- 1Department of Anesthesiology, Shanghai General Hospital, Shanghai Jiao Tong University School of Medicine, Shanghai, China
- 2Department of Anesthesiology, Gansu Provincial Maternity and Child Care Hospital, Lanzhou, China
Despite the significant progress in cancer treatment, new anticancer therapeutics drugs with new structures and/or mechanisms are still in urgent need to tackle many key challenges. Drug repurposing is a feasible strategy in discovering new drugs among the approved drugs by defining new indications. Recently, ropivacaine, a local anesthetic that has been applied in clinical practice for several decades, has been found to possess inhibitory activity and sensitizing effects when combined with conventional chemotherapeutics toward cancer cells. While its full applications and the exact targets remain to be revealed, it has been indicated that its anticancer potency was mediated by multiple mechanisms, such as modulating sodium channel, inducing mitochondria-associated apoptosis, cell cycle arrest, inhibiting autophagy, and/or regulating other key players in cancer cells, which can be termed as multi-targets/functions that require more in-depth studies. In this review, we attempted to summarize the research past decade of using ropivacaine in suppressing cancer growth and sensitizing anticancer drugs both in-vitro and in-vivo, and tried to interpret the underlying action modes. The information gained in these findings may inspire multidisciplinary efforts to develop/discover more novel anticancer agents via drug repurposing.
Introduction
Cancer has become a global health burden in both developing and developed countries. Despite the significant progress of chemotherapies and immunotherapies, unexpected low response rate, unfavorable adverse effects, multidrug resistance (MDR) and cancer recurrence are among the major challenges that undermine effective cancer treatment as summarized in Figure 1 (1–4). New drugs with novel structures and/or mechanisms and novel therapeutic strategies remain unmet clinical needs to tackle these issues. The discovery and development of one new drug, especially de novo drug discovery, may approximately take at least ten years and one billion dollars, rendering it a highly challenging and risky task due to the fact of high attrition rates (5–7). Potential strategies that may serve as shortcuts in drug discovery are 1) old drugs repurposing, 2) co-crystallization between lead compound and its target protein, which may fasten the identification and optimization of drug candidates, 3) artificial intelligence (AI) and machine learning, and 4) others such as high throughput screening (HTS) in natural products or other commercial available compound libraries (8–12). Recently, old drug repurposing has been proved to be a feasible strategy to develop new drugs from those approved drugs by defining new indications (13–18). More importantly, these approved drugs have already been evaluated in humans to possess favorable profiles of pharmacokinetic, pharmacodynamic profiles and safety, as well as controllable/acceptable adverse/toxic effects, which is a procedure of time-consuming (19). Drug repurposing may indeed shorten the overall time in developing and launching a new drug, and may substantially relief the financial burden as compared to de novo drug development (16, 17, 20, 21).
Retrospective clinical studies have suggested that the application of local anesthetics can improve the treatment outcomes of certain cancer patients in pain control and, more strikingly, in suppressing cancer growth (22, 23). In the past decade, researchers have studies intensively in discovering new agents with anticancer activities among local anesthetics, finding that several anesthetics possess broad-spectrum anticancer potencies (24), such as lidocaine (25–27), procaine (28–30), ropivacaine (31–35) and its stereoisomers bupivacaine (36, 37) and levobupivacaine (38, 39). Following our previous review of lidocaine in cancer treatment (11), in the current review, we aimed to summarize the anticancer studies of ropivacaine, another amide-linked local anesthetic (same as lidocaine) that has been widely used in perioperative period as a long acting local anesthetic (40, 41). Structurally, ropivacaine is the S-enantiomer of bupivacaine, while it has a weaker cardiotoxicity and other toxic effects than bupivacaine when used as an anesthetic (42, 43). Ropivacaine, when repurposed as anticancer agent that is administered by single or combination, inhibits cancer cells growth, proliferation, invasion and migration through multiple mechanisms, showing great potential in cancer treatment.
Ropivacaine Demonstrates Anticancer Efficacies
Growing evidence has suggested that at certain concentrations/doses, ropivacaine can work as an anticancer agent by monotherapy or combination. In this section, we summarized these studies categorized by the functions such as inhibiting proliferation or invasion, etc. Compared to lidocaine that has been evaluated in various cancer types in-vitro and in-vivo (11), relatively fewer studies were conducted using ropivacaine in cancer treatment. By far, it’s still not clear about the direct interactions of local anesthetics on cancer cells. Further applications can be significantly expanded after deciphering the exact mechanism and the target of ropivacaine.
Ropivacaine Inhibits Cancer Cells Proliferation
Via Regulating Ras and RhoA Signaling Pathway
There are studies that indicated the anticancer effects of ropivacaine are independent of its role in regulating sodium channel (44). RhoA and Ras are two important members of Ras superfamily that regulates many aspects of cancer cell biology including cell division, proliferation and migration, whose inhibitors hold promising activity against cancer (45, 46). Zheng et al. (44) found that ropivacaine (0.25, 0. 5, 1 and 2 mM) inhibited the proliferation and migration of human melanoma A375 and A431 cells in a concentration-dependent manner via inducing apoptosis (44). More importantly, it (0.5 mM) could also serve as chemosensitizer as it markedly enhanced the potencies of vemurafenib and dacarbazine, two widely used drugs in melanoma treatment, suggesting a broader screening of its potential in drug resistant cancers. Interestingly, its isomer bupivacaine didn’t show such sensitizing effect as ropivacaine. These effects were independent of sodium channel but were mediated by the inhibition of RhoA and Ras, which can be reversed by pre-treatment with the activator of Ras and Rho, calpeptin (44). The Western blot analysis also showed that ropivacaine treatment (1 and 2 mM) not only caused the down-regulation but also inhibited the activities of several downstream signaling of Ras and RhoA, such as MAPK/ERK Kinase (MEK) and myosin phosphatase target subunit 1 (MYPT1) MLC, further verifying its mechanism (44).
Via Regulating Integrin Alpha-2 (ITGα2) and ITGβ1
ITGα2 is a key protein that closely participates in cell adhesion. Serving as a therapeutic target, ITGA2 is found to be overexpressed in certain cancer cell lines and tumor tissues, which may cause the promotion of cancer aggression (47, 48). Ropivacaine (2.5-40 μM) inhibited the proliferation of gastric cancer AGS and BGC-823 cells as shown in a study by Qin et al. (49). Mechanistic study indicated that ropivacaine inhibited the expression of ITGA2 in a concentration-dependent manner, resulting in significant apoptosis as supported by the down-regulated anti-apoptotic B-cell lymphoma 2 (Bcl-2), and up-regulated pro-apoptotic Bcl-2-associated X protein (Bax), cleaved caspase 3/9. Importantly, these effects could be reversed by the overexpression of ITGα2, indicating that ropivacaine’s anticancer effects were mediated by inhibiting ITGα2 (49). In this study, ropivacaine was also found to suppress the invasion and metastasis of human papillary thyroid cancer (PTC) TPC-1 cells, suggesting it a multifunctional agent (49).
Another recent study by Wang and Li (50) showed that ropivacaine (200, 400, 800 μM) induced apoptosis and inhibited the proliferation and migration of colon cancer HCT116 and SW620 cells by targeting another subunit of integrin, ITGβ1 (50).
Via Regulating Wnt/β-Catenin
Cancer stem cells (CSCs) are a subpopulation of cancer cells that have self-renewing and highly proliferative properties, which can often cause cancer recurrence and drug resistance (51–53). Wnt/β-catenin pathway plays critical role in regulating the pluripotency and renewal of CSCs. In addition, Wnt/β-catenin is found to be dys-regulated in cancer patients, indicating its potential therapeutic implication (54, 55). At clinical relevant concentrations, ropivacaine exhibits inhibitory effects towards CSCs. A study conducted by Li et al. (28) showed that ropivacaine (10, 50 and 100 μM) inhibited leukemia stem cell (LSC) stronger than normal hematopoietic stem cell (HSC), although ropivacaine was found to be less potent than lidocaine or bupivacaine (56). At the same concentrations, ropivacaine significantly repressed the colony numbers as well as the serial replating of LSC, likely via inhibiting Wnt/β-catenin as confirmed by Western blot analysis, suggesting its potential capabilities in inhibiting CSCs and warranting further studies (56).
Via Regulating Autophagy Through Vascular Endothelial Growth Factor (VEGF)-A and Signal Transducer and Activator of Transcription 3 (STAT3)
Autophagy is a biological procedure that prompts cancer cells to respond nutrition changes by degrading and then recycling the intracellular biomacromolecules, serving as a promising therapeutic target in cancer (57–59). Combinational therapy of ropivacaine has also been attempted to inhibit pain relief and tumor growth simultaneously. Zhang et al. (20) developed a formulation of liposomes composed with ropivacaine (named as Rop-DPRL), and these liposomes, when combined with nutrition deprivation which may lead to activated autophagy, can suppress the tumor growth of melanoma B16 cells xenograft model and relieve the cancer pain (60). Further study indicated that these effects were mediated by reducing the expression of VEGF-A, and inhibiting the phosphorylation of STAT3 (60).
Via Apoptosis-Associated Pathways and Cell Cycle Arrest
Most of cancer cells die due to apoptosis induced by different therapeutic strategies. Apoptosis, the programmed cell death, can be categorized into external and internal apoptosis which are initiated via distinct pathways. Both of them can serve as therapeutic targets that can be attacked by small-molecule drugs or macromolecule drugs via intervening the key components, e.g., either activating the pro-apoptotic proteins or suppressing the anti-apoptotic ones (61, 62).
One of the hallmarks of cancer cells is the uncontrollable cell division and proliferation. Key enzymes such as cyclin-dependent kinases (CDKs) and members of cyclins are dynamically stimulated to regulate the active cell cycle, rending them to be attracting and druggable targets (63, 64). Growing evidence has showed that ropivacaine can kill cancer cells via inducing apoptosis or cell cycle arrest.
Castelli et al. (65) evaluated the cytotoxicity of ropivacaine on drug-resistant human triple-negative breast cancer MDA-MB-231 cells, and melanoma A375 cells (65). Ropivacaine (5-1000 µM) was found to concentration-dependently inhibit the proliferation of both cell lines, and suppressed the migration as confirmed by the transwell assay. Ropivacaine induced significant apoptosis by up-regulating the cleaved caspase 3 and 9, and it also caused cell arrest via inhibiting the expression of cyclins B2, D1 and E. These effects suggested that ropivacaine suppressed cancer cells proliferation via cell cycle arrest and activating apoptosis pathway (65).
Another study showed that ropivacaine possessed similar activity in human non-small cell lung cancer (NSCLC) A549 and H520 cells (66). Ropivacaine (2-12 mM) inhibited the cell viability, suppressed the invasion and migration at 4.06 and 2.62 mM (ED50 values) via inducing G0/G1 phase arrest and apoptosis by down-regulating anti-apoptotic but up-regulating pro-apoptotic proteins, provoking DNA damage and reactive oxygen species (ROS) production through activating mitogen-activated protein kinase (MAPK) pathways (66). It’s worth noting that this study using much higher concentration of ropivacaine that that of in Castelli et al.’s study (65), which is a common issue using local anesthetic as anticancer agent, further pharmacokinetic studies are needed.
Li et al. (28) reported that at plasma concentrations (10, 35 µM, much lower than 1 mM) for 72 h, ropivacaine failed to decrease cell viability and migration of breast cancer MDA-MB-231 and MCF7 cells, while at higher concentrations (more than 1 mM), it significantly inhibited cell viability and showed cytotoxicity without affecting the viability of a non-cancerous breast cell line, MCF10A, suggesting its selective profile (67). At 10-fold plasma concentrations, ropivacaine suppressed the migration of MDA-MB-231 by inducing cell arrest at the S phase (64).
Another similar result was found that ropivacaine at 1 mM decreased the viability and proliferation of hepatocellular carcinoma (HCC) HuH7 cells while spared the well-differentiated HepaRG cells (68). The levels of mRNA of several key cell-cycle regulators, including cyclin A2, B1/2, and CDK1, as well as the marker of proliferation Ki-67 (MKI67) were significantly suppressed, indicating a cell arrest-mediated mechanism (68).
Mitochondria are pivotal organelles in cancer cells for their roles in ATP production (mitochondrial respiration) and endogenous apoptosis pathway initiating and regulating, rendering them to be attractive therapeutic targets (67, 69, 70). Several studies have indicated that ropivacaine could exert its anticancer effects through inducing mitochondria-mediated apoptosis and/or impacting the respiration pathway (71, 72). In HCC Bel7402 and HLE cells, in the concentration- and time-dependent manners, ropivacaine markedly suppressed the cells proliferation and migration via damaging mitochondria by inducing endogenous apoptosis event as confirmed by the up-regulated caspase 3/9, apoptotic protease activating factor-1 (Apaf-1) and released cytochrome C from mitochondria and down-regulated anti-apoptotic Bcl-2 (73). Another study conducted by Yang et al. (32) showed that at clinically relevant concentrations, ropivacaine was able to suppress the angiogenesis of human lung tumor-associated endothelial cells (HLT-EC) via disturbing the complex II located in the mitochondrial respiration chain. The damaged mitochondrial respiration caused by ropivacaine further leads to ATP depletion, overproduced ROS and finally lethal damages to cells (74).
Another study by Gong et al. (33) showed that ropivacaine (0.5 and 1 mM) inhibited the activities of complex I and II in mitochondrial respiration chain in breast cancer MDA-MB-468 and SkBr cells, leading to the repressed growth, survival and colony formation through inducing oxidative stress (75). Further study indicated that ropivacaine could work as a chemo-sensitizing agent since it (0.5 mM) can enhance the sensitivity of 5-fluorouracil (5-FU) via inhibiting the phosphorylation of Akt, mammalian target of rapamycin (mTOR) and ErbB3 receptor-binding protein 1 (EBP1) (75).
Ropivacaine also exerts inhibitory effects towards mesenchymal stem cells (MSCs) which possess self-renewing property that may contribute in wounds healing and tumor growth (71, 72, 76). At 100 μM, ropivacaine induced proliferation inhibition, cell arrest at the G0/1-S phase, resulting in less colony formation and delayed wound healing via impacting mitochondrial respiration and reducing ATP production (77).
Via Regulating Extracellular Signal-Regulated Kinases 1/2 (ERK1/2)
ERK1/2 signal pathway is one of the central players in regulating cell biology, such as proliferation, differentiation, autophagy, stress response apoptosis and survival (78). Several selective ERK1/2 inhibitors are undergoing clinical trials, showing their great potentials in certain cancers treatment (79). Yang et al. (80) found that ropivacaine (1 mM) significantly inhibited the proliferation and migration of gastric cancer AGS and HG-27 cells via down-regulating phosphorylated ERK1/2 (80). Further studies are necessary to elucidate the details of impacted signal pathway and associated cancer cell biology, e.g., the interaction of ERK1/2 down-regulation with autophagy (81), apoptosis (82), and cell cycle (83), etc.
Via Micro RNAs/Long Non-Coding RNAs (lncRNAs) and Associated Signaling Pathways
Micro RNAs have drawn profound attentions for their roles in regulating cancer progression and migration, serving as therapeutic target (84). Zhang et al. (20) found that ropivacaine could up-regulate miR-520a-3p that can further suppressed the expression of WEE1 and phosphorylated PI3K, leading to concentration- and time-dependent inhibition of the proliferation of gastric cancer AGS and BGC-823 cells, suppression of the migration and invasion (85). More importantly, in the AGS cells xenograft mouse model, ropivacaine (20, 40, and 60 μM/kg) significantly reduced tumor growth, accompanied with up-regulated miR-520a-3p and decreased WEE1 and phosphorylated PI3K (85).
In breast cancer MDA-MB-231 and MCF-7 cells, ropivacaine (1 mM) induced apoptosis, leading to the time-dependent inhibition of the proliferation, and the reduction of the colony formation, as well as decreased cell invasion and migration (86). Ropivacaine was confirmed to up-regulate miR-27b-3p and its target gene YAP to exert its anticancer effects. Ropivacine (40 μM/Kg) inhibited the tumor growth of MDA-MB-231 cells xenograft model, which can be reversed by co-treatment of miR-27b-3p inhibitor (86).
Recently, lncRNAs have shown great potentials as key players in gene regulation and cancer progression. MEG2 lncRNA regulates epigenetic modifications through interacting with chromatin-modifying complexes, acting as a tumor suppressor that is down-regulated in various types of cancer (87). As a central player in cell biology and a therapeutic target, STAT3 is a transcription factor that regulates cell differentiation, proliferation and apoptosis, resulting in promoting cancer progression (88). Chen et al. (89) reported that ropivacaine (0.25, 0.5 and 1 mM) possessed inhibitory effects to cervical cancer SiHa, Caski cells via suppressing the expression of cyclin D1 and survivin, an anti-apoptotic protein (90, 91), by abrogating the phosphorylation and transcriptional activation of STAT3 whose overexpression could reverse the cytotoxicity of ropivacaine (89). These effects were mediated by up-regulating MEG2 and down-regulating microRNA96, suggesting ropivacaine as a potential therapeutic agent for cervical cancer (89).
Ropivacaine Inhibits Cancer Cells Invasion And Migration
As discussed above, ropivacaine at certain concentrations/doses could not only suppress the proliferation, but also the invasion and migration via similar multiple mechanisms. While there are also studies indicated that ropivacaine can only inhibit the invasion and migration, but not be able to kill cancer cells, probably due to the applied different concentrations, e.g., lower concentrations.
Via Regulating Sodium Channels
Proteins in regulating sodium channel such as NaV1.5 voltage-gated Na+ channel (VGSC), can also prompt the tumorigenesis including the proliferation and metastasis of cancers (92, 93). Certain types of metastatic cancer cells, including breast and colon cancer cells, express high level of NaV1.5 VGSC, which may lead to poor prognosis of patients (93–96). Consequently, the block of NaV1.5 VGSC leads to the cease of cancer cell invasion (93, 97). As a local anesthesia, ropivacaine works by inhibiting sodium channel that mediates the pain signal transduction (98). Accordingly, ropivacaine can also well exert its anticancer effects via the same mechanism of action through the inhibition of NaV1.5 VGSC. A study conducted by Baptista-Hon et al. (97) found that, ropivacaine blocked the NaV1.5 VGSC of both neonatal and adult splice variants in colon cancer SW620 cells, with IC50 values of 2.5 and 3.9 μM, respectively. Consequently, ropivacaine inhibited the invasion of SW620 cells (IC50 of 3.8 μM), suggesting its potential application in controlling colon cancer invasion (97).
In 2015, a systematic review by Koltai et al. was published, aiming in searching regulators of VGSCs (NaV1.1 to Nav1.9) and the potentials of their regulators in suppressing invasion and metastasis of cancers (99). In this review, they reported that a couple of local anesthetics, such as ropivacaine and lidocaine, as well as many other drugs, may serve as anticancer agents in suppressing metastasis and invasion of cancer cells (99). However, their further applications in clinic remain to be unveiled (99). This review also suggests a wider screening of this type of approved drugs for their potential in cancer treatment.
Via Attenuating the Axis of Rac1/c-Jun N-Terminal Kinase (JNK)/Paxillin/Focal Adhesion Kinase (FAK)
A study in Zheng’s group (2018) showed that at lower concentrations (less than 200 μM), ropivacaine didn’t impact cancer cell growth and survival but suppress the cell migration (100). As shown in this research, at the clinically relevant concentration (50, 100 and 200μM), ropivacaine inhibited the migration of esophageal cancer OE33, ESO26 and FLO-1 cells via decreasing the activities of GTPases of RhoA, Rac1 and Ras, and inhibiting the prenylation, which were independent of sodium channel. This work demonstrated that the potent anti-migratory effect of ropivacaine in esophageal cancer was mediated by attenuating the axis of Rac1/JNK/paxillin/FAK and prenylation-dependent migratory signaling pathways (100).
Via Regulating Matrix Metalloproteinase 9 (MMP9)/Akt/FAK
MMP9 is a key protein that plays key role in cancer cells invasion, which serves as a prognostic biomarker in certain cancer patients, implying its potential role as a therapeutic target (101–103). In NCI-H838 lung adenocarcinoma cells, ropivacaine (1 nM-100 μM) significantly reduced TNFα-induced activation/phosphorylation of Akt, FAK, caveolin-1 as well as MMP9 via attenuating tyrosine protein kinase Src-dependent inflammatory pathway. Ropivacaine (1 μM) completely inhibited the invasion of NCI-H838 cells, suggesting its potential in suppressing the metastasis (104).
Similar results were also confirmed by Piegeler et al. (2012) that ropivacaine, through its anti-inflammatory effects, suppressed the Src and vascular intercellular adhesion molecule-1 (VCAM-1), two important key players in tumor growth and metastasis (104, 105). Ropivacaine (100 μM for 20 min) decreased the Src activity by 62% through decreasing Src-activation and intercellular adhesion molecule-1 phosphorylation (106).
Via Nuclear Factor Kappa-Light-Chain-Enhancer of Activated B Cells (NF-κB) Pathway
NF-κB pathway regulates cancer cell proliferation, survival, and angiogenesis, playing pivotal role in cell biogenic activities and serving as an attracting target in cancer treatment (105). Su et al. (107) found that ropivacaine (10 μM), when combined with tumor necrosis factor α (TNFα), caused the inhibition of adhesion of three cancer cell lines, human hepatoma HepG2 cells, human colon cancer HT-29 cells and human leukemic monocyte THP-1 cells. These effects were mediated by down-regulating the expression of CD62E, a key protein in regulating adhesion (106, 108). Further mechanistic study showed that ropivacaine significantly suppressed the expression of several key components of NF-κB pathway, including the phosphorylation of p65, IκBα and IKKα/β, indicating that ropivacaine decreased the adhesion of cancer cells via modulating CD62E expression by inhibiting the NF-κB pathway (107).
Via DNA Demethylation
DNA methylation is a procedure through which bases are modified by methyl group, which is found to be highly active in many cancers (109). The inhibitors of DNA methylation can produce anticancer potencies and several of these inhibitors have been approved by FDA (110). Ropivacaine showed epigenetic regulatory effects via modulating DNA methylation. As shown in Lirk et al.’s study (111), ropivacaine at clinically relevant concentrations (3 and 30 µM) didn’t directly kill but decrease the DNA methylation in breast cancer BT-20 cells which lead to lower tumorigenesis properties (111).
Clinical Studies
A recent retrospective cohort study of 215 pancreatic cancer patients by Chen et al. (112) showed that 0.375%-0.5% of intraoperative epidural ropivacaine significantly improve the overall survival (112). Several clinical trials have already been conducted by applying ropivacaine as an adjuvant therapy in shortening the recovery time and other beneficial effects in surgical cancer patients. A clinical study revealed recently (2020) that in liver cancer patients, ropivacaine, when combined with dezocine, could significantly shorten the recovery time of anesthesia and inhibit pain factors secretion, with markedly less adverse reaction, and this combination therapy could reduce stress response, promote patients’ postoperative recovery after cancer surgery (113). Another study (NCT02256228) showed that via the anti-inflammatory and analgesic effects, intraperitoneal ropivacaine in ovarian cancer patients could prompt the postoperative recovery and shorten the time for chemotherapy, which may lead to better overall recovery (114). Similar results were also observed in breast cancer patients who underwent surgery. This study (NCT02691195) showed that in the treatment group, 25 ml of 0.5% ropivacaine could improve the quality of recovery as confirmed by analyzing the 40-item questionnaire, leading to higher patient satisfaction (115). Wang et al. (31) reported that ropivacaine treatment might improve the postoperative cognitive dysfunction in patients following thoracotomy for esophageal cancer by down-regulating inflammatory cytokines such as IL-6 and TNFα (116).
Recently (2020), a study conducted in New Zealand to explore the long term of application of intraperitoneal ropivacaine in colonic cancer patients has been reported. This study had enrolled 60 patients of both benign and malignant colon cancer (stages I-III), and was analyzed by evaluating the overall survival, disease-free survival and recurrence. However, the revealed results showed that the treatment group by ropivacaine didn’t exhibit better overall survival or reduced mortality than the placebo group treated by 0.9% saline solution. And even worse, higher incidence of cancer-specific mortality was found in the ropivacaine-treated group, indicating no beneficial effects by applying intraperitoneal ropivacaine in patients with colonic malignancy (117). While further studies are clearly needed to explore the indications, e.g., earlier stages of cancers, and certain combinational therapy with ropivacaine.
Discussion And Future Perspectives
The above studies indicate that local anesthetic ropivacaine may benefit cancer patients by two ways, 1) inhibit the proliferation or suppresses the migration of cancer cells as we summarized in Table 1 and Figure 2, and 2) shortens the recovery times and improve the quality of life.
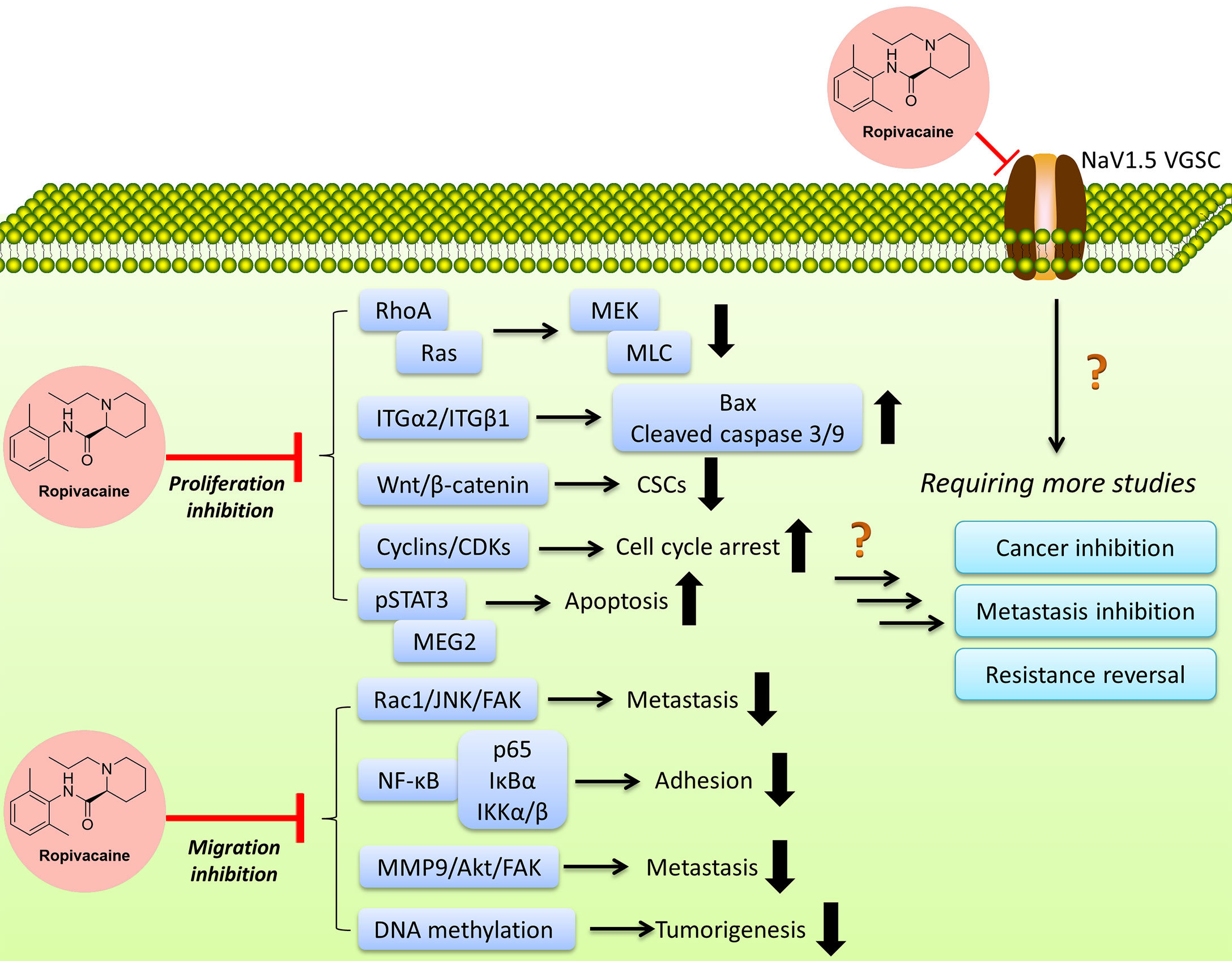
Figure 2 Ropivacaine suppresses cancer cells via multiple targets/functions by modulating multiple signal pathways. More efforts are needed to reveal the full map of the mechanisms.
The studies performed in-vitro and in-vivo have proved that ropivacaine represses the cancer cells invasion and migration at lower concentrations (usually less than 200 μM) (100), while it suppresses cancer growth or kills cancer cells via various acting modes at higher concentrations/doses (mostly more than 1 mM) (118). However, the concentrations/doses vary when it comes to different cancer cells, requiring further in-depth studies for a clear therapeutic window in certain cancer type. In addition, ropivacaine appears to be an enhancer of the sensitivity of certain chemotherapy, suggesting its potential in the treatment of certain resistant cancers (44, 75). The information above may 1) suggest an appealing strategy in screening and identifying certain combinational therapy with ropivacaine, and 2) evoke a broad screening among local anesthetics and related drugs for cancer treatment. However, we cannot overstate the therapeutic implication until more results especially in-vivo and clinical studies are revealed.
In addition to its role in killing cancer cells, ropivacaine also improves the quality of life of cancer patients who have undergone surgical treatment. Many retrospective studies conducted among cancer patients upon treatment of local anesthetics including lidocaine and ropivacaine demonstrate a favorable trend of decrease in tumor metastasis and recurrence. While the clinical trials focusing on the anticancer effects of ropivacaine have yielded limited successes, there are still several ongoing trials for cancer-related diseases (see at http://6tt.co/tjEU on ClinicalTrials.gov), its potentials in cancer treatment remain to be fully revealed.
One interesting finding is the difference of anticancer efficacies of the analogs or isomers of ropivacaine. Ropivacaine and bupivacaine are optical isomers, while they possess different potentials and targets, e.g., GTPases (42), though they also exhibit similar effects in regulating certain targets such as hypoxia-inducible factor 2α (HIF-2α) signaling (115). More efforts are needed to decipher the underlying mechanisms, such as the binding targets, the network through which ropivacaine regulates certain signals transduction in cancers, etc. The application of ropivacaine in cancer treatment is still in its infant stage, more effects are clearly needed to define its indications and administration strategies (either single use or combination). Meanwhile, there are still many questions to be answered, such as the exact targets, and the acting concentrations/doses, more efforts (such as more in-vivo models, combinations, and certain rescue experiments) are needed to fill the blanks to obtain a full view (Figure 2). As per the reported studies, ropivacaine appears to be a multi-target or multi-functional compound. Under clinical relevant (achievable) concentrations/doses, it exerts anti-metastatic, anti-CSCs via regulating sodium channel, anti-inflammatory function and signaling pathways that regulate these two and other associated pathways, leading to the inhibition of signaling transduction which may contribute in the metastasis and maintenance of CSCs (94). Under multiple-fold of clinical-achievable concentrations/doses, ropivacaine is able to kill cancer cells by suppressing key players (proteins or signal pathways) in prompting cancer cells growth, proliferation and migration, including those key proteins in regulating cell cycle, apoptosis, mRNA, epigenetics, autophagy, etc. (64, 116).
It’s noteworthy that ropivacaine appears to exert its anticancer effects via the regulation of ITGα2 and/or members of Ras superfamily, because in the original studies, two pivotal experiments, such as the overexpression of ITGα2 (49) or the co-treatment of calpeptin, an activator of Ras and Rho (44), although other pathways can’t be excluded. Another interesting thing is among all affected pathways, several of them are membrane-associated proteins, such as NaV1.5 VGSC, as well as ITG members, indicating that ropivacaine might preferably attack membrane proteins which require further investigations. Again, future studies of the direct interaction between associated proteins and ropivacaine are warranted, which may help finally explain and identify its exact targets/mechanisms.
Conclusions
Ropivacaine exerts anticancer and chemotherapeutic re-sensitizing effects, showing potentials in benefiting certain cancer patients. Further studies are warranted to explore the mechanisms, combinations and indications.
Author Contributions
PX conceived the idea. PX, SZ, LT, and LW wrote the manuscript. PX, SZ, and JL revised the manuscript. All authors read and approved the final manuscript.
Conflict of Interest
The authors declare that the research was conducted in the absence of any commercial or financial relationships that could be construed as a potential conflict of interest.
Publisher’s Note
All claims expressed in this article are solely those of the authors and do not necessarily represent those of their affiliated organizations, or those of the publisher, the editors and the reviewers. Any product that may be evaluated in this article, or claim that may be made by its manufacturer, is not guaranteed or endorsed by the publisher.
References
1. Assaraf YG, Brozovic A, Goncalves AC, Jurkovicova D, Line A, Machuqueiro M, et al. The Multi-Factorial Nature of Clinical Multidrug Resistance in Cancer. Drug Resist Updat (2019) 46:100645. doi: 10.1016/j.drup.2019.100645
2. Cui Q, Wang JQ, Assaraf YG, Ren L, Gupta P, Wei L, et al. Modulating ROS to Overcome Multidrug Resistance in Cancer. Drug Resist Updat (2018) 41:1–25. doi: 10.1016/j.drup.2018.11.001
3. Mahvi DA, Liu R, Grinstaff MW, Colson YL, Raut CP. Local Cancer Recurrence: The Realities, Challenges, and Opportunities for New Therapies. CA Cancer J Clin (2018) 68(6):488–505. doi: 10.3322/caac.21498
4. Zugazagoitia J, Guedes C, Ponce S, Ferrer I, Molina-Pinelo S, Paz-Ares L. Current Challenges in Cancer Treatment. Clin Ther (2016) 38(7):1551–66. doi: 10.1016/j.clinthera.2016.03.026
5. Takebe T, Imai R, Ono S. The Current Status of Drug Discovery and Development as Originated in United States Academia: The Influence of Industrial and Academic Collaboration on Drug Discovery and Development. Clin Transl Sci (2018) 11(6):597–606. doi: 10.1111/cts.12577
6. Munos B. Lessons From 60 Years of Pharmaceutical Innovation. Nat Rev Drug Discov (2009) 8(12):959–68. doi: 10.1038/nrd2961
7. Berdigaliyev N, Aljofan M. An Overview of Drug Discovery and Development. Future Med Chem (2020) 12(10):939–47. doi: 10.4155/fmc-2019-0307
8. Huang J. Can Drug Repositioning Work as a Systematical Business Model? ACS Med Chem Lett (2020) 11(6):1074–75. doi: 10.1021/acsmedchemlett.0c00122
9. Duggirala NK, Perry ML, Almarsson O, Zaworotko MJ. Pharmaceutical Cocrystals: Along the Path to Improved Medicines. Chem Commun (Camb) (2016) 52(4):640–55. doi: 10.1039/C5CC08216A
10. Cui Q, Yang DH, Chen ZS. Special Issue: Natural Products: Anticancer and Beyond. Molecules (2018) 23(6):1246–49. doi: 10.3390/molecules23061246
11. Zhou D, Wang L, Cui Q, Iftikhar R, Xia Y, Xu P. Repositioning Lidocaine as an Anticancer Drug: The Role Beyond Anesthesia. Front Cell Dev Biol (2020) 8:565/full. doi: 10.3389/fcell.2020.00565/full
12. Mak KK, Pichika MR. Artificial Intelligence in Drug Development: Present Status and Future Prospects. Drug Discov Today (2019) 24(3):773–80. doi: 10.1016/j.drudis.2018.11.014
13. Dinic J, Efferth T, Garcia-Sosa AT, Grahovac J, Padron JM, Pajeva I, et al. Repurposing Old Drugs to Fight Multidrug Resistant Cancers. Drug Resist Updat (2020) 52:100713. doi: 10.1016/j.drup.2020.100713
14. Krchniakova M, Skoda J, Neradil J, Chlapek P, Veselska R. Repurposing Tyrosine Kinase Inhibitors to Overcome Multidrug Resistance in Cancer: A Focus on Transporters and Lysosomal Sequestration. Int J Mol Sci (2020) 21(9):3157–75. doi: 10.3390/ijms21093157
15. Fan YF, Zhang W, Zeng L, Lei ZN, Cai CY, Gupta P, et al. Dacomitinib Antagonizes Multidrug Resistance (MDR) in Cancer Cells by Inhibiting The Efflux Activity of ABCB1 and ABCG2 Transporters. Cancer Lett (2018) 421:186–98. doi: 10.1016/j.canlet.2018.01.021
16. Parvathaneni V, Kulkarni NS, Muth A, Gupta V. Drug Repurposing: A Promising Tool to Accelerate the Drug Discovery Process. Drug Discov Today (2019) 24(10):2076–85. doi: 10.1016/j.drudis.2019.06.014
17. Polamreddy P, Gattu N. The Drug Repurposing Landscape From 2012 to 2017: Evolution, Challenges, and Possible Solutions. Drug Discov Today (2019) 24(3):789–95. doi: 10.1016/j.drudis.2018.11.022
18. Cui Q, Cai CY, Gao HL, Ren L, Ji N, Gupta P, et al. Glesatinib, a C-MET/SMO Dual Inhibitor, Antagonizes P-Glycoprotein Mediated Multidrug Resistance in Cancer Cells. Front Oncol (2019) 9:313. doi: 10.3389/fonc.2019.00313
19. Pushpakom S, Iorio F, Eyers PA, Escott KJ, Hopper S, Wells A, et al. Drug Repurposing: Progress, Challenges and Recommendations. Nat Rev Drug Discov (2018) 18(1):41–58. doi: 10.1038/nrd.2018.168
20. Zhang Z, Zhou L, Xie N, Nice EC, Zhang T, Cui Y, et al. Overcoming Cancer Therapeutic Bottleneck by Drug Repurposing. Signal Transduct Target Ther (2020) 5(1):113. doi: 10.1038/s41392-020-00213-8
21. Cui Q, Cai CY, Wang JQ, Zhang S, Gupta P, Ji N, et al. Chk1 Inhibitor MK-8776 Restores the Sensitivity of Chemotherapeutics in P-Glycoprotein Overexpressing Cancer Cells. Int J Mol Sci (2019) 20(17):4095–106. doi: 10.3390/ijms20174095
22. Siekmann W, Tina E, Von Sydow AK, Gupta A. Effect of Lidocaine and Ropivacaine on Primary (SW480) and Metastatic (SW620) Colon Cancer Cell Lines. Oncol Lett (2019) 18(1):395–401. doi: 10.3892/ol.2019.10332
23. Lynch CR. Local Anesthetics as...Cancer Therapy? Anesth Analg (2018) 127(3):601–02. doi: 10.1213/ANE.0000000000003659
24. Liu H, Dilger JP, Lin J. Effects of Local Anesthetics on Cancer Cells. Pharmacol Ther (2020) 212:107558. doi: 10.1016/j.pharmthera.2020.107558
25. Sun H, Sun Y. Lidocaine Inhibits Proliferation and Metastasis of Lung Cancer Cell. via Regul miR-539/EGFR axis Artif Cells Nanomed Biotechnol (2019) 47(1):2866–74. doi: 10.1080/21691401.2019.1636807
26. Chen J, Jiao Z, Wang A, Zhong W. Lidocaine Inhibits Melanoma Cell Proliferation by Regulating ERK Phosphorylation. J Cell Biochem (2019) 120(4):6402–08. doi: 10.1002/jcb.27927
27. Gao J, Hu H, Wang X. Clinically Relevant Concentrations of Lidocaine Inhibit Tumor Angiogenesis Through Suppressing VEGF/VEGFR2 Signaling. Cancer Chemother Pharmacol (2019) 83(6):1007–15. doi: 10.1007/s00280-019-03815-4
28. Li C, Gao S, Li X, Li C, Ma L. Procaine Inhibits the Proliferation and Migration of Colon Cancer Cells Through Inactivation of the ERK/MAPK/FAK Pathways by Regulation of RhoA. Oncol Res (2018) 26(2):209–17. doi: 10.3727/096504017X14944585873622
29. Li YC, Wang Y, Li DD, Zhang Y, Zhao TC, Li CF. Procaine Is a Specific DNA Methylation Inhibitor With Anti-Tumor Effect for Human Gastric Cancer. J Cell Biochem (2018) 119(2):2440–49. doi: 10.1002/jcb.26407
30. Ma XW, Li Y, Han XC, Xin QZ. The Effect of Low Dosage of Procaine on Lung Cancer Cell Proliferation. Eur Rev Med Pharmacol Sci (2016) 20(22):4791–95.
31. Wang W, Zhu M, Xu Z, Li W, Dong X, Chen Y, et al. Ropivacaine Promotes Apoptosis of Hepatocellular Carcinoma Cells Through Damaging Mitochondria and Activating Caspase-3 Activity. Biol Res (2019) 52(1):36. doi: 10.1186/s40659-019-0242-7
32. Yang J, Li G, Bao K, Liu W, Zhang Y, Ting W. Ropivacaine Inhibits Tumor Angiogenesis via Sodium-Channel-Independent Mitochondrial Dysfunction and Oxidative Stress. J Bioenerg Biomembr (2019) 51(3):231–38. doi: 10.1007/s10863-019-09793-9
33. Gong X, Dan J, Li F, Wang L. Suppression of Mitochondrial Respiration With Local Anesthetic Ropivacaine Targets Breast Cancer Cells. J Thorac Dis (2018) 10(5):2804–12. doi: 10.21037/jtd.2018.05.21
34. Piegeler T, Schlapfer M, Dull RO, Schwartz DE, Borgeat A, Minshall RD, et al. Clinically Relevant Concentrations of Lidocaine and Ropivacaine Inhibit TNFalpha-Induced Invasion of Lung Adenocarcinoma Cells In Vitro by Blocking the Activation of Akt and Focal Adhesion Kinase. Br J Anaesth (2015) 115(5):784–91. doi: 10.1093/bja/aev341
35. Zhu G, Zhang L, Dan J, Zhu Q. Differential Effects and Mechanisms of Local Anesthetics on Esophageal Carcinoma Cell Migration, Growth, Survival and Chemosensitivity. BMC Anesthesiol (2020) 20(1):126. doi: 10.1186/s12871-020-01039-1
36. Dan J, Gong X, Li D, Zhu G, Wang L, Li F. Inhibition of Gastric Cancer by Local Anesthetic Bupivacaine Through Multiple Mechanisms Independent of Sodium Channel Blockade. Biomed Pharmacother (2018) 103:823–28. doi: 10.1016/j.biopha.2018.04.106
37. Xuan W, Zhao H, Hankin J, Chen L, Yao S, Ma D. Local Anesthetic Bupivacaine Induced Ovarian and Prostate Cancer Apoptotic Cell Death and Underlying Mechanisms In Vitro. Sci Rep (2016) 6:26277. doi: 10.1038/srep26277
38. Li T, Chen L, Zhao H, Wu L, Masters J, Han C, et al. And Ma D.: Both Bupivacaine and Levobupivacaine Inhibit Colon Cancer Cell Growth But Not Melanoma Cells In Vitro. J Anesth (2019) 33(1):17–25. doi: 10.1007/s00540-018-2577-6
39. Jose C, Hebert-Chatelain E, Dias AN, Roche E, Obre E, Lacombe D, et al. And Rossignol R.: Redox Mechanism of Levobupivacaine Cytostatic Effect on Human Prostate Cancer Cells. Redox Biol (2018) 18:33–42. doi: 10.1016/j.redox.2018.05.014
40. Hansen TG. Ropivacaine: A Pharmacological Review. Expert Rev Neurother (2004) 4(5):781–91. doi: 10.1586/14737175.4.5.781
41. Casati A, Santorsola R, Cerchierini E, Moizo E. Ropivacaine. Minerva Anestesiol (2001) 67(9 Suppl 1):15–9.
42. Graf BM, Abraham I, Eberbach N, Kunst G, Stowe DF, Martin E. Differences in Cardiotoxicity of Bupivacaine and Ropivacaine Are the Result of Physicochemical and Stereoselective Properties. Anesthesiol (2002) 96(6):1427–34. doi: 10.1097/00000542-200206000-00023
43. Leone S, Di Cianni S, Casati A, Fanelli G. Pharmacology, Toxicology, and Clinical Use of New Long Acting Local Anesthetics, Ropivacaine and Levobupivacaine. Acta Biomed (2008) 79(2):92–105.
44. Zheng Q, Peng X, Zhang Y. Cytotoxicity of Amide-Linked Local Anesthetics on Melanoma Cells via Inhibition of Ras and RhoA Signaling Independent of Sodium Channel Blockade. BMC Anesthesiol (2020) 20(1):43. doi: 10.1186/s12871-020-00957-4
45. Phuyal S, Farhan H. Multifaceted Rho GTPase Signaling at the Endomembranes. Front Cell Dev Biol (2019) 7:127. doi: 10.3389/fcell.2019.00127
46. Qu L, Pan C, He SM, Lang B, Gao GD, Wang XL, et al. The Ras Superfamily of Small GTPases in Non-Neoplastic Cerebral Diseases. Front Mol Neurosci (2019) 12:121. doi: 10.3389/fnmol.2019.00121
47. Adorno-Cruz V, Liu H. Regulation and Functions of Integrin Alpha2 in Cell Adhesion and Disease. Genes Dis (2019) 6(1):16–24. doi: 10.1016/j.gendis.2018.12.003
48. Ren D, Zhao J, Sun Y, Li D, Meng Z, Wang B, et al. Overexpressed ITGA2 Promotes Malignant Tumor Aggression by Up-Regulating PD-L1 Expression Through the Activation of the STAT3 Signaling Pathway. J Exp Clin Cancer Res (2019) 38(1):485. doi: 10.1186/s13046-019-1496-1
49. Qin A, Liu Q, Wang J. Ropivacaine Inhibits Proliferation, Invasion, Migration and Promotes Apoptosis of Papillary Thyroid Cancer Cells via Regulating ITGA2 Expression. Drug Dev Res (2020) 81(6):700–7. doi: 10.1002/ddr.21671
50. Wang X, Li T. Ropivacaine Inhibits the Proliferation and Migration of Colorectal Cancer Cells Through ITGB1. Bioengineered (2021) 12(1):44–53. doi: 10.1080/21655979.2020.1857120
51. Yeldag G, Rice A, Del RHA. Chemoresistance and the Self-Maintaining Tumor Microenvironment. Cancers (Basel) (2018) 10(12):471–503. doi: 10.3390/cancers10120471
52. Prieto-Vila M, Takahashi RU, Usuba W, Kohama I, Ochiya T. Drug Resistance Driven by Cancer Stem Cells and Their Niche. Int J Mol Sci (2017) 18(12):2574–95. doi: 10.3390/ijms18122574
53. Suresh R, Ali S, Ahmad A, Philip PA, Sarkar FH. The Role of Cancer Stem Cells in Recurrent and Drug-Resistant Lung Cancer. Adv Exp Med Biol (2016) 890:57–74. doi: 10.1007/978-3-319-24932-2_4
54. Pai SG, Carneiro BA, Mota JM, Costa R, Leite CA, Barroso-Sousa R, et al. Wnt/beta-Catenin Pathway: Modulating Anticancer Immune Response. J Hematol Oncol (2017) 10(1):101. doi: 10.1186/s13045-017-0471-6
55. Li X, Xiang Y, Li F, Yin C, Li B, Ke X. WNT/beta-Catenin Signaling Pathway Regulating T Cell-Inflammation in the Tumor Microenvironment. Front Immunol (2019) 10:2293. doi: 10.3389/fimmu.2019.02293
56. Ni J, Xie T, Xiao M, Xiang W, Wang L. Amide-Linked Local Anesthetics Preferentially Target Leukemia Stem Cell Through Inhibition of Wnt/beta-Catenin. Biochem Biophys Res Commun (2018) 503(2):956–62. doi: 10.1016/j.bbrc.2018.06.102
57. Gao L, Jauregui CE, Teng Y. Targeting Autophagy as a Strategy for Drug Discovery and Therapeutic Modulation. Future Med Chem (2017) 9(3):335–45. doi: 10.4155/fmc-2016-0210
58. Mathiassen SG, De Zio D, Cecconi F. Autophagy and the Cell Cycle: A Complex Landscape. Front Oncol (2017) 7:51. doi: 10.3389/fonc.2017.00051
59. Yun CW, Lee SH. The Roles of Autophagy in Cancer. Int J Mol Sci (2018) 19(11):3466–83. doi: 10.3390/ijms19113466
60. Zhang J, Zhu S, Tan Q, Cheng D, Dai Q, Yang Z, et al. Combination Therapy With Ropivacaine-Loaded Liposomes and Nutrient Deprivation for Simultaneous Cancer Therapy and Cancer Pain Relief. Theranostics (2020) 10(11):4885–99. doi: 10.7150/thno.43932
61. Carneiro BA, El-Deiry WS. Targeting Apoptosis in Cancer Therapy. Nat Rev Clin Oncol (2020) 17(7):395–417. doi: 10.1038/s41571-020-0341-y
62. Shahar N, Larisch S. Inhibiting the Inhibitors: Targeting Anti-Apoptotic Proteins in Cancer and Therapy Resistance. Drug Resist Updat (2020) 52:100712. doi: 10.1016/j.drup.2020.100712
63. Otto T, Sicinski P. Cell Cycle Proteins as Promising Targets in Cancer Therapy. Nat Rev Cancer (2017) 17(2):93–115. doi: 10.1038/nrc.2016.138
64. Visconti R, Della MR, Grieco D. Cell Cycle Checkpoint in Cancer: A Therapeutically Targetable Double-Edged Sword. J Exp Clin Cancer Res (2016) 35(1):153. doi: 10.1186/s13046-016-0433-9
65. Castelli V, Piroli A, Marinangeli F, D'Angelo M, Benedetti E, Ippoliti R, et al. Local Anesthetics Counteract Cell Proliferation and Migration of Human Triple-Negative Breast Cancer and Melanoma Cells. J Cell Physiol (2020) 235(4):3474–84. doi: 10.1002/jcp.29236
66. Wang HW, Wang LY, Jiang L, Tian SM, Zhong TD, Fang XM. Amide-Linked Local Anesthetics Induce Apoptosis in Human Non-Small Cell Lung Cancer. J Thorac Dis (2016) 8(10):2748–57. doi: 10.21037/jtd.2016.09.66
67. Abate M, Festa A, Falco M, Lombardi A, Luce A, Grimaldi A, et al. Mitochondria as Playmakers of Apoptosis, Autophagy and Senescence. Semin Cell Dev Biol (2019) 98:139–53. doi: 10.1016/j.semcdb.2019.05.022
68. Le Gac G, Angenard G, Clement B, Laviolle B, Coulouarn C, Beloeil H. Local Anesthetics Inhibit the Growth of Human Hepatocellular Carcinoma Cells. Anesth Analg (2017) 125(5):1600–09. doi: 10.1213/ANE.0000000000002429
69. Cui Q, Wen S, Huang P. Targeting Cancer Cell Mitochondria as a Therapeutic Approach: Recent Updates. Future Med Chem (2017) 9(9):929–49. doi: 10.4155/fmc-2017-0011
70. Supinski GS, Schroder EA, Callahan LA. Mitochondria and Critical Illness. Chest (2019) 157(2):310–22. doi: 10.1016/j.chest.2019.08.2182
71. Pittenger MF, Discher DE, Peault BM, Phinney DG, Hare JM, Caplan AI. Mesenchymal Stem Cell Perspective: Cell Biology to Clinical Progress. NPJ Regener Med (2019) 4:22. doi: 10.1038/s41536-019-0083-6
72. Abdal DA, Lee SB, Kim K, Lim KM, Jeon TI, Seok J, et al. Production of Mesenchymal Stem Cells Through Stem Cell Reprogramming. Int J Mol Sci (2019) 20(8):1922–63. doi: 10.3390/ijms20081922
73. Wang W, Zhu M, Xu Z, Li W, Dong X, Chen Y, et al. Ropivacaine Promotes Apoptosis of Hepatocellular Carcinoma Cells Through Damaging Mitochondria and Activating Caspase-3 Activity. Biol Res (2019) 52(1):36. doi: 10.1186/s40659-019-0242-7
74. Yang J, Li G, Bao K, Liu W, Zhang Y, Ting W. Ropivacaine Inhibits Tumor Angiogenesis via Sodium-Channel-Independent Mitochondrial Dysfunction and Oxidative Stress. J Bioenerg Biomembr (2019) 51(3):231–38. doi: 10.1007/s10863-019-09793-9
75. Gong X, Dan J, Li F, Wang L. Suppression of Mitochondrial Respiration With Local Anesthetic Ropivacaine Targets Breast Cancer Cells. J Thorac Dis (2018) 10(5):2804–12. doi: 10.21037/jtd.2018.05.21
76. Chan TS, Shaked Y, Tsai KK. Targeting the Interplay Between Cancer Fibroblasts, Mesenchymal Stem Cells, and Cancer Stem Cells in Desmoplastic Cancers. Front Oncol (2019) 9:688. doi: 10.3389/fonc.2019.00688
77. Lucchinetti E, Awad AE, Rahman M, Feng J, Lou PH, Zhang L, et al. Antiproliferative Effects of Local Anesthetics on Mesenchymal Stem Cells: Potential Implications for Tumor Spreading and Wound Healing. Anesthesiol (2012) 116(4):841–56. doi: 10.1097/ALN.0b013e31824babfe
78. Wortzel I, Seger R. The ERK Cascade: Distinct Functions Within Various Subcellular Organelles. Genes Cancer (2011) 2(3):195–209. doi: 10.1177/1947601911407328
79. Kidger AM, Sipthorp J, Cook SJ. ERK1/2 Inhibitors: New Weapons to Inhibit the RAS-Regulated RAF-MEK1/2-ERK1/2 Pathway. Pharmacol Ther (2018) 187:45–60. doi: 10.1016/j.pharmthera.2018.02.007
80. Yang W, Cai J, Zhang H, Wang G, Jiang W. Effects of Lidocaine and Ropivacaine on Gastric Cancer Cells Through Down-Regulation of ERK1/2 Phosphorylation In Vitro. Anticancer Res (2018) 38(12):6729–35. doi: 10.21873/anticanres.13042
81. Xu C, Zhang W, Liu S, Wu W, Qin M, Huang J. Activation of the SphK1/ERK/p-ERK Pathway Promotes Autophagy in Colon Cancer Cells. Oncol Lett (2018) 15(6):9719–24. doi: 10.3892/ol.2018.8588
82. Melo-Lima S, Lopes MC, Mollinedo F. ERK1/2 Acts as a Switch Between Necrotic and Apoptotic Cell Death in Ether Phospholipid Edelfosine-Treated Glioblastoma Cells. Pharmacol Res (2015) 95-96:2–11. doi: 10.1016/j.phrs.2015.02.007
83. Lu Y, Liu B, Liu Y, Yu X, Cheng G. Dual Effects of Active ERK in Cancer: A Potential Target for Enhancing Radiosensitivity. Oncol Lett (2020) 20(2):993–1000. doi: 10.3892/ol.2020.11684
84. Ors-Kumoglu G, Gulce-Iz S, Biray-Avci C. Therapeutic microRNAs in Human Cancer. Cytotechnol (2019) 71(1):411–25. doi: 10.1007/s10616-018-0291-8
85. Zhang N, Xing X, Gu F, Zhou G, Liu X, Li B. Ropivacaine Inhibits the Growth, Migration and Invasion of Gastric Cancer Through Attenuation of WEE1 and PI3K/AKT Signaling via miR-520a-3p. Onco Targets Ther (2020) 13:5309–21. doi: 10.2147/OTT.S244550
86. Zhao L, Han S, Hou J, Shi W, Zhao Y, Chen Y. The Local Anesthetic Ropivacaine Suppresses Progression of Breast Cancer by Regulating miR-27b-3p/YAP Axis. Aging (Albany NY) (2021) 13(12):16341–52. doi: 10.18632/aging.203160
87. Sherpa C, Rausch JW, Le Grice SF. Structural Characterization of Maternally Expressed Gene 3 RNA Reveals Conserved Motifs and Potential Sites of Interaction With Polycomb Repressive Complex 2. Nucleic Acids Res (2018) 46(19):10432–47. doi: 10.1093/nar/gky722
88. Huynh J, Chand A, Gough D, Ernst M. Therapeutically Exploiting STAT3 Activity in Cancer - Using Tissue Repair as a Road Map. Nat Rev Cancer (2019) 19(2):82–96. doi: 10.1038/s41568-018-0090-8
89. Chen X, Liu W, Guo X, Huang S, Song X. Ropivacaine Inhibits Cervical Cancer Cell Growth via Suppression of the Mir96/MEG2/pSTAT3 Axis. Oncol Rep (2020) 43(5):1659–68. doi: 10.3892/or.2020.7521
90. Peery R, Kyei-Baffour K, Dong Z, Liu J, de Andrade HP, Dai M, et al. Synthesis and Identification of a Novel Lead Targeting Survivin Dimerization for Proteasome-Dependent Degradation. J Med Chem (2020) 63(1):7243–51. doi: 10.1021/acs.jmedchem.0c00475
91. Peery RC, Liu JY, Zhang JT. Targeting Survivin for Therapeutic Discovery: Past, Present, and Future Promises. Drug Discov Today (2017) 22(10):1466–77. doi: 10.1016/j.drudis.2017.05.009
92. Roger S, Gillet L, Le Guennec JY, Besson P. Voltage-Gated Sodium Channels and Cancer: Is Excitability Their Primary Role? Front Pharmacol (2015) 6:152. doi: 10.3389/fphar.2015.00152
93. Mao W, Zhang J, Korner H, Jiang Y, Ying S. The Emerging Role of Voltage-Gated Sodium Channels in Tumor Biology. Front Oncol (2019) 9:124. doi: 10.3389/fonc.2019.00124
94. Nelson M, Yang M, Millican-Slater R, Brackenbury WJ. Nav1.5 Regulates Breast Tumor Growth and Metastatic Dissemination In Vivo. Oncotarget (2015) 6(32):32914–29. doi: 10.18632/oncotarget.5441
95. Peng J, Ou Q, Wu X, Zhang R, Zhao Q, Jiang W, et al. Expression of Voltage-Gated Sodium Channel Nav1.5 in Non-Metastatic Colon Cancer and Its Associations With Estrogen Receptor (ER)-Beta Expression and Clinical Outcomes. Chin J Cancer (2017) 36(1):89. doi: 10.1186/s40880-017-0253-0
96. Djamgoz M, Fraser SP, Brackenbury WJ. In Vivo Evidence for Voltage-Gated Sodium Channel Expression in Carcinomas and Potentiation of Metastasis. Cancers (Basel) (2019) 11(11):1675–94. doi: 10.3390/cancers11111675
97. Baptista-Hon DT, Robertson FM, Robertson GB, Owen SJ, Rogers GW, Lydon EL, et al. Potent Inhibition by Ropivacaine of Metastatic Colon Cancer SW620 Cell Invasion and NaV1.5 Channel Function. Br J Anaesth (2014) 113(Suppl 1):i39–48. doi: 10.1093/bja/aeu104
98. Arlock P. Actions of Three Local Anaesthetics: Lidocaine, Bupivacaine and Ropivacaine on Guinea Pig Papillary Muscle Sodium Channels (Vmax). Pharmacol Toxicol (1988) 63(2):96–104. doi: 10.1111/j.1600-0773.1988.tb00918.x
99. Koltai T. Voltage-Gated Sodium Channel as a Target for Metastatic Risk Reduction With Re-Purposed Drugs. F1000Res (2015) 4:297. doi: 10.12688/f1000research.6789.1
100. Zhang Y, Peng X, Zheng Q. Ropivacaine Inhibits the Migration of Esophageal Cancer Cells via Sodium-Channel-Independent But Prenylation-Dependent Inhibition of Rac1/JNK/Paxillin/FAK. Biochem Biophys Res Commun (2018) 501(4):1074–79. doi: 10.1016/j.bbrc.2018.05.110
101. Huang H. Matrix Metalloproteinase-9 (MMP-9) as a Cancer Biomarker and MMP-9 Biosensors: Recent Advances. Sensors (Basel) (2018) 18(10):3249–67. doi: 10.3390/s18103249
102. Zarkesh M, Zadeh-Vakili A, Akbarzadeh M, Fanaei SA, Hedayati M, Azizi F. The Role of Matrix Metalloproteinase-9 as a Prognostic Biomarker in Papillary Thyroid Cancer. BMC Cancer (2018) 18(1):1199. doi: 10.1186/s12885-018-5112-0
103. Barillari G. The Impact of Matrix Metalloproteinase-9 on the Sequential Steps of the Metastatic Process. Int J Mol Sci (2020) 21(12):4526–53. doi: 10.3390/ijms21124526
104. Piegeler T, Schlapfer M, Dull RO, Schwartz DE, Borgeat A, Minshall RD, et al. Clinically Relevant Concentrations of Lidocaine and Ropivacaine Inhibit TNFalpha-Induced Invasion of Lung Adenocarcinoma Cells In Vitro by Blocking the Activation of Akt and Focal Adhesion Kinase. Br J Anaesth (2015) 115(5):784–91. doi: 10.1093/bja/aev341
105. Xia L, Tan S, Zhou Y, Lin J, Wang H, Oyang L, et al. Role of the NFkappaB-Signaling Pathway in Cancer. Onco Targets Ther (2018) 11:2063–73. doi: 10.2147/OTT.S161109
106. Dixon GL, Heyderman RS, van der Ley P, Klein NJ. High-Level Endothelial E-Selectin (CD62E) Cell Adhesion Molecule Expression by a Lipopolysaccharide-Deficient Strain of Neisseria Meningitidis Despite Poor Activation of NF-kappaB Transcription Factor. Clin Exp Immunol (2004) 135(1):85–93. doi: 10.1111/j.1365-2249.2004.02335.x
107. Su Z, Huang P, Ye X, Huang S, Li W, Yan Y, et al. Ropivacaine via Nuclear Factor Kappa B Signalling Modulates CD62E Expression and Diminishes Tumour Cell Arrest. J Anesth (2019) 33(6):685–93. doi: 10.1007/s00540-019-02699-1
108. Jassam SA, Maherally Z, Smith JR, Ashkan K, Roncaroli F, Fillmore HL, et al. CD15s/CD62E Interaction Mediates the Adhesion of Non-Small Cell Lung Cancer Cells on Brain Endothelial Cells: Implications for Cerebral Metastasis. Int J Mol Sci (2017) 18(7):1474–89. doi: 10.3390/ijms18071474
109. Kulis M, Esteller M. DNA Methylation and Cancer. Adv Genet (2010) 70:27–56. doi: 10.1016/B978-0-12-380866-0.60002-2
110. Giri AK, Aittokallio T. DNMT Inhibitors Increase Methylation in the Cancer Genome. Front Pharmacol (2019) 10:385. doi: 10.3389/fphar.2019.00385
111. Lirk P, Hollmann MW, Fleischer M, Weber NC. And Fiegl H.: Lidocaine and Ropivacaine, But Not Bupivacaine, Demethylate Deoxyribonucleic Acid in Breast Cancer Cells In Vitro. Br J Anaesth (2014) 113(Suppl 1):i32–38. doi: 10.1093/bja/aeu201
112. Chen W, Xu Y, Zhang Y, Lou W, Han X. Positive Impact of Intraoperative Epidural Ropivacaine Infusion on Oncologic Outcomes in Pancreatic Cancer Patients Undergoing Pancreatectomy: A Retrospective Cohort Study. J Cancer (2021) 12(15):4513–21. doi: 10.7150/jca.57661
113. Zhu R, Du T, Gao H. Effects of Dezocine and Ropivacaine Infiltration Anesthesia on Cellular Immune Function Indicators, Anesthesia Recovery Time and Pain Factors in Patients With Open Liver Resection. Cell Mol Biol (Noisy-le-grand) (2020) 66(3):149–54. doi: 10.14715/cmb/2020.66.3.23
114. Hayden JM, Oras J, Block L, Thorn SE, Palmqvist C, Salehi S, et al. Intraperitoneal Ropivacaine Reduces Time Interval to Initiation of Chemotherapy After Surgery for Advanced Ovarian Cancer: Randomised Controlled Double-Blind Pilot Study. Br J Anaesth (2020) 124(5):562–70. doi: 10.1016/j.bja.2020.01.026
115. Yao Y, Li J, Hu H, Xu T, Chen Y. Ultrasound-Guided Serratus Plane Block Enhances Pain Relief and Quality of Recovery After Breast Cancer Surgery: A Randomised Controlled Trial. Eur J Anaesthesiol (2019) 36(6):436–41. doi: 10.1097/EJA.0000000000001004
116. Wang Y, Cheng J, Yang L, Wang J, Liu H, Lv Z. Ropivacaine for Intercostal Nerve Block Improves Early Postoperative Cognitive Dysfunction in Patients Following Thoracotomy for Esophageal Cancer. Med Sci Monit (2019) 25:460–65. doi: 10.12659/MSM.912328
117. MacFater WS, Xia W, Barazanchi A, MacFater HS, Lightfoot N, Svirskis D, et al. Association Between Perioperative Intraperitoneal Local Anaesthetic Infusion and Long-Term Survival and Cancer Recurrence After Colectomy: Follow-Up Analysis of a Previous Randomized Controlled Trial. ANZ J Surg (2020) 90(5):802–06. doi: 10.1111/ans.15753
Keywords: drug repurposing, local anesthetics, ropivacaine, anticancer, mechanisms
Citation: Xu P, Zhang S, Tan L, Wang L, Yang Z and Li J (2022) Local Anesthetic Ropivacaine Exhibits Therapeutic Effects in Cancers. Front. Oncol. 12:836882. doi: 10.3389/fonc.2022.836882
Received: 16 December 2021; Accepted: 10 January 2022;
Published: 03 February 2022.
Edited by:
Leli Zeng, Sun Yat-sen University, ChinaReviewed by:
Enming Du, Henan Provincial People’s Hospital, ChinaKaiyan Liu, University of Maryland, Baltimore, United States
Copyright © 2022 Xu, Zhang, Tan, Wang, Yang and Li. This is an open-access article distributed under the terms of the Creative Commons Attribution License (CC BY). The use, distribution or reproduction in other forums is permitted, provided the original author(s) and the copyright owner(s) are credited and that the original publication in this journal is cited, in accordance with accepted academic practice. No use, distribution or reproduction is permitted which does not comply with these terms.
*Correspondence: Jinbao Li, bGlqaW5iYW9zaGFuZ2hhaUAxNjMuY29t
†These authors have contributed equally to this work