- 1Graduate Program in Translational Biology, Medicine and Health, Virginia Tech, Roanoke, VA, United States
- 2Department of Biomedical Sciences and Pathobiology, Virginia Tech, Blacksburg, VA, United States
- 3Institute for Critical Technology and Applied Sciences, Virginia Tech, Blacksburg, VA, United States
- 4Department of Biomedical Engineering and Mechanics, Virginia Tech, Blacksburg, VA, United States
- 5Department of Mechanical Engineering, Virginia Tech, Blacksburg, VA, United States
Advancements in medical sciences and technologies have significantly improved the survival of many cancers; however, pancreatic cancer remains a deadly diagnosis. This malignancy is often diagnosed late in the disease when metastases have already occurred. Additionally, the location of the pancreas near vital organs limits surgical candidacy, the tumor’s immunosuppressive environment limits immunotherapy success, and it is highly resistant to radiation and chemotherapy. Hence, clinicians and patients alike need a treatment paradigm that reduces primary tumor burden, activates systemic anti-tumor immunity, and reverses the local immunosuppressive microenvironment to eventually clear distant metastases. Irreversible electroporation (IRE), a novel non-thermal tumor ablation technique, applies high‐voltage ultra-short pulses to permeabilize targeted cell membranes and induce cell death. Progression with IRE technology and an array of research studies have shown that beyond tumor debulking, IRE can induce anti-tumor immune responses possibly through tumor neo-antigen release. However, the success of IRE treatment (i.e. full ablation and tumor recurrence) is variable. We believe that IRE treatment induces IFNγ expression, which then modulates immune checkpoint molecules and thus leads to tumor recurrence. This indicates a co-therapeutic use of IRE and immune checkpoint inhibitors as a promising treatment for pancreatic cancer patients. Here, we review the well-defined and speculated pathways involved in the immunostimulatory effects of IRE treatment for pancreatic cancer, as well as the regulatory pathways that may negate these anti-tumor responses. By defining these underlying mechanisms, future studies may identify improvements to systemic immune system engagement following local tumor ablation with IRE and beyond.
Introduction
The advances of cancer treatments have been staggering in the last 50 years. From advancing chemotherapeutics to cytokine and antibody treatments, many cancer patients have seen a significant increase in survival rates. However, some cancers have continued to be relatively unaltered by these new treatments (1–3). Dense tissue in later-stage cancers, as well as several other factors, can limit tumor penetration and response of drugs (4). In the past decade, immunotherapeutic developments, including checkpoint inhibitor monoclonal antibodies (mAbs) and adoptive cellular therapy, have shown promise in treating many cancer patients with advanced-stage tumors (5, 6). However, cancers such as pancreatic adenocarcinoma continue to have severely low survival rates even with new immunotherapeutic options in part due to the immunosuppressive tumor microenvironment that shields the tumors from the immune system’s attempts to identify and target the malignancy (7, 8). Pancreatic cancer has a low incidence rate (3.2% of all new cancer cases) but high death rate (7.9% of all cancer related death), making it the third-highest cause of cancer-related deaths in the United States (9). Additionally, pancreatic cancer is projected to become the second-highest cause of cancer-related deaths by 2030 (10, 11). It is often diagnosed late in the disease progression, which severely limits treatment options. Furthermore, due to close proximity to critical structures, the standard-of-care surgery is only available to 20% of total diagnosed patients.
These limitations have led to a rush of targeted ablation modalities to circumvent the challenges faced by surgeons and oncologists. Many of these modalities use thermal effects that burn or freeze the tumor. However, these technologies can lead to adverse effects and limited application by causing off-target damage to nearby healthy tissues, and heat sink effect making them unsuitable for many cancer patients (12, 13). The use of thermal energy can also denature proteins, which can limit immune signaling by destroying potential damage signals and tumor antigens (14, 15). Non-thermal ablation modalities, such as those utilizing electroporation, greatly limit damage to surrounding healthy tissues (16, 17). Moreover, immunogenic signaling could be preserved, allowing for damage signals and viable antigens to remain intact and elicit an anti-tumor immune response after treatment (18, 19). Preclinical and clinical reports of potential immunological effects to the primary treatment site and even metastatic lesions have led to many recent investigations on the impact of these electroporation-based modalities, such as irreversible electroporation (IRE), high-frequency irreversible electroporation (H-FIRE), electrochemotherapy (ECT), and nanosecond pulse electric fields (nsPEFs) on the tumor microenvironment and the immune system. Indeed, several reports suggested an abscopal-like effect where treatment of the primary tumor by electroporation-based modalities resulted in decreased tumor size and reduced distant metastases (20–22).
A plethora of signaling pathways are involved in the formation and progression of pancreatic cancer. Many of these pathways involve cell death/survival and immune system activation/suppression and have been observed after IRE treatment. Elucidating these pathways, which are poorly understood in the context of IRE ablation of pancreatic cancer, could potentially change its clinical application. Such discoveries could inform well-rounded treatment plans that limit IRE side effects (tumor lysis syndrome, organ damage from inflammation, and developing autoimmune diseases) and work alongside chemotherapies or immunotherapies. It would also provide much-needed information on how IRE impacts the tumor microenvironment and potential improvements to the treatment modality itself. A better understanding of these mechanisms can thus lead to improved patient outcomes and prolonged survival. In this review, we discuss pathways involved in cell death, survival, immune system activation, and immune suppression after IRE treatment.
IRE Ablation Releases Tumor Antigen
Electroporation-based ablations include many subtypes of ablative strategies, from ECT to nsPEFs (Figure 1). These different treatments require adjustments of pulsed electric fields (PEFs) to generate the desired tumor ablation. By adjusting the polarity, duration, electric field strength (V/cm), and number of pulses applied, electroporation can temporarily or permanently permeabilize cell membranes. European Standard Operating Procedure on Electrochemotherapy (ESOPE) multicenter trial has standardized Electrochemotherapy treatment parameters which was first published in 2006 and recently updated (23–25). The ESOPE protocol (8 rectangular pulses, 1000 V/cm, 100 microseconds) specifies the standard pulsing requirements on human patients. In recent years, IRE has been integral to many clinical trials targeting notoriously difficult-to-treat malignancies, including liver and pancreatic cancers (26–29). The significant results of recent clinical trials have propelled IRE into Phase III clinical trials in pancreatic cancer (clinicaltrials.gov, ID: NCT03899636) (30). IRE utilizes microsecond pulsed electric fields. Unlike ECT, IRE increases the number of applied pulses so that so that cells cannot recover from the membrane permeabilization and induce cell death through a disruption in homeostasis (31). IRE is often compared to thermal ablation treatments, but it is normally applied non-thermally and thereby reduces the risk of healthy tissue damage (16) and spares critical structures (29, 32).
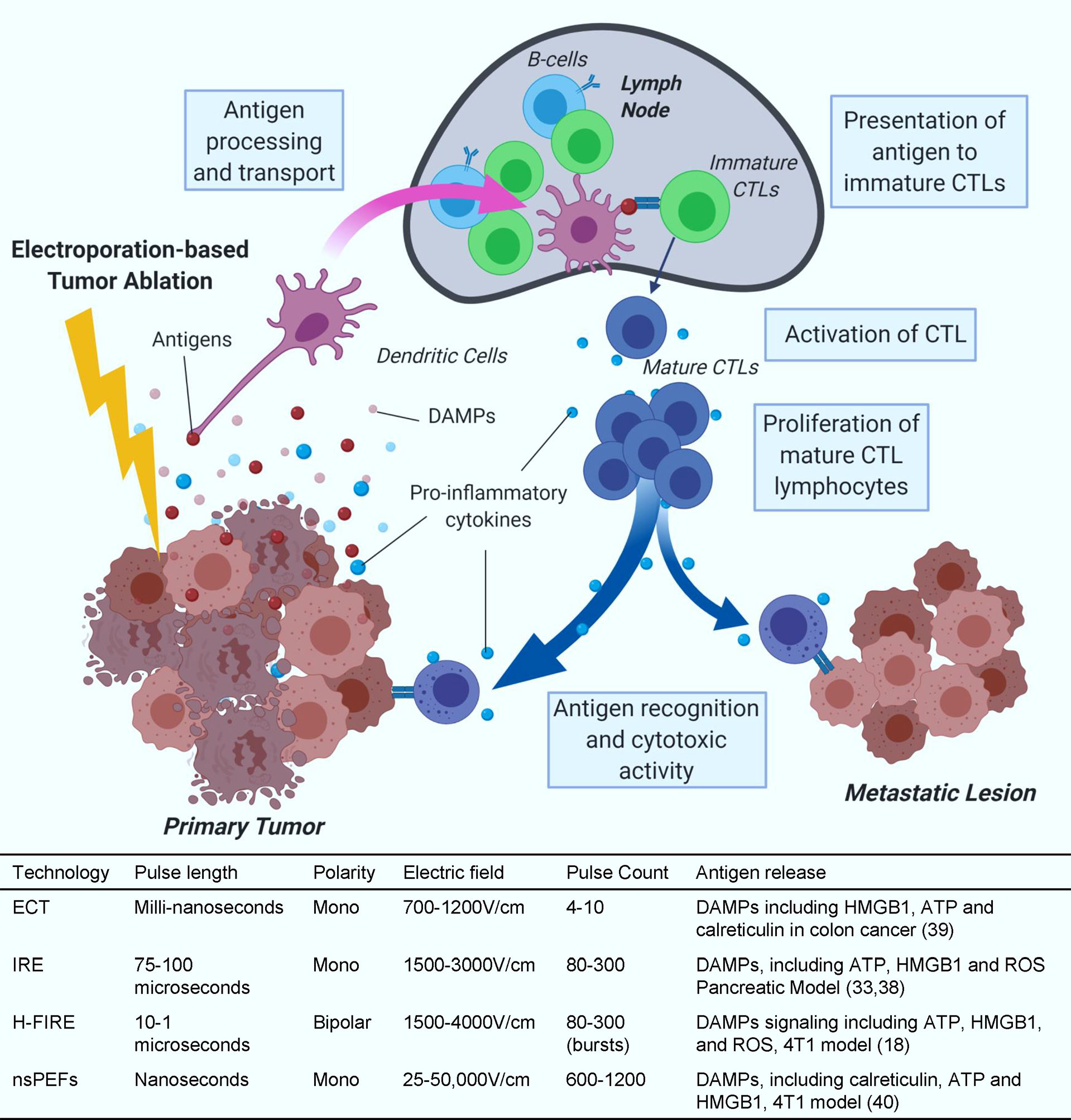
Figure 1 Antigen release and presentation. Collection and presentation of antigens by dendritic cells to cytotoxic T-cells (CTLs) and subsequent CTL activity after IRE treatment. Treatment parameter value ranges are based on commonly reported parameters in literature for in vivo preclinical and clinical applications. These ranges are not definite and applications outside of these ranges can occur.
IRE treatment induces different types of cell death, such as apoptosis and necrosis in pancreatic cancer cell lines (33). Treating with next-generation IRE, known as H-FIRE, induces apoptosis, necrosis and pyroptosis in liver and breast cancer (18, 34). After IRE treatment, cytoplasmic and antigenic materials are released through the permanent damage of the cell membrane and lead to different types of cell death. These findings were recently summarized by Brock et al. (35). Apoptosis is more controlled and promotes a weak immune response due, in part, to minimal debris and antigen released after programmed phagocytosis (36). In contrast, necrosis involves rapid accidental or mechanical lytic cell death that leads to the release of large amounts of damage-associated molecular patterns (DAMPs). Necrosis-released DAMPs can activate the innate immune system, induce inflammation, and recruit immune cells to the local tumor site. Pyroptosis is a tightly regulated inflammatory form of programmed cell death distinguished from necrosis by the cleavage and activation of Caspase-1 and Caspase-11 (37). During pyroptosis, a substantial quantity of DAMPs, including adenosine triphosphate (ATP), High mobility group box protein 1 (HMGB1), reactive oxygen species (ROS), and different potent proinflammatory cytokines are released, including IL-1β and IL-18 (38). IRE treatment to KrasLSL-G12D-p53LSL-R172H-Pdx-1-Cre (KPC) pancreatic cancer cells produces DAMPs validated by the production of ATP (33). Likewise, H-FIRE treatment has been shown to upregulate several DAMPs such as ATP, HMGB1, and ROS (18). ECT promotes LCs migration from the tumor to draining lymph nodes and pDCs and dDCs recruitment at the site of the lesion (39). Furthermore, ECT and nsPEF have also been shown to release several DAMPs including ATP, HMGB1, and calreticulin in different cancer models (40, 41). Although several studies have shown positive immune response after IRE for pancreatic cancer (22, 38, 42–44), more research needs to be done to determine the immune response after ECT due to different cell death pathways.
It is well established that DAMPs including HMGB1, heat shock protein (HSP), and antigens released via immunogenic cell death can activate the immune system against specific cancer cells (45, 46). It is evident that IRE causes immunogenic cell death and helps release tumor antigens which should, in turn, activate systemic anti-tumor immunity against pancreatic cancer and change the tumor microenvironment (Figure 1). By improving the microenvironment, the tumor is accessible to anti-tumor leukocytes such as neutrophils, macrophages, natural killer (NK) cells, T helper 1 cells, and CTLs (47–50). Indeed, many cancer patients show an increase in survival outcomes when more activated immune cells and less immune suppressive cells are observed (24, 28, 51–55),. These findings indicate the importance of monitoring and altering the immune system activation in cancer patients to improve survival.
Released Tumor Antigen by IRE Is Presented by APCs to Activate T Cells
Dendritic cells, macrophages, and B cells act as antigen-presenting cells (APCs) through interactions with T cells to link innate and adaptive immune responses. APCs display tumor antigens on the cell surface through major histocompatibility complexes to control the activation, differentiation, and effector functions of T cells. Electroporation-based ablation modalities do not use thermal energy to induce cell death and are believed to preserve viable tumor antigens that can be presented by APCs to T cells to activate systemic antitumor immunity (Figure 1). The lack of thermal energy is thought to preserve optimal antigen structure, size, and confirmation for APCs to present. When APCs encounter tumor antigens, they activate naïve T cells either by moving to nearby lymph nodes or locally in the tissue. Mature and activated T cells proliferate and migrate to the local tumor site and circulate systemically (56).
Immunocompetent mice demonstrate enhanced local and systemic anti-tumor efficacy following electroporation compared to immunodeficient mice, which indicates involvement of the immune system in the reduction of tumor burden by IRE treatment (57). We have recently shown that H-FIRE and IRE treated glioblastoma cells can activate CD8+ cytotoxic T cells (CTL) when cultured with CD4+ helper T cells and antigen-presenting dendritic cells (58). A recent report shows that IRE treatment using a mouse model of orthotopic pancreatic cancer resulted in an increased number of cytotoxic CD8+ T cells and memory T cells in the spleen, lymph nodes, and tumor, and induced an abscopal like effect through the synthesis and secretion of DAMPs (22). A significant increase in the CD8+ T cells and macrophages in the tumor has been noted after IRE and nsPEF treatment (59, 60). Macrophage and pan-T cell infiltration has been found from 6 hours to 14 days after IRE treatment (61, 62). A recent review compiled a timeline to show immune cell types and their upregulation or downregulation in the pancreatic tumor site after IRE treatment from both mouse models and human patients (43).
A murine pancreatic tumor model showed that after IRE treatment, there is evidence for antigen release and an increase in T cells in the lymph nodes and activated T cell infiltration in the local tumor site (22). However, direct evidence that tumor antigens released by IRE treatment then processed by APCs and their migration to the lymph nodes are not established. Furthermore, in situ T cell responses in the local tumor after IRE treatment are through antigen-mediated activation is not well demonstrated and requires further investigation.
T Cell-Mediated Cytokine Expression Pathway
Upon initial contact with tumor antigen, activated CD8+ cytotoxic T cells express a plethora of cytokines based on their subtypes and exposure to polarizing cytokines (63) including interferon gamma (IFNγ), Granzyme B, and Perforin (64–66). In a study with 34 locally advanced pancreatic cancer (LAPC) patients, significant upregulation was detected in the blood for IL-2, IL-6, and IL-10 after IRE treatment (62). In another study with 79 Stage III/IV pancreatic cancer patients, IRE treatment along with allogeneic natural killer cell therapy was tested and demonstrated that IRE alone and IRE with NK cell therapy increases IFNγ expression (67). In a murine model of pancreatic cancer, IRE treatment increased IFNγ expression compared to the sham control procedure (33). In an osteosarcoma rat model, IRE induced IFNγ expression in the serum (68). These findings indicate predominantly pro-inflammatory cytokine expression after electroporation-based tumor ablation treatment, presumably by activated lymphocytes.
IFNγ is primarily produced by lymphocyte populations, such as NK cells, innate lymphoid cells, T helper 1 (TH1) cells, and CTLs. For different cell types, the signaling can initiate through pattern recognition receptors, T cell receptors, and IFNγR. Stimulation of any of these receptors triggers the recognized Janus kinase (JAK) signal transducer and activator of transcription (STAT) signaling pathway (Figure 2). IFNγR ligand binding results in the initiation of JAK1 and JAK2 receptor association and activation followed by phosphorylation and activation of STAT1. Activated STAT1 moves to the nucleus, binds to the GAS site, and starts the transcription of interferon-stimulated genes (ISGs). ISGs not only encode cytokines and chemokines but also phagocytic receptors and antigen-presenting molecules (Figure 2). It is apparent that IRE produces tumor antigens that elicit an immune response, specifically via APCs that present the tumor antigens to IFNγ-expressing T cells. These activated T cells then kill tumor cells directly or activate alternative killing mechanisms, such as macrophage activation. However, the relationship between antigen presentation, T cell activation, and cytokine expression has not been fully elucidated in the context of IRE. We suggest this interface reveals an exciting co-therapy target, such as enhancing the release and subsequent presentation of a specific antigen and can also inform the timing of co-therapy application.
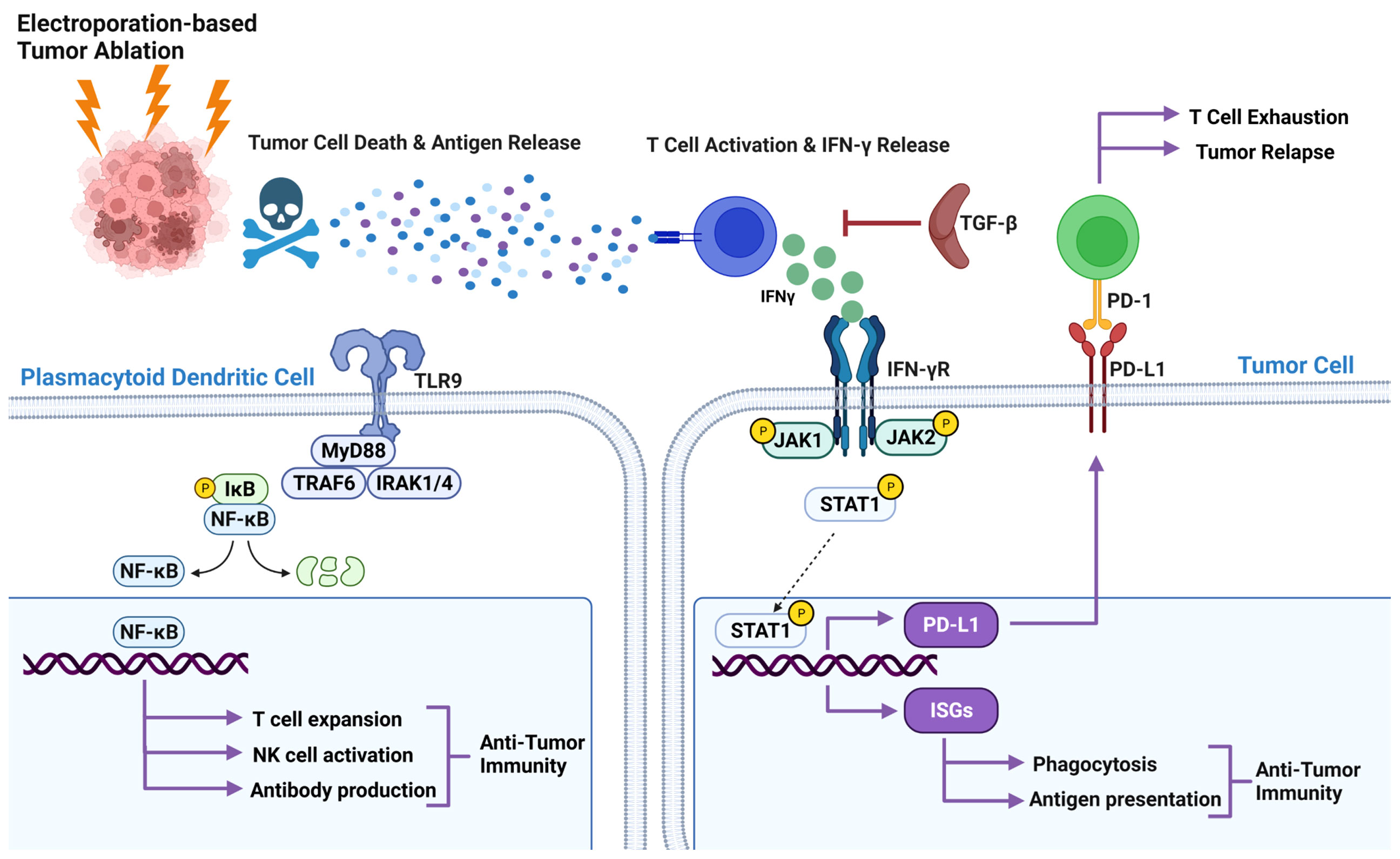
Figure 2 Pathways involved in the IRE treatment of pancreatic cancer. Released antigen after IRE treatment activates CTL and induces IFNγ expression. Binding IFNγ to IFNR recruit and phosphorylate JAK1, and JAK2 activates STAT1 by phosphorylation results in translocation of STAT1 into the nucleus. In the nucleus STAT1 binds to the GAS site and starts the transcription of interferon-stimulated genes (ISGs). ISGs not only encode cytokines and chemokines, but also phagocytic receptors and antigen-presenting molecules. IFNγ also induces PD-L1 expression by tumor cells through the JAK/STAT pathway. TLR9 agonists promote antitumor immunity through NK-κB signaling.
IFNγ Mediated PD1/PD-L1 Expression and CTL Inhibition Pathway
Unrestrained immune responses to tumor antigens can cause excessive inflammatory tissue damage and autoimmune diseases, therefore, immune homeostasis is critical for host survival. To maintain this homeostasis, the extent of the immune response is controlled by a balance between co-stimulatory and inhibitory signals of immune checkpoints. These checkpoints are often hijacked by tumor cells to evade the immune system. In a mouse model of pancreatic cancer, IFNγ expression by splenocytes after IRE and PD-L1 expression by tumor cells after IFNγ treatment has been demonstrated (69). An increase in CD4+PD1+ and CD8+PD1+ T cells 2 weeks post-IRE were found in the blood of all 10 LAPC patients (44). Enhanced antitumor efficacy of IRE and anti-PD1 immune checkpoint blockade in a murine orthotopic pancreatic adenocarcinoma (PDAC) model (KRAS model) were found where blocking of CD8a negates the benefit of IRE and blocking of CTLA4 did not enhance the efficacy of IRE. These results denote the importance of retaining CTL’s anti-tumor activity by targeting the right checkpoint molecule (38). IFNγ mediated PD-L1 expression is also regulated by JAK/STAT pathway (Figure 2), and emphasis has been put on why anti-PD-L1 immunotherapy might not work if IFNγ mediated PD-L1 is not expressed (70). IFNγ expression after IRE treatment due to T cell activation and PD1 by T cells and PD-L1 by tumor cells have been shown, but it is poorly understood what pathway is being activated and requires further investigation.
Fascinating genetic and biochemical results support crucial roles for JAK1, JAK2, STAT1, and ISGs in facilitating cellular IFNγ responses. The JAK-STAT pathway is also important in IFNγ function, as seen in host defense against pathogens, inflammatory and immune responses, tissue damage, and tumor immunosurveillance. In the context of pancreatic tumors treated with IRE, the IFNγ–JAK–STAT1–ISG pathway, immune functions of ISGs, and feedback inhibition of this pathway pose some key questions to be answered. Many components of this pathway are interlinked. For example, antigens released through IRE treatment activate T cells and produce IFNγ, and IFNγ can induce itself and/or PD-L1 presumably through JAK–STAT1–ISG pathway. IFNγ stimulates its own production through a positive feedback loop and induces PD-L1 through a negative feedback loop, which may be regulated through spacial, temporal and cell type dependent manner. This complex interaction is not yet fully defined in the context of IRE and pancreatic cancer. Hence, comprehensive understanding of this pathway might improve development of co-therapy targets for IRE.
TGF-β Pathway in Pancreatic Cancer and IRE
The transforming growth factor-β (TGF-β) pathway mediates diverse cellular processes and is a major contributor to cancer initiation and progression. In pancreatic cancer, TGF-β plays a paradoxical role as both a tumor suppressor and a tumor promoter. TGF-β family members are upregulated in pancreatic cancer, and increased expression of type II TGF-β receptor is linked with reduced survival in pancreatic cancer patients (71). Intriguingly, in the rabbit VX-2 breast cancer model, it was found that IRE improved the antitumor immune response by lowering the plasma levels of soluble interleukin-2 receptor (sIL-2R) and TGF-β1 (43). While TGF-β signaling was upstream of many crucial signaling pathways in pancreatic cancer, no significant impact of IRE treatment on TGF-β signaling was found in microarray analysis (23). However, microarray analysis was performed only on samples collected 24 hours post-IRE treatment, but the TGF-β signaling dynamics are dependent on many factors and require comprehensive investigation (72).
TGF-β signaling is important in pancreatic cancer and has been actively or passively implicated after IRE treatment but requires further investigation to fully interpret the immunosuppressive role of TGF-β. Common Treg and MDSC cells are subsets of suppressor cells known to have a role in tumor immunology. Treg1 (CD4+CD25−DX5+LAG-3+FoxP3−) is a subset of T regs and have been found to secrete high amounts of IL-10 and TGF-β. TGF-β and IL-10 expression decrease synthesis of IFNγ (Figure 2) and TNF-α by pro-inflammatory CD4 T cells, and in turn reduce tumor-specific cytotoxicity of CTLs, prevent activities of dendritic cells and NK cells, and result in tolerance to tumor cells (73–75). Reduction of Tregs after IRE treatment in LAPC patients has been observed (44). Previous studies indicated that MDSC mediate development of Treg cells in a TGF-β-dependent manner (76, 77). MDSCs remained the same on day 2 post-treatment with Nano-Pulse stimulation but significantly decreased on day 7 in LAPC patients (78). In agreement with the reduction of Tregs, IRE along with PD1 blockade and TLR7 agonist decreased MDSC levels on day 7 (21). This indicates the importance of elucidating underlying mechanism involving TGF-β, Tregs and MDSCs.
TLR3/TLR9 Pathway in Pancreatic Cancer and IRE
Toll like receptor signaling recognize a wide variety of pathogen associated molecular patterns (PAMPs) and activate subsequent immune signaling. CpG and IMO-2125 are TLR9 agonists. CpG motifs are considered PAMPs and can act as an agonist of TLR9 when unmethylated. When CpG binds to TLR9, it triggers a conformational shift in the receptor causing MyD88 recruitment and activation of signaling pathways downstream, culminating in NF-κB activation (79) to initiate a cascade of innate and adaptive immune responses (Figure 2). TLR9 agonists (CpG, IMO-2125) activate plasmacytoid dendritic cells to secrete type I interferon and to express increased levels of co-stimulatory molecules such as CD80 and CD86. This is thought to induce a variety of secondary effects, including secretion of cytokines/chemokines, activation of natural killer (NK) cells, and expansion of T-cell populations (80, 81). A humoral immune response is also initiated as TLR9 agonists enhance differentiation of B cells into antibody-secreting plasma cells, potentially promoting antibody-dependent cellular cytotoxicity (82).
In a mouse lymphoma model, IRE along with TLR3/9 agonist and PD1 blockade produced strong antigen specific CD8+ T cells and reduced the number of exhausted intratumoral CTLs, resulting in complete removal of primary tumors and distant tumors. IRE combination therapy efficiently altered the tumor microenvironment to promote anti-tumor signaling, as shown by decreased M2 macrophages, MDSCs, plasmacytoid dendritic cells, and Tregs and by increased M1 macrophages and CTLs (83). A phase II clinical trial PANFIRE-II (NCT01939665) showed that IRE resulted in a median overall survival of 17 months after diagnosis when combined with 5-fluorouracil, leucovorin, irinotecan, and oxaliplatin (84). PANFIRE-III trial (NCT04612530) is at present assessing safety and efficacy of IRE + systemic anti-PD-1 ± intratumoral TLR-9 agonist in metastatic PDAC patients (85). While clinical trials are underway it should still be noted that pattern recognition receptor pathways have not been well studied in the context of IRE and pancreatic cancer.
Conclusion
IRE is a promising and novel method to treat pancreatic cancer. Many important biochemical pathways have been implicated in pancreatic cancer and several of them were found altered after IRE treatment using human pancreatic cancer patients or animal models. For many of those pathways, starting and endpoints have been tested after IRE treatment but comprehensive knowledge of the alterations of the components of a whole pathway is crucial to understand and design an effective target for treatment. A timeline of initial tumor growth, tumor reduction after IRE treatment, IFNγ expression and its stimulation of PD-1/PD-L1, activation/suppression of pattern recognition receptors, and the TGF-β pathway would help the field develop co-therapy targets and design improved clinical trials.
Author Contributions
KI wrote the first draft of the manuscript. KI, MN-S, and RB contributed to the design of the figures. All authors reviewed and commented on the subsequent drafts of the manuscript. All authors contributed substantially to the article and approved the final version.
Funding
This work was supported, in part, by the Virginia Maryland College of Veterinary Medicine, The Virginia Tech Institute for Critical Technology and Applied Sciences Center for Engineered Health, and The National Institutes of Health R21EB028429 (ICA) R01CA213423 (RVD). The content is solely the responsibility of the authors and does not necessarily represent the official views of the NIH or any other funding agency.
Conflict of Interest
Authors IA, NA, and RD have patent applications on this work.
The remaining authors declare that the research was conducted in the absence of any commercial or financial relationships that could be construed as a potential conflict of interest.
Publisher’s Note
All claims expressed in this article are solely those of the authors and do not necessarily represent those of their affiliated organizations, or those of the publisher, the editors and the reviewers. Any product that may be evaluated in this article, or claim that may be made by its manufacturer, is not guaranteed or endorsed by the publisher.
Acknowledgments
Schematics were created with BioRender.com.
References
1. Khalil DN, Smith EL, Brentjens RJ, Wolchok JD. The Future of Cancer Treatment: Immunomodulation, CARs and Combination Immunotherapy. Nat Rev Clin Oncol (2016) 13:273–90. doi: 10.1038/nrclinonc.2016.25
2. Aroldi F, Zaniboni A. Immunotherapy for Pancreatic Cancer: Present and Future. Immunotherapy (2017) 9:607–16. doi: 10.2217/imt-2016-0142
3. Whiteside TL, Demaria S, Rodriguez-Ruiz ME, Zarour HM, Melero I. Emerging Opportunities and Challenges in Cancer Immunotherapy. Clin Cancer Res (2016) 22:1845–55. doi: 10.1158/1078-0432.CCR-16-0049
4. Ashdown ML, Robinson AP, Yatomi-Clarke SL, Ashdown ML, Allison A, Abbott D, et al. Chemotherapy for Late-Stage Cancer Patients: Meta-Analysis of Complete Response Rates. F1000Research (2015) 4. doi: 10.12688/f1000research.6760.1
5. Farkona S, Diamandis EP, Blasutig IM. Cancer Immunotherapy: The Beginning of the End of Cancer? BMC Med (2016) 14:73. doi: 10.1186/s12916-016-0623-5
6. Blattman JN, Greenberg PD. Cancer Immunotherapy: A Treatment for the Masses. Science (2004) 305:200–5. doi: 10.1126/science.1100369
7. Ansari D, Gustafsson A, Andersson R. Update on the Management of Pancreatic Cancer: Surgery is Not Enough. World J Gastroenterol WJG (2015) 21:3157. doi: 10.3748/wjg.v21.i11.3157
8. Ma Q, Long W, Xing C, Chu J, Luo M, Wang HY, et al. Cancer Stem Cells and Immunosuppressive Microenvironment in Glioma. Front Immunol (2018) 9:2924. doi: 10.3389/fimmu.2018.02924
9. seer.cancer.gov. Cancer Stat Facts: Pancreatic Cancer. Available at: https://seer.cancer.gov/statfacts/html/pancreas.html (Accessed January 29, 2022).
10. Rahib L, Smith BD, Aizenberg R, Rosenzweig AB, Fleshman JM, Matrisian LM. Projecting Cancer Incidence and Deaths to 2030: The Unexpected Burden of Thyroid, Liver, and Pancreas Cancers in the United States. Cancer Res (2014) 74:2913–21. doi: 10.1158/0008-5472.CAN-14-0155
11. Rahib L, Wehner MR, Matrisian LM, Nead KT. Estimated Projection of US Cancer Incidence and Death to 2040. JAMA Netw Open (2021) 4:e214708. doi: 10.1001/jamanetworkopen.2021.4708
12. Liang P, Wang Y. Treatment of Malignant Liver Tumors With Percutaneous Microwave Ablation: Complications Among a 1136 Patients Cohort. Radiology (2009) 251:933–40. doi: 10.1148/radiol.2513081740
13. Chen M-H, Yang W, Yan K, Gao W, Dai Y, Wang Y-B, et al. Treatment Efficacy of Radiofrequency Ablation of 338 Patients With Hepatic Malignant Tumor and the Relevant Complications. World J Gastroenterol WJG (2005) 11:6395. doi: 10.3748/wjg.v11.i40.6395
14. Zhang J, Dong B, Liang P, Yu X, Su L, Yu D, et al. Significance of Changes in Local Immunity in Patients With Hepatocellular Carcinoma After Percutaneous Microwave Coagulation Therapy. Chin Med J (Engl) (2002) 115:1367–71.
15. Hu Z, Yang XY, Liu Y, Sankin GN, Pua EC, Morse MA, et al. Investigation of HIFU-Induced Anti-Tumor Immunity in a Murine Tumor Model. J Transl Med (2007) 5:1–11. doi: 10.1186/1479-5876-5-34
16. Al-Sakere B, André F, Bernat C, Connault E, Opolon P, Davalos RV, et al. Tumor Ablation With Irreversible Electroporation. PloS One (2007) 2:e1135. doi: 10.1371/journal.pone.0001135
17. Belehradek M, Domenge C, Luboinski B, Orlowski S, Belehradek J, Mir LM. Electrochemotherapy, a New Antitumor Treatment. First Clinical Phase I-II Trial. Cancer (1993) 72:3694–700. doi: 10.1002/1097-0142(19931215)72:12<3694::AID-CNCR2820721222>3.0.CO;2-2
18. Ringel-Scaia VM, Beitel-White N, Lorenzo MF, Brock RM, Huie KE, Coutermarsh-Ott S, et al. High-Frequency Irreversible Electroporation is an Effective Tumor Ablation Strategy That Induces Immunologic Cell Death and Promotes Systemic Anti-Tumor Immunity. EBioMedicine (2019) 44:112–25. doi: 10.1016/j.ebiom.2019.05.036
19. Shao Q, O’Flanagan S, Lam T, Roy P, Pelaez F, Burbach BJ, et al. Engineering T Cell Response to Cancer Antigens by Choice of Focal Therapeutic Conditions. Int J Hyperth (2019) 36:130–8. doi: 10.1080/02656736.2018.1539253
20. Bulvik BE, Rozenblum N, Gourevich S, Ahmed M, Andriyanov AV, Galun E, et al. Irreversible Electroporation Versus Radiofrequency Ablation: A Comparison of Local and Systemic Effects in a Small-Animal Model. Radiology (2016) 280:413–24. doi: 10.1148/radiol.2015151166
21. Narayanan JSS, Ray P, Hayashi T, Whisenant TC, Vicente D, Carson DA, et al. Irreversible Electroporation Combined With Checkpoint Blockade and TLR7 Stimulation Induces Antitumor Immunity in a Murine Pancreatic Cancer Model. Cancer Immunol Res (2019) 7:1714–26. doi: 10.1158/2326-6066.CIR-19-0101
22. He C, Huang X, Zhang Y, Lin X. Li S. T-Cell Activation and Immune Memory Enhancement Induced by Irreversible Electroporation in Pancreatic Cancer. Clin Transl Med (2020) 10:e39. doi: 10.1002/ctm2.39
23. Romeo S, Sannino A, Scarfì MR, Vernier PT, Cadossi R, Gehl J, et al. ESOPE-Equivalent Pulsing Protocols for Calcium Electroporation: An In Vitro Optimization Study on 2 Cancer Cell Models. Technol Cancer Res Treat (2018) 17:1533033818788072. doi: 10.1177/1533033818788072
24. Marty M, Sersa G, Garbay JR, Gehl J, Collins CG, Snoj M, et al. Electrochemotherapy–An Easy, Highly Effective and Safe Treatment of Cutaneous and Subcutaneous Metastases: Results of ESOPE (European Standard Operating Procedures of Electrochemotherapy) Study. Eur J Cancer Suppl (2006) 4:3–13. doi: 10.1016/j.ejcsup.2006.08.002
25. Mir LM, Gehl J, Sersa G, Collins CG, Garbay J-R, Billard V, et al. Standard Operating Procedures of the Electrochemotherapy: Instructions for the Use of Bleomycin or Cisplatin Administered Either Systemically or Locally and Electric Pulses Delivered by the CliniporatorTM by Means of Invasive or non-Invasive Electrodes. Eur J Cancer Suppl (2006) 4:14–25. doi: 10.1016/j.ejcsup.2006.08.003
26. Belfiore MP, Ronza FM, Romano F, Pietro IG, De Lucia G, Gallo C, et al. Percutaneous CT-Guided Irreversible Electroporation Followed by Chemotherapy as a Novel Neoadjuvant Protocol in Locally Advanced Pancreatic Cancer: Our Preliminary Experience. Int J Surg (2015) 21:S34–9. doi: 10.1016/j.ijsu.2015.06.049
27. Sugimoto K, Kakimi K, Takeuchi H, Fujieda N, Saito K, Sato E, et al. Irreversible Electroporation Versus Radiofrequency Ablation: Comparison of Systemic Immune Responses in Patients With Hepatocellular Carcinoma. J Vasc Interv Radiol (2019) 30:845–53. doi: 10.1016/j.jvir.2019.03.002
28. Martin RC II, Kwon D, Chalikonda S, Sellers M, Kotz E, Scoggins C, et al. Treatment of 200 Locally Advanced (Stage III) Pancreatic Adenocarcinoma Patients With Irreversible Electroporation: Safety and Efficacy. Ann Surg (2015) 262:486–94. doi: 10.1097/SLA.0000000000001441
29. Cannon R, Ellis S, Hayes D, Narayanan G, Martin RCG. Safety and Early Efficacy of Irreversible Electroporation for Hepatic Tumors in Proximity to Vital Structures. J Surg Oncol (2013) 107:544–9. doi: 10.1002/jso.23280
30. Narayanan G, Bilimoria MM, Hosein PJ, Su Z, Mortimer KM, Martin RCG. Multicenter Randomized Controlled Trial and Registry Study to Assess the Safety and Efficacy of the NanoKnife® System for the Ablation of Stage 3 Pancreatic Adenocarcinoma: Overview of Study Protocols. BMC Cancer (2021) 21:1–10. doi: 10.1186/s12885-021-08474-4
31. Rubinsky B. Irreversible Electroporation. New York, NY United States: Springer Science & Business Media (2009).
32. Lee EW, Chen C, Prieto VE, Dry SM, Loh CT, Kee ST. Advanced Hepatic Ablation Technique for Creating Complete Cell Death: Irreversible Electroporation. Radiology (2010) 255:426–33. doi: 10.1148/radiol.10090337
33. Yang J, Eresen A, Shangguan J, Ma Q, Yaghmai V, Zhang Z. Irreversible Electroporation Ablation Overcomes Tumor-Associated Immunosuppression to Improve the Efficacy of DC Vaccination in a Mice Model of Pancreatic Cancer. Oncoimmunology (2021) 10:1875638. doi: 10.1080/2162402X.2021.1875638
34. Partridge BR, O’Brien TJ, Lorenzo MF, Coutermarsh-Ott SL, Barry SL, Stadler K, et al. High-Frequency Irreversible Electroporation for Treatment of Primary Liver Cancer: A Proof-Of-Principle Study in Canine Hepatocellular Carcinoma. J Vasc Interv Radiol (2020) 31:482–491.e4. doi: 10.1016/j.jvir.2019.10.015
35. Brock RM, Beitel-White N, Davalos RV, Allen IC. Starting a Fire Without Flame: The Induction of Cell Death and Inflammation in Electroporation-Based Tumor Ablation Strategies. Front Oncol (2020) 10:1235. doi: 10.3389/fonc.2020.01235
36. Elmore S. Apoptosis: A Review of Programmed Cell Death. Toxicol Pathol (2007) 35:495–516. doi: 10.1080/01926230701320337
37. Man SM, Karki R, Kanneganti T-D. Molecular Mechanisms and Functions of Pyroptosis, Inflammatory Caspases and Inflammasomes in Infectious Diseases. Immunol Rev (2017) 277:61–75. doi: 10.1111/imr.12534
38. Zhao J, Wen X, Tian L, Li T, Xu C, Wen X, et al. Irreversible Electroporation Reverses Resistance to Immune Checkpoint Blockade in Pancreatic Cancer. Nat Commun (2019) 10:1–14. doi: 10.1038/s41467-019-08782-1
39. Roux S, Bernat C, Al-Sakere B, Ghiringhelli F, Opolon P, Carpentier AF, et al. Tumor Destruction Using Electrochemotherapy Followed by CpG Oligodeoxynucleotide Injection Induces Distant Tumor Responses. Cancer Immunol Immunother (2008) 57:1291–300. doi: 10.1007/s00262-008-0462-0
40. Calvet CY, Famin D, André FM, Mir LM. Electrochemotherapy With Bleomycin Induces Hallmarks of Immunogenic Cell Death in Murine Colon Cancer Cells. Oncoimmunology (2014) 3:e28131. doi: 10.4161/onci.28131
41. Nuccitelli R, McDaniel A, Anand S, Cha J, Mallon Z, Berridge JC, et al. Nano-Pulse Stimulation is a Physical Modality That can Trigger Immunogenic Tumor Cell Death. J Immunother Cancer (2017) 5:1–13. doi: 10.1186/s40425-017-0234-5
42. Beitel-White N, Martin RCG, Li Y, Brock RM, Allen IC, Davalos RV. Real-Time Prediction of Patient Immune Cell Modulation During Irreversible Electroporation Therapy. Sci Rep (2019) 9:1–8. doi: 10.1038/s41598-019-53974-w
43. Tian G, Guan J, Chu Y, Zhao Q, Jiang T. Immunomodulatory Effect of Irreversible Electroporation Alone and Its Cooperating With Immunotherapy in Pancreatic Cancer. Front Oncol (2021) 11:712042. doi: 10.3389/fonc.2021.712042
44. Scheffer HJ, Stam AGM, Geboers B, Vroomen LGPH, Ruarus A, de Bruijn B, et al. Irreversible Electroporation of Locally Advanced Pancreatic Cancer Transiently Alleviates Immune Suppression and Creates a Window for Antitumor T Cell Activation. Oncoimmunology (2019) 8:1652532. doi: 10.1080/2162402X.2019.1652532
45. Kepp O, Senovilla L, Vitale I, Vacchelli E, Adjemian S, Agostinis P, et al. Consensus Guidelines for the Detection of Immunogenic Cell Death. Oncoimmunology (2014) 3:e955691. doi: 10.4161/21624011.2014.955691
46. Garg AD, Krysko DV, Vandenabeele P, Agostinis P. The Emergence of Phox-ER Stress Induced Immunogenic Apoptosis. Oncoimmunology (2012) 1:786–8. doi: 10.4161/onci.19750
47. Fridlender ZG, Sun J, Kim S, Kapoor V, Cheng G, Ling L, et al. Polarization of Tumor-Associated Neutrophil Phenotype by TGF-β:”N1” Versus “N2” TAN. Cancer Cell (2009) 16:183–94. doi: 10.1016/j.ccr.2009.06.017
48. Thorsson V, Gibbs DL, Brown SD, Wolf D, Bortone DS, Yang THO, et al. Cancer Genome Atlas Research, AJ Lazar, JS Serody, EG Demicco, ML Disis, BG Vincent and I. Shmulevich. Immunity (2018) 48:812–30. doi: 10.1016/j.immuni.2018.03.023
49. Dai M, Hellstrom I, Yip YY, Sjögren HO, Hellstrom KE. Tumor Regression and Cure Depends on Sustained Th1 Responses. J Immunother (Hagerstown Md 1997) (2018) 41:369. doi: 10.1097/CJI.0000000000000231
50. Dewan MZ, Terunuma H, Takada M, Tanaka Y, Abe H, Sata T, et al. Role of Natural Killer Cells in Hormone-Independent Rapid Tumor Formation and Spontaneous Metastasis of Breast Cancer Cells In Vivo. Breast Cancer Res Treat (2007) 104:267–75. doi: 10.1007/s10549-006-9416-4
51. Bingle L, Brown NJ, Lewis CE. The Role of Tumour-Associated Macrophages in Tumour Progression: Implications for New Anticancer Therapies. J Pathol A J Pathol Soc Gt Britain Irel (2002) 196:254–65. doi: 10.1002/path.1027
52. Mougiakakos D, Choudhury A, Lladser A, Kiessling R, Johansson CC. Regulatory T Cells in Cancer. Adv Cancer Res (2010) 107:57–117. doi: 10.1016/S0065-230X(10)07003-X
53. Diaz-Montero CM, Salem ML, Nishimura MI, Garrett-Mayer E, Cole DJ, Montero AJ. Increased Circulating Myeloid-Derived Suppressor Cells Correlate With Clinical Cancer Stage, Metastatic Tumor Burden, and Doxorubicin–Cyclophosphamide Chemotherapy. Cancer Immunol Immunother (2009) 58:49–59. doi: 10.1007/s00262-008-0523-4
54. Shree T, Olson OC, Elie BT, Kester JC, Garfall AL, Simpson K, et al. Macrophages and Cathepsin Proteases Blunt Chemotherapeutic Response in Breast Cancer. Genes Dev (2011) 25:2465–79. doi: 10.1101/gad.180331.111
55. Mirza N, Fishman M, Fricke I, Dunn M, Neuger AM, Frost TJ, et al. All-Trans-Retinoic Acid Improves Differentiation of Myeloid Cells and Immune Response in Cancer Patients. Cancer Res (2006) 66:9299–307. doi: 10.1158/0008-5472.CAN-06-1690
56. Hampton HR, Chtanova T. Lymphatic Migration of Immune Cells. Front Immunol (2019) 10:1168. doi: 10.3389/fimmu.2019.01168
57. Neal RE 2nd, Rossmeisl JHJ, Robertson JL, Arena CB, Davis EM, Singh RN, et al. Improved Local and Systemic Anti-Tumor Efficacy for Irreversible Electroporation in Immunocompetent Versus Immunodeficient Mice. PloS One (2013) 8:e64559. doi: 10.1371/journal.pone.0064559
58. Alinezhadbalalami N, Graybill PM, Imran KM, Verbridge SS, Allen IC, Davalos RV. Generation of Tumor-Activated T Cells Using Electroporation. Bioelectrochemistry (2021) 142:107886. doi: 10.1016/j.bioelechem.2021.107886
59. Zhao J, Chen S, Zhu L, Zhang L, Liu J, Xu D, et al. Antitumor Effect and Immune Response of Nanosecond Pulsed Electric Fields in Pancreatic Cancer. Front Oncol (2020) 10:621092. doi: 10.3389/fonc.2020.621092
60. Figini M, Wang X, Lyu T, Su Z, Wang B, Sun C, et al. Diffusion MRI Biomarkers Predict the Outcome of Irreversible Electroporation in a Pancreatic Tumor Mouse Model. Am J Cancer Res (2018) 8:1615–23.
61. White SB, Zhang Z, Chen J, Gogineni VR, Larson AC. Early Immunologic Response of Irreversible Electroporation Versus Cryoablation in a Rodent Model of Pancreatic Cancer. J Vasc Interv Radiol (2018) 29:1764–9. doi: 10.1016/j.jvir.2018.07.009
62. He C, Wang J, Sun S, Zhang Y, Li S. Immunomodulatory Effect After Irreversible Electroporation in Patients With Locally Advanced Pancreatic Cancer. J Oncol (2019) 2019:9346017. doi: 10.1155/2019/9346017
63. St Paul M, Ohashi PS. The Roles of CD8(+) T Cell Subsets in Antitumor Immunity. Trends Cell Biol (2020) 30:695–704. doi: 10.1016/j.tcb.2020.06.003
64. Jorgovanovic D, Song M, Wang L, Zhang Y. Roles of IFN-γ in Tumor Progression and Regression: A Review. Biomark Res (2020) 8:49. doi: 10.1186/s40364-020-00228-x
65. Tau GZ, Cowan SN, Weisburg J, Braunstein NS, Rothman PB. Regulation of IFN-Gamma Signaling is Essential for the Cytotoxic Activity of CD8(+) T Cells. J Immunol (2001) 167:5574–82. doi: 10.4049/jimmunol.167.10.5574
66. Maimela NR, Liu S, Zhang Y. Fates of CD8+ T Cells in Tumor Microenvironment. Comput Struct Biotechnol J (2019) 17:1–13. doi: 10.1016/j.csbj.2018.11.004
67. Lin M, Alnaggar M, Liang S, Wang X, Liang Y, Zhang M, et al. An Important Discovery on Combination of Irreversible Electroporation and Allogeneic Natural Killer Cell Immunotherapy for Unresectable Pancreatic Cancer. Oncotarget (2017) 8:101795–807. doi: 10.18632/oncotarget.21974
68. Li X, Xu K, Li W, Qiu X, Ma B, Fan Q, et al. Immunologic Response to Tumor Ablation With Irreversible Electroporation. PloS One (2012) 7:e48749. doi: 10.1371/journal.pone.0048749
69. O’Neill C, Hayat T, Hamm J, Healey M, Zheng Q, Li Y. Martin RCG 2nd. A Phase 1b Trial of Concurrent Immunotherapy and Irreversible Electroporation in the Treatment of Locally Advanced Pancreatic Adenocarcinoma. Surgery (2020) 168:610–6. doi: 10.1016/j.surg.2020.04.057
70. Kalbasi A, Ribas A. Tumour-Intrinsic Resistance to Immune Checkpoint Blockade. Nat Rev Immunol (2020) 20:25–39. doi: 10.1038/s41577-019-0218-4
71. Wagner M, Kleeff J, Friess H, Büchler MW, Korc M. Enhanced Expression of the Type II Transforming Growth Factor-Beta Receptor is Associated With Decreased Survival in Human Pancreatic Cancer. Pancreas (1999) 19:370–6. doi: 10.1097/00006676-199911000-00008
72. Zi Z, Chapnick DA, Liu X. Dynamics of TGF-β/Smad Signaling. FEBS Lett (2012) 586:1921–8. doi: 10.1016/j.febslet.2012.03.063
73. Han WGH, Schuurhuis DH, Fu N, Camps M, van Duivenvoorde LM, Louis-Plence P, et al. DC-Induced CD8(+) T-Cell Response is Inhibited by MHC Class II-Dependent DX5(+)CD4(+) Treg. Eur J Immunol (2009) 39:1765–73. doi: 10.1002/eji.200838842
74. Novickij V, Čėsna R, Perminaitė E, Zinkevičienė A, Characiejus D, Novickij J, et al. Antitumor Response and Immunomodulatory Effects of Sub-Microsecond Irreversible Electroporation and Its Combination With Calcium Electroporation. Cancers (Basel) (2019) 11:1763. doi: 10.3390/cancers11111763
75. Li Z, Liu X, Guo R, Wang P. CD4(+)Foxp3(-) Type 1 Regulatory T Cells in Glioblastoma Multiforme Suppress T Cell Responses Through Multiple Pathways and are Regulated by Tumor-Associated Macrophages. Int J Biochem Cell Biol (2016) 81:1–9. doi: 10.1016/j.biocel.2016.09.013
76. Yang R, Cai Z, Zhang Y, Yutzy WH4, Roby KF, Roden RBS. CD80 in Immune Suppression by Mouse Ovarian Carcinoma-Associated Gr-1+CD11b+ Myeloid Cells. Cancer Res (2006) 66:6807–15. doi: 10.1158/0008-5472.CAN-05-3755
77. Huang B, Pan P-Y, Li Q, Sato AI, Levy DE, Bromberg J, et al. Gr-1+CD115+ Immature Myeloid Suppressor Cells Mediate the Development of Tumor-Induced T Regulatory Cells and T-Cell Anergy in Tumor-Bearing Host. Cancer Res (2006) 66:1123–31. doi: 10.1158/0008-5472.CAN-05-1299
78. Guo S, Burcus NI, Hornef J, Jing Y, Jiang C, Heller R, et al. Nano-Pulse Stimulation for the Treatment of Pancreatic Cancer and the Changes in Immune Profile. Cancers (Basel) (2018) 10(7):217. doi: 10.3390/cancers10070217
79. Latz E, Verma A, Visintin A, Gong M, Sirois CM, Klein DCG, et al. Ligand-Induced Conformational Changes Allosterically Activate Toll-Like Receptor 9. Nat Immunol (2007) 8:772–9. doi: 10.1038/ni1479
80. Krieg AM. Antitumor Applications of Stimulating Toll-Like Receptor 9 With CpG Oligodeoxynucleotides. Curr Oncol Rep (2004) 6:88–95. doi: 10.1007/s11912-004-0019-0
81. Krieg AM. Therapeutic Potential of Toll-Like Receptor 9 Activation. Nat Rev Drug Discov (2006) 5:471–84. doi: 10.1038/nrd2059
82. Appay V, Jandus C, Voelter V, Reynard S, Coupland SE, Rimoldi D, et al. New Generation Vaccine Induces Effective Melanoma-Specific CD8+ T Cells in the Circulation But Not in the Tumor Site. J Immunol (2006) 177:1670–8. doi: 10.4049/jimmunol.177.3.1670
83. Babikr F, Wan J, Xu A, Wu Z, Ahmed S, Freywald A, et al. Distinct Roles But Cooperative Effect of TLR3/9 Agonists and PD-1 Blockade in Converting the Immunotolerant Microenvironment of Irreversible Electroporation-Ablated Tumors. Cell Mol Immunol (2021) 18:2632–47. doi: 10.1038/s41423-021-00796-4
84. Ruarus AH, Vroomen LGPH, Geboers B, van Veldhuisen E, Puijk RS, Nieuwenhuizen S, et al. Percutaneous Irreversible Electroporation in Locally Advanced and Recurrent Pancreatic Cancer (PANFIRE-2): A Multicenter, Prospective, Single-Arm, Phase II Study. Radiology (2020) 294:212–20. doi: 10.1148/radiol.2019191109
85. Geboers B, Timmer FEF, Ruarus AH, Pouw JEE, Schouten EAC, Bakker J, et al. Irreversible Electroporation and Nivolumab Combined With Intratumoral Administration of a Toll-Like Receptor Ligand, as a Means of In Vivo Vaccination for Metastatic Pancreatic Ductal Adenocarcinoma (PANFIRE-III). A Phase-I Study Protocol. Cancers (Basel) (2021) 13(15):3902. doi: 10.3390/cancers13153902
Keywords: pancreatic cancer, irreversible electroporation, anti-tumor immunity, immunomodulatory pathways, IFNγ-PD-L1 axis
Citation: Imran KM, Nagai-Singer MA, Brock RM, Alinezhadbalalami N, Davalos RV and Allen IC (2022) Exploration of Novel Pathways Underlying Irreversible Electroporation Induced Anti-Tumor Immunity in Pancreatic Cancer. Front. Oncol. 12:853779. doi: 10.3389/fonc.2022.853779
Received: 13 January 2022; Accepted: 28 February 2022;
Published: 18 March 2022.
Edited by:
Uei Pua, Tan Tock Seng Hospital, SingaporeReviewed by:
Marie-Pierre Rols, UMR5089 Institut de Pharmacologie et de Biologie Structurale (IPBS), FranceCopyright © 2022 Imran, Nagai-Singer, Brock, Alinezhadbalalami, Davalos and Allen. This is an open-access article distributed under the terms of the Creative Commons Attribution License (CC BY). The use, distribution or reproduction in other forums is permitted, provided the original author(s) and the copyright owner(s) are credited and that the original publication in this journal is cited, in accordance with accepted academic practice. No use, distribution or reproduction is permitted which does not comply with these terms.
*Correspondence: Irving Coy Allen, aWNhbGxlbkB2dC5lZHU=