- 1Department of Dermatology, Wuhan No. 1 Hospital, Tongji Medical College, Huazhong University of Science and Technology, Wuhan, China
- 2School of Materials Science and Engineering, Wuhan University of Technology, Wuhan, China
- 3Department of Hepatobiliary Surgery, Wuhan No. 1 Hospital, Tongji Medical College, Huazhong University of Science and Technology, Wuhan, China
Comprehensive cancer treatments have been widely studied. Traditional treatment methods (e.g., radiotherapy, chemotherapy), despite ablating tumors, inevitably damage normal cells and cause serious complications. Photodynamic therapy (PDT), with its low rate of trauma, accurate targeting, synergism, repeatability, has displayed great advantages in the treatment of tumors. In recent years, nanotech-based PDT has provided a new modality for cancer treatment. Direct modification of PSs by nanotechnology or the delivery of PSs by nanocarriers can improve their targeting, specificity, and PDT efficacy for tumors. In this review, we strive to provide the reader with a comprehensive overview, on various aspects of the types, characteristics, and research progress of photosensitizers and nanomaterials used in PDT. And the application progress and relative limitations of nanotech-PDT in non-melanoma skin cancer and melanoma are also summarized.
Introduction
Photodynamic therapy (PDT) is a non-invasive or minimally invasive treatment with verified safety, efficacy. In 2011, PDT has been listed as one of the primary methods for early esophageal cancer by NCCN in USA. Other tumors that have tried PDT strategy include gastric cancer, cholangiocarcinoma, squamous cell carcinoma on head and neck, early cervical cancer or precancerosis and urologic malignancies, and so on (1). At present, PDT is widely and maturely used in skin cancer and precancerous lesions with visual cosmetic effect.
PDT is based on photosensitizers (PSs), which intensely accumulate in pathological tissues, to produce cytotoxic substances under irradiation by light sources at a specific wavelength, and it acts on target tissues to kill pathological cells, induce microvascular injury, and stimulate immune responses. There are two types of photo-oxidative mechanism of PDT: PSs are converted from the singlet basic state into the excited singlet state after absorb the radiant energy, and then, partial energy direct PSs to the excited triplet state. On the one hand, PSs can transfer energy to the surrounding biomolecules, so reactive oxygen species (ROS) is further produced and tumor cells are destructed in this cascade of photodynamic reactions (Type I); on the other hand, PSs directly transfer energy to the ground state oxygen molecules, leading to the generation of singlet oxygen (1O2, Type II). Type II reaction is the most important process, as the amount of available oxygen decreases, Type I mechanism becomes significant (2). PDT has been mainly divided into tumor-targeted PDT, vascular-targeted PDT (VTP) and anti-microbial PDT. Most PDT are based on cellular-targeted photochemotherapy. VTP distinct from two other ways, and selectively disrupts vascular function by inducing oxidative damages to the vasculature, particularly endothelial cells, meanwhile, VTP-PSs remain predominantly confined within the circulation (3). Microorganisms thrive in well-organized biofilm ecosystems associated with infections. PDT overcomes biofilm producing pathogens by simultaneously acting on multiple target sites, such as damage to the cell membrane, DNA damage and protein/enzyme inactivation, and has been used against gram-negative and positive bacteria, fungi, viruses and parasites (4). In this paper, we reviewed the types, characteristics, and research progress of photosensitizers and nanomaterials used in PDT, and summarized the application outcomes of nanotech-PDT in non-melanoma skin cancer and melanoma.
Research Status of PSs
Since the 1980s, PDT has been systematically studied, a series of PSs and light sources have been developed. PS is a compound that can absorb photons and transfer energy to molecules, thereby promoting chemical reactions without participating directly in them (5).
Commonly, PSs are classified into three generations. First-generation PSs (PSs1) mainly include porphyrin compounds which characterized with low chemical purity, poor tissue penetration and selectivity, and long residence time in body limit their utilization.
The structures of second-generation PSs (PSs2) are clear compared with those of PSs1, and the photosensitizing activity, absorption spectra, and penetration to deeply located tissues of PSs2 have been greatly improved (2, 6). PSs2 include derivatives of porphyrins (e.g., 5-Aminolevulinic acid, 5-ALA), chlorin (e.g., Temoporfrin/m-THPC, bacteriochlorin derivative) and metallic phthalocyanines (Pcs). 5-ALA is the precursor of protoporphyrin IX (PpIX, with strong photosensitive activity), thus 5-ALA has widely been applied to port-wine stain, severe acne, warts, cSCC, and precancerous lesions (7). Compared with porphyrins, chlorins have lower phototoxicity, large molar extinction coefficient, higher yield of 1O2 formation, and the maximum absorption wavelength at 650-800 nm, which make the depth of tissue penetration is up to 1 cm. Researchers synthesized the alkylether analogs of chlorophylla derivatives, 2-[1-Hexyloxyethyl]-2-devinyl pyropheophorbide-a (HPPH) characterized by single chemical structure, long excitation wavelength, good photostability. Chen et al. developed a rat PBPK model of HPPH, and demonstrated that the appropriate time window to conduct light exposure for the treatment of digestive cancer and skin cancer were 24-48 h and 48-96 h, respectively (8).
Pcs, which are large conjugated systems consisting of four pyrrole units linked by four N-atoms, can be functionalized with various materials, and the complexes formed by Pcs and non-transition metals (Zn, Al, Si) have photobiological activity against tumors. Some Pcs exhibit strong absorption at 750–900 nm, the light transmission ratio of melanoma is significant in this range, and the absorption increased nearly twice as much as that of the 630nm wavelength. As reported previously, Pcs demonstrated better efficacy in PDT against melanoma in terms of tumor cell photokilling and decreased dark toxicity than porphyrins because of their stronger reactive oxygen species (ROS) generation and better spectroscopic properties (9, 10). Pcs have strong hydrophobicity and a tendency to aggregate, thereby reducing the efficacy of PDT, but PSs2 can be re-functionalized by core or peripheral modification to overcome these drawbacks. Scientists synthesized sugar-bound zinc Pcs (ZnPcs) and found that the aggregation of C-glycoside–modified Pcs in solution could be greatly reduced, thus improving the stability of PSs.
PSs3 are the products of PSs2 combined with functional molecules, including polypeptides, glycosylated compounds, and nanoparticles (NPs), as carriers to improve biocompatibility and targeting (11). The rapid proliferation of cancer cells requires a large number of carbohydrate compounds, and thus, glycosylated PSs inherently have enhanced targeting to cancer cells and better water solubility. Inactivating PSs are conjugated with a prodrug, such as a quenching agent or pH sensor, to activate the PSs to increase its specificity for cancer cells. Muttaqien et al. developed lower critical solution temperature polymers exerting pH-responsive phase transition for PDT. The PS-polymer conjugate exhibited significantly enhanced cellular uptake, and it augmented the efficacy of PDT in a tumor pH-selective manner (12, 13). Mechanism of action of PSs as presented in Figure 1.
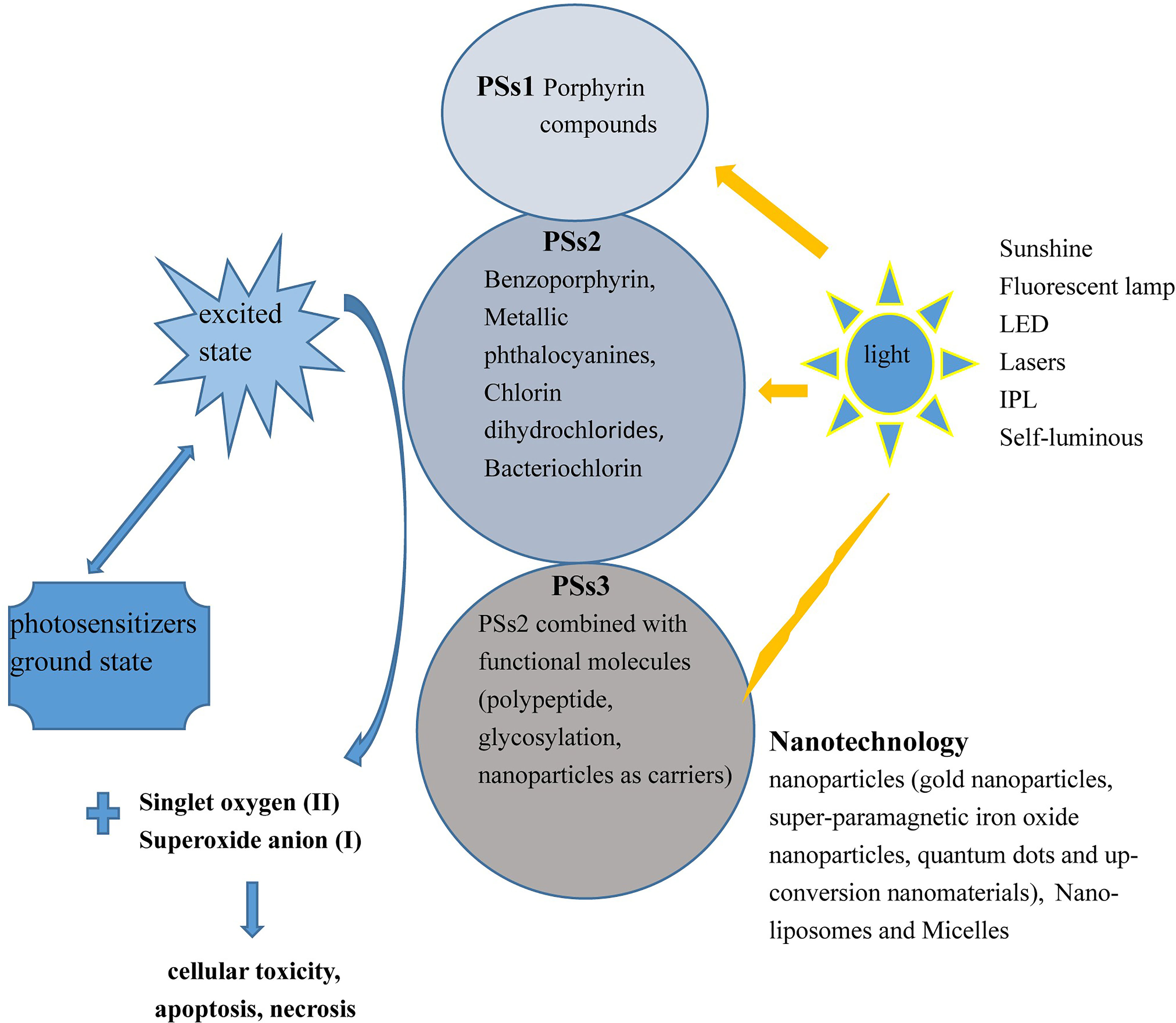
Figure 1 Action mechanism of PDT. First-, second-, and third-generation PSs absorb photons from different types of light sources and transfer energy (between the excited state and ground state) to generate 1O2 (II type) or superoxide anion (I type) to kill cells.
Nanotechnology
Polymerized PSs-NPs are the most widely studied PSs3, and they are usually produced via hydrophobic or electrostatic interactions between PSs and polymers, including polylactic acid, poly-D-lysine, polycaprolactone, and gelatin (14). The tumorous angiogenesis distinct from normal blood vessels in structure and morphology, with larger gaps between endothelial cells; and that, the lack of lymphatic in the tumors prevents lymphatic reflux, also known as enhanced permeability and retention (EPR) effect, consequently, NPs or other macromolecular substances can preferentially accumulate in tumor sites for a relative long time, thereby improving the efficiency of PDT and mitigating side effects in peripheral normal tissue.
PSs can be directly modified by nanotechnology or delivered by nanocarriers, such as NPs, nanoliposomes (LPs), and nanohydrogel particles. NPs include gold NPs (GNPs), super-paramagnetic iron oxide NPs (SPIONs), quantum dots (Qds), and upconverting nanomaterial, and so on (15).
NPs
NPs can be combined with PSs to obtain novel nano-PSs. Compared with conventional PSs, nano-PSs have many advantages, such as a small size, large specific surface area, high catalytic efficiency, a higher number of active centers, and strong adsorption capacity (16).
GNPs with good biocompatibility and hypotoxicity are generally used as passivating agents for SPIONs and carriers for PSs or anticarcinogen (17). GNPs modified by polyethylene glycol (PEG) have improved solubility and the ability to resist protein adsorption and avoid removal by the reticuloendothelial system, thereby prolonging the residence time of drugs in vivo. GNPs are divided into passive and active types according to whether they play a tumor-photosensitizing role in PDT. Passive GNPs, which are mainly used for drug transportation, have no substantial effects on the efficiency of PSs. Conversely, active GNPs can effectively absorb light energy to increase PS excitation and ROS production. The representative active GNPs are plasma active NPs, such as gold nanorods (AuNRs), shells, and cubes (18). Some GNPs have strong plasmonic resonance bands, which can effectively absorb near-infrared light and convert photons into heat energy, thus causing irreversible high-temperature ablation of cancer cells (19).
GNPs have been explored in various biological applications because of their chemical inertness and minimal toxicity. Li et al. (20) reported hexadecyl dimethylammonium bromide-coated AuNRs with positive charges that were bound to sulfonated aluminum Pcs (AlPcS) with negative charges to form AuNRs-AlPcS via electrostatic force. These constructs had 5-fold stronger fluorescence, and they caused typical light dose-dependent PDT-mediated damage in QGY liver cancer cells. This synergistic reaction simultaneously improved photodynamic detection and treatment efficacy.
Qds are powerful and versatile biological imaging probes. Qds possess wide light absorption bands, two-photon absorption cross-sections, and light resistance. The chromophores in biological system are quite complex. Photons with different energy densities may resonate with different chromophores to produce different electron transitions and photoluminescence (PL) reaction, so the tunable PL spectrum of Qds can match the requirements for diverse tumor tissues (21). However, Qds have lower ROS yields than traditional PSs, and some contain toxic cadmium, therefore, Qds and PSs are complementary modalities, and their advantages are magnified when they are used in combination. Samia et al. (22) proposed the concept of “Qds-PS for PDT”. Specifically, Qds as energy donors produce fluorescence resonance energy transfer (FRET) with traditional PSs as acceptors. The non-covalently linked Qds-Pc4 complexes were found to increase ROS production.
To date, a series of Qds-PS systems have been developed, such as Qds-porphyrins, Qds-Pcs, and Qds-organic or inorganic dyes. Qds with high quantum yield generally have poor water solubility, and thus, surface modification is required to improve their solubility. Karabanovas et al. (23) prepared CdSe/ZnS Qds loaded with chlorin e6 (Ce6) and coated with phospholipids, and the water solubility and colloidal stability of these compound were greatly improved.
PSs conjugated to water-soluble Qds result in FRET from Qds to PSs, thus improving the effect of PDT (24). Yueshu et al. (25)coordinated CuInS2/ZnS(CIS/ZnS) Qds with 5-ALA to form CIS/ZnS–5-ALA conjugates via chemical bonding. Their analysis revealed that the FRET efficiency of the conjugates was 58.49%, and the viability of cells in vitro was less than 40% after 800- and 1300-nm femtosecond excitation. Li et al. (26) reported the conjugation of AlPcSs with amine-dihydrolipoic acid-coated Qds via electrostatic binding, and compared with free AlPcSs, the AlPcS-Qd compounds had a greatly increased uptake rate in human nasopharyngeal carcinoma cells (KB cells) and achieved FRET with 84% efficiency.
SPIONs are magnetic particles with iron oxide NPs (mainly including Fe3O4 and Fe2O3) as their cores. The sizes of SPIONs have a linear relationship with their magnetization intensity indices (27). Studies illustrated that SPIONs with a crystal nucleus diameter < 10 nm exhibit superparamagnetism. However, the minimum pore size of microvessels in normal tissues is approximately 10 nm, and thus, an excessively small particle size may lead to drug leakage and affect the sedimentation rate. Oppositely, >200-nm particles in tissues were easily engulfed by the phagocytic system (28), therefore, an appropriate size is needed in the application of SPIONs. Magnetic NPs of 10–100 nm in size, which have the best stability and magnetism, can act as effective drug carriers (29). They have been successfully used for delivering porphyrins and non-porphyrin precursor drugs to targets during PDT, thereby promoting the development of multi-function SPION-PS conjugates (30).
To obtain better properties, SPIONs are often surface-modified and then further functionalized with a variety of functional groups (31), which mainly include synthetic polymers (e.g., PEG), neutral polymers (e.g., dextran, chitosan), inorganic metals, inorganic oxides (e.g., silica), and bioactive molecules (e.g., liposomes). Leyong et al. (32) developed multi-component nanocomplexes composed of SPIONs, upconverting NPs (UCNPs), and AlPcS4, which could kill 70% of MCF-7 cells under irradiation by a 980-nm laser. He et al. (33)synthesized Fe3O4@GNPs bound to hemagglutinin as bimodal contrast agents for imaging in colorectal cancer models. Wei et al. (34) constructed an integrin receptor-targeted cRGDfK-SPION nanomolecular probe via chemical methods to image HepG2 cells with strong αVβ3 integrin receptor expression, and the results indicated that cRGDfK-SPION significantly accumulated in HepG2 cells in vitro.
Silica-based mesoporous materials, which benefit from their special structure-ordered pore network, can induce promising controlled loading and release of PSs or drugs. Multifunctional nanocomposites conjugated with Pc4-magnetic NPs have been used as potential drugs for PDT application. Gauta and colleagues reported (35) ZnPc linked to folic acid and amine-functionalized magnetic NPs to form ZnPc-AMNPs-FA conjugates, and these constructs both localized at tumor sites with the aid of an external magnetic field and possessed the advantages of targeted PDT modes combined with MRI. Refilwe et al. (36) developed ZnTPPcQ-DNDs-BODIPY (conjugated with ZnPc, nanodiamond, and halogenated BODIPY) as PSs with higher 1O2 quantum yield in water solution by testing fluorescence lifetimes from time-correlated single photon counting methods, thus, the three-component nanoassembly worked better than the free individual components.
UCNPs can convert the near-infrared light (NIR) with strong tissue penetration into UV or visible light (VIS), providing a light converter for PDT activated by NIR, which is expected to solve the problem of shallow tissue penetration depth in traditional PDT. As a unique light conversion material, UCNPs have the strengths of low toxicity, a narrow emission band, long emission life, photobleaching resistance, non-autofluorescence background, and display great potential in biological imaging, biosensing, and drugs release control. In addition, UCNPs as carrier-loaded PSs can avoid the easy agglomeration of hydrophobic PSs. UCNPs can be effectively enriched in tumor sites by the EPR effect (37, 38).
SiO2 coating, self-assembly and covalent bonding are three common methods to construct UCNP-PDT system. Zhang et al. (39)constructed mesoporous silica-coated UCNPs that could convert deeply penetrating near-infrared to visible wavelengths. In this study, the composites loaded with two types of PSs (MC 540 and ZnPc) were designed to improve the ROS yield and enhance the PDT effects by using the polychromatic emission of UCNPs excited with 980-nm light source. Chen et al. (40)constructed complexes (UCNP@BSA-RB&IR825) coated with bovine serum albumin (BSA), which improved the solubility and stability of these complexes in the physiological environment. Emphatically, rose bengal (RB) and IR825 were simultaneously loaded in the BSA layer. Under NIR, these dual dye-loaded UCNPs were used for binary model imaging in vivo; meanwhile, photothermal therapy (PTT)/PDT synergistically ablated cancer cells. In UCNP-PDT system, UCNPs and PSs act as energy donor and acceptor, respectively. Ce6 and UCNP-NaYF4:Yb,Er@CaF2 were assembled into tumor pH-responsive composites, and after 980-nm excitation, NaYF4:Yb,Er@CaF2 produced upconversion fluorescence at 675 nm, which prompted Ce6 to produce ROS to selectively kill deep tumor tissues in a lower pH microenvironment (41). The proximity load and high content of PSs on UCNPs is the key to improve the energy transfer efficiency of UCNP-PDT.
LPs and Micelles
LPs are single or multi-lamellar nanosystems spontaneously formed by phospholipids dispersed in aqueous medium, and LPs accumulate passively in tumor cells via the EPR effect (42). Some LPs are biocompatible and biodegradable, and they can bind with a variety of hydrophilic and hydrophobic drugs. Among these moieties, PEGylated LPs exhibit longer blood circulation, and therefore, they have been widely investigated for drug delivery application (43).
Liposomal preparation can effectively prevent the aggregation of PSs, thereby enhancing their photoactivity. ICG green-loaded PSs that released the dye in response to NIR displayed greater ability to suppress tumor growth in a breast cancer model than the free dye (44). Scientists had prepared ICG-coated photothermal-sensitive LPs that damaged DNA via PDT-mediated ROS production and exerted strong inhibitory effect on triple-negative breast cancer cell lines (MDA-MB-468, HCC-1806) (45). The coupling of targeting moieties to the surface of LPs enabled LPs to selectively bind to specific tissues or cell types.
The efficiency of chemotherapy is restricted by the limited uptake of anti-cancer drugs in a bioavailable form. LPs can integrate PSs and drugs simultaneously to create co-delivery systems. It has been reported that doxorubicin (Dox)-loaded porphyrin-phospholipid LPs (Pop-LPs-Dox) had long circulation half-life and storage stability. Pop-LPs-Dox were injected into mouse subcutaneous pancreatic xenografts (3–7 mg/kg Dox) under near-infrared irradiation, and the results revealed 7-fold greater Dox accumulation in tumors (46). Because of rapid tumor growth and increased oxygen consumption, hypoxia microenvironment enormously restrict the efficiency of PDT. Shi et al. (47) encapsulated catalase, lyso-targeted NIR-PS, and Dox in LPs to form complexes that enhanced oxygenation in tumors and promoted 1O2 production through the catalysis of intratumoral H2O2, thus significantly inducing apoptosis in tumor cells.
Micelles are self-assembled nanoscale colloidal particles with a hydrophobic core and hydrophilic shell. PS-loaded polymeric micelles are characterized by a long bleeding cycle time and high accumulation in diseased tissues. Many PSs can be used in combination with LPs or micelles for PDT (48). Wu et al. (49)confirmed that porphyrin- and galactosyl-conjugated polymer micelles had better targeting and PDT efficiency in HepG2 cells. Elbayoumi et al. (50) investigated that tetraphenylporphyrin (TPP)-loaded PEG-PE micelles had greater cytotoxicity than TPP alone and better targeting in MCF-7 cells. Deng et al. (51)constructed PEGylated iridium-based nanomicelles (IP600−IP4000 NPs) and found that the micellar stability increased with increasing PEG length and cellular uptake decreased with increasing PEG length. IP2000 and IP4000 NPs had excellent biocompatibility, and further analysis revealed that IP2000 NPs displayed higher therapeutic efficacy in PDT.
Micelles carrying PSs may cause phototoxicity during blood circulation, such as damage to endothelial cells and adjacent vascular cells; therefore, it is necessary to diminish side effects in the untargeted region (52). Li et al. (53) designed PEG-b-poly (caprolactone) micelles containing pheophorbide A (PhA) as the PS and the antioxidant β-carotene (CAR) via simple physical incorporation. CAR removed 1O2 produced by PhA in blood circulation and reduced the phototoxicity. When the micelles were ingested by tumor cells, the spatial isolation of PhA and CAR hindered the scavenging of 1O2, resulting in remarkable tumor cell death. This strategy ameliorated the accuracy and safety of PDT in cancer treatment.
Regarding the advantages and drawbacks of nanomaterials, GNPs are easy to prepare, but their removal is slow for their poor biocompatibility. Qds can permit combined labeling, detection, and treatment, but some Qds contain toxic heavy metals. UCNPs are limited to in vitro use because of their weak drug loading and low upconversion efficiency. Micelles are commonly used to deliver hydrophobic drugs, but their stability in vivo needs to be improved.
Application of Nano-PDT in Skin Cancer
On the basis of pathological findings, squamous cell carcinoma (SCC), basal cell carcinoma (BCC), and malignant melanoma (MM) are among the most common skin cancers. The rest of skin cancer, such as Paget’s disease, sarcoma, and cutaneous T/B-cell lymphoma, are relatively rare. According to the origin of cells, skin cancer can also be divided into two types: MM and non-melanoma skin cancer (NMSC). Epidemiological analyses revealed an estimated 300 skin cancer cases per 100,000 people worldwide in 2018 (54). Skin cancer is common in Caucasians because of environmental pollution and other adverse factors, but the incidence of skin cancer in China is also increasing annually. Cutaneous SCC (cSCC) accounts for approximately 20% of skin tumors (55). Although primary cSCC can be successfully treated with surgery, whereas, 5% of patients with cSCC develop metastasis or local recurrence after complete resection.
The incidence of MM is increasing rapidly. Each year, there are an estimated 280,000 new cases of MM and more than 60,000 deaths worldwide. Globally, there are significant differences in the morbidity and mortality of MM, and these differences are mainly related to the timing of its detection and treatment (56). Traditional treatments for MM include surgery, chemotherapy, and radiotherapy. In recent years, targeted therapy and immunotherapy have made significant progress in extending survival and improving the quality of life of patients. Topical therapy can enhance the local drug concentration in diseased regions, meanwhile it has less potential toxicity than systemic drug therapy, making it an effective supplement to systemic therapy.
In 2010, Norwegian Photodynamic Therapy Group and medical specialists presented guidelines for the practical use of topical PDT in NMSC (57). PSs can preferentially aggregate in lesions and produce specific biological effects. This important property gives PDT great promise for the targeted therapy in skin cancer. PSs are delivered to patients topically, orally or intravenously.
5-ALA and methyl aminolevulinate (MAL, increased lipid solubility compared with ALA) have been approved by the FDA for dermatologic indications, but as prodrugs, they must be converted into active PpIX, which preferentially localize in endoplasmic reticulum to induce cell death upon irradiation. The major absorption peak of PpIX occurs in the blue light range at 410-420 nm, but other wavelengths with smaller absorption peaks can also be used to activate 5-ALA. A blue light source, potassium titanyl phosphate laser, pulsed dye laser, and intense pulsed light have broad applications for PDT. Red light with a wavelength of 630 nm (e.g., helium-neon laser) also served as a light source in some investigations (58). Generally, the depth of light penetration into skin increases at longer wavelengths, and thus, red light and NIR are more effective for thicker lesions.
The optimization of PDT depends on appropriate light parameters, oxygen, the kinetic distribution and concentration of PSs in tissues, but the low skin penetration of 5-ALA limits the efficacy of PDT. Champeau et al. (59) reviewed chemical (containing nanotechnology) and physical strategies for improving PSs/drugs penetration and PpIX metabolism in skin cancer.
Skin Barriers and Drug Penetration
Methods for enhancing topical drug penetration include changing the thickness of the stratum corneum and chemical absorption of skin barriers, and among these strategies, ablative fractional laser (AFL) can create microchannels in the skin surface to facilitate PS uptake. Scientists used three groups of far-infrared wavelength lasers (CO2 laser with 10,600 nm wavelength, Er : YAG laser with 2940 nm, and Er : YSSG laser with 2790 nm) to create vertical micropores in the epidermis to increase drug delivery via AFL; meanwhile, the depth of the micropores could be controlled by the laser energy density. Anderson’s group conducted a randomized clinical trial (60) using AFXL (10 mJ per pulse, 0.12 mm spot, 5% density; UltraPulse-DeepFx, Lumenis Inc.) combined with MAL cream to treat 212 patients with actinic keratosis (AK, severity grade I–III). After 3 months of follow-up, AFXL-PDT was significantly more effective than PDT for all AK grades. According to the theory of fractioinal photothermolysis, AFL equipped with a picture generator, which disperse the concentrated spots into dozens to hundreds of smaller focal spots, can vaporize the target tissues in matrix mode, so that the skin tissues between heat damage areas are not affected, simultaneously promote skin healing. Although AFL improved the permeability of PSs, they have some drawbacks, such as secondary skin infection, inflammatory hyper/hypopigmentation, and scarring (61).
Microneedles (MNs) with different materials and shapes can improve cutaneous delivery. Moreover, MNs shorter than 900 μm in length do not damage deep blood vessels and nerves, leading to low risks and minimal pain (62). Wu and co-workers constructed an analgesic MN patch that features dissolvable MNs to transdermally deliver calcitonin gene-related peptide antagonist to treat localized neuropathic pain. The study demonstrated that AMN patches did not cause skin irritation or systemic side effects (63). A randomized clinical trial confirmed that expedited MN-assisted PDT for the in situ treatment of AK significantly shortened the incubation time to 20 min achieved 76% clearance, similar to the findings for conventional 1-h 5-ALA incubation (64).
In addition to AFL and MNs, microdermabrasion, curettage, and keratolytics as physical methods can also promote the permeation of PSs/drugs into skin by reducing the thickness of the stratum corneum.
Extensive studies have been conducted in the past 20 years to explore the superiority of 5-ALA PDT and MAL PDT with different light sources in the in situ treatment of AK and cSCC compared with other topical therapy modalities, such as 5-FU, imiquimod, and cryotherapy (65). PDT is recommended to treat superficial and thin nodular BCC (thickness < 2mm), and the combination of superficial debridement can appropriately extend the depth of PDT (66). Roozeboom et al. (67) compared the long-term effectiveness of fractionated 20% 5-ALA PDT with prior partial debulking versus surgical excision in nBCC. The result revealed that the recurrence rate was higher in the 5-ALA PDT group than in the surgery group.
MAL exhibits lipotropic effects as well as better selectivity and deeper skin penetration than 5-ALA. Clinical trials (68)demonstrated the efficacy of MAL PDT (red light is preferred as the excitation light) in the treatment of severe acne, non-hyperkeratotic AK on the face and scalp, Bowen’s disease, and NMSCs such as BCC that are not suitable for conventional surgery. Rhodes et al. (69) confirmed that MAL PDT is not inferior to surgery for nBCC after 3 and 24 months of follow-up. Szeimies et al. (70) reached the same conclusion after 3 months of follow-up, but the recurrence rate was significantly higher in the MAL PDT group than in the surgery group at 12 months.
Prodrugs With Enhancing Strategies
PpIX has strong fluorescence and photosensitizing activity, and some tumors exhibit elevated 5-ALA–mediated PpIX levels; thus, increasing PpIX levels can improve the effects of PDT against tumors. Xue et al. (71) summarized the strategies for enhancing 5-ALA–based tumor detection and PDT. Iron chelators can remove ferrous iron and prevent the conversion of PpIX into heme, thus increasing PpIX accumulation.
Some differentiation agents, such as methotrexate and vitamin D, can increase 5-ALA–mediated PpIX production and improve 5-ALA PDT outcomes by upregulating CPOX. Anand et al. (72) found that using oral vitamin D3 prior to PDT, which increased PpIX levels by 3–4-fold, enhanced PDT-mediated cell death by 20-fold in cSCC without inducing unfavorable phototoxicity in normal tissues. PpIX is an endogenous substrate of the ABCG2 transporter. Sun et al. (73) confirmed that gefitinib (a potent ABCG2 inhibitor) could inhibit ABCG2-mediated PpIX efflux from malignant brain tumor cells, thereby increasing intracellular PpIX levels and the effect of PDT, as presented in Figure 2.
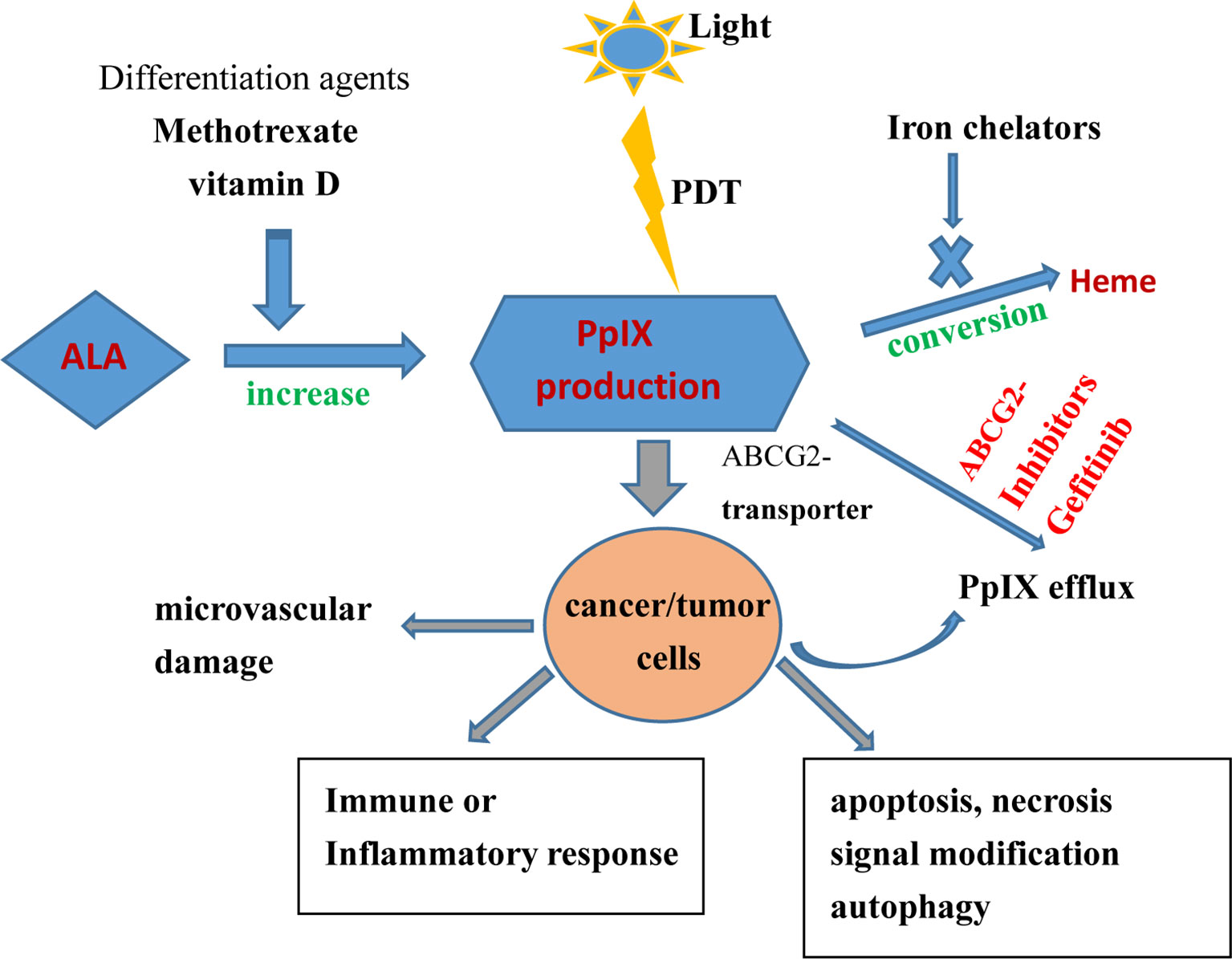
Figure 2 Effects of topical PDT on cancer/tumor cells and therapeutic strategies for enhancing ALA-based tumor therapy (increasing PpIX synthesis, reducing PpIX conversion, and inhibiting PpIX efflux).
Collier and Rhodes (74) provided an outline of knowledge based on an analysis of randomized controlled trials of topical PDT in BCC, introduced the mechanism of action of topical PDT, described the use 5-ALA/MAL as prodrugs, and discussed the development of strategies for enhancing penetration and optimizing PpIX accumulation (e.g., epigallocatechin-3-gallate, iron chelators). The correlation between BCC subtypes and the effect of PDT (superficial low-risk BCC displayed higher complete response rates to topical PDT than other subtypes of BCC) was revealed, and a comparison of topical PDT with other topical methods (e.g., surgical excision, cryosurgery, topical 5-FU, imiquimod) was reported.
Nanotechnology in PDT for NMSC Treatment
Due to the limited skin penetration and inferior luminescence efficiency of 5-ALA/MAL, microvehicles and nanodrug delivery systems such as NPs, LPs, and micelles have been developed (Table 1).
Studies reported that LPs increase the penetration of 5-ALA into skin relative to free 5-ALA and limited drug absorption into systemic circulation, thus reducing cytotoxicity and phototoxicity after treatment (75). Researchers assessed the possible toxicities of a liposomal formulation of PS (m-THPC) in feline cSCC. All cats responded to therapy (0.15 mg m-THPC/kg injection into the cephalic or femoral vein), and overall 1-year control rate was 75%. This outcome illustrated that liposomal PS was safety and efficacy (76). Scientists (77) investigated the long-term outcomes and prognostic factors of feline cSCC following the intravenous injection of liposomal phosphorylated mTHPC under irradiation with a 652-nm diode laser, and the overall response rate was 84% (complete remission, 61%; partial remission, 22%) with a mean progression-free interval of 35 months. Woźniak et al. (78) analyzed the photosensitizing effect of curcumin-loaded LPs acting in MUG-Mel2 (melanoma), SCC-25 (SCC), and HaCaT (normal keratinocytes) cells in vitro, revealing that the liposomal formulation improved the photosensitizing properties of curcumin-mediated PDT in skin cancers and reduced toxicity in normal keratinocytes. Alyssa et al. (79) reported that a nanoformulation of EGFR-targeted Pc4 packaged with polymeric micelles underwent faster and more extensive uptake in EGFR-overexpressing H&N SCC-15 cells, subsequently, they tested the PDT response of the Pc4 nanoformulation in subcutaneous SCC-15 xenografts in mice, and found that the higher level of Pc4 intra-tumoral uptake corresponded to the cell-study, thus improving PDT efficacy in vitro and in vivo.
PDT is limited by the sample size and tumor depth. For example, cSCC limited to the papillary dermis can be treated by PDT. To overcome these hurdles, pretreatment with AFLs or MNs and the encapsulation of PSs by LPs or other nanoplatforms have been attempted with remarkable efficacy (80). NPs have also been used as PDT enhancers because nanocarriers can passively accumulate at tumor sites via the EPR effect. Wang et al. (81)prepared 5-ALA–loaded polylactic-co-glycolic acid (PLGA) NPs as a cream formulation for application onto tumor surfaces in combination with helium–neon laser irradiation, finding that 5-ALA–PLGA NPs mediated PDT more effectively than free 5-ALA at the same concentration in cSCC. Loaded ZnPcs on chitosan/methoxy polyethylene glycol-polylactic acid (CPP) was used to form NP-(Z-CPP), and the dark toxicity and efficacy of topical PDT in SCC was examined using in vivo and in vitro tests. The results demonstrated that the compounds exhibited no dark toxicity, and the efficacy of PDT was significantly better using Z-CPP than using free ZnPcs (82).
PDT is an established treatment for low-risk BCC, mainly including primary nodular and superficial BCC. A randomized phase III trial evaluated the non-inferiority of BF-200 5-ALA (nanoemulsion gel containing 5-ALA) to MAL (cream containing MAL) in the treatment of sBCC or nBCC via PDT. The complete response rate in the BF-200 ALA group was 93.4%, compared with 91.8% in the MAL group, and BF-200 ALA-PDT proved non-inferior to MAL-PDT (83). Salmivuori et al. (84)conducted a prospective and double-blinded trial and confirmed that low-concentration hexyl aminolevulinate and BF-200 5-ALA had similar efficacy and tolerability as MAL in PDT for non-aggressive BCC.
PDT Based on Nanotechnology for Melanoma
Melanoma is an invasive and aggressive skin cancer, and can be classified in pigmented and unpigmented-type. In 2019, The European Interdisciplinary Guideline on melanoma made the recommendations on cutaneous melanoma diagnosis and treatment, and confirmed the primary treatment of melanoma is surgical excision, PDT hasn’t been mentioned in adjuvant therapy (85). However, PDT has become an effective treatment for superficial and localized cutaneous MM in some published literatures.
Tumor cells can induce the formation of their own defense system to some extent, and form an antioxidant defense system under the oxidative stress triggered by PDT. Nano-PSs have the advantages of high ROS yield, easy modification and good stability, and can overcome PDT resistance. Tang et al. observed that NPs could kill tumor cells directly via oxidative stress, DNA damage, and cell membrane damage in MM and demonstrated that they bind chemotherapeutic drugs or nucleotide fragments as carriers via electrostatic force or hydrophilic/hydrophobic interactions to enhance the biocompatibility and targeting of nanodrugs (86). Small interfering RNA (siRNA) can block gene expression to achieve high gene silencing for tumor treatment, but siRNAs are not stable, permitting their easy degradation in blood. Ragelle et al. (87)constructed PEGylated chitosan-based NPs to deliver siRNAs and confirmed that these combined NPs achieved high gene silencing, low cytotoxicity, and high stability in B16 melanoma cells expressing luciferase.
Monolayer cell culture models in vitro do not predict the efficiency of in vivo clinical studies, and in fact melanoma cells establish interactions with their neighbors in a three-dimensional (3D) space. 3D culture models (melanoma spheroids) recreate tumor heterogeneity by reconstructing natural gradients such as hypoxic environment (88), however, there are only a few reports of PDT on melanoma spheroids mimic tumor architecture.
PDT is less effective against pigmented melanoma than against NMSC because the main function of melanin synthesized in melanocytes is to protect against UV-induced damage, which prevents light from efficiently reaching the targets. Naidoo et al. (89) reported that PSs including verteporfin, halogenated porphyrins, 5-ALA, ruthenium porphyrins, and Pcs have been applied for PDT in MM. Cai et al. (90) found that 5-ALA PDT could significantly inhibit the survival of MM-A375 and A431 cells in a concentration-dependent and time-dependent manner, and the mechanism of action was related to the downregulation of Bcl-2 and upregulation of Bax and cleaved-PARP.
The incorporation of antibodies or targeting molecules to NPs improved passive or active PS delivery to target tumor cells. Functionalized NP platforms are beneficial for enhancing PS drug delivery, as presented in Figure 3.
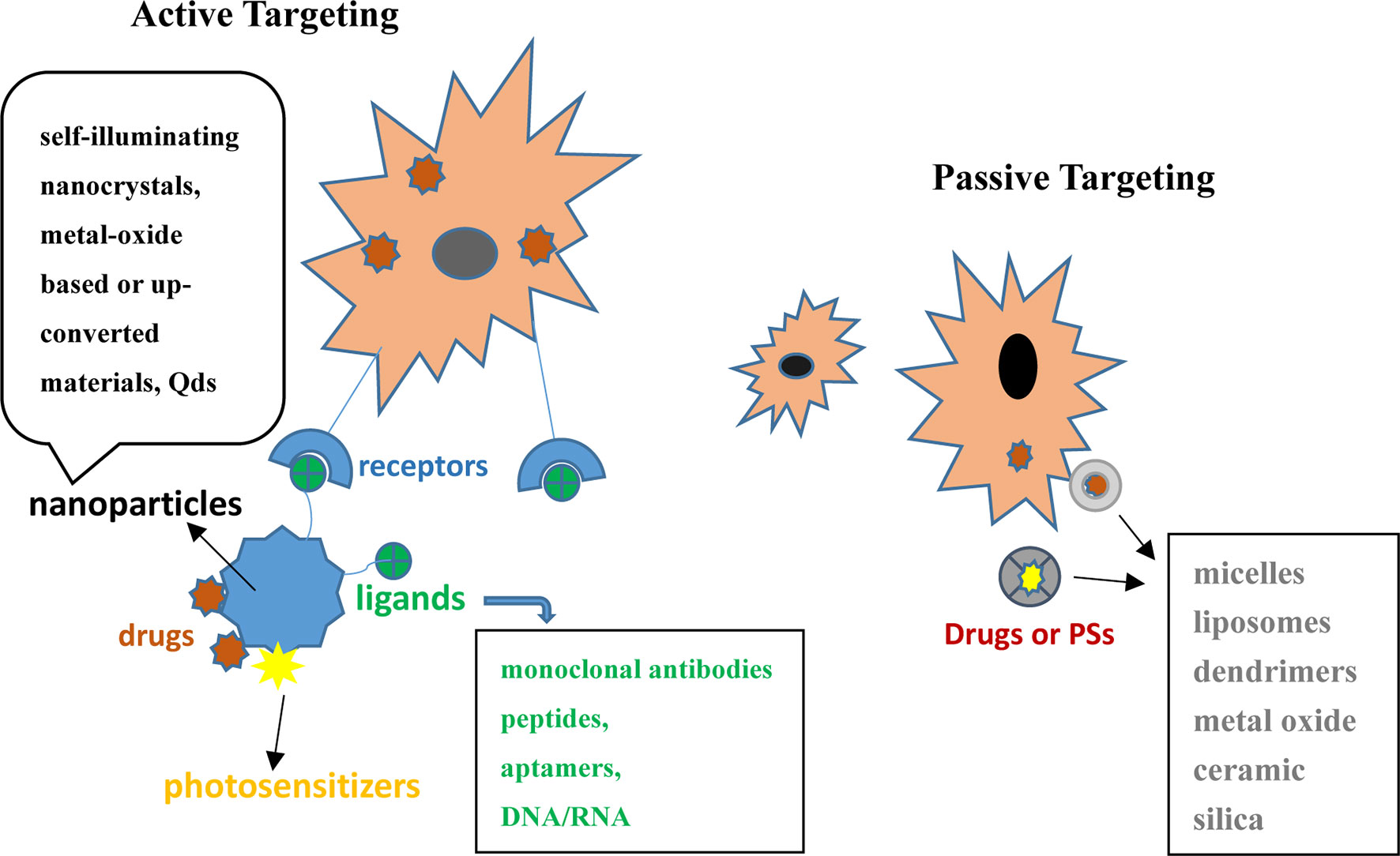
Figure 3 Active and passive forms of PSs or chemotherapeutics in combination with nanocarriers in PDT for skin cancer.
Nanocarriers can be divided into two types based on whether they are absorbed passively or actively. Passive drug delivery systems included micelles, LPs, dendrimers, metal oxide, ceramic, and silica (91).
Although the pigmentation of melanoma reduces the efficacy of PDT, researchers (92) used phenylthiourea (a melanin synthesis inhibitor) to inhibit melanin synthesis and obtain de-pigmented melanoma cells, and these cells were then co-incubated with LPs encapsulating sodium ferrous chlorophyllin (Fe-CHL). Transmission electron microscopy revealed an increase in the cellular uptake, and the compounds mainly accumulated in mitochondria and nuclei, thus increasing the efficacy of Fe-CHL–mediated PDT. Lee et al. (93) demonstrated that chitosan-coated LPs improved the stability and permeation of ICG, increased the cellular uptake of agent, and enhanced the efficacy of topical PDT in B16-F10 melanoma cells. Polish scientists designed a co-delivery system combining IR-768 with daunorubicin via mPEG-b-PLGA micelles, and the dual drug-loaded delivery system enhanced 1O2 generation during PDT in A375 cells (94). Chen et al. (95) encapsulated palladium porphyrin (PdTCPP) in layered metal oxide NPs (LDH), which improved the solubility of hydrophobic PS-PdTCPP and increased the biocompatibility and stability of the nanocomposites. Because LDH had higher loading capability, the surface could be modified with different functional groups. PdTCPP-LDH provided excellent anti-cancer efficacy during PDT in B16F10 cells.
NPs can be functionalized with targeting molecules that can selectively bind to receptors overexpressed on tumor cells, leading to enhanced PS/drug uptake. Monoclonal antibodies, peptides, aptamers, DNA/RNA, and other targeting molecules have been used for active targeting in PDT. In this process, modalities such as Qds, self-illuminating nanocrystals, and metal oxide-based or upconverting material have been selected as nanocarriers (96).
The peak absorption of melanin pigment is around 335 nm, and absorption is almost completely attenuated at the wavelengths longer than 700 nm. GNPs irradiated with NIR, tunable optics and photothermal properties can exert synergistic effects with PSs in PDT. Researchers assessed the dual efficacy of PDT/PTT using complexes of ZnPc attached to AuNRs in vitro with B16F10 melanotic cells and B16G4F amelanotic cells under irradiation at 635 nm. The results indicated that the photodynamic activity and photothermal effect could eliminate more than 90% of melanoma cells (97). Campu et al. (98)designed a near-infrared irradiation-responsive dual PTT/PDT therapeutic nanosystem featuring gold nanobipyramids (AuBPs) loaded with ICG. When the nanosystem was simulated by NIR, the 1O2 yield was doubled and the PTT effect was strongly increased compared with the findings for AuBPs alone. Urszula et al. (99) designed xanthene-originated RB co-encapsulated with upconverting NaYF4 NPs co-doped with lanthanide ions (Er3+ [2%] and Yb3+ [20%]) and then engineered them with PLGA and non-ionic surfactants (Span 80 and Cremophor A25). The outcome indicated that the double-core nanoplatform for the co-delivery of hybrid fluorophores had excellent selectivity, biocompatibility, and PDT effects in human melanoma cells (Me-45 and MeWo).
Summary
Thus far, numerous studies have investigated the combined efficacy of PSs with nanomaterials in PDT. Although the actual targeted transport efficiency of conventional PSs in vivo is hindered because of complex conjugated structures, poor water solubility and variational biological environment (e.g.pH, oxygen, nutrients), nano-PSs strategies open new ways for efficient PDT. Some local and superficial skin cancers and precancerosis are absolute indications for nanotech-mediated PDT. Furthermore, nanocarriers can better control drug release and significantly improve ROS-generating ability.
PDT still faces many challenges in clinical application. The excitation wavelength of PSs is mainly concentrated in UV or VIS, but biological tissues have strong absorption and scattering effect on light in the wavelength range, resulting in weak penetration ability, which fail to be directly applied in deep tumors or large solid tumors. NIR with preeminent penetration depth at 700-1000 nm, is an ideal PDT excitation light. Unfortunately, compared with UV-VIS, the molar absorption coefficient of PSs with the absorption peak in NIR is usually small, so one-photon excitation (OPE) PDT effect is non-ideal under NIR excitation with a safe radiation dose. Two-photon excitation (TPE) PDT has excellent spatial selectivity, and can achieve accurate target positioning, however, TPE-PDT PSs mainly occurs in the focal region, with small two-photon absorption cross section, thus resulting in low efficiency of TPE PDT, even using high-energy NIR technique, PDT efficacy cannot be improved significantly. Compared with TPE, the upconversion process does not need a high-power laser (98), and conventional continuous lasers can make UCNPs emit short-wavelength light, so as to reduce tissues damage caused by high-power light sources (100), but the photoquantum yield of UCNPs is relatively low. Weighing the pros and cons of various nanomaterials, it is crucial that constructing the ideal PSs compounds with the characteristics including high degree of chemical purity and stability, easy solubility in the tissues, high photochemical reactivity and photosensitive effect, and the maximum absorption of light should be at wavelengths 600 nm-800 nm, excellent selectivity and minimal cytotoxicity.
Due to oxygen consumption and microvascular damage, the anti-tumor efficacy of PDT is limited by tumorous hypoxic state. Scholars have designed a variety of nanodelivery systems to enhance the blood oxygen content of tumor sites, including direct oxygen delivery (by hemoglobin or perfluorocarbons NPs), catalytic oxygen production strategies (catalase oxygen-producing nanodelivery system), and so on. Direct oxygen delivery strategy and enzyme-catalyzed oxygen production strategy are affected by delivery efficiency and substrate concentration (101), in order to further improve the efficacy of oxygen-enhanced PDT, researchers used responsive materials to react with water in vivo to generate oxygen, which can effectively improve hypoxic internal environment. PDT has become one of the most important clinical therapies in dermatology. It is believed that with the development and integration of nanomedicine, biology and optics, more and more dermatological patients will benefit from PDT.
Author Contributions
ZP and DL helped draft the manuscript. All authors contributed to the article and approved the submitted version.
Funding
This work was supported by the National Natural Science Foundation of China (No. 81803157).
Conflict of Interest
The authors declare that the research was conducted in the absence of any commercial or financial relationships that could be construed as a potential conflict of interest.
Publisher’s Note
All claims expressed in this article are solely those of the authors and do not necessarily represent those of their affiliated organizations, or those of the publisher, the editors and the reviewers. Any product that may be evaluated in this article, or claim that may be made by its manufacturer, is not guaranteed or endorsed by the publisher.
Abbreviations
NPs, nanoparticles; EPR, enhanced permeability and retention effect; LPs, liposomes; SPIONs, super-paramagnetic iron oxide NPs; Qds, quantum dots; PEG, polyethylene glycol; AuNRs, gold nanorods; AlPcS, aluminum Pcs; PL, photoluminescence; FRET, fluorescence resonance energy transfer; Ce6, chlorin e6; UCNPs, upconverting NPs; NIR, near-infrared light; PTT, photothermal therapy; Dox, doxorubicin; TPP, tetraphenylporphyrin; PhA, pheophorbide A; CAR, carotene; SCC, squamous cell carcinoma; cSCC, Cutaneous SCC; BCC, basal cell carcinoma; MM, metastatic melanoma; NMSC, non-melanoma skin cancer; PpIX, protoporphyrin IX; AFL, ablative fractional laser; MAL, aminolevulinate.
References
1. Donohoe C, Senge MO, Arnaut LG, Gomes-da-Silva LC. Cell Death in Photodynamic Therapy: From Oxidative Stress to Anti-Tumor Immunity. Biochim Biophys Acta Rev Cancer (2019) 1872:188308. doi: 10.1016/j.bbcan.2019.07.003
2. Kwiatkowski S, Knap B, Przystupski D, Saczko J, Kedzierska E, Knap-Czop K, et al. Photodynamic Therapy - Mechanisms, Photosensitizers and Combinations. BioMed Pharmacother (2018) 106:1098–107. doi: 10.1016/j.biopha.2018.07.049
3. Nogueira L, Tracey AT, Alvim R, Reisz P, Scherz A, Coleman JA, et al. Developments in Vascular-Targeted Photodynamic Therapy for Urologic Malignancies. Molecules (2020) 25. doi: 10.3390/molecules25225417
4. Warrier A, Mazumder N, Prabhu S, Satyamoorthy K, Murali TS. Photodynamic Therapy to Control Microbial Biofilms. Photodiagnosis Photodyn Ther (2021) 33:102090. doi: 10.1016/j.pdpdt.2020.102090
5. Wilson BC. Photodynamic Therapy for Cancer: Principles. Can J Gastroenterol (2002) 16:393–6. doi: 10.1155/2002/743109
6. Dobson J, de Queiroz GF, Golding JP. Photodynamic Therapy and Diagnosis: Principles and Comparative Aspects. Vet J (2018) 233:8–18. doi: 10.1016/j.tvjl.2017.11.012
7. Wen X, Li Y, Hamblin MR. Photodynamic Therapy in Dermatology Beyond non-Melanoma Cancer: An Update. Photodiagnosis Photodyn Ther (2017) 19:140–52. doi: 10.1016/j.pdpdt.2017.06.010
8. Chen L, Zhang X, Cao Q, Wu Y, Zhang T, Tong H, et al. Development and Application of a Physiologically Based Pharmacokinetic Model for HPPH in Rats and Extrapolate to Humans. Eur J Pharm Sci (2019) 129:68–78. doi: 10.1016/j.ejps.2018.12.014
9. Almeida EDP, Dipieri LV, Rossetti FC, Marchetti JM, Bentley M, Nunes RS, et al. Skin Permeation, Biocompatibility and Antitumor Effect of Chloroaluminum Phthalocyanine Associated to Oleic Acid in Lipid Nanoparticles. Photodiagnosis Photodyn Ther (2018) 24:262–73. doi: 10.1016/j.pdpdt.2018.10.002
10. Li K, Qiu L, Liu Q, Lv G, Zhao X, Wang S, et al. Conjugate of Biotin With Silicon(IV) Phthalocyanine for Tumor-Targeting Photodynamic Therapy. J Photochem Photobiol B (2017) 174:243–50. doi: 10.1016/j.jphotobiol.2017.08.003
11. Ma X, Qu Q, Zhao Y. Targeted Delivery of 5-Aminolevulinic Acid by Multifunctional Hollow Mesoporous Silica Nanoparticles for Photodynamic Skin Cancer Therapy. ACS Appl Mater Interfaces (2015) 7:10671–6. doi: 10.1021/acsami.5b03087
12. Muttaqien SE, Nomoto T, Dou X, Takemoto H, Matsui M, Nishiyama N. Photodynamic Therapy Using LCST Polymers Exerting Ph-Responsive Isothermal Phase Transition. J Control Release (2020) 328:608–16. doi: 10.1016/j.jconrel.2020.09.036
13. Zhao B, Wang M, Wang X, Yu P, Wang N, Li F. Synthesis and Characterization of Novel Porphyrin-Cinnamic Acid Conjugates. Spectrochim Acta A Mol Biomol Spectrosc (2019) 223:117314. doi: 10.1016/j.saa.2019.117314
14. Avci P, Erdem SS, Hamblin MR. Photodynamic Therapy: One Step Ahead With Self-Assembled Nanoparticles. J BioMed Nanotechnol (2014) 10:1937–52. doi: 10.1166/jbn.2014.1953
15. Yang Z, Ma Y, Zhao H, Yuan Y, Kim BYS. Nanotechnology Platforms for Cancer Immunotherapy. Wiley Interdiscip Rev Nanomed Nanobiotechnol (2020) 12:e1590. doi: 10.1002/wnan.1590
16. Mishra S. Nanotechnology in Medicine. Indian Heart J (2016) 68:437–9. doi: 10.1016/j.ihj.2016.05.003
17. Fakayode OJ, Tsolekile N, Songca SP, Oluwafemi OS. Applications of Functionalized Nanomaterials in Photodynamic Therapy. Biophys Rev (2018) 10:49–67. doi: 10.1007/s12551-017-0383-2
18. Gamaleia NF, Shton IO. Gold Mining for PDT: Great Expectations From Tiny Nanoparticles. Photodiagnosis Photodyn Ther (2015) 12:221–31. doi: 10.1016/j.pdpdt.2015.03.002
19. Norouzi H, Khoshgard K, Akbarzadeh F. In Vitro Outlook of Gold Nanoparticles in Photo-Thermal Therapy: A Literature Review. Lasers Med Sci (2018) 33:917–26. doi: 10.1007/s10103-018-2467-z
20. Li L, Chen JY, Wu X, Wang PN, Peng Q. Plasmonic Gold Nanorods can Carry Sulfonated Aluminum Phthalocyanine to Improve Photodynamic Detection and Therapy of Cancers. J Phys Chem B (2010) 114:17194–200. doi: 10.1021/jp109363n
21. Skripka A, Dapkute D, Valanciunaite J, Karabanovas V, Rotomskis R. Impact of Quantum Dot Surface on Complex Formation With Chlorin E(6) and Photodynamic Therapy. Nanomaterials (Basel) (2018) 9. doi: 10.3390/nano9010009
22. Samia AC, Chen X, Burda C. Semiconductor Quantum Dots for Photodynamic Therapy. J Am Chem Soc (2003) 125:15736–7. doi: 10.1021/ja0386905
23. Karabanovas V, Skripka A, Valanciunaite J, Kubiliute R, Poderys V, Rotomskis R. Formation of Self-Assembled Quantum Dot–Chlorin E6 Complex: Influence of Nanoparticles Phospholipid Coating. J Nanoparticle Res (2014) 16. doi: 10.1007/s11051-014-2508-x
24. Yaghini E, Giuntini F, Eggleston IM, Suhling K, Seifalian AM, MacRobert AJ. Fluorescence Lifetime Imaging and FRET-Induced Intracellular Redistribution of Tat-Conjugated Quantum Dot Nanoparticles Through Interaction With a Phthalocyanine Photosensitiser. Small (2014) 10:782–92. doi: 10.1002/smll.201301459
25. Feng Y, Liu L, Hu S, Liu Y, Ren Y, Zhang X. Förster Resonance Energy Transfer Properties of a New Type of Near-Infrared Excitation PDT Photosensitizer: Cuins2/Zns Quantum Dots-5-Aminolevulinic Acid Conjugates. RSC Adv (2016) 6:55568–76. doi: 10.1039/C6RA06937A
26. Li L, Zhao JF, Won N, Jin H, Kim S, Chen JY. Quantum Dot-Aluminum Phthalocyanine Conjugates Perform Photodynamic Reactions to Kill Cancer Cells via Fluorescence Resonance Energy Transfer. Nanoscale Res Lett (2012) 7:386. doi: 10.1186/1556-276X-7-386
27. Mok H, Zhang M. Superparamagnetic Iron Oxide Nanoparticle-Based Delivery Systems for Biotherapeutics. Expert Opin Drug Deliv (2013) 10:73–87. doi: 10.1517/17425247.2013.747507
28. Hayashi K, Nakamura M, Sakamoto W, Yogo T, Miki H, Ozaki S, et al. Superparamagnetic Nanoparticle Clusters for Cancer Theranostics Combining Magnetic Resonance Imaging and Hyperthermia Treatment. Theranostics (2013) 3:366–76. doi: 10.7150/thno.5860
29. Gupta AK, Gupta M. Synthesis and Surface Engineering of Iron Oxide Nanoparticles for Biomedical Applications. Biomaterials (2005) 26:3995–4021. doi: 10.1016/j.biomaterials.2004.10.012
30. Yan L, Amirshaghaghi A, Huang D, Miller J, Stein JM, Busch TM, et al. Protoporphyrin Ix (Ppix)-Coated Superparamagnetic Iron Oxide Nanoparticle (Spion) Nanoclusters for Magnetic Resonance Imaging and Photodynamic Therapy. Adv Funct Mater (2018) 28:16. doi: 10.1002/adfm.201707030
31. Xu JK, Zhang FF, Sun JJ, Sheng J, Wang F, Sun M. Bio and Nanomaterials Based on Fe3O4. Molecules (2014) 19:21506–28. doi: 10.3390/molecules191221506
32. Zeng L, Xiang L, Ren W, Zheng J, Li T, Chen B, et al. Multifunctional Photosensitizer-Conjugated Core–Shell Fe3O4@Nayf4:Yb/Er Nanocomplexes and Their Applications in T2-Weighted Magnetic Resonance/Upconversion Luminescence Imaging and Photodynamic Therapy of Cancer Cells. RSC Adv (2013) 3. doi: 10.1039/c3ra41916a
33. He X, Liu F, Liu L, Duan T, Zhang H, Wang Z. Lectin-Conjugated Fe2O3@Au Core@Shell Nanoparticles as Dual Mode Contrast Agents for In Vivo Detection of Tumor. Mol Pharm (2014) 11:738–45. doi: 10.1021/mp400456j
34. Xinqing J. Integrin Receptor Targeted Crgd-Conjugated Magnetic Nanoparticles for Vitro Imaging Experimental Hepatic Cancer Cells. Chin J Clinicians (Electronic Edition) 05:783–7. doi: 10.3877/cma.j.issn.1674-0785.2015.05.019.
35. Matlou GG, Oluwole DO, Prinsloo E, Nyokong T. Photodynamic Therapy Activity of Zinc Phthalocyanine Linked to Folic Acid and Magnetic Nanoparticles. J Photochem Photobiol B (2018) 186:216–24. doi: 10.1016/j.jphotobiol.2018.07.025
36. Matshitse R, Ngoy BP, Managa M, Mack J, Nyokong T. Photophysical Properties and Photodynamic Therapy Activities of Detonated Nanodiamonds-BODIPY-Phthalocyanines Nanoassemblies. Photodiagnosis Photodyn Ther (2019) 26:101–10. doi: 10.1016/j.pdpdt.2019.03.007
37. Wang F, Han Y, Lim CS, Lu Y, Wang J, Xu J, et al. Simultaneous Phase and Size Control of Upconversion Nanocrystals Through Lanthanide Doping. Nature (2010) 463:1061–5. doi: 10.1038/nature08777
38. Su Q, Feng W, Yang D, Li F. Resonance Energy Transfer in Upconversion Nanoplatforms for Selective Biodetection. Acc Chem Res (2017) 50:32–40. doi: 10.1021/acs.accounts.6b00382
39. Idris NM, Gnanasammandhan MK, Zhang J, Ho PC, Mahendran R, Zhang Y. In Vivo Photodynamic Therapy Using Upconversion Nanoparticles as Remote-Controlled Nanotransducers. Nat Med (2012) 18:1580–5. doi: 10.1038/nm.2933
40. Chen Q, Wang C, Cheng L, He W, Cheng Z, Liu Z. Protein Modified Upconversion Nanoparticles for Imaging-Guided Combined Photothermal and Photodynamic Therapy. Biomaterials (2014) 35:2915–23. doi: 10.1016/j.biomaterials.2013.12.046
41. Li F, Du Y, Liu J, Sun H, Wang J, Li R, et al. Responsive Assembly of Upconversion Nanoparticles for Ph-Activated and Near-Infrared-Triggered Photodynamic Therapy of Deep Tumors. Adv Mater (2018) 30:e1802808. doi: 10.1002/adma.201802808
42. Torchilin VP. Recent Advances With Liposomes as Pharmaceutical Carriers. Nat Rev Drug Discovery (2005) 4:145–60. doi: 10.1038/nrd1632
43. Ghosh S, Carter KA, Lovell JF. Liposomal Formulations of Photosensitizers. Biomaterials (2019) 218:119341. doi: 10.1016/j.biomaterials.2019.119341
44. Shemesh CS, Moshkelani D, Zhang H. Thermosensitive Liposome Formulated Indocyanine Green for Near-Infrared Triggered Photodynamic Therapy: In Vivo Evaluation for Triple-Negative Breast Cancer. Pharm Res (2015) 32:1604–14. doi: 10.1007/s11095-014-1560-7
45. Luo D, Carter KA, Razi A, Geng J, Shao S, Giraldo D, et al. Doxorubicin Encapsulated in Stealth Liposomes Conferred With Light-Triggered Drug Release. Biomaterials (2016) 75:193–202. doi: 10.1016/j.biomaterials.2015.10.027
46. Shemesh CS, Hardy CW, Yu DS, Fernandez B, Zhang H. Indocyanine Green Loaded Liposome Nanocarriers for Photodynamic Therapy Using Human Triple Negative Breast Cancer Cells. Photodiagnosis Photodyn Ther (2014) 11:193–203. doi: 10.1016/j.pdpdt.2014.02.001
47. Shi C, Li M, Zhang Z, Yao Q, Shao K, Xu F, et al. Catalase-Based Liposomal for Reversing Immunosuppressive Tumor Microenvironment and Enhanced Cancer Chemo-Photodynamic Therapy. Biomaterials (2020) 233:119755. doi: 10.1016/j.biomaterials.2020.119755
48. Nascimento BFO, Pereira NAM, Valente AJM, Pinho EMT, Pineiro M. A Review on (Hydro)Porphyrin-Loaded Polymer Micelles: Interesting and Valuable Platforms for Enhanced Cancer Nanotheranostics. Pharmaceutics (2019) 11. doi: 10.3390/pharmaceutics11020081
49. Wu DQ, Li ZY, Li C, Fan JJ, Lu B, Chang C, et al. Porphyrin and Galactosyl Conjugated Micelles for Targeting Photodynamic Therapy. Pharm Res (2010) 27:187–99. doi: 10.1007/s11095-009-9998-8
50. Elbayoumi TA, Pabba S, Roby A, Torchilin VP. Antinucleosome Antibody-Modified Liposomes and Lipid-Core Micelles for Tumor-Targeted Delivery of Therapeutic and Diagnostic Agents. J Liposome Res (2007) 17:1–14. doi: 10.1080/08982100601186474
51. Deng Y, Huang F, Zhang J, Liu J, Li B, Ouyang R, et al. Pegylated Iridium-Based Nano-Micelle: Self-Assembly, Selective Tumor Fluorescence Imaging and Photodynamic Therapy. Dyes Pigments (2020) 182. doi: 10.1016/j.dyepig.2020.108651
52. Bugaj AM. Targeted Photodynamic Therapy–a Promising Strategy of Tumor Treatment. Photochem Photobiol Sci (2011) 10:1097–109. doi: 10.1039/c0pp00147c
53. Li L, Cho H, Yoon KH, Kang HC, Huh KM. Antioxidant-Photosensitizer Dual-Loaded Polymeric Micelles With Controllable Production of Reactive Oxygen Species. Int J Pharm (2014) 471:339–48. doi: 10.1016/j.ijpharm.2014.05.064
54. Bray F, Ferlay J, Soerjomataram I, Siegel RL, Torre LA, Jemal A. Global Cancer Statistics 2018: GLOBOCAN Estimates of Incidence and Mortality Worldwide for 36 Cancers in 185 Countries. CA Cancer J Clin (2018) 68:394–424. doi: 10.3322/caac.21492
55. Que SKT, Zwald FO, Schmults CD. Cutaneous Squamous Cell Carcinoma: Incidence, Risk Factors, Diagnosis, and Staging. J Am Acad Dermatol (2018) 78:237–47. doi: 10.1016/j.jaad.2017.08.059
56. Tas F, Erturk K. Recurrence Behavior in Early-Stage Cutaneous Melanoma: Pattern, Timing, Survival, and Influencing Factors. Melanoma Res (2017) 27:134–9. doi: 10.1097/CMR.0000000000000332
57. Christensen E, Warloe T, Kroon S, Funk J, Helsing P, Soler AM, et al. Guidelines for Practical Use of MAL-PDT in non-Melanoma Skin Cancer. J Eur Acad Dermatol Venereol (2010) 24:505–12. doi: 10.1111/j.1468-3083.2009.03430.x
58. Gold MH. Therapeutic and Aesthetic Uses of Photodynamic Therapy Part Five of a Five-Part Series: ALA-PDT and MAL-PDT What Makes Them Different. J Clin Aesthet Dermatol (2009) 2:44–7.
59. Champeau M, Vignoud S, Mortier L, Mordon S. Photodynamic Therapy for Skin Cancer: How to Enhance Drug Penetration? J Photochem Photobiol B (2019) 197:111544. doi: 10.1016/j.jphotobiol.2019.111544
60. Togsverd-Bo K, Haak CS, Thaysen-Petersen D, Wulf HC, Anderson RR, Haedersdal M. Intensified Photodynamic Therapy of Actinic Keratoses With Fractional CO2 Laser: A Randomized Clinical Trial. Br J Dermatol (2012) 166:1262–9. doi: 10.1111/j.1365-2133.2012.10893.x
61. Haak CS, Christiansen K, Erlendsson AM, Taudorf EH, Thaysen-Petersen D, Wulf HC, et al. Ablative Fractional Laser Enhances MAL-Induced Ppix Accumulation: Impact of Laser Channel Density, Incubation Time and Drug Concentration. J Photochem Photobiol B (2016) 159:42–8. doi: 10.1016/j.jphotobiol.2016.03.021
62. Mikolajewska P, Donnelly RF, Garland MJ, Morrow DI, Singh TR, Iani V, et al. Microneedle Pre-Treatment of Human Skin Improves 5-Aminolevulininc Acid (ALA)- and 5-Aminolevulinic Acid Methyl Ester (MAL)-Induced Ppix Production for Topical Photodynamic Therapy Without Increase in Pain or Erythema. Pharm Res (2010) 27(10):2213–20. doi: 10.1007/s11095-010-0227-2
63. Xie X, Pascual C, Lieu C, Oh S, Wang J, Zou B, et al. Analgesic Microneedle Patch for Neuropathic Pain Therapy. ACS Nano (2017) 11:395–406. doi: 10.1021/acsnano.6b06104
64. Petukhova TA, Hassoun LA, Foolad N, Barath M, Sivamani RK. Effect of Expedited Microneedle-Assisted Photodynamic Therapy for Field Treatment of Actinic Keratoses: A Randomized Clinical Trial. JAMA Dermatol (2017) 153:637–43. doi: 10.1001/jamadermatol.2017.0849
65. Serra-Guillen C, Nagore E, Hueso L, Traves V, Messeguer F, Sanmartin O, et al. A Randomized Pilot Comparative Study of Topical Methyl Aminolevulinate Photodynamic Therapy Versus Imiquimod 5% Versus Sequential Application of Both Therapies in Immunocompetent Patients With Actinic Keratosis: Clinical and Histologic Outcomes. J Am Acad Dermatol (2012) 66:e131–7. doi: 10.1016/j.jaad.2011.11.933
66. Christensen E, Mork C, Skogvoll E. High and Sustained Efficacy After Two Sessions of Topical 5-Aminolaevulinic Acid Photodynamic Therapy for Basal Cell Carcinoma: A Prospective, Clinical and Histological 10-Year Follow-Up Study. Br J Dermatol (2012) 166:1342–8. doi: 10.1111/j.1365-2133.2012.10878.x
67. Roozeboom MH, Aardoom MA, Nelemans PJ, Thissen MR, Kelleners-Smeets NW, Kuijpers DI, et al. Fractionated 5-Aminolevulinic Acid Photodynamic Therapy After Partial Debulking Versus Surgical Excision for Nodular Basal Cell Carcinoma: A Randomized Controlled Trial With at Least 5-Year Follow-Up. J Am Acad Dermatol (2013) 69:280–7. doi: 10.1016/j.jaad.2013.02.014
68. Wan MT, Lin JY. Current Evidence and Applications of Photodynamic Therapy in Dermatology. Clin Cosmet Investig Dermatol (2014) 7:145–63. doi: 10.2147/CCID.S35334
69. Rhodes LE, de Rie M, Enstrom Y, Groves R, Morken T, Goulden V, et al. Photodynamic Therapy Using Topical Methyl Aminolevulinate vs Surgery for Nodular Basal Cell Carcinoma: Results of a Multicenter Randomized Prospective Trial. Arch Dermatol (2004) 140:17–23. doi: 10.1001/archderm.140.1.17
70. Szeimies RM, Ibbotson S, Murrell DF, Rubel D, Frambach Y, de Berker D, et al. A Clinical Study Comparing Methyl Aminolevulinate Photodynamic Therapy and Surgery in Small Superficial Basal Cell Carcinoma (8-20 Mm), With a 12-Month Follow-Up. J Eur Acad Dermatol Venereol (2008) 22:1302–11. doi: 10.1111/j.1468-3083.2008.02803.x
71. Yang X, Palasuberniam P, Kraus D, Chen B. Aminolevulinic Acid-Based Tumor Detection and Therapy: Molecular Mechanisms and Strategies for Enhancement. Int J Mol Sci (2015) 16:25865–80. doi: 10.3390/ijms161025865
72. Anand S, Rollakanti KR, Horst RL, Hasan T, Maytin EV. Combination of Oral Vitamin D3 With Photodynamic Therapy Enhances Tumor Cell Death in a Murine Model of Cutaneous Squamous Cell Carcinoma. Photochem Photobiol (2014) 90:1126–35. doi: 10.1111/php.12286
73. Sun W, Kajimoto Y, Inoue H, Miyatake S, Ishikawa T, Kuroiwa T. Gefitinib Enhances the Efficacy of Photodynamic Therapy Using 5-Aminolevulinic Acid in Malignant Brain Tumor Cells. Photodiagnosis Photodyn Ther (2013) 10:42–50. doi: 10.1016/j.pdpdt.2012.06.003
74. Collier NJ, Rhodes LE. Photodynamic Therapy for Basal Cell Carcinoma: The Clinical Context for Future Research Priorities. Molecules (2020) 25. doi: 10.3390/molecules25225398
75. Dragicevic-Curic N, Fahr A. Liposomes in Topical Photodynamic Therapy. Expert Opin Drug Deliv (2012) 9:1015–32. doi: 10.1517/17425247.2012.697894
76. Buchholz J, Wergin M, Walt H, Grafe S, Bley CR, Kaser-Hotz B. Photodynamic Therapy of Feline Cutaneous Squamous Cell Carcinoma Using a Newly Developed Liposomal Photosensitizer: Preliminary Results Concerning Drug Safety and Efficacy. J Vet Intern Med (2007) 21:770–5. doi: 10.1111/j.1939-1676.2007.tb03020.x
77. Flickinger I, Gasymova E, Dietiker-Moretti S, Tichy A, Rohrer Bley C. Evaluation of Long-Term Outcome and Prognostic Factors of Feline Squamous Cell Carcinomas Treated With Photodynamic Therapy Using Liposomal Phosphorylated Meta-Tetra(Hydroxylphenyl)Chlorine. J Feline Med Surg (2018) 20:1100–4. doi: 10.1177/1098612X17752196
78. Wozniak M, Nowak M, Lazebna A, Wiecek K, Jablonska I, Szpadel K, et al. The Comparison of In Vitro Photosensitizing Efficacy of Curcumin-Loaded Liposomes Following Photodynamic Therapy on Melanoma MUG-Mel2, Squamous Cell Carcinoma SCC-25, and Normal Keratinocyte Hacat Cells. Pharmaceuticals (Basel) (2021) 14. doi: 10.3390/ph14040374
79. Master A, Malamas A, Solanki R, Clausen DM, Eiseman JL, Sen Gupta A. A Cell-Targeted Photodynamic Nanomedicine Strategy for Head and Neck Cancers. Mol Pharm (2013) 10:1988–97. doi: 10.1021/mp400007k
80. Keyal U, Bhatta AK, Zhang G, Wang XL. Present and Future Perspectives of Photodynamic Therapy for Cutaneous Squamous Cell Carcinoma. J Am Acad Dermatol (2019) 80:765–73. doi: 10.1016/j.jaad.2018.10.042
81. Wang X, Shi L, Tu Q, Wang H, Zhang H, Wang P, et al. Treating Cutaneous Squamous Cell Carcinoma Using 5-Aminolevulinic Acid Polylactic-Co-Glycolic Acid Nanoparticle-Mediated Photodynamic Therapy in a Mouse Model. Int J Nanomedicine (2015) 10:347–55. doi: 10.2147/IJN.S71245
82. Keyal U, Luo Q, Bhatta AK, Luan H, Zhang P, Wu Q, et al. Zinc Pthalocyanine-Loaded Chitosan/Mpeg-PLA Nanoparticles-Mediated Photodynamic Therapy for the Treatment of Cutaneous Squamous Cell Carcinoma. J Biophotonics (2018) 11:e201800114. doi: 10.1002/jbio.201800114
83. Morton CA, Dominicus R, Radny P, Dirschka T, Hauschild A, Reinhold U, et al. A Randomized, Multinational, Noninferiority, Phase III Trial to Evaluate the Safety and Efficacy of BF-200 Aminolaevulinic Acid Gel vs. Methyl Aminolaevulinate Cream in the Treatment of Nonaggressive Basal Cell Carcinoma With Photodynamic Therapy. Br J Dermatol (2018) 179:309–19. doi: 10.1111/bjd.16441
84. Salmivuori M, Gronroos M, Tani T, Polonen I, Rasanen J, Annala L, et al. Hexyl Aminolevulinate, 5-Aminolevulinic Acid Nanoemulsion and Methyl Aminolevulinate in Photodynamic Therapy of non-Aggressive Basal Cell Carcinomas: A non-Sponsored, Randomized, Prospective and Double-Blinded Trial. J Eur Acad Dermatol Venereol (2020) 34:2781–8. doi: 10.1111/jdv.16357
85. Garbe C, Amaral T, Peris K, Hauschild A, Arenberger P, Bastholt L, et al. Treatment of, European Consensus-Based Interdisciplinary Guideline for Melanoma. Part 2: Treatment - Update 2019. Eur J Cancer (2020) 126:159–77. doi: 10.1016/j.ejca.2019.11.015
86. Tang JQ, Hou XY, Yang CS, Li YX, Xin Y, Guo WW, et al. Recent Developments in Nanomedicine for Melanoma Treatment. Int J Cancer (2017) 141:646–53. doi: 10.1002/ijc.30708
87. Ragelle H, Riva R, Vandermeulen G, Naeye B, Pourcelle V, Le Duff CS, et al. Chitosan Nanoparticles for Sirna Delivery: Optimizing Formulation to Increase Stability and Efficiency. J Control Release (2014) 176:54–63. doi: 10.1016/j.jconrel.2013.12.026
88. Vera RE, Lamberti MJ, Rivarola VA, Rumie Vittar NB. Developing Strategies to Predict Photodynamic Therapy Outcome: The Role of Melanoma Microenvironment. Tumour Biol (2015) 36:9127–36. doi: 10.1007/s13277-015-4059-x
89. Naidoo C, Kruger CA, Abrahamse H. Photodynamic Therapy for Metastatic Melanoma Treatment: A Review. Technol Cancer Res Treat (2018) 17:1533033818791795. doi: 10.1177/1533033818791795
90. Cai J, Zheng Q, Huang H, Li B. 5-Aminolevulinic Acid Mediated Photodynamic Therapy Inhibits Survival Activity and Promotes Apoptosis of A375 and A431 Cells. Photodiagnosis Photodyn Ther (2018) 21:257–62. doi: 10.1016/j.pdpdt.2018.01.004
91. Ashfaq UA, Riaz M, Yasmeen E, Yousaf MZ. Recent Advances in Nanoparticle-Based Targeted Drug-Delivery Systems Against Cancer and Role of Tumor Microenvironment. Crit Rev Ther Drug Carrier Syst (2017) 34:317–53. doi: 10.1615/CritRevTherDrugCarrierSyst.2017017845
92. Gomaa I, Sebak A, Afifi N, Abdel-Kader M. Liposomal Delivery of Ferrous Chlorophyllin: A Novel Third Generation Photosensitizer for In Vitro PDT of Melanoma. Photodiagnosis Photodyn Ther (2017) 18:162–70. doi: 10.1016/j.pdpdt.2017.01.186
93. Lee EH, Lim SJ, Lee MK. Chitosan-Coated Liposomes to Stabilize and Enhance Transdermal Delivery of Indocyanine Green for Photodynamic Therapy of Melanoma. Carbohydr Polym (2019) 224:115143. doi: 10.1016/j.carbpol.2019.115143
94. Tokarska K, Lamch L, Piechota B, Zukowski K, Chudy M, Wilk KA, et al. Co-Delivery of IR-768 and Daunorubicin Using Mpeg-B-PLGA Micelles for Synergistic Enhancement of Combination Therapy of Melanoma. J Photochem Photobiol B (2020) 211:111981. doi: 10.1016/j.jphotobiol.2020.111981
95. Chen ZA, Kuthati Y, Kankala RK, Chang YC, Liu CL, Weng CF, et al. Encapsulation of Palladium Porphyrin Photosensitizer in Layered Metal Oxide Nanoparticles for Photodynamic Therapy Against Skin Melanoma. Sci Technol Adv Mater (2015) 16:054205. doi: 10.1088/1468-6996/16/5/054205
96. Bi Y, Hao F, Yan G, Teng L, Lee RJ, Xie J. Actively Targeted Nanoparticles for Drug Delivery to Tumor. Curr Drug Metab (2016) 17:763–82. doi: 10.2174/1389200217666160619191853
97. Freitas LF, Hamblin MR, Anzengruber F, Perussi JR, Ribeiro AO, Martins VCA, et al. Zinc Phthalocyanines Attached to Gold Nanorods for Simultaneous Hyperthermic and Photodynamic Therapies Against Melanoma In Vitro. J Photochem Photobiol B (2017) 173:181–6. doi: 10.1016/j.jphotobiol.2017.05.037
98. Campu A, Focsan M, Lerouge F, Borlan R, Tie L, Rugina D, et al. ICG-Loaded Gold Nano-Bipyramids With NIR Activatable Dual PTT-PDT Therapeutic Potential in Melanoma Cells. Colloids Surf B Biointerfaces (2020) 194:111213. doi: 10.1016/j.colsurfb.2020.111213
99. Bazylinska U, Wawrzynczyk D, Szewczyk A, Kulbacka J. Engineering and Biological Assessment of Double Core Nanoplatform for Co-Delivery of Hybrid Fluorophores to Human Melanoma. J Inorg Biochem (2020) 208:111088. doi: 10.1016/j.jinorgbio.2020.111088
100. Hamblin MR. Upconversion in Photodynamic Therapy: Plumbing the Depths. Dalton Trans (2018) 47:8571–80. doi: 10.1039/C8DT00087E
Keywords: photodynamic therapy, photosensitizer, nanotechnology, nanoparticle, nonmelanoma skin cancer, metastatic melanoma
Citation: Zhang P, Han T, Xia H, Dong L, Chen L and Lei L (2022) Advances in Photodynamic Therapy Based on Nanotechnology and Its Application in Skin Cancer. Front. Oncol. 12:836397. doi: 10.3389/fonc.2022.836397
Received: 15 December 2021; Accepted: 03 February 2022;
Published: 16 March 2022.
Edited by:
Nihal Ahmad, University of Wisconsin-Madison, United StatesReviewed by:
Maria Julia Lamberti, National University of Río Cuarto, ArgentinaSanjay Anand, Cleveland Clinic, United States
Copyright © 2022 Zhang, Han, Xia, Dong, Chen and Lei. This is an open-access article distributed under the terms of the Creative Commons Attribution License (CC BY). The use, distribution or reproduction in other forums is permitted, provided the original author(s) and the copyright owner(s) are credited and that the original publication in this journal is cited, in accordance with accepted academic practice. No use, distribution or reproduction is permitted which does not comply with these terms.
*Correspondence: Lijie Dong, ZG9uZ0B3aHV0LmVkdS5jbg==; Liuqing Chen, Y2xxMzVAMTI2LmNvbQ==