- 1Graduate Biomedical Sciences Program, University of Alabama at Birmingham, Birmingham, AL, United States
- 2Department of Biophysics, Faculty of Biochemistry, Biophysics and Biotechnology, Jagiellonian University, Krakow, Poland
- 3Department of Biophysics and Cancer Biology, Faculty of Biochemistry, Biophysics and Biotechnology, Jagiellonian University, Krakow, Poland
- 4Department of Dermatology, University of Alabama at Birmingham, Birmingham, AL, United States
- 5Department of Human Biology, Institute of Biology, Faculty of Biological and Veterinary Sciences, Nicolaus Copernicus University, Toruń, Poland
- 6Pathology Laboratory Service, Veteran Administration Medical Center at Birmingham, Birmingham, AL, United States
Melanin pigment plays a critical role in the protection against the harmful effects of ultraviolet radiation and other environmental stressors. It is produced by the enzymatic transformation of L-tyrosine to dopaquinone and subsequent chemical and biochemical reactions resulting in the formation of various 5,6-dihydroxyindole-2-carboxylic acid (DHICA) and 5,6-dihydroxyindole (DHI) oligomers—main constituents of eumelanin, and benzothiazine and benzothiazole units of pheomelanin. The biosynthesis of melanin is regulated by sun exposure and by many hormonal factors at the tissue, cellular, and subcellular levels. While the presence of melanin protects against the development of skin cancers including cutaneous melanoma, its presence may be necessary for the malignant transformation of melanocytes. This shows a complex role of melanogenesis in melanoma development defined by chemical properties of melanin and the nature of generating pathways such as eu- and pheomelanogenesis. While eumelanin is believed to provide radioprotection and photoprotection by acting as an efficient antioxidant and sunscreen, pheomelanin, being less photostable, can generate mutagenic environment after exposure to the short-wavelength UVR. Melanogenesis by itself and its highly reactive intermediates show cytotoxic, genotoxic, and mutagenic activities, and it can stimulate glycolysis and hypoxia-inducible factor 1-alpha (HIF-1α) activation, which, combined with their immunosuppressive effects, can lead to melanoma progression and resistance to immunotherapy. On the other hand, melanogenesis-related proteins can be a target for immunotherapy. Interestingly, clinicopathological analyses on advanced melanomas have shown a negative correlation between tumor pigmentation and diseases outcome as defined by overall survival and disease-free time. This indicates a “Yin and Yang” role for melanin and active melanogenesis in melanoma development, progression, and therapy. Furthermore, based on the clinical, experimental data and diverse effects of melanogenesis, we propose that inhibition of melanogenesis in advanced melanotic melanoma represents a realistic adjuvant strategy to enhance immuno-, radio-, and chemotherapy.
Biochemistry and Chemistry of Melanin Pigmentation
Melanin pigmentation of mammals is regulated by a number of factors at the systemic, tissue, cellular, and subcellular levels (1). The solar radiation is the main environmental factor regulating melanin pigmentation of the skin directly or indirectly via different mechanisms (2, 3), while melanin pigment is the main protective factor against ultraviolet (UVR)-induced damage (4). In addition, the skin pigmentary responses are affected by endocrine, nutritional, paracrine, autocrine, and intracrine factors and involve precise interactions between epidermal or follicular melanocytes and keratinocytes (1, 5–10). On the cellular level, melanin synthesis takes place in highly specialized organelles, regulated through precise mechanisms involving organelle formation, synthesis, delivery of enzymes, structural and regulatory proteins and co-factors, substrates, copper, and final activation and dynamic modification and velocity of the process (1, 5).
In mammalian melanocytes, two main types of melanin are synthesized—eumelanin and pheomelanin (11, 12). While the level of melanin synthesis and the type of synthesized melanin in most mammalian species are predominantly determined by two key factors, namely, melanotropins and agouti signaling protein, these are also regulated by several other factors utilizing different signaling transduction pathways including cAMP, calcium, and protein kinase A and C (1, 13–23). Although eumelanin and pheomelanin derive from the common precursor dopaquinone, which is formed by tyrosinase-catalyzed transformation of tyrosine to DOPA and dopaquinone (24–26), biosynthesis of pheomelanin occurs without additional catalytical action of enzymes, requiring only cysteine to produce benzothiazine and benzothiazole units (11, 27–29). Biosynthesis of eumelanin, on the other hand, requires two additional tyrosinase-related proteins (TRPs or TYRPs), which catalyze the conversion of dopachrome to 5,6-dihydroxyindole-2-carboxylic acid (DHICA) and oxidation of 5,6-dihydroxyindole (DHI) and DHICA (30–36). They were named as TRP-1—DHICA oxidase (37)—and TRP-2—dopachrome tautomerase (Dct) (38). The latter contains zinc (II) cation in its active center (39). Cupric and other ions can also stimulate the rearrangement of dopachrome to DHICA (40, 41). Interestingly, metal cations such as Mn+2, Cu+2, and others can stimulate DOPA auto-oxidation to melanin without any enzyme needed with velocity of the process depending on the pH and physicochemical properties of the solution (6). Moreover, zinc (II) cations, which are known to inhibit melanogenesis via inhibiting TYR (42, 43), may stimulate polymerization of pheomelanin monomers in vitro (44).
In addition, exposure of L-tyrosine dissolved in water to solar light induces its photochemical transformation leading to gradual production of melanin in this purely in vitro condition. The pivotal role of dopaquinone in controlling melanogenesis was demonstrated by pulse radiolysis studies (45–47). Thus, although the intramolecular addition of the amino group giving cyclodopa was shown to be relatively slow, it rapidly oxidized to dopachrome through redox exchange. On the other hand, in the presence of cysteine, 5-S-cysteinyldopa was quickly formed, which, via redox exchange, gave cysteinyldopaquinone. In most cases, a mixed melanogenesis occurs giving rise to mixed melanin (48).
It is believed that the main subunits of eumelanin—derivatives of DHI and DHICA—and in case of pheomelanin—derivatives of benzothiazine and benzothiazole—polymerize to relatively small oligomers, which, via π−π interaction form protomolecules, and via secondary and tertiary aggregation, form pigment granules (49, 50). The hierarchical aggregate structure of melanin, particularly eumelanin, has been discussed in recent reviews (12, 51) Typical melanosome, depending on its origin, is a membrane-bound oval or spheroidal structure of submicrometer to a few micrometer size (1, 52). Eumelanin, pheomelanin, or most commonly mixed-type melanin is deposited on a fibrillar matrix formed by the amyloid core of the melanocyte-specific protein PMEL (53). Importantly, chemistry and photochemistry of melanin granules should be viewed as relatively separated processes that take place in a special milieu provided by the melanized melanosome. The melanosomal membrane limits the access of reagents that could interact with melanin and reduces the outflow of the reaction products. Considering that melanogenesis itself is accompanied by the formation of highly reactive species (1, 11, 54, 55), such spatial separation between the melanin and cytosol is sensible and justified.
In conclusion, melanin synthesis is a highly complex process developed through billions of years of evolution to protect living organisms in educated manner from damaging effects of different spectrum of the solar light in highly dynamic fashions involving several feedback mechanisms and regulatory processes affecting cell, tissue, and organismal homeostasis.
Electron-Exchange and Metal Ion-Binding Properties of Melanin Relevant for its Antioxidant and Photoprotective Action
Although melanin is usually considered a very stable organic material and chemical evidence for eumelanin pigment from the Jurassic period was presented (56), different studies have demonstrated that melanin exhibits substantial chemical reactivity (1, 57, 58) and can undergo physicochemical changes even under in vivo conditions (59, 60). Arguably, one of the most distinct chemical properties of melanin is its ability to participate in redox reactions. This is consistent with the composition of melanin, which contains significant number of redox-active groups such as DHI and DHICA in case of eumelanin and benzothiazine and benzothiazole in pheomelanin. They also contain fully oxidized forms of the corresponding hydroquinones and aminophenols, i.e., ortho-quinones and ortho-quinonimines, and a very small percentage of ortho-semiquinones and ortho-semiquinonimines, which arise from so-called comproportionation equilibrium of the corresponding fully reduced and fully oxidized melanin subunits (12, 61, 62). The fully reduced melanin building blocks are good electron donors, while the fully oxidized units are responsible for oxidizing properties of the melanin. One of the major differences between free ortho-quinones, ortho-quinonimines, and their corresponding radical forms, and the oxidized melanin subunits and melanin radicals is their effective stability and reactivity. While in solution, ortho-quinones and ortho-semiquinones are extremely unstable and very reactive (63, 64), in melanin, these functional groups exhibit only modest reactivity (65). Although many factors may be responsible for such a dramatic modification of the reactivity of melanin quinone (quinonimine) groups, steric hindrance of the groups and changes in their one-electron reduction potential, after incorporation into the forming oligomers, could play significant role.
The reducing ability of natural melanin has been recognized long ago and was exploited in a histological test to detect melanin in situ; the presence of melanin in the biological material was deduced from the ability of the specimen to reduce Ag+ ions to metallic silver (66). Oxidation of NADH by melanin was reported in 1968 (67), and electron transfer properties of melanin were demonstrated in several redox systems (68–71). Melanin is considered an efficient antioxidant that scavenges reactive free radicals (72, 73). The issue has been addressed in a systematic way by employing the most direct experimental approach—pulse radiolysis (72, 73). It was demonstrated that a number of reducing and oxidizing radicals interacted with synthetic DOPA-melanin and cysteinyldopa-melanin with the efficiency that correlated with the absolute value of the radical one-electron reduction potential (72). In most cases, melanin interacted with the radicals via simple one-electron transfer processes, consistent with the presence of melanin oxidized and reduced subunits. The obtained data indicated that synthetic pheomelanin was more efficient in oxidizing the reducing radicals, while synthetic eumelanin could interact more efficiently with the oxidizing radicals. The effects of synthetic eumelanin on iron-catalyzed free radical decomposition of hydrogen peroxide was studied by EPR-spin trapping (74). At low iron concentration, melanin dramatically decreased the yield of hydroxyl radicals due to binding of ferrous ions; however, it increased the rate of hydroxyl radicals particularly in the excess of ferric ions due to the ability of melanin to reduce iron to ferrous ions. Distinct inhibition of lipid peroxidation induced by ferrous ions or a water-soluble free radical initiator by synthetic neuromelanin, prepared by autooxidation of dopamine, was reported (75). Although scavenging of oxidizing radicals could in part be responsible for the observed antioxidant action of melanin, sequestration of redox active iron ions plays the dominant role as shown in a related study (76). Interestingly, the protective action of melanin against peroxidation of lipids induced by iron/ascorbate significantly diminished after bovine retinal pigmented epithelium (RPE) melanosomes were subjected to experimental photobleaching—an in vitro model for melanosomes photoaging (77).
Several attempts to determine the oxidation (and reduction) potential of synthetic and natural melanin by cyclic voltammetry measurements gave somewhat different results, with the melanin oxidation potential ranging between 0.125 and 0.6V and the melanin reduction potential being in the range −0.5 V–+0.4V (72–76). Distinct pro-oxidizing activity of synthetic pheomelanins was demonstrated in a study, in which the efficiency of melanin containing different amount of benzothiazole and benzothiazine to photooxidize reduced glutathione was compared (78). The researchers found that benzothiazole-rich pheomelanin was more efficient in depleting glutathione (GSH) upon irradiation with UVA than benzothiazine-rich melanin. Interestingly, in a non-related study, it was demonstrated that partially photodegraded 5-S-cysteinyldopa melanin, which exhibited higher percentage of benzothiazole derivatives, compared to control non-photolyzed melanin, photogenerated singlet oxygen with significantly higher yield (79). A detailed discussion of the possible UV-dependent and UV-independent chemical mechanisms underlying pheomelanin-mediated oxidative stress, with special reference to the oxygen-dependent depletion of glutathione and other cell antioxidants, was presented in a review by Napolitano et al. (80).
The ability of melanin to reversibly bind metal ions, behaving as a weak acid cation exchanger, has been recognized for over a half century (81). Binding by melanin of cupric and other paramagnetic metal ions is accompanied by significant quenching of the EPR signal of melanin radicals (82). On the other hand, the interaction of melanin with multivalent diamagnetic metal ions, such as zinc(II), brings about a significant increase in the melanin radical EPR signal (83). The effect was explained as being due to a metal-ion-induced shift in the comproportionation equilibrium between the fully reduced and fully oxidized melanin subunits and melanin radicals stabilized by the metal ions (61). Using EPR, Mossbauer, and resonance Raman spectroscopies, atomic absorption measurements, potentiometric titration, and selective chemical blocking of specific functional groups, the role of phenolic hydroxyl, ortho-semiquinone, amine, and carboxyl groups in binding of copper(II) and iron(III) and zinc(II), at different pH, was analyzed (84–86). It is important to realize that although melanin is a vivid chelator of redox-active metal ions, such as copper (II) and iron(III), under acidic conditions or in the case of metal ion overload, melanin will tend to release bound metal ions (84, 87). In addition, it was demonstrated that experimentally photoaged RPE melanosomes from pig and bovine eyes lost part of their metal ion binding capacity, which resulted in a reduced antioxidant efficiency of such pigment granules (77, 88). It is postulated that exposure of pigmented tissues to significant fluxes of UV radiation or even short-wavelength visible light energy can lead to physicochemical modification of the melanin, particularly its metal ion binding and redox properties, which could lower the protective ability of melanin and even increase its pro-oxidizing potential (77, 89–91).
Thus, melanin pigment, being unquestionably protective against light, can be a part of damaging circuity that is context dependent in its physicochemical and biological environments. We could speculate that billions of years of evolution established precise biophysical mechanisms placed into a proper biological context to protect integumental cells from the damage, destruction or malignant transformation, or to destroy the same cells that are beyond repair and would represent a danger to the local and perhaps global homeostasis (2, 92). This would make pigmentary responses crucial to organismal survival and/or adaptation requiring very precise mechanisms regulating them and leaving no room for random reactions in biological context. Based on the biochemistry, physiochemistry, and biology, it has already been postulated that melanogenically active melanosomes serve and signaling molecules regulate epidermal functions (93, 94) with concomitant function of melanocytes and melanosomes in sensing, traducing, and computing solar radiation (2).
Melanin, While Protecting Against UVR-Induced Melanomagenesis, Also Contributes to the Initiation of Malignant Transformation of Melanocytes
One of the important distinguishing features of melanoma is its neuro-ectodermal, neural crest origin. This is actually a transient organ, active in the embryonal development, and of the role of producing embryonic cells that are able, and this is their main role, to wander around the organism, settle various niches, and differentiate toward the terminally differentiated cells (95). This feature may be preserved during the whole lifetime, the example of which are melanocytes, in particular the ones of the hair follicle. Their progenitors preserve the possibility of migrating and settling niches (96), so no wonder that the same feature is very early manifested during the progression of melanoma, which makes this tumor particularly invasive.
The neural crest is believed to be the only important distinguishing feature of vertebrates, surprisingly not the spine (95). The possibility to transform to melanoma cells should be, consequently, preserved only to vertebrates. This seems to be the case. Interestingly, other melanin-producing cells in vertebrates such as the RPE transform mainly to benign tumors [this is more of hyperplasia than neoplasia (97)], while the malignant tumors are of a different histopathological character (98, 99). Meanwhile, one of the most dangerous and invasive tumor in children, neuroblastoma, reveals the character of immature, non-differentiated, and not pigmented neural crest cells, not being melanocytes (100).
Clearly, the development of melanoma is related to the melanocytic line of neural crest development, and other tumors of melanocytic origin are unknown. Nevertheless, there are important in vivo models developed in non-vertebrates, often used in research, namely, in Drosophila melanogaster (101). The tumors can be there easily induced by mutation in particular genes regulating cellular development (101). This melanomagenesis is clearly non-melanin related. On the other hand, one cannot find such melanomas developing spontaneously or under physical conditions in D. melanogaster, only by mutations, which even further couples melanomagenesis with melanin-producing melanocytes or their progenitors. The decisions whether a particular part of neuroectoderm should differentiate towards neural crest and, consequently, a part of them towards pigmented melanocytes, is taken very early in embryogenesis, on the stage of gastrulation (95), which also anchorages the evolutionary pathways conditioning development of melanoma very early in evolution. Melanoma cells very often reveal various forms of mal-pigmentation, starting from the level of melanogenesis (102–104), toward cytology (105, 106), and transfer of melanosomes to the target tissues in human (107) or in amphibians (108). Clearly, the possibility to develop melanoma is a long-established toll paid for controlling melanogenesis by specialized cells. One of the theories of evolution of melanogenesis assumes that, due to the special chemical features of quinones, melanogenesis evolved initially as a non-enzymatic, side pathway, a consequence of the occurrence of oxygen in the atmosphere, and the necessity to depose toxic quinones in a less aggressive form (109). The primordial cells must have initially “learn” how to inhibit melanogenesis and, later on, how to control melanogenesis and its more efficient variant—eumelanogenesis (110). The protective role of melanin must have developed in parallel with the development of the endangerment by the photooxidative stress; otherwise, the threat of destabilization of genetic material might have quickly led to the Eigen error catastrophe (111, 112). This process must have taken a substantial part of evolution, and the process of melanomagenesis is, according to our latest suggestion, the step back not only in ontogenesis but also in phylogenesis (113).
Despite the fact that in clinically detectable melanomas there are numerous mutations (114), of whom many play a critical role in controlling melanogenesis, the actual genetic risk factors of melanoma in humans concern mainly the three types of genes: those controlling cell cycling and proliferation, those controlling telomerase, and those controlling tumor–immunity interactions (115). Even the typically melanoma-associated BRAF V600E/K mutation is not 100% associated with malignant tumors and is present in benign nevi (116). However, the data collected in this paper convincingly show that melanogenesis is a risk factor of malignant melanoma. This apparent paradox can be analyzed with special attention to the progression of the tumor and risk factors leading to massive metastasizing disease and consequently death of the patient. In dissecting this problem, it is important to pay attention to the processes of genetic regulation of melanogenesis and of cell proliferation and inhibition of apoptosis, which are in many cross-points entangled (5, 117, 118). To initiate melanomagenesis, some key mutations must be already present in the cellular genome, the genome of cells particularly predestinated to melanin production (115). Melanogenesis-activating factors (e.g., UV) may, at the same time, activate the pathways controlling melanogenesis, inhibiting apoptosis (because melanogenesis in cells undergoing apoptosis makes a little sense) and facilitating cell proliferation. If the two latter are mutated, this must enhance the “local error catastrophe” (111, 112), enhance proliferation of mutated cells, and, if the melanomagenic factor is still present, cumulate secondary mutations, destabilizing the full control of melanogenesis, leading to the release of toxic melanogenesis intermediates, decrease in melanin production, and enhancement of the full process in the loop of positive feedback, according to Figure 1.
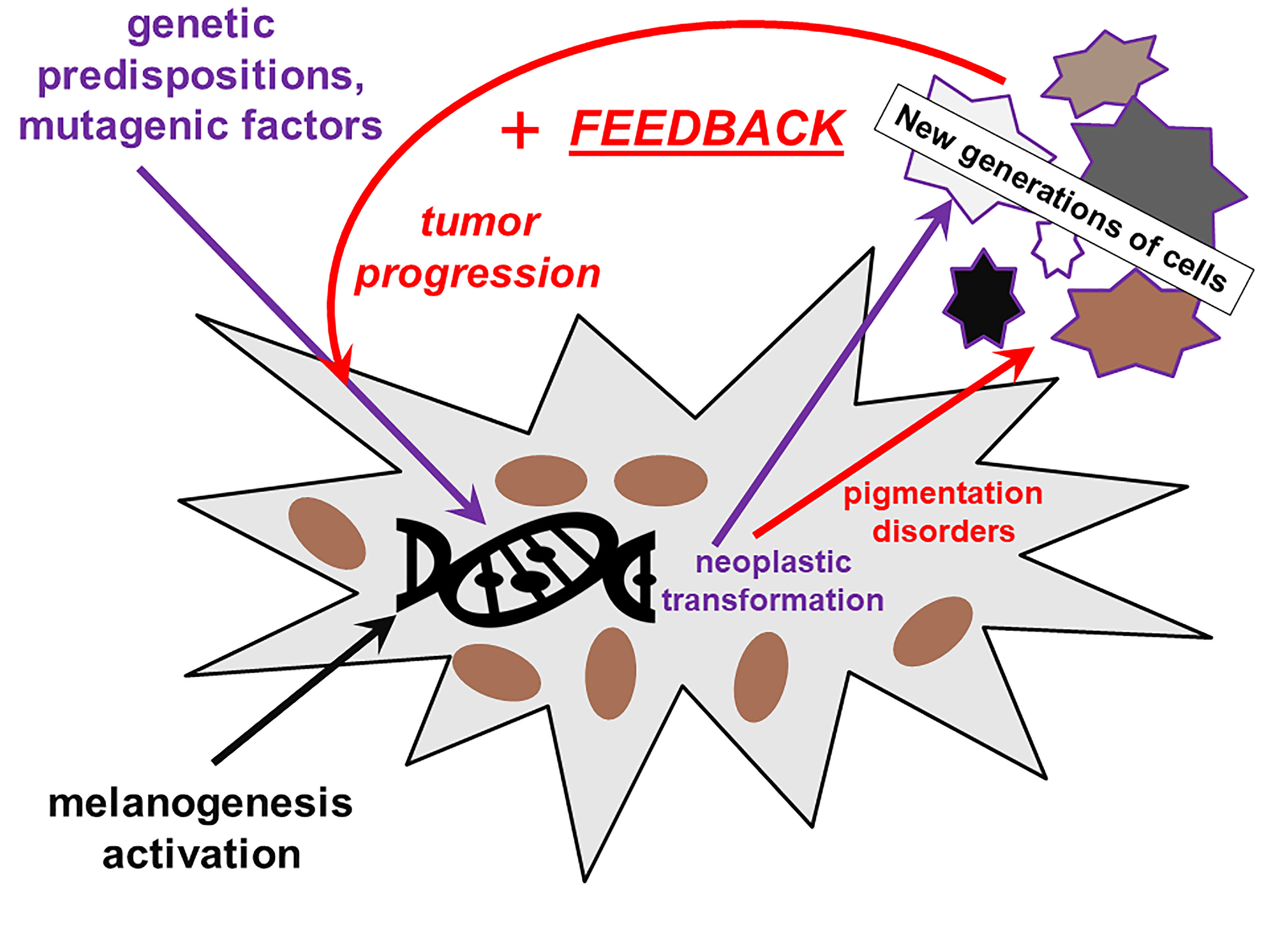
Figure 1 The role of melanin and of impairment of melanin synthesis in the initiation and progression of melanoma. The defective melanin synthesis in connection with external factors (UV) and genetic predispositions plays a role in melanomagenesis in a positive feedback loop.
The presence of melanin paradoxically does not exclude transformation of the melanocyte to the malignant state. In addition, some studies have indicated that melanin is necessary to induce melanoma. It has been called “a photocarcinogen for cutaneous malignant melanoma” by Moan et al. (117). Indeed, it was shown in mice that melanoma induction by ultraviolet A (320–400 nm) required the presence of melanin pigment and was associated with oxidative DNA damage within melanocytes (119). However, UVB initiated melanoma in a pigment-independent manner. It must be noted that among various types and subtypes of natural melanins, pheomelanin is the most dangerous one, as shown by Mitra et al. (120). In this paper, an Mc1R−/− on the background of C57BL/6 was used. Such mice do not express the melanocortin receptor type 1, but melanin is generated due to the activation of “rescue” melanogenetic pathways (5, 118). It leads to a limited activity of tyrosinase and synthesis of low amounts of pigment with a big proportion of pheomelanin. The mice produce yellow fur (just like in the “lethal yellow” mutants) and develop melanomas with no relations to UV when additionally transfected with the BRafV600E “oncogenic driver”. In black and albino (c/c) controls fewer melanomas developed with slower process of melanomagenesis (120). This had been predicted by in vitro studies on toxicity and phototoxicity of pheomelanin (80). More recently, melanin “in the dark” turned out to be toxic also for non-related keratinocytes (121, 122), which provides further argument for potentially toxic character of melanin, under certain conditions.
Melanin Pigment Can Attenuate Chemo- and Radiotherapy
Almost 50 years ago, the different effects of ionizing of pigmented and amelanotic melanoma cells were observed, and further studies confirmed these observations and the possibilities to enhance the melanoma cells sensitivity chemo- and radiotherapy by targeting the melanogenesis (123–127).
Melanins, acting as protective molecules with metal chelating properties, affect the anti-tumor drug chemosensitivity of melanoma cells. Studies of the Cichorek group on Bomirski hamster amelanotic and pigmented transplantable melanomas (103, 106, 128, 129) reported the differences in biology of these lines. They differed with ultrastructure, metabolism, growth rate, ability to undergo apoptosis, and others (103, 104, 130–134). The authors noticed the higher proliferation rate accompanied by decreased ability to undergo spontaneous apoptosis in amelanotic melanoma cells (130, 131, 135) and suggested that these properties reflect more aggressive phenotype. However, further studies of this group indicated higher susceptibility of amelanotic melanoma cells to camptothecin-induced apoptosis, with crucial role of caspases (136). The killing effects of camptothecin in melanoma cells depended on cell cycle phase with strongest effects on amelanotic than pigmented cells (137). In this same model, the higher expression of FasL, involved in the induction of cytotoxic T lymphocytes and NK cells death, on pigmented melanoma cells was observed (138). Furthermore, induced melanogenesis in amelanotic cell line changed the melanoma cells’ morphology and metabolism, decreased the number of cells, and provoked the displacement of cells to the subG0/G1 fraction, indicating the cell death pathway (139–142). Our study on SKMel-188 cell line with inducible melanogenesis had also showed the diversification of responses of melanomas with different melanization level to the treatment with chemotherapeutics (143). In this model, pigmented cells were more resistant to cyclophosphamide sensitized to cytotoxic action of cyclophosphamide with melanogenesis inhibitors (143). The inhibition of melanogenesis with N-phenylthiourea and D-penicillamine sensitized the pigmented melanomas to cyclophosphamide, with strongest effects of the latter (143) and to radiotherapy (144). We also observed the weaker effects of activity of 20(OH)D3 on melanoma cell with active melanogenesis (145). Protein-bound polysaccharides (PBPs) from Coriolus Versicolor fungus in human SKMel-188 melanoma cell line induced cytotoxicity in non-pigmented melanoma cells (146). This effect was caspase independent, accompanied by an increased intracellular reactive oxygen species, and was mediated by pathway involving RIP1 (146). Further studies revealed that in amelanotic melanoma cells, PBPs-induced death is related to inducing the RIPK1/RIPK3/MLKL-mediated necroptosis (147). In melanoma cells with active melanogenesis, the use of melanin synthesis inhibitors to induce depigmentation could also restore the susceptibility of melanoma cells to RIPK1/RIPK3/MLKL-mediated necroptosis (148). On the contrary, moderately pigmentated mouse and hamster melanoma cells were more susceptible to antiproliferative effect of vitamin D analogs (149). However, in human melanomas, induction of pigmentation led to an increased resistance to anticancer activity of vitamin D3 hydroxyderivatives (145). In this study, active forms of vitamin D were found to inhibit nuclear factor kappa B (NF-κB) activity in non-pigmented cells while having no effect on pigmented cells, and biopsies of non-pigmented and slightly pigmented melanomas displayed higher nuclear NF-κB p65 expression than highly pigmented melanomas.
A very probable hypothesis on the biological origin of melanin from the point of view of their adaptative values is their function as a radioprotector. Currently, this is difficult to imagine due to a relatively low level of natural background radiation, but in the past, there may have been periods of time when exposition of the Earth and all the living organisms on the ionizing radiation was much higher (150). The pigmented tissues and organs should be particularly resistant to ionizing radiation as compared with amelanotic materials.
This aspect brings about some notorious practical implications in tumor therapy. Radiotherapy belongs to the accepted and effective methods of tumor eradication, but this is not always the case for the pigmented tumors, in particular, skin melanoma (151). Indeed, research carried out at Jagiellonian University in Krakow, Poland in the 1970s revealed that the pigmented variants of skin melanoma obtained in Syrian golden hamsters (BHM) were much more resistant to radiotherapy than their amelanotic variants, although without treatment growing much faster that their pigmented counterparts (152). Soon, it turned out that this distinction is only valid for radiations of a low LET (linear energy transfer coefficient), such as X-ray. As a therapy, its effect strongly depends on the concentration of dioxygen. The effects of radiation are here clearly derivatives of active oxygen species generated as radiation products and sequestrated by melanin, if present. High-LET radiation (fast neutrons) causes damage to direct biological targets (DNA) and does not depend on dioxygen concentration. This subject was described in detail in a recent review (152).
Application of a proton beam turned out, consequently, to be an effective mode of therapy of melanotic tumors of the eye (uveal melanoma) (153, 154), and inhibition of melanogenesis (e.g., by the inhibition of tyrosinase activity via sequestration of copper) or increase in oxygen level became a promising way to sensitize melanoma tumors for radiotherapy (152, 155).
As the most dangerous factor associated with anticipated long-way cosmic travels (e.g., the manned mission to Mars) is the piercing component of the cosmic irradiation and solar wind (which does not reach the Earth surface thanks to our magnetosphere), melanin is recently being considered as an important radioprotector (156). It must be noted that the melanin is of fungal origin, and it plays a very special role in biological divagations as believed to be a new step in the biologic evolution (157). It turned out that some pigmented strains of Cryptococci develop better and grow faster under sublethal doses of gamma irradiation than their albino mutants (158). As the production of biomass is also improved, it looks as if a new type of metabolism—radiotrophy—has been described and identified. This fact may also be of a crucial importance for astrobiology. Also because of similar roles postulated for the so-called phytomelanins, substances of polyphenolic character produced in some groups of higher plants loosely related to animals and fungi (159, 160).
Intermediates of Melanogenesis Inhibit Immune Activity, While Melanogenesis-Related Proteins Are Targets for Immune Response
The intermediates of melanogenesis including quinones, semiquinones, quinonimines, and their corresponding radical form and reactive oxygen species (ROS) generated during this process are highly cytotoxic, therefore, they affect the viability of immune cells (reviewed in (1, 161). L-DOPA, an intermediate of melanogenesis, significantly inhibits proliferation of activated murine and human T and B lymphocytes while having less pronounced effects against fibroblasts or non-activated lymphocytes (162). Anti-proliferative effect against human T lymphocytes was also demonstrated with cell cycle arrest at the G1/0 phase with concomitant inhibition of interleukin (IL)-1, IL-6, tumor necrosis factor alpha (TNF-α), and IL-10 gene expression (143). These inhibitory effects were observed at a concentration of DOPA ranging from 1 to 100 µM, and the effects were independent whether L- or D-DOPA was used. Furthermore, cytotoxicity by IL-2-activated peripheral blood lymphocytes was low in pigmented vs. non-pigmented melanoma, and lymphocyte-mediated killing effect was significantly increased by inhibition of melanogenesis by N-phenylthiourea or D-penicillamine (D-pen) sensitized melanoma cells (143). Similarly, the inhibition of melanogenesis by D-pen or kojic acid in melanoma cells stimulated the IL-1, IL-2, IL-6, and IL-12 cytokine expression when co-cultured with peripheral blood mononuclear cells (148).
Separate studies have shown that incubation with either L-DOPA or dopamine resulted in a dose-dependent inhibition of lymphocyte proliferation and differentiation (163). L-DOPA, dopamine (DA) and norepinephrine dose-dependently suppressed mitogen-induced proliferation and differentiation of mouse lymphocytes, suppressed lymphocyte proliferation and cytokine production, and induced apoptosis (164). Others showed that DA suppressed expression of non-receptor tyrosine kinases, Lck and Fyn, and caused inhibition of anti-CD3 mAb-induced release of Th1 and Th2 cytokines, IL-2, interferon gamma (IFN-γ), and IL-4 from T cells (165). While these authors indicated involvement of dopamine receptors in these effects, other authors proposed that DA-induced inhibition of T-cell proliferation represented nonspecific cell killing (166–169). Although we cannot completely exclude a receptor-mediated effect for L-DOPA in immunosuppression, we favor nonspecific killing because DOPA and catecholamines undergo autoxidative transformation to melanin or neuromelanin, in a process that is regulated by pH and presence of metal cations. For example, L-DOPA inhibited glycoproteins phosphorylation (6, 170), which was dependent on Mn+2 (cation that induces DOPA oxidation) in the reaction mixture (171).
In other systems, the downregulation of the afferent phase of T-cell-mediated pulmonary inflammation and immunity was associated with melanin production by Cryptococcus neoformans (172) and DOPA-melanin pathway was associated with fungal resistance to phagocytosis by macrophages (173). In addition, immune cells can undergo apoptosis in response to the oxidative stress generated in the tumor environment (174). Note that melanogenesis generates a highly oxidative environment. Therefore, the above studies clearly indicate that melanogenesis either starting from DOPA or catecholamines will have an immunosuppressive effect within the tumor environment and/or systemically.
Melanogenesis-related proteins (MRPs) including tyrosinase, TRP-1, TRP-2, gp100, and MART-1 are classified as major histocompatibility complex (MHC)-restricted tumor antigens, and specific peptides derived from processing of MRPs can activate T-lymphocyte responses against melanoma cells (175–180). Such T-cell immune responses are variable because peptides derived from MRPs recognized by T cells are associated with specific MHC haplotypes and therefore limits their therapeutic utility (181, 182). Although the experimental effort for vaccination against melanoma using tyrosinase is being investigated (183), the major clinical effort is currently focused on checkpoint inhibitors (184, 185). In this context, the immunosuppressive effects of intermediates and byproducts of melanogenesis must be seriously considered by physicians, since immunotherapy is the most promising strategy in handling melanomas (186). We recommend inhibition of active melanogenesis in metastatic melanoma to improve the immune responses against the tumor. Interestingly, patients with advanced desmoplastic melanoma (amelanotic phenotype) had substantial clinical benefit from PD-1 or PD-L1 immune checkpoint blockade therapy (187), which is consistent with a recommendation presented above. Metastatic melanotic melanomas not only can release immunosuppressive intermediates but also tyrosinase and other enzymes secondary to cell damage or death leading to uncontrolled melanogenesis in the tumor environment or at the systemic level. In this context, immunization against tyrosinase may represent an additional step in eliminating this enzyme from the extracellular environment (182, 188). Moreover, during progression of advanced melanotic melanomas, levels of tyrosinase or different intermediates of melanogenesis are increased in the serum (189, 190), contributing to general melanosis (182).
Melanogenesis Can Enhance Melanoma Progression
The basic properties of melanin pigment and biochemistry of melanogenesis that are contributing to malignant transformation of melanocytes and their progression has been previously discussed earlier in this review. Briefly, active melanogenesis generates free radicals and highly reactive intermediates with genotoxic and mutagenic activities (55, 161, 191–195), while melanin, its monomers, pheomelanin in particular, under specific conditions can generate pro-oxidative environment and induce DNA damage (58, 60, 80, 196, 197). Melanin pigments can also have direct proinflammatory and pro-oxidant effects in keratinocytes, independently from light exposure (121). Thus, uncontrolled melanogenesis in the melanosome or outside (through autooxidation of its soluble metabolites), via depletion of major cell antioxidants and generation of ROS, and the direct action of quinone and semiquinone intermediates on RNA, DNA, and regulatory proteins will generate pro-mutagenic environment contributing to melanomagenesis (Figure 2).
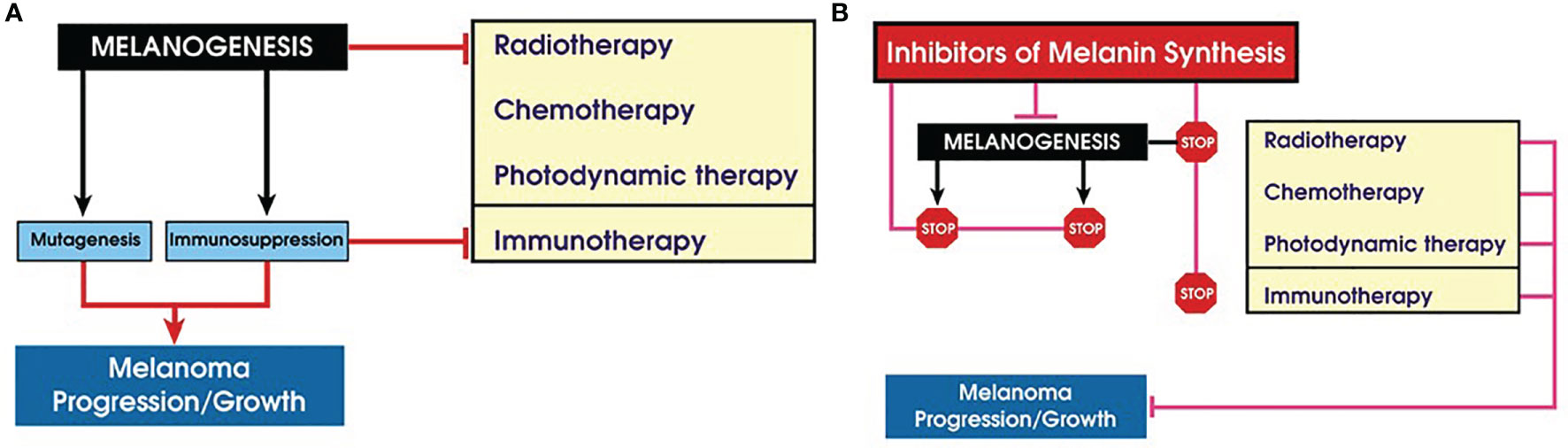
Figure 2 Role of melanogenesis and melanin in melanoma progression and therapy. (A) Melanogenesis stimulates melanoma progression and attenuates therapy; (B) inhibition of melanogenes sensitizes melanotic melanoma to diverse therapeutic modes.
Most malignant tumors rely on aerobic glycolysis for its growth, expansion, and progression (198–201). Different types of cellular and glucose metabolism play a central role in the natural history of tumors and their resistance to the therapy (200, 202, 203). Melanin pigment consumes oxygen (204, 205), while its intermediate L-DOPA can stimulate glycolysis in melanotic melanomas (133). It also stimulated pentose phosphate pathway with melanogenesis being involved in this process (206). Melanogenesis and L-DOPA oxidation can also lead to dramatic changes in glycoproteins phosphorylation pattern (171). The use of high-resolution magic angle spinning (HRMAS) nuclear magnetic resonance (NMR) has also shown that induction of melanogenesis is associated with changes in glucose and sodium acetate metabolism (142). We have also shown that induction of melanogenesis in melanoma cells leads not only to increased HIF-1α accumulation but also to the robust upregulation of HIF-1-dependent and independent pathways, suggesting a role for melanogenesis in the regulation of cellular metabolism and behavior of melanoma cells (Figure 3) (207). Furthermore, immunohistochemistry performed in the above study revealed higher levels of HIF-1α and GLUT-1 in advanced melanomas in comparison to melanocytic nevi or thin melanomas localized to the skin.
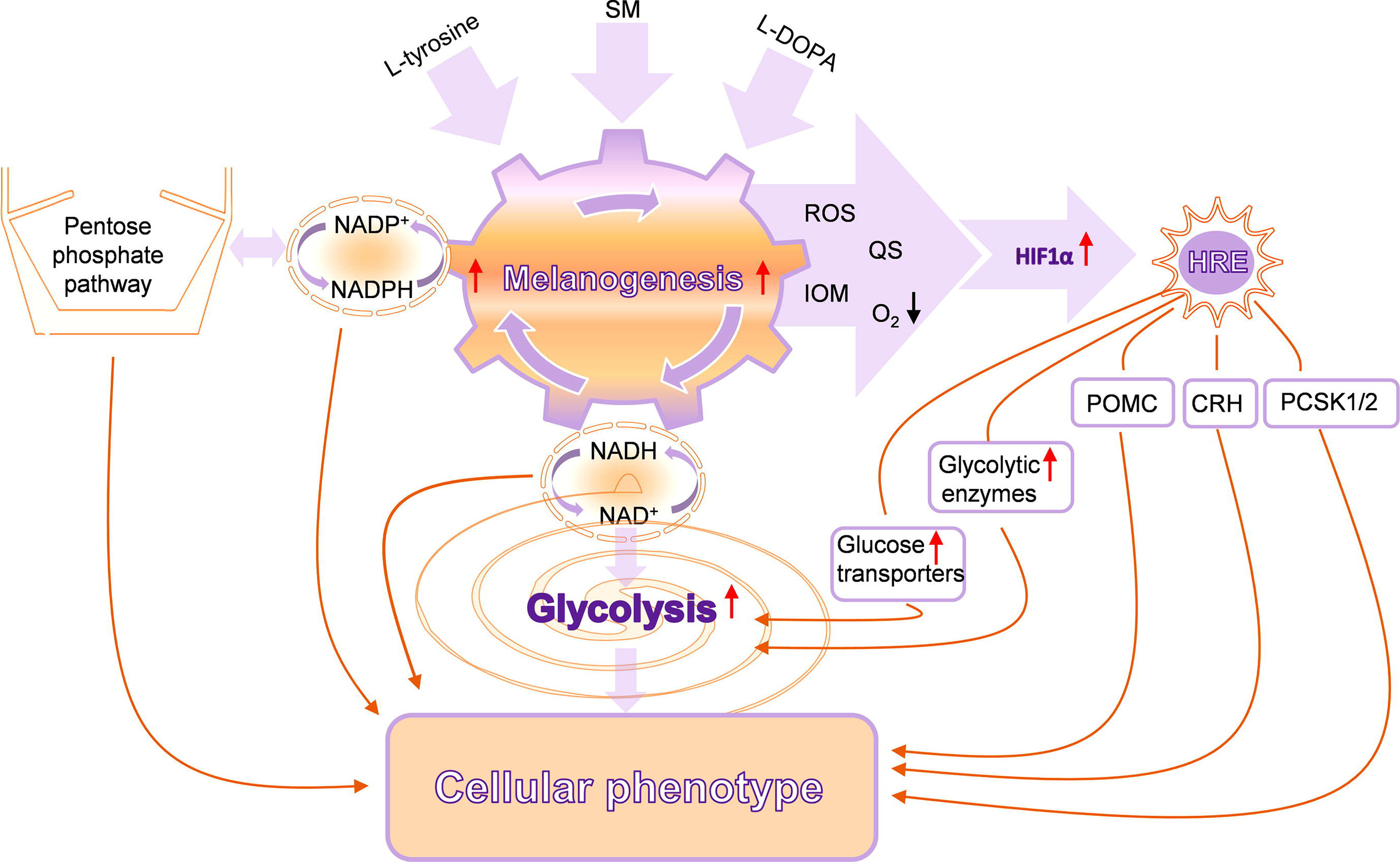
Figure 3 Complex interactions between melanogenesis, glucose metabolism, and HIF-1-dependent pathways. SM, stimulators of melanogenesis; ROS, reactive oxygen species; QS, quinones and semiquinones; IOM, intermediates of melanogenesis; POMC, proopiomelanocortin; CRH, corticotropin-releasing hormone; PCSK1/2, proprotein convertase subtilisin/kexin types 1 and 2. Reproduced from (207) with a permission from the publisher.
In addition, precursors to melanin are not only able to stimulate melanogenesis (208–210) but also stimulate expression and activity of its regulators such as melanocyte-stimulating hormone (MSH) receptors (210–212) and production of proopiomelanocortin (POMC) and POMC-derived peptides (213). Of note, POMC peptides including MSH are immunosuppressive (214–216), and increased expression of POMC peptides was noted during progression of melanomas to advanced stages (217–222).
In summary, stimulation of melanogenesis leads to a pro-oxidative and mutagenic environment and rewires cellular metabolism, which includes stimulation of glycolysis and HIF-1α activation that, combined with immunosuppressive effects, would lead to melanoma progression and resistance to immunotherapy. The biophysical properties of melanin would also make melanoma resistant to chemo- and radiotherapy. This indicates that inhibition of melanogenesis in advanced melanotic melanomas would be an educated approach to improve immunotherapy, chemotherapy, and radiotherapy or perhaps by itself will attenuate melanoma growth (Figure 2B). However, there is another aspect of melanin pigmentation that makes the Yin and Yang issue in the case of melanoma even more intriguing. It is related to mechanistic effects of melanosomes that apparently play a role in the trans-migration abilities of melanoma cells in vitro (223). In a follow-up study, it was demonstrated that human melanoma cells containing melanin were less capable to spread in nude mice than melanoma cells without the pigment (224). These results suggest that the presence of melanin can inhibit formation of melanoma metastases. However, it remains to be tested whether either in vivo simulation or inhibition of melanogenesis would affect the metastatic cascade. Under in vitro conditions, stimulation of melanogenesis leads to changes in adhesive properties of melanoma cells and detachment of heavily melanized cells from the substratum (141, 152, 207, 225). Such process in vivo could lead to the detachment of cells from the primary or secondary tumors, a hypothesis that remains to be tested experimentally. Therefore, further research is needed on the relative contribution of the pro-oxidizing conditions induced by melanogenesis or melanin itself or the inhibitory effects of melanin granules, due to their mechanical properties, in melanoma progression to metastatic stage.
Clinicopathological Correlation Between Melanogenesis and Melanoma Progression
Melanin can affect the clinical course of both cutaneous (Figure 4) and uveal melanomas. Our previous studies revealed that patients with cutaneous pigmented metastasizing melanomas were characterized by poorer prognosis as assessed by both shorter disease-free survival (DFS) and overall survival (OS) than amelanotic cases (226). Similarly, the pigmentation of lymph node melanoma metastases was related to a worse prognosis (shorter OS and DFS (226). In addition, decreasing pigmentation in metastatic tumors versus primary melanomas was related to longer DFS (226). In this same group of patients, we found a significant lower level of melanin in primary pT3-4 versus pT1-2 melanoma, with its concomitant significantly elevated level in reticular versus papillary dermis. In addition, pT3–4 primary melanomas that developed metastases were characterized by significantly higher pigmentation than pN0 melanomas. The melanization of lymph node melanoma metastases of pT4 tumors were more pronounced than those of pT2–3 tumors similarly to melanization of lymph node melanoma metastases in patients that developed distant metastases (pM1) (227). Since significantly shorter OS and DFS in stage III and IV pigmented melanomas was observed, we analyzed the radiotherapy efficiency in melanoma patients in relation to the melanization level and found better OS in patients with amelanotic melanoma treated with RTH and CHTH or RTH (227).
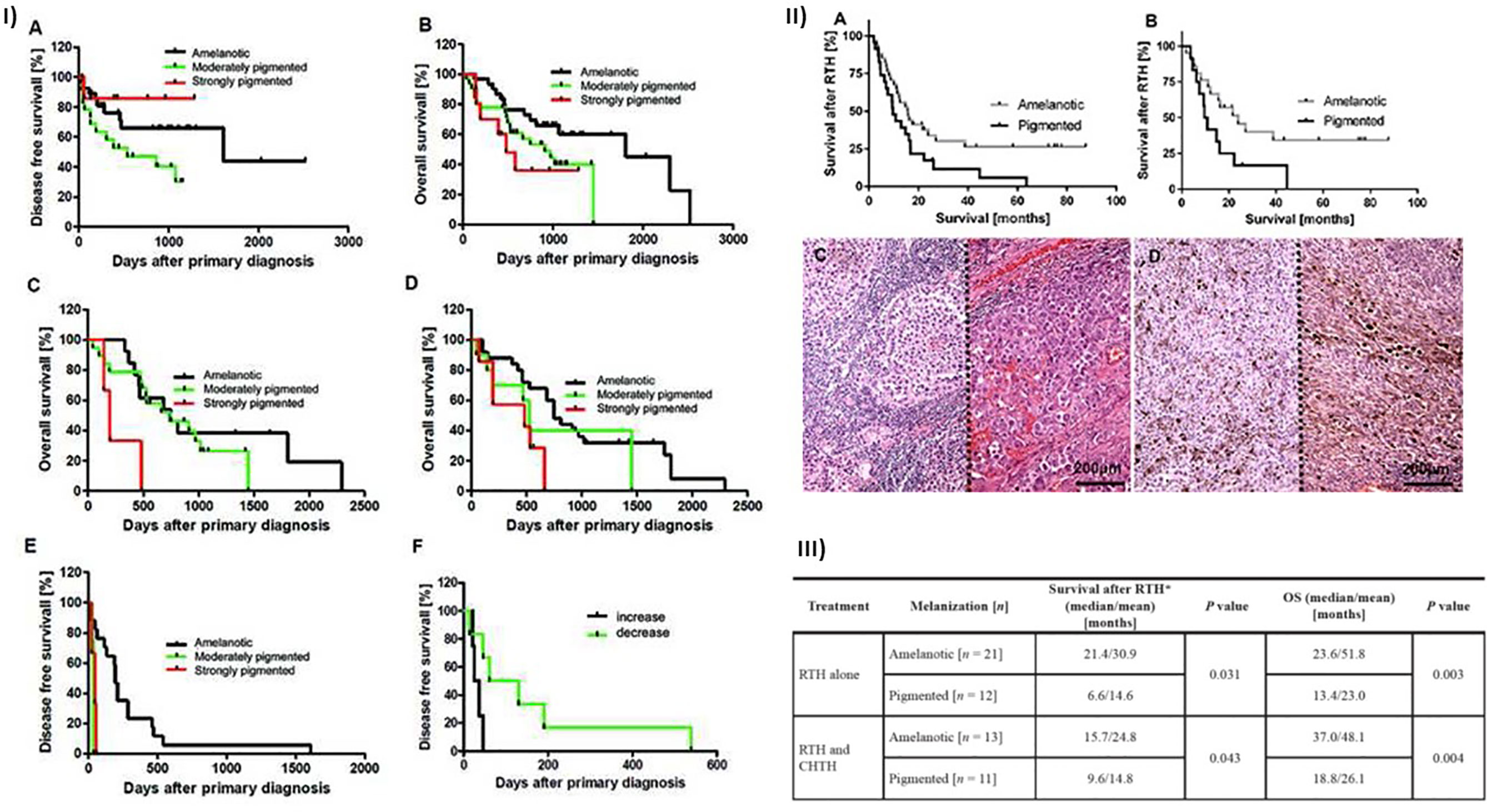
Figure 4 Relationship between melanization and survival of cutaneous melanoma patients. (I) Correlation between melanin level and disease-free (DFS) and overall (OS) survival in patients with melanomas (n = 73). Reproduced from (226) with a permission from the publisher. (A) DFS and (B) OS curves in all melanoma patients (localized [n = 37]) and metastatic disease [n = 36]) stratified according to melanin content. OS curves of primary metastasizing melanomas [(C); χ2 = 7.554, p = 0.0229; amelanotic vs. strongly pigmented: χ2 = 6.113, p = 0.0134, χ2 = 6.570, p = 0.0104; amelanotic vs. moderately pigmented: χ2 = 5.656, p = 0.0174] and lymph node metastases [(D); χ2 = 3.972, p = 0.0463; amelanotic vs. strongly pigmented: χ2 = 6.603, p = 0.0102] stratified according to melanin content. (E) DFS curves of melanoma lymph node metastases stratified according to melanin content in metastatic tumors (χ2 = 11.43, p = 0.0033; amelanotic vs. moderately pigmented: χ2 = 10.23, p = 0.0014; amelanotic vs. strongly pigmented: χ2 = 7.812, p = 0.0052). (F) DFS curves of metastatic melanomas stratified into groups with decreased or increased melanin content in metastases relative to primary melanomas (log-rank test, χ2 = 4.071, p = 0.0436). (II) Survival time of melanoma patients after radiotherapy (RTH). (A) Survival of melanoma patients received both RTH and CHTH or only RTH (n = 57; χ2 = 4.62, p = 0.03). (B) Survival of melanoma patients received only RTH treatment (n = 33; χ2 = 4.33, p = 0.04). Melanomas were stratified according to melanin level in melanoma metastases. Representative amelanotic (C) and pigmented [(D); two cases separated with dotted line] lymph node melanoma metastases. Scale bars = 200 µm. Reproduced from (227) with a permission from the publisher. (III) Survival after RTH and OS in melanoma patients with pigmented and amelanotic metastatic melanomas that were confirmed histologically, and who received radiotherapy (n = 57). CTHT, chemotherapy; RTH, radiotherapy; OS, overall survival time from primary diagnosis to the end of observation or death of patient, *survival time from the end of radiotherapy treatment to the end of observation or death of patient. Reproduced from (227) with a permission from the publisher.
Shields’s group published the results consistent with our data (228, 229). For uveal melanomas stage III, pigmentation was one of the factors predicting metastases, while for stage II melanomas, pigmentation was one factor predicting death (228). The other paper based on multivariate analysis reported the pigmentation as a factor related to higher risk of death and increased metastases rate independently on race (229). In addition, the continuous increase in the pigmentation with tumor advancement was also reported (228).
In addition, the vitamin D receptor (VDR) and retinoic acid orphan receptors (ROR)α and γ expression decreased in melanized melanoma cells in comparison to amelanotic or poorly pigmented cells (230, 231). Similarly, in uveal melanomas, melanin level inversely correlated with VDR expression (232). In addition, the expression of the enzyme-activating vitamin D (CYP27B1) was inversely related to melanin in melanoma cells in vivo and melanoma cells cultured in vitro (233), while the expression of CYP24A1 was lower in non-pigmented melanomas vs. highly melanized ones (234). These clinical–pathological studies indicate that melanization level can affect the local vitamin D endocrine system, which plays an important role in melanoma biology (235, 236).
Concluding Remarks and Future Directions
It is commonly believed that the most important biological function of melanin in humans is the protection against noxious insults including UVR-induced cancerogenesis and melanomagenesis. However, under certain conditions, melanin can also be phototoxic. Although such different actions of melanin may appear difficult to reconcile, conceivable explanation for both photoprotective and phototoxic properties of melanin is based on unusual physicochemical and photochemical properties of melanin pigments. Over the last decades, major advances have been made on the communication between melanogenesis and cell energy yielding metabolism, immune functions, and other networks regulating local homeostasis and melanocytes activities in negative and positive fashions. For example, the biosynthesis of melanin affects cellular metabolism because this pathway consists of a series of tightly coupled oxidoreduction reaction, and active melanogenesis and melanin consume oxygen, leading to relative intracellular hypoxia. Its intermediates, such as free radicals and highly reactive quinone compounds, can display cytotoxic, genotoxic, and mutagenic activities or other regulatory functions. In addition, melanin acts as a scavenger of free radicals, metal cations, and cellular toxins including chemotherapeutics (5, 237, 238). In normal melanocytes, the process of melanin synthesis is highly controlled, since it takes place within the boundaries of specialized membrane-bound organelles, the melanosomes. In such conditions, the process of melanin synthesis plays a protective role against environmental insults and protects against UVR-induced cancers. However, despite its protective role against UVR, melanin pigment appears to have the contribution to malignant transformation of melanocytes (117, 120, 239). In melanoma cells, this process can be dysregulated with intermediates of melanogenesis leaking outside melanosomes, which will affect the behavior of melanoma cells or their surrounding environment (Figure 3), which is further discussed in (161, 240). Therefore, an uncontrolled melanogenesis has a role, perhaps critical, in the progression of melanotic melanoma, and, together with melanin pigment, it can attenuate radio-, chemo- and phototherapy and immunotherapy (Figure 2). This hypothesis is supported by clinicopathological data showing that increased melanin pigmentation leads to shorter OST and DFST (226, 227) and identifying melanogenesis as a risk factor in uveal melanomas (228, 229). Thus, the inhibition of melanogenesis can improve diverse therapeutic modes or perhaps may even directly improve the clinical outcome of melanotic melanomas (Figure 2).
There are several challenges in understanding a broader context of the Yin and Yang action of melanogenesis and melanin pigment that is protective under physiological and destructive under pathological conditions (Figure 5). These actions could depend on physicochemical and biochemical properties described above. However, the simple linear interactions are unlikely and should be placed in the larger context of local regulatory networks including bidirectional communication with CRH/POMC/MSH&ACTH axis, HIF-1α, NF-κβ, MITF, PKA, PKC, second messengers, phosphorylation cascades, and nuclear receptor signaling (including vitamin D) that would act in a nonlinear fashion involving direct and indirect effects. These pathways would act as sensors of melanogenesis distress signals and their regulators under physiological conditions. When melanogenesis is deregulated, regulatory pathways will be out of tune, leading to the amplification of the disruptive signals and “cellular chaos” on the local level facilitating tumor progression and resistance to therapy (241). The effects of melanogenesis on the local and systemic immune functions can be crucial in the context of immunotherapy and immune checkpoint inhibitors that are emerging as a frontline treatment for melanoma.
Therefore, one can envision use of well-established inhibitors of melanogenesis such as N-phenylthiourea, D-penicillamine (copper chelator), kojic acid, or other non-toxic inhibitors of tyrosinase to enhance radio-, chemo-, or immunotherapy of melanotic melanomas. Furthermore, diet deficient in melanin precursors such as L-phenylalanine and L-tyrosine can be used to systematically inhibit melanogenesis during treatment of melanotic melanomas, however, with some limitations (242–244). We also acknowledge that an opposite strategy, use of melanin precursors for experimental therapy of melanoma, was proposed (245–249), however, this strategy did not reach the patient's bed.
In summary, the Yin and Yang effects of melanogenesis should be considered in mechanism-oriented preclinical studies on melanomagenesis and melanoma progression and clinical effort to handle this devastating disease for the benefit of the patients.
Author Contributions
All authors listed have made a substantial, direct, and intellectual contribution to the work and approved it for publication.
Funding
Writing of the review was supported in part by grants from NIH R01AR073004-01A1, R01AR071189-01A1, and R21AI149267-01A1, and VA merit award (1I01BX004293-01A1) to ATS, from the National Science Center, Poland no. 2014/15/B/NZ4/00751 to AAB and 2017/27/B/ST5/02631 to TS.
Conflict of Interest
The authors declare that the research was conducted in the absence of any commercial or financial relationships that could be construed as a potential conflict of interest.
Publisher’s Note
All claims expressed in this article are solely those of the authors and do not necessarily represent those of their affiliated organizations, or those of the publisher, the editors and the reviewers. Any product that may be evaluated in this article, or claim that may be made by its manufacturer, is not guaranteed or endorsed by the publisher.
References
1. Nordlund JJ, Boissy RE, Hearing VJ, King RA, Oetting WS, Ortonne JP. The Pigmentary System: Physiology and Pathophysiology. 2nd ed. Oxford: Blackwell Publishing Ltd (2006).
2. Slominski AT, Zmijewski MA, Plonka PM, Szaflarski JP, Paus R. How UV Light Touches the Brain and Endocrine System Through Skin, and Why. Endocrinology (2018) 159:1992–2007. doi: 10.1210/en.2017-03230
3. Jablonski NG. The Evolution of Human Skin Pigmentation Involved the Interactions of Genetic, Environmental, and Cultural Variables. Pigment Cell Melanoma Res (2021) 34:707–29. doi: 10.1111/pcmr.12976
4. Fajuyigbe D, Lwin SM, Diffey BL, Baker R, Tobin DJ, Sarkany RPE, et al. Melanin Distribution in Human Epidermis Affords Localized Protection Against DNA Photodamage and Concurs With Skin Cancer Incidence Difference in Extreme Phototypes. FASEB J (2018) 32:3700–6. doi: 10.1096/fj.201701472R
5. Slominski A, Tobin DJ, Shibahara S, Wortsman J. Melanin Pigmentation in Mammalian Skin and Its Hormonal Regulation. Physiol Rev (2004) 84:1155–228. doi: 10.1152/physrev.00044.2003
6. Slominski A, Zmijewski MA, Pawelek J. L-Tyrosine and L-Dihydroxyphenylalanine as Hormone-Like Regulators of Melanocyte Functions. Pigment Cell Melanoma Res (2012) 25:14–27. doi: 10.1111/j.1755-148X.2011.00898.x
7. Slominski A, Wortsman J, Plonka PM, Schallreuter KU, Paus R, Tobin DJ. Hair Follicle Pigmentation. J Invest Dermatol (2005) 124:13–21. doi: 10.1111/j.0022-202X.2004.23528.x
8. Singh SK, Kurfurst R, Nizard C, Schnebert S, Perrier E, Tobin DJ. Melanin Transfer in Human Skin Cells Is Mediated by Filopodia–A Model for Homotypic and Heterotypic Lysosome-Related Organelle Transfer. FASEB J (2010) 24:3756–69. doi: 10.1096/fj.10-159046
9. O'Sullivan JDB, Nicu C, Picard M, Cheret J, Bedogni B, Tobin DJ, et al. The Biology of Human Hair Greying. Biol Rev Camb Philos Soc (2021) 96:107–28. doi: 10.1111/brv.12648
10. Joly-Tonetti N, Wibawa JID, Bell M, Tobin DJ. An Explanation for the Mysterious Distribution of Melanin in Human Skin: A Rare Example of Asymmetric (Melanin) Organelle Distribution During Mitosis of Basal Layer Progenitor Keratinocytes. Br J Dermatol (2018) 179:1115–26. doi: 10.1111/bjd.16926
11. Prota G. The Chemistry of Melanins and Melanogenesis. Fortschr Chem Org Naturst (1995) 64:93–148. doi: 10.1007/978-3-7091-9337-2_2
12. d'Ischia M, Wakamatsu K, Napolitano A, Briganti S, Garcia-Borron JC, Kovacs D, et al. Melanins and Melanogenesis: Methods, Standards, Protocols. Pigment Cell Melanoma Res (2013) 26:616–33. doi: 10.1111/pcmr.12121
13. Hida T, Wakamatsu K, Sviderskaya EV, Donkin AJ, Montoliu L, Lynn Lamoreux M, et al. Agouti Protein, Mahogunin, and Attractin in Pheomelanogenesis and Melanoblast-Like Alteration of Melanocytes: A cAMP-Independent Pathway. Pigment Cell Melanoma Res (2009) 22:623–34. doi: 10.1111/j.1755-148X.2009.00582.x
14. Berryere TG, Kerns JA, Barsh GS, Schmutz SM. Association of an Agouti Allele With Fawn or Sable Coat Color in Domestic Dogs. Mamm Genome (2005) 16:262–72. doi: 10.1007/s00335-004-2445-6
15. He L, Gunn TM, Bouley DM, Lu XY, Watson SJ, Schlossman SF, et al. A Biochemical Function for Attractin in Agouti-Induced Pigmentation and Obesity. Nat Genet (2001) 27:40–7. doi: 10.1038/83741
16. Kerns JA, Olivier M, Lust G, Barsh GS. Exclusion of Melanocortin-1 Receptor (Mc1r) and Agouti as Candidates for Dominant Black in Dogs. J Hered (2003) 94:75–9. doi: 10.1093/jhered/esg01
17. Kaelin CB, Xu X, Hong LZ, David VA, McGowan KA, Schmidt-Kuntzel A, et al. Specifying and Sustaining Pigmentation Patterns in Domestic and Wild Cats. Science (2012) 337:1536–41. doi: 10.1126/science.1220893
18. Van Raamsdonk CD, Barsh GS, Wakamatsu K, Ito S. Independent Regulation of Hair and Skin Color by Two G Protein-Coupled Pathways. Pigment Cell Melanoma Res (2009) 22:819–26. doi: 10.1111/j.1755-148X.2009.00609.x
19. Hyter S, Coleman DJ, Ganguli-Indra G, Merrill GF, Ma S, Yanagisawa M, et al. Endothelin-1 Is a Transcriptional Target of P53 in Epidermal Keratinocytes and Regulates Ultraviolet-Induced Melanocyte Homeostasis. Pigment Cell Melanoma Res (2013) 26:247–58. doi: 10.1111/pcmr.12063
20. Wang Z, Coleman DJ, Bajaj G, Liang X, Ganguli-Indra G, Indra AK. RXRalpha Ablation in Epidermal Keratinocytes Enhances UVR-Induced DNA Damage, Apoptosis, and Proliferation of Keratinocytes and Melanocytes. J Invest Dermatol (2011) 131:177–87. doi: 10.1038/jid.2010.290
21. Hyter S, Bajaj G, Liang X, Barbacid M, Ganguli-Indra G, Indra AK. Loss of Nuclear Receptor RXRalpha in Epidermal Keratinocytes Promotes the Formation of Cdk4-Activated Invasive Melanomas. Pigment Cell Melanoma Res (2010) 23:635–48. doi: 10.1111/j.1755-148X.2010.00732.x
22. Slominski AT, Zmijewski MA, Zbytek B, Tobin DJ, Theoharides TC, Rivier J. Key Role of CRF in the Skin Stress Response System. Endocr Rev (2013) 34:827–84. doi: 10.1210/er.2012-1092
23. Slominski A, Plonka PM, Pisarchik A, Smart JL, Tolle V, Wortsman J, et al. Preservation of Eumelanin Hair Pigmentation in Proopiomelanocortin-Deficient Mice on a Nonagouti (a/a) Genetic Background. Endocrinology (2005) 146:1245–53. doi: 10.1210/en.2004-0733
24. Korner A, Pawelek J. Mammalian Tyrosinase Catalyzes Three Reactions in the Biosynthesis of Melanin. Science (1982) 217:1163–5. doi: 10.1126/science.6810464
25. Lerner AB, Fitzpatrick TB. Biochemistry of Melanin Formation. Physiol Rev (1950) 30:91–126. doi: 10.1152/physrev.1950.30.1.91
26. Hearing VJ, Tsukamoto K. Enzymatic Control of Pigmentation in Mammals. FASEB J (1991) 5:2902–9. doi: 10.1096/fasebj.5.14.1752358
27. d'Ischia M, Wakamatsu K, Cicoira F, Di Mauro E, Garcia-Borron JC, Commo S, et al. Melanins and Melanogenesis: From Pigment Cells to Human Health and Technological Applications. Pigment Cell Melanoma Res (2015) 28:520–44. doi: 10.1111/pcmr.12393
28. Ito S. The IFPCS Presidential Lecture: A Chemist's View of Melanogenesis. Pigment Cell Res (2003) 16:230–6. doi: 10.1034/j.1600-0749.2003.00037.x
29. Ito S, Wakamatsu K. Melanin Chemistry and Melanin Precursors in Melanoma. J Invest Dermatol (1989) 92:261S–5S. doi: 10.1111/1523-1747.ep13076587
30. Tsukamoto K, Palumbo A, D'Ischia M, Hearing VJ, Prota G. 5,6-Dihydroxyindole-2-Carboxylic Acid Is Incorporated in Mammalian Melanin. Biochem J (1992) 286(Pt 2):491–5. doi: 10.1042/bj2860491
31. Tsukamoto K, Jackson IJ, Urabe K, Montague PM, Hearing VJ. A Second Tyrosinase-Related Protein, TRP-2, Is a Melanogenic Enzyme Termed DOPAchrome Tautomerase. EMBO J (1992) 11:519–26. doi: 10.1002/j.1460-2075.1992.tb05082.x
32. Orlow SJ, Osber MP, Pawelek JM. Synthesis and Characterization of Melanins From Dihydroxyindole-2-Carboxylic Acid and Dihydroxyindole. Pigment Cell Res (1992) 5:113–21. doi: 10.1111/j.1600-0749.1992.tb00007.x
33. Chakraborty AK, Orlow SJ, Pawelek JM. Evidence That Dopachrome Tautomerase Is a Ferrous Iron-Binding Glycoprotein. FEBS Lett (1992) 302:126–8. doi: 10.1016/0014-5793(92)80421-C
34. Pawelek JM. After Dopachrome? Pigment Cell Res (1991) 4:53–62. doi: 10.1111/j.1600-0749.1991.tb00315.x
35. Pawelek JM. Dopachrome Conversion Factor Functions as an Isomerase. Biochem Biophys Res Commun (1990) 166:1328–33. doi: 10.1016/0006-291X(90)91011-G
36. Chakraborty AK, Platt JT, Kim KK, Kwon BS, Bennett DC, Pawelek JM. Polymerization of 5,6-Dihydroxyindole-2-Carboxylic Acid to Melanin by the Pmel 17/Silver Locus Protein. Eur J Biochem (1996) 236:180–8. doi: 10.1111/j.1432-1033.1996.t01-1-00180.x
37. Aroca P, Garcia-Borron JC, Solano F, Lozano JA. Regulation of Mammalian Melanogenesis. I: Partial Purification and Characterization of a Dopachrome Converting Factor: Dopachrome Tautomerase. Biochim Biophys Acta (1990) 1035:266–75. doi: 10.1016/0304-4165(90)90088-E
38. Jimenez-Cervantes C, Solano F, Kobayashi T, Urabe K, Hearing VJ, Lozano JA, et al. A New Enzymatic Function in the Melanogenic Pathway. The 5,6-Dihydroxyindole-2-Carboxylic Acid Oxidase Activity of Tyrosinase-Related Protein-1 (TRP1). J Biol Chem (1994) 269:17993–8000. doi: 10.1016/S0021-9258(17)32408-0
39. Solano F, Martinez-Liarte JH, Jimenez-Cervantes C, Garcia-Borron JC, Lozano JA. Dopachrome Tautomerase Is a Zinc-Containing Enzyme. Biochem Biophys Res Commun (1994) 204:1243–50. doi: 10.1006/bbrc.1994.2596
40. Palumbo A, Solano F, Misuraca G, Aroca P, Garcia Borron JC, Lozano JA, et al. Comparative Action of Dopachrome Tautomerase and Metal Ions on the Rearrangement of Dopachrome. Biochim Biophys Acta (BBA) - Gen Subj (1991) 1115:1–5. doi: 10.1016/0304-4165(91)90003-Y
41. Ito S, Suzuki N, Takebayashi S, Commo S, Wakamatsu K. Neutral pH and Copper Ions Promote Eumelanogenesis After the Dopachrome Stage. Pigment Cell Melanoma Res (2013) 26:817–25. doi: 10.1111/pcmr.12137
42. Jara JR, Solano F, Garcia-Borron JC, Aroca P, Lozano JA. Regulation of Mammalian Melanogenesis. II: The Role of Metal Cations. Biochim Biophys Acta (1990) 1035:276–85. doi: 10.1016/0304-4165(90)90089-F
43. Plonka P, Handjiski B, Michalczyk D, Popik M, Paus R. Oral Zinc Sulphate Causes Murine Hair Hypopigmentation and Is a Potent Inhibitor of Eumelanogenesis In Vivo. Br J Dermatol (2006) 155:39–49. doi: 10.1111/j.1365-2133.2006.07376.x
44. Napolitano A, Di Donato P, Prota G. Zinc-Catalyzed Oxidation of 5-S-Cysteinyldopa to 2,2'-Bi(2H-1,4-Benzothiazine): Tracking the Biosynthetic Pathway of Trichochromes, the Characteristic Pigments of Red Hair. J Org Chem (2001) 66:6958–66. doi: 10.1021/jo010320g
45. Land EJ, Riley PA. Spontaneous Redox Reactions of Dopaquinone and the Balance Between the Eumelanic and Phaeomelanic Pathways. Pigment Cell Res (2000) 13:273–7. doi: 10.1034/j.1600-0749.2000.130409.x
46. Land EJ, Ito S, Wakamatsu K, Riley PA. Rate Constants for the First Two Chemical Steps of Eumelanogenesis. Pigment Cell Res (2003) 16:487–93. doi: 10.1034/j.1600-0749.2003.00082.x
47. Land EJ, Ramsden CA, Riley PA. Tyrosinase Autoactivation and the Chemistry of Ortho-Quinone Amines. Acc Chem Res (2003) 36:300–8. doi: 10.1021/ar020062p
48. Ito S, Wakamatsu K. Quantitative Analysis of Eumelanin and Pheomelanin in Humans, Mice, and Other Animals: A Comparative Review. Pigment Cell Res (2003) 16:523–31. doi: 10.1034/j.1600-0749.2003.00072.x
49. Clancy CMR, Simon JD. Ultrastructural Organization of Eumelanin From Sepia Officinalis Measured by Atomic Force Microscopy. Biochemistry (2001) 40:13353–60. doi: 10.1021/bi010786t
50. Lin WP, Lai HL, Liu YL, Chiung YM, Shiau CY, Han JM, et al. Effect of Melanin Produced by a Recombinant Escherichia Coli on Antibacterial Activity of Antibiotics. J Microbiol Immunol Infect (2005) 38:320–6.
51. d'Ischia M, Napolitano A, Pezzella A, Meredith P, Sarna T. Chemical and Structural Diversity in Eumelanins: Unexplored Bio-Optoelectronic Materials. Angewandte Chemie Int Edition (2009) 48:3914–21. doi: 10.1002/anie.200803786
52. Moellmann G, Slominski A, Kuklinska E, A. B. L. Regulation of Melanogenesis in Melanocytes. Pigment Cell Res (1988) 1:79–87. doi: 10.1111/j.1600-0749.1988.tb00798.x
53. Hee JS, Mitchell SM, Liu X, Leonhardt RM. Melanosomal Formation of PMEL Core Amyloid Is Driven by Aromatic Residues. Sci Rep (2017) 7:44064. doi: 10.1038/srep44064
54. Land EJ, Ramsden CA, Riley PA. Quinone Chemistry and Melanogenesis. Methods Enzymol (2004) 378:88–109. doi: 10.1016/S0076-6879(04)78005-2
55. Pawelek JM, Lerner AB. 5,6-Dihydroxyindole Is a Melanin Precursor Showing Potent Cytotoxicity. Nature (1978) 276:626–8. doi: 10.1038/276627a0
56. Glass K, Ito S, Wilby PR, Sota T, Nakamura A, Bowers CR, et al. Direct Chemical Evidence for Eumelanin Pigment From the Jurassic Period. Proc Natl Acad Sci USA (2012) 109:10218–23. doi: 10.1073/pnas.1118448109
57. Kim E, Leverage WT, Liu Y, Panzella L, Alfieri ML, Napolitano A, et al. Paraquat-Melanin Redox-Cycling: Evidence From Electrochemical Reverse Engineering. ACS Chem Neurosci (2016) 7:1057–67. doi: 10.1021/acschemneuro.6b00007
58. Kim E, Panzella L, Napolitano A, Payne GF. Redox Activities of Melanins Investigated by Electrochemical Reverse Engineering: Implications for Their Roles in Oxidative Stress. J Invest Dermatol (2020) 140:537–43. doi: 10.1016/j.jid.2019.09.010
59. Ito S, Pilat A, Gerwat W, Skumatz CM, Ito M, Kiyono A, et al. Photoaging of Human Retinal Pigment Epithelium Is Accompanied by Oxidative Modifications of Its Eumelanin. Pigment Cell Melanoma Res (2013) 26:357–66. doi: 10.1111/pcmr.12078
60. Ito S, Wakamatsu K, Sarna T. Photodegradation of Eumelanin and Pheomelanin and Its Pathophysiological Implications. Photochem Photobiol (2018) 94:409–20. doi: 10.1111/php.12837
61. Felix CC, Hyde JS, Sarna T, Sealy RC. Melanin Photoreactions in Aerated Media: Electron Spin Resonance Evidence for Production of Superoxide and Hydrogen Peroxide. Biochem Biophys Res Commun (1978) 84:335–41. doi: 10.1016/0006-291X(78)90175-4
62. Sealy RC, Hyde JS, Felix CC, Menon IA, Prota G, Swartz HM, et al. Novel Free Radicals in Synthetic and Natural Pheomelanins: Distinction Between Dopa Melanins and Cysteinyldopa Melanins by ESR Spectroscopy. Proc Natl Acad Sci USA (1982) 79:2885–9. doi: 10.1073/pnas.79.9.2885
63. Monks TJ, Hanzlik RP, Cohen GM, Ross D, Graham DG. Quinone Chemistry and Toxicity. Toxicol Appl Pharmacol (1992) 112:2–16. doi: 10.1016/0041-008X(92)90273-U
64. Pezzella A, Panzella L, Crescenzi O, Napolitano A, Navaratman S, Edge R, et al. Short-Lived Quinonoid Species From 5,6-Dihydroxyindole Dimers En Route to Eumelanin Polymers: Integrated Chemical, Pulse Radiolytic, and Quantum Mechanical Investigation. J Am Chem Soc (2006) 128:15490–8. doi: 10.1021/ja0650246
65. Meredith P, Sarna T. The Physical and Chemical Properties of Eumelanin. Pigment Cell Res (2006) 19:572–94. doi: 10.1111/j.1600-0749.2006.00345.x
66. Lillie RD, Donaldson PT, Jirge SK, Pizzolato PC. Primary Acid Silver Nitrate Fixation of Enterochromaffin and Murine Mast Cells. J Histochem Cytochem (1976) 24:1039–41. doi: 10.1177/24.9.965715
67. Van Woert MH. Reduced Nicotinamide-Adenine Dinucleotide Oxidation by Melanin: Inhibition by Phenothiazines. Proc Soc Exp Biol Med (1968) 129:165–71. doi: 10.3181/00379727-129-33275
68. Gan EV, Haberman HF, Menon IA. Electron Transfer Properties of Melanin. Arch Biochem Biophys (1976) 173:666–72. doi: 10.1016/0003-9861(76)90304-0
69. Sarna T, Menon IA, Sealy RC. Photosensitization of Melanins: A Comparative Study. Photochem Photobiol (1985) 42:529–32. doi: 10.1111/j.1751-1097.1985.tb01605.x
70. Sarna T, Korytowski W, Sealy RC. Nitroxides as Redox Probes of Melanins: Dark-Induced and Photoinduced Changes in Redox Equilibria. Arch Biochem Biophys (1985) 239:226–33. doi: 10.1016/0003-9861(85)90830-6
71. Rozanowska M, Bober A, Burke JM, Sarna T. The Role of Retinal Pigment Epithelium Melanin in Photoinduced Oxidation of Ascorbate. Photochem Photobiol (1997) 65:472–9. doi: 10.1111/j.1751-1097.1997.tb08593.x
72. Rozanowska M, Sarna T, Land EJ, Truscott TG. Free Radical Scavenging Properties of Melanin Interaction of Eu- and Pheo-Melanin Models With Reducing and Oxidising Radicals. Free Radic Biol Med (1999) 26:518–25. doi: 10.1016/S0891-5849(98)00234-2
73. Sarna T, Pilas B, Land EJ, Truscott TG. Interaction of Radicals From Water Radiolysis With Melanin. Biochim Biophys Acta (1986) 883:162–7. doi: 10.1016/0304-4165(86)90147-9
74. Pilas B, Sarna T, Kalyanaraman B, Swartz HM. The Effect of Melanin on Iron Associated Decomposition of Hydrogen Peroxide. Free Radic Biol Med (1988) 4:285–93. doi: 10.1016/0891-5849(88)90049-4
75. Korytowski W, Sarna T, Zar ba M. Antioxidant Action of Neuromelanin: The Mechanism of Inhibitory Effect on Lipid Peroxidation. Arch Biochem Biophys (1995) 319:142–8. doi: 10.1006/abbi.1995.1276
76. Zareba M, Bober A, Korytowski W, Zecca L, Sarna T. The Effect of a Synthetic Neuromelanin on Yield of Free Hydroxyl Radicals Generated in Model Systems. Biochim Biophys Acta (1995) 1271:343–8. doi: 10.1016/0925-4439(95)00058-C
77. Zadlo A, Rozanowska MB, Burke JM, Sarna TJ. Photobleaching of Retinal Pigment Epithelium Melanosomes Reduces Their Ability to Inhibit Iron-Induced Peroxidation of Lipids. Pigment Cell Res (2007) 20:52–60. doi: 10.1111/j.1600-0749.2006.00350.x
78. Tanaka H, Yamashita Y, Umezawa K, Hirobe T, Ito S, Wakamatsu K. The Pro-Oxidant Activity of Pheomelanin Is Significantly Enhanced by UVA Irradiation: Benzothiazole Moieties Are More Reactive Than Benzothiazine Moieties. Int J Mol Sci (2018) 19:2889. doi: 10.20944/preprints201809.0091.v1
79. Zadlo A, Szewczyk G, Sarna M, Camenisch TG, Sidabras JW, Ito S, et al. Photobleaching of Pheomelanin Increases Its Phototoxic Potential: Physicochemical Studies of Synthetic Pheomelanin Subjected to Aerobic Photolysis. Pigment Cell Melanoma Res (2019) 32:359–72. doi: 10.1111/pcmr.12752
80. Napolitano A, Panzella L, Monfrecola G, d'Ischia M. Pheomelanin-Induced Oxidative Stress: Bright and Dark Chemistry Bridging Red Hair Phenotype and Melanoma. Pigment Cell Melanoma Res (2014) 27:721–33. doi: 10.1111/pcmr.12262
81. Bruenger FW, Stover BJ, Atherton DR. The Incorporation of Various Metal Ions Into In Vivo- and In Vitro-Produced Melanin. Radiat Res (1967) 32:1–12. doi: 10.2307/3572300
82. Samokhvalov A, Hong L, Liu Y, Garguilo J, Nemanich RJ, Edwards GS, et al. Oxidation Potentials of Human Eumelanosomes and Pheomelanosomes. Photochem Photobiol (2005) 81:145–8. doi: 10.1562/2004-07-23-RC-245.1
83. Sarna T, Lukiewicz S. Electron Spin Resonance Studies on Living Cells. IV. Pathological Changes in Amphibian Eggs and Embryos. Folia Histochem Cytochem (Krakow) (1972) 10:265–78.
84. Sarna T, Swartz HM, Zadlo A. Interaction of Melanin With Metal Ions Modulates Their Cytotoxic Potential. Appl Magnetic Resonance (2021) 53:105–21. doi: 10.1007/s00723-021-01386-3
85. Sarna T, Froncisz W, Hyde JS. Cu2+ Probe of Metal-Ion Binding Sites in Melanin Using Electron Paramagentic Resonance Spectroscopy. II. Natural Melanin. Arch Biochem Biophys (1980) 202:304–13. doi: 10.1016/0003-9861(80)90431-2
86. Froncisz W, Sarna T, Hyde JS. Cu2+ Probe of Metal-Ion Binding Sites in Melanin Using Electron Paramagnetic Resonance Spectroscopy. I. Synthetic Melanins. Arch Biochem Biophys (1980) 202:289–303. doi: 10.1016/0003-9861(80)90430-0
87. Zadlo A, Mokrzynski K, Ito S, Wakamatsu K, Sarna T. The Influence of Iron on Selected Properties of Synthetic Pheomelanin. Cell Biochem Biophys (2020) 78:181–9. doi: 10.1007/s12013-020-00918-1
88. Zareba M, Szewczyk G, Sarna T, Hong L, Simon JD, Henry MM, et al. Effects of Photodegradation on the Physical and Antioxidant Properties of Melanosomes Isolated From Retinal Pigment Epithelium. Photochem Photobiol (2006) 82:1024–9. doi: 10.1562/2006-03-08-RA-836
89. Zadlo A, Burke JM, Sarna T. Effect of Untreated and Photobleached Bovine RPE Melanosomes on the Photoinduced Peroxidation of Lipids. Photochem Photobiol Sci (2009) 8:830–7. doi: 10.1039/b901820d
90. Zareba M, Sarna T, Szewczyk G, Burke JM. Photobleaching of Melanosomes From Retinal Pigment Epithelium: II. Effects on the Response of Living Cells to Photic Stress. Photochem Photobiol (2007) 83:925–30. doi: 10.1111/j.1751-1097.2007.00080.x
91. Burke JM, Henry MM, Zareba M, Sarna T. Photobleaching of Melanosomes From Retinal Pigment Epithelium: I. Effects on Protein Oxidation. Photochem Photobiol (2007) 83:920–4. doi: 10.1111/j.1751-1097.2007.00081.x
92. Slominski A, Wortsman J. Neuroendocrinology of the Skin. Endocr Rev (2000) 21:457–87. doi: 10.1210/er.21.5.457
93. Slominski A, Paus R, Schadendorf D. Melanocytes as "Sensory" and Regulatory Cells in the Epidermis. J Theor Biol (1993) 164:103–20. doi: 10.1006/jtbi.1993.1142
94. Slominski A, Paus R. Are L-Tyrosine and L-Dopa Hormone-Like Bioregulators? J Theor Biol (1990) 143:123–38. doi: 10.1016/S0022-5193(05)80292-9
95. Donoghue PC, Graham A, Kelsh RN. The Origin and Evolution of the Neural Crest. Bioessays (2008) 30:530–41. doi: 10.1002/bies.20767
96. Nishimura EK, Jordan SA, Oshima H, Yoshida H, Osawa M, Moriyama M, et al. Dominant Role of the Niche in Melanocyte Stem-Cell Fate Determination. Nature (2002) 416:854–60. doi: 10.1038/416854a
97. Schalenbourg A, Zografos L. Retinal Pigment Epithelial Tumors. In: Singh A, Seregard S, editors. Ocular Tumors. Karger, Basel (2016). p. 60–72.
98. Sreenivasan J, Rishi P, Das K, Krishnakumar S, Biswas J. Retinal Pigment Epithelium Adenoma and Adenocarcinoma: A Review. Ocul Oncol Pathol (2021) 7:121–32. doi: 10.1159/000509484
99. Kaprinis K, Bobat H, De Salvo G. MultiColor(TM) Imaging in Combined Hamartoma of the Retina and Retinal Pigment Epithelium. Eye (Lond) (2018) 32:1478–82. doi: 10.1038/s41433-018-0123-2
100. Johnsen JI, Dyberg C, Wickstrom M. Neuroblastoma-A Neural Crest Derived Embryonal Malignancy. Front Mol Neurosci (2019) 12:9. doi: 10.3389/fnmol.2019.00009
101. Bennett D, Lyulcheva E, Cobbe N. Drosophila as a Potential Model for Ocular Tumors. Ocul Oncol Pathol (2015) 1:190–9. doi: 10.1159/000370155
102. Pajak S, Cieszka K, Plonka P, Lukiewicz S, Mihm M, Slominski A. Transplantable Melanomas in Gerbils (Meriones Unguiculatus). I. Origin, Morphology and Growth Rate. Anticancer Res (1996) 16:1203–8.
103. Bomirski A, Slominski A, Bigda J. The Natural History of a Family of Transplantable Melanomas in Hamsters. Cancer Metastasis Rev (1988) 7:95–118. doi: 10.1007/BF00046481
104. Slominski A, Paus R. Bomirski Melanomas - A Versatile and Powerful Model for Pigment Cell and Melanoma Research (Review). Int J Oncol (1993) 2:221–8. doi: 10.3892/ijo.2.2.221
105. Plonka PM, Slominski AT, Pajak S, Urbanska K. Transplantable Melanomas in Gerbils (Meriones Unguiculatus). II: Melanogenesis. Exp Dermatol (2003) 12:356–64. doi: 10.1034/j.1600-0625.2002.120401.x
106. Bomirski A, Wrzolkowa T, Arendarczyk M, Bomirska M, Kuklinska E, Slominski A, et al. Pathology and Ultrastructural Characteristics of a Hypomelanotic Variant of Transplantable Hamster Melanoma With Elevated Tyrosinase Activity. J Invest Dermatol (1987) 89:469–73. doi: 10.1111/1523-1747.ep12460928
107. Lazova R, Pawelek JM. Why do Melanomas Get So Dark? Exp Dermatol (2009) 18:934–8. doi: 10.1111/j.1600-0625.2009.00933.x
108. Aspengren S, Hedberg D, Wallin M. Studies of Pigment Transfer Between Xenopus Laevis Melanophores and Fibroblasts In Vitro and In Vivo. Pigment Cell Res (2006) 19:136–45. doi: 10.1111/j.1600-0749.2005.00290.x
109. Riley PA. Materia Melanica: Further Dark Thoughts. Pigment Cell Res (1992) 5:101–6. doi: 10.1111/j.1600-0749.1992.tb00005.x
110. Krzywda A, Petelenz E, Michalczyk D, Plonka PM. Sclerotia of the Acellular (True) Slime Mould Fuligo Septica as a Model to Study Melanization and Anabiosis. Cell Mol Biol Lett (2008) 13:130–43. doi: 10.2478/s11658-007-0047-5
111. Biebricher CK, Eigen M. The Error Threshold. Virus Res (2005) 107:117–27. doi: 10.1016/j.virusres.2004.11.002
112. Eigen M. Selforganization of Matter and the Evolution of Biological Macromolecules. Naturwissenschaften (1971) 58:465–523. doi: 10.1007/BF00623322
113. Grabacka M, Plonka P. Mitochondrial Sirtuins at the Crossroads of Energy Metabolism and Oncogenic Transformation. In: Maiese K, editor. Sirtuin Biology in Cancer and Metabolic Disease Cellular Pathways for Clinical Discovery. London – San Diego-Cambridge-Oxford: Academic Press Elsevier (2021). doi: 10.1016/B978-0-12-822467-0.00001-2
114. Davis EJ, Johnson DB, Sosman JA, Chandra S. Melanoma: What Do All the Mutations Mean? Cancer (2018) 124:3490–9. doi: 10.1002/cncr.31345
115. Sini MC, Doneddu V, Paliogiannis P, Casula M, Colombino M, Manca A, et al. Genetic Alterations in Main Candidate Genes During Melanoma Progression. Oncotarget (2018) 9:8531–41. doi: 10.18632/oncotarget.23989
116. Uribe P, Wistuba I, Gonzalez S. BRAF Mutation: A Frequent Event in Benign, Atypical, and Malignant Melanocytic Lesions of the Skin. Am J Dermatopathol (2003) 25:365–70. doi: 10.1097/00000372-200310000-00001
117. Moan J, Dahlback A, Setlow RB. Epidemiological Support for an Hypothesis for Melanoma Induction Indicating a Role for UVA Radiation. Photochem Photobiol (1999) 70:243–7. doi: 10.1111/j.1751-1097.1999.tb07995.x
118. Ortonne J-P, Ballotti R. Melanocyte Biology and Melanogenesis: What's New? J Dermatol Treat (2000) 11(Suppl 1):S15–26. doi: 10.1080/09546630050517621
119. Noonan FP, Zaidi MR, Wolnicka-Glubisz A, Anver MR, Bahn J, Wielgus A, et al. Melanoma Induction by Ultraviolet A But Not Ultraviolet B Radiation Requires Melanin Pigment. Nat Commun (2012) 3:884. doi: 10.1038/ncomms1893
120. Mitra D, Luo X, Morgan A, Wang J, Hoang MP, Lo J, et al. An Ultraviolet-Radiation-Independent Pathway to Melanoma Carcinogenesis in the Red Hair/Fair Skin Background. Nature (2012) 491:449–53. doi: 10.1038/nature11624
121. Lembo S, Di Caprio R, Micillo R, Balato A, Monfrecola G, Panzella L, et al. Light-Independent Pro-Inflammatory and Pro-Oxidant Effects of Purified Human Hair Melanins on Keratinocyte Cell Cultures. Exp Dermatol (2017) 26:592–4. doi: 10.1111/exd.13122
122. Plonka PM, Picardo M, Slominski AT. Does Melanin Matter in the Dark? Exp Dermatol (2017) 26:595–7. doi: 10.1111/exd.13171
123. Lukiewicz S. The Biological Role of Melanin. I. New Concepts and Methodical Approaches. Folia Histochem Cytochem (Krakow) (1972) 10:93–108.
124. Mileo AM, Mattei E, Fanuele M, Delpino A, Ferrini U. Differential Radiosensitivity in Cultured B-16 Melanoma Cells Following Interrupted Melanogenesis Induced by Glucosamine. Pigment Cell Res (1989) 2:167–70. doi: 10.1111/j.1600-0749.1989.tb00182.x
125. Zygulska-Mach H, Maciejewski Z, Lukiewicz S, Iwasiow B, Link E. Clinical Trials on Chemical Radiosensitization of Malignant Melanoma of the Choroid. Ophthalmologica (1979) 178:194–7. doi: 10.1159/000308823
126. Lukiewicz S. Electron Spin Resonance Studies on the Detectability of Radiation Damage and Radiosensitization of Neoplastic Cells. Coordinated Programme on Improvement of Radiotherapy of Cancer Using Modifiers of Radiosensitivity of Cells. Julich, Federal Republic of Germany: International Atomic Energy Agency (IAEA (1981) p. 1–34.
127. Kinnaert E, Morandini R, Simon S, Hill HZ, Ghanem G, Van Houtte P. The Degree of Pigmentation Modulates the Radiosensitivity of Human Melanoma Cells. Radiat Res (2000) 154:497–502. doi: 10.1667/0033-7587(2000)154[0497:TDOPMT]2.0.CO;2
128. Bomirski A, Dominiczak T, Nowinska L. Spontaneous Transplantable Melanoma in the Golden Hamster (Mesocricetus Auratus). Acta Unio Int Contra Cancrum (1962) 18:178–80.
129. Bomirski A, Zawrocka-Wrzolkowa T, Pautsch F. Ultrastructure of Transplantable Melanotic and Amelanotic Melanoma of Hamsters. Acta Med Pol (1971) 12:101–5.
130. Cichorek M, Kozlowska K, Wachulska M, Zielinska K. Spontaneous Apoptosis of Melanotic and Amelanotic Melanoma Cells in Different Phases of Cell Cycle: Relation to Tumor Growth. Folia Histochem Cytobiol (2006) 44:31–6. doi: 10.5603/4585
131. Kozlowska K, Zarzeczna M, Cichorek M. Sensitivity of Transplantable Melanoma Cells to Cytokines With Regard to Their Spontaneous Apoptosis. Pathobiology (2001) 69:249–57. doi: 10.1159/000064335
132. Kozlowska K, Cichorek M, Zarzeczna M, Brozek J, Witkowski JM. Heterogeneous Susceptibility to Spontaneous and Induced Apoptosis Characterizes Two Related Transplantable Melanomas With Different Biological Properties. Pigment Cell Res (2002) 15:233–8. doi: 10.1034/j.1600-0749.2002.02020.x
133. Scislowski PW, Slominski A, Bomirski A. Biochemical Characterization of Three Hamster Melanoma Variants–II. Glycolysis and Oxygen Consumption. Int J Biochem (1984) 16:327–31. doi: 10.1016/0020-711X(84)90107-1
134. Slominski A, Scislowski PW, Bomirski A. Biochemical Characterization of Three Hamster Melanoma Variants–I. Tyrosinase Activity and Melanin Content. Int J Biochem (1984) 16:323–6. doi: 10.1016/0020-711X(84)90106-X
135. Cichorek M, Zarzeczna M, Kozlowska K. Tumoricidal Effect of Macrophages on Transplantable Melanoma Cells With Regard to Their Sensitivity to Exogenous Cytokines. Folia Histochem Cytobiol (2002) 40:191–2.
136. Cichorek M, Kozlowska K, Bryl E. The Activity of Caspases in Spontaneous and Camptothecin-Induced Death of Melanotic and Amelanotic Melanoma Cell. Cancer Biol Ther (2007) 6:346–53. doi: 10.4161/cbt.6.3.3701
137. Cichorek M. Camptothecin-Induced Death of Amelanotic and Melanotic Melanoma Cells in Different Phases of Cell Cycle. Neoplasma (2011) 58:227–34. doi: 10.4149/neo_2011_03_227
138. Zielinska K, Kozlowska K, Cichorek M, Wachulska M. Fas and FasL Expression on Cells of Two Transplantable Melanoma Lines According to Their Different Biological Properties. Folia Histochem Cytobiol (2008) 46:337–43. doi: 10.2478/v10042-008-0041-4
139. Skoniecka A, Cichorek M, Tyminska A, Pelikant-Malecka I, Dziewiatkowski J. Melanization as Unfavorable Factor in Amelanotic Melanoma Cell Biology. Protoplasma (2021) 258:935–48. doi: 10.1007/s00709-021-01613-5
140. Slominski A. Rapid Melanization of Bomirski Amelanotic Melanoma Cells in Cell Culture. Biosci Rep (1983) 3:189–94. doi: 10.1007/BF01121951
141. Slominski A. Some Properties of Bomirski Ab Amelanotic Melanoma Cells, Which Underwent Spontaneous Melanization in Primary Cell Culture. Growth Kinetics, Cell Morphology, Melanin Content and Tumorigenicity. J Cancer Res Clin Oncol (1985) 109:29–37. doi: 10.1007/BF01884251
142. Li W, Slominski R, Slominski AT. High-Resolution Magic Angle Spinning Nuclear Magnetic Resonance Analysis of Metabolic Changes in Melanoma Cells After Induction of Melanogenesis. Anal Biochem (2009) 386:282–4. doi: 10.1016/j.ab.2008.12.017
143. Slominski A, Zbytek B, Slominski R. Inhibitors of Melanogenesis Increase Toxicity of Cyclophosphamide and Lymphocytes Against Melanoma Cells. Int J Cancer (2009) 124:1470–7. doi: 10.1002/ijc.24005
144. Brozyna AA, VanMiddlesworth L, Slominski AT. Inhibition of Melanogenesis as a Radiation Sensitizer for Melanoma Therapy. Int J Cancer (2008) 123:1448–56. doi: 10.1002/ijc.23664
145. Janjetovic Z, Brozyna AA, Tuckey RC, Kim TK, Nguyen MN, Jozwicki W, et al. High Basal NF-kappaB Activity in Nonpigmented Melanoma Cells Is Associated With an Enhanced Sensitivity to Vitamin D3 Derivatives. Br J Cancer (2011) 105:1874–84. doi: 10.1038/bjc.2011.458
146. Pawlikowska M, Piotrowski J, Jedrzejewski T, Kozak W, Slominski AT, Brozyna AA. Coriolus Versicolor-Derived Protein-Bound Polysaccharides Trigger the Caspase-Independent Cell Death Pathway in Amelanotic But Not Melanotic Melanoma Cells. Phytother Res (2020) 34:173–83. doi: 10.1002/ptr.6513
147. Pawlikowska M, Jedrzejewski T, Brozyna AA, Wrotek S. Protein-Bound Polysaccharides From Coriolus Versicolor Induce RIPK1/RIPK3/MLKL-Mediated Necroptosis in ER-Positive Breast Cancer and Amelanotic Melanoma Cells. Cell Physiol Biochem (2020) 54:591–604. doi: 10.33594/000000242
148. Pawlikowska M, Jedrzejewski T, Slominski AT, Brozyna AA, Wrotek S. Pigmentation Levels Affect Melanoma Responses to Coriolus Versicolor Extract and Play a Crucial Role in Melanoma-Mononuclear Cell Crosstalk. Int J Mol Sci (2021) 22:5735. doi: 10.3390/ijms22115735
149. Wasiewicz T, Piotrowska A, Wierzbicka J, Slominski AT, Zmijewski MA. Antiproliferative Activity of Non-Calcemic Vitamin D Analogs on Human Melanoma Lines in Relation to VDR and PDIA3 Receptors. Int J Mol Sci (2018) 19:2583. doi: 10.3390/ijms19092583
150. Doglioni C, Pignatti J, Coleman M. Why Did Life Develop on the Surface of the Earth in the Cambrian? Geosci Front (2016) 7:865–73. doi: 10.1016/j.gsf.2016.02.001
151. Oxenberg J, Kane JM. 3rd, The Role of Radiation Therapy in Melanoma. Surg Clin North Am (2014) 94:1031–47, viii. doi: 10.1016/j.suc.2014.07.006
152. Sniegocka M, Podgorska E, Plonka PM, Elas M, Romanowska-Dixon B, Szczygiel M, et al. Transplantable Melanomas in Hamsters and Gerbils as Models for Human Melanoma. Sensitization in Melanoma Radiotherapy-From Animal Models to Clinical Trials. Int J Mol Sci (2018) 19:1048. doi: 10.3390/ijms19041048
153. Kedracka-Krok S, Jankowska U, Elas M, Sowa U, Swakon J, Cierniak A, et al. Proteomic Analysis of Proton Beam Irradiated Human Melanoma Cells. PloS One (2014) 9:e84621. doi: 10.1371/journal.pone.0084621
154. Podgorska E, Drzal A, Matuszak Z, Swakon J, Slominski A, Elas M, et al. Calcitriol and Calcidiol Can Sensitize Melanoma Cells to Low(-)LET Proton Beam Irradiation. Int J Mol Sci (2018) 19:2236. doi: 10.3390/ijms19082236
155. Cascinelli N, Heerlyn M, Schneeberger A, Kuwert C, Slominski A, Armstrong C, et al. What Is the Most Promising Strategy for the Treatment of Metastasizing Melanoma? Exp Dermatol (2000) 9:439–51. doi: 10.1034/j.1600-0625.2000.009006439.x
156. Vasileiou T, Summerer L. A Biomimetic Approach to Shielding From Ionizing Radiation: The Case of Melanized Fungi. PloS One (2020) 15:e0229921. doi: 10.1371/journal.pone.0229921
157. Pinkert S, Zeuss D. Thermal Biology: Melanin-Based Energy Harvesting Across the Tree of Life. Curr Biol (2018) 28:R887–9. doi: 10.1016/j.cub.2018.07.026
158. Dadachova E, Bryan RA, Huang X, Moadel T, Schweitzer AD, Aisen P, et al. Ionizing Radiation Changes the Electronic Properties of Melanin and Enhances the Growth of Melanized Fungi. PloS One (2007) 2:e457. doi: 10.1371/journal.pone.0000457
159. Nick P. Black Is Beautiful (and Protective): Melanin Synthesis in Animals and Plants. Protoplasma (2021) 258:923–4. doi: 10.1007/s00709-021-01693-3
160. Coutinho JW, Rodrigues AC, Appezzato-da-Gloria B, Oliveira EM, Oliveira FMC, Lusa MG. Plastid Role in Phytomelanin Synthesis in Piptocarpha Axillaris (Less.) Baker Stems (Asteraceae, Vernonieae). Protoplasma (2021) 258:963–77. doi: 10.1007/s00709-021-01615-3
161. Slominski A, Paus R, Mihm MC. Inhibition of Melanogenesis as an Adjuvant Strategy in the Treatment of Melanotic Melanomas: Selective Review and Hypothesis. Anticancer Res (1998) 18:3709–15.
162. Slominski A, Goodman-Snitkoff GG. Dopa Inhibits Induced Proliferative Activity of Murine and Human Lymphocytes. Anticancer Res (1992) 12:753–6.
163. Bergquist J, Tarkowski A, Ekman R, Ewing A. Discovery of Endogenous Catecholamines in Lymphocytes and Evidence for Catecholamine Regulation of Lymphocyte Function via an Autocrine Loop. Proc Natl Acad Sci USA (1994) 91:12912–6. doi: 10.1073/pnas.91.26.12912
164. Josefsson E, Bergquist J, Ekman R, Tarkowski A. Catecholamines Are Synthesized by Mouse Lymphocytes and Regulate Function of These Cells by Induction of Apoptosis. Immunology (1996) 88:140–6. doi: 10.1046/j.1365-2567.1996.d01-653.x
165. Ghosh MC, Mondal AC, Basu S, Banerjee S, Majumder J, Bhattacharya D, et al. Dopamine Inhibits Cytokine Release and Expression of Tyrosine Kinases, Lck and Fyn in Activated T Cells. Int Immunopharmacol (2003) 3:1019–26. doi: 10.1016/S1567-5769(03)00100-0
166. Cook-Mills JM, Cohen RL, Perlman RL, Chambers DA. Inhibition of Lymphocyte Activation by Catecholamines: Evidence for a Non-Classical Mechanism of Catecholamine Action. Immunology (1995) 85:544–9.
167. Offen D, Ziv I, Gorodin S, Barzilai A, Malik Z, Melamed E. Dopamine-Induced Programmed Cell Death in Mouse Thymocytes. Biochim Biophys Acta (1995) 1268:171–7. doi: 10.1016/0167-4889(95)00075-4
168. Bergquist J, Tarkowski A, Ewing A, Ekman R. Catecholaminergic Suppression of Immunocompetent Cells. Immunol Today (1998) 19:562–7. doi: 10.1016/S0167-5699(98)01367-X
169. Cioca DP, Watanabe N, Isobe M. Apoptosis of Peripheral Blood Lymphocytes Is Induced by Catecholamines. Jpn Heart J (2000) 41:385–98. doi: 10.1536/jhj.41.385
170. Slominski A, Pruski D. L-Dopa Binding Sites in Rodent Melanoma Cells. Biochim Biophys Acta (1992) 1139:324–8. doi: 10.1016/0925-4439(92)90109-Z
171. Slominski A, Friedrich T. L-Dopa Inhibits In Vitro Phosphorylation of Melanoma Glycoproteins. Pigment Cell Res (1992) 5:396–9. doi: 10.1111/j.1600-0749.1992.tb00569.x
172. Huffnagle GB, Chen GH, Curtis JL, McDonald RA, Strieter RM, Toews GB. Down-Regulation of the Afferent Phase of T Cell-Mediated Pulmonary Inflammation and Immunity by a High Melanin-Producing Strain of Cryptococcus Neoformans. J Immunol (1995) 155:3507–16.
173. Liu D, Wei L, Guo T, Tan W. Detection of DOPA-Melanin in the Dimorphic Fungal Pathogen Penicillium Marneffei and Its Effect on Macrophage Phagocytosis In Vitro. PloS One (2014) 9:e92610. doi: 10.1371/journal.pone.0092610
174. Mehrotra S, Mougiakakos D, Johansson CC, Voelkel-Johnson C, Kiessling R. Oxidative Stress and Lymphocyte Persistence: Implications in Immunotherapy. Adv Cancer Res (2009) 102:197–227. doi: 10.1016/S0065-230X(09)02006-5
175. Ochsenreither S, Fusi A, Busse A, Letsch A, Haase D, Thiel E, et al. Long Term Presence of a Single Predominant Tyrosinase-Specific T-Cell Clone Associated With Disease Control in a Patient With Metastatic Melanoma. Int J Cancer (2010) 126:2497–502. doi: 10.1002/ijc.24939
176. Michaeli Y, Denkberg G, Sinik K, Lantzy L, Chih-Sheng C, Beauverd C, et al. Expression Hierarchy of T Cell Epitopes From Melanoma Differentiation Antigens: Unexpected High Level Presentation of Tyrosinase-HLA-A2 Complexes Revealed by Peptide-Specific, MHC-Restricted, TCR-Like Antibodies. J Immunol (2009) 182:6328–41. doi: 10.4049/jimmunol.0801898
177. Liu Y, Peng Y, Mi M, Guevara-Patino J, Munn DH, Fu N, et al. Lentivector Immunization Stimulates Potent CD8 T Cell Responses Against Melanoma Self-Antigen Tyrosinase-Related Protein 1 and Generates Antitumor Immunity in Mice. J Immunol (2009) 182:5960–9. doi: 10.4049/jimmunol.0900008
178. Reynolds SR, Celis E, Sette A, Oratz R, Shapiro RL, Johnston D, et al. HLA-Independent Heterogeneity of CD8+ T Cell Responses to MAGE-3, Melan-A/MART-1, Gp100, Tyrosinase, MC1R, and TRP-2 in Vaccine-Treated Melanoma Patients. J Immunol (1998) 161:6970–6. doi: 10.1016/S0923-1811(98)83940-6
179. Parkhurst MR, Fitzgerald EB, Southwood S, Sette A, Rosenberg SA, Kawakami Y. Identification of a Shared HLA-A*0201-Restricted T-Cell Epitope From the Melanoma Antigen Tyrosinase-Related Protein 2 (TRP2). Cancer Res (1998) 58:4895–901. doi: 10.1002/1097-0215(20000801)87:3<399::AID-IJC14>3.0.CO;2-9
180. Di Nicola M, Carlo-Stella C, Mortarini R, Baldassari P, Guidetti A, Gallino GF, et al. Boosting T Cell-Mediated Immunity to Tyrosinase by Vaccinia Virus-Transduced, CD34(+)-Derived Dendritic Cell Vaccination: A Phase I Trial in Metastatic Melanoma. Clin Cancer Res (2004) 10:5381–90. doi: 10.1158/1078-0432.CCR-04-0602
181. Smith C, Cerundolo V. Immunotherapy of Melanoma. Immunology (2001) 104:1–7. doi: 10.1046/j.1365-2567.2001.01297.x
182. Slominski A, Wortsman J, Carlson AJ, Matsuoka LY, Balch CM, Mihm MC. Malignant Melanoma. Arch Pathol Lab Med (2001) 125:1295–306. doi: 10.5858/2001-125-1295-MM
183. Liao JC, Gregor P, Wolchok JD, Orlandi F, Craft D, Leung C, et al. Vaccination With Human Tyrosinase DNA Induces Antibody Responses in Dogs With Advanced Melanoma. Cancer Immun (2006) 6:8.
184. Marconcini R, Spagnolo F, Stucci LS, Ribero S, Marra E, Rosa F, et al. Current Status and Perspectives in Immunotherapy for Metastatic Melanoma. Oncotarget (2018) 9:12452–70. doi: 10.18632/oncotarget.23746
185. Perez-Guijarro E, Yang HH, Araya RE, El Meskini R, Michael HT, Vodnala SK, et al. Multimodel Preclinical Platform Predicts Clinical Response of Melanoma to Immunotherapy. Nat Med (2020) 26:781–91. doi: 10.1038/s41591-020-0818-3
186. Davis L, Tarduno A, Lu Y-C. Neoantigen-Reactive T Cells: The Driving Force Behind Successful Melanoma Immunotherapy. Cancers (2021) 13:6061. doi: 10.3390/cancers13236061
187. Eroglu Z, Zaretsky JM, Hu-Lieskovan S, Kim DW, Algazi A, Johnson DB, et al. High Response Rate to PD-1 Blockade in Desmoplastic Melanomas. Nature (2018) 553:347–50. doi: 10.1038/nature25187
188. Slominski AT, Paus R. Inhibition of Melanogenesis for Melanoma Therapy? J Invest Dermatol (1994) 103:742. doi: 10.1111/1523-1747.ep12398639
189. Wakamatsu K, Fukushima S, Minagawa A, Omodaka T, Hida T, Hatta N, et al. Significance of 5-S-Cysteinyldopa as a Marker for Melanoma. Int J Mol Sci (2020) 21:432. doi: 10.3390/ijms21020432
190. Sonesson B, Eide S, Ringborg U, Rorsman H, Rosengren E. Tyrosinase Activity in the Serum of Patients With Malignant Melanoma. Melanoma Res (1995) 5:113–6. doi: 10.1097/00008390-199504000-00007
191. Miranda M, Ligas C, Amicarelli F, D'Alessandro E, Brisdelli F, Zarivi O, et al. Sister Chromatid Exchange (SCE) Rates in Human Melanoma Cells as an Index of Mutagenesis. Mutagenesis (1997) 12:233–6. doi: 10.1093/mutage/12.4.233
192. Miranda M, Botti D, Di Cola M. Possible Genotoxicity of Melanin Synthesis Intermediates: Tyrosinase Reaction Products Interact With DNA In Vitro. Mol Gen Genet (1984) 193:395–9. doi: 10.1007/BF00382074
193. Graham DG, Tiffany SM, Vogel FS. The Toxicity of Melanin Precursors. J Invest Dermatol (1978) 70:113–6. doi: 1523-1747.ep12541249
194. Wick MM. Levodopa/dopamine Analogs as Inhibitors of DNA Synthesis in Human Melanoma Cells. J Invest Dermatol (1989) 92:329S–31S. doi: 10.1111/1523-1747.ep13076753
195. Wick MM, Fitzgerald G. Inhibition of Reverse Transcriptase by Tyrosinase Generated Quinones Related to Levodopa and Dopamine. Chem Biol Interact (1981) 38:99–107. doi: 10.1016/0009-2797(81)90156-3
196. Brash DE. UV-Induced Melanin Chemiexcitation: A New Mode of Melanoma Pathogenesis. Toxicol Pathol (2016) 44:552–4. doi: 10.1177/0192623316632072
197. Premi S, Wallisch S, Mano CM, Weiner AB, Bacchiocchi A, Wakamatsu K, et al. Photochemistry. Chemiexcitation of Melanin Derivatives Induces DNA Photoproducts Long After UV Exposure. Science (2015) 347:842–7. doi: 10.1126/science.1256022
198. Warburg O. On the Origin of Cancer Cells. Science (1956) 123:309–14. doi: 10.1126/science.123.3191.309
199. Kroemer G, Pouyssegur J. Tumor Cell Metabolism: Cancer's Achilles' Heel. Cancer Cell (2008) 13:472–82. doi: 10.1016/j.ccr.2008.05.005
200. Semenza GL. Evaluation of HIF-1 Inhibitors as Anticancer Agents. Drug Discovery Today (2007) 12:853–9. doi: 10.1016/j.drudis.2007.08.006
201. Hanahan D, Robert A. Weinberg, Hallmarks of Cancer: The Next Generation. Cell (2011) 144:646–74. doi: 10.1016/j.cell.2011.02.013
202. Bailey KM, Wojtkowiak JW, Hashim AI, Gillies RJ. Chapter Four - Targeting the Metabolic Microenvironment of Tumors. In: Smalley KSM, editor. Advances in Pharmacology. Academic Press (2012). p. 63–107.
203. Dang TN-H, Singla KA, Mackay ME, Jirik RF, Weljie MA. Targeted Cancer Therapeutics: Biosynthetic and Energetic Pathways Characterized by Metabolomics and the Interplay With Key Cancer Regulatory Factors. Curr Pharm Design (2014) 20:2637–47. doi: 10.2174/13816128113199990489
204. Sarna T, Duleba A, Korytowski W, Swartz H. Interaction of Melanin With Oxygen. Arch Biochem Biophys (1980) 200:140–8. doi: 10.1016/0003-9861(80)90340-9
205. Sarna T, Sealy RC. Photoinduced Oxygen Consumption in Melanin Systems. Action Spectra and Quantum Yields for Eumelanin and Synthetic Melanin. Photochem Photobiol (1984) 39:69–74. doi: 10.1111/j.1751-1097.1984.tb03406.x
206. Scislowski PW, Slominski A, Bomirski A, Zydowo M. Metabolic Characterization of Three Hamster Melanoma Variants. Neoplasma (1985) 32:593–8.
207. Slominski A, Kim TK, Brozyna AA, Janjetovic Z, Brooks DL, Schwab LP, et al. The Role of Melanogenesis in Regulation of Melanoma Behavior: Melanogenesis Leads to Stimulation of HIF-1alpha Expression and HIF-Dependent Attendant Pathways. Arch Biochem Biophys (2014) 563:79–93. doi: 10.1016/j.abb.2014.06.030
208. Slominski A, Moellmann G, Kuklinska E. L-Tyrosine, L-Dopa, and Tyrosinase as Positive Regulators of the Subcellular Apparatus of Melanogenesis in Bomirski Ab Amelanotic Melanoma Cells. Pigment Cell Res (1989) 2:109–16. doi: 10.1111/j.1600-0749.1989.tb00170.x
209. Slominski A, Moellmann G, Kuklinska E, Bomirski A, Pawelek J. Positive Regulation of Melanin Pigmentation by Two Key Substrates of the Melanogenic Pathway, L-Tyrosine and L-Dopa. J Cell Sci (1988) 89(Pt 3):287–96. doi: 10.1242/jcs.89.3.287
210. Pawelek J, Bolognia J, McLane J, Murray M, Osber M, Slominski A. A Possible Role for Melanin Precursors in Regulating Both Pigmentation and Proliferation of Melanocytes. Prog Clin Biol Res (1988) 256:143–54.
211. Slominski A, Jastreboff P, Pawelek J. L-Tyrosine Stimulates Induction of Tyrosinase Activity by MSH and Reduces Cooperative Interactions Between MSH Receptors in Hamster Melanoma Cells. Biosci Rep (1989) 9:579–86. doi: 10.1007/BF01119801
212. McLane J, Osber M, Pawelek JM. Phosphorylated Isomers of L-Dopa Stimulate MSH Binding Capacity and Responsiveness to MSH in Cultured Melanoma Cells. Biochem Biophys Res Commun (1987) 145:719–25. doi: 10.1016/0006-291X(87)91024-2
213. Slominski A. POMC Gene Expression in Mouse and Hamster Melanoma Cells. FEBS Lett (1991) 291:165–8. doi: 10.1016/0014-5793(91)81274-C
214. Bohm M, Luger TA, Tobin DJ, Garcia-Borron JC. Melanocortin Receptor Ligands: New Horizons for Skin Biology and Clinical Dermatology. J Invest Dermatol (2006) 126:1966–75. doi: 10.1038/sj.jid.5700421
215. Slominski A, Wortsman J, Luger T, Paus R, Solomon S. Corticotropin Releasing Hormone and Proopiomelanocortin Involvement in the Cutaneous Response to Stress. Physiol Rev (2000) 80:979–1020. doi: 10.1152/physrev.2000.80.3.979
216. Hedley SJ, Murray A, Sisley K, Ghanem G, Morandini R, Gawkrodger DJ, et al. Alpha-Melanocyte Stimulating Hormone can Reduce T-Cell Interaction With Melanoma Cells In Vitro. Melanoma Res (2000) 10:323–30. doi: 10.1097/00008390-200008000-00003
217. Slominski A, Wortsman J, Mazurkiewicz JE, Matsuoka L, Dietrich J, Lawrence K, et al. Detection of Proopiomelanocortin-Derived Antigens in Normal and Pathologic Human Skin. J Lab Clin Med (1993) 122:658–66. doi: 10.5555/uri:pii:002221439390247V
218. Nagahama M, Funasaka Y, Fernandez-Frez ML, Ohashi A, Chakraborty AK, Ueda M, et al. Immunoreactivity of Alpha-Melanocyte-Stimulating Hormone, Adrenocorticotrophic Hormone and Beta-Endorphin in Cutaneous Malignant Melanoma and Benign Melanocytic Naevi. Br J Dermatol (1998) 138:981–5. doi: 10.1046/j.1365-2133.1998.02263.x
219. Ghanem G, Lienard D, Hanson P, Lejeune F, Fruhling J. Increased Serum Alpha-Melanocyte Stimulating Hormone (Alpha-MSH) in Human Malignant Melanoma. Eur J Cancer Clin Oncol (1986) 22:535–6. doi: 10.1016/0277-5379(86)90125-2
220. Loir B, Bouchard B, Morandini R, Del Marmol V, Deraemaecker R, Garcia-Borron JC, et al. Immunoreactive Alpha-Melanotropin as an Autocrine Effector in Human Melanoma Cells. Eur J Biochem (1997) 244:923–30. doi: 10.1111/j.1432-1033.1997.00923.x
221. Funasaka Y, Sato H, Chakraborty AK, Ohashi A, Chrousos GP, Ichihashi M. Expression of Proopiomelanocortin, Corticotropin-Releasing Hormone (CRH), and CRH Receptor in Melanoma Cells, Nevus Cells, and Normal Human Melanocytes. J Investig Dermatol Symp Proc (1999) 4:105–9. doi: 10.1038/sj.jidsp.5640192
222. Sato H, Nagashima Y, Chrousos GP, Ichihashi M, Funasak Y. The Expression of Corticotropin-Releasing Hormone in Melanoma. Pigment Cell Res (2002) 15:98–103. doi: 10.1034/j.1600-0749.2002.1o063.x
223. Sarna M, Zadlo A, Hermanowicz P, Madeja Z, Burda K, Sarna T. Cell Elasticity Is an Important Indicator of the Metastatic Phenotype of Melanoma Cells. Exp Dermatol (2014) 23:813–8. doi: 10.1111/exd.12535
224. Sarna M, Krzykawska-Serda M, Jakubowska M, Zadlo A, Urbanska K. Melanin Presence Inhibits Melanoma Cell Spread in Mice in a Unique Mechanical Fashion. Sci Rep (2019) 9:9280. doi: 10.1038/s41598-019-45643-9
225. Slominski A, Ermak G, Wortsman J. Modification of Melanogenesis in Cultured Human Melanoma Cells. In Vitro Cell Dev Biol Anim (1999) 35:564–5. doi: 10.1007/s11626-999-0093-6
226. Brozyna AA, Jozwicki W, Carlson JA, Slominski AT. Melanogenesis Affects Overall and Disease-Free Survival in Patients With Stage III and IV Melanoma. Hum Pathol (2013) 44:2071–4. doi: 10.1016/j.humpath.2013.02.022
227. Brozyna AA, Jozwicki W, Roszkowski K, Filipiak J, Slominski AT. Melanin Content in Melanoma Metastases Affects the Outcome of Radiotherapy. Oncotarget (2016) 7:17844–53. doi: 10.18632/oncotarget.7528
228. Shields CL, Kaliki S, Furuta M, Fulco E, Alarcon C, Shields JA. American Joint Committee on Cancer Classification of Uveal Melanoma (Anatomic Stage) Predicts Prognosis in 7,731 Patients: The 2013 Zimmerman Lecture. Ophthalmology (2015) 122:1180–6. doi: 10.1016/j.ophtha.2015.01.026
229. Shields CL, Kaliki S, Cohen MN, Shields PW, Furuta M, Shields JA. Prognosis of Uveal Melanoma Based on Race in 8100 Patients: The 2015 Doyne Lecture. Eye (Lond) (2015) 29:1027–35. doi: 10.1038/eye.2015.51
230. Brozyna AA, Jozwicki W, Janjetovic Z, Slominski AT. Expression of Vitamin D Receptor Decreases During Progression of Pigmented Skin Lesions. Hum Pathol (2011) 42:618–31. doi: 10.1016/j.humpath.2010.09.014
231. Brozyna AA, Jozwicki W, Skobowiat C, Jetten A, Slominski AT. RORalpha and RORgamma Expression Inversely Correlates With Human Melanoma Progression. Oncotarget (2016) 7:63261–82. doi: 10.18632/oncotarget.11211
232. Markiewicz A, Brozyna AA, Podgorska E, Elas M, Urbanska K, Jetten AM, et al. Vitamin D Receptors (VDR), Hydroxylases CYP27B1 and CYP24A1 and Retinoid-Related Orphan Receptors (ROR) Level in Human Uveal Tract and Ocular Melanoma With Different Melanization Levels. Sci Rep (2019) 9:9142. doi: 10.1038/s41598-019-45161-8
233. Brozyna AA, Jozwicki W, Janjetovic Z, Slominski AT. Expression of the Vitamin D-Activating Enzyme 1alpha-Hydroxylase (CYP27B1) Decreases During Melanoma Progression. Hum Pathol (2013) 44:374–87. doi: 10.1016/j.humpath.2012.03.031
234. Brozyna AA, Jochymski C, Janjetovic Z, Jozwicki W, Tuckey RC, Slominski AT. CYP24A1 Expression Inversely Correlates With Melanoma Progression: Clinic-Pathological Studies. Int J Mol Sci (2014) 15:19000–17. doi: 10.3390/ijms151019000
235. Slominski AT, Brozyna AA, Zmijewski MA, Jozwicki W, Jetten AM, Mason RS, et al. Vitamin D Signaling and Melanoma: Role of Vitamin D and its Receptors in Melanoma Progression and Management. Lab Invest (2017) 97:706–24. doi: 10.1038/labinvest.2017.3
236. Brozyna AA, Hoffman RM, Slominski AT. Relevance of Vitamin D in Melanoma Development, Progression and Therapy. Anticancer Res (2020) 40:473–89. doi: 10.21873/anticanres.13976
237. Fruehauf JP, Meyskens FL Jr. Reactive Oxygen Species: A Breath of Life or Death? Clin Cancer Res (2007) 13:789–94. doi: 10.1158/1078-0432.CCR-06-2082
238. Meyskens FL Jr., Farmer PJ, Yang S, Anton-Culver H. New Perspectives on Melanoma Pathogenesis and Chemoprevention. Recent Results Cancer Res (2007) 174:191–5. doi: 10.1007/978-3-540-37696-5_16
239. Hill HZ, Hill GJ. UVA, Pheomelanin and the Carcinogenesis of Melanoma. Pigment Cell Res (2000) 13(Suppl 8):140–4. doi: 10.1034/j.1600-0749.13.s8.25.x
240. Slominski RM, Zmijewski MA, Slominski AT. The Role of Melanin Pigment in Melanoma. Exp Dermatol (2015) 24:258–9. doi: 10.1111/exd.12618
241. Slominski AT, Carlson JA. Melanoma Resistance: A Bright Future for Academicians and a Challenge for Patient Advocates. Mayo Clin Proc (2014) 89:429–33. doi: 10.1016/j.mayocp.2014.02.009
242. Demopoulos HB. Effects of Reducing the Phenylalanine-Tyrosine Intake of Patients With Advanced Malignant Melanoma. Cancer (1966) 19:657–664. doi: 10.1002/1097-0142(196605)19:5%3C657::AID-CNCR2820190509%3E3.0.CO;2-J
243. Jensen OA, Egeberg J, Edmund J. The Effect of a Phenylalanine-Tyrosine Low Diet on the Growth and Morphology of Transplantable Malignant Melanomas of the Syrian Golden Hamster (Mesocricetus Auratus). Acta Pathol Microbiol Scand A (1973) 81:559–68. doi: 10.1111/j.1699-0463.1973.tb00502.x
244. Elstad CA, Meadows GG, Abdallah RM. Specificity of the Suppression of Metastatic Phenotype by Tyrosine and Phenylalanine Restriction. Clin Exp Metastasis (1990) 8:393–416. doi: 10.1007/bf00058152
245. Riley PA. Melanogenesis: A Realistic Target for Antimelanoma Therapy? Eur J Cancer (1991) 27:1172–7. doi: 10.1016/0277-5379(91)90319-9
246. Jawaid S, Khan TH, Osborn HM, Williams NA. Tyrosinase Activated Melanoma Prodrugs. Anticancer Agents Med Chem (2019) 9:717–27. doi: 10.2174/187152009789056886
247. Tandon M, Thomas PD, Shokravi M, Singh S, Samra S, Chang D, et al. Synthesis and Antitumour Effect of the Melanogenesis-Based Antimelanoma Agent N-Propionyl-4-S-Cysteaminylphenol. Biochem Pharmacol (1998) 55:2023–9. doi: 10.1016/s0006-2952(98)00090-2
248. Riley PA. Melanogenesis and Melanoma. Pigment Cell Res (2003) 16:548–52. doi: 10.1034/j.1600-0749.2003.00069.x
Keywords: melanoma, melanocytes, melanin, melanogenesis, immune responses, oxidative stress, melanoma progression, melanoma therapy
Citation: Slominski RM, Sarna T, Płonka PM, Raman C, Brożyna AA and Slominski AT (2022) Melanoma, Melanin, and Melanogenesis: The Yin and Yang Relationship. Front. Oncol. 12:842496. doi: 10.3389/fonc.2022.842496
Received: 23 December 2021; Accepted: 24 January 2022;
Published: 14 March 2022.
Edited by:
Giuseppe Palmieri, University of Sassari, ItalyReviewed by:
Francisco Solano, University of Murcia, SpainArtur Beberok, Medical University of Silesia, Poland
Copyright © 2022 Slominski, Sarna, Płonka, Raman, Brożyna and Slominski. This is an open-access article distributed under the terms of the Creative Commons Attribution License (CC BY). The use, distribution or reproduction in other forums is permitted, provided the original author(s) and the copyright owner(s) are credited and that the original publication in this journal is cited, in accordance with accepted academic practice. No use, distribution or reproduction is permitted which does not comply with these terms.
*Correspondence: Andrzej T. Slominski, aslominski@uabmc.edu
†ORCID: Radomir M. Slominski, orcid.org/0000-0002-2929-1118
Tadeusz Sarna, orcid.org/0000-0002-7693-5756
Przemysław M. Płonka, orcid.org/0000-0002-0261-3439
Chander Raman, orcid.org/0000-0001-7775-9988
Anna A. Brożyna, orcid.org/0000-0002-3195-9965
Andrzej T. Slominski, orcid.org/0000-0001-8963-3995
‡These authors have contributed equally to this work