- 1Biology Science Institutes, Chongqing Medical University, Chongqing, China
- 2Clinical Hematology, Third Military Medical University (Army Medical University), Chongqing, China
- 3School of Medicine, Chongqing University, Chongqing, China
- 4Key Laboratory of Laboratory Medical Diagnostics Designated by the Ministry of Education, School of Laboratory Medicine, Chongqing Medical University, Chongqing, China
Acute myeloid leukemia (AML) is a heterogeneous hematologic malignancy characterized by multiple cytogenetic and molecular abnormalities, with a very poor prognosis. Current treatments for AML often fail to eliminate leukemic stem cells (LSCs), which perpetuate the disease. LSCs exhibit a unique metabolic profile, especially dependent on oxidative phosphorylation (OXPHOS) for energy production. Whereas, normal hematopoietic stem cells (HSCs) and leukemic blasts rely on glycolysis for adenosine triphosphate (ATP) production. Thus, understanding the regulation of OXPHOS in LSCs may offer effective targets for developing clinical therapies in AML. This review summarizes these studies with a focus on the regulation of the electron transport chain (ETC) and tricarboxylic acid (TCA) cycle in OXPHOS and discusses potential therapies for eliminating LSCs.
Introduction
Acute myeloid leukemia (AML) is a common type of acute leukemia with high lethality. Although conventional chemotherapy induces remission in up to 70% of AML patients, the majority of patients will relapse (1, 2). Leukemic stem cells (LSCs), originating from the hematopoietic stem cells (HSCs) and progenitor cells (multipotent progenitor cells, MPPs; common myeloid progenitor cells, CMPs), have self-renewal and infinite proliferative capacities and could block the function and development of mature blood cells and initiate leukemia when transplanted into a new host (3–6). Additionally, LSCs are resilient to the cytotoxic effects of chemotherapy via mechanisms that include resistance to apoptosis, increased capacities to efflux drugs, and relative quiescence (7, 8). Thus, the main treatment strategy for AML is the removal of LSCs to promote hematopoietic recovery and reduce recurrence.
In recent years, plenty of strategies have been developed to eliminate LSCs, such as blocking self-renewal pathways (9, 10), disturbing some signaling pathways (11–13), etc. However, these strategies show limited potential for curing AML and removing LSCs in clinical practice. Researchers are committed to finding new targets for eliminating LSCs. Metabolism reprogramming, especially energy metabolism, is considered a hallmark of cancer. In particular, energy metabolism may be the Achilles’ heel of cancer stem cells (14). To find an effective target for clearing LSCs, an increasing number of researchers are focused on finding the distinctive feature of energy metabolism. Lagadinou et al. revealed that LSCs relied on oxidative phosphorylation (OXPHOS) significantly more than normal bone marrow progenitors (15). Also, Kuntz et al. found that LSCs increased mitochondrial respiration and tricarboxylic acid (TCA) cycle flux compared with their counterparts (16). These studies indicate that LSCs depend on OXPHOS for survival, and understanding the metabolic properties of OXPHOS and further developing effective therapies to eradicate LSCs are required to improve the prognosis in AML patients.
We discussed here the metabolic properties of OXPHOS in LSCs.
LSCs Depend on OXPHOS for Energy Demand
Mammalian cells produce adenosine triphosphate (ATP), the universal energy currency, mainly through glycolysis and OXPHOS. OXPHOS is the preferred method of energy production in mature cells with more metabolic efficiency than glycolysis, as glucose produces 38 ATP molecules via OXPHOS and two ATP molecules via glycolysis (17). Since the twentieth century, researchers have found that cancer cells, including both solid tumors and hematological malignancies (16, 18), preferred to depend on glycolysis for ATP production (19). However, several drug candidates targeting glycolysis in leukemic cells do not have a significant effect on tumor growth as expected. In 2018, Jones et al. performed global metabolic profiling in LSCs and AML blasts from 15 primary AML specimens by mass spectrometry and found that 39 metabolites were significantly increased in LSCs. Among the 39 metabolites, two were TCA cycle intermediates, and five were glutathione homeostasis metabolites, which were all related to OXPHOS (20). In 2020, Raffel et al. performed quantitative proteomics of LSCs and hematopoietic stem and progenitor cells (HSPCs) and found that the predominant utilization of OXPHOS and not glycolysis may be a general feature of LSCs (21). Also, many other studies disclosed that tumors are metabolically heterogeneous and LSCs paradoxically depend more on OXPHOS to meet their energy demands and maintain their survival (15, 22, 23), which may be the reason that drug candidates targeting tumor glycolysis sometimes lead to cancer treatment failure. Moreover, HSCs tend to be glycolytic for survival. Thus, targeting OXPHOS may kill LSCs effectively.
OXPHOS, a fundamental mitochondrial process, is carried out by the electron transport chain (ETC) to the production of ATP. In more detail, the metabolites NADH and FADH2, mainly from the TCA cycle, could fuel the complex in ETC and contribute to the proton-motive force, which drives the ATP synthesis. Thus, OXPHOS is generally considered to link the TCA cycle and ETC (24). As the metabolites from glucose, amino acids, and fatty acids can enter the TCA cycle, OXPHOS generates energy not only via glucose metabolism but also through amino acid and fatty acid metabolism. Many researchers are working on exploring the specific metabolic regulation of LSCs. In 2012, Lagadinou et al. found that the mitochondrial oxidative metabolism was activated in LSCs. Also, they used the mitochondrial respiration inhibitors oligomycin and FCCP to treat LSCs and found that the cells failed to activate the glycolytic pathway to satisfy energy demand even though OXPHOS was inhibited (15). Thus, LSCs are metabolically inflexible and cannot depend on glycolysis for energy demand, which leads to glucose no longer being considered a fuel for OXPHOS. As a result, LSCs use nutrients, amino acids, and/or fatty acids to fuel OXPHOS. This discovery was also confirmed by Jones et al. in 2018 (20).
The metabolic properties of OXPHOS in LSCs vary in different stages of disease pathogenesis. The de novo AML LSCs use amino acid metabolism to fuel OXPHOS. It has been reported that some specific amino acids, such as glutamine, cysteine, and branched-chain amino acids, are important in many hematologic malignancies, but the mechanism remains unknown (25–27). Until 2018, Jones et al. revealed that LSCs isolated from de novo AML patients showed increased amino acid levels and uptake. Moreover, targeting amino acid metabolism decreased TCA cycle flux and induced LSC death (20). Whereas, both fatty acid oxidation (FAO) and amino acid metabolism are important to drive OXPHOS in relapsed LSCs. Stevens et al. reported that relapsed LSCs were resistant to amino acid metabolism inhibition, and the inhibition of both amino acid metabolism and FAO led to a decreased OXPHOS and eliminated LSCs more clearly than one inhibition (23, 28). The difference in metabolism properties between de novo AML LSCs and relapsed AML LSCs may represent potential targets for individualized treatment.
There are many factors influencing OXPHOS in LSCs, and these factors may present potential targets for eliminating LSCs.
Regulation of ETC in LSCs
Composition of ETC
ETC is composed of four major multienzymatic complexes and supported by two mobile cosubstrates (coenzyme Q and cytochrome c) in the inner mitochondrial membrane. As shown in Figure 1, nutrient oxidation can produce NADH and FADH2, the electron donor molecules that are also called high-energy-reducing equivalents, mainly from the TCA cycle. NADH2 provides electrons to the NADH dehydrogenase:ubiquinone oxidoreductase (complex I (CI)), and FADH2 transfers electrons to the succinate:ubiquinone oxidoreductase (complex II (CII)). Coenzyme Q uptakes the electrons from CI and CII and transfers them to the ubiquinol:cytochrome c oxidoreductase or cytochrome bc1 complex (complex III (CIII)). Cytochrome c then uptakes the electrons from CIII and transfers them to cytochrome c oxidase (complex IV), which transfers the electrons to molecular oxygen. Ultimately, ATPs are produced at the ATP synthase with the help of the electrochemical gradient caused by a proton pump from the mitochondrial matrix to the intermembrane space (29–32).
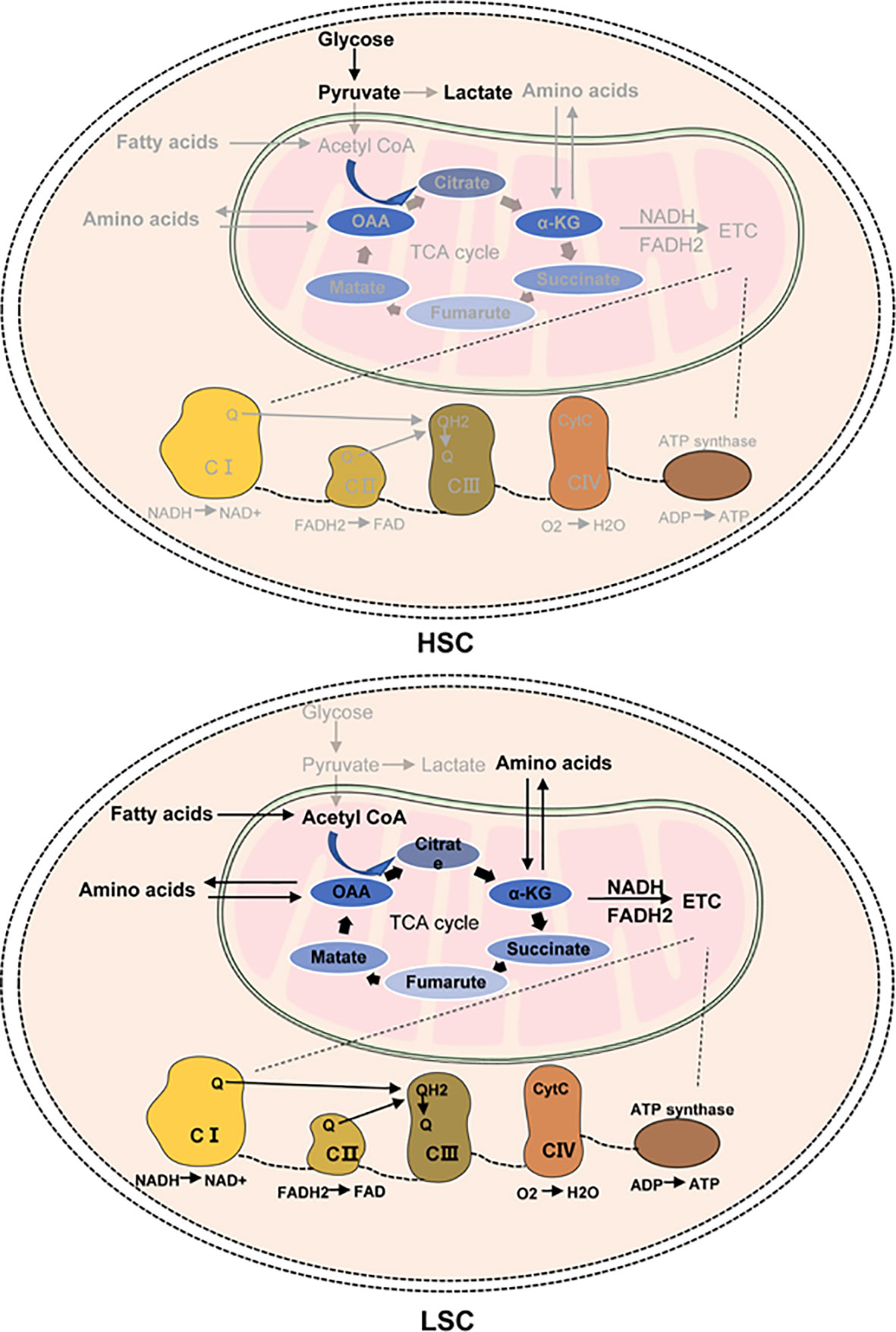
Figure 1 The energy metabolism in HSCs and LSCs. LSCs mainly depend on OXPHOS for energy demand. OXPHOS is a fundamental mitochondrial process, linking the tricarboxylic acid (TCA) cycle to the production of ATP via the electron transport chain (ETC). In LSCs, glucose cannot enter the TCA cycle. Amino acids enter the TCA cycle through conversion to the TCA cycle intermediates (such as oxaloacetate (OAA) and alphaketoglutarate (α-KG)). Fatty acids are converted to acetyl-CoA through β-oxidation in the mitochondria. The increased NAD+ levels from nicotinamide metabolism are necessary for the sufficient function of the TCA cycle enzymes. NADH and FADH2 produced from the TCA cycle generate ATP via ETC. TCA, tricarboxylic acid; ATP, adenosine triphosphate; acetyl-CoA, acetyl coenzyme A; CytC, cytochrome c; ETC, electron transport chain; Q, coenzyme Q/ubiquinone; QH2, ubiquinol; FADH2, flavin adenine dinucleotide; NADH, nicotinamide adenine dinucleotide; CI, complex I; CII, complex II; CIII, complex III; CIV, complex IV; ADP, adenosine diphosphate.
Mitochondrial-Encoded Translation of ETC Proteins in LSCs
There are about 90 proteins in ETC, and 13 of these are encoded by mitochondrial DNA, which is composed of a double-stranded circular genome without introns. Mitochondrial DNA-encoded proteins are translated into mitochondrial ribosomes using a unique protein translation machinery, differing from nucleus DNA-encoded proteins, which are translated into cytosolic ribosomes. Although most ETC-related proteins are obtained from cytoplasmic translation, most of these proteins are not located in the key sites of ETC. Also, cytoplasmic translation has a wide range of functions. Thus, it is not a good choice to intervene in ETC via targeting cytoplasmic translation (33). Inversely, most of the 13 mitochondrial-encoded proteins are functional components of 4/5 ETC complexes, and targeting mitochondrial-encoded translation can inhibit the activity of ETC effectively (34). In 2011, Skrtic et al. found that antimicrobial tigecycline, an inhibitor of mitochondrial protein translation, selectively killed LSCs rather than their normal counterparts via inhibiting OXPHOS (33). Importantly, antimicrobial tigecycline is an FDA-approved agent. While Jhas et al. reported that the metabolic shift with decreased OXPHOS resulting from antimicrobial tigecycline was reversible upon removal, the long-term use of antimicrobial tigecycline treatment may cause clinical resistance (35). In 2017, Liyanage et al. revealed that inhibiting the replication of mitochondrial DNA by ddC (a selective inhibitor of the mitochondrial DNA polymerase) repressed ETC-related proteins and induced cell death in both subsets of primary leukemic cells and LSCs (36). Also, there was no toxicity of ddC for normal cells in xenotransplantation assays. However, it remains unknown whether ddC could be effective in patients. Thus, mitochondrial-translated proteins may be a potential target for LSCs, while the effective treatment strategy targeting them to eliminate LSCs needs further study.
Posttranslational Modifications of ETC Proteins in LSCs
Glutathionylation, one of the posttranslational modifications, has a critical role in sustaining the activity of OXPHOS (37, 38). For example, glutathionylation of hMIA40, an intermembrane space import receptor of mitochondria, maintains the optimum function of CIII and CIV in ETC (39). Also, Jones et al. revealed that glutathionylation of succinate dehydrogenase complex flavoprotein subunit A (SDHA), a key component of ETC CII, enhanced OXPHOS in LSCs (40). Cysteine is the precursor of glutathione in LSCs. Inhibition of SDHA glutathionylation via cysteine depletion or using cysteine-degrading enzyme impairs ETC CII activity and OXPHOS and leads to LSC death with no detectable effect on HSCs (40). However, the glutathionylation of ETC proteins is reversible and can be removed by potent reducing agents (such as β-mercaptoethanol) as detected in HEK293T cells, indicating that long-term clinical use of some of these potent reducing agents may cause resistance. Thus, to explore more effective targets, more studies should focus on the mechanism of glutathionylation in ETC-related proteins. In 2020, van Gastel et al. found that residual mouse AML cells in the bone marrow required glutamine to fuel glutathione synthesis and generate pyrimidine nucleotides, but not the TCA cycle (41), and whether enhanced glutathione synthesis promotes OXPHOS via ETC-related protein glutathionylation remains unknown.
Other posttranslational modifications of ETC exist, such as phosphorylation of the complex I subunits (42, 43), methylation of the NDUFB3 subunit in complex I (44), acetylation of the complex II subunits (45), etc. However, whether these posttranslational modifications of ETC exist in LSCs remains unknown.
Mitochondrial Protease Activity Regulating the ETC Complex in LSCs
Mitochondrial proteases, including four core ATP-dependent proteases (m-AAA, i-AAA, LonP, and ClpXP), regulate protein homeostasis in the mitochondrial membrane and matrix. m-AAA is encoded by AFG3L2, and AFG3L2 mutation or knockdown leads to unfolded mitochondrial protein accumulation and complex IV deficiency, resulting in respiratory deficiency mainly in neurological diseases (46, 47). i-AAA is encoded by YME1L, and the knockdown of YME1L by short hairpin RNA leads to excessive accumulation of non-assembled respiratory chain subunits and impairs cell proliferation in human embryonic kidney 293 cells (48), whereas the function of i-AAA is not found in other cells. As for LonP, there is no related research about its function. ClpXP, containing serine protease ClpP and the AAA+ATPase C1pX (49), is the most important protease to maintain protein quality in mitochondria via degrading damaged or misfolded substrates involved in ETC and the TCA cycle (50–53). Moreover, ClpP is highly expressed in LSCs and is tight with ETC activity and cell function. The knockdown of ClpP reduces the growth and viability of AML progenitors via degrading SDHA and impairing complex II activity (50). Moreover, A2-32-01, a ClpP inhibitor, decreases the number of leukemic cells in primary and secondary engraftment in mice (33). Ishizawa et al. revealed that ONC201 and ONC212, activators of ClpP, also decreased OXPHOS in AML through uncontrolled degradation of respiratory chain proteins in complexes I, II, and IV (54). Moreover, ONC201 is being studied in phase I clinical trials, and ONC212 has shown promising preclinical results in AML (55–57). Thus, these studies show that not only inhibition but also hyperactivation of ClpP impair the ETC activity and induce cell death, and whether inhibiting or activating ClpP is the preferred therapeutic strategy of eradicating LSCs remains unknown.
Additionally, neurolysin (NLN) is a zinc metallopeptidase with secretion into the circulation or location in the mitochondria. The function of NLN in the circulation has been well studied, while the mitochondrial function is unclear. Until 2020, Mirali et al. found that NLN was necessary for the formation of respiratory complexes I, III, and IV in AML cells (58). 3-[(2S)-1-[(3R)-3-(2-Chlorophenyl)-2-(2-fluorophenyl)pyrazolidin-1-yl]-1-oxopropan-2-yl]-1-(adamantan-2-yl)urea (R2), an inhibitor of NLN, reduces the viability of LSCs in vitro and in vivo, but does not influence normal hematopoietic cells. Whether NLN inhibitor could be used in the clinic and if there are other effective targets relating to mitochondrial proteases in the removal of LSCs, need further study.
Other Factors Regulating ETC
FAO supplies FADH2 to ETC. Cytosolic fatty acids could convert into fatty acyl-CoA esters by acyl-CoA synthases, and the fatty acyl-CoA is transported to the mitochondria to perform FAO, thus resulting in FADH2 production. This metabolism signaling indicates that FAO could fuel ETC directly, not through the TCA cycle, but whether this metabolism pathway exists in LSCs remains unknown.
Also, there are some other strategies targeting ETC in LSCs to treat AML: IACS-010759 (59) and mubritinib (60) have been used to inhibit complex I and ME-344 to inhibit complexes I and III (61). Though the mechanisms remain unclear, IACS-010759, mubritinib, and ME-344 have achieved a good result in clearing LSCs in vitro or in vivo. Notably, these treatments do not influence the survival of normal stem and progenitor cells, suggesting that these therapies may be more selective for LSCs. Furthermore, IACS-010759 is currently in phase I clinical trials in R/R AML (62). A ribonucleoside analog, 8-chloro-adenosine (8-Cl-Ado), can remove LSCs while sparing HSC via targeting the ATP synthase. Also, 8-Cl-Ado has been used in phase I clinical trials and has yielded encouraging results in AML patients (23).
Regulation of the TCA Cycle in LSCs
Glycolysis as Fuels to the TCA Cycle
Not only can glucose be oxidized to produce ATP and regenerate NADH, it can also enter the TCA cycle through glycolysis. In 2019, Jones et al. revealed that the AML blast cells were dependent on glycolysis to produce energy and upon glucose deprivation to decrease viability in culture. Glucose depletion did not affect the viability or colony-forming potential of LSCs, which indicated that LSCs are not dependent on glucose to fuel the TCA cycle and survival (20). In the same year, Chu et al. reported that six1 played a role in LSC maintenance in AML, and six1-KD led to a significant decrease in the glycolytic gene expression and pyruvate and lactate production, but did not affect the ATP level (63). This research indicated that glycolysis was important for LSC maintenance but not through energy suppling. In 2020, Pulikkottil and Bamezai revealed that quiescent LSCs preferentially depended on OXPHOS, while non-quiescent, cycling AML LSCs may likely depend on glucose metabolism (64). Also, Zhao et al. reported that the hypoxic endosteal niche led to LSCs undergoing a metabolic change (abandoning OXPHOS and switching to glycolysis) in chronic myeloid leukemia (65). Whether the metabolic change exists in LSCs of AML is unclear. Thus, further study of glycolysis in AML LSCs is needed.
Fructose is the most abundant blood sugar in humans apart from glucose. In 2016, Chen et al. found that AML cells were prone to fructose utilization to fuel glycolytic flux and result in increased proliferation and colony growth when glucose was deficient (66). As colony formation is linked to cancer stem cell properties (67), the increased fructose utilization may contribute to maintaining LSCs. While further study about fructose metabolism in LSCs was needed.
PPP as Fuels to the TCA Cycle
Glucose is involved in the pentose phosphate pathway (PPP) to generate pentose phosphate for ribonucleotide synthesis, serine biosynthesis, and one-carbon metabolism (68). The metabolites of PPP can also enter glycolysis via glyceraldehyde 3-phosphate and fructose 6-phosphate. As reported, PPP had a critical role in leukemic cell survival, and the suppression of PPP enzymes by shRNA-mediated knockdown or inhibitor selectively decreased leukemogenesis in vitro (69, 70). In particular, Mizuno et al. found that EVI1 can both upregulate the PPP pathway and OXPHOS in AML (70). Also, Pei et al. reported that PPP was a cytoprotective mechanism of AML cells in response to the treatment of parthenolide (71). Thus, whether the PPP pathway exists in LSCs and is related to glycolysis or the TCA cycle need further study.
Amino Acid Metabolism as Fuels to the TCA Cycle in LSCs
Glutamate is the key metabolite in the process of amino acid metabolism fueling the TCA cycle. It was reported that arginine, glutamine, histidine, branched-chain amino acids, and proline were precursors to glutamate. Glutamate could enter the TCA cycle by converting to α-ketoglutarate with the help of glutamate dehydrogenase and drive OXPHOS through the production of NADH and succinate (the substrate for ETC complex II) (72). Jones et al. revealed that deprivation of amino acids could decrease fumarate, malate, citrate, succinate, and α-ketoglutarate in the TCA cycle and induce the death of de novo AML LSCs, but not HSCs, indicating that de novo AML LSCs use amino acid metabolism to fuel the TCA cycle and OXPHOS (20). Moreover, the pharmacological inhibition of amino acid metabolism with a combination of venetoclax and azacytidine (ven/aza) reduces OXPHOS and eliminates LSCs by measuring the engraftment ability of primary AML specimens after 24 h of ex vivo treatment (20). Notably, in 2018, FDA approved venetoclax as an AML treatment agent in combination with other hypomethylating agents. Thus, amino acid metabolism may be a potential target to treat AML patients in the clinic.
FAO as Fuels to the TCA Cycle in LSCs
FAO, a process of breaking down fatty acids into acetyl-CoA, fuels the TCA cycle. Within the mitochondria, fatty acyl-CoA enters the FAO spiral resulting in the generation of acetyl-CoA that contributes to the overall mitochondrial pool due to acetyl-CoA being used in the TCA cycle (Figure 1). The relapsed AML patients have inferior outcomes compared to the de novo AML patients after receiving ven/aza treatment, which may be due to FAO also fueling the TCA cycle (73). Shafat et al. revealed that the AML blasts could alter the metabolic processes of adipocytes to activate lipolysis, and then the fatty acids were transferred from adipocytes to the AML blasts and sustained AML proliferation in the bone marrow microenvironment (74). Also, leukemic cells could create an inflammatory microenvironment in adipose tissue, triggering lipolysis. More importantly, the released fatty acids fuel the chemoresistance of LSCs by inducing the fatty acid transporter CD36 expression (75). Interestingly, the inhibition of fatty acid synthesis has also been shown to potentiate the effects of the BCL-2/BCL-XL dual inhibitor, ABT-737, in AML (76). Inhibiting carnitine palmitoyltransferase 1a (CPT1a), the rate-limiting enzyme of the essential step of FAO, enhances the antileukemic activity of Bcl-2 inhibitor in AML (77, 78). Also, in relapsed LSCs, sorbitan sesquioleate, a CD36 inhibitor to inhibit fatty acid uptake, in combination with ven/aza, results in a significant decrease in OXPHOS (20, 28). Thus, the inhibition of FAO and its role in fueling OXPHOS will likely become an exciting new direction in fighting therapeutic resistance in AML.
Other Metabolism Pathways as Fuels to the TCA Cycle in LSCs
In addition to amino acid metabolism and FAO fueling the TCA cycle and OXPHOS, other metabolisms exist. For example, Anderson et al. revealed that dihydrolipoamide S-succinyltransferase (DLST), a TCA cycle-related transferase, was important for the TCA cycle flux and MYC-mediated leukemogenesis of T-cell lymphoblastic leukemia (79). Anderson et al. also found that heme export was closely related to the TCA cycle and OXPHOS (80). Whether these regulations exist in the LSCs remains unknown. In 2020, Jones et al. revealed that elevated NAD+ from nicotinamide drives OXPHOS by regulating NAD+-dependent enzymes (isocitrate dehydrogenase, 2-oxoglutarate dehydrogenase, and malate dehydrogenase) in the TCA cycle in relapsed LSCs (81). Notably, the inhibition of nicotinamide phosphoribosyltransferase (NAMPT), the rate-limiting enzyme of nicotinamide, converted to NAD+ in mammals, decreased the TCA cycle flux, and enhanced ven/aza sensibility. Thus, the finding provides a means for LSCs to circumvent the cytotoxic effects of ven/aza therapy and suggests that inhibition of NAMPT may be an effective strategy to target LSCs in relapsed AML patients.
Resistance to OXPHOS Inhibition
Based on the important role of OXPHOS in LSCs, many therapies targeting OXPHOS have been used to eliminate LSCs. However, none of them can erase LSCs. In 2021, Saito et al. found that OXPHOS inhibition triggered a mitochondrial transfer from the mesenchymal stem cells (MSCs) to AML cells via tunneling nanotubes, which induced mitochondrial fission and increased functional mitochondria in AML cells, leading to secondary resistance to OXPHOS inhibition (82). Thus, interference with these processes is required to boost the antitumor potency of OXPHOS inhibition. In 2022, You et al. reported that AML cells took up functional mitochondria from MSCs to sustain the resistance to cytarabine (83). Thus, targeting mitochondrial transfer in a leukemic microenvironment may be an effective strategy for curing AML, and an effective target needs further study.
Also, some noncanonical energy fuels exist in leukemic cells. Creatine, as a noncanonical energy fuel, can satisfy the cellular energy requirement in an acute manner coupled with the ATP–ADP transition when a nutrient deficiency occurs. Creatine-dependent metabolism was found important for the survival of EVI1-positive AML and FLT3-ITD mutant AML (84, 85). Whether creatine metabolism contributes to LSC survival is unclear and needs further exploration.
Conclusions
The crucial role of LSCs in the progression and relapse of AML has resulted to investigations into the LSC unique metabolic profile, which may help develop novel and effective treatment strategies. OXPHOS may be a potential target for AML treatment. In this review, we discussed OXPHOS in LSCs, focusing especially on the regulation of the TCA cycle and ETC (Figure 1) and the intervention strategies targeting OXPHOS (Table 1). However, several questions and perspectives remain to be considered in this field. The dysregulated metabolism and the metabolic changes after OXPHOS inhibition in LSCs should be further characterized. In addition, toxicities from OXPHOS-targeted therapies should be evaluated as metabolism is a ubiquitous process.
Data Availability Statement
The original contributions presented in the study are included in the article/supplementary material. Further inquiries can be directed to the corresponding author.
Author Contributions
MP reviewed the literature and wrote the manuscript. YoH reviewed the literature. YuH, XZ, and LZ designed the review and revised the manuscript. All authors listed have made a substantial, direct, and intellectual contribution to the work and approved it for publication
Funding
This work was supported by grants from the National Natural Science Foundation of China (Grant No. 82170115 and No. 1900588).
Conflict of Interest
The authors declare that the research was conducted in the absence of any commercial or financial relationships that could be construed as a potential conflict of interest.
Publisher’s Note
All claims expressed in this article are solely those of the authors and do not necessarily represent those of their affiliated organizations, or those of the publisher, the editors and the reviewers. Any product that may be evaluated in this article, or claim that may be made by its manufacturer, is not guaranteed or endorsed by the publisher.
References
1. Dombret H, Gardin C. An Update of Current Treatments for Adult Acute Myeloid Leukemia. Blood (2016) 127(1):53–61. doi: 10.1182/blood-2015-08-604520
2. Shlush L, Mitchell A, Heisler L, Abelson S, Ng S, Trotman-Grant A, et al. Tracing the Origins of Relapse in Acute Myeloid Leukaemia to Stem Cells. Nature (2017) 547(7661):104–8. doi: 10.1038/nature22993
3. Bonnet D, Dick J. Human Acute Myeloid Leukemia is Organized as a Hierarchy That Originates From a Primitive Hematopoietic Cell. Nat Med (1997) 3(7):730–7. doi: 10.1038/nm0797-730
4. Hope KJ, Jin L, Dick JE. Human Acute Myeloid Leukemia Stem Cells. Arch Med Res (2003) 34(6):507–14. doi: 10.1016/j.arcmed.2003.08.007
5. Passegué E, Jamieson CH, Ailles LE, Weissman IL. Normal and Leukemic Hematopoiesis: Are Leukemias a Stem Cell Disorder or a Reacquisition of Stem Cell Characteristics? Proc Natl Acad Sci USA (2003) 100(Suppl 1):11842–9. doi: 10.1073/pnas.2034201100
6. Tenen DG. Disruption of Differentiation in Human Cancer: AML Shows the Way. Nat Rev Cancer (2003) 3(2):89–101. doi: 10.1038/nrc989
7. O'Reilly E, Zeinabad H, Szegezdi E. Hematopoietic Versus Leukemic Stem Cell Quiescence: Challenges and Therapeutic Opportunities. Blood Rev (2021) 50:100850. doi: 10.1016/j.blre.2021.100850
8. Thomas D, Majeti R. Biology and Relevance of Human Acute Myeloid Leukemia Stem Cells. Blood (2017) 129(12):1577–85. doi: 10.1182/blood-2016-10-696054
9. Wojiski S, Guibal F, Kindler T, Lee B, Jesneck J, Fabian A, et al. PML-RARalpha Initiates Leukemia by Conferring Properties of Self-Renewal to Committed Promyelocytic Progenitors. Leukemia (2009) 23(8):1462–71. doi: 10.1038/leu.2009.63
10. Han Y, Park C, Bhagat G, Zhang J, Wang Y, Fan J, et al. microRNA-29a Induces Aberrant Self-Renewal Capacity in Hematopoietic Progenitors, Biased Myeloid Development, and Acute Myeloid Leukemia. J Exp Med (2010) 207(3):475–89. doi: 10.1084/jem.20090831
11. Zhou J, Bi C, Cheong L, Mahara S, Liu S, Tay K, et al. The Histone Methyltransferase Inhibitor, DZNep, Up-Regulates TXNIP, Increases ROS Production, and Targets Leukemia Cells in AML. Blood (2011) 118(10):2830–9. doi: 10.1182/blood-2010-07-294827
12. Hosen N, Park C, Tatsumi N, Oji Y, Sugiyama H, Gramatzki M, et al. CD96 is a Leukemic Stem Cell-Specific Marker in Human Acute Myeloid Leukemia. Proc Natl Acad Sci United States America (2007) 104(26):11008–13. doi: 10.1073/pnas.0704271104
13. Majeti R, Chao M, Alizadeh A, Pang W, Jaiswal S, Gibbs K, et al. CD47 is an Adverse Prognostic Factor and Therapeutic Antibody Target on Human Acute Myeloid Leukemia Stem Cells. Cell (2009) 138(2):286–99. doi: 10.1016/j.cell.2009.05.045
14. Hanahan D, Weinberg RA. Hallmarks of Cancer: The Next Generation. Cell (2011) 144(5):646–74. doi: 10.1016/j.cell.2011.02.013
15. Lagadinou ED, Sach A, Callahan K, Rossi RM, Neering SJ, Minhajuddin M, et al. BCL-2 Inhibition Targets Oxidative Phosphorylation and Selectively Eradicates Quiescent Human Leukemia Stem Cells. Cell Stem Cell (2013) 12(3):329–41. doi: 10.1016/j.stem.2012.12.013
16. Kuntz EM, Baquero P, Michie AM, Dunn K, Tardito S, Holyoake TL, et al. Targeting Mitochondrial Oxidative Phosphorylation Eradicates Therapy-Resistant Chronic Myeloid Leukemia Stem Cells. Nat Med (2017) 23(10):1234–40. doi: 10.1038/nm.4399
17. Zheng J. Energy Metabolism of Cancer: Glycolysis Versus Oxidative Phosphorylation (Review). Oncol Lett (2012) 4(6):1151–7. doi: 10.3892/ol.2012.928
18. Lapidot T, Sirard C, Vormoor J, Murdoch B, Hoang T, Caceres-Cortes J, et al. A Cell Initiating Human Acute Myeloid Leukaemia After Transplantation Into SCID Mice. Nature (1994) 367(6464):645–8. doi: 10.1038/367645a0
19. Warburg O. On the Origin of Cancer Cells. Sci (New York NY) (1956) 123(3191):309–14. doi: 10.1126/science.123.3191.309
20. Jones C, Stevens B, D'Alessandro A, Reisz J, Culp-Hill R, Nemkov T, et al. Inhibition of Amino Acid Metabolism Selectively Targets Human Leukemia Stem Cells. Cancer Cell (2019) 35(2):333–5. doi: 10.1016/j.ccell.2019.01.013
21. Raffel S, Klimmeck D, Falcone M, Demir A, Pouya A, Zeisberger P, et al. Quantitative Proteomics Reveals Specific Metabolic Features of Acute Myeloid Leukemia Stem Cells. Blood (2020) 136(13):1507–19. doi: 10.1182/blood.2019003654
22. Polak A, Bialopiotrowicz E, Krzymieniewska B, Wozniak J, Stojak M, Cybulska M, et al. SYK Inhibition Targets Acute Myeloid Leukemia Stem Cells by Blocking Their Oxidative Metabolism. Cell Death Dis (2020) 11(11):956. doi: 10.1038/s41419-020-03156-8
23. Buettner R, Nguyen LXT, Morales C, Chen MH, Wu X, Chen LS, et al. Targeting the Metabolic Vulnerability of Acute Myeloid Leukemia Blasts With a Combination of Venetoclax and 8-Chloro-Adenosine. J Hematol Oncol (2021) 14(1):70. doi: 10.1186/s13045-021-01076-4
24. Tang JX, Thompson K, Taylor RW, Oláhová M. Mitochondrial OXPHOS Biogenesis: Co-Regulation of Protein Synthesis, Import, and Assembly Pathways. Int J Mol Sci (2020) 21(11):3820. doi: 10.3390/ijms21113820
25. Gregory MA, D'Alessandro A, Alvarez-Calderon F, Kim J, Nemkov T, Adane B, et al. ATM/G6PD-Driven Redox Metabolism Promotes FLT3 Inhibitor Resistance in Acute Myeloid Leukemia. Proc Natl Acad Sci United States America (2016) 113(43):E6669–78. doi: 10.1073/pnas.1603876113
26. Cramer SL, Saha A, Liu J, Tadi S, Tiziani S, Yan W, et al. Systemic Depletion of L-Cyst(E)Ine With Cyst(E)Inase Increases Reactive Oxygen Species and Suppresses Tumor Growth. Nat Med (2017) 23(1):120–7. doi: 10.1038/nm.4232
27. Raffel S, Falcone M, Kneisel N, Hansson J, Wang W, Lutz C, et al. BCAT1 Restricts αkg Levels in AML Stem Cells Leading to IDHmut-Like DNA Hypermethylation. Nature (2017) 551(7680):384–8. doi: 10.1038/nature24294
28. Stevens BM, Jones CL, Pollyea DA, Culp-Hill R, D'Alessandro A, Winters A, et al. Fatty Acid Metabolism Underlies Venetoclax Resistance in Acute Myeloid Leukemia Stem Cells. Nat Cancer (2020) 1(12):1176–87. doi: 10.1038/s43018-020-00126-z
29. Chance B, Williams GR. A Method for the Localization of Sites for Oxidative Phosphorylation. Nature (1955) 176(4475):250–4. doi: 10.1038/176250a0
30. Hatefi Y, Haavik AG, Fowler LR, Griffiths DE. Studies on the Electron Transfer System. XLII. Reconstitution of the Electron Transfer System. J Biol Chem (1962) 237:2661–9. doi: 10.1016/S0021-9258(19)73804-6
31. Kröger A, Klingenberg M. The Kinetics of the Redox Reactions of Ubiquinone Related to the Electron-Transport Activity in the Respiratory Chain. Eur J Biochem (1973) 34(2):358–68. doi: 10.1111/j.1432-1033.1973.tb02767.x
32. Margoliash E, Ferguson-Miller S, Tulloss J, Kang CH, Feinberg BA, Brautigan DL, et al. Separate Intramolecular Pathways for Reduction and Oxidation of Cytochrome C in Electron Transport Chain Reactions. Proc Natl Acad Sci USA (1973) 70(11):3245–9. doi: 10.1073/pnas.70.11.3245
33. Skrtić M, Sriskanthadevan S, Jhas B, Gebbia M, Wang X, Wang Z, et al. Inhibition of Mitochondrial Translation as a Therapeutic Strategy for Human Acute Myeloid Leukemia. Cancer Cell (2011) 20(5):674–88. doi: 10.1016/j.ccr.2011.10.015
34. Fukuda R, Zhang H, Kim JW, Shimoda L, Dang CV, Semenza GL. HIF-1 Regulates Cytochrome Oxidase Subunits to Optimize Efficiency of Respiration in Hypoxic Cells. Cell (2007) 129(1):111–22. doi: 10.1016/j.cell.2007.01.047
35. Jhas B, Sriskanthadevan S, Skrtic M, Sukhai MA, Voisin V, Jitkova Y, et al. Metabolic Adaptation to Chronic Inhibition of Mitochondrial Protein Synthesis in Acute Myeloid Leukemia Cells. PloS One (2013) 8(3):e58367. doi: 10.1371/journal.pone.0058367
36. Liyanage SU, Hurren R, Voisin V, Bridon G, Wang X, Xu C, et al. Leveraging Increased Cytoplasmic Nucleoside Kinase Activity to Target mtDNA and Oxidative Phosphorylation in AML. Blood (2017) 129(19):2657–66. doi: 10.1182/blood-2016-10-741207
37. O'Brien M, Chalker J, Slade L, Gardiner D, Mailloux RJ. Protein S-Glutathionylation Alters Superoxide/Hydrogen Peroxide Emission From Pyruvate Dehydrogenase Complex. Free Radical Biol Med (2017) 106:302–14. doi: 10.1016/j.freeradbiomed.2017.02.046
38. Cortés-Rojo C, Vargas-Vargas MA, Olmos-Orizaba BE, Rodríguez-Orozco AR, Calderón-Cortés E. Interplay Between NADH Oxidation by Complex I, Glutathione Redox State and Sirtuin-3, and its Role in the Development of Insulin Resistance. Biochim Biophys Acta Mol Basis Dis (2020) 1866(8):165801. doi: 10.1016/j.bbadis.2020.165801
39. Thiriveedi VR, Mattam U, Pattabhi P, Bisoyi V, Talari NK, Krishnamoorthy T, et al. Glutathionylated and Fe-S Cluster Containing Hmia40 (CHCHD4) Regulates ROS and Mitochondrial Complex III and IV Activities of the Electron Transport Chain. Redox Biol (2020) 37:101725. doi: 10.1016/j.redox.2020.101725
40. Jones CL, Stevens BM, D'Alessandro A. Cysteine Depletion Targets Leukemia Stem Cells Through Inhibition of Electron Transport Complex II. Blood (2019) 134(4):389–94. doi: 10.1182/blood.2019898114
41. van Gastel N, Spinelli JB, Sharda A, Schajnovitz A, Baryawno N, Rhee C, et al. Induction of a Timed Metabolic Collapse to Overcome Cancer Chemoresistance. Cell Metab (2020) 32(3):391–403.e6. doi: 10.1016/j.cmet.2020.07.009
42. Wang Z, Fan M, Candas D, Zhang TQ, Qin L, Eldridge A, et al. Cyclin B1/Cdk1 Coordinates Mitochondrial Respiration for Cell-Cycle G2/M Progression. Dev Cell (2014) 29(2):217–32. doi: 10.1016/j.devcel.2014.03.012
43. Liu J, Peng Y, Wei W. Cell Cycle on the Crossroad of Tumorigenesis and Cancer Therapy. Trends Cell Biol (2022) 32(1):30–44. doi: 10.1016/j.tcb.2021.07.001
44. Davydova E, Shimazu T, Schuhmacher MK. The Methyltransferase METTL9 Mediates Pervasive 1-Methylhistidine Modification in Mammalian Proteomes. Nat Commun (2021) 12(1):891. doi: 10.1038/s41467-020-20670-7
45. Finley LW, Haas W, Desquiret-Dumas V, Wallace DC, Procaccio V, Gygi SP, et al. Succinate Dehydrogenase Is a Direct Target of Sirtuin 3 Deacetylase Activity. PloS One (2011) 6(8):e23295. doi: 10.1371/journal.pone.0023295
46. Di Bella D, Lazzaro F, Brusco A, Plumari M, Battaglia G, Pastore A, et al. Mutations in the Mitochondrial Protease Gene AFG3L2 Cause Dominant Hereditary Ataxia SCA28. Nat Genet (2010) 42(4):313–21. doi: 10.1038/ng.544
47. Pareek G, Pallanck LJ. Inactivation of the Mitochondrial Protease Afg3l2 Results in Severely Diminished Respiratory Chain Activity and Widespread Defects in Mitochondrial Gene Expression. PloS Genet (2020) 16(10):e1009118. doi: 10.1371/journal.pgen.1009118
48. Stiburek L, Cesnekova J, Kostkova O, Fornuskova D, Vinsova K, Wenchich L, et al. YME1L Controls the Accumulation of Respiratory Chain Subunits and is Required for Apoptotic Resistance, Cristae Morphogenesis, and Cell Proliferation. Mol Biol Cell (2012) 23(6):1010–23. doi: 10.1091/mbc.e11-08-0674
49. Deshwal S, Fiedler KU, Langer T. Mitochondrial Proteases: Multifaceted Regulators of Mitochondrial Plasticity. Annu Rev Biochem (2020) 89:501–28. doi: 10.1146/annurev-biochem-062917-012739
50. Cole A, Wang Z, Coyaud E, Voisin V, Gronda M, Jitkova Y, et al. Inhibition of the Mitochondrial Protease ClpP as a Therapeutic Strategy for Human Acute Myeloid Leukemia. Cancer Cell (2015) 27(6):864–76. doi: 10.1016/j.ccell.2015.05.004
51. Seo JH, Rivadeneira DB, Caino MC, Chae YC, Speicher DW, Tang HY. The Mitochondrial Unfoldase-Peptidase Complex ClpXP Controls Bioenergetics Stress and Metastasis. PLoS Biol (2016) 14(7):. doi: 10.1371/journal.pbio.1002507
52. Fischer F, Langer JD, Osiewacz HD. Identification of Potential Mitochondrial CLPXP Protease Interactors and Substrates Suggests its Central Role in Energy Metabolism. Sci Rep (2015) 5:18375. doi: 10.1038/srep18375
53. Fischer F, Hamann A, Osiewacz HD. Mitochondrial Quality Control: An Integrated Network of Pathways. Trends Biochem Sci (2012) 37(7):284–92. doi: 10.1016/j.tibs.2012.02.004
54. Ishizawa J, Zarabi SF, Davis RE, Halgas O, Nii T, Jitkova Y, et al. Mitochondrial ClpP-Mediated Proteolysis Induces Selective Cancer Cell Lethality. Cancer Cell (2019) 35(5):721–37.e9. doi: 10.1016/j.ccell.2019.03.014
55. Kline CL, Van den Heuvel AP, Allen JE, Prabhu VV, Dicker DT, El-Deiry WS. ONC201 Kills Solid Tumor Cells by Triggering an Integrated Stress Response Dependent on ATF4 Activation by Specific Eif2α Kinases. Sci Signaling (2016) 9(415):ra18. doi: 10.1126/scisignal.aac4374
56. Stein MN, Bertino JR, Kaufman HL, Mayer T, Moss R, Silk A, et al. First-In-Human Clinical Trial of Oral ONC201 in Patients With Refractory Solid Tumors. Clin Cancer Res (2017) 23(15):4163–9. doi: 10.1158/1078-0432.CCR-16-2658
57. Nii T, Prabhu VV, Ruvolo V, Madhukar N, Zhao R, Mu H, et al. Imipridone ONC212 Activates Orphan G Protein-Coupled Receptor GPR132 and Integrated Stress Response in Acute Myeloid Leukemia. Leukemia (2019) 33(12):2805–16. doi: 10.1038/s41375-019-0491-z
58. Mirali S, Botham A, Voisin V, Xu C, St-Germain J. The Mitochondrial Peptidase, Neurolysin, Regulates Respiratory Chain Supercomplex Formation and is Necessary for AML Viability. Sci Transl Med (2020) 12(538):eaaz8264. doi: 10.1126/scitranslmed.aaz8264
59. Molina JR, Sun Y, Protopopova M, Gera S, Bandi M, Bristow C, et al. An Inhibitor of Oxidative Phosphorylation Exploits Cancer Vulnerability. Nat Med (2018) 24(7):1036–46. doi: 10.1038/s41591-018-0052-4
60. Baccelli I, Gareau Y, Lehnertz B, Gingras S, Spinella JF, Corneau S, et al. Mubritinib Targets the Electron Transport Chain Complex I and Reveals the Landscape of OXPHOS Dependency in Acute Myeloid Leukemia. Cancer Cell (2019) 36(1):84–99.e8. doi: 10.1016/j.ccell.2019.06.003
61. Jeyaraju DV, Hurren R, Wang X, MacLean N, Gronda M, Shamas-Din A, et al. A Novel Isoflavone, ME-344, Targets the Cytoskeleton in Acute Myeloid Leukemia. Oncotarget (2016) 7(31):49777–85. doi: 10.18632/oncotarget.10446
62. Carter JL, Hege K, Kalpage HA, Edwards H, Hüttemann M, Taub JW, et al. Targeting Mitochondrial Respiration for the Treatment of Acute Myeloid Leukemia. Biochem Pharmacol (2020) 182:114253. doi: 10.1016/j.bcp.2020.114253
63. Chu Y, Chen Y, Li M, Shi D, Wang B, Lian Y, et al. Six1 Regulates Leukemia Stem Cell Maintenance in Acute Myeloid Leukemia. Cancer Sci (2019) 110(7):2200–10. doi: 10.1111/cas.14033
64. Pulikkottil AJ, Bamezai S. TET3 Promotes AML Growth and Epigenetically Regulates Glucose Metabolism and Leukemic Stem Cell Associated Pathways. Leukemia (2022) 36(2):416–25. doi: 10.1038/s41375-021-01390-3
65. Zhao F, Mancuso A, Bui TV, Tong X, Gruber JJ, Swider CR, et al. Imatinib Resistance Associated With BCR-ABL Upregulation is Dependent on HIF-1alpha-Induced Metabolic Reprograming. Oncogene (2010) 29(20):2962–72. doi: 10.1038/onc.2010.67
66. Chen WL, Wang YY, Zhao A, Xia L, Xie G, Su M, et al. Enhanced Fructose Utilization Mediated by SLC2A5 Is a Unique Metabolic Feature of Acute Myeloid Leukemia With Therapeutic Potential. Cancer Cell (2016) 30(5):779–91. doi: 10.1016/j.ccell.2016.09.006
67. Matsubara S, Ding Q, Miyazaki Y, Kuwahata T, Tsukasa K, Takao S. mTOR Plays Critical Roles in Pancreatic Cancer Stem Cells Through Specific and Stemness-Related Functions. Sci Rep (2013) 3:3230. doi: 10.1038/srep03230
68. Patra KC, Hay N. The Pentose Phosphate Pathway and Cancer. Trends Biochem Sci (2014) 39(8):347–54. doi: 10.1016/j.tibs.2014.06.005
69. Poulain L, Sujobert P, Zylbersztejn F, Barreau S, Stuani L, Lambert M, et al. High Mtorc1 Activity Drives Glycolysis Addiction and Sensitivity to G6PD Inhibition in Acute Myeloid Leukemia Cells. Leukemia (2017) 31(11):2326–35. doi: 10.1038/leu.2017.81
70. Mizuno H, Koya J, Masamoto Y, Kagoya Y, Kurokawa M. Evi1 Upregulates Fbp1 and Supports Progression of Acute Myeloid Leukemia Through Pentose Phosphate Pathway Activation. Cancer Sci (2021) 112(10):4112–26. doi: 10.1111/cas.15098
71. Pei S, Minhajuddin M, D'Alessandro A, Nemkov T, Stevens B, Adane B, et al. Rational Design of a Parthenolide-Based Drug Regimen That Selectively Eradicates Acute Myelogenous Leukemia Stem Cells. J Biol Chem (2016) 291(42):21984–2000. doi: 10.1074/jbc.M116.750653
72. Tardito S, Oudin A, Ahmed SU, Fack F, Keunen O, Zheng L, et al. Glutamine Synthetase Activity Fuels Nucleotide Biosynthesis and Supports Growth of Glutamine-Restricted Glioblastoma. Nat Cell Biol (2015) 17(12):1556–68. doi: 10.1038/ncb3272
73. DiNardo CD, Rausch CR. Clinical Experience With the BCL2-Inhibitor Venetoclax in Combination Therapy for Relapsed and Refractory Acute Myeloid Leukemia and Related Myeloid Malignancies. Am J Hematol (2018) 93(3):401–7. doi: 10.1002/ajh.25000
74. Shafat MS, Oellerich T, Mohr S, Robinson SD, Edwards DR, Marlein CR, et al. Leukemic Blasts Program Bone Marrow Adipocytes to Generate a Protumoral Microenvironment. Blood (2017) 129(10):1320–32. doi: 10.1182/blood-2016-08-734798
75. Ye H, Adane B, Khan N, Sullivan T, Minhajuddin M, Gasparetto M, et al. Leukemic Stem Cells Evade Chemotherapy by Metabolic Adaptation to an Adipose Tissue Niche. Cell Stem Cell (2016) 19(1):23–37. doi: 10.1016/j.stem.2016.06.001
76. Samudio I, Harmancey R, Fiegl M, Kantarjian H, Konopleva M, Korchin B, et al. Pharmacologic Inhibition of Fatty Acid Oxidation Sensitizes Human Leukemia Cells to Apoptosis Induction. J Clin Invest (2010) 120(1):142–56. doi: 10.1172/JCI38942
77. Mao S, Ling Q, Pan J, Li F, Huang S, Ye W, et al. Inhibition of CPT1a as a Prognostic Marker Can Synergistically Enhance the Antileukemic Activity of ABT199. J Transl Med (2021) 19(1):181. doi: 10.1186/s12967-021-02848-9
78. Ricciardi MR, Mirabilii S. Targeting the Leukemia Cell Metabolism by the CPT1a Inhibition: Functional Preclinical Effects in Leukemias. Blood (2015) 126(16):1925–9. doi: 10.1182/blood-2014-12-617498
79. Anderson NM, Li D, Peng HL, Laroche FJ, Mansour MR, Gjini E, et al. The TCA Cycle Transferase DLST Is Important for MYC-Mediated Leukemogenesis. Leukemia (2016) 30(6):1365–74. doi: 10.1038/leu.2016.26
80. Fiorito V, Allocco AL, Petrillo S, Gazzano E, Torretta S, Marchi S, et al. The Heme Synthesis-Export System Regulates the Tricarboxylic Acid Cycle Flux and Oxidative Phosphorylation. Cell Rep (2021) 35(11):109252. doi: 10.1016/j.celrep.2021.109252
81. Jones CL, Stevens BM, Pollyea DA, Culp-Hill R, Reisz JA, Nemkov T, et al. Nicotinamide Metabolism Mediates Resistance to Venetoclax in Relapsed Acute Myeloid Leukemia Stem Cells. Cell Stem Cell (2020) 27(5):748–64.e4. doi: 10.1016/j.stem.2020.07.021
82. Saito K, Zhang Q, Yang H, Yamatani K. Exogenous Mitochondrial Transfer and Endogenous Mitochondrial Fission Facilitate AML Resistance to OxPhos Inhibition. Blood Adv (2021) 5(20):4233–55. doi: 10.1182/bloodadvances.2020003661
83. You R, Wang B, Chen P, Zheng X, Hou D, Wang X, et al. Metformin Sensitizes AML Cells to Chemotherapy Through Blocking Mitochondrial Transfer From Stromal Cells to AML Cells. Cancer Lett (2022) 532:215582. doi: 10.1016/j.canlet.2022.215582
84. Fenouille N, Bassil CF, Ben-Sahra I, Benajiba L, Alexe G, Ramos A, et al. The Creatine Kinase Pathway Is a Metabolic Vulnerability in EVI1-Positive Acute Myeloid Leukemia. Nat Med (2017) 23(3):301–13. doi: 10.1038/nm.4283
Keywords: leukemic stem cells (LSCs), oxidative phosphorylation (OXPHOS), electron transport chain, tricarboxylic acid cycle (TCA cycle), mitochondria
Citation: Peng M, Huang Y, Zhang L, Zhao X and Hou Y (2022) Targeting Mitochondrial Oxidative Phosphorylation Eradicates Acute Myeloid Leukemic Stem Cells. Front. Oncol. 12:899502. doi: 10.3389/fonc.2022.899502
Received: 18 March 2022; Accepted: 01 April 2022;
Published: 29 April 2022.
Edited by:
Haojian Zhang, Wuhan University, ChinaReviewed by:
Junke Zheng, Shanghai Jiaotong University School of Medicine, ChinaYiguo Hu, Sichuan University, China
Shanshan Pei, University of Colorado, United States
Copyright © 2022 Peng, Huang, Zhang, Zhao and Hou. This is an open-access article distributed under the terms of the Creative Commons Attribution License (CC BY). The use, distribution or reproduction in other forums is permitted, provided the original author(s) and the copyright owner(s) are credited and that the original publication in this journal is cited, in accordance with accepted academic practice. No use, distribution or reproduction is permitted which does not comply with these terms.
*Correspondence: Yu Hou, aG91eXVAY3FtdS5lZHUuY24=
†These authors have contributed equally to this work