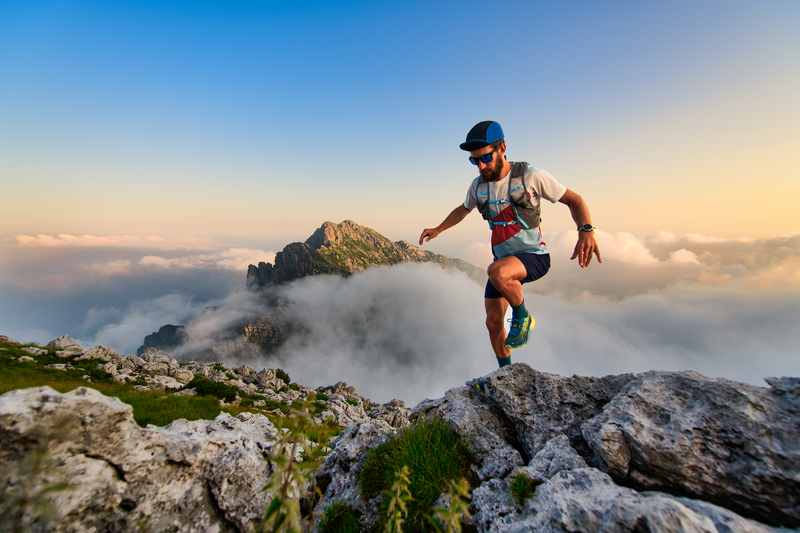
95% of researchers rate our articles as excellent or good
Learn more about the work of our research integrity team to safeguard the quality of each article we publish.
Find out more
REVIEW article
Front. Oncol. , 17 June 2022
Sec. Cancer Metabolism
Volume 12 - 2022 | https://doi.org/10.3389/fonc.2022.867271
This article is part of the Research Topic Women in Cancer Metabolism: 2021/2022 View all 13 articles
Siderophores are iron chelating molecules produced by nearly all organisms, most notably by bacteria, to efficiently sequester the limited iron that is available in the environment. Siderophores are an essential component of mammalian iron homeostasis and the ongoing interspecies competition for iron. Bacteria produce a broad repertoire of siderophores with a canonical role in iron chelation and the capacity to perform versatile functions such as interacting with other microbes and the host immune system. Siderophores are a vast area of untapped potential in the field of cancer research because cancer cells demand increased iron concentrations to sustain rapid proliferation. Studies investigating siderophores as therapeutics in cancer generally focused on the role of a few siderophores as iron chelators; however, these studies are limited and some show conflicting results. Moreover, siderophores are biologically conserved, structurally diverse molecules that perform additional functions related to iron chelation. Siderophores also have a role in inflammation due to their iron acquisition and chelation properties. These diverse functions may contribute to both risks and benefits as therapeutic agents in cancer. The potential of siderophore-mediated iron and bacterial modulation to be used in the treatment of cancer warrants further investigation. This review discusses the wide range of bacterial siderophore functions and their utilization in cancer treatment to further expand their functional relevance in cancer detection and treatment.
Siderophores are iron chelating molecules produced by nearly all organisms to enhance iron acquisition from the environment (1–3). Iron is an essential micronutrient for biological and metabolic cellular functions, but has limited availability in the environment (2, 3). Iron can accept and donate electrons and primarily exists in two states in biological systems, ferric (Fe3+) and ferrous (Fe2+), which allows iron to bind different ligands. Ferrous iron (Fe2+) can generate reactive oxygen species (ROS) through the Fenton and Haber-Weiss reactions (4, 5). At physiological pH, ferrous (Fe2+) iron is oxidized to the low solubility ferric (Fe3+) state (6). Iron levels are tightly regulated through the production of various binding molecules and transporters such as transferrin, ferritin and ferroportin, which decrease the potential of ferrous iron to generate ROS (4, 7) (Figure 1A). This regulation results in an ongoing battle for iron between mammalian hosts and their microbial inhabitants (8–11). Bacteria evolved to improve their odds in this battle by producing an extensive repertoire of siderophores that bind ferric (Fe3+) iron (2, 12). These siderophores are recognized for their diverse functionality beyond iron binding, including roles in signaling, virulence, protection against oxidative stress, metal acquisition, and competition with other microbes and their hosts (13–15).
Figure 1 (A) Mechanisms of iron acquisition between bacteria and normal epithelial cells. Bacteria-secreted siderophores and mammalian siderophores (2,5-DHBA) acquire ferric iron (Fe3+) for bacteria or host uptake. Siderophores can also chelate iron away from transferrin. LCN2 can bind the bacteria-secreted siderophores to sequester ferric iron (Fe3+) away from bacteria. Transferrin and LCN2 bind ferric iron in the extracellular space, and by binding the transferrin receptor or the LCN2 receptor (respectively) in the cell surface, the transferrin/LCN2-iron complex enters the cell through endocytosis. Once iron is in the cytoplasm, it is converted to the ferrous form (Fe2+) by the STEAP2 enzyme, and exits the endosome through the DMT1 transporter. In the cytosol, iron can be stored in ferritin back in the ferric form (Fe3+). Iron can exit the cell via ferroportin, which is regulated by hephaestin. This process is tightly regulated to avoid the generation of ROS from the labile iron pool (free ferrous iron (Fe2+) in the cytoplasm). (B) Mechanisms of iron acquisition between bacteria and cancer cells. Many cancers are characterized by increased bacterial growth and dysbiosis. Iron uptake is increased in cancer cells, which is accomplished by increasing the function of transferrin, the transferrin receptor, ferritin iron storage, and decreasing the function of ferroportin. LCN2 and its receptor are also increased during cancer. Increased ferrous iron (Fe2+) accumulation in the cytosol generates ROS.
Siderophores and iron have become relevant in carcinogenesis because cancer cells demand increased iron concentrations to sustain rapid proliferation, which increases the activity of many iron-binding molecules [transferrin, the transferrin receptor, ferritin and lipocalin 2 (Lcn2)] while decreasing the activity of the cell iron exporter ferroportin (Figure 1B) (11, 16–18). Bacterial dysbiosis is also common during cancer (19–22), which could affect siderophore secretion in this disease (Figure 1B). Iron accumulation is usually observed in tumors, which has been linked to worse cancer prognosis and increased invasion and metastasis (11, 23) (Figure 2A). There is extensive evidence that iron supports various steps of cancer progression, and modulating iron levels has been considered as a promising alternative cancer therapy. Bacterial and synthetic siderophores have been used as iron chelating agents to reduce iron levels in tumors (24) but the function and potential of siderophores in cancer continues to be severely underexplored. Published preclinical data and some clinical studies using siderophores in cancer reported both beneficial and inconsistent results (Figure 2B; Tables 1, 2) (35, 46, 68–70), suggesting that their role in cancer warrants further investigation.
Figure 2 Potential effects and mechanisms of an exogenous siderophore treatment in cancer cells. (A) Under normal conditions, cancer cells increase their iron uptake, which increases the iron labile pool (Fe2+) and ROS generation, and has been related to increased invasion, proliferation and tumor growth. (B) During exogenous siderophore treatment, siderophores bind ferric iron (Fe3+) and decrease the levels of free iron available for bacteria, LCN2 and cancer cells. As a result, cancer cells display reduced proliferation and tumor growth, and induction of apoptosis. Proposed mechanisms include: reduction of ferric iron (Fe3+) availability, the intracellular iron pool (Fe2+), ROS generation and expression of the anti-apoptotic gene Bcl-2, increasing expression of p53 and the pro-apoptotic genes Bax and Fas, activating the pro-apoptotic pathway through DDIT3, and inhibiting HDAC.
Table 2 Clinical trials that investigated siderophores and siderophore analogs as cancer therapeutics.
Siderophore interactions with the immune system contribute to the ongoing struggle for iron homeostasis. Immune cells enhance the production of siderophore-binding proteins and proinflammatory cytokines (71–73). In response, bacteria upregulate siderophore production and synthesize stealth siderophores to evade host immune defenses which will be described in detail below (3, 72). These siderophore-mediated adjustments in iron availability suggest a link between the immune response and iron accumulation in cancer cells. Insight into the balance between iron, siderophores, and immune function could contribute to a more comprehensive understanding of the role of siderophores in cancer.
This review focuses on the current research investigating the role and therapeutic potential of bacterial siderophores in cancer, highlighting their functions and interactions with the microbiome and the immune system that could be relevant in cancer research. While we provide brief summaries on siderophore functionality and the relationship between iron and cancer, we would like to direct the readers to the following reviews for a deeper understanding of these topics (4, 13, 14, 23). This review also lists the clinical trials that use siderophores and analogs as interventions in cancer. We compare and discuss their settings and regimens to provide a broader perspective of how siderophores are administered to patients, and what factors need to be taken into consideration in cancer. We searched the following databases: clinicaltrials.gov, the European Society of Medical Oncology (ESMO), and the World Health Organization. We used the following search terms: “siderophores” OR “deferoxamine” OR “deferasirox” OR “enterobactin” OR “desferrithiocin” OR “ferrichrome” OR “deferiprone” OR “2,3‑dihydroxybenzoic acid” AND “cancer”. By highlighting the role of these siderophore interactions and how they are used in preclinical cancer models, we aim to promote novel research that leverages these connections in cancer detection and treatment.
Iron is an essential element for nearly all life forms, with critical roles in various biological and metabolic processes for cell survival. Iron availability within aerobic environments is severely limited due to low ferric (Fe3+) iron solubility and low ferrous (Fe2+) iron availability (2, 3). Essentially all microorganisms, bacteria, fungi, plants, and animals produce siderophores to sequester iron from the environment. Siderophores are high-affinity iron chelators that bind iron to maintain iron levels required for survival (1, 3, 8, 12, 14). Siderophores are small molecules, around 500–1500 Daltons in molecular weight that bind primarily to ferric iron (Fe3+) (2) and exhibit high structural diversity (hundreds have been structurally characterized and described) (2, 74). Thus, siderophores are biologically important and have the potential to selectively compete in cellular processes, which makes them an interesting target in cancer research. Understanding their functions and interactions in the microbiome and with the host will be needed to explore their role and potential in cancer.
Free ferric iron (Fe3+) is tightly regulated in human hosts; most iron is bound to transferrin in serum, or to lactoferrin primarily in secretory fluids, thereby limiting iron availability for bacterial acquisition (8–10). Mammals produce a limited number of siderophores, including 2,5-dihydroxybenzoic acid (2,5-DHBA) (8, 75) and catechols (76, 77), which enable iron acquisition from transferrin, lactoferrin, and the environment Figure 1A (71, 77, 78). Synthesis of the mammalian siderophore 2,5-DHBA is catalyzed by the enzyme 3-hydroxybutyrate dehydrogenase-2 (Bdh2) (8). During the innate immune response to lipopolysaccharide exposure, toll-like receptor 4 (TLR4) suppresses Bdh2 through the transcriptional repressor B lymphocyte–induced maturation protein (Blimp-1) (8, 79). This reduces the circulating 2,5-DHBA levels during bacterial infection and limits the host-derived iron complexes available for pathogens to sequester (76, 77, 79). Embedded in the role of iron binding and transport, 2,5-DHBA is involved in intracellular iron homeostasis, erythrocyte maturation, and mitochondrial iron uptake (8, 80, 81). Decreased 2,5-DHBA through reduction of Bdh2 can lead to anemia, cytoplasmic iron accumulation, mitochondrial dysfunction, and potential apoptosis (8, 80, 81). A group of siderophore-binding proteins called lipocalins or siderocalins bind mammalian and bacterial siderophores with specificity for nearly all catecholate siderophores and some carboxylate siderophores (71, 72). Thus, mammalian siderophores, alongside lipocalins and transferrin, have a critical role in mammalian iron homeostasis through intracellular iron balance and mitochondrial function (8, 71, 77, 78, 80). Future work might uncover new siderophores and functionalities within the mammalian iron chelator protein family that could be used to alter iron availability within cancer cells.
Bacteria produce siderophores to obtain iron from their hosts or environment and to outcompete other microbes within their environment (3, 14, 82). The ferric uptake regulator (Fur) is a bacterial transcription factor that upregulates siderophore production based on low intracellular iron availability to rapidly acquire iron for metabolic processes and virulence (9, 83, 84). When iron availability is high, Fur suppresses iron acquisition and transport genes, including TonB, thereby preventing iron toxicity and intracellular oxidative stress (83–85). Siderophores enable bacteria to bind ferric iron (Fe3+) from their hosts or environment when under low iron availability, and then selectively import iron via specific cognate receptors. The specificity of siderophores and siderophore receptors enable microbes to support their own proliferation while competing with other microbial populations for limited iron resources (12, 14). However, there is evidence of both cooperation and competition among bacterial populations for iron, and bacteria can modulate siderophore production in specific hosts or environments (14, 86, 87). Cooperation in bacterial iron uptake involves the production of siderophores that can be taken up by bacterial species other than the initial producer, although there is a correlation between bacterial relatedness and cooperation. Competition in bacterial iron uptake occurs when bacteria that do not produce specific siderophores express receptors that enable siderophore uptake, thereby exploiting the siderophore production of other bacteria. Competition also happens when bacteria secrete specific siderophores for which other bacteria lack the matching receptors for uptake or when the secreted siderophores have higher affinity for iron. Therefore, the bacteria that secreted the specific siderophore (or higher affinity siderophores) decreases the iron available to other species (14, 88). Cooperation and competition has been extensively studied in Pseudomonas aeruginosa and its siderophore pyoverdine (PVD) (14, 15, 88–91).
Bacteria can synthesize multiple siderophores with varying iron affinities and minor structural variations, which can enhance iron uptake, reduce the frequency of competitive theft, and improve the competitive advantage over other microbes (3, 12, 82). Several microbes produce stealth siderophores, which are essential in evading the mammalian host innate immune molecule, siderophore binding Lcn2 (3, 72). For example, E. coli and Salmonella spp. can modify the siderophore enterobactin, which can be neutralized by Lcn2, to form salmochelin, a stealth siderophore that cannot be bound by Lcn2, thereby enhancing their survival when the acute phase response of infection and inflammation has been triggered (2, 3). Enterobactin from E. coli is usually associated with a negative impact on iron homeostasis and host health. Mice deficient in Lcn2 have increased susceptibility to E. coli-induced septicemia (73). At the same time, enterobactin increases the host iron pool and promotes mitochondrial iron uptake by binding to the ATP synthase α subunit in mammalian cells and C. elegans (92). This positive relationship between enterobactin and the host could explain why the host continues to tolerate enterobactin-producing bacteria in their microbiome.
To further showcase the multifunctionality of siderophores, PVD, a siderophore produced by P. aeruginosa, can enter C. elegans and induce death by acting as a toxin. PVD and P. aeruginosa can also disrupt C. elegans’ mitochondrial homeostasis inducing mitophagy, which is accomplished by the host as a mechanism to resist damages by PVD or P. aeruginosa (93). Exogenous iron chelators attenuate P. aeruginosa-mediated C. elegans killing by limiting bacterial growth. Interestingly, the same iron chelators reduce mitochondrial mass and induce mitochondrial fragmentation in C. elegans, and induce mitochondrial turnover and degradation in mammalian cells (93). This same group found that the siderophore PVD (but not pyochelin) was required for P. aeruginosa to induce cell death in C. elegans, which occurs through hypoxia induction. Similarly, exogenous iron chelators at higher concentrations also trigger a hypoxic response and death in C. elegans (94). The flexibility and diversity of bacterial siderophore production indicates that these molecules have important and complex roles in iron acquisition that should be taken into consideration in the context of cancer.
Both plants and fungi produce hundreds of unique siderophores. Plant siderophores (phytosiderophores) are most notably secreted by gramineous plants to obtain ferric iron (Fe3+), and likely zinc and copper, from soils that are deficient in these metals (95). Fungi utilize siderophores for iron import and can even upregulate bacterial siderophore transporters to compete for iron in the environment (2, 96–98). The focus of this review is bacterial siderophores and their role in cancer; however, the interactions among fungal, bacterial, and host iron acquisition systems may be disrupted in cancer and should be considered.
Siderophores perform a multitude of functions in addition to iron acquisition. These include roles in signaling, protection against oxidative stress, sequestration of other metals, and siderophore moieties as antibiotics (13). Although iron chelation is the most important and well-characterized role of siderophores, these additional functionalities indicate their biological significance and structural diversity (12). Understanding whether these functions can be applied or studied in the context of cancer will elucidate the potential of siderophores as cancer research targets.
Siderophores have been rigorously linked to pathogen virulence (99–104). As mentioned earlier, Pseudomonas aeruginosa produces PVD, a siderophore that stimulates its own production along with the virulence factors exotoxin A and lysyl endoprotease through a transmembrane signaling pathway described by Lamont et al. (99, 105). Klebsiella pneumoniae strain CG43 carries the virulence plasmid pLVPK, which expresses genes involved in iron acquisition, pathogenicity, capsular polysaccharide synthesis, and genes that confer resistance to lead and tellurite (106). K. pneumoniae genes for the siderophores enterobactin, aerobactin, and salmochelin are encoded in the chromosome, the plasmid, or both; aerobactin and salmochelin are associated with hypervirulence (104). Siderophore synthesis promotes iron acquisition in an iron-limited environment, which enhances microbial proliferation and pathogenic virulence during invasion and colonization of the host.
Microbes can use siderophores as intraspecies signaling molecules that are responsive to their environment and modify their own iron acquisition capabilities, and as interspecies signaling molecules between microbial populations across the environment (13). The siderophore PVD from P. aeruginosa is produced through a complex signaling pathway, described by Lamont et al. and Beare et al., that also initiates transcriptional activation of the endotoxin A (toxA) gene (99, 107, 108). Another signaling mechanism of P. aeruginosa involves siderophore-induced upregulation of the TonB-dependent receptors FoxA and FiuA in response to the heterologous siderophores ferrioxamine B and ferrichrome (109). This confers a competitive advantage to P. aeruginosa by enhancing iron sequestration from the environment. The E. coli TonB-dependent receptor FecA is activated by binding to ferric citrate, and then initiates the transcription of ferric citrate transport proteins to facilitate transport of citrate-bound iron (ferric citrate) into the cytoplasm (110, 111). Some microbial siderophores act as signaling molecules that regulate the production of their own virulence factors, stimulate iron transport, and communicate within and between microbial communities, an important consideration when factoring in disrupted iron homeostasis in hosts with cancer.
ROS are a lethal threat to microbes, and are often synthesized by mammalian polymorphonuclear lymphocytes that utilize iron as part of the innate immune response (112, 113). Siderophores have been implicated in reducing the levels of ROS produced by the host and minimizing oxidative stress through multiple mechanisms (7, 114, 115). For example, the siderophore yersiniabactin, which is produced by some Yersinia spp. and other Enterobacteriaceae, inhibits ROS production in human and mouse white blood cells by binding iron more effectively than mammalian lactoferrin and transferrin (7). This subsequently blocks the innate immune system by blocking iron acquisition for the production of ROS, which ultimately reduces oxidative stress in the presence of yersiniabactin (7). E. coli produces the enterobactin siderophore, which protects against oxidative stress (116). Enterobactin is internalized and hydrolyzed in the bacteria cytoplasm to relieve oxidative stress by scavenging radicals (116, 117). The role of siderophores in oxidative stress will be discussed in greater detail later.
Siderophores primarily bind iron, but they also can bind other metals based on their major structural group (118). P. aeruginosa produces two major siderophores, PVD and pyochelin, which form complexes with Fe3+, Ag+, Al3+, Cd2+, Co2+, Cr2+, Cu2+, Eu3+, Ga3+, Hg2+, Mn2+, Ni2+, Pb2+, Sn2+, Tb3+, Tl+, and Zn2+, although many of these metals are not efficiently transported into the cell (119, 120). The ability of siderophores to bind and selectively transport specific metals into cells allows these metals to act as cofactors for biological processes (119, 120). However, toxic metals may remain bound to siderophores with limited uptake into cells, while potentially triggering the production of additional siderophores (120–122). Toxic metals that are transported into the microbial cytoplasm can be expelled via efflux pumps, thereby enhancing microbial survival in environments containing toxic metals (119, 121–123). This is seen during E. coli infections, where E. coli secretes the siderophore yersiniabactin as a protective mechanism against copper toxicity (124). Yersiniabactin was found to be a favorable copper (II) ligand that prevents copper (II) reduction to copper (I) and helps E. coli resist toxicity during infections (7, 124).
With a different purpose, gallium (Ga3+) can replace ferric iron (Fe3+) already bound to siderophores (125). Gallium and iron share similar chemical properties, which allows gallium to also bind iron-binding molecules like transferrin and ferritin, and to be taken up by cells through the transferrin receptor (126–129). Gallium and iron differ in their pharmacokinetics and cellular functions, iron is eliminated at a faster rate than gallium and they cannot be interchanged in essential iron-catalyzed reactions (130). The ability of siderophores to bind other metals and interfere with iron metabolism suggests a therapeutic potential for gallium to control and treat bacterial infections (see section titled “Antibiotic Activity of Siderophores”). The mechanisms explored here could potentially be applied for siderophores in cancer research. Gallium treatment is already used as a cancer treatment in pre-clinical models, which we describe in the section titled “Siderophores as Iron Chelating Anticancer Agents”.
Targeting siderophore-mediated iron acquisition has become an attractive approach to treat bacteria, as they become more resistant to drug antibiotics. For example, gallium was found to reduce microbial iron uptake and hamper bacterial growth. By binding endogenous siderophores like PVD and pyochelin, gallium can be taken up instead of iron by P. aeruginosa, which disrupts iron metabolism and inhibits bacterial growth (131–134). Gallium coupled with the siderophore deferrioxamine is a successful bactericidal therapy against P. aeruginosa infection (135). Importantly, the antibacterial activity of gallium on P. aeruginosa is greatly influenced by the siderophore ligand type and the bacterial carbon source, which is important to consider when using this mechanism to develop medical therapies (136). Therefore, siderophores may have antibacterial effects by starving pathogens for iron. Similarly, pathogens can control the growth of bacteria by limiting iron acquisition through competition, discussed in the “Bacterial Siderophores” section (14). Additionally, siderophores exert antimicrobial activity against competing microbes through the formation of sideromycins (2, 13). Sideromycins are generated by linking a siderophore to an antibiotic component, although naturally occurring sideromycins are limited (2, 13, 137). A number of sideromycins have been synthesized to use as therapeutics against bacterial infections due to their targeted antimicrobial activity (10, 118, 137). Burkholderia thailandensis produces the natural sideromycin malleonitrone (138), which exhibits antibiotic activity against Gram-negative bacteria and inhibits P. aeruginosa (138). Streptomyces spp. produce the sideromycin albomycin, which inhibits protein synthesis and exerts a broad range of antibiotic and antimicrobial activities (2, 13, 137). Nonetheless, pathogenic bacteria can still develop resistance to sideromycins. This is seen in many bacteria mainly through the loss of siderophore receptors (139, 140), and therefore understanding the mechanisms of resistance is vital to develop more effective and targeted treatments. Salmycins, ferromycins, and microcins are sideromycins that exhibit more limited antimicrobial effects, typically against Gram-positive bacteria (13). The antibiotic and antimicrobial activities of sideromycins suggest that the extensive variety of siderophores produced by microbes has biological relevance beyond the acquisition of iron.
Iron has a key role in immune competency (13). As previously discussed, iron availability within hosts is limited and results in pathogen upregulation of siderophores to confer a survival advantage (83, 141). Iron availability and acquisition are critical for bacterial pathogenesis (103, 142, 143). Increased iron availability in normal and once-immunized mice was associated with lethal infection regardless of Salmonella typhimurium virulence, and the observed reduction in mortality of twice-immunized mice was associated with timing of the iron injection in relation to infection (142). Although excess siderophores in normal mice increased mortality, the injection of siderophores into immune mice did not enhance the lethality of infection (142). Siderophores enhance microbial iron acquisition and may induce competition that alters the balance of the microbiome and iron homeostasis in the host (10, 14, 144).
Iron availability within hosts potentiates pathogen infection/proliferation and cancer, so bound iron is essential for immunity. Iron regulation is more tightly controlled during host disease states as a form of nonspecific immunity used to mitigate the proliferation of pathogens or cancer (77, 145, 146). As previously mentioned, Lcn2 represents a method of iron regulation during immune stimulation as part of the acute phase response (72, 77, 147), and can be synthesized by a variety of cells, including neutrophils and epithelial cells (147–149). Mouse Lcn2 was upregulated in macrophages (in vitro) and in serum (in vivo) via TLR4 receptor signaling when stimulated or injected, respectively, with lipopolysaccharide (73). Lcn2 transcription was increased in blood cells, hepatocytes, macrophages, fibroblasts, and endothelial cells of mice infected with E. coli H9049, further implicating its role in innate immune function (73). Lcn2 has been extensively reviewed in the literature regarding its relation to cancer (150–155), whereas interactions between siderophores and the immune system have not been as comprehensively studied.
Some siderophores interact directly with the immune system by interfering with host defense mechanisms. For example, the enterobactin siderophore produced by E. coli impeded the defensive functionality of neutrophils by inhibiting ROS that prevented the formation of neutrophil extracellular traps (156). Enterobactin also inhibited myeloperoxidase activity by binding directly to the enzyme, thereby preventing an oxidative burst (157). Lcn2 can bind enterobactin and counterbalance its immune-inhibiting activity in ROS production, neutrophil extracellular trap formation, and myeloperoxidase activity (156–158). By binding siderophores, Lcn2 aids in limiting microbial iron acquisition while simultaneously enhancing the innate immune system (157, 158). However, this competition triggers the production of stealth siderophores that cannot be bound by Lcn2 (2, 157), perpetuating the battle for iron and forcing the host to utilize additional defenses. The host defense peptide LL-37 is produced ubiquitously at epithelial surfaces and binds stealth siderophores such as aerobactin and rhizoferrin to defend against pathogenic microbes (159). Siderophores and Lcn2 also have roles in stimulating the immune response (71, 160, 161). Enterobactin enhanced the secretion of Lcn2 and triggered the release of the inflammatory cytokine IL-6 (161). Siderophores and siderophore-Lcn2 complexes also increase IL-8 expression (160, 161). The Lcn2-induced secretion of proinflammatory cytokines IL-6, IL-8, and CCL20 was enhanced through superfluous siderophore iron chelation (161). Immune cells are sensitive to the levels of iron and other metals that modulate signaling, cytokine production, and antimicrobial functionality (162). Siderophores can initiate the innate immune response and modulate the immune response system through various mechanisms related to iron homeostasis. The ability of hosts to respond to siderophores and control iron homeostasis could be crucial for controlling iron availability in cancer.
The potential of siderophores as therapeutics for cancer warrants further investigation, but the complex interactions between siderophores and the immune system could complicate any beneficial effects. Therefore, it is necessary to understand the role of siderophores in iron chelation and immune response mechanisms before developing innovative cancer therapeutics.
There is a complex relationship between iron and cancer, as different cancers display distinct and altered iron regulation and metabolism (11). Health status and associated diseases have an important role in determining the fate of iron in the body and during carcinogenesis. Most solid tumors accumulate iron within the cancer site (11). Tissue-specific transcriptomic analyses showed that excess ferrous iron (Fe2+) and H2O2 undergo Fenton reactions in the cytosol and mitochondria of 14 different cancers (163). This study predicted that cytosolic Fenton reactions increase intracellular pH by producing OH–, thereby increasing glycolytic ATP and nucleotide synthesis, which are key mediators of rapid cancer proliferation (23, 164). The Fenton reaction also generates hydroxyl radicals (•OH), which promotes inflammation and metabolic rewiring, dysregulates cell signals, damages lipids in the cell membrane, and can ultimately induce iron-dependent ferroptotic cell death (23).
The relationship between iron and cancer is clear in hereditary hemochromatosis, a genetic disorder that causes increased iron absorption. Hemochromatosis is a known risk factor for hepatocellular carcinoma (HCC) (165, 166), and previous studies investigated the mechanisms underlying hemochromatosis-mediated excess iron accumulation and tumor development (167). Reducing iron levels in vitro and in vivo suppresses HCC cell growth (168), and iron chelation therapy is advised for patients with hemochromatosis. The evidence suggests that excess iron can induce p53 mutations (169), which are the primary causes for increased risk of HCC along with increased oxidative stress (170).
One of the most widely accepted hypotheses for the increased iron levels in tumors is the need for increased iron to support and sustain the rapid growth and proliferation of cancer cells compared to non-neoplastic cells. Consistent with this proposal, many cancer cells display upregulation of the iron import protein transferrin, its receptor, and the iron storage protein ferritin Figure 1B (16, 18, 171, 172). HCC, breast cancer, ovarian cancer, and colorectal cancer (CRC) display increased levels of transferrin receptor and aberrant expression of other iron transport–related proteins such as ferroportin (iron export transmembrane protein) and the divalent metal transporter (DMT1), required for iron uptake into enterocytes and transport across the endosomal membrane once iron is taken up by the cell (173) Figure 1B (174–176). Suppression of the transferrin receptor or reduction of intracellular iron levels in breast and ovarian cancers reduces cancer cell proliferation in vitro, inhibits tumor growth in vivo, and decreases metastases (175, 176). Conversely, increased cellular iron accumulation from iron loading is associated with increased proliferation in CRC cells and decreased mRNA and protein expression of E-cadherin (174). CRC cells can increase motility and invasiveness by reducing E-cadherin expression, suggesting a role for iron in promoting invasion (174). The Carotene and Retinol Efficacy Trial revealed a trend relating higher iron intake with increased risk of clinically aggressive prostate cancer (177). Although there was no significant association between iron intake and overall risk for prostate cancer in this study, it proposes that iron intake could have a prominent role in prostate cancer (177). Iron dysregulation and excess have been implicated in other cancers, including pancreas (178), lung (179), and bladder cancer (180), melanoma (36), and hematological malignancies (181, 182). These studies support the role of iron in increasing cancer risk by promoting cancer cell proliferation, invasion, tumor growth, and inhibiting apoptosis Figure 2A.
Dietary iron supplementation can modulate iron availability in the host, thereby increasing iron availability to cancer cells, promoting microbial siderophore-mediated iron acquisition, changing the intestinal microbiome, and potentially increasing inflammation (183–186). In particular, iron fortification increases the growth of enterobacteria over bifidobacteria or lactobacilli, and increases pathogenic E. coli in the microbiome of children (187). Most bifidobacteria and lactobacilli do not secrete siderophores and provide a barrier against pathogenic invasion (188, 189), whereas enterobacteria usually secrete siderophores for iron uptake. This demonstrates a powerful role of dietary iron and siderophores in bacterial growth, and the potential for these changes to influence cancer development.
There is extensive evidence, reviewed here (4, 23), that iron supports cancer progression, and that modulating iron levels can be considered a promising cancer therapy. Iron chelators have been tested as an anticancer treatment to reduce iron levels (190). These chelators consist of bidentate, tridentate, and hexadentate ligands that form octahedral complexes with ferric iron (Fe3+) (24). Siderophores secreted by bacteria are considered iron chelators, and siderophore-like molecules have been synthesized to mimic the iron chelation activity of bacterial siderophores and tested in preclinical models (Table 1) and patients (Table 2). Reducing iron levels through iron chelation has successfully reduced proliferation, tumor growth, and metastasis in preclinical models of pancreatic (191), liver (34, 38), gastric (41), breast (37, 192), prostate (193), esophageal (69), and melanoma (36) cancers (Table 1; Figure 2B); however, the results from the few patient interventions have had conflicting outcomes (Table 2). The following sections evaluate cases and studies where siderophores were involved in cancer.
There is scarce evidence on whether siderophores can be detected in tumors or in the microbiome of subjects with cancer. A small pilot study in 2017 evaluated the potential of the sputum microbiome for diagnosing lung cancer status and stage (194). The study included 10 patients referred with possible lung cancer; four were diagnosed with lung cancer after one year, and the other six remained cancer free (194). The study investigated differences in the bacterial species present in patients that developed lung cancer and those that did not. Seven bacterial species were found in both cohorts, and from those, five species were more abundant in the patients that developed lung cancer than those that did not, but only the average percentage abundance of Streptococcus viridans was significantly higher. The abundances of Granulicatella adiacens, Streptococcus intermedius, Mycobacterium tuberculosis, Streptococcus viridans, and Mycobacterium bovis were significantly higher in patients that developed lung cancer than those that did not. Of note, Mycobacterium tuberculosis and Mycobacterium bovis secrete mycobactin siderophores for iron uptake (143, 195). The researchers used metagenomics sequencing and functional alignment analyses to determine that iron siderophore receptors were higher in patients that developed lung cancer than those that did not (194). Since excess iron has been implicated in lung carcinogenesis (179), understanding the reason for sputum microbiome upregulation of iron siderophore receptors in lung cancer is relevant to understand disease progression. This was the only study that identified resident siderophores or their receptors in cancer. Future studies should characterize these molecules to further understand tumor-microbiome interactions.
Natural and synthetic siderophores have been extensively studied as potential therapies and therapy vehicles for different cancers due to their iron chelating capabilities (Table 1; Figure 2B). These approaches are discussed in the following sections.
One of the most popular bacterial siderophores in cancer therapy research is deferoxamine (Desferal, desferrioxamine, desferrioxamine-B, or DFO), which is a water-soluble trihydroxamate hexadentate siderophore secreted by many Streptomyces species (196, 197). DFO is commercially available and has been used to treat iron overload diseases (70, 198–200). In leukemia, DFO promotes apoptosis in vitro by upregulating the tumor suppressor gene p53 and the pro-apoptotic genes Bax and Fas, and reducing the expression of the anti-apoptotic gene Bcl-2 (35). DFO alone and in combination with doxorubicin chemotherapy inhibited breast tumor growth in xenograft mouse models (37). When combined with doxorubicin, DFO inhibited the cardiotoxic side effects that commonly occur in doxorubicin therapy without compromising the treatment efficacy, suggesting that siderophores have a beneficial role as an adjunct treatment with chemotherapy (37). DFO administration during the initial stages of tumor formation in a subcutaneous xenograft mouse model of HCC regressed or slowed tumor growth due to a decrease in intracellular iron concentration (Figure 2B; Table 1) (39).
Most preclinical studies demonstrated the antitumor effects of DFO. By contrast, studies in cancer patients had conflicting outcomes, with many clinical trials terminating due to difficulties in patient enrollment (Table 2). An early clinical study of ten children with recurrent neuroblastoma (NB) after 1–3 treatment regimens administered continuous IV DFO infusion at 150 mg/kg/day for five consecutive days every other week (42). Within one month from the initiation of therapy, nine patients had progressive disease, and one patient had stable disease. This study concluded that the selected dosage and interval of DFO was ineffective as a single therapy for NB patients. Higher DFO doses such as 240 mg/kg/day have serious adverse effects and are not recommended for use (42). Another clinical trial of ten children with unresectable NB administered DFO at 150 mg/kg/day followed by preoperative chemotherapy, surgery and postoperative chemotherapy (43). There were three complete responses, six partial responses, and one minor response. Nine out of ten patients underwent complete remission following surgery, and the tenth patient underwent complete remission after postoperative chemotherapy. The authors concluded that the DFO regimen used was effective in achieving complete tumor resection (43). A more recent study of ten adult patients with advanced HCC, who did not have a response to hepatic arterial infusion chemotherapy, were administered 10–80 mg/kg of DFO during 24 hours on alternate days an average of 27 times (40). The overall response rate and the 1-year cumulative survival for these patients was 20% (40), indicating that DFO treatment was not successful in the majority of cases. Only four of the clinical trials registered, that used siderophores and iron chelation therapy in cancer, used DFO for the intervention (Table 2). One of these studies focused on patients with leukemia and myelodysplastic syndrome and reported low accrual (n=5) due to the need for home administration of deferoxamine (50 mg/kg/day) and the considerable intervention window (60). All patients enrolled had iron overload and were scheduled to undergo myeloablative allogeneic hematopoietic stem cell transplantation (60). No serious adverse side effects were reported and serum ferritin decreased after the treatments, but liver iron content remained unchanged. The estimated progression-free survival after transplantation was 100% (60), but the authors are not able to draw any reliable conclusions due to the low number of patients enrolled. The other three clinical trials are in pediatric cancers with nephropathy, HCC patients, or pancreatic cancer patients. The HCC trial is currently recruiting while the pancreatic cancer trial was completed/terminated and did not provide the DFO dosage or preliminary results. Conclusions from the clinical studies described here are limited and should be taken with caution due to the low enrollment, lack of standardized dose, and absence of appropriate control groups.
DFO is a hydrophilic molecule that presents many disadvantages including poor membrane permeability, poor oral viability, and a short plasma half-life of ~12 minutes (201, 202). This requires that DFO is continuously administered subcutaneously or intravenously (68, 203) according to a rigorous infusion regime 8–12 hours/day 3–7 days/week (204, 205). As expected, this administration method has a compliance of <50% (206). The inconsistent success of DFO as an antitumor agent has encouraged the design of more effective iron chelators, with particular focus on chelator lipophilicity, membrane permeability, and selective antitumor activity.
To improve the efficacy of DFO as a cancer therapy, researchers analyzed the effects of a synthesized DFO variation bound to caffeine called desferrioxamine-caffeine dimer (DFCAF), which has greater cell permeability than DFO alone (44). DFCAF cytotoxicity was tested against cancer stem cells (CSCs, the source of biological variability within a tumor), which often determine neoplasm resistance to traditional therapies. CSCs give rise to diverse tumor cell populations and can initiate metastasis, and therefore demand a high intake of nutrients such as iron (44). DFCAF conferred greater ability to sequester iron within breast CSCs, which was measured as the concentration of an intracellular iron storage protein remaining after treatment. The CSCs also had reduced viability, reversed epithelial-to-mesenchymal transition, and increased clearance after DFCAF treatment in vitro.
Another bacterial siderophore that has been studied is desferrithiocin (DFT) (Table 1). DFT is a tridentate natural siderophore from Streptomyces antibioticus DSM (207), which displays antineoplastic activity in HCCs in vitro and has high oral effectiveness (45). However, it also confers severe nephrotoxicity, which makes it unsuitable for chelation therapy.
Enterobactin is produced by Gram-negative Enterobacteriaceae (208) (E. coli, Salmonella enterica, Shigella dysenteriae, and Klebsiella pneumoniae) and is reported to have anticancer properties (Table 1). This siderophore does not have polar properties and is permeable across the cell membrane. Although there is a lack of evidence relating this siderophore to cancer, enterobactin displays anticancer properties by disrupting the generation of ROS and disturbing the homeostasis of the labile iron (redox-active iron that can be chelated) pool in vitro. (Figure 2B) (46) Enterobactin showed efficacy in selectively chelating iron in monocyte-derived cancer cell lines and refraining from sequestering iron pools in bone marrow–derived macrophages (46). Iron-bound enterobactin exhibits cytotoxic capabilities in rapidly dividing cell lines, whereas unbound enterobactin does not display cytotoxic effects. The observed increase in intracellular Lcn2 concentrations in noncancerous cells could reflect the reduced tendency of enterobactin-induced cytotoxicity in these cells, whereas the lower Lcn2 levels in cancerous cells may allow the increased free enterobactin to exhibit its anticancer properties (46). Lcn2 levels are usually higher in many types of cancers (152) (not the case in this study), which might prevent these results from being translated to other tumor types.
Ferrichrome is another bacterial siderophore with anticancer properties (Table 1). Ferrichrome originates from the common probiotic strain Lactobacillus casei (47), and was studied for potential cytotoxic effects on gastric and colon cancer cell lines and xenograft mouse models (47, 48). Unbound ferrichrome has antitumor activity through activation of the pro-apoptotic pathway c-Jun N-terminal (JNK)-DNA damage–inducible transcript 3 (DDIT3) (Figure 2B). In this study, iron-bound ferrichrome did not display the same antitumor suppression activity. The authors suggested that the mechanism of antitumor activity in ferrichrome is likely located in the iron-chelation site (47). Another study used a xenograft model of CRC and reported similar results for the tumor suppression activity of unbound ferrichrome through upregulated DDIT3 (49). Ferrichrome treatment did not alter serum iron levels in mice, suggesting that it does not necessarily reduce systemic iron levels (49).
A recent study investigated the effects of three bacterial siderophores on the proliferation of malignant and non-malignant mouse and human cell lines: exochelin-MS (Exo-MS), mycobactin S (MBS), and deferoxamine B (DFO) (34). Exo-MS and MBS are water-soluble and lipid-soluble siderophores, respectively, and both are produced by Mycobacterium smegmatis. DFO is a water-soluble siderophore. Exo-MS inhibited the growth of mouse cancer cell lines, but not human cancer cell lines (34). DFO inhibited the growth of mouse cancer cells and human breast and leukemia cancer cells (34). MBS decreased the survival of human liver cancer cells, which was not observed with DFO or Exo-MS. The authors suggested that conjugating water-soluble siderophores with lipid molecules could increase their effectiveness against tumor cells (34).
The antitumor effects of siderophore-like molecules derived from bacteria have also been investigated. Amamistatin A and B were isolated from the actinomycete Nocardia asteroids, and have similar structures as mycobacterial siderophores. Amamistatin A displayed antiproliferative effects against the human tumor cell lines MCF-7 breast, A549 lung, and MKN45 stomach. Amamistatin A and B displayed cytotoxicity against mouse lymphocytic leukemia cells (50). The authors suggested that the antitumor activity of amamistatins could be explained by their iron chelating properties, similar to the bacterial siderophores. Amamistatins might act as histone deacetylase (HDAC) inhibitors through their N-formyl hydroxylamine or retrohydroxamate moiety. HDAC inhibition prevents tumor growth (209), and the retrohydroxamate ligand has been utilized in small molecule HDAC inhibitors (210), suggesting that amamistatins may be useful in cancer therapy and warrant further investigation.
Since siderophores are also able to bind gallium, which prevents the growth of pathogenic bacteria, research has expanded to understand this interaction in cancer. Gallium can be taken up by tumors exhibiting antineoplastic activity (211, 212). In human lymphoma cells, gallium induced cell death by activating pro-apoptotic Bax and inducing mitochondria-generated ROS (168). Gallium nitrate is approved by the FDA and is being studied in clinical trials to treat cancers like Non-Hodgkin’s Lymphoma, where it had favorable results (213). Cancer patients treated with gallium have a disrupted iron metabolism (214) which indicates that the antitumor function of gallium might be due to iron-related effects. However, the mechanism of action in cancer is still not clear, and patients treated with gallium are at higher risk of iron deficiency and complications like anemia. Continuous treatment with gallium nitrate promotes the development of resistance, which is accompanied by changes in iron trafficking and metabolism (134). Interestingly, other types of gallium, like gallium maltolate, can still inhibit the growth of lymphoma cells that become resistant to gallium nitrate (215). Since some bacteria can become resistant to sideromycins, it will be important to assess whether bacterial and synthetic siderophores could generate resistance in cancer cells.
Recent research focus has shifted to the development of synthetic iron chelators with superior pharmacological properties and similar preclinical results (211, 212), that avoid the adverse effects of siderophores in humans. Optimal iron chelators for cancer treatment must be readily absorbable, with a long-half life in the blood and higher affinity for iron over other metals, although we have reported the benefits of siderophores being able to bind other metals. Selectivity for or against cancer cells is also an important characteristic that should be investigated to avoid systemic iron deprivation when it is not needed (212, 216). The synthetic iron chelator thiosemicarbazone-24 (TSC24) suppressed human HCC tumor growth by disrupting iron homeostasis, reducing available iron, and triggering cell cycle arrest and apoptosis without any apparent host toxicity (168). Other synthetic iron chelators currently in preclinical studies as anticancer agents include deferiprone (193) and deferasirox (191, 192, 217), which are also in clinical trials to treat cancers related to iron overload (Table 2).
All clinical trials using iron chelation as a cancer therapy are summarized in Table 2. A number of bacterial siderophores and siderophore analogs are studied in preclinical studies; however, only DFO, deferasirox (synthetic), and deferiprone (synthetic) are undergoing clinical trials. Deferasirox is more commonly used than DFO, probably due to its more convenient oral administration. None of these clinical trials are investigating the effects of chelators/siderophores in solid tumors (other than one HCC and one pancreatic cancer study without results), whereas most of the research in preclinical studies is focused on tumors. All conditions and diseases studied in the clinical trials involve systemic iron overload, including beta-thalassemia, leukemia, and myelodysplastic syndrome (which requires increased blood transfusions). The clinical studies are not reflective of the preclinical data generated in mouse models and in vitro cell cultures, where siderophores are studied for tumor treatment regardless of systemic iron overload. A potential reason for this is that these chelators act systemically and do not specifically target the tumor. Therefore, other tissues could be damaged and anemia could be developed by a drastic reduction in iron levels. Future studies should address chelator targeting for non-systemic reduction in tumor iron levels and obtain further evidence to increase the translation of these studies to the clinic.
Siderophores and their analogs are also used for iron transport–mediated drug delivery through sideromycins (Trojan horse antibiotics). Microbes can develop antibiotic resistance by altering permeability barriers, altering the drug target binding sites, synthesizing enzymes that destroy antibiotics, and developing mechanisms to transport antibiotics out of the cell before they induce damage (218). Siderophores can evade membrane-associated drug resistance because microbes require nutrient uptake from the environment for survival, which enables a route and target of drug delivery. Siderophores were investigated as a drug transport agent to overcome antibiotic resistance and target pathogenic bacteria. Successful results in targeting antibiotic-resistant bacteria suggested that this mechanism could be used to target cancer cells. This seems to be a promising proposition, as siderophore-iron complexes can bind Lcn2 and travel in circulation (219), and iron-loaded Lcn2 can be taken up by cancer cells where Lcn2 is commonly upregulated (220), creating a pathway for the implementation of a modified “Trojan horse” drug delivery.
A recent study conjugated the siderophore PVD from P. aeruginosa, a mixed-type siderophore with hydroxamate and catecholate groups, to synthesize superparamagnetic iron oxide nanoparticles (SPION) (221). The PVD-iron complex has strong binding and high stability, which protects it from hydrolysis and enzymatic degradation. PVD-SPION was covalently conjugated to a mucine 1 aptamer (MUC1Apt) and loaded with doxorubicin, which is used in chemotherapy. MUC1Apt is one of the most studied aptamers as it specifically recognizes the mucin 1 (MUC1) protein that is strongly upregulated in most cancer cell surfaces, which makes it a great target in cancer (222). The investigators showed that the PVD-SPION-MUC1Apt complex was successfully taken up by cancer cells, and conferred tumor inhibitory growth effects and improved survival in mice bearing C26 colon carcinoma (221). This complex also served as a diagnostic agent that improved contrast at the tumor site in magnetic resonance images (221). This study is one of the first to show the potential of siderophores as chemotherapy delivery agents and diagnostic tools in cancer.
While siderophore treatment in cancer has shown some beneficial results in pre-clinical models, and some siderophores and analogs have been used in clinical trials, additional studies are needed to determine side effects or potential risks of these interventions. Understanding siderophore functions in mammalian cells will help assess the risk of siderophore implementation in cancer. We previously mentioned that iron chelation reduces mitochondrial mass and induces mitochondrial fragmentation in C. elegans, and that it promotes mitochondrial degradation in mammalian cells (93) In addition, iron chelation induces C. elegans’ death through hypoxia (94). Iron chelation therapy is commonly used to lower systemic iron levels in iron overload diseases (202), and data from the literature suggest that chelation therapy could also enhance iron absorption, which might be undesirable in the context of cancer (223). Therefore, investigating the effects of siderophore therapy on the microbiome, the mitochondria (in not only cancer cells, but also other cells of the body) and systemic and cellular iron levels, will be vital when evaluating the feasibility of these interventions.
Another factor to consider is whether cancer cells can become resistant to siderophore treatment, as bacteria are known to become resistant to sideromycins by losing their siderophore receptors (139, 140), and to gallium nitrate treatment (134). Moreover, it is supported that cancer cells employ Lcn2 to collect extracellular iron to support cancer growth in renal cell carcinoma (220) and leptomeningeal metastasis (224). Thus, understanding the function of Lcn2 as a result of a siderophore treatment will further elucidate additional risks related to these treatments.
Siderophores are secreted by many organisms including bacteria, to sequester essential iron from the environment to sustain their growth. This function has gained interest in cancer research because excess iron availability is linked to increased cancer risk, and cancer cells require higher iron levels to sustain their rapid proliferation and growth Figure 1 (71, 225). Therefore, bacterial siderophores and siderophore analogs are being utilized to chelate iron and prevent it from being taken up by cancer cells, which inhibits the proliferation and growth of many different cancers in preclinical studies Figure 2.
Siderophores also function as signaling molecules that can lead to the production of virulence factors and the regulation of siderophore synthesis. Siderophores can interact with the immune system, mitigate oxidative stress, protect microbes from ROS and bind other metals in addition to ferric iron (Fe3+). Finally, siderophores can form sideromycins and have antimicrobial effects against other microbes in the environment. Each of these described siderophore functions does not fully consider the interactions between microbes in a competitive environment, and how these functions may be enhanced or suppressed through these interactions.
There is still much to learn about the effects and interactions of siderophores in cancer. Most of the preclinical and clinical studies use inconsistent dosages, conditions, and safety and outcome measures, which reduce their translational value for additional studies in patients. Data regarding endogenous siderophores in cancer and related changes to the microbiome are also lacking. Additional studies are needed in these areas to assess the potential efficacy of siderophores in cancer detection and therapeutics. This review discussed bacterial siderophores and analogs that have potential benefits for cancer therapy, but even less is known about mammalian or fungal siderophores in cancer and immunity. It will be crucial to map the relationships between siderophores, iron, immune function, and cancer to develop stable, effective, and targeted therapeutics. These efforts should leverage the functions of siderophores and translate the outcomes to the clinical setting.
VP-G: study concept and design, development of methodology, drafting of initial manuscript, writing, review, and/or revision of the manuscript, administrative, technical, or material support, and final approval of the version to be submitted. KC: study concept and design, development of methodology, drafting of initial manuscript, writing, review, and/or revision of the manuscript, administrative, technical, or material support, and final approval of the version to be submitted. TS: drafting of initial manuscript and writing, review, and/or revision of the manuscript. ZC-M: study concept and design, development of methodology, drafting of initial manuscript, writing, review, and/or revision of the manuscript, administrative, technical, or material support, final approval of the version to be submitted, and study supervision.
This publication was supported by The National Cancer Institute (NCI) R01CA223204 (ZC-M) and the Pelotonia Fellowship Program (VP-G and TS). The content is solely the responsibility of the authors and does not necessarily represent the official views of the National Institutes of Health. Any opinions, findings, and conclusions expressed in this material are those of the author(s) and do not necessarily reflect those of the Pelotonia Fellowship Program or The Ohio State University.
The authors declare that the research was conducted in the absence of any commercial or financial relationships that could be construed as a potential conflict of interest.
All claims expressed in this article are solely those of the authors and do not necessarily represent those of their affiliated organizations, or those of the publisher, the editors and the reviewers. Any product that may be evaluated in this article, or claim that may be made by its manufacturer, is not guaranteed or endorsed by the publisher.
All figures were created with BioRender.com.
1. Neilands JB. Microbial Iron Compounds. Annu Rev Biochem (1981) 50:715–31. doi: 10.1146/annurev.bi.50.070181.003435
2. Hider RC, Kong X. Chemistry and Biology of Siderophores. Nat Prod Rep (2010) 27(5):637–57. doi: 10.1039/b906679a
3. Wilson BR, Bogdan AR, Miyazawa M, Hashimoto K, Tsuji Y. Siderophores in Iron Metabolism: From Mechanism to Therapy Potential. Trends Mol Med (2016) 22(12):1077–90. doi: 10.1016/j.molmed.2016.10.005
4. Torti SV, Manz DH, Paul BT, Blanchette-Farra N, Torti FM. Iron and Cancer. Annu Rev Nutr (2018) 38:97–125. doi: 10.1146/annurev-nutr-082117-051732
5. Fenton HJH. LXXIII—Oxidation of Tartaric Acid in Presence of Iron. J Chem Soc Trans (1894) 65:899–910. doi: 10.1039/CT8946500899
6. Ems T, St Lucia K, Huecker MR. Biochemistry, Iron Absorption. Treasure Island, FL: StatPearls (2022).
7. Paauw A, Leverstein-van Hall MA, van Kessel KP, Verhoef J, Fluit AC. Yersiniabactin Reduces the Respiratory Oxidative Stress Response of Innate Immune Cells. PloS One (2009) 4(12):e8240. doi: 10.1371/journal.pone.0008240
8. Devireddy LR, Hart DO, Goetz DH, Green MR. A Mammalian Siderophore Synthesized by an Enzyme With a Bacterial Homolog Involved in Enterobactin Production. Cell (2010) 141(6):1006–17. doi: 10.1016/j.cell.2010.04.040
9. Raymond KN, Dertz EA, Kim SS. Enterobactin: An Archetype for Microbial Iron Transport. Proc Natl Acad Sci USA (2003) 100(7):3584–8. doi: 10.1073/pnas.0630018100
10. Page MGP. The Role of Iron and Siderophores in Infection, and the Development of Siderophore Antibiotics. Clin Infect Dis (2019) 69(Suppl 7):S529–S37. doi: 10.1093/cid/ciz825
11. Torti SV, Torti FM. Iron and Cancer: More Ore to be Mined. Nat Rev Cancer (2013) 13(5):342–55. doi: 10.1038/nrc3495
12. McRose DL, Seyedsayamdost MR, Morel FMM. Multiple Siderophores: Bug or Feature? J Biol Inorg Chem (2018) 23(7):983–93. doi: 10.1007/s00775-018-1617-x
13. Johnstone TC, Nolan EM. Beyond Iron: Non-Classical Biological Functions of Bacterial Siderophores. Dalton Trans (2015) 44(14):6320–39. doi: 10.1039/C4DT03559C
14. Kramer J, Özkaya Ö, Kümmerli R. Bacterial Siderophores in Community and Host Interactions. Nat Rev Microbiol (2020) 18(3):152–63. doi: 10.1038/s41579-019-0284-4
15. Kümmerli R, Jiricny N, Clarke LS, West SA, Griffin AS. Phenotypic Plasticity of a Cooperative Behaviour in Bacteria. J Evol Biol (2009) 22(3):589–98. doi: 10.1111/j.1420-9101.2008.01666.x
16. Kew MC, Torrance JD, Derman D, Simon M, Macnab GM, Charlton RW, et al. Serum and Tumour Ferritins in Primary Liver Cancer. Gut (1978) 19(4):294–9. doi: 10.1136/gut.19.4.294
17. Marcus DM, Zinberg N. Isolation of Ferritin From Human Mammary and Pancreatic Carcinomas by Means of Antibody Immunoadsorbents. Arch Biochem Biophys (1974) 162(2):493–501. doi: 10.1016/0003-9861(74)90209-4
18. Weinstein RE, Bond BH, Silberberg BK. Tissue Ferritin Concentration in Carcinoma of the Breast. Cancer (1982) 50(11):2406–9. doi: 10.1002/1097-0142(19821201)50:11<2406::AID-CNCR2820501127>3.0.CO;2-S
19. Mendez R, Kesh K, Arora N, Di Martino L, McAllister F, Merchant N, et al. Microbial Dysbiosis and Polyamine Metabolism as Predictive Markers for Early Detection of Pancreatic Cancer. Carcinogenesis (2020) 41(5):561–70. doi: 10.1093/carcin/bgz116
20. Riquelme E, Zhang Y, Zhang L, Montiel M, Zoltan M, Dong W, et al. Tumor Microbiome Diversity and Composition Influence Pancreatic Cancer Outcomes. Cell (2019) 178(4):795–806.e12. doi: 10.1016/j.cell.2019.07.008
21. Koliarakis I, Messaritakis I, Nikolouzakis TK, Hamilos G, Souglakos J, Tsiaoussis J. Oral Bacteria and Intestinal Dysbiosis in Colorectal Cancer. Int J Mol Sci (2019) 20(17):4146. doi: 10.3390/ijms20174146
22. Gopalakrishnan V, Helmink BA, Spencer CN, Reuben A, Wargo JA. The Influence of the Gut Microbiome on Cancer, Immunity, and Cancer Immunotherapy. Cancer Cell (2018) 33(4):570–80. doi: 10.1016/j.ccell.2018.03.015
23. Brown RAM, Richardson KL, Kabir TD, Trinder D, Ganss R, Leedman PJ. Altered Iron Metabolism and Impact in Cancer Biology, Metastasis, and Immunology. Front Oncol (2020) 10:476. doi: 10.3389/fonc.2020.00476
24. Zhou T, Ma Y, Kong X, Hider RC. Design of Iron Chelators With Therapeutic Application. Dalton Trans (2012) 41(21):6371–89. doi: 10.1039/c2dt12159j
25. National Center for Biotechnology Information (2022). PubChem Compound Summary for CID 2973, Deferoxamine. Retrieved May 24, 2022 from https://pubchem.ncbi.nlm.nih.gov/compound/Deferoxamine.
26. National Center for Biotechnology Information (2022). PubChem Compound Summary for CID 2519, Caffeine. Retrieved May 24, 2022 from https://pubchem.ncbi.nlm.nih.gov/compound/Caffeine.
27. National Center for Biotechnology Information (2022). PubChem Compound Summary for CID 101609363. Retrieved May 24, 2022 from https://pubchem.ncbi.nlm.nih.gov/compound/101609363.
28. National Center for Biotechnology Information (2022). PubChem Compound Summary for CID 34231, Enterobactin. Retrieved May 24, 2022 from https://pubchem.ncbi.nlm.nih.gov/compound/Enterobactin.
29. National Center for Biotechnology Information (2022). PubChem Compound Summary for CID 644246, [55Fe] Ferrichrome. Retrieved May 24, 2022 from https://pubchem.ncbi.nlm.nih.gov/compound/55Fe_-Ferrichrome.
30. National Center for Biotechnology Information (2022). PubChem Compound Summary for CID 139583168, Exochelin MS. Retrieved May 24, 2022 from https://pubchem.ncbi.nlm.nih.gov/compound/Exochelin-MS.
31. National Center for Biotechnology Information (2022). PubChem Compound Summary for CID 3083702, Mycobactin. Retrieved May 24, 2022 from https://pubchem.ncbi.nlm.nih.gov/compound/Mycobactin.
32. National Center for Biotechnology Information. (2022). PubChemCompound Summary for CID 13543048, Amamistatin A. Retrieved May 24. Available at: https://pubchem.ncbi.nlm.nih.gov/compound/amamistatin_a..
33. National Center for Biotechnology Information. (2022). PubChemCompound Summary for CID 135438025, Amamistatin B. Retrieved May 24, Available at: https://pubchem.ncbi.nlm.nih.gov/compound/amamistatin_b.
34. Gokarn K, Sarangdhar V, Pal RB. Effect of Microbial Siderophores on Mammalian Non-Malignant and Malignant Cell Lines. BMC Complement Altern Med (2017) 17(1):145. doi: 10.1186/s12906-017-1657-8
35. Yang Y, Xu Y, Su A, Yang D, Zhang X. Effects of Deferoxamine on Leukemia In Vitro and Its Related Mechanism. Med Sci Monit (2018) 24:6735–41. doi: 10.12659/MSM.910325
36. Whitnall M, Howard J, Ponka P, Richardson DR. A Class of Iron Chelators With a Wide Spectrum of Potent Antitumor Activity That Overcomes Resistance to Chemotherapeutics. Proc Natl Acad Sci USA (2006) 103(40):14901–6. doi: 10.1073/pnas.0604979103
37. Hoke EM, Maylock CA, Shacter E. Desferal Inhibits Breast Tumor Growth and Does Not Interfere With the Tumoricidal Activity of Doxorubicin. Free Radic Biol Med (2005) 39(3):403–11. doi: 10.1016/j.freeradbiomed.2005.03.029
38. Saeki I, Yamamoto N, Yamasaki T, Takami T, Maeda M, Fujisawa K, et al. Effects of an Oral Iron Chelator, Deferasirox, on Advanced Hepatocellular Carcinoma. World J Gastroenterol (2016) 22(40):8967–77. doi: 10.3748/wjg.v22.i40.8967
39. Hann HW, Stahlhut MW, Rubin R, Maddrey WC. Antitumor Effect of Deferoxamine on Human Hepatocellular Carcinoma Growing in Athymic Nude Mice. Cancer (1992) 70(8):2051–6. doi: 10.1002/1097-0142(19921015)70:8<2051::AID-CNCR2820700806>3.0.CO;2-1
40. Yamasaki T, Terai S, Sakaida I. Deferoxamine for Advanced Hepatocellular Carcinoma. N Engl J Med (2011) 365(6):576–8. doi: 10.1056/NEJMc1105726
41. Kim JL, Lee DH, Na YJ, Kim BR, Jeong YA, Lee SI, et al. Iron Chelator-Induced Apoptosis via the ER Stress Pathway in Gastric Cancer Cells. Tumour Biol (2016) 37(7):9709–19. doi: 10.1007/s13277-016-4878-4
42. Blatt J. Deferoxamine in Children With Recurrent Neuroblastoma. Anticancer Res (1994) 14(5B):2109–12.
43. Donfrancesco A, Deb G, Dominici C, De Sio L, Inserra A, Boglino C, et al. D-CECaT as Preoperative Chemotherapy for Unresectable Neuroblastoma in Children Over One Year of Age. Anticancer Res (1995) 15(5B):2347–50.
44. Li B, Esposito BP, Wang S, Zhang J, Xu M, Zhang S, et al. Desferrioxamine-Caffeine Shows Improved Efficacy in Chelating Iron and Depleting Cancer Stem Cells. J Trace Elem Med Biol (2019) 52:232–8. doi: 10.1016/j.jtemb.2019.01.004
45. Bergeron RJ, Wiegand J, Dionis JB, Egli-Karmakka M, Frei J, Huxley-Tencer A, et al. Evaluation of Desferrithiocin and its Synthetic Analogues as Orally Effective Iron Chelators. J Med Chem (1991) 34(7):2072–8. doi: 10.1021/jm00111a023
46. Saha P, Yeoh BS, Xiao X, Golonka RM, Kumarasamy S, Vijay-Kumar M. Enterobactin, an Iron Chelating Bacterial Siderophore, Arrests Cancer Cell Proliferation. Biochem Pharmacol (2019) 168:71–81. doi: 10.1016/j.bcp.2019.06.017
47. Ijiri M, Fujiya M, Konishi H, Tanaka H, Ueno N, Kashima S, et al. Ferrichrome Identified From Lactobacillus Casei ATCC334 Induces Apoptosis Through its Iron-Binding Site in Gastric Cancer Cells. Tumour Biol (2017) 39(6):1010428317711311. doi: 10.1177/1010428317711311
48. Konishi H, Fujiya M, Tanaka H, Ueno N, Moriichi K, Sasajima J, et al. Probiotic-Derived Ferrichrome Inhibits Colon Cancer Progression via JNK-Mediated Apoptosis. Nat Commun (2016) 7:12365. doi: 10.1038/ncomms12365
49. Iwama T, Fujiya M, Konishi H, Tanaka H, Murakami Y, Kunogi T, et al. Bacteria-Derived Ferrichrome Inhibits Tumor Progression in Sporadic Colorectal Neoplasms and Colitis-Associated Cancer. Cancer Cell Int (2021) 21(1):21. doi: 10.1186/s12935-020-01723-9
50. Fennell KA, Mollmann U, Miller MJ. Syntheses and Biological Activity of Amamistatin B and Analogs. J Org Chem (2008) 73(3):1018–24. doi: 10.1021/jo7020532
51. Angelucci E, Li J, Greenberg P, Wu D, Hou M, Montano Figueroa EH, et al. Iron Chelation in Transfusion-Dependent Patients With Low- to Intermediate-1-Risk Myelodysplastic Syndromes: A Randomized Trial. Ann Intern Med (2020) 172(8):513–22. doi: 10.7326/M19-0916
52. Cappellini MD, Porter J, El-Beshlawy A, Li CK, Seymour JF, Elalfy M, et al. Tailoring Iron Chelation by Iron Intake and Serum Ferritin: The Prospective EPIC Study of Deferasirox in 1744 Patients With Transfusion-Dependent Anemias. Haematologica (2010) 95(4):557–66. doi: 10.3324/haematol.2009.014696
53. Nolte F, Hochsmann B, Giagounidis A, Lubbert M, Platzbecker U, Haase D, et al. Results From a 1-Year, Open-Label, Single Arm, Multi-Center Trial Evaluating the Efficacy and Safety of Oral Deferasirox in Patients Diagnosed With Low and Int-1 Risk Myelodysplastic Syndrome (MDS) and Transfusion-Dependent Iron Overload. Ann Hematol (2013) 92(2):191–8. doi: 10.1007/s00277-012-1594-z
54. Borgna-Pignatti C, Franchini M, Gandini G, Vassanelli A, De Gironcoli M, Aprili G. Subcutaneous Bolus Injection of Deferoxamine in Adult Patients Affected by Onco-Hematologic Diseases and Iron Overload. Haematologica (1998) 83(9):788–90.
55. Galanello R. Evaluation of ICL670, a Once-Daily Oral Iron Chelator in a Phase III Clinical Trial of Beta-Thalassemia Patients With Transfusional Iron Overload. Ann N Y Acad Sci (2005) 1054:183–5. doi: 10.1196/annals.1345.021
56. Efficace F, Santini V, La Nasa G, Cottone F, Finelli C, Borin L, et al. Health-Related Quality of Life in Transfusion-Dependent Patients With Myelodysplastic Syndromes: A Prospective Study to Assess the Impact of Iron Chelation Therapy. BMJ Support Palliat Care (2016) 6(1):80–8. doi: 10.1136/bmjspcare-2014-000726
57. Ho PJ, Tay L, Teo J, Marlton P, Grigg A, St Pierre T, et al. Cardiac Iron Load and Function in Transfused Patients Treated With Deferasirox (the MILE Study). Eur J Haematol (2017) 98(2):97–105. doi: 10.1111/ejh.12793
58. Greenberg PL, Koller CA, Cabantchik ZI, Warsi G, Glynos T, Paley C, et al. Prospective Assessment of Effects on Iron-Overload Parameters of Deferasirox Therapy in Patients With Myelodysplastic Syndromes. Leuk Res (2010) 34(12):1560–5. doi: 10.1016/j.leukres.2010.06.013
59. Ghoti H, Fibach E, Merkel D, Perez-Avraham G, Grisariu S, Rachmilewitz EA. Changes in Parameters of Oxidative Stress and Free Iron Biomarkers During Treatment With Deferasirox in Iron-Overloaded Patients With Myelodysplastic Syndromes. Haematologica (2010) 95(8):1433–4. doi: 10.3324/haematol.2010.024992
60. Armand P, Sainvil MM, Kim HT, Rhodes J, Cutler C, Ho VT, et al. Pre-Transplantation Iron Chelation in Patients With MDS or Acute Leukemia and Iron Overload Undergoing Myeloablative Allo-SCT. Bone Marrow Transplant (2013) 48(1):146–7. doi: 10.1038/bmt.2012.94
61. Messa E, Carturan S, Maffe C, Pautasso M, Bracco E, Roetto A, et al. Deferasirox Is a Powerful NF-kappaB Inhibitor in Myelodysplastic Cells and in Leukemia Cell Lines Acting Independently From Cell Iron Deprivation by Chelation and Reactive Oxygen Species Scavenging. Haematologica (2010) 95(8):1308–16. doi: 10.3324/haematol.2009.016824
62. Brissot P, Ball S, Rofail D, Cannon H, Jin VW. Hereditary Hemochromatosis: Patient Experiences of the Disease and Phlebotomy Treatment. Transfusion (2011) 51(6):1331–8. doi: 10.1111/j.1537-2995.2010.02997.x
63. Phatak P, Brissot P, Wurster M, Adams PC, Bonkovsky HL, Gross J, et al. A Phase 1/2, Dose-Escalation Trial of Deferasirox for the Treatment of Iron Overload in HFE-Related Hereditary Hemochromatosis. Hepatology (2010) 52(5):1671–779. doi: 10.1002/hep.23879
64. Barany E, Bergdahl IA, Bratteby LE, Lundh T, Samuelson G, Skerfving S, et al. Iron Status Influences Trace Element Levels in Human Blood and Serum. Environ Res (2005) 98(2):215–23. doi: 10.1016/j.envres.2004.09.010
65. Hori A, Mizoue T, Kasai H, Kawai K, Matsushita Y, Nanri A, et al. Body Iron Store as a Predictor of Oxidative DNA Damage in Healthy Men and Women. Cancer Sci (2010) 101(2):517–22. doi: 10.1111/j.1349-7006.2009.01394.x
66. Fukushima T, Kawabata H, Nakamura T, Iwao H, Nakajima A, Miki M, et al. Iron Chelation Therapy With Deferasirox Induced Complete Remission in a Patient With Chemotherapy-Resistant Acute Monocytic Leukemia. Anticancer Res (2011) 31(5):1741–4.
67. Paubelle E, Zylbersztejn F, Alkhaeir S, Suarez F, Callens C, Dussiot M, et al. Deferasirox and Vitamin D Improves Overall Survival in Elderly Patients With Acute Myeloid Leukemia After Demethylating Agents Failure. PloS One (2013) 8(6):e65998. doi: 10.1371/journal.pone.0065998
68. Donfrancesco A, Deb G, De Sio L, Cozza R, Castellano A. Role of Deferoxamine in Tumor Therapy. Acta Haematol (1996) 95(1):66–9. doi: 10.1159/000203951
69. Ford SJ, Obeidy P, Lovejoy DB, Bedford M, Nichols L, Chadwick C, et al. Deferasirox (ICL670A) Effectively Inhibits Oesophageal Cancer Growth In Vitro and In Vivo. Br J Pharmacol (2013) 168(6):1316–28. doi: 10.1111/bph.12045
70. Gattermann N. Overview of Guidelines on Iron Chelation Therapy in Patients With Myelodysplastic Syndromes and Transfusional Iron Overload. Int J Hematol (2008) 88(1):24–9. doi: 10.1007/s12185-008-0118-z
71. Golonka R, Yeoh BS, Vijay-Kumar M. The Iron Tug-Of-War Between Bacterial Siderophores and Innate Immunity. J Innate Immun (2019) 11(3):249–62. doi: 10.1159/000494627
72. Xiao X, Yeoh BS, Vijay-Kumar M. Lipocalin 2: An Emerging Player in Iron Homeostasis and Inflammation. Annu Rev Nutr (2017) 37:103–30. doi: 10.1146/annurev-nutr-071816-064559
73. Flo TH, Smith KD, Sato S, Rodriguez DJ, Holmes MA, Strong RK, et al. Lipocalin 2 Mediates an Innate Immune Response to Bacterial Infection by Sequestrating Iron. Nature (2004) 432(7019):917–21. doi: 10.1038/nature03104
74. Saha M, Sarkar S, Sarkar B, Sharma BK, Bhattacharjee S, Tribedi P. Microbial Siderophores and Their Potential Applications: A Review. Environ Sci Pollut Res Int (2016) 23(5):3984–99. doi: 10.1007/s11356-015-4294-0
75. Liu Z, Lanford R, Mueller S, Gerhard GS, Luscieti S, Sanchez M, et al. Siderophore-Mediated Iron Trafficking in Humans Is Regulated by Iron. J Mol Med (Berl) (2012) 90(10):1209–21. doi: 10.1007/s00109-012-0899-7
76. Caza M, Kronstad JW. Shared and Distinct Mechanisms of Iron Acquisition by Bacterial and Fungal Pathogens of Humans. Front Cell Infect Microbiol (2013) 3:80. doi: 10.3389/fcimb.2013.00080
77. Correnti C, Strong RK. Mammalian Siderophores, Siderophore-Binding Lipocalins, and the Labile Iron Pool. J Biol Chem (2012) 287(17):13524–31. doi: 10.1074/jbc.R111.311829
78. Khan A, Singh P, Srivastava A. Synthesis, Nature and Utility of Universal Iron Chelator - Siderophore: A Review. Microbiol Res (2018) 212-213:103–11. doi: 10.1016/j.micres.2017.10.012
79. Liu Z, Reba S, Chen WD, Porwal SK, Boom WH, Petersen RB, et al. Regulation of Mammalian Siderophore 2,5-DHBA in the Innate Immune Response to Infection. J Exp Med (2014) 211(6):1197–213. doi: 10.1084/jem.20132629
80. Liu Z, Ciocea A, Devireddy L. Endogenous Siderophore 2,5-Dihydroxybenzoic Acid Deficiency Promotes Anemia and Splenic Iron Overload in Mice. Mol Cell Biol (2014) 34(13):2533–46. doi: 10.1128/MCB.00231-14
81. Davuluri G, Song P, Liu Z, Wald D, Sakaguchi TF, Green MR, et al. Inactivation of 3-Hydroxybutyrate Dehydrogenase 2 Delays Zebrafish Erythroid Maturation by Conferring Premature Mitophagy. Proc Natl Acad Sci USA (2016) 113(11):E1460–9. doi: 10.1073/pnas.1600077113
82. Holden VI, Bachman MA. Diverging Roles of Bacterial Siderophores During Infection. Metallomics (2015) 7(6):986–95. doi: 10.1039/C4MT00333K
83. Santos R, Batista BB, da Silva Neto JF. Ferric Uptake Regulator Fur Coordinates Siderophore Production and Defense Against Iron Toxicity and Oxidative Stress and Contributes to Virulence in Chromobacterium Violaceum. Appl Environ Microbiol (2020) 86(21):e01620–20. doi: 10.1128/AEM.01620-20
84. Fillat MF, The FUR. (Ferric Uptake Regulator) Superfamily: Diversity and Versatility of Key Transcriptional Regulators. Arch Biochem Biophys (2014) 546:41–52. doi: 10.1016/j.abb.2014.01.029
85. da Silva Neto JF, Braz VS, Italiani VC, Marques MV. Fur Controls Iron Homeostasis and Oxidative Stress Defense in the Oligotrophic Alpha-Proteobacterium Caulobacter Crescentus. Nucleic Acids Res (2009) 37(14):4812–25. doi: 10.1093/nar/gkp509
86. West SA, Buckling A. Cooperation, Virulence and Siderophore Production in Bacterial Parasites. Proc Biol Sci (2003) 270(1510):37–44. doi: 10.1098/rspb.2002.2209
87. Niehus R, Picot A, Oliveira NM, Mitri S, Foster KR. The Evolution of Siderophore Production as a Competitive Trait. Evolution (2017) 71(6):1443–55. doi: 10.1111/evo.13230
88. Stilwell P, Lowe C, Buckling A. The Effect of Cheats on Siderophore Diversity in Pseudomonas Aeruginosa. J Evol Biol (2018) 31(9):1330–9. doi: 10.1111/jeb.13307
89. Buckling A, Harrison F, Vos M, Brockhurst MA, Gardner A, West SA, et al. Siderophore-Mediated Cooperation and Virulence in Pseudomonas Aeruginosa. FEMS Microbiol Ecol (2007) 62(2):135–41. doi: 10.1111/j.1574-6941.2007.00388.x
90. Butaitė E, Baumgartner M, Wyder S, Kümmerli R. Siderophore Cheating and Cheating Resistance Shape Competition for Iron in Soil and Freshwater Pseudomonas Communities. Nat Commun (2017) 8(1):414. doi: 10.1038/s41467-017-00509-4
91. Harrison F, Paul J, Massey RC, Buckling A. Interspecific Competition and Siderophore-Mediated Cooperation in Pseudomonas Aeruginosa. Isme J (2008) 2(1):49–55. doi: 10.1038/ismej.2007.96
92. Qi B, Han M. Microbial Siderophore Enterobactin Promotes Mitochondrial Iron Uptake and Development of the Host via Interaction With ATP Synthase. Cell (2018) 175(2):571–82.e11. doi: 10.1016/j.cell.2018.07.032
93. Kirienko NV, Ausubel FM, Ruvkun G. Mitophagy Confers Resistance to Siderophore-Mediated Killing by Pseudomonas Aeruginosa. Proc Natl Acad Sci USA (2015) 112(6):1821–6. doi: 10.1073/pnas.1424954112
94. Kirienko NV, Kirienko DR, Larkins-Ford J, Wahlby C, Ruvkun G, Ausubel FM. Pseudomonas Aeruginosa Disrupts Caenorhabditis Elegans Iron Homeostasis, Causing a Hypoxic Response and Death. Cell Host Microbe (2013) 13(4):406–16. doi: 10.1016/j.chom.2013.03.003
95. Kratena N, Gökler T, Maltrovsky L, Oburger E, Stanetty C. A Unified Approach to Phytosiderophore Natural Products. Chemistry (2021) 27(2):577–80. doi: 10.1002/chem.202004004
96. Martínez-Pastor MT, Puig S. Adaptation to Iron Deficiency in Human Pathogenic Fungi. Biochim Biophys Acta Mol Cell Res (2020) 1867(10):118797. doi: 10.1016/j.bbamcr.2020.118797
97. Misslinger M, Hortschansky P, Brakhage AA, Haas H. Fungal Iron Homeostasis With a Focus on Aspergillus Fumigatus. Biochim Biophys Acta Mol Cell Res (2021) 1868(1):118885. doi: 10.1016/j.bbamcr.2020.118885
98. Ipcho S, Sundelin T, Erbs G, Kistler HC, Newman MA, Olsson S. Fungal Innate Immunity Induced by Bacterial Microbe-Associated Molecular Patterns (MAMPs). G3 (Bethesda) (2016) 6(6):1585–95. doi: 10.1534/g3.116.027987
99. Lamont IL, Beare PA, Ochsner U, Vasil AI, Vasil ML. Siderophore-Mediated Signaling Regulates Virulence Factor Production in Pseudomonasaeruginosa. Proc Natl Acad Sci USA (2002) 99(10):7072–7. doi: 10.1073/pnas.092016999
100. Naves P, del Prado G, Huelves L, Gracia M, Ruiz V, Blanco J, et al. Correlation Between Virulence Factors and In Vitro Biofilm Formation by Escherichia Coli Strains. Microb Pathog (2008) 45(2):86–91. doi: 10.1016/j.micpath.2008.03.003
101. Kang D, Kirienko NV. Interdependence Between Iron Acquisition and Biofilm Formation in Pseudomonas Aeruginosa. J Microbiol (2018) 56(7):449–57. doi: 10.1007/s12275-018-8114-3
102. Dietl AM, Binder U, Bauer I, Shadkchan Y, Osherov N, Haas H. Arginine Auxotrophy Affects Siderophore Biosynthesis and Attenuates Virulence of Aspergillus Fumigatus. Genes (Basel) (2020) 11(4):423. doi: 10.3390/genes11040423
103. Batista BB, Santos R, Ricci-Azevedo R, da Silva Neto JF. Production and Uptake of Distinct Endogenous Catecholate-Type Siderophores Are Required for Iron Acquisition and Virulence in Chromobacterium Violaceum. Infect Immun (2019) 87(12):e00577–19. doi: 10.1128/IAI.00577-19
104. Lam MMC, Wyres KL, Judd LM, Wick RR, Jenney A, Brisse S, et al. Tracking Key Virulence Loci Encoding Aerobactin and Salmochelin Siderophore Synthesis in Klebsiella Pneumoniae. Genome Med (2018) 10(1):77. doi: 10.1186/s13073-018-0587-5
105. Wilderman PJ, Vasil AI, Johnson Z, Wilson MJ, Cunliffe HE, Lamont IL, et al. Characterization of an Endoprotease (PrpL) Encoded by a PvdS-Regulated Gene in Pseudomonas Aeruginosa. Infect Immun (2001) 69(9):5385–94. doi: 10.1128/IAI.69.9.5385-5394.2001
106. Chen YT, Chang HY, Lai YC, Pan CC, Tsai SF, Peng HL. Sequencing and Analysis of the Large Virulence Plasmid pLVPK of Klebsiella Pneumoniae CG43. Gene (2004) 337:189–98. doi: 10.1016/j.gene.2004.05.008
107. Shirley M, Lamont IL. Role of TonB1 in Pyoverdine-Mediated Signaling in Pseudomonas Aeruginosa. J Bacteriol (2009) 191(18):5634–40. doi: 10.1128/JB.00742-09
108. Beare PA, For RJ, Martin LW, Lamont IL. Siderophore-Mediated Cell Signalling in Pseudomonas Aeruginosa: Divergent Pathways Regulate Virulence Factor Production and Siderophore Receptor Synthesis. Mol Microbiol (2003) 47(1):195–207. doi: 10.1046/j.1365-2958.2003.03288.x
109. Llamas MA, Sparrius M, Kloet R, Jiménez CR, Vandenbroucke-Grauls C, Bitter W. The Heterologous Siderophores Ferrioxamine B and Ferrichrome Activate Signaling Pathways in Pseudomonas Aeruginosa. J Bacteriol (2006) 188(5):1882–91. doi: 10.1128/JB.188.5.1882-1891.2006
110. Welz D, Braun V. Ferric Citrate Transport of Escherichia Coli: Functional Regions of the FecR Transmembrane Regulatory Protein. J Bacteriol (1998) 180(9):2387–94. doi: 10.1128/JB.180.9.2387-2394.1998
111. Braun V, Braun M. Iron Transport and Signaling in Escherichia Coli. FEBS Lett (2002) 529(1):78–85. doi: 10.1016/S0014-5793(02)03185-X
113. Rosa L, Cutone A, Lepanto MS, Paesano R, Valenti P. Lactoferrin: A Natural Glycoprotein Involved in Iron and Inflammatory Homeostasis. Int J Mol Sci (2017) 18(9):1985. doi: 10.3390/ijms18091985
114. Adler C, Corbalan NS, Peralta DR, Pomares MF, de Cristóbal RE, Vincent PA. The Alternative Role of Enterobactin as an Oxidative Stress Protector Allows Escherichia Coli Colony Development. PloS One (2014) 9(1):e84734. doi: 10.1371/journal.pone.0084734
115. Zhang W, Zhang Y, Wang X, Ding F, Fu Y, Zhao J, et al. Siderophores in Clinical Isolates of Klebsiella Pneumoniae Promote Ciprofloxacin Resistance by Inhibiting the Oxidative Stress. Biochem Biophys Res Commun (2017) 491(3):855–61. doi: 10.1016/j.bbrc.2017.04.108
116. Peralta DR, Adler C, Corbalán NS, Paz García EC, Pomares MF, Vincent PA. Enterobactin as Part of the Oxidative Stress Response Repertoire. PloS One (2016) 11(6):e0157799. doi: 10.1371/journal.pone.0157799
117. Adler C, Corbalán NS, Seyedsayamdost MR, Pomares MF, de Cristóbal RE, Clardy J, et al. Catecholate Siderophores Protect Bacteria From Pyochelin Toxicity. PloS One (2012) 7(10):e46754. doi: 10.1371/journal.pone.0046754
118. Swayambhu G, Bruno M, Gulick AM, Pfeifer BA. Siderophore Natural Products as Pharmaceutical Agents. Curr Opin Biotechnol (2021) 69:242–51. doi: 10.1016/j.copbio.2021.01.021
119. Braud A, Hannauer M, Mislin GL, Schalk IJ. The Pseudomonas Aeruginosa Pyochelin-Iron Uptake Pathway and its Metal Specificity. J Bacteriol (2009) 191(11):3517–25. doi: 10.1128/JB.00010-09
120. Braud A, Hoegy F, Jezequel K, Lebeau T, Schalk IJ. New Insights Into the Metal Specificity of the Pseudomonas Aeruginosa Pyoverdine-Iron Uptake Pathway. Environ Microbiol (2009) 11(5):1079–91. doi: 10.1111/j.1462-2920.2008.01838.x
121. Wichard T, Bellenger JP, Loison A, Kraepiel AM. Catechol Siderophores Control Tungsten Uptake and Toxicity in the Nitrogen-Fixing Bacterium Azotobacter Vinelandii. Environ Sci Technol (2008) 42(7):2408–13. doi: 10.1021/es702651f
122. Teitzel GM, Geddie A, De Long SK, Kirisits MJ, Whiteley M, Parsek MR. Survival and Growth in the Presence of Elevated Copper: Transcriptional Profiling of Copper-Stressed Pseudomonas Aeruginosa. J Bacteriol (2006) 188(20):7242–56. doi: 10.1128/JB.00837-06
123. McRose DL, Baars O, Morel FMM, Kraepiel AML. Siderophore Production in Azotobacter Vinelandii in Response to Fe-, Mo- and V-Limitation. Environ Microbiol (2017) 19(9):3595–605. doi: 10.1111/1462-2920.13857
124. Chaturvedi KS, Hung CS, Crowley JR, Stapleton AE, Henderson JP. The Siderophore Yersiniabactin Binds Copper to Protect Pathogens During Infection. Nat Chem Biol (2012) 8(8):731–6. doi: 10.1038/nchembio.1020
125. Emery T. Exchange of Iron by Gallium in Siderophores. Biochemistry (1986) 25(16):4629–33. doi: 10.1021/bi00364a026
126. Harris AW, Sephton RG. Transferrin Promotion of 67Ga and 59Fe Uptake by Cultured Mouse Myeloma Cells. Cancer Res (1977) 37(10):3634–8.
127. Bernstein LR. Mechanisms of Therapeutic Activity for Gallium. Pharmacol Rev (1998) 50(4):665–82.
128. Weiner RE, Schreiber GJ, Hoffer PB. In Vitro Transfer of Ga-67 From Transferrin to Ferritin. J Nucl Med (1983) 24(7):608–14.
129. Sephton RG, Kraft N. 67Ga and 59Fe Uptakes by Cultured Human Lymphoblasts and Lymphocytes. Cancer Res (1978) 38(5):1213–6.
130. Logan KJ, Ng PK, Turner CJ, Schmidt RP, Terner UK, Scott JR, et al. Comparative Pharmacokinetics of Ga-67 and Fe-59 in Humans. Int J Nucl Med Biol (1981) 8(4):271–6. doi: 10.1016/0047-0740(81)90033-4
131. Frangipani E, Bonchi C, Minandri F, Imperi F, Visca P. Pyochelin Potentiates the Inhibitory Activity of Gallium on Pseudomonas Aeruginosa. Antimicrob Agents Chemother (2014) 58(9):5572–5. doi: 10.1128/AAC.03154-14
132. Olakanmi O, Britigan BE, Schlesinger LS. Gallium Disrupts Iron Metabolism of Mycobacteria Residing Within Human Macrophages. Infect Immun (2000) 68(10):5619–27. doi: 10.1128/IAI.68.10.5619-5627.2000
133. Kaneko Y, Thoendel M, Olakanmi O, Britigan BE, Singh PK. The Transition Metal Gallium Disrupts Pseudomonas Aeruginosa Iron Metabolism and has Antimicrobial and Antibiofilm Activity. J Clin Invest (2007) 117(4):877–88. doi: 10.1172/JCI30783
134. Davies NP, Suryo Rahmanto Y, Chitambar CR, Richardson DR. Resistance to the Antineoplastic Agent Gallium Nitrate Results in Marked Alterations in Intracellular Iron and Gallium Trafficking: Identification of Novel Intermediates. J Pharmacol Exp Ther (2006) 317(1):153–62. doi: 10.1124/jpet.105.099044
135. Banin E, Lozinski A, Brady KM, Berenshtein E, Butterfield PW, Moshe M, et al. The Potential of Desferrioxamine-Gallium as an Anti-Pseudomonas Therapeutic Agent. Proc Natl Acad Sci USA (2008) 105(43):16761–6. doi: 10.1073/pnas.0808608105
136. Rzhepishevska O, Ekstrand-Hammarstrom B, Popp M, Bjorn E, Bucht A, Sjostedt A, et al. The Antibacterial Activity of Ga3+ Is Influenced by Ligand Complexation as Well as the Bacterial Carbon Source. Antimicrob Agents Chemother (2011) 55(12):5568–80. doi: 10.1128/AAC.00386-11
137. Braun V, Pramanik A, Gwinner T, Köberle M, Bohn E. Sideromycins: Tools and Antibiotics. Biometals (2009) 22(1):3–13. doi: 10.1007/s10534-008-9199-7
138. Trottmann F, Franke J, Ishida K, García-Altares M, Hertweck C. A Pair of Bacterial Siderophores Releases and Traps an Intercellular Signal Molecule: An Unusual Case of Natural Nitrone Bioconjugation. Angew Chem Int Ed Engl (2019) 58(1):200–4. doi: 10.1002/anie.201811131
139. Tomaras AP, Crandon JL, McPherson CJ, Banevicius MA, Finegan SM, Irvine RL, et al. Adaptation-Based Resistance to Siderophore-Conjugated Antibacterial Agents by Pseudomonas Aeruginosa. Antimicrob Agents Chemother (2013) 57(9):4197–207. doi: 10.1128/AAC.00629-13
140. Kim A, Kutschke A, Ehmann DE, Patey SA, Crandon JL, Gorseth E, et al. Pharmacodynamic Profiling of a Siderophore-Conjugated Monocarbam in Pseudomonas Aeruginosa: Assessing the Risk for Resistance and Attenuated Efficacy. Antimicrob Agents Chemother (2015) 59(12):7743–52. doi: 10.1128/AAC.00831-15
141. Zughaier SM, Kandler JL, Shafer WM. Neisseria Gonorrhoeae Modulates Iron-Limiting Innate Immune Defenses in Macrophages. PloS One (2014) 9(1):e87688. doi: 10.1371/journal.pone.0087688
142. Kochan I, Wasynczuk J, McCabe MA. Effects of Injected Iron and Siderophores on Infections in Normal and Immune Mice. Infect Immun (1978) 22(2):560–7. doi: 10.1128/iai.22.2.560-567.1978
143. Chao A, Sieminski PJ, Owens CP, Goulding CW. Iron Acquisition in Mycobacterium Tuberculosis. Chem Rev (2019) 119(2):1193–220. doi: 10.1021/acs.chemrev.8b00285
144. Ellermann M, Arthur JC. Siderophore-Mediated Iron Acquisition and Modulation of Host-Bacterial Interactions. Free Radic Biol Med (2017) 105:68–78. doi: 10.1016/j.freeradbiomed.2016.10.489
145. Jurado RL. Iron, Infections, and Anemia of Inflammation. Clin Infect Dis (1997) 25(4):888–95. doi: 10.1086/515549
146. Pieracci FM, Barie PS. Iron and the Risk of Infection. Surg Infect (Larchmt) (2005) 6 Suppl 1:S41–6. doi: 10.1089/sur.2005.6.s1-41
147. Goetz DH, Holmes MA, Borregaard N, Bluhm ME, Raymond KN, Strong RK. The Neutrophil Lipocalin NGAL Is a Bacteriostatic Agent That Interferes With Siderophore-Mediated Iron Acquisition. Mol Cell (2002) 10(5):1033–43. doi: 10.1016/S1097-2765(02)00708-6
148. Bachman MA, Miller VL, Weiser JN. Mucosal Lipocalin 2 has Pro-Inflammatory and Iron-Sequestering Effects in Response to Bacterial Enterobactin. PloS Pathog (2009) 5(10):e1000622. doi: 10.1371/journal.ppat.1000622
149. Borregaard N, Cowland JB. Neutrophil Gelatinase-Associated Lipocalin, a Siderophore-Binding Eukaryotic Protein. Biometals (2006) 19(2):211–5. doi: 10.1007/s10534-005-3251-7
150. Jaberi SA, Cohen A, D'Souza C, Abdulrazzaq YM, Ojha S, Bastaki S, et al. Lipocalin-2: Structure, Function, Distribution and Role in Metabolic Disorders. BioMed Pharmacother (2021) 142:112002. doi: 10.1016/j.biopha.2021.112002
151. Krizanac M, Mass Sanchez PB, Weiskirchen R, Asimakopoulos A. A Scoping Review on Lipocalin-2 and Its Role in Non-Alcoholic Steatohepatitis and Hepatocellular Carcinoma. Int J Mol Sci (2021) 22(6). doi: 10.3390/ijms22062865
152. Santiago-Sanchez GS, Pita-Grisanti V, Quinones-Diaz B, Gumpper K, Cruz-Monserrate Z, Vivas-Mejia PE. Biological Functions and Therapeutic Potential of Lipocalin 2 in Cancer. Int J Mol Sci (2020) 21(12). doi: 10.3390/ijms21124365
153. Rahimi S, Roushandeh AM, Ahmadzadeh E, Jahanian-Najafabadi A, Roudkenar MH. Implication and Role of Neutrophil Gelatinase-Associated Lipocalin in Cancer: Lipocalin-2 as a Potential Novel Emerging Comprehensive Therapeutic Target for a Variety of Cancer Types. Mol Biol Rep (2020) 47(3):2327–46. doi: 10.1007/s11033-020-05261-5
154. Gumpper K, Dangel AW, Pita-Grisanti V, Krishna SG, Lara LF, Mace T, et al. Lipocalin-2 Expression and Function in Pancreatic Diseases. Pancreatology (2020) 20(3):419–24. doi: 10.1016/j.pan.2020.01.002
155. Jung M, Mertens C, Tomat E, Brune B. Iron as a Central Player and Promising Target in Cancer Progression. Int J Mol Sci (2019) 20(2). doi: 10.3390/ijms20020273
156. Saha P, Yeoh BS, Olvera RA, Xiao X, Singh V, Awasthi D, et al. Bacterial Siderophores Hijack Neutrophil Functions. J Immunol (2017) 198(11):4293–303. doi: 10.4049/jimmunol.1700261
157. Singh V, Yeoh BS, Xiao X, Kumar M, Bachman M, Borregaard N, et al. Interplay Between Enterobactin, Myeloperoxidase and Lipocalin 2 Regulates E. Coli Survival in the Inflamed Gut. Nat Commun (2015) 6:7113. doi: 10.1038/ncomms8113
158. Clifton MC, Corrent C, Strong RK. Siderocalins: Siderophore-Binding Proteins of the Innate Immune System. Biometals (2009) 22(4):557–64. doi: 10.1007/s10534-009-9207-6
159. Zsila F, Beke-Somfai T. Human Host-Defense Peptide LL-37 Targets Stealth Siderophores. Biochem Biophys Res Commun (2020) 526(3):780–5. doi: 10.1016/j.bbrc.2020.03.162
160. Choi EY, Kim EC, Oh HM, Kim S, Lee HJ, Cho EY, et al. Iron Chelator Triggers Inflammatory Signals in Human Intestinal Epithelial Cells: Involvement of P38 and Extracellular Signal-Regulated Kinase Signaling Pathways. J Immunol (2004) 172(11):7069–77. doi: 10.4049/jimmunol.172.11.7069
161. Holden VI, Lenio S, Kuick R, Ramakrishnan SK, Shah YM, Bachman MA. Bacterial Siderophores That Evade or Overwhelm Lipocalin 2 Induce Hypoxia Inducible Factor 1α and Proinflammatory Cytokine Secretion in Cultured Respiratory Epithelial Cells. Infect Immun (2014) 82(9):3826–36. doi: 10.1128/IAI.01849-14
162. Monteith AJ, Skaar EP. The Impact of Metal Availability on Immune Function During Infection. Trends Endocrinol Metab (2021) 32(11):916–28. doi: 10.1016/j.tem.2021.08.004
163. Sun H, Zhang C, Cao S, Sheng T, Dong N, Xu Y. Fenton Reactions Drive Nucleotide and ATP Syntheses in Cancer. J Mol Cell Biol (2018) 10(5):448–59. doi: 10.1093/jmcb/mjy039
164. Vander Heiden MG, DeBerardinis RJ. Understanding the Intersections Between Metabolism and Cancer Biology. Cell (2017) 168(4):657–69. doi: 10.1016/j.cell.2016.12.039
165. El-Serag HB. Hepatocellular Carcinoma. N Engl J Med (2011) 365(12):1118–27. doi: 10.1056/NEJMra1001683
166. Ko C, Siddaiah N, Berger J, Gish R, Brandhagen D, Sterling RK, et al. Prevalence of Hepatic Iron Overload and Association With Hepatocellular Cancer in End-Stage Liver Disease: Results From the National Hemochromatosis Transplant Registry. Liver Int (2007) 27(10):1394–401. doi: 10.1111/j.1478-3231.2007.01596.x
167. Muto Y, Moroishi T, Ichihara K, Nishiyama M, Shimizu H, Eguchi H, et al. Disruption of FBXL5-Mediated Cellular Iron Homeostasis Promotes Liver Carcinogenesis. J Exp Med (2019) 216(4):950–65. doi: 10.1084/jem.20180900
168. Ba Q, Hao M, Huang H, Hou J, Ge S, Zhang Z, et al. Iron Deprivation Suppresses Hepatocellular Carcinoma Growth in Experimental Studies. Clin Cancer Res (2011) 17(24):7625–33. doi: 10.1158/1078-0432.CCR-10-3099
169. Hussain SP, Raja K, Amstad PA, Sawyer M, Trudel LJ, Wogan GN, et al. Increased P53 Mutation Load in Nontumorous Human Liver of Wilson Disease and Hemochromatosis: Oxyradical Overload Diseases. Proc Natl Acad Sci USA (2000) 97(23):12770–5. doi: 10.1073/pnas.220416097
170. Marrogi AJ, Khan MA, van Gijssel HE, Welsh JA, Rahim H, Demetris AJ, et al. Oxidative Stress and P53 Mutations in the Carcinogenesis of Iron Overload-Associated Hepatocellular Carcinoma. J Natl Cancer Inst (2001) 93(21):1652–5. doi: 10.1093/jnci/93.21.1652
171. Shen Y, Li X, Dong D, Zhang B, Xue Y, Shang P. Transferrin Receptor 1 in Cancer: A New Sight for Cancer Therapy. Am J Cancer Res (2018) 8(6):916–31.
172. Weinstein RE, Bond BH, Silberberg BK, Vaughn CB, Subbaiah P, Pieper DR. Tissue Ferritin Concentration and Prognosis in Carcinoma of the Breast. Breast Cancer Res Treat (1989) 14(3):349–53. doi: 10.1007/BF01806307
173. Canonne-Hergaux F, Zhang AS, Ponka P, Gros P. Characterization of the Iron Transporter DMT1 (NRAMP2/DCT1) in Red Blood Cells of Normal and Anemic Mk/Mk Mice. Blood (2001) 98(13):3823–30. doi: 10.1182/blood.V98.13.3823
174. Brookes MJ, Hughes S, Turner FE, Reynolds G, Sharma N, Ismail T, et al. Modulation of Iron Transport Proteins in Human Colorectal Carcinogenesis. Gut (2006) 55(10):1449–60. doi: 10.1136/gut.2006.094060
175. Basuli D, Tesfay L, Deng Z, Paul B, Yamamoto Y, Ning G, et al. Iron Addiction: A Novel Therapeutic Target in Ovarian Cancer. Oncogene (2017) 36(29):4089–99. doi: 10.1038/onc.2017.11
176. Jiang XP, Elliott RL, Head JF. Manipulation of Iron Transporter Genes Results in the Suppression of Human and Mouse Mammary Adenocarcinomas. Anticancer Res (2010) 30(3):759–65.
177. Choi JY, Neuhouser ML, Barnett MJ, Hong CC, Kristal AR, Thornquist MD, et al. Iron Intake, Oxidative Stress-Related Genes (MnSOD and MPO) and Prostate Cancer Risk in CARET Cohort. Carcinogenesis (2008) 29(5):964–70. doi: 10.1093/carcin/bgn056
178. Richardson A, Kovacevic Z, Richardson DR. Iron Chelation: Inhibition of Key Signaling Pathways in the Induction of the Epithelial Mesenchymal Transition in Pancreatic Cancer and Other Tumors. Crit Rev Oncog (2013) 18(5):409–34. doi: 10.1615/CritRevOncog.2013007921
179. Xiong W, Wang L, Yu F. Regulation of Cellular Iron Metabolism and its Implications in Lung Cancer Progression. Med Oncol (2014) 31(7):28. doi: 10.1007/s12032-014-0028-2
180. Torti SV, Torti FM, Whitman SP, Brechbiel MW, Park G, Planalp RP. Tumor Cell Cytotoxicity of a Novel Metal Chelator. Blood (1998) 92(4):1384–9. doi: 10.1182/blood.V92.4.1384
181. Song S, Christova T, Perusini S, Alizadeh S, Bao RY, Miller BW, et al. Wnt Inhibitor Screen Reveals Iron Dependence of Beta-Catenin Signaling in Cancers. Cancer Res (2011) 71(24):7628–39. doi: 10.1158/0008-5472.CAN-11-2745
182. Franke GN, Kubasch AS, Cross M, Vucinic V, Platzbecker U. Iron Overload and its Impact on Outcome of Patients With Hematological Diseases. Mol Aspects Med (2020) 75:100868. doi: 10.1016/j.mam.2020.100868
183. Krebs NF, Sherlock LG, Westcott J, Culbertson D, Hambidge KM, Feazel LM, et al. Effects of Different Complementary Feeding Regimens on Iron Status and Enteric Microbiota in Breastfed Infants. J Pediatr (2013) 163(2):416–23. doi: 10.1016/j.jpeds.2013.01.024
184. Seril DN, Liao J, Ho KL, Warsi A, Yang CS, Yang GY. Dietary Iron Supplementation Enhances DSS-Induced Colitis and Associated Colorectal Carcinoma Development in Mice. Dig Dis Sci (2002) 47(6):1266–78. doi: 10.1023/A:1015362228659
185. Carrier JC, Aghdassi E, Jeejeebhoy K, Allard JP. Exacerbation of Dextran Sulfate Sodium-Induced Colitis by Dietary Iron Supplementation: Role of NF-Kappab. Int J Colorectal Dis (2006) 21(4):381–7. doi: 10.1007/s00384-005-0011-7
186. Lee T, Clavel T, Smirnov K, Schmidt A, Lagkouvardos I, Walker A, et al. Oral Versus Intravenous Iron Replacement Therapy Distinctly Alters the Gut Microbiota and Metabolome in Patients With IBD. Gut (2017) 66(5):863–71. doi: 10.1136/gutjnl-2015-309940
187. Jaeggi T, Kortman GA, Moretti D, Chassard C, Holding P, Dostal A, et al. Iron Fortification Adversely Affects the Gut Microbiome, Increases Pathogen Abundance and Induces Intestinal Inflammation in Kenyan Infants. Gut (2015) 64(5):731–42. doi: 10.1136/gutjnl-2014-307720
188. Lievin V, Peiffer I, Hudault S, Rochat F, Brassart D, Neeser JR, et al. Bifidobacterium Strains From Resident Infant Human Gastrointestinal Microflora Exert Antimicrobial Activity. Gut (2000) 47(5):646–52. doi: 10.1136/gut.47.5.646
189. Coconnier MH, Lievin V, Bernet-Camard MF, Hudault S, Servin AL. Antibacterial Effect of the Adhering Human Lactobacillus Acidophilus Strain LB. Antimicrob Agents Chemother (1997) 41(5):1046–52. doi: 10.1128/AAC.41.5.1046
190. Ibrahim O, O'Sullivan J. Iron Chelators in Cancer Therapy. Biometals (2020) 33(4-5):201–15. doi: 10.1007/s10534-020-00243-3
191. Harima H, Kaino S, Takami T, Shinoda S, Matsumoto T, Fujisawa K, et al. Deferasirox, a Novel Oral Iron Chelator, Shows Antiproliferative Activity Against Pancreatic Cancer In Vitro and In Vivo. BMC Cancer (2016) 16:702. doi: 10.1186/s12885-016-2744-9
192. Tury S, Assayag F, Bonin F, Chateau-Joubert S, Servely JL, Vacher S, et al. The Iron Chelator Deferasirox Synergises With Chemotherapy to Treat Triple-Negative Breast Cancers. J Pathol (2018) 246(1):103–14. doi: 10.1002/path.5104
193. Leftin A, Zhao H, Turkekul M, de Stanchina E, Manova K, Koutcher JA. Iron Deposition is Associated With Differential Macrophage Infiltration and Therapeutic Response to Iron Chelation in Prostate Cancer. Sci Rep (2017) 7(1):11632. doi: 10.1038/s41598-017-11899-2
194. Cameron SJS, Lewis KE, Huws SA, Hegarty MJ, Lewis PD, Pachebat JA, et al. A Pilot Study Using Metagenomic Sequencing of the Sputum Microbiome Suggests Potential Bacterial Biomarkers for Lung Cancer. PloS One (2017) 12(5):e0177062. doi: 10.1371/journal.pone.0177062
195. Gobin J, Wong DK, Gibson BW, Horwitz MA. Characterization of Exochelins of the Mycobacterium Bovis Type Strain and BCG Substrains. Infect Immun (1999) 67(4):2035–9. doi: 10.1128/IAI.67.4.2035-2039.1999
196. Schupp T, Toupet C, Divers M. Cloning and Expression of Two Genes of Streptomyces Pilosus Involved in the Biosynthesis of the Siderophore Desferrioxamine B. Gene (1988) 64(2):179–88. doi: 10.1016/0378-1119(88)90333-2
197. Blondeau R, Imbert M, Béchet M. Comparison of the Main Siderophores Produced by Some Species of Streptomyces. Curr Microbiol (1995) 31:129–33. doi: 10.1007/BF00294289
198. Maggio A. Light and Shadows in the Iron Chelation Treatment of Haematological Diseases. Br J Haematol (2007) 138(4):407–21. doi: 10.1111/j.1365-2141.2007.06666.x
199. Leitch HA. Improving Clinical Outcome in Patients With Myelodysplastic Syndrome and Iron Overload Using Iron Chelation Therapy. Leuk Res (2007) 31 Suppl 3:S7–9. doi: 10.1016/S0145-2126(07)70460-5
200. Lee KH, Choi E, Chun YS, Kim MS, Park JW. Differential Responses of Two Degradation Domains of HIF-1alpha to Hypoxia and Iron Deficiency. Biochimie (2006) 88(2):163–9. doi: 10.1016/j.biochi.2005.07.011
201. Richardson D, Ponka P, Baker E. The Effect of the Iron(III) Chelator, Desferrioxamine, on Iron and Transferrin Uptake by the Human Malignant Melanoma Cell. Cancer Res (1994) 54(3):685–9.
202. Kalinowski DS, Richardson DR. The Evolution of Iron Chelators for the Treatment of Iron Overload Disease and Cancer. Pharmacol Rev (2005) 57(4):547–83. doi: 10.1124/pr.57.4.2
203. Keberle H. The Biochemistry of Desferrioxamine and Its Relation to Iron Metabolism. Ann N Y Acad Sci (1964) 119:758–68. doi: 10.1111/j.1749-6632.1965.tb54077.x
204. Hershko C, Hershkov A, Konijn A, Breuer W, Cabantchik I Z, Pootrakul P, et al. Objectives and Methods of Iron Chelation Therapy. Bioinorg Chem Appl (2003). 1(2):151–68. doi: 10.1155/S1565363303000128
205. Olivieri NF, Brittenham GM. Iron-Chelating Therapy and the Treatment of Thalassemia. Blood (1997) 89(3):739–61. doi: 10.1182/blood.V89.3.739
206. Kontoghiorghes GJ. Comparative Efficacy and Toxicity of Desferrioxamine, Deferiprone and Other Iron and Aluminium Chelating Drugs. Toxicol Lett (1995) 80(1-3):1–18. doi: 10.1016/0378-4274(95)03415-H
208. Fischbach MA, Lin H, Liu DR, Walsh CT. How Pathogenic Bacteria Evade Mammalian Sabotage in the Battle for Iron. Nat Chem Biol (2006) 2(3):132–8. doi: 10.1038/nchembio771
209. McClure JJ, Li X, Chou CJ. Advances and Challenges of HDAC Inhibitors in Cancer Therapeutics. Adv Cancer Res (2018) 138:183–211. doi: 10.1016/bs.acr.2018.02.006
210. Nishino N, Yoshikawa D, Watanabe LA, Kato T, Jose B, Komatsu Y, et al. Synthesis and Histone Deacetylase Inhibitory Activity of Cyclic Tetrapeptides Containing a Retrohydroxamate as Zinc Ligand. Bioorg Med Chem Lett (2004) 14(10):2427–31. doi: 10.1016/j.bmcl.2004.03.018
211. Yu Y, Wong J, Lovejoy DB, Kalinowski DS, Richardson DR. Chelators at the Cancer Coalface: Desferrioxamine to Triapine and Beyond. Clin Cancer Res (2006) 12(23):6876–83. doi: 10.1158/1078-0432.CCR-06-1954
212. Hatcher HC, Singh RN, Torti FM, Torti SV. Synthetic and Natural Iron Chelators: Therapeutic Potential and Clinical Use. Future Med Chem (2009) 1(9):1643–70. doi: 10.4155/fmc.09.121
213. Chitambar CR. Gallium Nitrate for the Treatment of non-Hodgkin's Lymphoma. Expert Opin Investig Drugs (2004) 13(5):531–41. doi: 10.1517/13543784.13.5.531
214. Seligman PA, Moran PL, Schleicher RB, Crawford ED. Treatment With Gallium Nitrate: Evidence for Interference With Iron Metabolism In Vivo. Am J Hematol (1992) 41(4):232–40. doi: 10.1002/ajh.2830410403
215. Chitambar CR, Purpi DP, Woodliff J, Yang M, Wereley JP. Development of Gallium Compounds for Treatment of Lymphoma: Gallium Maltolate, a Novel Hydroxypyrone Gallium Compound, Induces Apoptosis and Circumvents Lymphoma Cell Resistance to Gallium Nitrate. J Pharmacol Exp Ther (2007) 322(3):1228–36. doi: 10.1124/jpet.107.126342
216. Cunningham MJ, Nathan DG. New Developments in Iron Chelators. Curr Opin Hematol (2005) 12(2):129–34. doi: 10.1097/01.moh.0000152631.63469.07
217. Lui GY, Obeidy P, Ford SJ, Tselepis C, Sharp DM, Jansson PJ, et al. The Iron Chelator, Deferasirox, as a Novel Strategy for Cancer Treatment: Oral Activity Against Human Lung Tumor Xenografts and Molecular Mechanism of Action. Mol Pharmacol (2013) 83(1):179–90. doi: 10.1124/mol.112.081893
218. Miller MJ, Zhu H, Xu Y, Wu C, Walz A, Vergne A, et al. Utilization of Microbial Iron Assimilation Processes for the Development of New Antibiotics and Inspiration for the Design of New Anticancer Agents. Biometals: Int J Role Metal Ions Biol Biochem Med (2009) 22(1):61–75. doi: 10.1007/s10534-008-9185-0
219. Bao G, Clifton M, Hoette TM, Mori K, Deng SX, Qiu A, et al. Iron Traffics in Circulation Bound to a Siderocalin (Ngal)-Catechol Complex. Nat Chem Biol (2010) 6(8):602–9. doi: 10.1038/nchembio.402
220. Rehwald C, Schnetz M, Urbschat A, Mertens C, Meier JK, Bauer R, et al. The Iron Load of Lipocalin-2 (LCN-2) Defines Its Pro-Tumour Function in Clear-Cell Renal Cell Carcinoma. Br J Cancer (2020) 122(3):421–33. doi: 10.1038/s41416-019-0655-7
221. Nosrati R, Abnous K, Alibolandi M, Mosafer J, Dehghani S, Taghdisi SM, et al. Targeted SPION Siderophore Conjugate Loaded With Doxorubicin as a Theranostic Agent for Imaging and Treatment of Colon Carcinoma. Sci Rep (2021) 11(1):13065. doi: 10.1038/s41598-021-92391-w
222. Horm TM, Schroeder JA. MUC1 and Metastatic Cancer: Expression, Function and Therapeutic Targeting. Cell Adh Migr (2013) 7(2):187–98. doi: 10.4161/cam.23131
223. Feng X, Jiang S, Zhang F, Wang R, Zhao Y, Zeng M. Siderophore (From Synechococcus Sp. PCC 7002)-Chelated Iron Promotes Iron Uptake in Caco-2 Cells and Ameliorates Iron Deficiency in Rats. Mar Drugs (2019) 17(12). doi: 10.3390/md17120709
224. Chi Y, Remsik J, Kiseliovas V, Derderian C, Sener U, Alghader M, et al. Cancer Cells Deploy Lipocalin-2 to Collect Limiting Iron in Leptomeningeal Metastasis. Science (2020) 369(6501):276–82. doi: 10.1126/science.aaz2193
Keywords: microbiome, bacteria, siderophores, enterobactin, deferoxamine, cancer, tumor, iron
Citation: Pita-Grisanti V, Chasser K, Sobol T and Cruz-Monserrate Z (2022) Understanding the Potential and Risk of Bacterial Siderophores in Cancer. Front. Oncol. 12:867271. doi: 10.3389/fonc.2022.867271
Received: 10 February 2022; Accepted: 06 April 2022;
Published: 17 June 2022.
Edited by:
Wen Zhou, Case Western Reserve University, United StatesReviewed by:
Rolf Kümmerli, University of Zurich, SwitzerlandCopyright © 2022 Pita-Grisanti, Chasser, Sobol and Cruz-Monserrate. This is an open-access article distributed under the terms of the Creative Commons Attribution License (CC BY). The use, distribution or reproduction in other forums is permitted, provided the original author(s) and the copyright owner(s) are credited and that the original publication in this journal is cited, in accordance with accepted academic practice. No use, distribution or reproduction is permitted which does not comply with these terms.
*Correspondence: Zobeida Cruz-Monserrate, em9iZWlkYS5jcnV6LW1vbnNlcnJhdGVAb3N1bWMuZWR1
Disclaimer: All claims expressed in this article are solely those of the authors and do not necessarily represent those of their affiliated organizations, or those of the publisher, the editors and the reviewers. Any product that may be evaluated in this article or claim that may be made by its manufacturer is not guaranteed or endorsed by the publisher.
Research integrity at Frontiers
Learn more about the work of our research integrity team to safeguard the quality of each article we publish.