- 1Department of Clinical Immunology, Jagiellonian University Medical College, Cracow, Poland
- 2University Centre of Veterinary Medicine, Jagiellonian University - University of Agriculture, Cracow, Poland
Prostate cancer (PC) is the second most often diagnosed malignancy in men and one of the major causes of cancer death worldwide. Despite genetic predispositions, environmental factors, including a high-fat diet, obesity, a sedentary lifestyle, infections of the prostate, and exposure to chemicals or ionizing radiation, play a crucial role in PC development. Moreover, due to a lack of, or insufficient T-cell infiltration and its immunosuppressive microenvironment, PC is frequently classified as a “cold” tumor. This is related to the absence of tumor-associated antigens, the lack of T-cell activation and their homing into the tumor bed, and the presence of immunological cells with regulatory functions, including myeloid-derived suppressor cells (MDSCs), regulatory T cells (Treg), and tumor-associated macrophages (TAMs). All of them, by a variety of means, hamper anti-tumor immune response in the tumor microenvironment (TME), stimulating tumor growth and the formation of metastases. Therefore, they emerge as potential anti-cancer therapy targets. This article is focused on the function and role of MDSCs in the initiation and progression of PC. Clinical trials directly targeting this cell population or affecting its biological functions, thus limiting its pro-tumorigenic activity, are also presented.
Prostate Cancer—Epidemiology
Prostate cancer (PC) is the most common, after lung cancer, malignancy in men—in 2020, more than 1.4 million new cases of PC were diagnosed worldwide (1, 2). Advanced age, race, and ethnicities such as African descent and family history are well-established risk factors of PC (3–6). Additionally, a higher incidence of PC has been associated with a diet rich in saturated animal fat and red meat, low intake of fruits/vegetables, obesity, hyperglycemia, lack of physical activity, prostate inflammation, as well as exposure to chemicals or ionizing radiation (6–8). The most common genetic predispositions for PC development are related to aberrations of the PTEN tumor suppressor gene. Inactivation of PTEN by deletion or mutations is identified in ∼20% of primary PC and as many as 50% of advanced castration-resistant tumors (9). The role of the immune system and prostatitis in PC development was also confirmed, indicating that inflammatory mediators may promote prostatic carcinogenesis via inhibition of apoptosis, promotion of cell proliferation, and even loss of the tumor suppressor genes (10). Importantly, not only the local, prostate inflammation, but also systemic reaction associated with chronic inflammatory diseases, including asthma and allergies, are associated with the higher risk of PC (11).
Most of the patients develop a low-risk neoplasm (12); however, approximately 15% of men with localized PC present with high-risk tumors, which will progress, metastasize, and finally result in death (13). In men with advanced metastatic prostate cancer (mPC), hormonal–androgen deprivation therapy is a method of choice with a good response rate. In some patients, however, the mPC will evolve into metastatic castration-resistant prostate cancer (mCRPC) (14). While a radical prostatectomy may be beneficial for patients with high-risk PC (15), only multimodal treatment, including surgery, radiation, and systemic therapy, gives the best chance for a long-term progression-free outcome (13). Nowadays, immunotherapy options, including anti-PC vaccines, e.g., Sipuleucel-T (Provenge), and the use of immune checkpoint inhibitors (anti-CTLA-4 and anti-PD-1/PD-L1 monoclonal antibodies or antagonists) further improve the effectiveness of the PC treatment (16).
PC is often considered a “cold” tumor, meaning that due to the reduced or complete lack of T-cell infiltration, e.g., because of the missing tumor-associated antigens, lack of T-cell activation and their homing into the tumor bed, and local immunosuppression, it does not trigger a strong immune response. This term emphasizes the role of the immune system in PC progression (16, 17). Studies indicate that regulatory T cells (Tregs) and other cell populations, namely, myeloid-derived suppressor cells (MDSCs; attracted to TME by low-grade chronic inflammatory signals) and tumor-associated macrophages (TAMs) (17), are mainly responsible for the immunosuppression observed in PC (18). Among them, MDSCs emerge as potential therapeutic targets (19).
Myeloid-Derived Suppressor Cells—Their Origin and Activity
The term “myeloid-derived suppressor cells” has been used in the literature since 2007; however, the history of these cells dates back to the early 20th century, when it was shown that cancer is often accompanied by extra-medullary hematopoiesis (EMH) and neutrophilia (20, 21). These immature leukocytes were further characterized by their suppressive activity and called myeloid suppressor cells (MSC) (22). This term was further changed to MDSCs (22), and although current, the progress in resolution techniques, including a high-dimensional single-cell analysis, has raised concerns regarding the development and activation state of MDSCs (23); it is still accepted that MDSCs represent a heterogeneous population of immature myeloid cells, promptly expanding during pathological conditions, including infection, inflammation, and cancer (24). With respect to their origin, MDSCs have been divided into two main subsets—monocytic (Mo-MDSCs) and granulocytic or polymorphonuclear (PMN-MDSCs). Recently, a third population of the so-called early-stage MDSCs (e-MDSCs) was also described (25). In cancer, the accumulation of MDCSs is inseparably related to the production of pro-inflammatory mediators by the tumor microenvironment (TME), which activate and drive their suppressive activity (26). The immunosuppressive mechanisms developed by MDSCs are diverse and may include arginase-1 (ARG1) and inducible nitric oxide synthase (iNOS) activity; secretion of TGFβ, IL-10, and cyclooxygenase-2 (COX-2); and depletion of tryptophan by indoleamine 2,3-dioxygenase (IDO) (27). Although the immunosuppressive nature and the induction of antigen-specific T-cell tolerance is common for all the MDSCs subsets (28), they differ in the mechanism of action. In this context, Mo-MDSCs suppress T-cell response in both an antigen-specific and an unspecific manner, utilizing the mechanisms associated with iNOS activity and production of nitric oxide (NO) (29, 30). In contrast, PMN-MDSCs suppress immune response primarily in an antigen-specific manner, using the STAT3-mediated mechanisms of NADPH-oxidase and ARG1 activities (31). PMN-MDSCs store ARG1 in the granules and release it to the extracellular milieu, leading to the local depletion of L-arginine, affecting T-cell functionality. Both MDSCs subsets release ROS, which are essential for their immunosuppressive activity, and for retaining their undifferentiated status. Numerous studies confirmed the interplay between chronic inflammatory factors and expansion of MDSCs (24, 32). The transcription factor STAT3 plays a central role in the generation and functioning of MDSCs (33–35). Various cytokines, including IL-6, IL-1β, IL-10, GM-CSF, and VEGF, secreted mainly in the TME by tumor cells (26), are involved in the activation of pSTAT3. Conversely, chronic inflammation is associated with the initiation and progression of the tumor (10). In this context, chemokines and their receptors, e.g., CCL2/CCL12-CCR2, CXCL5/2/1-CXCR2, CCL3/4/5-CCR5, CCL15-CCR1, and CXCL8-CXCR1/2, are relevant for a rapid progression of PC and the recruitment of MDSCs (36, 37). PC patients were shown to have higher MDSCs infiltration than those with a benign prostate hyperplasia (38). Therefore, the role of inflammation in the development and expansion of MDSCs, and hence in PC progression, is unquestionable.
Expansion of MDSCs in PC
Studies with the use of PTEN KO murine PC model documented that lack of this gene was associated with upregulated inflammatory response (enhanced production of CSF-1 and IL-1β), and an extensive MDSCs tumor infiltration (39). Another mechanism involved in the recruitment of MDSCs in PC could be linked to the Hippo–YAP signaling. This pathway, relevant for the regulation of cell proliferation and apoptosis, is often deregulated in human solid tumors and associated with enhanced cancer cell proliferation (40). In PC, the hyperactivated Hippo–YAP signaling causes the upregulation of CXCL5 in cancer cells, which promotes the MDSCs recruitment via the CXCL5–CXCR2 axis (41, 42). The recruitment of MDSCs to the tumor mass may also benefit from the tumor-related hypoxia. This is supported by the observation that the hypoxia-targeted therapy may lead to a long-lasting decrease in the accumulation of MDSCs in the tumor (43). A significant role in the recruitment of MDSCs to PC has also been assigned to chromodomain helicase DNA-binding protein 1 (CHD1), an essential tumor suppressor (44). Its depletion was found in 29.7% of cases in African Americans, and 11.0% of European PC patients (45). It has been shown that CHD1 deficiency may recruit MDSCs via an IL-6-dependent mechanism (46). Interestingly, a positive correlation between CHD1 and CD15 expression (a surface marker of PMN-MDSCs) in PC was also documented (46).
A growing list of evidence suggests that miRNA carried by tumor-derived extracellular vesicles (TEVs) may also play a role in the generation of MDSCs in many types of cancer (47–49). Although there are no data confirming such a role of EV miRNA in PC, some miRNAs already shown as relevant in the induction of MDSCs in other cancers have also been considered for PC (50).
The crosstalk between MDSCs and the TME in PC is schematically presented in Figure 1.
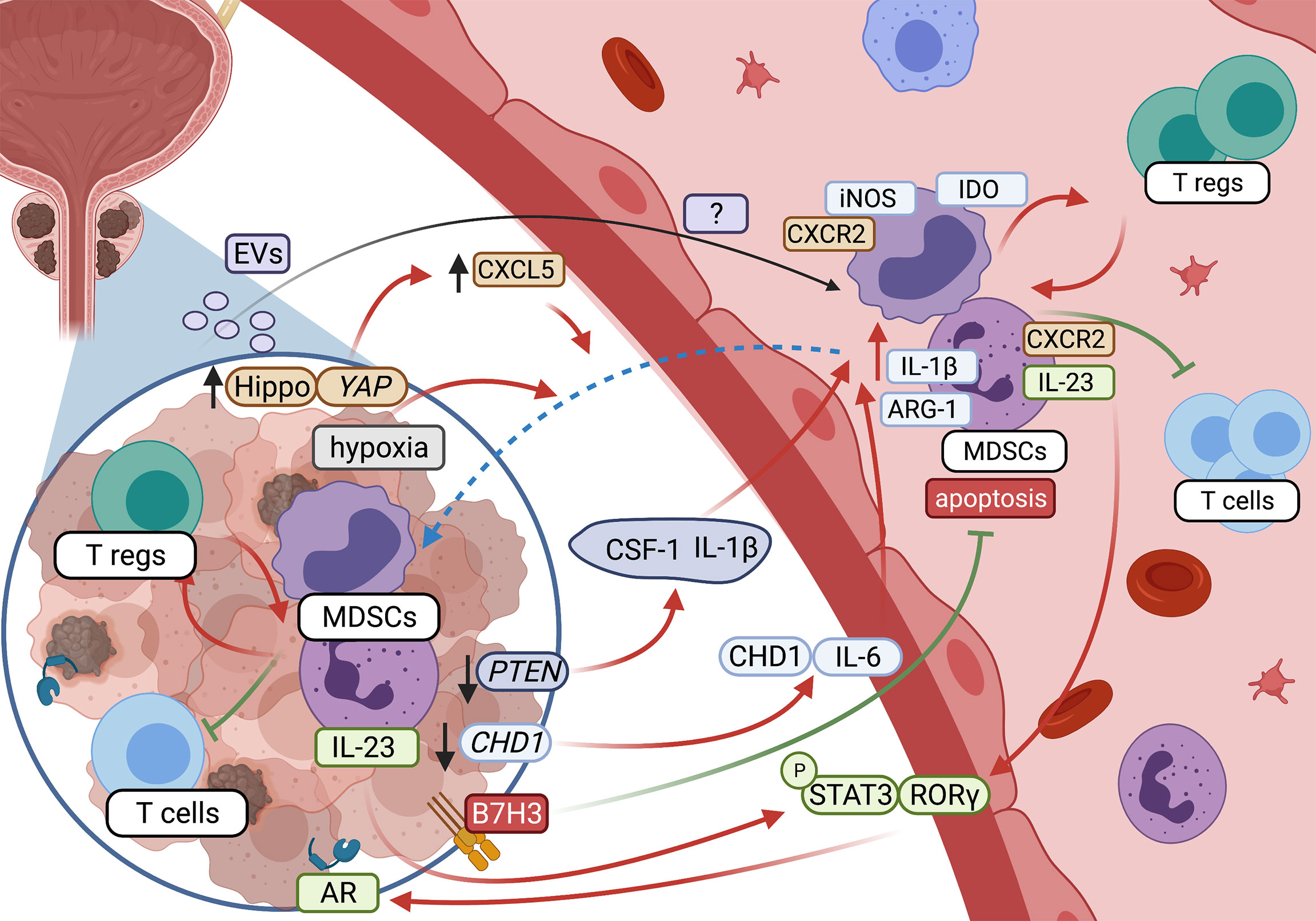
Figure 1 Crosstalk between MDSCs and tumor microenvironment in prostate cancer (created with BioRender.com).
Role of MDSCs in PC Development and Progression
In various cancers, the level of tumor-infiltrating MDSCs has been proposed as a prognostic marker (51, 52). In PC, however, such data are scarce and refer mainly to the PTEN mouse model (39). In contrast, there are observations that the MDSCs’ blood level could be a useful parameter for monitoring the disease burden in PC, allowing researchers to distinguish between metastatic cancer, localized PC, and cancer-free men (53). Additionally, circulating MDSCs correlate well with PSA level and metastasis (33, 54). The pivotal role of MDSCs in the development and progression of PC was further confirmed in randomized clinical studies showing that the increased level of MDSCs after the treatment is associated with the overall worse patients’ survival (55, 56). Moreover, in a mouse model of PC, the lung infiltration by MDSCs was associated with the formation of lung metastases (57). However, what type of MDSCs subpopulation is pivotal and prevalent in PC remains controversial, mainly due to the lack of reproducibility and standardization of such research. The work showing MDSCs as a negative prognostic marker in mCRPC indicates only blood Mo-MDSCs as relevant (58). Furthermore, in patients with mCRPC, a positive correlation between Mo-MDSCs and Treg cells has been described (58), suggesting a mutual positive feedback loop (59). Generally, most of the studies in PC have focused on Mo-MDSCs rather than on PMN-MDSCs (55, 58, 60). Even early reports on circulating immunosuppressive cells in patients with PC were concentrated on CD14+HLA-DRlow/- monocytes (54). This may result from the fact that Mo-MDSCs are more frequent in peripheral blood than PMN-MDSCs (61, 62). Another reason could be the fact that, in many studies, a cryopreserved material was used (63), affecting the recovery of PMN-MDSCs (64). Recently, Wen et al. documented infiltration of the primary prostate tumor by cells referred to as PMN-MDSCs (65); however, the markers used for their identification did not allow researchers to distinguish them from the population of tumor-associated neutrophils (TANs) (25). In this context, the phenotype definition of circulating blood PMN-MDSCs seems to be more reliable, but still, this should be further confirmed by functional tests that document the immunosuppressive nature of these cells (25).
Studies in PC showed that Mo-MDSCs and PMN-MDSCs are transcriptomically different (61), pointing out the ARG1 as typical for PMN-MDSCs (66) and iNOS or IDO for Mo-MDSCs (58, 60). Moreover, PMN-MDSCs can exert their immunosuppressive action also by the release of neutrophil elastase (NE), which was shown to stimulate the proliferation, migration, and invasion of cancer cells both in vitro and in vivo in a mouse model of PC (67, 68).
It is proposed that, in PC, the tumor-infiltrating PMN-MDSCs express upregulated IL-1β and IL-23a (66). Although the IL-1β-restrained antitumor immunity was described before for other tumors (69), the secretion of IL-23 by PMN-MDSCs so far has been documented only for PC. In this context, it was shown that IL-23 preserves the androgen receptor’s (AR) functionality, enabling survival and proliferation of PC in the androgen-deprived environment. The same mechanism is postulated as a driving force in the development of castration resistance (40). However, castration resistance may also be related to the secretion of IL-8 and subsequent tumor infiltration by PMN-MDSCs (66).
Targeting MDSCs in PC
Due to a lack of, or insufficient T-cell infiltration and immunosuppressive microenvironment in PC, there is a need to design new therapies that could “turn up the heat on the cold immune microenvironment” (17), to enhance the local anti-tumor immune response (16). Radiation per se has been found to activate the immune response (70); however, studies using the animal models of PC revealed that radiotherapy induces a rapid increase in the tumor-infiltrating MDSCs (71). Our previous studies showed that surgery or hormonal therapy alone did not reduce the level of circulating Mo-MDSCs in PC patients (62). In this context, in addition to the standard treatment, immunotherapy (72) or dietary strategies (73) are implemented, targeting cells with immunosuppressive potential, including MDSCs. One of the major challenges in targeting human MDSCs is their heterogeneous nature, e.g., differences in phenotype and mechanisms of suppression. A type of “universal” approach, covering the above aspects, may be the use of gemtuzumab ozogamicin, a calicheamicin-conjugated anti-CD33 humanized monoclonal antibody, already approved to treat a subset of patients with acute myeloid leukemia, which has also been highly effective against MDSCs in many solid tumors, including PC in vitro (61).
Clinically, MDSCs may be targeted by different approaches, including, e.g., inhibition of MDSCs expansion, MDSCs depletion, induction of their differentiation, functional inhibition, or multifactorial treatment. The clinical trials concerning all these potentially therapeutic strategies in PC have been described below Table 1.
Inhibition of MDSCs Expansion
Currently, there are three registered clinical trials, aiming at the inhibition of MDSCs expansion in PC. As mentioned, chemokines and their receptors are pivotal for the recruitment of MDSCs and the rapid progression of PC (36, 37); therefore, targeting the chemokine receptors or the use of chemokine inhibitors seems to be a promising form of immunotherapy in PC (74). One of the ongoing clinical trials (NCT03177187) seems to verify this hypothesis by using the CXCR2 antagonist AZD5069 in combination with enzalutamide—the androgen receptor’s antagonist in patients with mCRPC (75). An important additional factor associated with MDSCs expansion is VEGF (26); thus, administration of cabozantinib (a small-molecule inhibitor of tyrosine kinase receptor, including the VEFG pathway) followed by radical prostatectomy vs. prostatectomy alone (NCT03964337) is being tested in men with high-risk PC. Moreover, cabozantinib has already shown inhibitory effects on MDSCs (76). Another trial concerning dietary intervention, NCT03654638, is focused on soy bread, containing isoflavones, which were shown to reduce the level of pro-inflammatory cytokines and MDSCs (77).
MDSCs Depletion
MDSCs isolated from both mice and humans display elevated levels of STAT3, while inhibition of its pathway resulted in enhanced antitumor activity (28, 78). Circulating Mo-MDSCs maintain high levels of STAT3 until they reach the tumor, where hypoxia induces its rapid downregulation, causing differentiation of MDSCs to TAMs (79). STAT3 regulates the expression of the main factors of MDSCs activity, e.g., IDO, ARG1, IL-6, IL-10, IL-1β, and VEGF, among others, suggesting this pathway as an attractive therapeutic option (26). In this context, a fungal-derived pSTAT3 inhibitor, galiellalactone, was recently assessed for its ability to prevent PC-induced generation of MDSCs in vitro (53). In keeping with this, the clinical trial NCT03709550, aiming at testing decitabine (5-aza-2′-deoxycytidine), a hypomethylating agent with the ability to selectively deplete Mo-MDSCs, in mCRPC patients was implemented (80).
Inhibition of MDSCs Differentiation
Another therapeutic option involves a controlled differentiation of MDSCs towards the M1 anti-tumor macrophages with the use of curcumin (81). This approach will be considered in the recruiting clinical trial (NCT03769766). A similar approach will be used in the phase I clinical study in patients with biochemically recurrent PC, testing the effectiveness of the white button mushroom (WBM) extract containing β-glucan (NCT04519879). β-glucans, the most abundant carbohydrates found in yeast and mushrooms (82), may induce MDSCs differentiation to antigen-presenting cells, eliminating their suppressive abilities (83). The rationale for this concept was additionally grounded on the preclinical data showing that dietary WBM powder reduced not only the frequency of circulating MDSCs but also the level of prostate-specific antigen (PSA) (84).
Inhibition of MDSCs Induced Suppressive Circuits
There is also a possibility to inhibit some of the MDSCs-induced suppressive mechanisms operating in PC. One of such approaches is represented by a combination of abiraterone, a novel hormone therapy available for CRPC (85), and tildrakizumab (anti-IL-23 mAb) (NCT04458311), altering the production of IL-23 and therefore having a potential to target the MDSCs function specific for PC (41). In another clinical trial, a combination of ipatasertib (inhibitor of all three isoforms of protein kinase AKT, which blocks the PI3K/AKT signaling pathway—a key driver of cancer cell growth and proliferation in PC), atezolizumab (anti-PD-L1 monoclonal antibodies)—a checkpoint inhibitor on MDSCs (86), and docetaxel (NCT03673787) will be tested in patients with mCRPC. Currently, in Europe, there is one registered clinical trial focused on blocking the MDSCs function in PC patients (no. 2017-001857-14). It tests the combination of vinorelbine, a cytostatic drug, and two checkpoint inhibitors, durvalumab and tremelimumab, which are anti-PD-L1 and anti-CTLA-4 mAb, respectively.
Multifactorial Intervention: Inhibition of MDSCs Expansion and Blocking Their Suppressive Activity
Combinations of both the inhibition of MDSCs expansion and blocking their suppressive activity provide the opportunity for multifactorial interventions with potential better therapeutic effectiveness. One of such trials tests the combination of STAT3 inhibitor (AZD9150), a selective CXCR2 antagonist (AZD5069), and the PD-L1 inhibitor (MEDI4736) (no. 2015-002525-19), where each can inhibit either MDSCs expansion or function. Another drug combination that is being tested is gemcitabine and RQ-00000007 (grapiprant), where gemcitabine inhibits MDSCs expansion (87), while grapiprant—an inhibitor of PGE2-receptor—reduces the differentiation, expansion, and suppressive activities of Mo-MDSCs (88), confirming its role in MDSCs functioning (26).
Potential New Targets
Despite a wide scope of the ongoing clinical research, there are other available potential therapeutic options targeting MDSCs in PC. One, yet unexplored route, concerns the angiotensin-converting enzyme (ACE)–angiotensin pathway, where the overexpression of ACE in monocytic cells was shown to reduce the generation of MDSCs (89), while angiotensin was able to reduce the tumor malignancy in PC (90). Nowadays, during the SARS-CoV-2 pandemic, this pathway, however, takes on a quite different significance. However, other forms of angiotensin may impact the biological properties of PC cells by modulating inflammatory reaction, or even genes, including downregulation of HIF1a and upregulation of CDH-1 (91) expression, both associated with MDSCs recruitment. Another potential approach involves estrogen, used previously in PC therapy (92). The combined therapy, linking activation of estrogen receptor β (ERβ) and the checkpoint inhibitor anti-PD-1 mAb, diminishes MDSCs infiltration in mouse models of colorectal and breast cancer (93). Interestingly, apoptosis and/or differentiation of PC cells may be promoted during the ERβ activation (94). Additionally, studies confirmed the benefits of ERβ activation in androgen-dependent CRPC, decreasing the viability of the tumor cells (95). Also, ARG1 is a potential therapeutic target in PC, and its inactivation through STAT3 inhibition was already confirmed (34). The ongoing clinical trials aiming at targeting MDSCs may be a trigger for more frequent use of immunotherapy in combination with other forms of PC treatment.
Conclusion
Although the first observations reporting the negative role of MDSCs in antitumor responses in PC date back from the beginning of the 21st century, the last decade saw an upsurge of studies indicating their mechanisms of action and clinical relevance (96). Although several questions remain unanswered, the role of MDSCs in the development and progression of PC seems unquestionable, suggesting their potential as a therapeutic target. Hence, the implementation of the combination therapy, e.g., radiotherapy and immunotherapy, targeting both the tumor and MDSCs in PC seems crucial. Such therapy may increase the frequency of the abscopal response, which is a phenomenon associated with tumor shrinkage, occurring not only locally at the site of the treatment but also in other locations, where the tumor has already spread (97).
Author Contributions
IS wrote the draft version of the manuscript. JB revised and edited the final version of the manuscript. All authors contributed to the article and approved the submitted version.
Funding
The authors declare and acknowledge financial support from EU H2020-MSCA-RISE-2017 program - grant “CANCER” (GA 777682).
Conflict of Interest
The authors declare that the research was conducted in the absence of any commercial or financial relationships that could be construed as a potential conflict of interest.
Publisher’s Note
All claims expressed in this article are solely those of the authors and do not necessarily represent those of their affiliated organizations, or those of the publisher, the editors and the reviewers. Any product that may be evaluated in this article, or claim that may be made by its manufacturer, is not guaranteed or endorsed by the publisher.
Acknowledgments
Figure created with BioRender.com.
References
1. Sung H, Ferlay J, Siegel RL, Laversanne M, Soerjomataram I, Jemal A, et al. Global Cancer Statistics 2020: GLOBOCAN Estimates of Incidence and Mortality Worldwide for 36 Cancers in 185 Countries. CA: A Cancer J Clin (2021) 71:209–49. doi: 10.3322/caac.21660
3. Rebbeck TR. Prostate Cancer Genetics: Variation by Race, Ethnicity, and Geography. Semin Radiat Oncol (2017) 27:3. doi: 10.1016/J.SEMRADONC.2016.08.002
4. Pienta KJ, Esper PS. Risk Factors for Prostate Cancer. Ann Internal Med (1993) 118:793–803. doi: 10.7326/0003-4819-118-10-199305150-00007
5. Bostwick DG, Burke HB, Djakiew D, Euling S, Ho SM, Landolph J, et al. Human Prostate Cancer Risk Factors. Cancer (2004) 101:2371–490. doi: 10.1002/CNCR.20408
6. Dagnelie PC, Schuurman AG, Goldbohm RA, van den Brandt PA. Diet, Anthropometric Measures and Prostate Cancer Risk: A Review of Prospective Cohort and Intervention Studies. BJU Int (2004) 93:1139–50. doi: 10.1111/J.1464-410X.2004.04795.X
7. Kolonel LN. Fat, Meat, and Prostate Cancer. Epidemiol Rev (2001) 23:72–81. doi: 10.1093/OXFORDJOURNALS.EPIREV.A000798
8. Wolk A. Diet, Lifestyle and Risk of Prostate Cancer. Acta Oncol (Stockholm Sweden) (2005) 44:277–81. doi: 10.1080/02841860510029572
9. Jamaspishvili T, Berman DM, Ross AE, Scher HI, de Marzo AM, Squire JA, et al. Clinical Implications of PTEN Loss in Prostate Cancer. Nat Rev Urol (2018) 15:222–34. doi: 10.1038/NRUROL.2018.9
10. Jiang J, Li J, Zhang Y, Zhu H, Liu J, Pumill C. The Role of Prostatitis in Prostate Cancer: Meta-Analysis. PLos One (2013) 8:85179. doi: 10.1371/JOURNAL.PONE.0085179
11. Beckmann K, Russell B, Josephs D, Garmo H, Haggstrom C, Holmberg L, et al. Chronic Inflammatory Diseases, Anti-Inflammatory Medications and Risk of Prostate Cancer: A Population-Based Case-Control Study. BMC Cancer (2019) 19:1–9. doi: 10.1186/S12885-019-5846-3/TABLES/5
12. Punnen S, Cooperberg MR. The Epidemiology of High-Risk Prostate Cancer. Curr Opin Urol (2013) 23:331–6. doi: 10.1097/MOU.0B013E328361D48
13. McKay RR, Feng FY, Wang AY, Wallis CJD, Moses KA. Recent Advances in the Management of High-Risk Localized Prostate Cancer: Local Therapy, Systemic Therapy, and Biomarkers to Guide Treatment Decisions. ASCO Educ Book (2020) 40:241–52. doi: 10.1200/EDBK_279459
14. Shelley M, Harrison C, Coles B, Stafforth J, Wilt T, Mason M. Chemotherapy for Hormone-Refractory Prostate Cancer. Cochrane Database Syst Rev (2006) 4. doi: 10.1002/14651858.CD005247.PUB2
15. Ghodoussipour S, Cacciamani GE, Luis A, Abreu C. Radical Prostatectomy for High-Risk Prostate Cancer | Opinion: No. Int Braz J Urol (2019) 45(3):428–34. doi: 10.1590/S1677-5538.IBJU.2019.03.03
16. Fay EK, Graff JN. Immunotherapy in Prostate Cancer. Cancers (2020) 12:1–17. doi: 10.3390/CANCERS12071752
17. Stultz J, Fong L. How to Turn Up the Heat on the Cold Immune Microenvironment of Metastatic Prostate Cancer. Prostate Cancer Prostatic Dis (2021) 24:697–717. doi: 10.1038/s41391-021-00340-5
18. Sfanos KS, Bruno TC, Maris CH, Xu L, Thoburn CJ, Demarzo AM, et al. Phenotypic Analysis of Prostate-Infiltrating Lymphocytes Reveals TH17 and Treg Skewing. Clin Cancer Res (2008) 14:3254–61. doi: 10.1158/1078-0432.CCR-07-5164
19. Li X, Zhong J, Deng X, Guo X, Lu Y, Lin J, et al. Targeting Myeloid-Derived Suppressor Cells to Enhance the Antitumor Efficacy of Immune Checkpoint Blockade Therapy. Front Immunol (2021) 0:754196. doi: 10.3389/FIMMU.2021.754196
21. Talmadge JE, Gabrilovich DI. History of Myeloid Derived Suppressor Cells (MDSCs) in the Macro-and Micro-Environment of Tumour-Bearing Hosts. Nat Rev Cancer (2013) 13:739–52. doi: 10.1038/nrc3581
22. Gabrilovich DI, Bronte V, Chen S-H, Colombo MP, Ochoa A, Ostrand-Rosenberg S, et al. The Terminology Issue for Myeloid-Derived Suppressor Cells. Cancer Res (2007) 67:425–5. doi: 10.1158/0008-5472.CAN-06-3037
23. Hegde S, Leader AM, Merad M. MDSCs: Markers, Development, States, and Unaddressed Complexity. Immunity (2021) 54:875–84. doi: 10.1016/J.IMMUNI.2021.04.004
24. Sinha P, Chornoguz O, Clements VK, Artemenko KA, Zubarev RA, Ostrand-Rosenberg S. Myeloid-Derived Suppressor Cells Express the Death Receptor Fas and Apoptose in Response to T Cell-Expressed FasL. Blood (2011) 117:5381–90. doi: 10.1182/blood-2010-11-321752
25. Bronte V, Brandau S, Chen S, Colombo MP, Frey AB, Greten TF, et al. Recommendations for Myeloid-Derived Suppressor Cell Nomenclature and Characterization Standards. Nat Commun (2016) 7:1–10. doi: 10.1038/ncomms12150
26. Parker KH, Beury DW, Ostrand-Rosenberg S. Myeloid-Derived Suppressor Cells: Critical Cells Driving Immune Suppression in the Tumor Microenvironment. Adv Cancer Res (2015) 128:95–139. doi: 10.1016/bs.acr.2015.04.002
27. Shackleton EG, Ali HY, Khan M, Pockley GA, McArdle SE. Novel Combinatorial Approaches to Tackle the Immunosuppressive Microenvironment of Prostate Cancer. (2021) 13:1145. doi: 10.3390/CANCERS13051145
28. Marigo I, Bosio E, Solito S, Mesa C, Fernandez A, Dolcetti L, et al. Tumor-Induced Tolerance and Immune Suppression Depend on the C/Ebpβ Transcription Factor. Immunity (2010) 32:790–802. doi: 10.1016/j.immuni.2010.05.010
29. Kumar V, Patel S, Tcyganov E, Gabrilovich DI. The Nature of Myeloid-Derived Suppressor Cells in the Tumor Microenvironment. Trends Immunol (2016) 37:1–13. doi: 10.1016/j.it.2016.01.004
30. Gabrilovich DI. Myeloid-Derived Suppressor Cells. Cancer Immunol Res (2017) 5:3–8. doi: 10.1158/2326-6066.CIR-16-0297
31. Grzywa TM, Sosnowska A, Matryba P, Rydzynska Z, Jasinski M, Nowis D, et al. Myeloid Cell-Derived Arginase in Cancer Immune Response. Front Immunol (2020) 11:938. doi: 10.3389/fimmu.2020.00938
32. Gabrilovich DI, Ostrand-Rosenberg S, Bronte V. Coordinated Regulation of Myeloid Cells by Tumours. Nat Rev Immunol (2012) 12:253–68. doi: 10.1038/nri3175
33. Hossain DMS, Pal SK, Moreira D, Duttagupta P, Zhang Q, Won H, et al. TLR9-Targeted STAT3 Silencing Abrogates Immunosuppressive Activity of Myeloid-Derived Suppressor Cells From Prostate Cancer Patients. Clin Cancer Res (2015) 21:3771–82. doi: 10.1158/1078-0432.CCR-14-3145
34. Rébé C, Végran F, Berger H, Ghiringhelli F. STAT3 Activation: A Key Factor in Tumor Immunoescape. JAK-STAT (2013) 2:e23010. doi: 10.4161/JKST.23010
35. de Haas N, de Koning C, Spilgies L, de Vries IJM, Hato S V. Improving Cancer Immunotherapy by Targeting the STATe of MDSCs. Oncoimmunology (2016) 5. doi: 10.1080/2162402X.2016.1196312
36. Li BH, Garstka MA, Li ZF. Chemokines and Their Receptors Promoting the Recruitment of Myeloid-Derived Suppressor Cells Into the Tumor. Mol Immunol (2020) 117:201–15. doi: 10.1016/J.MOLIMM.2019.11.014
37. Maynard JP, Ertunc O, Kulac I, Valle JAB-D, de Marzo AM, Sfanos KS. IL8 Expression Is Associated With Prostate Cancer Aggressiveness and Androgen Receptor Loss in Primary and Metastatic Prostate Cancer. Mol Cancer Res (2020) 18:153–65. doi: 10.1158/1541-7786.MCR-19-0595
38. Sanaei MJ, Taheri F, Heshmati M, Bashash D, Nazmabadi R, Mohammad-Alibeigi F, et al. Comparing the Frequency of CD33 + Pstat3 + Myeloid-Derived Suppressor Cells and IL-17 + Lymphocytes in Patients With Prostate Cancer and Benign Prostatic Hyperplasia. Cell Biol Int (2021) 45:2086–95. doi: 10.1002/CBIN.11651
39. Garcia AJ, Ruscetti M, Arenzana TL, Tran LM, Bianci-Frias D, Sybert E, et al. Pten Null Prostate Epithelium Promotes Localized Myeloid-Derived Suppressor Cell Expansion and Immune Suppression During Tumor Initiation and Progression. Mol Cell Biol (2014) 34:2017. doi: 10.1128/MCB.00090-14
40. Johnson R, Halder G. The Two Faces of Hippo: Targeting the Hippo Pathway for Regenerative Medicine and Cancer Treatment. Nat Rev Drug Discovery (2014) 13:63. doi: 10.1038/NRD4161
41. Calcinotto A, Spataro C, Zagato E, di Mitri D, Gil V, Crespo M, et al. IL-23 Secreted by Myeloid Cells Drives Castration-Resistant Prostate Cancer. (2018) 559:363–9. doi: 10.1038/s41586-018-0266-0
42. Wang G, Lu X, Dey P, Deng P, Wu CC, Jiang S, et al. Targeting YAP-Dependent MDSCs Infiltration Impairs Tumor Progression. Cancer Discovery (2016) 6:80–95. doi: 10.1158/2159-8290.CD-15-0224
43. Jayaprakash P, Ai M, Liu A, Budhani P, Bartkowiak T, Sheng J, et al. Targeted Hypoxia Reduction Restores T Cell Infiltration and Sensitizes Prostate Cancer to Immunotherapy. J Clin Invest (2018) 128:5137–49. doi: 10.1172/JCI96268
44. Burkhardt L, Fuchs S, Krohn A, Masser S, Mader M, Kluth M, et al. CHD1 Is a 5q21 Tumor Suppressor Required for ERG Rearrangement in Prostate Cancer. Cancer Res (2013) 73:2795–805. doi: 10.1158/0008-5472.CAN-12-1342
45. Diossy M, Tisza V, Li H, Zhou J, Sztupinszki Z, Young D, et al. Increased Frequency of CHD1 Deletions in Prostate Cancers of African American Men is Associated With Distinct Homologous Recombination Deficiency Associated DNA Aberration Profiles. medRxiv (2021) 21251199. doi: 10.1101/2021.02.08.21251199
46. Zhao D, Cai L, Lu X, Liang X, Li J, Chen P, et al. Chromatin Regulator CHD1 Remodels the Immunosuppressive Tumor Microenvironment in PTEN-Deficient Prostate Cancer. Cancer Discov (2020) 10:1374–87. doi: 10.1158/2159-8290.CD-19-1352
47. Ren W, Zhang X, Li W, Feng Q, Feng H, Tong Y, et al. Exosomal miRNA-107 Induces Myeloid-Derived Suppressor Cell Expansion in Gastric Cancer. Cancer Manage Res (2019) 11:4023. doi: 10.2147/CMAR.S198886
48. Huber V, Vallacchi V, Fleming V, Hu X, Cova A, Dugo M, et al. Tumor-Derived microRNAs Induce Myeloid Suppressor Cells and Predict Immunotherapy Resistance in Melanoma. J Clin Invest (2018) 128:5517–30. doi: 10.1172/JCI98060
49. Daveri E, Vergani E, Shahaj E, Bergamaschi L, la Magra S, Dosi M, et al. microRNAs Shape Myeloid Cell-Mediated Resistance to Cancer Immunotherapy. Front Immunol (2020) 0:1214. doi: 10.3389/FIMMU.2020.01214
50. Cochetti G, Poli G, Guelfi G, Boni A, Egidi MG, Mearini E. Different Levels of Serum microRNAs in Prostate Cancer and Benign Prostatic Hyperplasia: Evaluation of Potential Diagnostic and Prognostic Role. OncoTargets Ther (2016) 9:7545–53. doi: 10.2147/OTT.S119027
51. Ai L, Mu S, Wang Y, Wang H, Cai L, Li W, et al. Prognostic Role of Myeloid-Derived Suppressor Cells in Cancers: A Systematic Review and Meta-Analysis. BMC Cancer (2018) 18:1–9. doi: 10.1186/s12885-018-5086-y
52. Zhang X, Fu X, Li T, Yan H. The Prognostic Value of Myeloid Derived Suppressor Cell Level in Hepatocellular Carcinoma: A Systematic Review and Meta-Analysis. PLos One (2019) 14:12:e0225327. doi: 10.1371/journal.pone.0225327
53. Chang G, Kang I, Shahabi A, Nadadur M, Athreya K, Suer D, et al. MDSCs Clinical Assay for Disease Surveillance in Prostate Cancer. J Clin Oncol (2015) 33:e16091–1. doi: 10.1200/jco.2015.33.15_suppl.e16091
54. Vuk-Pavlović S, Bulur PA, Lin Y, Qin R, Szumlanski CL, Zhao X, et al. Immunosuppressive CD14+HLA-DRlow/– Monocytes in Prostate Cancer. Prostate (2010) 70:443–55. doi: 10.1002/PROS.21078
55. Santegoets SJ, Stam AG, Lougheed SM, Gall H, Jooss K, Sacks N, et al. Myeloid Derived Suppressor and Dendritic Cell Subsets are Related to Clinical Outcome in Prostate Cancer Patients Treated With Prostate GVAX and Ipilimumab. J ImmunoTher Cancer (2014) 2:31. doi: 10.1186/S40425-014-0031-3
56. Takahashi R, Amano H, Ito Y, Eshima K, Satoh T, Iwamura M, et al. Microsomal Prostaglandin E Synthase-1 Promotes Lung Metastasis via SDF-1/CXCR4-Mediated Recruitment of CD11b+Gr1+MDSCs From Bone Marrow. Biomed Pharmacother (2020) 121:109581. doi: 10.1016/J.BIOPHA.2019.109581
57. Koga N, Moriya F, Waki K, Yamada A, Itoh K, Noguchi M. Immunological Efficacy of Herbal Medicines in Prostate Cancer Patients Treated by Personalized Peptide Vaccine. Cancer Sci (2017) 108:2326. doi: 10.1111/CAS.13397
58. Idorn M, Køllgaard T, Kongsted P, Sengeløv L, thor Straten P. Correlation Between Frequencies of Blood Monocytic Myeloid-Derived Suppressor Cells, Regulatory T Cells and Negative Prognostic Markers in Patients With Castration-Resistant Metastatic Prostate Cancer. Cancer Immunol Immunother (2014) 63:1177–87. doi: 10.1007/S00262-014-1591-2
59. Lee C-R, Kwak Y, Yang T, Han JH, Park S-H, Ye MB, et al. Myeloid-Derived Suppressor Cells Are Controlled by Regulatory T Cells via TGF-β During Murine Colitis. Cell Rep (2016) 17:3219–32. doi: 10.1016/j.celrep.2016.11.062
60. Hellsten R, Lilljebjörn L, Johansson M, Leandersson K, Bjartell A. The STAT3 Inhibitor Galiellalactone Inhibits the Generation of MDSCs-Like Monocytes by Prostate Cancer Cells and Decreases Immunosuppressive and Tumorigenic Factors. Prostate (2019) 79:1611–21. doi: 10.1002/PROS.23885
61. Fultang L, Panetti S, Ng M, Collins P, Graef S, Rizkalla N, et al. MDSCs Targeting With Gemtuzumab Ozogamicin Restores T Cell Immunity and Immunotherapy Against Cancers. EBioMedicine (2019) 47:235–46. doi: 10.1016/j.ebiom.2019.08.025
62. Siemińska I, Rychlicka-Buniowska E, Jaszczyński J, Palaczyński M, Bukowska-Strakova K, Ryś J, et al. The Level of Myeloid Derived-Suppressor Cells in Peripheral Blood of Patients With Prostate Cancerafter Various Types of Therapy. Polish J Pathol (2020) 71:46–54. doi: 10.5114/PJP.2020.95415
63. Trellakis S, Bruderek K, Hü J, Elian M, Hoffmann TK, Lang S, et al. Granulocytic Myeloid-Derived Suppressor Cells are Cryosensitive and Their Frequency Does Not Correlate With Serum Concentrations of Colony-Stimulating Factors in Head and Neck Cancer. Innate Immun (2013) 19:328–36 doi: 10.1177/1753425912463618
64. Grützner E, Stirner R, Arenz L, Athanasoulia AP, Schrödl K, Berking C, et al. Kinetics of Human Myeloid-Derived Suppressor Cells After Blood Draw. J Trans Med (2016) 14. doi: 10.1186/S12967-015-0755-Y
65. Wen J, Huang G, Liu S, Wan J, Wang X, Zhu Y, et al. Polymorphonuclear MDSCs are Enriched in the Stroma and Expanded in Metastases of Prostate Cancer. J Pathol: Clin Res (2020) 6:171. doi: 10.1002/CJP2.160
66. Lopez-Bujanda ZA, Haffner MC, Chaimowitz MG, Chowdhury N, Venturini NJ, Obradovic A, et al. Castration-Mediated IL-8 Promotes Myeloid Infiltration and Prostate Cancer Progression. bioRxiv (2019) 651083. doi: 10.1101/651083
67. Zhiguang X, Lerman I, Hammes SR. SAT-138 Neutrophil Elastase Promotes Proliferative Signals in Prostate Cells Through EGFR and DDR1. J Endocr Soc (2020) 4:1. doi: 10.1210/jendso/bvaa046.140
68. Lerman I, de la Luz Garcia-Hernandez M, Rangel-Moreno J, Chiriboga L, Pan C, Nastiuk KL, et al. Infiltrating Myeloid Cells Exert Protumorigenic Actions via Neutrophil Elastase. Mol Cancer Res (2017) 15:1138–52. doi: 10.1158/1541-7786.MCR-17-0003
69. Bruchard M, Mignot G, Derangère V, Chalmin F, Chevriaux A, Végran F, et al. Chemotherapy-Triggered Cathepsin B Release in Myeloid-Derived Suppressor Cells Activates the Nlrp3 Inflammasome and Promotes Tumor Growth. Nat Med (2012) 19:57–64. doi: 10.1038/nm.2999
70. Kaur P, Asea A. Radiation-Induced Effects and the Immune System in Cancer. Front Oncol (2012) 0:191. doi: 10.3389/FONC.2012.00191
71. Lin L, Kane N, Kobayashi N, Kono EA, Yamashiro JM, Nickols NG, et al. High-Dose Per Fraction Radiotherapy Induces Both Antitumor Immunity and Immunosuppressive Responses in Prostate Tumors. Clin Cancer Res (2021) 27:1505–15. doi: 10.1158/1078-0432.CCR-20-2293
72. May KF Jr., Gulley JL, Drake CG, Dranoff G, Kantoff PW. Prostate Cancer Immunotherapy. Clin Cancer Res (2011) 17:5233. doi: 10.1158/1078-0432.CCR-10-3402
73. Chen F, Zhao X. Prostate Cancer: Current Treatment and Prevention Strategies. Iranian Red Crescent Med J (2013) 15:279. doi: 10.5812/IRCMJ.6499
74. Poeta VM, Massara M, Capucetti A, Bonecchi R. Chemokines and Chemokine Receptors: New Targets for Cancer Immunotherapy. Front Immunol (2019) 10:379. doi: 10.3389/FIMMU.2019.00379
75. Koivisto CS, Parrish M, Bonala SB, Ngoi S, Torres A, Gallagher J, et al. Evaluating the Efficacy of Enzalutamide and the Development of Resistance in a Preclinical Mouse Model of Type-I Endometrial Carcinoma. bioRxiv (2019). doi: 10.1101/2019.12.06.86818
76. Kwilas AR, Ardiani A, Donahue RN, Aftab DT, Hodge JW. Dual Effects of a Targeted Small-Molecule Inhibitor (Cabozantinib) on Immune-Mediated Killing of Tumor Cells and Immune Tumor Microenvironment Permissiveness When Combined With a Cancer Vaccine. J Transl Med (2014) 12:1–15. doi: 10.1186/s12967-014-0294-y
77. Lesinski GB, Reville PK, Mace TA, Young GS, Ahn-Jarvis J, Thomas-Ahner J, et al. Consumption of Soy Isoflavone Enriched Bread in Men With Prostate Cancer Is Associated With Reduced Proinflammatory Cytokines and Immunosuppressive Cells. Cancer Prev Res (Phila) (2015)8:1036–44. doi: 10.1158/1940-6207.CAPR-14-0464
78. Moreira D, Adamus T, Zhao X, Su YL, Zhang Z, White SV, et al. STAT3 Inhibition Combined With CpG Immunostimulation Activates Antitumor Immunity to Eradicate Genetically Distinct Castration-Resistant Prostate Cancers. Clin Cancer Res (2018) 24:5948–62. doi: 10.1158/1078-0432.CCR-18-1277
79. Kumar V, Cheng P, Condamine T, Mony S, Languino LR, McCaffrey JC, et al. CD45 Phosphatase Inhibits STAT3 Transcription Factor Activity in Myeloid Cells and Promotes Tumor-Associated Macrophage Differentiation. Immunity (2016) 44:303. doi: 10.1016/J.IMMUNI.2016.01.014
80. Zhou J, Shen Q, Lin H, Hu L, Li G, Zhang X. Decitabine Shows Potent Anti-Myeloma Activity by Depleting Monocytic Myeloid-Derived Suppressor Cells in the Myeloma Microenvironment. J Cancer Res Clin Oncol (2019) 145:329–36. doi: 10.1007/s00432-018-2790-6
81. Tu SP, Jin H, Shi JD, Zhu LM, Suo Y, Lu G, et al. Curcumin Induces the Differentiation of Myeloid-Derived Suppressor Cells and Inhibits Their Interaction With Cancer Cells and Related Tumor Growth. Cancer Prev Res (2012) 5:205–15. doi: 10.1158/1940-6207.CAPR-11-0247
82. Murphy EJ, Rezoagli E, Major I, Rowan NJ, Laffey JG. β-Glucan Metabolic and Immunomodulatory Properties and Potential for Clinical Application. J Fungi (2020) 10:356. doi: 10.3390/jof6040356
83. Albeituni SH, Ding C, Liu M, Hu X, Luo F, Kloecker G, et al. Yeast-Derived Particulate β-Glucan Treatment Subverts the Suppression of Myeloid-Derived Suppressor Cells (MDSCs) by Inducing Polymorphonuclear MDSCs Apoptosis and Monocytic MDSCs Differentiation to APC in Cancer. J Immunol (2016) 196:2167–80. doi: 10.4049/jimmunol.1501853
84. Twardowski P, Kanaya N, Frankel P, Synold T, Ruel C, Pal SK, et al. A Phase I Trial of Mushroom Powder in Patients With Biochemically Recurrent Prostate Cancer: Roles of Cytokines and Myeloid-Derived Suppressor Cells for Agaricus Bisporus-Induced Prostate-Specific Antigen Responses. Cancer (2015) 121:2942–50. doi: 10.1002/cncr.29421
85. Tran C, Ouk S, Clegg NJ, Chen Y, Watson PA, Arora V, et al. Development of a Second-Generation Antiandrogen for Treatment of Advanced Prostate Cancer. Science (2009) 324:787–90. doi: 10.1126/SCIENCE.1168175
86. Weber R, Fleming V, Hu X, Nagibin V, Groth C, Altevogt P, et al. Myeloid-Derived Suppressor Cells Hinder the Anti-Cancer Activity of Immune Checkpoint Inhibitors. (2018) 9:1. doi: 10.3389/fimmu.2018.01310
87. Le HK, Graham L, Cha E, Morales JK, Manjili MH, Bear HD. Gemcitabine Directly Inhibits Myeloid Derived Suppressor Cells in BALB/c Mice Bearing 4T1 Mammary Carcinoma and Augments Expansion of T Cells From Tumor-Bearing Mice. Int Immunopharmacol (2009) 9:900–9. doi: 10.1016/J.INTIMP.2009.03.015
88. Lu W, Yu W, He J, Liu W, Yang J, Lin X, et al. Reprogramming Immunosuppressive Myeloid Cells Facilitates Immunotherapy for Colorectal Cancer. EMBO Mol Med (2021) 13:e12798. doi: 10.15252/EMMM.202012798
89. Shen XZ, Okwan-Duodu D, Blackwell WL, Ong FS, Janjulia T, Bernstein EA, et al. Myeloid Expression of Angiotensin-Converting Enzyme Facilitates Myeloid Maturation and Inhibits the Development of Myeloid-Derived Suppressor Cells. Lab Invest (2014) 94:536–44. doi: 10.1038/LABINVEST.2014.41
90. Domińska K, Okła P, Kowalska K, Habrowska-Górczyńska DE, Urbanek KA, Ochędalski T, et al. Angiotensin 1–7 Modulates Molecular and Cellular Processes Central to the Pathogenesis of Prostate Cancer. Sci Rep (2018) 8:1–12. doi: 10.1038/s41598-018-34049-8
91. Domińska K, Kowalska K, Anna Urbanek K, Ewa Habrowska-Górczy D, Och T, Wanda Piastowska Ciesielska A. The Impact of Ang-(1-9) and Ang-(3-7) on the Biological Properties of Prostate Cancer Cells by Modulation of Inflammatory and Steroidogenesis Pathway Genes. Int J Mol Sci Article (2020) 21:6227. doi: 10.3390/ijms21176227
92. Ockrim J, Lalani EN, Abel P. Therapy Insight: Parenteral Estrogen Treatment for Prostate Cancer - A New Dawn for an Old Therapy. Nat Clin Pract Oncol (2006) 3:552–63. doi: 10.1038/NCPONC060
93. Huang S, Zhou N, Zhao L, Gimple RC, Ahn YH, Zhang P, et al. Pharmacological Activation of Estrogen Receptor Beta Overcomes Tumor Resistance to Immune Checkpoint Blockade Therapy. iScience (2020) 23:101458. doi: 10.1016/J.ISCI.2020.101458
94. di Zazzo E, Galasso G, Giovannelli P, di Donato M, Castoria G. Estrogens and Their Receptors in Prostate Cancer: Therapeutic Implications. Front Oncol (2018) 8:2. doi: 10.3389/FONC.2018.00002
95. Gehrig J, Kaulfuß S, Jarry H, Bremmer F, Stettner M, Burfeind P, et al. Prospects of Estrogen Receptor β Activation in the Treatment of Castration-Resistant Prostate Cancer. Oncotarget (2017) 8:34971–9. doi: 10.18632/ONCOTARGET.16496
96. Sanaei MJ, Salimzadeh L, Bagheri N. Crosstalk Between Myeloid-Derived Suppressor Cells and the Immune System in Prostate Cancer: MDSCs and Immune System in Prostate Cancer. J Leukoc Biol (2020) 107:43–56. doi: 10.1002/JLB.4RU0819-150RR
Keywords: prostate cancer, myeloid-derived suppressor cells, immunosuppression, immunotherapy, anti-tumor immune response
Citation: Siemińska I and Baran J (2022) Myeloid-Derived Suppressor Cells as Key Players and Promising Therapy Targets in Prostate Cancer. Front. Oncol. 12:862416. doi: 10.3389/fonc.2022.862416
Received: 25 January 2022; Accepted: 06 June 2022;
Published: 04 July 2022.
Edited by:
Hua Li, Henry M. Jackson Foundation for the Advancement of Military Medicine (HJF), United StatesReviewed by:
Suman Kapur, Birla Institute of Technology and Science, IndiaNader Bagheri, Shahrekord University of Medical Sciences, Iran
Copyright © 2022 Siemińska and Baran. This is an open-access article distributed under the terms of the Creative Commons Attribution License (CC BY). The use, distribution or reproduction in other forums is permitted, provided the original author(s) and the copyright owner(s) are credited and that the original publication in this journal is cited, in accordance with accepted academic practice. No use, distribution or reproduction is permitted which does not comply with these terms.
*Correspondence: Jarek Baran, bWliYXJhbkBjeWYta3IuZWR1LnBs