- 1Department of Cancer Biology, Wake Forest University School of Medicine, Winston-Salem, NC, United States
- 2Wake Forest Baptist Comprehensive Cancer Center, Wake Forest University School of Medicine, Winston-Salem, NC, United States
Breast cancer is the most commonly diagnosed cancer in women. Metastasis is the primary cause of mortality for breast cancer patients. Multiple mechanisms underlie breast cancer metastatic dissemination, including the interleukin-6 (IL-6)-mediated signaling pathway. IL-6 is a pleiotropic cytokine that plays an important role in multiple physiological processes including cell proliferation, immune surveillance, acute inflammation, metabolism, and bone remodeling. IL-6 binds to the IL-6 receptor (IL-6Rα) which subsequently binds to the glycoprotein 130 (gp130) receptor creating a signal transducing hexameric receptor complex. Janus kinases (JAKs) are recruited and activated; activated JAKs, in turn, phosphorylate signal transducer and activator of transcription 3 (STAT3) for activation, leading to gene regulation. Constitutively active IL-6/JAK/STAT3 signaling drives cancer cell proliferation and invasiveness while suppressing apoptosis, and STAT3 enhances IL-6 signaling to promote a vicious inflammatory loop. Aberrant expression of IL-6 occurs in multiple cancer types and is associated with poor clinical prognosis and metastasis. In breast cancer, the IL-6 pathway is frequently activated, which can promote breast cancer metastasis while simultaneously suppressing the anti-tumor immune response. Given these important roles in human cancers, multiple components of the IL-6 pathway are promising targets for cancer therapeutics and are currently being evaluated preclinically and clinically for breast cancer. This review covers the current biological understanding of the IL-6 signaling pathway and its impact on breast cancer metastasis, as well as, therapeutic interventions that target components of the IL-6 pathway including: IL-6, IL-6Rα, gp130 receptor, JAKs, and STAT3.
Introduction
Breast cancer affects 1 in 8 women in the United States and is the second leading cause of cancer-related deaths in women behind lung cancer (1). Breast cancers are diverse and often identified by molecular subtype via immunohistochemical (IHC) expression of prognostic markers: estrogen receptor (ER), progesterone receptor (PR), and the human epidermal growth factor receptor 2 (HER2). The molecular subtypes of breast cancer range in both receptor expression and prognoses: luminal A (ER and/or PR+/HER2-), luminal B (ER and/or PR+/HER+), HER2-enriched (ER-/PR-/HER2+), and triple negative (ER-/PR-/HER2-) (2). HER2-enriched breast cancer and triple-negative breast cancer (TNBC) are the most aggressive subtypes represented by a higher Ki67 staining, poorer patient survival, and the highest propensity to metastasize (3). Primary breast cancer patients have a 5-year survival rate of 99%; however, the development of metastases diminishes survival rates to 28% (1). 20-30% of breast cancer cases metastasize to distant organs, which accounts for 90% of breast cancer-related deaths (4). The most common sites of metastasis for breast cancer patients include the bone, lung, brain, and liver (5). The development of metastases is a complex process comprised of multiple steps including epithelial-mesenchymal transition (EMT), local invasion, migration, intravasation, extravasation, mesenchymal-epithelial transition, and colonization to a distant organ. During many of these steps, breast cancer cells secrete small soluble proteins, such as cytokines, to promote cancer cells (autocrine effect) and prime microenvironmental cells (paracrine effect) (6). Cytokines are secreted, pleiotropic proteins (15-20 kDa) that mediate a myriad of immunological and inflammatory responses that are often hijacked in cancer. Many cytokines can exhibit either pro- or anti-cancer properties, including interleukin-6 (IL-6). Under homeostatic conditions, IL-6 plays fundamental roles in immune response, inflammation, hematopoiesis, and bone homeostasis; however, dysregulation of IL-6 promotes the pathogenesis of multiple inflammatory and immune-mediated diseases, as well as, cancer (7, 8). The IL-6 signaling pathway is one of the most dysregulated pathways in cancer. For example, IL-6 is elevated in sera of ovarian, cervical, colorectal, esophageal, head-and-neck, pancreatic, prostate, liver, lung, gastric, and breast cancer patients (9–24). In breast cancer, IL-6 expression correlates with poor patient survival, promotes growth and invasion, and mediates metastatic progression, which identifies the IL-6 signaling axis as a potential therapeutic target (25, 26). Consequently, many IL-6-pathway-targeted therapies have been developed and evaluated for breast cancer. Herein, we summarize the biology of the IL-6 signaling pathway, its roles in breast cancer metastasis, and therapeutic advancements in targeting the IL-6/JAK/STAT3 signaling axis.
IL-6 Signaling
IL-6 was first identified as a 26 kDa T cell-secreted factor that stimulates B cells for antibody production. Since the cloning of IL-6 cDNA by the Hirano and Kishimoto group in 1986 (27, 28), it became evident that IL-6 function was not limited to the immune system as the cDNA sequence maintained homology to other identified proteins: B cell stimulatory factor-2, Hepatocyte-stimulating factor, Hybridoma plasmacytoma growth factor, and interferon β2 (28–32). IL-6 is a member of the IL-6 cytokine family, a four-α helical bundle cytokine, and is secreted by both immune and non-immune cells. The IL-6 cytokine family encompasses eight cytokines, namely, IL-6, IL-11, ciliary neurotrophic factor (CNTF), leukemia inhibitory factor (LIF), oncostatin M (OSM), cardiotrophin 1 (CT-1), cardiotrophin-like cytokine (CLC), and IL-27. These cytokines bind to their respective receptors, but all utilize the signal-transducing co-receptor, glycoprotein 130 (gp130, CD130, IL-6Rβ, IL6ST, 130 kDa). More recently, two additional cytokines were added to the IL-6 cytokine family, IL-35 and IL-39, which utilize gp130 for signal transduction (33, 34). Specifically, IL-6 requires both interleukin-6 receptor α (IL-6Rα, CD80, 80 kDa) and gp130 to activate the downstream pathways via classic signaling, trans-signaling, or trans-presentation (35–37).
Classic Signaling
Classic IL-6 signaling is mediated strictly through membrane-bound receptors, IL-6Rα and gp130 (Figure 1, left) (38). IL-6 first binds to IL-6Rα on the cell surface which creates a high affinity for transmembrane gp130. Two trimeric receptor complexes (IL-6/IL-6Rα/gp130) homodimerize; IL-6 of one trimeric complex binds to the D1 domain of gp130 of the second trimeric complex, forming a signal transducing hexameric receptor complex (39). The IL-6/IL-6Rα/gp130 receptor complex activates mitogen activated protein kinase (MAPK), phosphatidylinositide-3-kinase (PI3K), Janus kinases (JAKs), and signal transducer and activator of transcription (STATs) signaling cascades. Formation of the IL-6/IL-6Rα/gp130 hexameric complex recruits the JAK family of non-receptor tyrosine kinases (JAK1, JAK2, and TYK2) to the membrane which associate with and phosphorylate the cytoplasmic tail of gp130 at five tyrosine residues (Y759, Y767, Y814, Y905, and Y915) (40). Phosphorylated gp130 serves as a docking site for STAT1 and STAT3 transcription factors that are subsequently phosphorylated by JAKs at Y701 and Y705, respectively (41, 42). Notably, IL-6 activates STAT3 more potently when compared to STAT1 (43). Upon phosphorylation, STAT3 undergoes a conformational change, detaches from the receptor complex, and homodimerizes allowing for STAT3 translocation into the nucleus to promote transcriptional activation (44). STAT3 is negatively regulated by tyrosine phosphatases, disruption of JAKs and/or cytokine receptors by suppressors of cytokine signaling (SOCS), or direct protein inhibitors of activated STATs (PIAS) (45–47). Receptor availability can also become a limiting factor for IL-6 signaling since IL-6 must be complexed with IL-6Rα in order to bind to gp130 receptor for signal transduction (38). Interestingly, transmembrane gp130 expression is ubiquitously expressed on most cell types; however, expression of membrane-bound IL-6Rα is restricted, therefore, limiting classic signaling to a small subset of cells (48, 49). Since IL-6 modulates pleiotropic effects beyond immune cells, it quickly became evident that IL-6 signals via alternative mechanisms outside of membrane-bound receptors, termed trans-signaling.
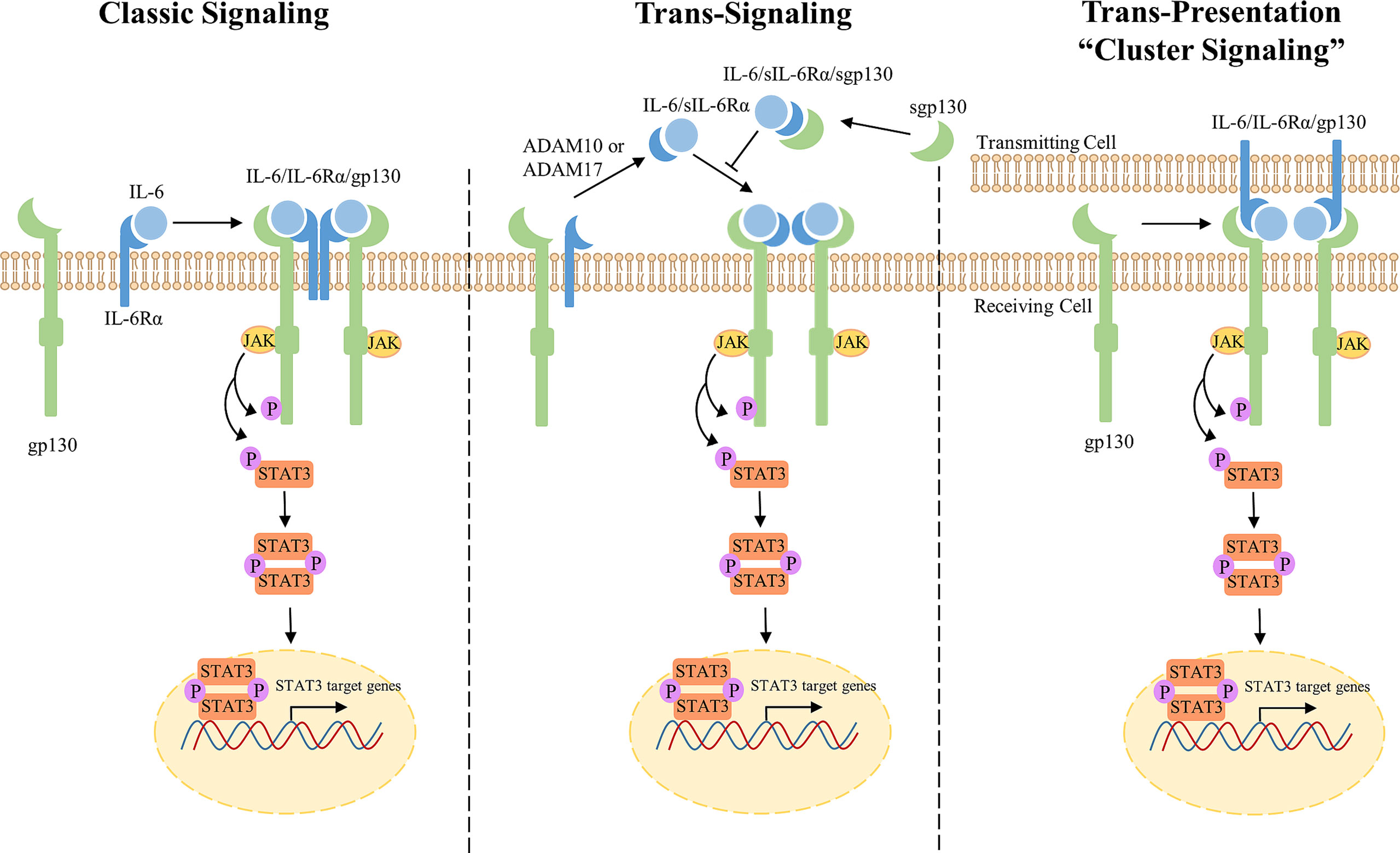
Figure 1 Overview of IL-6/JAK/STAT3 Classic, Trans-signaling, and Trans-presentation. Classic Signaling (left) occurs when IL-6 binds membrane-bound IL-6Rα leading to the subsequent formation of a trimeric receptor complex with signal-transducing subunit, gp130. Two trimeric IL-6/IL-6Rα/gp130 complexes bind through the D1 domain of gp130 to form a hexameric receptor complex for intracellular signaling through the JAK/STAT3 pathway. JAKs are recruited to the membrane and phosphorylate the cytoplasmic tail of gp130 and STAT3. pSTAT3 homodimerizes and translocates into the nucleus for activation of transcription. Trans-signaling (middle) occurs when IL-6Rα presents in a soluble form through mRNA alternative splicing or proteolysis by ADAM10/17. IL-6 binds sIL-6Rα to form a hexameric receptor complex through membrane-bound gp130 for signal transduction. Sgp130 antagonizes IL-6 signaling through sequestration of IL-6/sIL-6Rα. Trans-presentation (right), or “cluster signaling,” occurs between two different cells. A gp130 receptor complex on a receiving cell responds to a IL-6/IL-6Rα complex on a transmitting cell to induce downstream STAT3 signaling.
Trans-Signaling
IL-6 trans-signaling is mediated through a soluble form of IL-6Rα (sIL-6Rα) to potentiate IL-6 signaling in cells lacking sufficient expression of membrane-bound IL-6Rα (Figure 1, middle) (38, 50). Originally detected in serum and urine samples, sIL-6Rα functions as an agonist for IL-6 signaling. sIL-6Rα is produced either by proteolytic cleavage of the membrane-bound IL-6Rα or alternative splicing of pre-mRNA (50–54). Membrane-bound IL-6Rα undergoes proteolysis, or shedding, by disintegrin and metalloproteinase domain-containing proteins ADAM10 or ADAM17 to form sIL-6Rα (50, 55–57). Secreted IL-6 binds to sIL-6Rα which binds transmembrane gp130. Subsequently, two trimeric receptor complexes homodimerize to activate downstream signaling. Interestingly, gp130 can also present in a soluble form (sgp130) and sequesters IL-6/sIL6Rα, thus antagonizing IL-6 trans-signaling without impacting IL-6 classic signaling. However, sgp130 levels are almost negligible when compared to sIL-6Rα (52, 58). Trans-signaling regulates the IL-6 immune response and mediates pro-inflammatory responses through recruitment of mononuclear cells, stimulation of endothelial cells, T-cell survival, and inhibition of regulatory T-cell differentiation (59, 60). Administration of IL-6 and sIL-6Rα activates STAT3 in endothelial cells, solely expressing membrane-bound gp130, to recruit leukocytes for local inflammation in vitro and in vivo (61). Since trans-signaling mediates the pro-inflammatory responses induced by IL-6, trans-signaling is referred to as the primary mechanism by which IL-6 signaling promotes tumorigenesis in multiple cancers (59, 62, 63). In cancer, IL-6 trans-signaling induces therapeutic resistance, angiogenesis, and is associated with poor clinical outcome (64).
Cluster Signaling
More recently, a third mechanism of IL-6 signaling has been reported where IL-6 signals between two interacting cells termed, trans-presentation or “cluster signaling” (37). Originally discovered in 2017, Heink et al. discovered IL-6 binds membrane-bound IL-6Rα on one cell (transmitting cell) and is able to bind a gp130 receptor on another cell type (receiving cell) for signal transduction (Figure 1, right) (37). Co-culture experiments identified that gp130 receptors on T cells responded to IL-6/IL-6Rα complexes on the membrane of dendritic cells resulting in robust activation of STAT3. Functionally, IL-6 cluster signaling allowed dendritic cells to prime pathogenic T helper 17 cells. Since sgp130 is known to antagonize IL-6 trans-signaling, but not classic signaling, Heink et al. also investigated whether sgp130 is able to neutralize IL-6 cluster signaling. Although sgp130 did not show inhibitory effects in this model, Lamertz et al. reported sgp130 to neutralize IL-6 cluster signaling by directly binding to the IL-6/IL-6Rα complex on a transmitting cell (65). Given these contradictory findings, the IL-6 trans-presentation mechanism in addition to its biological and pathogenic roles remains to be characterized and elucidated.
IL-6 Signaling in Breast Cancer
Breast cancer is the most commonly diagnosed cancer in women. Despite recent advancements in targeted therapeutics, remission and survival in metastatic breast cancer patients remains poor (1). Metastatic dissemination can be regulated by multiple mechanisms including uncontrolled inflammation in the breast tumor microenvironment through the secretion of chemokines, growth factors, and cytokines to mediate immune evasion and promote tumor progression (66, 67). Importantly, the tumor microenvironment is comprised of a myriad of cell types, such as tumor-associated macrophages (TAMs), helper T cells, bone marrow-derived cells, adipocytes, fibroblasts, and cancer cells which secrete pro-inflammatory cytokines, such as IL-6. IL-6 can be secreted in an autocrine or paracrine manner by both immune and non-immune cells in the tumor microenvironment. The mechanisms by which IL-6 mediates crosstalk between the tumor microenvironment and tumor cells continues to be investigated to develop therapeutic targets in breast cancer. Specifically, IL-6 is of particular interest due to its increased levels in sera of breast cancer patients when compared to normal sera or tissue of healthy patients (68, 69). Increased IL-6 levels can be a result of single nucleotide polymorphisms (SNPs) in the promoter region of IL-6 gene, and have been demonstrated to predict poor prognoses in breast cancer patients. IL-6 SNPs are significantly associated with ER-positivity and results in a worse disease-free survival (70). Specifically, the rs1800795 SNP in IL-6 is associated with an increased risk of breast cancer metastasis, irrespective of ER status (71). Furthermore, IL-6 is upregulated in sera of patients with advanced stages of breast cancer and in patients presenting with metastases (68, 72). Notably, patients with metastases at two or more sites have increased IL-6 sera levels compared to patients presenting with one metastasis, and high levels of IL-6 correlates with significantly worse survival in metastatic breast cancer patients (72–74). In addition to IL-6, clinicopathological analyses have been conducted on IL-6’s corresponding receptor, IL-6R. Labovsky et al. identified IL-6 to positively correlate with IL-6R in breast cancer specimens, and that IL-6/IL-6R are co-overexpressed in breast carcinomas when compared to normal mammary tissues (75). Consequently, higher serum levels of sIL-6R predicts a shorter relapse-free survival in ER+ breast cancer patients (76). To complement above findings, stromal expression of IL-6Rα in primary breast carcinomas has been reported to be significantly correlated with metastatic occurrence, and a worse disease-free and overall patient survival (77). Moreover, IL-6 has also been reported to correlate with therapeutic resistance in breast cancer patients highlighting the IL-6/JAK/STAT3 pathway as an important prognostic marker in breast cancer progression, chemoresistance, and metastatic formation (78).
IL-6 Activation of STAT3
In pathophysiological states, IL-6 mediates inflammation while concurrently regulating MAPK, PI3K, and JAK/STAT oncogenic pathways (79). STAT3 is a primary downstream regulator of IL-6 signaling with its distinct role in regulating inflammation and neoplastic transformation (80, 81). Although STAT3 activation is tightly regulated under homeostatic conditions, overexpression of upstream effectors such as IL-6, IL-6Rα, or gp130 or loss of negative regulators (SOCS, PIAS, etc.) can lead to aberrantly activated STAT3 (82). IL-6 and phosphorylated-STAT3 (phospho-STAT3, pSTAT3) are co-overexpressed in primary breast cancer specimens (83). Upstream regulators that mediate STAT3 activation include canonical cytokines, growth factors, G-protein-coupled receptors, and microRNAs (miRNAs); however, IL-6 remains the primary activator of STAT3 signaling (84). For example, conditioned medium from IL-6-positive breast cancer cells stimulated STAT3 phosphorylation in IL-6-negative breast cancer and non-cancerous epithelial cells, while administration of anti-IL-6 antibodies abrogated these effects (85). Furthermore, homozygous STAT3 knockout tumors presented with decreased tumoral IL-6 expression and reduced systemic IL-6 levels in an orthotopic mammary fat pad (MFP) syngeneic mouse model (86). Functionally, the IL-6/JAK/STAT3 signaling axis promotes proliferation, angiogenesis, EMT, and the cancer stem cell (CSC) subpopulation, while concurrently suppressing the antitumor immune response (87–90). Secreted IL-6 induces expression of STAT3 target genes such as cyclin D1, Bcl-2, Bcl-xL, VEGF, VEGFR2, and matrix metalloproteinases (MMPs) (91–94). STAT3 is aberrantly active in breast cancer and promotes cancer growth through transcriptionally regulating target gene expression resulting in induction of G1 cell cycle progression, proliferation, anti-apoptosis, angiogenesis, and metastasis (95–97). Dysregulated STAT3 activates immunosuppressive tumor-infiltrating myeloid-derived suppressor cells (MDSCs), TAMs, and T regulatory cells; STAT3 further induces expression of upstream cytokines and growth factors creating a vicious autocrine and paracrine positive feedback loop (86, 98–101). In addition to STAT3, IL-6 can be further activated through nuclear factor kappa B (NF-κB) signaling in breast cancer. IL-6 is repressed by the let-7 miRNA, which targets the 3’-untranslated region (UTR) of IL-6 mRNA. Activation of NF-κB represses let-7 and results in super-activation of IL-6 and subsequent activation of STAT3 (102). OSM can also further activate IL-6/JAK/STAT3 signaling both in vitro and in vivo to promote breast cancer progression. More specifically, OSM synergizes with IL-1β to induce IL-6 secretion in ER+ and TNBC cells for further STAT3 activation (25). Because STAT3 is constitutively active in the majority of breast cancers and plays an important role in mediating breast cancer growth, migration, and metastasis, this review will focus on the IL-6/JAK/STAT3 signaling cascade (95, 103, 104).
IL-6/JAK/STAT3 Role in Breast Cancer EMT and CSCs
Whether IL-6 enhances or inhibits breast cancer cell proliferation in vitro remained controversial for several years. Early studies reported recombinant IL-6 to inhibit or have no significant effect on breast cancer cell proliferation (105–107). More recently, it has become widely accepted IL-6 mediates an oncogenic role in multiple cancers, including breast cancer, primarily through the activation of STAT3. Since IL-6Rα and gp130 are required for signal transduction, the previous contradictory results may have been attributed to a lack of receptor expression in tested breast cancer cell lines, or that IL-6’s pleiotropic effects may depend on JAK/STAT3 pathway activation. For example, gp130 suppresses cell-cycle progression by upregulating G1 cyclin/cyclin-dependent kinase (CDK) inhibitor, p21, independent of STAT3. In stark contrast, gp130-induced STAT3 signaling regulates cell cycle transition from G1 to S phase by upregulating cyclins D2, D3, A, and cell division cycle 25 A (CDC25A) while simultaneously downregulating CDK inhibitors, p21 and p27, indicating contradictory roles that may be regulated by the balance of STAT3 (108).
STAT3 is involved in proliferation and suppression of apoptosis of breast tumor cells through the upregulation of target genes cyclin D1, c-myc, Mcl-1, Bcl-2 and Bcl-xL (92, 109, 110). STAT3 also upregulates MMP2, MMP9, Twist, Snail, and vimentin expression to mediate an EMT phenotype (87, 95, 111, 112). To complement these findings, STAT3 knockdown resulted in a decrease in CD44+ subpopulation, mammosphere formation, and protein expression of stemness genes Oct-4 and Sox-2 in breast cancer cells (113). Sullivan et al. also reported IL-6 induces the EMT phenotype in breast cancer cells, and found IL-6 overexpressing breast cancer xenografts exhibited decreased E-cadherin and increased vimentin protein expression (114). More recently, Cho et al. utilized a microfluidic chip, which mimics the breast cancer microenvironment and breast metastatic phenotypes in vitro, and found that IL-6-treated breast cancer cells successfully invaded into blood and lymph vessels mimicking breast cancer lymphatic metastasis in vitro (115). Others have reported IL-6 to promote breast cancer cell proliferation, migration and invasion, and CSCs in breast cancer (116–118). Notably, breast CSCs are known to play a significant role in tumor recurrence and therapeutic resistance (119). Interestingly, mammospheres derived from node-positive breast carcinomas express higher levels of IL-6 when compared to their respective non-malignant matched mammary tissues indicating IL-6 may play a role in CSC renewal (120). Many studies have further validated IL-6 as a key regulator of breast CSCs. For example, IL-6 enriches the breast CSC subpopulation where administration of IL-6 stimulates spheroid growth in MCF-7 cells (120). To complement these findings, IL-6 enriches CD44+ cells as well as an EMT phenotype in breast cancer in vitro (114, 121). To determine if IL-6-mediated breast CSCs translates in vivo utilizing mouse metastasis models, Korkaya et al. demonstrated PTEN knockdown activates the IL-6 inflammatory loop which, in turn, promotes the breast CSC subpopulation in HER2+/trastuzumab-resistant cells, tumor growth, and secondary metastases in vivo (122).
In addition to breast CSCs, surrounding microenvironmental cells are known to communicate with and prime secondary organs for metastatic dissemination. Multiple mechanisms mediate IL-6-induced breast cancer progression, such as activation of autocrine/paracrine loops under inflammatory conditions and IL-6’s impact on the surrounding tumor microenvironment. For example, the oncogene Multiple Copies in T-cell Malignancy-1 (MCT-1), a recently identified prognostic biomarker in aggressive breast cancers, stimulates M2 macrophages in the tumor microenvironment through stimulation of IL-6. IL-6 promotes M2 macrophage polarization while concurrently stimulating TNBC stemness and tumor progression (123). IL-6 also functions in a paracrine manner to promote an invasive phenotype in breast cancer. For example, adipose stromal cells secrete IL-6 to promote breast cancer migration and invasion in vitro using the TNBC cell line, MDA-MB-231 (124). Further findings confirmed adipocyte-secreted IL-6 induces EMT in luminal A and TNBC cells through the activation of STAT3 (125). Additionally, isolated fibroblasts from breast tissue and breast cancer metastases secrete significantly more IL-6, enhance breast cancer cell growth, and induce pSTAT3 when compared to normal skin fibroblasts supporting IL-6’s role in priming the “soil” for organ-specific metastasis (126). Taken together, the IL-6/JAK/STAT3 pathway is a major regulator of breast cancer metastasis through promoting breast cancer cell proliferation, EMT, enriching the breast CSCs, and suppressing apoptosis.
IL-6/JAK/STAT3 in Metastatic Breast Cancer Mouse Models
Given that the IL-6/JAK/STAT3 signaling axis promotes metastatic phenotypes of breast cancer, researchers have investigated the role of IL-6/JAK/STAT3 in breast cancer metastasis in vivo to identify key drivers and therapeutic interventions. IL-6 has been demonstrated to prime distant sites for metastatic formation. For example, tumor secreted IL-6 has been recently reported to enhance metastatic potential through educating monocyte-dendritic progenitors to prime distant organs for breast cancer metastasis. Magidey-Klein et al. demonstrates IL-6 plays a functional role in mediating crosstalk between primary tumors and the bone marrow to promote monocyte-dendritic progenitors to give rise to immunosuppressive macrophages which, in turn, promotes metastasis in vivo (127). Whether IL-6 mediates organ-specific breast cancer metastasis remains to be conclusively elucidated. However, due to the prominent role of IL-6 in bone metabolism and homeostasis, it is accepted IL-6 is associated with bone metastases and osteoclastogenesis. Dysregulated IL-6 promotes a pro-tumorigenic role in the bone microenvironment allowing breast cancer cells to invade the bone. Consequently, breast tumor cells secrete IL-6 in a paracrine manner to activate osteolytic target genes, namely, PTHrP, RANKL, and DKK-1 (128). In addition to breast cancer bone metastases, recent functional studies have demonstrated IL-6 to promote lung metastases in breast cancer in vivo. Siersbæk et al. conducted an intraductal xenograft implantation of an ER+ breast cancer cell line overexpressing IL-6 and found a significant increase in pSTAT3 in the primary tumors, as well as, an increase in metastases in the lung in vivo (129). Moreover, another group investigated metastatic potential of IL-6 via an intravenous injection of a TNBC cell line overexpressing IL-6, and found tumors to significantly increase lung metastases (86). To complement these findings, Lin et al. reports CGI-99, or C14orf166, enhances IL-6 transcription resulting in hyperactivation of IL-6/STAT3 signaling to promote lung metastases (130). There are multiple downstream effectors under current mechanistic investigation for their role in mediating IL-6-induced metastatic phenotypes in breast cancer. For example, Nyati et al. identified a novel downstream long noncoding RNA, AU021063, which is induced by IL-6 to promote breast cancer metastasis in vivo (131). While multiple models are are currently under investigation, mechanistic mouse models expand beyond the scope of this review. The emerging role of IL-6/JAK/STAT3 in promoting the breast CSC subpopulation and breast cancer metastases in vivo underscores the therapeutic potential in exploiting the IL-6/JAK/STAT3 signaling axis in metastatic breast cancer.
IL-6/JAK/STAT3 in Therapeutic Resistance
Since IL-6/JAK/STAT3 signaling upregulates breast CSCs which are known to mediate metastasis and therapeutic resistance, it is to no surprise that IL-6 has been shown to play a role in chemoresistance. IL-6 secretion and expression is significantly elevated in therapeutically resistant breast cancer cells when compared to their respective parental lines. Furthermore, administration of recombinant IL-6 induced chemoresistance through upregulation of drug-resistant gene, mdr1, in breast cancer cells (132). Wang et al. identified STAT3 induces breast CSC renewal and chemoresistance through upregulation of fatty acid β-oxidation; administration of leptin resensitized breast tumors to chemotherapy in vivo (133). Furthermore, activation of the IL-6 inflammatory loop induces trastuzumab-resistance in HER2+ breast cancer cells indicating that IL-6’s pro-inflammatory role mediates breast cancer therapeutic resistance (122). Given these findings, studies have aimed to utilize IL-6/JAK/STAT3 signaling inhibition in combination with current standard-of-care (SOC) treatment (Tables 1, 2). Administration of Bcl-2 antagonist, sabutoclax, concurrently suppresses IL-6/STAT3 signaling to resensitize chemoresistant breast cancer cells to chemotherapeutic agents (175). Using a resistant ER+ patient-derived xenograft (PDX) mouse model, Siersbæk et al. reported a significant reduction in tumor growth with treatment of STAT3 inhibitor, ruxolitinib, but not fulvestrant alone, a SOC treatment for ER+ breast cancer (129). Clinically, cytoplasmic staining of IL-6Rα is significantly correlated with tamoxifen resistance in ER+ breast cancer patients suggesting IL-6/JAK/STAT3 is an actionable therapeutic target to sensitize tumor cells to current SOC treatment (78). To summarize, the IL-6/JAK/STAT3 signaling pathway mediates breast cancer progression, metastasis, and therapeutic resistance thus justifying investigations into IL-6/JAK/STAT3 as a targeted therapy for breast cancer patients.
Current Therapeutic Applications of IL-6 Signaling in Breast Cancer
Due to the heterogeneity of breast cancer, molecular classifications help determine which tumors may respond to targeted therapy. Each breast cancer subtype corresponds to a different prognosis and treatment regimen. Patients with luminal A and B breast cancer subtypes typically respond to targeted treatments such as tamoxifen, fulvestrant, or aromatase inhibitors; luminal patients have the most treatment options and better prognoses (196). HER2-enriched breast cancers initially respond to anti-HER2 antibodies such as Food and Drug Administration (FDA)-approved traustuzumab, lapatinib, pertuzumab, ado-trastuzumab emtansinse, and fam-trastuzumab (197–200), while TNBC patients have limited to no treatment options. Since TNBC tumors lack expression of ER, PR, and HER2, TNBC patients lack sensitivity to endocrine and molecular targeted treatments. Current SOC for TNBC patients includes systemic neoadjuvant chemotherapy and surgical resection (201). HER2-enriched breast cancer and TNBC are considered to be the most aggressive subtypes and maintain a higher propensity to metastasize (202). Metastatic HER2-enriched patients commonly acquire resistance to HER2-targeted therapies within one year, emphasizing the importance in developing novel therapeutics to treat or sensitize metastatic HER2-enriched tumors to current SOC treatment (203). Current findings elucidating the role of IL-6 in breast cancer progression, metastasis, and anti-cancer immunity, suggest the IL-6/JAK/STAT3 signaling pathway is an actionable target with preclinical and clinical studies demonstrating therapeutic potential in both primary and metastatic breast cancer. Notably, inhibiting the IL-6/JAK/STAT3 signaling axis has been investigated through directly targeting either IL-6, IL-6Rα, gp130 receptor, JAKs, or STAT3.
IL-6 Inhibitors
The FDA has yet to approve IL-6/JAK/STAT3 pathway inhibitors for breast cancer. However, monoclonal antibodies (mAb) and small molecule inhibitors are under preclinical (Table 1) and clinical (Table 2) investigation. Siltuximab is a chimeric IL-6 mAb which received FDA-approval for the treatment of multicentric Castlemans disease in 2014 (204). Morancho and others examined the efficacy of siltuximab in several PDX models, and found only two of the six lines responded to siltuximab treatment. Contradictory to previous findings, they did not find a significant reduction in pSTAT3 in all PDX cultures after inhibiting IL-6, indicating that identification of IL-6-dependent tumors is important for anti-IL-6 therapies to be efficacious (134). For example, serum IL-6 may be used as a biomarker for IL-6-mediated treatment. Casneuf et al. analyzed the IL-6 serum levels of ERα-positive breast cancer patients and found IL-6 sera levels to be significantly correlated with intratumoral pSTAT3 protein expression. Furthermore, pretreatment of siltuximab reduced tumor growth in an ERα-positive breast cancer xenograft mouse model. Casneuf et al. also investigated a combination treatment using siltuximab and fulvestrant, and found combination treatment to attenuate tumor growth suggesting that IL-6/JAK/STAT3 combination therapy may sensitize tumors to SOC treatment (135). MEDI5116 is a novel anti-IL-6 mAb which neutralizes IL-6, is efficacious against HER2+ trastuzumab-resistant tumors, suppresses NF-κB signaling, and lung metastases (136). NF-κB promotes an IL-6 feed-forward inflammatory loop, whereas interruption of IL-6/NF-κB signaling may counteract IL-6-induced breast cancer chemoresistance and requires further investigation (102).
IL-6Rα Inhibitors
Multiple anti-rheumatic agents targeting IL-6, IL-6Rα, and JAKs have gained FDA-approval and have transformed treatment outcomes for autoimmune and inflammatory diseases. In 2010, tocilizumab was the first approved in the United States for the treatment of rheumatoid arthritis (RA). Tocilizumab is a humanized anti-IL-6Rα mAb that competitively binds IL-6Rα and disrupts the IL-6/IL-6Rα complex in both classic and trans-signaling. Tociluzumab has a favorable safety and toxicity profile, and is now used for the treatment of juvenile idiopathic arthritis, adult-onset still’s disease, giant cell arthritis, chimeric antigen receptor T cell-induced cytokine release syndrome, and systemic associated-interstitial lung disease (205–207). In 2021, Tocilizumab received an emergency use authorization for the COVID-19 patients above the age of 2 years old (208).
Due to its diverse application, tocilizumab has been investigated in multiple cancers extensively, including breast cancer. Direct inhibition of IL-6Rα using tocilizumab effectively sensitizes resistant ER+ cells to tamoxifen in vitro and in vivo (78). HER2+ cells treated with tocilizumab or ruxolitinib, a JAK1/2 inhibitor, had reduced pSTAT3 protein expression, and increased cell apoptosis. Tocilizumab suppresses tumor volume, pSTAT3 protein expression, and cell proliferation (Ki67) in HER2+ orthotopic xenograft tumors (138). Administration of tocilizumab also reduces IL-6-mediated tumor growth, breast CSCs, and the development of secondary metastases in a PTEN-/HER2+/trastuzumab-resistant xenograft mouse model (122). Furthermore, tocilizumab inhibits TNBC mammosphere formation and suppresses mRNA expression of stemness markers: CD44, CD133, ALDH-1, EpCAM, Snail, Nanog, Oct-4, and Sox2 (123). Utilizing a TNBC intracardiac mouse model to model metastases, tocilizumab significantly suppresses bone metastases and osteoclast formation in vivo (137). Additionally, Jin et al. reported TNBC cells to secrete IL-6 in order to communicate with lymphatic endothelial cells to produce chemokine (C-C motif) ligand 5 (CCL5) to upregulate breast cancer lymph node metastasis. Combination treatment of tocilizumab and maraviroc, a CCR5 inhibitor, significantly reduces migratory and invasive phenotypes in TNBC cells in vitro, and breast cancer metastases in a TNBC xenograft mouse model in vivo (209). Given these results, tocilizumab proceeded to multiple clinical investigations (Table 2).
Tocilizumab was recently investigated in combination with trastuzumab and pertuzumab in metastatic trastuzumab-resistant HER2+ breast cancer patients in a Phase I clinical trial. This clinical study was completed March 20, 2020 and results are still under review (NCT03135171). Interestingly, tocilizumab is also under clinical investigation for severe COVID-19 treatment in breast cancer versus non-cancer patients where SOC chemotherapy may exacerbate severity of COVID-19 infection (NCT04871854). Tocilizumab in combination with atezolizumab and nab-paclitaxel is also under clinical investigation for safety of immunotherapy-based combination treatment in metastatic or inoperable locally advanced TNBC (NCT03424005). Sarilumab, an additional FDA-approved anti-IL-6Rα mAb for RA, which blocks both membrane-bound and soluble IL-6Rα, is under current Phase I and II clinical investigation in combination with capecitabine in stage I-III TNBC and metastatic TNBC patients (NCT04333706).
While IL-6Rα mAbs, tocilizumab and sarilumab, have made recent headway in clinical studies for breast cancer, drug repurposing remains an attractive therapeutic strategy to minimize the expensive, time-consuming drug development process (210). Diacerin, a non-steroidal anti-inflammatory drug used to treat osteoarthritis, directly interacts with IL-6Rα to suppress IL-6-induced phosphorylation of gp130, JAK1/2, STAT3, and MAPK in two TNBC cell lines. Furthermore, diacerin inhibits IL-6-induced STAT3 nuclear localization and transcriptional activity in TNBC cells, and significantly reduces tumor volume and induces apoptosis when compared to vehicle treated mice. Diacerin treatment reduces protein expression of IL-6Rα, pSTAT3, pMAPK, pAKT in TNBC tumor sections indicating diacerin could inhibit multiple IL-6-regulated oncogenic pathways (139).
Another strategy uses natural products as anti-cancer therapies. Aryappalli and colleagues report Manuka honey antagonizes IL-6Rα which inhibits downstream gp130, pJAK2, and pSTAT3; Manuka honey flavonoids, luteolin, chrysin, quercetin, and galangin disrupt IL-6 binding to IL-6Rα (141). Investigation of anti-cancer mechanisms of tubulosine, originally isolated from bark of Pogonopus tubulosus in 1964, identified tubulosine as a potent inhibitor of JAK2/STAT3 signaling through disruption of IL-6/IL-6Rα/gp130 complex formation (142). Furthermore, a triterpenoid saponin extracted from traditional Chinese medicine, Chikusetsusaponin IVa Butyl Ester (CS-IVa-Be), exhibits immunomodulatory effects by directly binding and antagonizing IL-6Rα. CS-Iva-Be reduces IL-6-induced STAT3 transactivation, TNBC cell viability, and synergizes with TRAIL to induce apoptosis in MDA-MB-231 cells (143). Overall, modulating IL-6/IL-6Rα interaction shows promising results in all subtypes of breast cancer mediated by IL-6/JAK/STAT3 signaling.
gp130 Inhibitors
The gp130 receptor has evolved as an attractive therapeutic target to prevent downstream IL-6 signaling. Interestingly, small molecules which are FDA-approved for other therapeutic implications have been identified to have gp130 inhibitory effects. Since gp130 is the signal-transducing subunit for all IL-6 family cytokines, few gp130 inhibitors are able to maintain selectivity against IL-6. Bazedoxifene, an FDA-approved selective estrogen receptor modulator (SERM) with conjugated estrogens, was previously identified to reduce breast cancer cell proliferation and downregulate ERα and cyclin D1; however, its antitumor mechanism was not elucidated until recently (211). Interestingly, since the IL-6 family of cytokines bind different regions on the surface of gp130, bazedoxifene is able to selectively inhibit IL-6-induced STAT3 in TNBC both in vitro and in vivo through direct binding of the gp130’s D1 domain (144, 145). Bazedoxifene was identified using a multiple-ligand simultaneous docking and drug repositioning approach in order to identify a small molecule that was able to directly bind into “hot-spot” residues on gp130 to prevent protein-protein interactions between IL-6 and gp130. Bazedoxifene inhibits STAT3-mediated transcriptional activity and, in turn, suppresses breast cancer colony formation, migration, and invasion. Bazedoxifene also reduces TNBC tumor volume suggesting the translational potential of the compound as an IL-6/JAK/STAT3 inhibitor (145). Whether bazedoxifene can inhibit IL-6-induced metastatic formation in TNBC is not known. Since bazedoxifene is FDA-approved with a favorable safety profile, bazedoxifene may provide clinical utility as a repurposed compound for the treatment of TNBC, but requires further investigation. Raloxifene, an additional FDA-approved SERM, has also been identified to directly bind to gp130 and suppress STAT3 activation in a TNBC cell line, SUM-159 (144). To further complement these findings, another group identified raloxifene to suppress breast cancer cell viability using another TNBC cell line, MDA-MB-231 (146). In 2007, raloxifene gained FDA-approval for the prevention of invasive breast cancer in postmenopausal women (212, 213). Currently, raloxifene is not approved for treatment of breast cancer. Of note, bazedoxifene and raloxifene are both FDA-approved for the prevention of postmenopausal osteoporosis, and have been reported to prevent bone loss and increase bone mineral density (214). Since the bone is a common distant site of metastasis in breast cancer, and breast cancer bone metastatic patients suffer from microfractures and severe pain, raloxifene and bazedoxifene may provide additional benefits in addition to treating primary breast tumors (215).
JAK Inhibitors
Another approach to targeting the IL-6/JAK/STAT3 signaling axis is through direct JAK inhibition of one or multiple JAK family of enzymes. Tofacitinib, ruxolitinib, baricitinib, and upadacitinib are all FDA-approved JAK inhibitors for implications other than breast cancer, e.g. RA, psoriatic arthritis, severe ulcerative colitis, polyarticular course juvenile idiopathic arthritis, myelofibrosis (216–220). Ruxolitinib is a bioavailable tyrosine kinase inhibitor of both JAK1 and JAK2, and was investigated in metastatic TNBC patients (NCT01562873). pSTAT3-positive metastatic TNBC patients were enrolled in a non-randomized Phase II study to examine ruxolitinib safety and efficacy. Although ruxolitinib was well-tolerated and exhibited on-target activity, this clinical study did not reach its primary efficacy endpoint indicating alternative mechanisms may mediate resistance (193). To potentially overcome this barrier, ruxolitinib is under current clinical investigations to examine combination treatments with paclitaxel, doxorubicin, cyclophosphamide, or pembrolizumab in TNBC patients (Table 2) (NCT03012230; NCT02928978). Furthermore, additional JAK inhibitors have been investigated preclinically and are demonstrated to be efficacious in vivo. Glyceryl trinitrate inhibits JAK2 through s-nitrosylation to suppress IL-6-induced migration and invasion in TNBC cells. Additionally, glyceryl trinitrate infusion decreases lung metastatic lesions in a TNBC syngeneic mouse model (147). Pentadecanoic acid suppresses the CSC subpopulation through inhibition of IL-6/JAK/STAT3 signaling and increases apoptosis in ER+ breast cancer cell line; however, the exact mechanism remains unknown (148). In vitro evidence identified a ferrocene derivative, 1- ferrocenyl-3-(4-methylsulfonylphenyl)propen-1-one (FMSP), that reduces IL-6-induced downstream effectors, CSC renewal, and downregulates stemness markers: Wnt1, Notch1, β-catenin, SOX2, CXCR4, and ALDH1A1 (149). Liu et al. investigated multiple derivatives of 2-phenyl-1,8-naphthyridin-4-one and identified LYF-11 which blocked IL-6-mediated EMT through the suppression of phosphorylated JAK2 (150). Direct mechanisms and efficacy in vivo of inhibitors listed above remain to be investigated. Other JAK inhibitors investigated preclinically in breast cancer include withaferin A, AG490, naphtho[1,2-b]furan-4,5-dione, 3-deoxy-2β,16-dihydroxynagilactone E, tagalide A, ganoderic acid A, methylseleninic acid, and 7β-(3-Ethyl-cis-crotonoyloxy)-1α-(2-methylbutyryloxy)-3,14-dehydro-Z-notonipetranone (Table 1). While JAK inhibition remains heavily studied in multiple cancers, the FDA has administered safety warnings against JAK inhibitors underscoring the need to investigate additional approaches to target the IL-6/JAK/STAT3 pathway (221).
STAT3 Inhibitors
STAT3 has gained significant attraction as an actionable anti-cancer therapeutic; however, there are currently no FDA-approved STAT3-targeted therapies for the treatment of cancer. Therefore, multiple studies have investigated novel small-molecule compounds which negatively regulate STAT3 activation in breast cancer (222) (Table 1). Strategies for STAT3 inhibition include disruption of STAT3 phosphorylation, dimerization, nuclear translocation, or prevention of DNA binding. In 2006, stattic was identified as a small-molecule that disrupts the src homology-2 (SH2) domain of STAT3, and thereby prevents STAT3 recruitment to gp130 on the cell membrane (158). Functionally, increasing doses of stattic was able to prevent STAT3 dimerization and nuclear translocation resulting in a subsequent decrease in IL-6-induced pSTAT3. Stattic induces apoptosis in TNBC cells (158). Since its discovery, others have identified stattic to be efficacious against breast CSCs through the downregulation of STAT3 stemness genes Oct-4, Sox-2, and Slug (113). Combination studies also reveal stattic is synergistic with SOC therapeutic, doxorubicin, and suppresses anti-apoptotic genes, Bcl-2 and Bcl-xL, to promote breast cancer cell apoptosis (159). Interestingly, another STAT3 inhibitor, STA-21, also directly binds to the SH2 domain of STAT3 to repress STAT3 transcriptional activity, and is efficacious in TNBC cells in vitro (160). Utilizing a structural-based computational screening approach, S3I-201 was identified to target the SH2 domain of STAT3 and suppress downstream signaling to induce breast cancer cell apoptosis and exhibit activity in vivo in a TNBC mouse model (172). Additionally, small molecule STAT3 inhibitors, FLLL31 and FLLL32, are derivatives of curcumin and selectively bind to the JAK2 and STAT3 SH2 domain. The JAK2 and STAT3 SH2 domain is essential for STAT3 phosphorylation, therefore, inhibition disrupts STAT3 dimerization and translocation required for activation of STAT3 transcriptional activity. Subsequently, downstream STAT3 target genes are significantly downregulated upon increasing doses of FLLL31 and FLLL32. FLLL31 exhibits efficacy in vivo where systemic administration reduced tumor growth and vascularity in a TNBC xenograft mouse model (161).
Other novel molecules have been identified to exhibit anti-STAT3 activity by inhibiting STAT3 phosphorylation. LLL12, prevents IL-6-induced STAT3 phosphorylation at Y705, and demonstrates efficacy in vivo in a TNBC MFP mouse model with a concomitant reduction in tumor volume and pSTAT3 expression (163). Interestingly, novel JAK2/STAT3 inhibitor, WP1066, can penetrate the blood-brain-barrier, suppress brain metastases in vivo, and prolong overall survival in mice inoculated with brain-trophic TNBC breast cancer cells via an intracardiac injection. WP1066 also reduces breast cancer cell viability and cell invasion in brain-trophic breast cancer cells through the reduction of STAT3 target genes, MMP-9 and VEGFR2 (94). Furthermore, Zinzalla and colleagues synthesized multiple pyrrolidinesulphonylaryl molecules and identified compound, 6a, to selectively inhibit IL-6-induced pSTAT3 in TNBC cells, and inhibit cell growth in STAT3-dependent but not STAT3-null cells demonstrating its dependency on STAT3 for inhibition (162). Additional novel STAT3 inhibitors that have been investigated preclinically for the treatment of breast cancer include: LLY17, 6Br-6a, napabucasin, and coumarin-benzo[b]thiophene 1, 1-dioxide conjugates (Table 1) (179, 181, 187, 188).
Interestingly, multiple natural compounds have been identified to suppress STAT3 activity and exhibit anti-cancer properties. For example, CDDO-Me is a triterpenoid with anti-inflammatory properties and suppresses activated STAT3 protein expression, nuclear translocation, and STAT3 anti-apoptotic genes in ovarian and breast cancer in vitro (164). Other natural compounds which have exhibited anti-cancer properties in breast cancer through modulation of STAT3 activity include: naringenin, ilamycin C, esculentoside A, catechol, dihydrotanshinone, DT-13, cucurbitacin E, galiellalactone, schisandrin A, pectolinarigenin, eupalinolide J, betulinic acid, deguelin, picrasidine G, and cantharidin (Table 1) (166–171, 173, 177, 180, 183, 185, 186, 190–192). Compounds have also been repositioned for the treatment of breast cancer due to their anti-cancer activity through inhibition of STAT3, and are under preclinical investigation. For example, niclosamide is currently FDA-approved as an anti-parasitic drug, yet treatment exhibited inhibition of IL-6-induced STAT3 activation resulting in suppression of adipocyte-induced EMT in breast cancer cells (176). Additionally, nifuroxazide, an antibiotic, exhibits anti-STAT3 activity and suppresses breast cancer tumor growth and lung metastases (178). Additional repurposed compounds under preclinical investigation for inhibition of STAT3 activity include pyrimethamine, flubendazole, and carfilzomib (Table 1) (182, 184, 189). While STAT3 inhibitors have been extensively investigated preclinically, only one compound is under current clinical investigation. TTI-101 is a novel small molecule STAT3 inhibitor, and is in a Phase I clinical trial examining pharmacokinetics and compound safety in advanced breast cancer patients as well as patients with unresectable solid tumors (NCT03195699).
Conclusions
Under normal conditions, IL-6 is an important regulator in acute phase immune responses and modulates both anti- and pro-inflammatory reactions. Breast cancer cells can hijack the IL-6/JAK/STAT3 signaling to evade normal immune responses and further promote tumor growth by activating surrounding microenvironmental cells. Therefore, it remains pertinent to maintain a homeostatic balance of IL-6/JAK/STAT3 as dysregulation creates a vicious autocrine and paracrine inflammatory loop which promotes breast cancer metastasis and therapeutic resistance. Recent reports extensively elaborate on IL-6’s pleiotropic effects and pro-metastatic role in breast cancer; however, current evidence on whether IL-6 promotes site-specific metastases requires further investigation. Due to recent evidence of IL-6 inducing the CSC subpopulation and mediating therapeutic resistance in breast cancer, preclinical investigations in metastatic breast cancer focus on targeting this pathway with either mAbs, novel small molecule compounds, or by repurposing current FDA-approved compounds. Multiple actionable therapeutic targets reside in the IL-6 pathway including inhibition of IL-6 directly, IL-6Rα, gp130 receptor, JAKs, or STAT3. While there remains a plethora of preclinical studies analyzing IL-6/JAK/STAT3 inhibitors on breast cancer growth, there remains an urgent gap analyzing compound efficacy against breast cancer metastases in vivo. Additionally, since IL-6 modulates multiple physiological processes and oncogenic pathways, elucidating effective biomarkers for breast cancer patients who could benefit from targeted IL-6/JAK/STAT3 inhibitors could aid in the development of therapeutics for metastatic breast cancer patients.
Author Contributions
Designed the manuscript, SM. Selected the reviewed literature, SM. Compiled the review tables and figures, SM. Wrote the manuscript, SM. Contributed literature search, DD. Table preparation, DD, GW, and SM. Manuscript writing and editing, DD and GW. Supervised, H-WL. Helped design figures, H-WL. Edited, H-WL. Funded this publication, H-WL. All authors have read and agreed to the published version of the manuscript.
Funding
The authors acknowledge funding support by NIH grants P30CA012197 (BP), 1R01CA228137-01A1 (H-WL), as well as, DoD grants, W81XWH-17-1-0044 (H-WL), W81XWH-19-1-0072 (H-WL), W81XWH-19-1-0753 (H-WL), W81XWH-20-1-0044 (H-WL) and F31CA261027-01A1 (DLD).
Conflict of Interest
The authors declare that the research was conducted in the absence of any commercial or financial relationships that could be construed as a potential conflict of interest.
Publisher’s Note
All claims expressed in this article are solely those of the authors and do not necessarily represent those of their affiliated organizations, or those of the publisher, the editors and the reviewers. Any product that may be evaluated in this article, or claim that may be made by its manufacturer, is not guaranteed or endorsed by the publisher.
Acknowledgments
The authors would like to thank the Carpenter Library at Wake Forest University School of Medicine for open-access literature support.
References
1. Siegel RL, Miller KD, Fuchs HE, Jemal A. Cancer Statistics, 2021. CA Cancer J Clin (2021) 71(1):7–33. doi: 10.3322/caac.21654
2. Perou CM, Sørlie T, Eisen MB, van de Rijn M, Jeffrey SS, Rees CA, et al. Molecular Portraits of Human Breast Tumours. Nature (2000) 406(6797):747–52. doi: 10.1038/35021093
3. Sørlie T, Perou CM, Tibshirani R, Aas T, Geisler S, Johnsen H, et al. Gene Expression Patterns of Breast Carcinomas Distinguish Tumor Subclasses With Clinical Implications. Proc Natl Acad Sci USA (2001) 98(19):10869–74. doi: 10.1073/pnas.191367098
4. Chaffer CL, Weinberg RA. A Perspective on Cancer Cell Metastasis. Science (2011) 331(6024):1559–64. doi: 10.1126/science.1203543
5. Wu Q, Li J, Zhu S, Wu J, Chen C, Liu Q, et al. Breast Cancer Subtypes Predict the Preferential Site of Distant Metastases: A SEER Based Study. Oncotarget (2017) 8(17):27990–6. doi: 10.18632/oncotarget.15856
6. Scully OJ, Bay BH, Yip G, Yu Y. Breast Cancer Metastasis. Cancer Genomics Proteomics (2012) 9(5):311–20.
7. Hirano T, Matsuda T, Turner M, Miyasaka N, Buchan G, Tang B, et al. Excessive Production of Interleukin 6/B Cell Stimulatory Factor-2 in Rheumatoid Arthritis. Eur J Immunol (1988) 18(11):1797–801. doi: 10.1002/eji.1830181122
8. Katsume A, Saito H, Yamada Y, Yorozu K, Ueda O, Akamatsu K, et al. Anti-Interleukin 6 (IL-6) Receptor Antibody Suppresses Castleman's Disease Like Symptoms Emerged in IL-6 Transgenic Mice. Cytokine (2002) 20(6):304–11. doi: 10.1006/cyto.2002.2012
9. Sanguinete MMM, Oliveira PH, Martins-Filho A, Micheli DC, Tavares-Murta BM, Murta EFC, et al. Serum IL-6 and IL-8 Correlate With Prognostic Factors in Ovarian Cancer. Immunol Invest (2017) 46(7):677–88. doi: 10.1080/08820139.2017.1360342
10. Dobrzycka B, Mackowiak-Matejczyk B, Terlikowska KM, Kulesza-Bronczyk B, Kinalski M, Terlikowski SJ. Serum Levels of IL-6, IL-8 and CRP as Prognostic Factors in Epithelial Ovarian Cancer. Eur Cytokine Netw (2013) 24(3):106–13. doi: 10.1684/ecn.2013.0340
11. Kotowicz B, Fuksiewicz M, Jonska-Gmyrek J, Bidzinski M, Kowalska M. The Assessment of the Prognostic Value of Tumor Markers and Cytokines as SCCAg, CYFRA 21.1, IL-6, VEGF and sTNF Receptors in Patients With Squamous Cell Cervical Cancer, Particularly With Early Stage of the Disease. Tumour Biol (2016) 37(1):1271–8. doi: 10.1007/s13277-015-3914-0
12. Yeh KY, Li YY, Hsieh LL, Lu CH, Chou WC, Liaw CC, et al. Analysis of the Effect of Serum Interleukin-6 (IL-6) and Soluble IL-6 Receptor Levels on Survival of Patients With Colorectal Cancer. Jpn J Clin Oncol (2010) 40(6):580–7. doi: 10.1093/jjco/hyq010
13. Chung YC, Chang YF. Serum Interleukin-6 Levels Reflect the Disease Status of Colorectal Cancer. J Surg Oncol (2003) 83(4):222–6. doi: 10.1002/jso.10269
14. Chen MF, Chen PT, Lu MS, Lin PY, Chen WC, Lee KD. IL-6 Expression Predicts Treatment Response and Outcome in Squamous Cell Carcinoma of the Esophagus. Mol Cancer (2013) 12:26. doi: 10.1186/1476-4598-12-26
15. Riedel F, Zaiss I, Herzog D, Götte K, Naim R, Hörmann K. Serum Levels of Interleukin-6 in Patients With Primary Head and Neck Squamous Cell Carcinoma. Anticancer Res (2005) 25(4):2761–5.
16. Miura T, Mitsunaga S, Ikeda M, Shimizu S, Ohno I, Takahashi H, et al. Characterization of Patients With Advanced Pancreatic Cancer and High Serum Interleukin-6 Levels. Pancreas (2015) 44(5):756–63. doi: 10.1097/MPA.0000000000000335
17. Feng L, Qi Q, Wang P, Chen H, Chen Z, Meng Z, et al. Serum Levels of IL-6, IL-8, and IL-10 are Indicators of Prognosis in Pancreatic Cancer. J Int Med Res (2018) 46(12):5228–36. doi: 10.1177/0300060518800588
18. Okada S, Okusaka T, Ishii H, Kyogoku A, Yoshimori M, Kajimura N, et al. Elevated Serum Interleukin-6 Levels in Patients With Pancreatic Cancer. Jpn J Clin Oncol (1998) 28(1):12–5. doi: 10.1093/jjco/28.1.12
19. Michalaki V, Syrigos K, Charles P, Waxman J. Serum Levels of IL-6 and TNF-Alpha Correlate With Clinicopathological Features and Patient Survival in Patients With Prostate Cancer. Br J Cancer (2004) 90(12):2312–6. doi: 10.1038/sj.bjc.6601814
20. Loosen SH, Schulze-Hagen M, Leyh C, Benz F, Vucur M, Kuhl C, et al. IL-6 and IL-8 Serum Levels Predict Tumor Response and Overall Survival After TACE for Primary and Secondary Hepatic Malignancies. Int J Mol Sci (2018) 19(6). doi: 10.3390/ijms19061766
21. Chang CH, Hsiao CF, Yeh YM, Chang GC, Tsai YH, Chen YM, et al. Circulating Interleukin-6 Level Is a Prognostic Marker for Survival in Advanced Nonsmall Cell Lung Cancer Patients Treated With Chemotherapy. Int J Cancer (2013) 132(9):1977–85. doi: 10.1002/ijc.27892
22. An J, Gu Q, Cao L, Yang H, Deng P, Hu C, et al. Serum IL-6 as a Vital Predictor of Severe Lung Cancer. Ann Palliat Med (2021) 10(1):202–9. doi: 10.21037/apm-20-2229
23. Ikeguchi M, Hatada T, Yamamoto M, Miyake T, Matsunaga T, Fukumoto Y, et al. Serum Interleukin-6 and -10 Levels in Patients With Gastric Cancer. Gastric Cancer (2009) 12(2):95–100. doi: 10.1007/s10120-009-0509-8
24. Ahmed OI, Adel AM, Diab DR, Gobran NS. Prognostic Value of Serum Level of Interleukin-6 and Interleukin-8 in Metastatic Breast Cancer Patients. Egypt J Immunol (2006) 13(2):61–8.
25. Tawara K, Scott H, Emathinger J, Wolf C, LaJoie D, Hedeen D, et al. HIGH Expression of OSM and IL-6 are Associated With Decreased Breast Cancer Survival: Synergistic Induction of IL-6 Secretion by OSM and IL-1β. Oncotarget (2019) 10(21):2068–85. doi: 10.18632/oncotarget.26699
26. Wolfe AR, Trenton NJ, Debeb BG, Larson R, Ruffell B, Chu K, et al. Mesenchymal Stem Cells and Macrophages Interact Through IL-6 to Promote Inflammatory Breast Cancer in Pre-Clinical Models. Oncotarget (2016) 7(50):82482–92. doi: 10.18632/oncotarget.12694
27. Kishimoto T, Ishizaka K. Regulation of Antibody Response In Vitro. X. Biphasic Effect of Cyclic AMP on the Secondary Anti-Hapten Antibody Response to Anti-Immunoglobulin and Enhancing Soluble Factor. J Immunol (1976) 116(2):534–41.
28. Hirano T, Yasukawa K, Harada H, Taga T, Watanabe Y, Matsuda T, et al. Complementary DNA for a Novel Human Interleukin (BSF-2) That Induces B Lymphocytes to Produce Immunoglobulin. Nature (1986) 324(6092):73–6. doi: 10.1038/324073a0
29. Gauldie J, Richards C, Harnish D, Lansdorp P, Baumann H. Interferon Beta 2/B-Cell Stimulatory Factor Type 2 Shares Identity With Monocyte-Derived Hepatocyte-Stimulating Factor and Regulates the Major Acute Phase Protein Response in Liver Cells. Proc Natl Acad Sci USA (1987) 84(20):7251–5. doi: 10.1073/pnas.84.20.7251
30. Brakenhoff JP, de Groot ER, Evers RF, Pannekoek H, Aarden LA. Molecular Cloning and Expression of Hybridoma Growth Factor in Escherichia Coli. J Immunol (1987) 139(12):4116–21.
31. Zilberstein A, Ruggieri R, Korn JH, Revel M. Structure and Expression of cDNA and Genes for Human Interferon-Beta-2, a Distinct Species Inducible by Growth-Stimulatory Cytokines. EMBO J (1986) 5(10):2529–37. doi: 10.1002/j.1460-2075.1986.tb04531.x
32. Haegeman G, Content J, Volckaert G, Derynck R, Tavernier J, Fiers W. Structural Analysis of the Sequence Coding for an Inducible 26-kDa Protein in Human Fibroblasts. Eur J Biochem (1986) 159(3):625–32. doi: 10.1111/j.1432-1033.1986.tb09931.x
33. Collison LW, Delgoffe GM, Guy CS, Vignali KM, Chaturvedi V, Fairweather D, et al. The Composition and Signaling of the IL-35 Receptor Are Unconventional. Nat Immunol (2012) 13(3):290–9. doi: 10.1038/ni.2227
34. Wang X, Wei Y, Xiao H, Liu X, Zhang Y, Han G, et al. A Novel IL-23p19/Ebi3 (IL-39) Cytokine Mediates Inflammation in Lupus-Like Mice. Eur J Immunol (2016) 46(6):1343–50. doi: 10.1002/eji.201546095
35. Rose-John S. Interleukin-6 Family Cytokines. Cold Spring Harb Perspect Biol (2018) 10(2). doi: 10.1101/cshperspect.a028415
36. Hibi M, Murakami M, Saito M, Hirano T, Taga T, Kishimoto T. Molecular Cloning and Expression of an IL-6 Signal Transducer, Gp130. Cell (1990) 63(6):1149–57. doi: 10.1016/0092-8674(90)90411-7
37. Heink S, Yogev N, Garbers C, Herwerth M, Aly L, Gasperi C, et al. Trans-Presentation of IL-6 by Dendritic Cells Is Required for the Priming of Pathogenic T(H)17 Cells. Nat Immunol (2017) 18(1):74–85. doi: 10.1038/ni.3632
38. Taga T, Hibi M, Hirata Y, Yamasaki K, Yasukawa K, Matsuda T, et al. Interleukin-6 Triggers the Association of Its Receptor With a Possible Signal Transducer, Gp130. Cell (1989) 58(3):573–81. doi: 10.1016/0092-8674(89)90438-8
39. Boulanger MJ, Chow DC, Brevnova EE, Garcia KC. Hexameric Structure and Assembly of the Interleukin-6/IL-6 Alpha-Receptor/Gp130 Complex. Science (2003) 300(5628):2101–4. doi: 10.1126/science.1083901
40. Guschin D, Rogers N, Briscoe J, Witthuhn B, Watling D, Horn F, et al. A Major Role for the Protein Tyrosine Kinase JAK1 in the JAK/STAT Signal Transduction Pathway in Response to Interleukin-6. EMBO J (1995) 14(7):1421–9. doi: 10.1002/j.1460-2075.1995.tb07128.x
41. Kaptein A, Paillard V, Saunders M. Dominant Negative Stat3 Mutant Inhibits Interleukin-6-Induced Jak-STAT Signal Transduction. J Biol Chem (1996) 271(11):5961–4. doi: 10.1074/jbc.271.11.5961
42. Shuai K, Ziemiecki A, Wilks AF, Harpur AG, Sadowski HB, Gilman MZ, et al. Polypeptide Signalling to the Nucleus Through Tyrosine Phosphorylation of Jak and Stat Proteins. Nature (1993) 366(6455):580–3. doi: 10.1038/366580a0
43. Haan S, Keller JF, Behrmann I, Heinrich PC, Haan C. Multiple Reasons for an Inefficient STAT1 Response Upon IL-6-Type Cytokine Stimulation. Cell Signal (2005) 17(12):1542–50. doi: 10.1016/j.cellsig.2005.03.010
44. Morris R, Kershaw NJ, Babon JJ. The Molecular Details of Cytokine Signaling via the JAK/STAT Pathway. Protein Sci (2018) 27(12):1984–2009. doi: 10.1002/pro.3519
45. Chung CD, Liao J, Liu B, Rao X, Jay P, Berta P, et al. Specific Inhibition of Stat3 Signal Transduction by PIAS3. Science (1997) 278(5344):1803–5. doi: 10.1126/science.278.5344.1803
46. Endo TA, Masuhara M, Yokouchi M, Suzuki R, Sakamoto H, Mitsui K, et al. A New Protein Containing an SH2 Domain That Inhibits JAK Kinases. Nature (1997) 387(6636):921–4. doi: 10.1038/43213
47. Xu D, Qu CK. Protein Tyrosine Phosphatases in the JAK/STAT Pathway. Front Biosci (2008) 13:4925–32. doi: 10.2741/3051
48. Dmitrieva OS, Shilovskiy IP, Khaitov MR, Grivennikov SI. Interleukins 1 and 6 as Main Mediators of Inflammation and Cancer. Biochem (Mosc) (2016) 81(2):80–90. doi: 10.1134/S0006297916020024
49. Oberg HH, Wesch D, Grüssel S, Rose-John S, Kabelitz D. Differential Expression of CD126 and CD130 Mediates Different STAT-3 Phosphorylation in CD4+CD25- and CD25high Regulatory T Cells. Int Immunol (2006) 18(4):555–63. doi: 10.1093/intimm/dxh396
50. Müllberg J, Schooltink H, Stoyan T, Günther M, Graeve L, Buse G, et al. The Soluble Interleukin-6 Receptor Is Generated by Shedding. Eur J Immunol (1993) 23(2):473–80. doi: 10.1002/eji.1830230226
51. Müller-Newen G, Köhne C, Keul R, Hemmann U, Müller-Esterl W, Wijdenes J, et al. Purification and Characterization of the Soluble Interleukin-6 Receptor From Human Plasma and Identification of an Isoform Generated Through Alternative Splicing. Eur J Biochem (1996) 236(3):837–42. doi: 10.1111/j.1432-1033.1996.00837.x
52. Müllberg J, Dittrich E, Graeve L, Gerhartz C, Yasukawa K, Taga T, et al. Differential Shedding of the Two Subunits of the Interleukin-6 Receptor. FEBS Lett (1993) 332(1-2):174–8. doi: 10.1016/0014-5793(93)80507-Q
53. Novick D, Engelmann H, Wallach D, Rubinstein M. Soluble Cytokine Receptors Are Present in Normal Human Urine. J Exp Med (1989) 170(4):1409–14. doi: 10.1084/jem.170.4.1409
54. Oh JW, Revel M, Chebath J. A Soluble Interleukin 6 Receptor Isolated From Conditioned Medium of Human Breast Cancer Cells Is Encoded by a Differentially Spliced mRNA. Cytokine (1996) 8(5):401–9. doi: 10.1006/cyto.1996.0055
55. Lust JA, Donovan KA, Kline MP, Greipp PR, Kyle RA, Maihle NJ. Isolation of an mRNA Encoding a Soluble Form of the Human Interleukin-6 Receptor. Cytokine (1992) 4(2):96–100. doi: 10.1016/1043-4666(92)90043-Q
56. Yan I, Schwarz J, Lücke K, Schumacher N, Schumacher V, Schmidt S, et al. ADAM17 Controls IL-6 Signaling by Cleavage of the Murine IL-6rα From the Cell Surface of Leukocytes During Inflammatory Responses. J Leukoc Biol (2016) 99(5):749–60. doi: 10.1189/jlb.3A0515-207R
57. Riethmueller S, Ehlers JC, Lokau J, Düsterhöft S, Knittler K, Dombrowsky G, et al. Cleavage Site Localization Differentially Controls Interleukin-6 Receptor Proteolysis by ADAM10 and ADAM17. Sci Rep (2016) 6:25550. doi: 10.1038/srep25550
58. Jostock T, Müllberg J, Ozbek S, Atreya R, Blinn G, Voltz N, et al. Soluble Gp130 Is the Natural Inhibitor of Soluble Interleukin-6 Receptor Transsignaling Responses. Eur J Biochem (2001) 268(1):160–7. doi: 10.1046/j.1432-1327.2001.01867.x
59. McLoughlin RM, Jenkins BJ, Grail D, Williams AS, Fielding CA, Parker CR, et al. IL-6 Trans-Signaling via STAT3 Directs T Cell Infiltration in Acute Inflammation. Proc Natl Acad Sci USA (2005) 102(27):9589–94. doi: 10.1073/pnas.0501794102
60. Schaper F, Rose-John S. Interleukin-6: Biology, Signaling and Strategies of Blockade. Cytokine Growth Factor Rev (2015) 26(5):475–87. doi: 10.1016/j.cytogfr.2015.07.004
61. Romano M, Sironi M, Toniatti C, Polentarutti N, Fruscella P, Ghezzi P, et al. Role of IL-6 and its Soluble Receptor in Induction of Chemokines and Leukocyte Recruitment. Immunity (1997) 6(3):315–25. doi: 10.1016/S1074-7613(00)80334-9
62. Böttcher JP, Schanz O, Garbers C, Zaremba A, Hegenbarth S, Kurts C, et al. IL-6 Trans-Signaling-Dependent Rapid Development of Cytotoxic CD8+ T Cell Function. Cell Rep (2014) 8(5):1318–27. doi: 10.1016/j.celrep.2014.07.008
63. Chalaris A, Garbers C, Rabe B, Rose-John S, Scheller J. The Soluble Interleukin 6 Receptor: Generation and Role in Inflammation and Cancer. Eur J Cell Biol (2011) 90(6-7):484–94. doi: 10.1016/j.ejcb.2010.10.007
64. Lo CW, Chen MW, Hsiao M, Wang S, Chen CA, Hsiao SM, et al. IL-6 Trans-Signaling in Formation and Progression of Malignant Ascites in Ovarian Cancer. Cancer Res (2011) 71(2):424–34. doi: 10.1158/0008-5472.CAN-10-1496
65. Lamertz L, Rummel F, Polz R, Baran P, Hansen S, Waetzig GH, et al. Soluble Gp130 Prevents Interleukin-6 and Interleukin-11 Cluster Signaling But Not Intracellular Autocrine Responses. Sci Signal (2018) 11(550). doi: 10.1126/scisignal.aar7388
66. Quail DF, Joyce JA. Microenvironmental Regulation of Tumor Progression and Metastasis. Nat Med (2013) 19(11):1423–37. doi: 10.1038/nm.3394
67. Bates JP, Derakhshandeh R, Jones L, Webb TJ. Mechanisms of Immune Evasion in Breast Cancer. BMC Cancer (2018) 18(1):556. doi: 10.1186/s12885-018-4441-3
68. Kozłowski L, Zakrzewska I, Tokajuk P, Wojtukiewicz MZ. Concentration of Interleukin-6 (IL-6), Interleukin-8 (IL-8) and Interleukin-10 (IL-10) in Blood Serum of Breast Cancer Patients. Rocz Akad Med Bialymst (2003) 48:82–4.
69. Jiang XP, Yang DC, Elliott RL, Head JF. Reduction in Serum IL-6 After Vacination of Breast Cancer Patients With Tumour-Associated Antigens is Related to Estrogen Receptor Status. Cytokine (2000) 12(5):458–65. doi: 10.1006/cyto.1999.0591
70. DeMichele A, Gray R, Horn M, Chen J, Aplenc R, Vaughan WP, et al. Host Genetic Variants in the Interleukin-6 Promoter Predict Poor Outcome in Patients With Estrogen Receptor-Positive, Node-Positive Breast Cancer. Cancer Res (2009) 69(10):4184–91. doi: 10.1158/0008-5472.CAN-08-2989
71. Abana CO, Bingham BS, Cho JH, Graves AJ, Koyama T, Pilarski RT, et al. IL-6 Variant Is Associated With Metastasis in Breast Cancer Patients. PloS One (2017) 12(7):e0181725. doi: 10.1371/journal.pone.0181725
72. Salgado R, Junius S, Benoy I, Van Dam P, Vermeulen P, Van Marck E, et al. Circulating Interleukin-6 Predicts Survival in Patients With Metastatic Breast Cancer. Int J Cancer (2003) 103(5):642–6. doi: 10.1002/ijc.10833
73. Bachelot T, Ray-Coquard I, Menetrier-Caux C, Rastkha M, Duc A, Blay JY. Prognostic Value of Serum Levels of Interleukin 6 and of Serum and Plasma Levels of Vascular Endothelial Growth Factor in Hormone-Refractory Metastatic Breast Cancer Patients. Br J Cancer (2003) 88(11):1721–6. doi: 10.1038/sj.bjc.6600956
74. Bozcuk H, Uslu G, Samur M, Yildiz M, Ozben T, Ozdoğan M, et al. Tumour Necrosis Factor-Alpha, Interleukin-6, and Fasting Serum Insulin Correlate With Clinical Outcome in Metastatic Breast Cancer Patients Treated With Chemotherapy. Cytokine (2004) 27(2-3):58–65. doi: 10.1016/j.cyto.2004.04.002
75. Labovsky V, Martinez LM, Davies KM, García-Rivello H, Calcagno Mde L, Matas A, et al. Association Between Ligands and Receptors Related to the Progression of Early Breast Cancer in Tumor Epithelial and Stromal Cells. Clin Breast Cancer (2015) 15(1):e13–21. doi: 10.1016/j.clbc.2014.05.006
76. Won HS, Kim YA, Lee JS, Jeon EK, An HJ, Sun DS, et al. Soluble Interleukin-6 Receptor Is a Prognostic Marker for Relapse-Free Survival in Estrogen Receptor-Positive Breast Cancer. Cancer Invest (2013) 31(8):516–21. doi: 10.3109/07357907.2013.826239
77. Labovsky V, Martinez LM, Calcagno ML, Davies KM, García-Rivello H, Wernicke A, et al. Interleukin-6 Receptor in Spindle-Shaped Stromal Cells, a Prognostic Determinant of Early Breast Cancer. Tumour Biol (2016) 37(10):13377–84. doi: 10.1007/s13277-016-5268-7
78. Tsoi H, Man EPS, Chau KM, Khoo US. Targeting the IL-6/STAT3 Signalling Cascade to Reverse Tamoxifen Resistance in Estrogen Receptor Positive Breast Cancer. Cancers (Basel) (2021) 13(7). doi: 10.3390/cancers13071511
79. Yang L, Wang L, Lin HK, Kan PY, Xie S, Tsai MY, et al. Interleukin-6 Differentially Regulates Androgen Receptor Transactivation via PI3K-Akt, STAT3, and MAPK, Three Distinct Signal Pathways in Prostate Cancer Cells. Biochem Biophys Res Commun (2003) 305(3):462–9. doi: 10.1016/S0006-291X(03)00792-7
80. Yu H, Pardoll D, Jove R. STATs in Cancer Inflammation and Immunity: A Leading Role for STAT3. Nat Rev Cancer (2009) 9(11):798–809. doi: 10.1038/nrc2734
81. Catlett-Falcone R, Landowski TH, Oshiro MM, Turkson J, Levitzki A, Savino R, et al. Constitutive Activation of Stat3 Signaling Confers Resistance to Apoptosis in Human U266 Myeloma Cells. Immunity (1999) 10(1):105–15. doi: 10.1016/S1074-7613(00)80011-4
82. Kubo M, Hanada T, Yoshimura A. Suppressors of Cytokine Signaling and Immunity. Nat Immunol (2003) 4(12):1169–76. doi: 10.1038/ni1012
83. Berishaj M, Gao SP, Ahmed S, Leslie K, Al-Ahmadie H, Gerald WL, et al. Stat3 is Tyrosine-Phosphorylated Through the Interleukin-6/Glycoprotein 130/Janus Kinase Pathway in Breast Cancer. Breast Cancer Res (2007) 9(3):R32. doi: 10.1186/bcr1680
84. Zhong Z, Wen Z, Darnell JE Jr. Stat3: A STAT Family Member Activated by Tyrosine Phosphorylation in Response to Epidermal Growth Factor and Interleukin-6. Science (1994) 264(5155):95–8. doi: 10.1126/science.8140422
85. Lieblein JC, Ball S, Hutzen B, Sasser AK, Lin HJ, Huang TH, et al. STAT3 can be Activated Through Paracrine Signaling in Breast Epithelial Cells. BMC Cancer (2008) 8:302. doi: 10.1186/1471-2407-8-302
86. Chang Q, Bournazou E, Sansone P, Berishaj M, Gao SP, Daly L, et al. The IL-6/JAK/Stat3 Feed-Forward Loop Drives Tumorigenesis and Metastasis. Neoplasia (2013) 15(7):848–62. doi: 10.1593/neo.13706
87. Lo HW, Hsu SC, Xia W, Cao X, Shih JY, Wei Y, et al. Epidermal Growth Factor Receptor Cooperates With Signal Transducer and Activator of Transcription 3 to Induce Epithelial-Mesenchymal Transition in Cancer Cells via Up-Regulation of TWIST Gene Expression. Cancer Res (2007) 67(19):9066–76. doi: 10.1158/0008-5472.CAN-07-0575
88. Niu G, Wright KL, Huang M, Song L, Haura E, Turkson J, et al. Constitutive Stat3 Activity Up-Regulates VEGF Expression and Tumor Angiogenesis. Oncogene (2002) 21(13):2000–8. doi: 10.1038/sj.onc.1205260
89. Kortylewski M, Yu H. Role of Stat3 in Suppressing Anti-Tumor Immunity. Curr Opin Immunol (2008) 20(2):228–33. doi: 10.1016/j.coi.2008.03.010
90. Wang T, Niu G, Kortylewski M, Burdelya L, Shain K, Zhang S, et al. Regulation of the Innate and Adaptive Immune Responses by Stat-3 Signaling in Tumor Cells. Nat Med (2004) 10(1):48–54. doi: 10.1038/nm976
91. Fukada T, Hibi M, Yamanaka Y, Takahashi-Tezuka M, Fujitani Y, Yamaguchi T, et al. Two Signals are Necessary for Cell Proliferation Induced by a Cytokine Receptor Gp130: Involvement of STAT3 in Anti-Apoptosis. Immunity (1996) 5(5):449–60. doi: 10.1016/S1074-7613(00)80501-4
92. Leslie K, Lang C, Devgan G, Azare J, Berishaj M, Gerald W, et al. Cyclin D1 Is Transcriptionally Regulated by and Required for Transformation by Activated Signal Transducer and Activator of Transcription 3. Cancer Res (2006) 66(5):2544–52. doi: 10.1158/0008-5472.CAN-05-2203
93. Burke WM, Jin X, Lin HJ, Huang M, Liu R, Reynolds RK, et al. Inhibition of Constitutively Active Stat3 Suppresses Growth of Human Ovarian and Breast Cancer Cells. Oncogene (2001) 20(55):7925–34. doi: 10.1038/sj.onc.1204990
94. Lee HT, Xue J, Chou PC, Zhou A, Yang P, Conrad CA, et al. Stat3 Orchestrates Interaction Between Endothelial and Tumor Cells and Inhibition of Stat3 Suppresses Brain Metastasis of Breast Cancer Cells. Oncotarget (2015) 6(12):10016–29. doi: 10.18632/oncotarget.3540
95. Carpenter RL, Lo HW. STAT3 Target Genes Relevant to Human Cancers. Cancers (Basel) (2014) 6(2):897–925. doi: 10.3390/cancers6020897
96. Alvarez JV, Febbo PG, Ramaswamy S, Loda M, Richardson A, Frank DA. Identification of a Genetic Signature of Activated Signal Transducer and Activator of Transcription 3 in Human Tumors. Cancer Res (2005) 65(12):5054–62. doi: 10.1158/0008-5472.CAN-04-4281
97. Dechow TN, Pedranzini L, Leitch A, Leslie K, Gerald WL, Linkov I, et al. Requirement of Matrix Metalloproteinase-9 for the Transformation of Human Mammary Epithelial Cells by Stat3-C. Proc Natl Acad Sci USA (2004) 101(29):10602–7. doi: 10.1073/pnas.0404100101
98. Kujawski M, Kortylewski M, Lee H, Herrmann A, Kay H, Yu H. Stat3 Mediates Myeloid Cell-Dependent Tumor Angiogenesis in Mice. J Clin Invest (2008) 118(10):3367–77. doi: 10.1172/JCI35213
99. Jiang M, Chen J, Zhang W, Zhang R, Ye Y, Liu P, et al. Interleukin-6 Trans-Signaling Pathway Promotes Immunosuppressive Myeloid-Derived Suppressor Cells via Suppression of Suppressor of Cytokine Signaling 3 in Breast Cancer. Front Immunol (2017) 8:1840. doi: 10.3389/fimmu.2017.01840
100. Sun Z, Yao Z, Liu S, Tang H, Yan X. An Oligonucleotide Decoy for Stat3 Activates the Immune Response of Macrophages to Breast Cancer. Immunobiology (2006) 211(3):199–209. doi: 10.1016/j.imbio.2005.11.004
101. Jones LM, Broz ML, Ranger JJ, Ozcelik J, Ahn R, Zuo D, et al. STAT3 Establishes an Immunosuppressive Microenvironment During the Early Stages of Breast Carcinogenesis to Promote Tumor Growth and Metastasis. Cancer Res (2016) 76(6):1416–28. doi: 10.1158/0008-5472.CAN-15-2770
102. Iliopoulos D, Hirsch HA, Struhl K. An Epigenetic Switch Involving NF-Kappab, Lin28, Let-7 MicroRNA, and IL6 Links Inflammation to Cell Transformation. Cell (2009) 139(4):693–706. doi: 10.1016/j.cell.2009.10.014
103. Barbieri I, Pensa S, Pannellini T, Quaglino E, Maritano D, Demaria M, et al. Constitutively Active Stat3 Enhances Neu-Mediated Migration and Metastasis in Mammary Tumors via Upregulation of Cten. Cancer Res (2010) 70(6):2558–67. doi: 10.1158/0008-5472.CAN-09-2840
104. Yu H, Kortylewski M, Pardoll D. Crosstalk Between Cancer and Immune Cells: Role of STAT3 in the Tumour Microenvironment. Nat Rev Immunol (2007) 7(1):41–51. doi: 10.1038/nri1995
105. Danforth DN Jr., Sgagias MK. Interleukin-1 Alpha and Interleukin-6 Act Additively to Inhibit Growth of MCF-7 Breast Cancer Cells In Vitro. Cancer Res (1993) 53(7):1538–45.
106. Badache A, Hynes NE. Interleukin 6 Inhibits Proliferation and, in Cooperation With an Epidermal Growth Factor Receptor Autocrine Loop, Increases Migration of T47D Breast Cancer Cells. Cancer Res (2001) 61(1):383–91.
107. Jiang XP, Yang DC, Elliott RL, Head JF. Down-Regulation of Expression of Interleukin-6 and its Receptor Results in Growth Inhibition of MCF-7 Breast Cancer Cells. Anticancer Res (2011) 31(9):2899–906.
108. Fukada T, Ohtani T, Yoshida Y, Shirogane T, Nishida K, Nakajima K, et al. STAT3 Orchestrates Contradictory Signals in Cytokine-Induced G1 to S Cell-Cycle Transition. EMBO J (1998) 17(22):6670–7. doi: 10.1093/emboj/17.22.6670
109. Real PJ, Sierra A, De Juan A, Segovia JC, Lopez-Vega JM, Fernandez-Luna JL. Resistance to Chemotherapy via Stat3-Dependent Overexpression of Bcl-2 in Metastatic Breast Cancer Cells. Oncogene (2002) 21(50):7611–8. doi: 10.1038/sj.onc.1206004
110. Hsieh FC, Cheng G, Lin J. Evaluation of Potential Stat3-Regulated Genes in Human Breast Cancer. Biochem Biophys Res Commun (2005) 335(2):292–9. doi: 10.1016/j.bbrc.2005.07.075
111. Zhang F, Wang Z, Fan Y, Xu Q, Ji W, Tian R, et al. Elevated STAT3 Signaling-Mediated Upregulation of MMP-2/9 Confers Enhanced Invasion Ability in Multidrug-Resistant Breast Cancer Cells. Int J Mol Sci (2015) 16(10):24772–90. doi: 10.3390/ijms161024772
112. Saitoh M, Endo K, Furuya S, Minami M, Fukasawa A, Imamura T, et al. STAT3 Integrates Cooperative Ras and TGF-β Signals That Induce Snail Expression. Oncogene (2016) 35(8):1049–57. doi: 10.1038/onc.2015.161
113. Chung SS, Giehl N, Wu Y, Vadgama JV. STAT3 Activation in HER2-Overexpressing Breast Cancer Promotes Epithelial-Mesenchymal Transition and Cancer Stem Cell Traits. Int J Oncol (2014) 44(2):403–11. doi: 10.3892/ijo.2013.2195
114. Sullivan NJ, Sasser AK, Axel AE, Vesuna F, Raman V, Ramirez N, et al. Interleukin-6 Induces an Epithelial-Mesenchymal Transition Phenotype in Human Breast Cancer Cells. Oncogene (2009) 28(33):2940–7. doi: 10.1038/onc.2009.180
115. Cho HY, Choi JH, Kim KJ, Shin M, Choi JW. Microfluidic System to Analyze the Effects of Interleukin 6 on Lymphatic Breast Cancer Metastasis. Front Bioeng Biotechnol (2020) 8:611802. doi: 10.3389/fbioe.2020.611802
116. Tamm I, Cardinale I, Krueger J, Murphy JS, May LT, Sehgal PB. Interleukin 6 Decreases Cell-Cell Association and Increases Motility of Ductal Breast Carcinoma Cells. J Exp Med (1989) 170(5):1649–69. doi: 10.1084/jem.170.5.1649
117. Sanguinetti A, Santini D, Bonafè M, Taffurelli M, Avenia N. Interleukin-6 and Pro Inflammatory Status in the Breast Tumor Microenvironment. World J Surg Oncol (2015) 13:129. doi: 10.1186/s12957-015-0529-2
118. Oh K, Lee OY, Park Y, Seo MW, Lee DS. IL-1β Induces IL-6 Production and Increases Invasiveness and Estrogen-Independent Growth in a TG2-Dependent Manner in Human Breast Cancer Cells. BMC Cancer (2016) 16(1):724. doi: 10.1186/s12885-016-2746-7
119. Babaei G, Aziz SG, Jaghi NZZ. EMT, Cancer Stem Cells and Autophagy; The Three Main Axes of Metastasis. BioMed Pharmacother (2021) 133:110909. doi: 10.1016/j.biopha.2020.110909
120. Sansone P, Storci G, Tavolari S, Guarnieri T, Giovannini C, Taffurelli M, et al. IL-6 Triggers Malignant Features in Mammospheres From Human Ductal Breast Carcinoma and Normal Mammary Gland. J Clin Invest (2007) 117(12):3988–4002. doi: 10.1172/JCI32533
121. Xie G, Yao Q, Liu Y, Du S, Liu A, Guo Z, et al. IL-6-Induced Epithelial-Mesenchymal Transition Promotes the Generation of Breast Cancer Stem-Like Cells Analogous to Mammosphere Cultures. Int J Oncol (2012) 40(4):1171–9. doi: 10.3892/ijo.2011.1275
122. Korkaya H, Kim GI, Davis A, Malik F, Henry NL, Ithimakin S, et al. Activation of an IL6 Inflammatory Loop Mediates Trastuzumab Resistance in HER2+ Breast Cancer by Expanding the Cancer Stem Cell Population. Mol Cell (2012) 47(4):570–84. doi: 10.1016/j.molcel.2012.06.014
123. Weng YS, Tseng HY, Chen YA, Shen PC, Al Haq AT, Chen LM, et al. MCT-1/miR-34a/IL-6/IL-6R Signaling Axis Promotes EMT Progression, Cancer Stemness and M2 Macrophage Polarization in Triple-Negative Breast Cancer. Mol Cancer (2019) 18(1):42. doi: 10.1186/s12943-019-0988-0
124. Walter M, Liang S, Ghosh S, Hornsby PJ, Li R. Interleukin 6 Secreted From Adipose Stromal Cells Promotes Migration and Invasion of Breast Cancer Cells. Oncogene (2009) 28(30):2745–55. doi: 10.1038/onc.2009.130
125. Gyamfi J, Lee YH, Eom M, Choi J. Interleukin-6/STAT3 Signalling Regulates Adipocyte Induced Epithelial-Mesenchymal Transition in Breast Cancer Cells. Sci Rep (2018) 8(1):8859. doi: 10.1038/s41598-018-27184-9
126. Studebaker AW, Storci G, Werbeck JL, Sansone P, Sasser AK, Tavolari S, et al. Fibroblasts Isolated From Common Sites of Breast Cancer Metastasis Enhance Cancer Cell Growth Rates and Invasiveness in an Interleukin-6-Dependent Manner. Cancer Res (2008) 68(21):9087–95. doi: 10.1158/0008-5472.CAN-08-0400
127. Magidey-Klein K, Cooper TJ, Kveler K, Normand R, Zhang T, Timaner M, et al. IL-6 Contributes to Metastatic Switch via the Differentiation of Monocytic-Dendritic Progenitors Into Prometastatic Immune Cells. J Immunother Cancer (2021) 9(6). doi: 10.1136/jitc-2021-002856
128. Ara T, Declerck YA. Interleukin-6 in Bone Metastasis and Cancer Progression. Eur J Cancer (2010) 46(7):1223–31. doi: 10.1016/j.ejca.2010.02.026
129. Siersbæk R, Scabia V, Nagarajan S, Chernukhin I, Papachristou EK, Broome R, et al. IL6/STAT3 Signaling Hijacks Estrogen Receptor α Enhancers to Drive Breast Cancer Metastasis. Cancer Cell (2020) 38(3):412–23.e9. doi: 10.1016/j.ccell.2020.06.007
130. Lin C, Liao W, Jian Y, Peng Y, Zhang X, Ye L, et al. CGI-99 Promotes Breast Cancer Metastasis via Autocrine Interleukin-6 Signaling. Oncogene (2017) 36(26):3695–705. doi: 10.1038/onc.2016.525
131. Nyati KK, Hashimoto S, Singh SK, Tekguc M, Metwally H, Liu YC, et al. The Novel Long Noncoding RNA AU021063, Induced by IL-6/Arid5a Signaling, Exacerbates Breast Cancer Invasion and Metastasis by Stabilizing Trib3 and Activating the Mek/Erk Pathway. Cancer Lett (2021) 520:295–306. doi: 10.1016/j.canlet.2021.08.004
132. Conze D, Weiss L, Regen PS, Bhushan A, Weaver D, Johnson P, et al. Autocrine Production of Interleukin 6 Causes Multidrug Resistance in Breast Cancer Cells. Cancer Res (2001) 61(24):8851–8.
133. Wang T, Fahrmann JF, Lee H, Li YJ, Tripathi SC, Yue C, et al. JAK/STAT3-Regulated Fatty Acid β-Oxidation Is Critical for Breast Cancer Stem Cell Self-Renewal and Chemoresistance. Cell Metab (2018) 27(1):136–50.e5. doi: 10.1016/j.cmet.2017.11.001
134. Morancho B, Zacarías-Fluck M, Esgueva A, Bernadó-Morales C, Di Cosimo S, Prat A, et al. Modeling Anti-IL-6 Therapy Using Breast Cancer Patient-Derived Xenografts. Oncotarget (2016) 7(42):67956–65. doi: 10.18632/oncotarget.11815
135. Casneuf T, Axel AE, King P, Alvarez JD, Werbeck JL, Verhulst T, et al. Interleukin-6 is a Potential Therapeutic Target in Interleukin-6 Dependent, Estrogen Receptor-α-Positive Breast Cancer. Breast Cancer (Dove Med Press) (2016) 8:13–27. doi: 10.2147/BCTT.S92414
136. Zhong H, Davis A, Ouzounova M, Carrasco RA, Chen C, Breen S, et al. A Novel IL6 Antibody Sensitizes Multiple Tumor Types to Chemotherapy Including Trastuzumab-Resistant Tumors. Cancer Res (2016) 76(2):480–90. doi: 10.1158/0008-5472.CAN-15-0883
137. Wakabayashi H, Hamaguchi T, Nagao N, Kato S, Iino T, Nakamura T, et al. Interleukin-6 Receptor Inhibitor Suppresses Bone Metastases in a Breast Cancer Cell Line. Breast Cancer (2018) 25(5):566–74. doi: 10.1007/s12282-018-0853-9
138. Rodriguez-Barrueco R, Yu J, Saucedo-Cuevas LP, Olivan M, Llobet-Navas D, Putcha P, et al. Inhibition of the Autocrine IL-6-JAK2-STAT3-Calprotectin Axis as Targeted Therapy for HR-/HER2+ Breast Cancers. Genes Dev (2015) 29(15):1631–48. doi: 10.1101/gad.262642.115
139. Bharti R, Dey G, Ojha PK, Rajput S, Jaganathan SK, Sen R, et al. Diacerein-Mediated Inhibition of IL-6/IL-6R Signaling Induces Apoptotic Effects on Breast Cancer. Oncogene (2016) 35(30):3965–75. doi: 10.1038/onc.2015.466
140. Bharti R, Dey G, Banerjee I, Dey KK, Parida S, Kumar BN, et al. Somatostatin Receptor Targeted Liposomes With Diacerein Inhibit IL-6 for Breast Cancer Therapy. Cancer Lett (2017) 388:292–302. doi: 10.1016/j.canlet.2016.12.021
141. Aryappalli P, Shabbiri K, Masad RJ, Al-Marri RH, Haneefa SM, Mohamed YA, et al. Inhibition of Tyrosine-Phosphorylated STAT3 in Human Breast and Lung Cancer Cells by Manuka Honey is Mediated by Selective Antagonism of the IL-6 Receptor. Int J Mol Sci (2019) 20(18). doi: 10.3390/ijms20184340
142. Kim BH, Yi EH, Li YC, Park IC, Park JY, Ye SK. Anticancer Activity of Tubulosine Through Suppression of Interleukin-6-Induced Janus Kinase 2/Signal Transducer and Activation of Transcription 3 Signaling. J Breast Cancer (2019) 22(3):362–74. doi: 10.4048/jbc.2019.22.e34
143. Yang J, Qian S, Cai X, Lu W, Hu C, Sun X, et al. Chikusetsusaponin IVa Butyl Ester (CS-IVa-Be), a Novel IL6R Antagonist, Inhibits IL6/STAT3 Signaling Pathway and Induces Cancer Cell Apoptosis. Mol Cancer Ther (2016) 15(6):1190–200. doi: 10.1158/1535-7163.MCT-15-0551
144. Li H, Xiao H, Lin L, Jou D, Kumari V, Lin J, et al. Drug Design Targeting Protein-Protein Interactions (PPIs) Using Multiple Ligand Simultaneous Docking (MLSD) and Drug Repositioning: Discovery of Raloxifene and Bazedoxifene as Novel Inhibitors of IL-6/GP130 Interface. J Med Chem (2014) 57(3):632–41. doi: 10.1021/jm401144z
145. Tian J, Chen X, Fu S, Zhang R, Pan L, Cao Y, et al. Bazedoxifene Is a Novel IL-6/GP130 Inhibitor for Treating Triple-Negative Breast Cancer. Breast Cancer Res Treat (2019) 175(3):553–66. doi: 10.1007/s10549-019-05183-2
146. Shi W, Yan D, Zhao C, Xiao M, Wang Y, Ma H, et al. Inhibition of IL-6/STAT3 Signaling in Human Cancer Cells Using Evista. Biochem Biophys Res Commun (2017) 491(1):159–65. doi: 10.1016/j.bbrc.2017.07.067
147. Bouaouiche S, Ghione S, Sghaier R, Burgy O, Racoeur C, Derangère V, et al. Nitric Oxide-Releasing Drug Glyceryl Trinitrate Targets JAK2/STAT3 Signaling, Migration and Invasion of Triple-Negative Breast Cancer Cells. Int J Mol Sci (2021) 22(16). doi: 10.3390/ijms22168449
148. To NB, Nguyen YT, Moon JY, Ediriweera MK, Cho SK. Pentadecanoic Acid, an Odd-Chain Fatty Acid, Suppresses the Stemness of MCF-7/SC Human Breast Cancer Stem-Like Cells Through JAK2/STAT3 Signaling. Nutrients (2020) 12(6). doi: 10.3390/nu12061663
149. Nourbakhsh M, Farzaneh S, Taghikhani A, Zarghi A, Noori S. The Effect of a Newly Synthesized Ferrocene Derivative Against MCF-7 Breast Cancer Cells and Spheroid Stem Cells Through ROS Production and Inhibition of JAK2/STAT3 Signaling Pathway. Anticancer Agents Med Chem (2020) 20(7):875–86. doi: 10.2174/1871520620666200101151743
150. Liu LC, Wu YC, Kuo SC, Ho CT, Way TD, Chen ST. 2-Phenylnaphthyridin-4-One Derivative LYF-11 Inhibits Interleukin-6-Mediated Epithelial-To-Mesenchymal Transition via the Inhibition of JAK2/STAT3 Signaling Pathway in MCF-7 Cells. Anticancer Res (2018) 38(5):2849–59. doi: 10.21873/anticanres.12530
151. Lee J, Hahm ER, Singh SV. Withaferin A Inhibits Activation of Signal Transducer and Activator of Transcription 3 in Human Breast Cancer Cells. Carcinogenesis (2010) 31(11):1991–8. doi: 10.1093/carcin/bgq175
152. Khanna P, Lee JS, Sereemaspun A, Lee H, Baeg GH. GRAMD1B Regulates Cell Migration in Breast Cancer Cells Through JAK/STAT and Akt Signaling. Sci Rep (2018) 8(1):9511. doi: 10.1038/s41598-018-27864-6
153. Lin KL, Su JC, Chien CM, Tseng CH, Chen YL, Chang LS, et al. Naphtho[1,2-B]Furan-4,5-Dione Disrupts Janus Kinase-2 and Induces Apoptosis in Breast Cancer MDA-MB-231 Cells. Toxicol In Vitro (2010) 24(4):1158–67. doi: 10.1016/j.tiv.2010.02.019
154. Shan H, Yao S, Ye Y, Yu Q. 3-Deoxy-2β,16-Dihydroxynagilactone E, a Natural Compound From Podocarpus Nagi, Preferentially Inhibits JAK2/STAT3 Signaling by Allosterically Interacting With the Regulatory Domain of JAK2 and Induces Apoptosis of Cancer Cells. Acta Pharmacol Sin (2019) 40(12):1578–86. doi: 10.1038/s41401-019-0254-4
155. Zhang X-H, Yang Y, Liu J-J, Shen L, Shi Z, Wu J. Tagalide A and Tagalol A, Naturally Occurring 5/6/6/6- and 5/6/6-Fused Cyclic Dolabrane-Type Diterpenes: A New Insight Into the Anti-Breast Cancer Activity of the Dolabrane Scaffold. Organic Chem Front (2018) 5(7):1176–83. doi: 10.1039/C8QO00010G
156. Yang Y, Zhou H, Liu W, Wu J, Yue X, Wang J, et al. Ganoderic Acid A Exerts Antitumor Activity Against MDA-MB-231 Human Breast Cancer Cells by Inhibiting the Janus Kinase 2/Signal Transducer and Activator of Transcription 3 Signaling Pathway. Oncol Lett (2018) 16(5):6515–21. doi: 10.3892/ol.2018.9475
157. Qiu C, Zhang T, Zhu X, Qiu J, Jiang K, Zhao G, et al. Methylseleninic Acid Suppresses Breast Cancer Growth via the JAK2/STAT3 Pathway. Reprod Sci (2019) 26(6):829–38. doi: 10.1177/1933719118815582
158. Schust J, Sperl B, Hollis A, Mayer TU, Berg T. Stattic: A Small-Molecule Inhibitor of STAT3 Activation and Dimerization. Chem Biol (2006) 13(11):1235–42. doi: 10.1016/j.chembiol.2006.09.018
159. Khaki-Khatibi F, Ghorbani M, Sabzichi M, Ramezani F, Mohammadian J. Adjuvant Therapy With Stattic Enriches the Anti-Proliferative Effect of Doxorubicin in Human ZR-75-1 Breast Cancer Cells via Arresting Cell Cycle and Inducing Apoptosis. BioMed Pharmacother (2019) 109:1240–8. doi: 10.1016/j.biopha.2018.10.183
160. Song H, Wang R, Wang S, Lin J. A Low-Molecular-Weight Compound Discovered Through Virtual Database Screening Inhibits Stat3 Function in Breast Cancer Cells. Proc Natl Acad Sci USA (2005) 102(13):4700–5. doi: 10.1073/pnas.0409894102
161. Lin L, Hutzen B, Zuo M, Ball S, Deangelis S, Foust E, et al. Novel STAT3 Phosphorylation Inhibitors Exhibit Potent Growth-Suppressive Activity in Pancreatic and Breast Cancer Cells. Cancer Res (2010) 70(6):2445–54. doi: 10.1158/0008-5472.CAN-09-2468
162. Zinzalla G, Haque MR, Basu BP, Anderson J, Kaye SL, Haider S, et al. A Novel Small-Molecule Inhibitor of IL-6 Signalling. Bioorg Med Chem Lett (2010) 20(23):7029–32. doi: 10.1016/j.bmcl.2010.09.117
163. Lin L, Hutzen B, Li PK, Ball S, Zuo M, DeAngelis S, et al. A Novel Small Molecule, LLL12, Inhibits STAT3 Phosphorylation and Activities and Exhibits Potent Growth-Suppressive Activity in Human Cancer Cells. Neoplasia (2010) 12(1):39–50. doi: 10.1593/neo.91196
164. Duan Z, Ames RY, Ryan M, Hornicek FJ, Mankin H, Seiden MV. CDDO-Me, a Synthetic Triterpenoid, Inhibits Expression of IL-6 and Stat3 Phosphorylation in Multi-Drug Resistant Ovarian Cancer Cells. Cancer Chemother Pharmacol (2009) 63(4):681–9. doi: 10.1007/s00280-008-0785-8
165. Ball MS, Bhandari R, Torres GM, Martyanov V, ElTanbouly MA, Archambault K, et al. CDDO-Me Alters the Tumor Microenvironment in Estrogen Receptor Negative Breast Cancer. Sci Rep (2020) 10(1):6560. doi: 10.1038/s41598-020-63482-x
166. Noori S, Rezaei Tavirani M, Deravi N, Mahboobi Rabbani MI, Zarghi A. Naringenin Enhances the Anti-Cancer Effect of Cyclophosphamide Against MDA-MB-231 Breast Cancer Cells Via Targeting the STAT3 Signaling Pathway. Iran J Pharm Res (2020) 19(3):122–33. doi: 10.22037/ijpr.2020.113103.14112
167. Xie Q, Yang Z, Huang X, Zhang Z, Li J, Ju J, et al. Ilamycin C Induces Apoptosis and Inhibits Migration and Invasion in Triple-Negative Breast Cancer by Suppressing IL-6/STAT3 Pathway. J Hematol Oncol (2019) 12(1):60. doi: 10.1186/s13045-019-0744-3
168. Liu C, Dong L, Sun Z, Wang L, Wang Q, Li H, et al. Esculentoside A Suppresses Breast Cancer Stem Cell Growth Through Stemness Attenuation and Apoptosis Induction by Blocking IL-6/STAT3 Signaling Pathway. Phytother Res (2018) 32(11):2299–311. doi: 10.1002/ptr.6172
169. Choi HS, Kim JH, Kim SL, Deng HY, Lee D, Kim CS, et al. Catechol Derived From Aronia Juice Through Lactic Acid Bacteria Fermentation Inhibits Breast Cancer Stem Cell Formation via Modulation Stat3/IL-6 Signaling Pathway. Mol Carcinog (2018) 57(11):1467–79. doi: 10.1002/mc.22870
170. Kim SL, Choi HS, Kim JH, Jeong DK, Kim KS, Lee DS. Dihydrotanshinone-Induced NOX5 Activation Inhibits Breast Cancer Stem Cell Through the ROS/Stat3 Signaling Pathway. Oxid Med Cell Longev (2019) 2019:9296439. doi: 10.1155/2019/9296439
171. He J, Wei X, Li S, Quan X, Li R, Du H, et al. DT-13 Suppresses Breast Cancer Metastasis by Modulating PLOD2 in the Adipocytes Microenvironment. Phytomedicine (2019) 59:152778. doi: 10.1016/j.phymed.2018.12.001
172. Siddiquee K, Zhang S, Guida WC, Blaskovich MA, Greedy B, Lawrence HR, et al. Selective Chemical Probe Inhibitor of Stat3, Identified Through Structure-Based Virtual Screening, Induces Antitumor Activity. Proc Natl Acad Sci USA (2007) 104(18):7391–6. doi: 10.1073/pnas.0609757104
173. Lan T, Wang L, Xu Q, Liu W, Jin H, Mao W, et al. Growth Inhibitory Effect of Cucurbitacin E on Breast Cancer Cells. Int J Clin Exp Pathol (2013) 6(9):1799–805.
174. Fujimoto M, Kito H, Kajikuri J, Ohya S. Transcriptional Repression of Human Epidermal Growth Factor Receptor 2 by ClC-3 Cl(-) /H(+) Transporter Inhibition in Human Breast Cancer Cells. Cancer Sci (2018) 109(9):2781–91. doi: 10.1111/cas.13715
175. Hu Y, Yagüe E, Zhao J, Wang L, Bai J, Yang Q, et al. Sabutoclax, Pan-Active BCL-2 Protein Family Antagonist, Overcomes Drug Resistance and Eliminates Cancer Stem Cells in Breast Cancer. Cancer Lett (2018) 423:47–59. doi: 10.1016/j.canlet.2018.02.036
176. Gyamfi J, Lee YH, Min BS, Choi J. Niclosamide Reverses Adipocyte Induced Epithelial-Mesenchymal Transition in Breast Cancer Cells via Suppression of the Interleukin-6/STAT3 Signalling Axis. Sci Rep (2019) 9(1):11336. doi: 10.1038/s41598-019-47707-2
177. Ko H, Lee JH, Kim HS, Kim T, Han YT, Suh YG, et al. Novel Galiellalactone Analogues Can Target STAT3 Phosphorylation and Cause Apoptosis in Triple-Negative Breast Cancer. Biomolecules (2019) 9(5). doi: 10.3390/biom9050170
178. Yang F, Hu M, Lei Q, Xia Y, Zhu Y, Song X, et al. Nifuroxazide Induces Apoptosis and Impairs Pulmonary Metastasis in Breast Cancer Model. Cell Death Dis (2015) 6(3):e1701. doi: 10.1038/cddis.2015.63
179. Pan L, Chen X, Fu S, Yu W, Li C, Wang T, et al. LLY17, a Novel Small Molecule STAT3 Inhibitor Induces Apoptosis and Suppresses Cell Migration and Tumor Growth in Triple-Negative Breast Cancer. Breast Cancer Res Treat (2020) 181(1):31–41. doi: 10.1007/s10549-020-05613-6
180. Zhang ZL, Jiang QC, Wang SR. Schisandrin A Reverses Doxorubicin-Resistant Human Breast Cancer Cell Line by the Inhibition of P65 and Stat3 Phosphorylation. Breast Cancer (2018) 25(2):233–42. doi: 10.1007/s12282-017-0821-9
181. Liu Z, Ge X, Gu Y, Huang Y, Liu H, Yu M, et al. Small Molecule STAT3 Inhibitor, 6Br-6a Suppresses Breast Cancer Growth In Vitro and In Vivo. BioMed Pharmacother (2020) 121:109502. doi: 10.1016/j.biopha.2019.109502
182. Khan MW, Saadalla A, Ewida AH, Al-Katranji K, Al-Saoudi G, Giaccone ZT, et al. The STAT3 Inhibitor Pyrimethamine Displays Anti-Cancer and Immune Stimulatory Effects in Murine Models of Breast Cancer. Cancer Immunol Immunother (2018) 67(1):13–23. doi: 10.1007/s00262-017-2057-0
183. Li Y, Gan C, Zhang Y, Yu Y, Fan C, Deng Y, et al. Inhibition of Stat3 Signaling Pathway by Natural Product Pectolinarigenin Attenuates Breast Cancer Metastasis. Front Pharmacol (2019) 10:1195. doi: 10.3389/fphar.2019.01195
184. Oh E, Kim YJ, An H, Sung D, Cho TM, Farrand L, et al. Flubendazole Elicits Anti-Metastatic Effects in Triple-Negative Breast Cancer via STAT3 Inhibition. Int J Cancer (2018) 143(8):1978–93. doi: 10.1002/ijc.31585
185. Yang B, Shen JW, Zhou DH, Zhao YP, Wang WQ, Zhu Y, et al. Precise Discovery of a STAT3 Inhibitor From Eupatorium Lindleyanum and Evaluation of Its Activity of Anti-Triple-Negative Breast Cancer. Nat Prod Res (2019) 33(4):477–85. doi: 10.1080/14786419.2017.1396596
186. Zeng AQ, Yu Y, Yao YQ, Yang FF, Liao M, Song LJ, et al. Betulinic Acid Impairs Metastasis and Reduces Immunosuppressive Cells in Breast Cancer Models. Oncotarget (2018) 9(3):3794–804. doi: 10.18632/oncotarget.23376
187. Löcken H, Clamor C, Müller K. Napabucasin and Related Heterocycle-Fused Naphthoquinones as STAT3 Inhibitors With Antiproliferative Activity Against Cancer Cells. J Nat Prod (2018) 81(7):1636–44. doi: 10.1021/acs.jnatprod.8b00247
188. Cai G, Yu W, Song D, Zhang W, Guo J, Zhu J, et al. Discovery of Fluorescent Coumarin-Benzo[B]Thiophene 1, 1-Dioxide Conjugates as Mitochondria-Targeting Antitumor STAT3 Inhibitors. Eur J Med Chem (2019) 174:236–51. doi: 10.1016/j.ejmech.2019.04.024
189. Vyas D, Lopez-Hisijos N, Shah P, Deshpande KS, Basson MD, Vyas A, et al. A Second-Generation Proteasome Inhibitor and Doxorubicin Modulates IL-6, pSTAT-3 and NF-kB Activity in MDA-MB-231 Breast Cancer Cells. J Nanosci Nanotechnol (2017) 17(1):175–85. doi: 10.1166/jnn.2017.12427
190. Mehta R, Katta H, Alimirah F, Patel R, Murillo G, Peng X, et al. Deguelin Action Involves C-Met and EGFR Signaling Pathways in Triple Negative Breast Cancer Cells. PloS One (2013) 8(6):e65113. doi: 10.1371/journal.pone.0065113
191. Yamashita N, Kondo M, Zhao S, Li W, Koike K, Nemoto K, et al. Picrasidine G Decreases Viability of MDA-MB 468 EGFR-Overexpressing Triple-Negative Breast Cancer Cells Through Inhibition of EGFR/STAT3 Signaling Pathway. Bioorg Med Chem Lett (2017) 27(11):2608–12. doi: 10.1016/j.bmcl.2017.03.061
192. Chun J, Park MK, Ko H, Lee K, Kim YS. Bioassay-Guided Isolation of Cantharidin From Blister Beetles and its Anticancer Activity Through Inhibition of Epidermal Growth Factor Receptor-Mediated STAT3 and Akt Pathways. J Nat Med (2018) 72(4):937–45. doi: 10.1007/s11418-018-1226-6
193. Stover DG, Gil Del Alcazar CR, Brock J, Guo H, Overmoyer B, Balko J, et al. Phase II Study of Ruxolitinib, a Selective JAK1/2 Inhibitor, in Patients With Metastatic Triple-Negative Breast Cancer. NPJ Breast Cancer (2018) 4:10. doi: 10.1038/s41523-018-0060-z
194. O'Shaughnessy J, DeMichele A, Ma CX, Richards P, Yardley DA, Wright GS, et al. A Randomized, Double-Blind, Phase 2 Study of Ruxolitinib or Placebo in Combination With Capecitabine in Patients With Advanced HER2-Negative Breast Cancer and Elevated C-Reactive Protein, a Marker of Systemic Inflammation. Breast Cancer Res Treat (2018) 170(3):547–57. doi: 10.1007/s10549-018-4770-6
195. Kearney M, Franks L, Lee S, Tiersten A, Makower DF, Cigler T, et al. Phase I/II Trial of Ruxolitinib in Combination With Trastuzumab in Metastatic HER2 Positive Breast Cancer. Breast Cancer Res Treat (2021) 189(1):177–85. doi: 10.1007/s10549-021-06306-4
196. Hennigs A, Riedel F, Gondos A, Sinn P, Schirmacher P, Marmé F, et al. Prognosis of Breast Cancer Molecular Subtypes in Routine Clinical Care: A Large Prospective Cohort Study. BMC Cancer (2016) 16(1):734. doi: 10.1186/s12885-016-2766-3
197. Cobleigh MA, Vogel CL, Tripathy D, Robert NJ, Scholl S, Fehrenbacher L, et al. Multinational Study of the Efficacy and Safety of Humanized Anti-HER2 Monoclonal Antibody in Women Who Have HER2-Overexpressing Metastatic Breast Cancer That has Progressed After Chemotherapy for Metastatic Disease. J Clin Oncol (1999) 17(9):2639–48. doi: 10.1200/JCO.1999.17.9.2639
198. Ryan Q, Ibrahim A, Cohen MH, Johnson J, Ko CW, Sridhara R, et al. FDA Drug Approval Summary: Lapatinib in Combination With Capecitabine for Previously Treated Metastatic Breast Cancer That Overexpresses HER-2. Oncologist (2008) 13(10):1114–9. doi: 10.1634/theoncologist.2008-0816
199. Howie LJ, Scher NS, Amiri-Kordestani L, Zhang L, King-Kallimanis BL, Choudhry Y, et al. FDA Approval Summary: Pertuzumab for Adjuvant Treatment of HER2-Positive Early Breast Cancer. Clin Cancer Res (2019) 25(10):2949–55. doi: 10.1158/1078-0432.CCR-18-3003
200. Baron JM, Boster BL, Barnett CM. Ado-Trastuzumab Emtansine (T-DM1): A Novel Antibody-Drug Conjugate for the Treatment of HER2-Positive Metastatic Breast Cancer. J Oncol Pharm Pract (2015) 21(2):132–42. doi: 10.1177/1078155214527144
201. Yin L, Duan JJ, Bian XW, Yu SC. Triple-Negative Breast Cancer Molecular Subtyping and Treatment Progress. Breast Cancer Res (2020) 22(1):61. doi: 10.1186/s13058-020-01296-5
202. Kast K, Link T, Friedrich K, Petzold A, Niedostatek A, Schoffer O, et al. Impact of Breast Cancer Subtypes and Patterns of Metastasis on Outcome. Breast Cancer Res Treat (2015) 150(3):621–9. doi: 10.1007/s10549-015-3341-3
203. Slamon DJ, Leyland-Jones B, Shak S, Fuchs H, Paton V, Bajamonde A, et al. Use of Chemotherapy Plus a Monoclonal Antibody Against HER2 for Metastatic Breast Cancer That Overexpresses HER2. N Engl J Med (2001) 344(11):783–92. doi: 10.1056/NEJM200103153441101
204. Deisseroth A, Ko CW, Nie L, Zirkelbach JF, Zhao L, Bullock J, et al. FDA Approval: Siltuximab for the Treatment of Patients With Multicentric Castleman Disease. Clin Cancer Res (2015) 21(5):950–4. doi: 10.1158/1078-0432.CCR-14-1678
205. Le RQ, Li L, Yuan W, Shord SS, Nie L, Habtemariam BA, et al. FDA Approval Summary: Tocilizumab for Treatment of Chimeric Antigen Receptor T Cell-Induced Severe or Life-Threatening Cytokine Release Syndrome. Oncologist (2018) 23(8):943–7. doi: 10.1634/theoncologist.2018-0028
206. Thompson CA. FDA Approves Tocilizumab to Treat Rheumatoid Arthritis. Am J Health Syst Pharm (2010) 67(4):254. doi: 10.2146/news100012
207. Sheppard M, Laskou F, Stapleton PP, Hadavi S, Dasgupta B. Tocilizumab (Actemra). Hum Vaccin Immunother (2017) 13(9):1972–88. doi: 10.1080/21645515.2017.1316909
209. Jin K, Pandey NB, Popel AS. Simultaneous Blockade of IL-6 and CCL5 Signaling for Synergistic Inhibition of Triple-Negative Breast Cancer Growth and Metastasis. Breast Cancer Res (2018) 20(1):54. doi: 10.1186/s13058-018-0981-3
210. Pushpakom S, Iorio F, Eyers PA, Escott KJ, Hopper S, Wells A, et al. Drug Repurposing: Progress, Challenges and Recommendations. Nat Rev Drug Discov (2019) 18(1):41–58. doi: 10.1038/nrd.2018.168
211. Lewis-Wambi JS, Kim H, Curpan R, Grigg R, Sarker MA, Jordan VC. The Selective Estrogen Receptor Modulator Bazedoxifene Inhibits Hormone-Independent Breast Cancer Cell Growth and Down-Regulates Estrogen Receptor α and Cyclin D1. Mol Pharmacol (2011) 80(4):610–20. doi: 10.1124/mol.111.072249
212. Vogel VG. The NSABP Study of Tamoxifen and Raloxifene (STAR) Trial. Expert Rev Anticancer Ther (2009) 9(1):51–60. doi: 10.1586/14737140.9.1.51
213. Gennari L, Merlotti D, Paola VD, Nuti R. Raloxifene in Breast Cancer Prevention. Expert Opin Drug Saf (2008) 7(3):259–70. doi: 10.1517/14740338.7.3.259
214. Barrionuevo P, Kapoor E, Asi N, Alahdab F, Mohammed K, Benkhadra K, et al. Efficacy of Pharmacological Therapies for the Prevention of Fractures in Postmenopausal Women: A Network Meta-Analysis. J Clin Endocrinol Metab (2019) 104(5):1623–30. doi: 10.1210/jc.2019-00192
215. Brook N, Brook E, Dharmarajan A, Dass CR, Chan A. Breast Cancer Bone Metastases: Pathogenesis and Therapeutic Targets. Int J Biochem Cell Biol (2018) 96:63–78. doi: 10.1016/j.biocel.2018.01.003
216. Sandborn WJ, Su C, Sands BE, D'Haens GR, Vermeire S, Schreiber S, et al. Tofacitinib as Induction and Maintenance Therapy for Ulcerative Colitis. N Engl J Med (2017) 376(18):1723–36. doi: 10.1056/NEJMoa1606910
217. Wollenhaupt J, Lee EB, Curtis JR, Silverfield J, Terry K, Soma K, et al. Safety and Efficacy of Tofacitinib for Up to 9.5 Years in the Treatment of Rheumatoid Arthritis: Final Results of a Global, Open-Label, Long-Term Extension Study. Arthritis Res Ther (2019) 21(1):89. doi: 10.1186/s13075-019-1866-2
218. Mease P, Hall S, FitzGerald O, van der Heijde D, Merola JF, Avila-Zapata F, et al. Tofacitinib or Adalimumab Versus Placebo for Psoriatic Arthritis. N Engl J Med (2017) 377(16):1537–50. doi: 10.1056/NEJMoa1615975
219. Ruperto N, Brunner HI, Zuber Z, Tzaribachev N, Kingsbury DJ, Foeldvari I, et al. Pharmacokinetic and Safety Profile of Tofacitinib in Children With Polyarticular Course Juvenile Idiopathic Arthritis: Results of a Phase 1, Open-Label, Multicenter Study. Pediatr Rheumatol Online J (2017) 15(1):86. doi: 10.1186/s12969-017-0212-y
220. Verstovsek S, Mesa RA, Gotlib J, Levy RS, Gupta V, DiPersio JF, et al. A Double-Blind, Placebo-Controlled Trial of Ruxolitinib for Myelofibrosis. N Engl J Med (2012) 366(9):799–807. doi: 10.1056/NEJMoa1110557
221. Nash P, Kerschbaumer A, Dörner T, Dougados M, Fleischmann RM, Geissler K, et al. Points to Consider for the Treatment of Immune-Mediated Inflammatory Diseases With Janus Kinase Inhibitors: A Consensus Statement. Ann Rheum Dis (2021) 80(1):71–87. doi: 10.1136/annrheumdis-2020-218398
Keywords: breast cancer, metastasis, interleukin-6, JAKs, STAT3, therapeutics
Citation: Manore SG, Doheny DL, Wong GL and Lo H-W (2022) IL-6/JAK/STAT3 Signaling in Breast Cancer Metastasis: Biology and Treatment. Front. Oncol. 12:866014. doi: 10.3389/fonc.2022.866014
Received: 30 January 2022; Accepted: 16 February 2022;
Published: 15 March 2022.
Edited by:
João Pessoa, University of Coimbra, PortugalReviewed by:
Giacomo Canesin, Beth Israel Deaconess Medical Center and Harvard Medical School, United StatesWenwen Du, First Affiliated Hospital of Soochow University, China
Copyright © 2022 Manore, Doheny, Wong and Lo. This is an open-access article distributed under the terms of the Creative Commons Attribution License (CC BY). The use, distribution or reproduction in other forums is permitted, provided the original author(s) and the copyright owner(s) are credited and that the original publication in this journal is cited, in accordance with accepted academic practice. No use, distribution or reproduction is permitted which does not comply with these terms.
*Correspondence: Hui-Wen Lo, aGxvQHdha2VoZWFsdGguZWR1