient delivery of drugs. In particular, regulation based on the response of the TME including multifunctional nanoparticles has a broad development prospect for improving BC drug delivery. TME-responsive NPs cleverly utilize the abnormal features of BC tissues to achieve targeted transport, stable release, and improved drug efficacy.
However, there is still room for improvement in TME-responsive nanotechnology. First, there are limitations of the nanomaterials themselves, including limited understanding of the mechanism underlying the interaction between materials and other systems in the body, which makes clinical translation difficult. In addition, the interaction and influence of different components in the nanosystem represent important questions that have not yet been elucidated. Therefore, the design of improved NPs including multifunctional NPs is a promising direction in the future.
All authors contributed to the design of the study and writing of the manuscript. XL, KH, and HC undertook the research, YW and ZN wrote the main manuscript text and prepared figures. QZ and YW revised the article critically for important intellectual content and final approval of the version to be submitted. All authors reviewed the manuscript.
The authors declare that the research was conducted in the absence of any commercial or financial relationships that could be construed as a potential conflict of interest.
All claims expressed in this article are solely those of the authors and do not necessarily represent those of their affiliated organizations, or those of the publisher, the editors and the reviewers. Any product that may be evaluated in this article, or claim that may be made by its manufacturer, is not guaranteed or endorsed by the publisher.
BC, breast cancer; TME, tumor microenvironment; HER2, human epidermal growth factor receptor 2; ER, estrogen receptors; PR, progesterone receptors; TNBC, triple negative breast cancer; CAFs, cancer-associated fibroblasts; ECM, extracellular matrix; NPs, nanoparticles; ROS, reactive oxygen species; DOX, doxorubicin.
1. Ferlay J, Colombet M, Soerjomataram I, Parkin DM, Pineros M, Znaor A, et al. Cancer Statistics for the Year 2020: An Overview. Int J Cancer (2021). doi: 10.1002/ijc.33588
2. Siegel RL, Miller KD, Fuchs HE, Jemal A. Cancer Statistics, 2021. CA Cancer J Clin (2021) 71(1):7–33. doi: 10.3322/caac.21654
3. Shao H, Varamini P. Breast Cancer Bone Metastasis: A Narrative Review of Emerging Targeted Drug Delivery Systems. Cells (2022) 11(3):388. doi: 10.3390/cells11030388
4. Azamjah N, Soltan-Zadeh Y, Zayeri F. Global Trend of Breast Cancer Mortality Rate: A 25-Year Study. Asian Pac J Cancer Prev (2019) 20(7):2015–20. doi: 10.31557/APJCP.2019.20.7.2015
5. Gradishar WJ, Anderson BO, Abraham J, Aft R, Agnese D, Allison KH, et al. Breast Cancer, Version 3.2020, NCCN Clinical Practice Guidelines in Oncology. J Natl Compr Canc Netw (2020) 18(4):452–78. doi: 10.6004/jnccn.2020.0016
6. Cardoso F, Kyriakides S, Ohno S, Penault-Llorca F, Poortmans P, Rubio IT, et al. Early Breast Cancer: ESMO Clinical Practice Guidelines for Diagnosis, Treatment and Follow-Up. Ann Oncol (2019) 30(10):1674. doi: 10.1093/annonc/mdz189
7. Tryfonidis K, Senkus E, Cardoso MJ, Cardoso F. Management of Locally Advanced Breast Cancer-Perspectives and Future Directions. Nat Rev Clin Oncol (2015) 12(3):147–62. doi: 10.1038/nrclinonc.2015.13
8. Newman LA. Epidemiology of Locally Advanced Breast Cancer. Semin Radiat Oncol (2009) 19(4):195–203. doi: 10.1016/j.semradonc.2009.05.003
9. Fodor A, Brombin C, Mangili P, Borroni F, Pasetti M, Tummineri R, et al. Impact of Molecular Subtype on 1325 Early-Stage Breast Cancer Patients Homogeneously Treated With Hypofractionated Radiotherapy Without Boost: Should the Indications for Radiotherapy be More Personalized? Breast (2021) 55:45–54. doi: 10.1016/j.breast.2020.12.004
10. van der Meer DJ, Kramer I, van Maaren MC, van Diest PJ, Linn SC, Maduro JH, et al. Comprehensive Trends in Incidence, Treatment, Survival and Mortality of First Primary Invasive Breast Cancer Stratified by Age, Stage and Receptor Subtype in the Netherlands Between 1989 and 2017. Int J Cancer (2021) 148(9):2289–303. doi: 10.1002/ijc.33417
11. Tadros AB, Sevilimedu V, Giri DD, Zabor EC, Morrow M, Plitas G. Survival Outcomes for Metaplastic Breast Cancer Differ by Histologic Subtype. Ann Surg Oncol (2021) 28(8):4245–53. doi: 10.1245/s10434-020-09430-5
12. Yalcin B. Overview on Locally Advanced Breast Cancer: Defining, Epidemiology, and Overview on Neoadjuvant Therapy. Exp Oncol (2013) 35(4):250–2.
13. Russnes HG, Lingjaerde OC, Borresen-Dale AL, Caldas C. Breast Cancer Molecular Stratification: From Intrinsic Subtypes to Integrative Clusters. Am J Pathol (2017) 187(10):2152–62. doi: 10.1016/j.ajpath.2017.04.022
14. Bayani J, Yao CQ, Quintayo MA, Yan F, Haider S, D'Costa A, et al. Molecular Stratification of Early Breast Cancer Identifies Drug Targets to Drive Stratified Medicine. NPJ Breast Cancer (2017) 3:3. doi: 10.1038/s41523-016-0003-5
15. Yao K, Goldschmidt R, Turk M, Wesseling J, Stork-Sloots L, de Snoo F, et al. Molecular Subtyping Improves Diagnostic Stratification of Patients With Primary Breast Cancer Into Prognostically Defined Risk Groups. Breast Cancer Res Treat (2015) 154(1):81–8. doi: 10.1007/s10549-015-3587-9
16. Wang DY, Jiang Z, Ben-David Y, Woodgett JR, Zacksenhaus E. Molecular Stratification Within Triple-Negative Breast Cancer Subtypes. Sci Rep (2019) 9(1):19107. doi: 10.1038/s41598-019-55710-w
17. Ruswendro D, Syamsu SA, Thabry R, Seweng A, Usman AN. Association Between Molecular Subtype of Local Advanced Breast Cancer With Ca 15-3 Level. Breast Dis (2021) 40(S1):S119–22. doi: 10.3233/BD-219018
18. Waks AG, Winer EP. Breast Cancer Treatment: A Review. JAMA (2019) 321(3):288–300. doi: 10.1001/jama.2018.19323
19. Tyagi NK, Dhesy-Thind S. Clinical Practice Guidelines in Breast Cancer. Curr Oncol (2018) 25(Suppl 1):S151–60. doi: 10.3747/co.25.3729
20. Xie P, An R, Yu S, He J, Zhang H. A Novel Immune Subtype Classification of ER-Positive, PR-Negative and HER2-Negative Breast Cancer Based on the Genomic and Transcriptomic Landscape. J Transl Med (2021) 19(1):398. doi: 10.1186/s12967-021-03076-x
21. Reid S, Haddad D, Tezak A, Weidner A, Wang X, Mautz B, et al. Impact of Molecular Subtype and Race on HR+, HER2- Breast Cancer Survival. Breast Cancer Res Treat (2021) 189(3):845–52. doi: 10.1007/s10549-021-06342-0
22. Mills MN, Thawani C, Figura NB, Oliver DE, Soyano AE, Etame A, et al. Breast Cancer Subtype Predicts Clinical Outcomes After Stereotactic Radiation for Brain Metastases. J Neurooncol (2021) 152(3):591–601. doi: 10.1007/s11060-021-03735-5
23. Li Y, Wang S, Yang W, Liu H. Prognostic Significance of Molecular Subtype, Metastatic Site and Primary Tumor Surgery for Survival in Primary Metastatic Breast Cancer: A SEER-Based Study. Med (Baltimore) (2021) 100(27):e26619. doi: 10.1097/MD.0000000000026619
24. Hong J, Tong Y, He J, Chen X, Shen K. Association Between Tumor Molecular Subtype, Clinical Stage and Axillary Pathological Response in Breast Cancer Patients Undergoing Complete Pathological Remission After Neoadjuvant Chemotherapy: Potential Implications for De-Escalation of Axillary Surgery. Ther Adv Med Oncol (2021) 13:1758835921996673. doi: 10.1177/1758835921996673
25. Ding S, Sun X, Lu S, Wang Z, Chen X, Shen K. Association of Molecular Subtype Concordance and Survival Outcome in Synchronous and Metachronous Bilateral Breast Cancer. Breast (2021) 57:71–9. doi: 10.1016/j.breast.2021.03.005
26. Im SA, Xu B, Li W, Robson M, Ouyang Q, Yeh DC, et al. Olaparib Monotherapy for Asian Patients With a Germline BRCA Mutation and HER2-Negative Metastatic Breast Cancer: OlympiAD Randomized Trial Subgroup Analysis. Sci Rep (2020) 10(1):8753. doi: 10.1038/s41598-020-63033-4
27. Metzger-Filho O, Collier K, Asad S, Ansell PJ, Watson M, Bae J, et al. Matched Cohort Study of Germline BRCA Mutation Carriers With Triple Negative Breast Cancer in Brightness. NPJ Breast Cancer (2021) 7(1):142. doi: 10.1038/s41523-021-00349-y
28. Su Y, Yao Q, Xu Y, Yu C, Zhang J, Wang Q, et al. Characteristics of Germline Non-BRCA Mutation Status of High-Risk Breast Cancer Patients in China and Correlation With High-Risk Factors and Multigene Testing Suggestions. Front Genet (2021) 12:674094. doi: 10.3389/fgene.2021.674094
29. Atci MM, Geredeli C, Ay S, Sakin A, Erturk B, Secmeler S, et al. Clinical and Pathological Characteristics of Patients With High-Risk Breast Cancer Based on BRCA Mutation Profiles: A Retrospective Study. Eur J Breast Health (2021) 17(2):123–7. doi: 10.4274/ejbh.galenos.2020.6346
30. Spring LM, Wander SA, Andre F, Moy B, Turner NC, Bardia A. Cyclin-Dependent Kinase 4 and 6 Inhibitors for Hormone Receptor-Positive Breast Cancer: Past, Present, and Future. Lancet (2020) 395(10226):817–27. doi: 10.1016/S0140-6736(20)30165-3
31. Shashni B, Nagasaki Y. Nitroxide Radical-Containing Nanoparticles Attenuate Tumorigenic Potential of Triple Negative Breast Cancer. Biomaterials (2018) 178:48–62. doi: 10.1016/j.biomaterials.2018.05.042
32. Reinert T, Barrios CH. Optimal Management of Hormone Receptor Positive Metastatic Breast Cancer in 2016. Ther Adv Med Oncol (2015) 7(6):304–20. doi: 10.1177/1758834015608993
33. Raman D, Foo CH, Clement MV, Pervaiz S. Breast Cancer: A Molecular and Redox Snapshot. Antioxid Redox Signal (2016) 25(6):337–70. doi: 10.1089/ars.2015.6546
34. Wen N, Qiu J, Xu L, Wang Y, Zhang J, Xie Y, et al. Adjuvant Chemotherapy Guidance for Pt1-3N0-1 Breast Cancer Patients With HR(+), HER2(-) Subtype: A Cohort Study Based on the SEER Database. Ann Transl Med (2021) 9(24):1779. doi: 10.21037/atm-21-5937
35. Truffi M, Mazzucchelli S, Bonizzi A, Sorrentino L, Allevi R, Vanna R, et al. Nano-Strategies to Target Breast Cancer-Associated Fibroblasts: Rearranging the Tumor Microenvironment to Achieve Antitumor Efficacy. Int J Mol Sci (2019) 20(6):1263. doi: 10.3390/ijms20061263
36. Chen X, Song E. Turning Foes to Friends: Targeting Cancer-Associated Fibroblasts. Nat Rev Drug Discov (2019) 18(2):99–115. doi: 10.1038/s41573-018-0004-1
37. Tchou J, Conejo-Garcia J. Targeting the Tumor Stroma as a Novel Treatment Strategy for Breast Cancer: Shifting From the Neoplastic Cell-Centric to a Stroma-Centric Paradigm. Adv Pharmacol (2012) 65:45–61. doi: 10.1016/B978-0-12-397927-8.00003-8
38. Stingl J, Caldas C. Molecular Heterogeneity of Breast Carcinomas and the Cancer Stem Cell Hypothesis. Nat Rev Cancer (2007) 7(10):791–9. doi: 10.1038/nrc2212
39. Lu P, Wang G, Qian T, Cai X, Zhang P, Li M, et al. The Balanced Microenvironment Regulated by the Degradants of Appropriate PLGA Scaffolds and Chitosan Conduit Promotes Peripheral Nerve Regeneration. Mater Today Bio (2021) 12:100158. doi: 10.1016/j.mtbio.2021.100158
40. Han Z, Gong C, Li J, Guo H, Chen X, Jin Y, et al. Immunologically Modified Enzyme-Responsive Micelles Regulate the Tumor Microenvironment for Cancer Immunotherapy. Mater Today Bio (2022) 13:100170. doi: 10.1016/j.mtbio.2021.100170
41. Batra H, Pawar S, Bahl D. Curcumin in Combination With Anti-Cancer Drugs: A Nanomedicine Review. Pharmacol Res (2019) 139:91–105. doi: 10.1016/j.phrs.2018.11.005
42. Truffi M, Fiandra L, Sorrentino L, Monieri M, Corsi F, Mazzucchelli S. Ferritin Nanocages: A Biological Platform for Drug Delivery, Imaging and Theranostics in Cancer. Pharmacol Res (2016) 107:57–65. doi: 10.1016/j.phrs.2016.03.002
43. Colombo M, Rizzuto MA, Pacini C, Pandolfi L, Bonizzi A, Truffi M, et al. Half-Chain Cetuximab Nanoconjugates Allow Multitarget Therapy of Triple Negative Breast Cancer. Bioconjug Chem (2018) 29(11):3817–32. doi: 10.1021/acs.bioconjchem.8b00667
44. Tong R, Langer R. Nanomedicines Targeting the Tumor Microenvironment. Cancer J (2015) 21(4):314–21. doi: 10.1097/PPO.0000000000000123
45. Omidi Y, Barar J. Targeting Tumor Microenvironment: Crossing Tumor Interstitial Fluid by Multifunctional Nanomedicines. Bioimpacts (2014) 4(2):55–67. doi: 10.5681/bi.2014.021
46. Bezdenezhnykh N, Semesiuk N, Lykhova O, Zhylchuk V, Kudryavets Y. Impact of Stromal Cell Components of Tumor Microenvironment on Epithelial-Mesenchymal Transition in Breast Cancer Cells. Exp Oncol (2014) 36(2):72–8.
47. Mao Y, Keller ET, Garfield DH, Shen K, Wang J. Stromal Cells in Tumor Microenvironment and Breast Cancer. Cancer Metastasis Rev (2013) 32(1-2):303–15. doi: 10.1007/s10555-012-9415-3
48. Dong M, Shen W, Yang G, Yang Z, Li X. Analysis of M6a Methylation Modification Patterns and Tumor Immune Microenvironment in Breast Cancer. Front Cell Dev Biol (2022) 10:785058. doi: 10.3389/fcell.2022.785058
49. Munkacsy G, Santarpia L, Gyorffy B. Gene Expression Profiling in Early Breast Cancer-Patient Stratification Based on Molecular and Tumor Microenvironment Features. Biomedicines (2022) 10(2):248. doi: 10.3390/biomedicines10020248
50. Gu X, Gao Y, Wang P, Wang L, Peng H, He Y, et al. Nano-Delivery Systems Focused on Tumor Microenvironment Regulation and Biomimetic Strategies for Treatment of Breast Cancer Metastasis. J Control Release (2021) 333:374–90. doi: 10.1016/j.jconrel.2021.03.039
51. Xu Q, Yan X, Han Z, Jin X, Jin Y, Sun H, et al. Immune Cell Infiltration and Relevant Gene Signatures in the Tumor Microenvironment That Significantly Associates With the Prognosis of Patients With Breast Cancer. Front Mol Biosci (2022) 9:823911. doi: 10.3389/fmolb.2022.823911
52. Zhu J, Shen Y, Wang L, Qiao J, Zhao Y, Wang Q. A Novel 12-Gene Prognostic Signature in Breast Cancer Based on the Tumor Microenvironment. Ann Transl Med (2022) 10(3):143. doi: 10.21037/atm-21-6748
53. Wang X, Xie T, Luo J, Zhou Z, Yu X, Guo X. Radiomics Predicts the Prognosis of Patients With Locally Advanced Breast Cancer by Reflecting the Heterogeneity of Tumor Cells and the Tumor Microenvironment. Breast Cancer Res (2022) 24(1):20. doi: 10.1186/s13058-022-01516-0
54. Xu L, Hu Y, Liu W. Pyroptosis-Mediated Molecular Subtypes Are Characterized by Distinct Tumor Microenvironment Infiltration Characteristics in Breast Cancer. J Inflammation Res (2022) 15:345–62. doi: 10.2147/JIR.S349186
55. Du Y, Cao M, Liu Y, He Y, Yang C, Zhang G, et al. Tumor Microenvironment Remodeling Modulates Macrophage Phenotype in Breast Cancer Lymphangiogenesis. FASEB J (2022) 36(4):e22248. doi: 10.1096/fj.202101230R
56. Zeng N, Chen X, Liu Z. Natural Products and Nanotechnology Against Coronavirus Disease 2019. Front Chem (2022) 10:819969. doi: 10.3389/fchem.2022.819969
57. Li Y, Liu Z, Zeng W, Wang Z, Liu C, Zeng N, et al. A Novel H2O2 Generator for Tumor Chemotherapy-Enhanced CO Gas Therapy. Front Oncol (2021) 11:738567. doi: 10.3389/fonc.2021.738567
58. Huang C, Liu Z, Chen M, Du L, Liu C, Wang S, et al. Tumor-Derived Biomimetic Nanozyme With Immune Evasion Ability for Synergistically Enhanced Low Dose Radiotherapy. J Nanobiotechnology (2021) 19(1):457. doi: 10.1186/s12951-021-01182-y
59. Hou K, Ning Z, Chen H, Wu Y. Nanomaterial Technology and Triple Negative Breast Cancer. Front Oncol (2021) 11:828810. doi: 10.3389/fonc.2021.828810
60. Zhao T, Wu W, Sui L, Huang Q, Nan Y, Liu J, et al. Reactive Oxygen Species-Based Nanomaterials for the Treatment of Myocardial Ischemia Reperfusion Injuries. Bioact Mater (2022) 7:47–72. doi: 10.1016/j.bioactmat.2021.06.006
61. Choi HW, Lim JH, Kim CW, Lee E, Kim JM, Chang K, et al. Near-Infrared Light-Triggered Generation of Reactive Oxygen Species and Induction of Local Hyperthermia From Indocyanine Green Encapsulated Mesoporous Silica-Coated Graphene Oxide for Colorectal Cancer Therapy. Antioxid (Basel) (2022) 11(1):174. doi: 10.3390/antiox11010174
62. Liu L, Liu F, Liu D, Yuan W, Zhang M, Wei P, et al. A Smart Theranostic Prodrug System Activated by Reactive Oxygen Species for Regional Chemotherapy of Metastatic Cancer. Angew Chem Int Ed Engl (2022) 61(12):e202116807. doi: 10.1002/anie.202116807
63. Casella D, Palumbo P, Sandroni S, Caponi C, Littori F, Capuano F, et al. Positive ROS (Reactive Oxygen Species) Modulator Engineered Device Support Skin Treatment in Locally Advanced Breast Cancer (LABC) Enhancing Patient Quality of Life. J Clin Med (2021) 11(1):126. doi: 10.3390/jcm11010126
64. Wang G, Duan P, Wei Z, Liu F. Curcumin Sensitizes Carboplatin Treatment in Triple Negative Breast Cancer Through Reactive Oxygen Species Induced DNA Repair Pathway. Mol Biol Rep (2022) 49(4):3259–70. doi: 10.1007/s11033-022-07162-1
65. Wang C, Guo J, Wu Z. Combinative Treatment of Curdione and Docetaxel Triggers Reactive Oxygen Species (ROS)-Mediated Intrinsic Apoptosis of Triple-Negative Breast Cancer Cells. Bioengineered (2021) 12(2):10037–48. doi: 10.1080/21655979.2021.1994737
66. Mishra R, Yuan L, Patel H, Karve AS, Zhu H, White A, et al. Phosphoinositide 3-Kinase (PI3K) Reactive Oxygen Species (ROS)-Activated Prodrug in Combination With Anthracycline Impairs PI3K Signaling, Increases DNA Damage Response and Reduces Breast Cancer Cell Growth. Int J Mol Sci (2021) 22(4):2088. doi: 10.3390/ijms22042088
67. Ullah I, Khalil AT, Ali M, Iqbal J, Ali W, Alarifi S, et al. Green-Synthesized Silver Nanoparticles Induced Apoptotic Cell Death in MCF-7 Breast Cancer Cells by Generating Reactive Oxygen Species and Activating Caspase 3 and 9 Enzyme Activities. Oxid Med Cell Longev (2020) 2020:1215395. doi: 10.1155/2020/1215395
68. Aggarwal V, Tuli HS, Varol A, Thakral F, Yerer MB, Sak K, et al. Role of Reactive Oxygen Species in Cancer Progression: Molecular Mechanisms and Recent Advancements. Biomolecules (2019) 9(11):735. doi: 10.3390/biom9110735
69. Li J, Wang Y, Xu C, Yu Q, Wang X, Xie H, et al. Rapid pH-Responsive Self-Disintegrating Nanoassemblies Balance Tumor Accumulation and Penetration for Enhanced Anti-Breast Cancer Therapy. Acta Biomater (2021) 134:546–58. doi: 10.1016/j.actbio.2021.04.022
70. Gong C, Yu X, Zhang W, Han L, Wang R, Wang Y, et al. Regulating the Immunosuppressive Tumor Microenvironment to Enhance Breast Cancer Immunotherapy Using pH-Responsive Hybrid Membrane-Coated Nanoparticles. J Nanobiotechnol (2021) 19(1):58. doi: 10.1186/s12951-021-00805-8
71. Shen Q, Xu L, Li R, Wu G, Li S, Saw PE, et al. A Tumor Microenvironment (TME)-Responsive Nanoplatform for Systemic Saporin Delivery and Effective Breast Cancer Therapy. Chem Commun (Camb) (2021) 57(20):2563–6. doi: 10.1039/d0cc07808e
72. Qiao Y, Wan J, Zhou L, Ma W, Yang Y, Luo W, et al. Stimuli-Responsive Nanotherapeutics for Precision Drug Delivery and Cancer Therapy. Wiley Interdiscip Rev Nanomed Nanobiotechnol (2019) 11(1):e1527. doi: 10.1002/wnan.1527
73. Dong X, Yin W, Zhang X, Zhu S, He X, Yu J, et al. Intelligent MoS2 Nanotheranostic for Targeted and Enzyme-/pH-/NIR-Responsive Drug Delivery To Overcome Cancer Chemotherapy Resistance Guided by PET Imaging. ACS Appl Mater Interfaces (2018) 10(4):4271–84. doi: 10.1021/acsami.7b17506
74. Kashyap S, Singh N, Surnar B, Jayakannan M. Enzyme and Thermal Dual Responsive Amphiphilic Polymer Core-Shell Nanoparticle for Doxorubicin Delivery to Cancer Cells. Biomacromolecules (2016) 17(1):384–98. doi: 10.1021/acs.biomac.5b01545
75. Nosrati H, Mojtahedi A, Danafar H, Kheiri Manjili H. Enzymatic Stimuli-Responsive Methotrexate-Conjugated Magnetic Nanoparticles for Target Delivery to Breast Cancer Cells and Release Study in Lysosomal Condition. J BioMed Mater Res A (2018) 106(6):1646–54. doi: 10.1002/jbm.a.36364
76. Wojtkowiak JW, Verduzco D, Schramm KJ, Gillies RJ. Drug Resistance and Cellular Adaptation to Tumor Acidic pH Microenvironment. Mol Pharm (2011) 8(6):2032–8. doi: 10.1021/mp200292c
77. Mpekris F, Panagi M, Voutouri C, Martin JD, Samuel R, Takahashi S, et al. Normalizing the Microenvironment Overcomes Vessel Compression and Resistance to Nano-Immunotherapy in Breast Cancer Lung Metastasis. Adv Sci (Weinh) (2021) 8(3):2001917. doi: 10.1002/advs.202001917
78. Rao NV, Ko H, Lee J, Park JH. Recent Progress and Advances in Stimuli-Responsive Polymers for Cancer Therapy. Front Bioeng Biotechnol (2018) 6:110. doi: 10.3389/fbioe.2018.00110
79. Ding Y, Su S, Zhang R, Shao L, Zhang Y, Wang B, et al. Precision Combination Therapy for Triple Negative Breast Cancer via Biomimetic Polydopamine Polymer Core-Shell Nanostructures. Biomaterials (2017) 113:243–52. doi: 10.1016/j.biomaterials.2016.10.053
80. Hu Q, Shang L, Wang M, Tu K, Hu M, Yu Y, et al. Co-Delivery of Paclitaxel and Interleukin-12 Regulating Tumor Microenvironment for Cancer Immunochemotherapy. Adv Healthc Mater (2020) 9(10):e1901858. doi: 10.1002/adhm.201901858
81. Niu S, Williams GR, Wu J, Wu J, Zhang X, Chen X, et al. A Chitosan-Based Cascade-Responsive Drug Delivery System for Triple-Negative Breast Cancer Therapy. J Nanobiotechnol (2019) 17(1):95. doi: 10.1186/s12951-019-0529-4
82. Huang J, Huang Q, Liu M, Chen Q, Ai K. Emerging Bismuth Chalcogenides Based Nanodrugs for Cancer Radiotherapy. Front Pharmacol (2022) 13:844037. doi: 10.3389/fphar.2022.844037
83. Qiao A, Gu F, Guo X, Zhang X, Fu L. Breast Cancer-Associated Fibroblasts: Their Roles in Tumor Initiation, Progression and Clinical Applications. Front Med (2016) 10(1):33–40. doi: 10.1007/s11684-016-0431-5
84. Alkasalias T, Moyano-Galceran L, Arsenian-Henriksson M, Lehti K. Fibroblasts in the Tumor Microenvironment: Shield or Spear? Int J Mol Sci (2018) 19(5):1532. doi: 10.3390/ijms19051532
85. Costa A, Kieffer Y, Scholer-Dahirel A, Pelon F, Bourachot B, Cardon M, et al. Fibroblast Heterogeneity and Immunosuppressive Environment in Human Breast Cancer. Cancer Cell (2018) 33(3):463–79.e10. doi: 10.1016/j.ccell.2018.01.011
86. Bochet L, Lehuede C, Dauvillier S, Wang YY, Dirat B, Laurent V, et al. Adipocyte-Derived Fibroblasts Promote Tumor Progression and Contribute to the Desmoplastic Reaction in Breast Cancer. Cancer Res (2013) 73(18):5657–68. doi: 10.1158/0008-5472.CAN-13-0530
87. Ao Z, Shah SH, Machlin LM, Parajuli R, Miller PC, Rawal S, et al. Identification of Cancer-Associated Fibroblasts in Circulating Blood From Patients With Metastatic Breast Cancer. Cancer Res (2015) 75(22):4681–7. doi: 10.1158/0008-5472.CAN-15-1633
88. LeBleu VS, Kalluri R. A Peek Into Cancer-Associated Fibroblasts: Origins, Functions and Translational Impact. Dis Model Mech (2018) 11(4):dmm029447. doi: 10.1242/dmm.029447
Keywords: nanomaterial, nanotechnology, nanobiosensors, drug delivery, tumor microenvironment, smart nanoparticles
Citation: Luo X, Zhang Q, Chen H, Hou K, Zeng N and Wu Y (2022) Smart Nanoparticles for Breast Cancer Treatment Based on the Tumor Microenvironment. Front. Oncol. 12:907684. doi: 10.3389/fonc.2022.907684
Received: 30 March 2022; Accepted: 28 April 2022;
Published: 26 May 2022.
Edited by:
Kelong Ai, Central South University, ChinaReviewed by:
Qiong Huang, Central South University, ChinaCopyright © 2022 Luo, Zhang, Chen, Hou, Zeng and Wu. This is an open-access article distributed under the terms of the Creative Commons Attribution License (CC BY). The use, distribution or reproduction in other forums is permitted, provided the original author(s) and the copyright owner(s) are credited and that the original publication in this journal is cited, in accordance with accepted academic practice. No use, distribution or reproduction is permitted which does not comply with these terms.
*Correspondence: Yiping Wu, d3V5aXBpbmd0akAxNjMuY29t; Ning Zeng, emVuZ25pbmd3aHRqQDE2My5jb20=
†These authors have contributed equally to this work
Disclaimer: All claims expressed in this article are solely those of the authors and do not necessarily represent those of their affiliated organizations, or those of the publisher, the editors and the reviewers. Any product that may be evaluated in this article or claim that may be made by its manufacturer is not guaranteed or endorsed by the publisher.
Research integrity at Frontiers
Learn more about the work of our research integrity team to safeguard the quality of each article we publish.