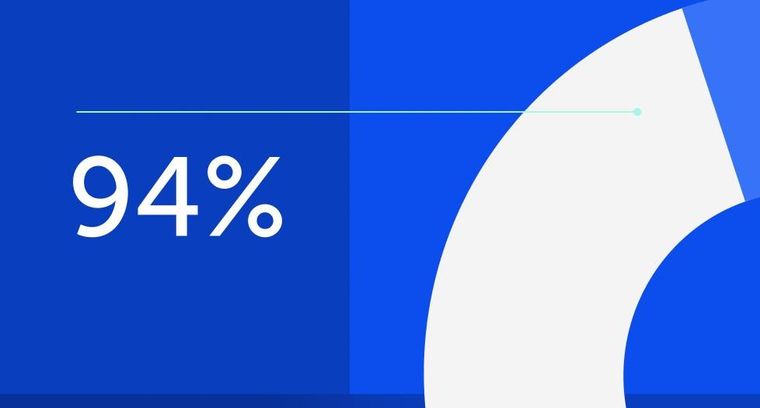
94% of researchers rate our articles as excellent or good
Learn more about the work of our research integrity team to safeguard the quality of each article we publish.
Find out more
REVIEW article
Front. Oncol., 01 April 2022
Sec. Pediatric Oncology
Volume 12 - 2022 | https://doi.org/10.3389/fonc.2022.822330
The management of retinoblastoma, the most common intraocular malignancy in children, has changed drastically over the last decade. Landmark developments in local drug delivery, namely, safer techniques for intravitreal chemotherapy injection and ophthalmic artery chemosurgery, have resulted in eye globe salvages that were not previously attainable using systemic chemotherapy or external beam irradiation. Novel drugs, oncolytic viruses, and immunotherapy are promising approaches in the treatment of intraocular retinoblastoma. Importantly, emerging studies of the pattern of tumor dissemination and local drug delivery may provide the first steps toward new treatments for metastatic disease. Here, we review recent advances in retinoblastoma treatment, especially with regard to local drug delivery, that have enabled successful conservative management of intraocular retinoblastoma. We also review emerging data from preclinical and clinical studies on innovative approaches that promise to lead to further improvement in outcomes, namely, the mechanisms and potential uses of new and repurposed drugs and non-chemotherapy treatments, and discuss future directions for therapeutic development.
Retinoblastoma is a highly curable neoplasm in high-income countries, where patient survival exceeds 99%, making it the most curable of all pediatric cancers. In striking contrast, in many lower-income countries, most patients present with disseminated and metastatic disease, which is almost always fatal (1, 2).
In high-income countries, eye-sparing treatments have been used for decades. In the late 1990s there was an evolution in conservative treatment from local, eye-directed therapies such as external beam radiotherapy (EBRT) toward systemic chemotherapy combined with aggressive focal therapies. This change in approach aimed to reduce the use of EBRT, which has been consistently associated with a higher risk of second malignancies (3). The use of systemic chemoreduction was effective for tumor control in eyes with less advanced tumors, avoiding the use of EBRT (4, 5). However, the majority of eyes worldwide (75%) present with more advanced tumors with massive vitreous or subretinal seeding and retinal detachment, where both radiation and systemic chemotherapy can rarely save the eye or vision (6). Although this treatment results in reduced long-term toxicity by avoiding EBRT in many patients, its acute toxicity, though manageable in developed countries can be fatal in up to 4–5% of patients in less resourced settings (7). Moreover, most studies showed an additive risk for secondary malignancies when chemotherapy is combined with EBRT (8). Ototoxicity caused by carboplatin and cases of fatal chemotherapy-induced leukemia were also reported in non-irradiated children with retinoblastoma (9–12). So, from the mid-2000s on, most groups moved away from systemic chemotherapy to advanced techniques for more selective ocular delivery to increase drug exposure in the tumor, maximizing efficacy and minimizing the probability of adverse events.
The introduction of ophthalmic artery chemosurgery (OAC) led to remarkable success in treating eyes with more advanced disease in which systemic chemotherapy had poorer results (13–20). In the 2010s intravitreous chemotherapy (IVi) was added as another eye-directed therapy, which in conjunction with OAC became the current standard therapy utilized by many centers in high- and middle-income countries, achieving unprecedented success in eye preservation and completely eliminating the use of EBRT (19, 21–24).
Despite the changing paradigm of retinoblastoma treatment from systemic to OAC and IVi chemotherapy, eyes that relapse or that are initially refractory to conventional therapy are still difficult to treat with currently available drug options and most undergo enucleation. Thus, drug discovery in retinoblastoma is of paramount importance. Recent studies have used innovative multi-omics technology to identify deregulated pathways that could be targeted via novel treatment strategies in retinoblastoma. Moreover, targets for immunotherapy such as GD2 ganglioside have been under evaluation despite limited translation into the clinic. Other innovative approaches include conditional replicating oncolytic adenovirus targeting the RB1 pathway, which is currently under Phase I evaluation with promising early results.
In the present review we aimed to describe the latest innovations in retinoblastoma treatment, including both preclinical and clinical studies that have led to new local drug delivery routes and the discovery of promising drugs and non-chemotherapy strategies.
In the 1950s, systemic triethylenemelamine was used in patients with retinoblastoma to reduce the dose of EBRT, but was abandoned due to severe side effects (fever with neutropenia was then a life-threatening condition). In the 1970s, systemic chemotherapy was reintroduced for retinoblastoma using vincristine, cyclophosphamide, cisplatin, and doxorubicin based on clinical responses in metastatic patients (25, 26). It was not until the mid-1990s that systemic chemotherapy became widely adopted based on work in England and the US showing the efficacy of carboplatin for intraocular disease (27). Drug selection was mainly on an empirical basis. During the last two decades, etoposide for systemic chemotherapy and both topotecan and melphalan, and also etoposide and vincristine, have been incorporated in the clinic.
After intravenous (i.v) administration, the entire drug dose enters the systemic circulation but only a small fraction becomes available at the ocular tumor after metabolism in major organs and penetration through the blood-ocular barrier. For instance, vitreous topotecan drug exposure was only one-third of the systemic exposure after i.v infusion in rats, whereas etoposide vitreous concentrations barely attained 10% of the systemic exposure (28). Although clinical responses were universal and often dramatic with these drugs, tumors regrew if they could not be controlled with additional focal treatments. The majority of retinoblastoma eyes worldwide at diagnosis have vitreous and sub-retinal seeding which is rarely controlled by systemic chemotherapy (2, 10). Thus, local drug delivery is preferred to increase drug exposure in the eye while limiting the amount that reaches the bloodstream, which reduces systemic toxicity and risk of second malignancies being particularly important for children with germline mutations (29, 30).
During the last decade, rates of globe salvage have dramatically improved, even in eyes with advanced retinoblastoma, as a result of routine local chemotherapy administration. For example, at the Memorial Sloan Kettering Cancer Center (MSKCC) in New York, the enucleation rate dropped from 95% to less than 10% in a ten-year period (18). This success is most probably related to an increase in the bioavailability of the drugs in tumor tissues.
Intra-arterial delivery of chemotherapy was first achieved 70 years ago by Reese, who delivered a nitrogen mustard derivative to the eye by puncturing the internal carotid artery and injecting the drug into that artery. Although responses were dramatic, tumors regrew; he added EBRT to cure those patients. Japanese investigators then discovered that melphalan appeared to be the most effective chemotherapy against retinoblastoma and began delivering intra-arterially (31). They designed a microcatheter similar to a miniature Foley catheter, which was inserted in the femoral artery and passed through the body until just above the orifice of the ophthalmic artery. After occluding the internal carotid with the balloon catheter, melphalan was injected. The success rate was high and long-term follow-up showed no adverse consequences from the technique, drug, or X-ray exposure during the procedure (32). These patients also received combinations of EBRT, IVi melphalan, and hyperthermia, making it difficult to discern the true contribution of intra-arterial chemotherapy. Despite having experience for more than 30 years with over 400 eyes, the technique was only performed in Japan. The modern era for OAC began following establishment of the technique by Abramson and Gobin at MSK in 2006 (15, 33). Since then, it has been adopted as primary and salvage therapy for both unilateral and bilateral retinoblastoma worldwide and is performed in more than 45 countries. Every series published to date, from the US, China, India, Argentina, Italy, Switzerland, Malaysia, and others, has concluded that the technique is better, safer, and more effective (33–39).
Key outcomes of the widespread adoption of OAC include:
1. Eliminating the use of EBRT, which has decreased the incidence of second, non-ocular tumors and thereby improved long-term survival (2).
2. Eliminating the use of systemic multiagent chemotherapy completely in many centers. This eliminates the need for a port, which requires prophylactic antibiotics to prevent infections, and also for transfusions to treat associated neutropenia. Also, it prevents chemotherapy-induced hair loss and permanent hearing loss (caused by carboplatin). With OAC, fever/neutropenia requiring transfusions develops in <1% of all patients. Associated benefits include:
a. Likely eliminating the development of secondary leukemia in retinoblastoma patients; to date no cases have been reported worldwide (30).
b. Reducing expense in most settings by avoiding the costs of systemic chemotherapy: ports, antibiotics, hospitalization, and treatment for febrile neutropenia such as transfusions (33).
c. Reduced impact on immune function, allowing patients to continue to receive routine vaccinations (40).
d. Avoiding associated hindrance of patient growth (height and weight (41)).
3. Drastically increasing the rate of eye salvage. At MSKCC, this rate has increased from 5 to 95% (42).
4. Enabling salvage of eyes that have failed multiagent systemic chemotherapy (13, 18).
5. Reducing the time from initiating to completing therapy (2).
6. Successful treatment of choroidal invasion, orbital retinoblastoma and optic nerve invasion in select cases (43, 44).
7. Enabling cure of eyes with vitreous and or subretinal seeding which was nearly impossible with systemic chemotherapy and usually failed with external beam radiation.
8. Avoidance of increased risk of orbital disease or second cancers (45).
9. Allowing almost 25% of all eyes with retinal detachment and extinguished ERGs to regain more than 25 μV of activity (46).
The aim in developing OAC was to deliver the drug directly into the artery that irrigates the ocular tissues to increase local bioavailability while minimizing systemic exposure. An initial proof-of-concept study in a swine model reported that topotecan vitreous exposure was almost 30 times higher than that in the bloodstream (47). Moreover, vitreous levels exceeded the drug concentration needed to cause a 50% decrease in in vitro tumor cell proliferation (IC50) for a period of time greatly exceeding that needed to exert cytotoxicity. A later similar study showed that the OAC route selectively delivers chemotherapy to the eye: topotecan concentrations in the vitreous and retina of non-tumor bearing pigs were 243 and 146 times higher after OAC than after IV, respectively, while systemic exposure was comparable between the two routes (48). The favorable disposition of topotecan in ocular tissues after OAC would have predicted successful tumor control; however, this has not been clinically observed. Failure to achieve adequate tumor control despite vitreous levels above the IC50, considered a surrogate of active pharmacological threshold, may be related to the administration schedule. Because topotecan exerts its cytotoxic activity by inhibiting the nuclear enzyme topoisomerase I, resulting in double-strand breaks during cell replication, it acts on cells in the S-phase and subsequent doses are required to target quiescent tumor cells that enter cell replication. Thus, protracted schedules have been associated with greater antitumor effects compared to intermittent, higher-dose schedules (49, 50). However, repeated daily OAC to deliver topotecan is impractical, limiting its efficacy via this delivery route.
OAC infusion was also shown to allow more selective ocular delivery of the alkylating agent melphalan; concentrations in the retina and vitreous were 12 times and 26 times higher, respectively, after OAC than after IV infusion in non-tumor-bearing rabbits (51). Interestingly, as these values are much lower than those for topotecan, and considering that vitreous-to-plasma exposure of melphalan also in pigs is 10-times less than that for topotecan, melphalan appears to display a lower capacity to penetrate or be retained in the vitreous and retina than topotecan (52, 53). This limited penetration and/or retention of melphalan in the vitreous after OAC was evident in a pharmacokinetic study in pigs showing that the drug barely attains cytotoxic levels in the vitreous (53).
Despite restricted melphalan vitreal penetration in non-tumor bearing models, with the caveat of potential differences in drug disposition between eyes filled with tumor and healthy eyes, melphalan displays a marked antitumor effect after OAC that is not observed after topotecan. Nonetheless, the higher ocular exposure to chemotherapy attained after OAC delivery than after IV infusion is the reasonable explanation for a better tumor control in eyes with retinoblastoma. This hypothesis was confirmed in tumor-bearing rabbits treated with a high dose of OAC melphalan (1.2 mg/kg), which showed substantial antitumor effects, in contrast to a lack of tumor cell killing in eyes treated with the same IV dose of melphalan or with the standard combination of carboplatin, vincristine, and etoposide that is still widely used in clinical practice (51). Nonetheless, these results may be biased by the use of a single dose of melphalan almost 3 times higher than that used for patients with retinoblastoma. Melphalan cytotoxicity is schedule and dose-dependent and these factors may have an impact on the drug efficacy and toxicity.
Other results that support the penetration of melphalan after OAC in ocular tissues were obtained in pigs in which melphalan accumulated in the retinal pigment epithelium (RPE)-choroid, probably due to its affinity to melanin and perhaps explaining the efficacy against subretinal seeds, and the choroidal toxicity associated with the drug in the clinic (53).
Although OAC primarily delivers drug locally, because the drug is injected into an artery, detectable concentrations in the bloodstream are expected as shown in animal models (47, 51, 53). After OAC of melphalan to rabbits, drug concentrations in the contralateral eye were 50–80 times lower than in the treated eye, but similar to those in plasma (51). Importantly, a clinical pharmacokinetic study in patients receiving single-agent melphalan by OAC reported that melphalan dose-normalized systemic exposure was 165 ng ∗ h/ml/mg. As it is well described for IV infusions, melphalan systemic exposure after OAC is proportional to the dose (between 3 and 7 mg), and a threshold of 0.48 mg/kg is associated with an increased probability of developing severe neutropenia (53, 54). In a subsequent study, the pharmacokinetics of melphalan was unaltered by concomitant administration of topotecan. Consistent with similar systemic exposure to melphalan, the incidence of grade III/IV neutropenia (12%) was comparable to that obtained in patients receiving OAC of single-agent melphalan at doses less than 0.5 mg/kg, and no patient had fever or needed transfusions or hospitalization (55). In addition, topotecan systemic exposure was far less than that previously reported to be associated with severe neutropenia (56).
Recent work showed that OAC is also a selective route for carboplatin delivery to the retina, resulting in an almost 4-fold higher exposure in this tissue compared to that in plasma (51). Nonetheless, the blood-retinal barrier may hinder free drug penetration, as carboplatin exposure in the vitreous was almost 3 times lower than in the retina. Despite potential ocular barriers limiting free diffusion, OAC led to 123 and 131 times higher exposure in the retina and vitreous, respectively, compared with those following IV infusion of the same dose (51).
The most common toxicity of OAC results from the high dose of chemotherapy in the eye, namely choroidal vascular toxicity, which arises in approximately 3–5% of patients (57). Central retinal artery occlusion is also possible after OAC, but has been reported in isolated cases and is clearly related to the experience of the treating team (58). Other side effects such as eyelid edema are seen in 10–15% of infusions, but do not give rise to permanent sequelae (42). Some patients present hypotension and bronchospasm during the anesthetic procedure, which may occasionally lead to respiratory arrest (59). Stroke has not been seen in most large series, but single cases have been reported after OAC (60).
The optic nerve is a crucial route of tumor cell dissemination to the CNS in eyes with advanced disease, and thus patients with optic nerve tumor involvement would benefit from high local exposure to chemotherapy (61). Because the ophthalmic artery supplies blood to the intraorbital segment of the optic nerve, in a porcine model, OAC administration of topotecan led to drug concentrations that were 80 times higher in the proximal and distal portions of the optic nerve than after IV infusion, with concentrations greatly exceeding the topotecan IC50 (48).
These concepts were translated to the treatment of a patient with a massive orbital and chiasmatic mass with positive CSF cytology. This patient received high-dose OAC targeting the optic nerve and chiasm, along with intrathecal topotecan for leptomeningeal dissemination (44). Histologically confirmed complete response was achieved after three cycles of this treatment. Orbital retinoblastoma has also been treated with OAC in a limited number of patients, either as monotherapy after extraocular relapse or in combination with systemic chemotherapy to avoid the use of orbital EBRT (62, 63).
Typically, massive choroidal invasion is detected upon pathological examination of the enucleated eye and has been associated with up to 4% risk of extraocular relapse (64). OAC has also been recently used for the treatment of patients in whom choroidal invasion was detectable during ocular examination (43). In these patients, tumor response included the choroidal invasion and eyes were preserved without extraocular relapse. Though these are encouraging results, more data are needed to confirm the safety of this therapy.
Vitreous seeding may occur in eyes with endophytic features or after conservative therapy. Until the advent of OAC in combination with IVi, vitreous seeding was extremely difficult to control with systemic chemotherapy or EBRT, and thus most of those eyes were enucleated. Modifying IVi to enhance safety by injecting the drug in a tumor-free pars plana site using an anti-reflux technique made it possible to safely administer chemotherapy and, more recently, biological agents (21, 65, 66). A worldwide survey of thousands of cases revealed no extraocular spread after IVi (65). Similarly, using a sensitive method for the quantification of RNA from photoreceptors collected via sterile filter paper pieces placed on the site of injection after IVi, no tumor cell reflux was detected after IVi chemotherapy (61, 67).
Melphalan and topotecan have been the most commonly used drugs which may be injected every 7–21 days; the number of injections varies depending on response and toxicity (23, 68–70). In general, response of vitreous seeds to IVi injections varies from 85 to 100% efficacy (23, 69). Response to IVi chemotherapy, including time to regression, is associated with seed classification according to appearance on indirect ophthalmoscopy as clouds, dust, or spheres (69, 71). For instance, spheres regress in a shorter amount of time and receive less cumulative drug compared with clouds.
Retinal toxicity has been reported with IVi melphalan: specifically, each 30 µg of melphalan is associated with an approximately 5 µV decrement in retinal function as evaluated by electroretinogram (68). Because melphalan physically associates with melanin, eyes with deeper inherent pigmentation appear to develop more retinal toxicity following IVi, i.e., worse electroretinogram recordings and more apparent fundus abnormalities (23, 68, 70), due to uptake into the RPE. IVi topotecan does not appear to confer the same retinal toxicity (72), although its clinical utility as a single agent through IVi is still being investigated. There are only limited data on the efficacy and toxicity of IVi carboplatin (73).
IVi injection is ideal for targeting vitreous disease, as this route avoids losing active drug due to restricted penetration through the ocular barriers and systemic metabolism. IVi injection of only 15 μg of melphalan, equivalent to a human dose of between 30 and 35 μg, resulted in vitreous concentrations above the IC50 for several hours, consistent with IVi administration allowing superior control of vitreous seeds in patients (74). Importantly, melphalan was undetectable in plasma, probably explaining the lack of hematologic toxicity associated with IVi chemotherapy. Although beneficial for controlling vitreous seeds, high drug concentrations in the vitreous were associated with morphological and functional retinal changes in white and pigmented rabbits and also a reduction in the ERG response in patients (23, 68, 75–78). As mentioned earlier, this toxicity likely results from the melanin-binding capacity of melphalan and resulting retention in the RPE (53, 68, 79).
Some practical considerations that hinder the use of melphalan include its spontaneous hydrolysis; significant activity is lost after 1 h of reconstitution of the commercial vial. Moreover, as the commercial vial contains 50 mg of melphalan but doses for IVi delivered in 0.1 ml are typically between 20 and 30 μg, about 94% of the drug would be wasted at every single procedure. To avoid this unnecessary cost, pharmacists have shown that storing reconstituted melphalan solution (300 ng/ml) in prefilled syringes at −20°C maintains activity (80). In recent years, a new propylene glycol-free injectable melphalan formulation has shown improved drug stability compared to the original product (81) without affecting the frequency of toxicity and ocular survival in patients receiving IVi melphalan (76).
A safer cytotoxic agent for IVi is the topoisomerase inhibitor topotecan, as shown in preclinical models and in human eyes of the single agent at doses up to 30 μg or in combination with melphalan (72, 75, 82–84). As previously discussed, protracted schemes of topotecan and thus, high and prolonged vitreous exposure would be desirable to leverage antitumor efficacy without the need for further IVi injections (50, 85). Three weekly doses of 15 μg or 30 μg topotecan were reported as nontoxic to the rabbit eye (75). In our experience repeated doses of up to 50 μg of topotecan in rabbits (equivalent to a 100 μg human dose) can be intravitreally injected without morphological or functional retinal changes (86).
Lastly, an advantage of using topotecan is that institutions with limited resources may opt to use a solution of 300ng/mL in prefilled syringes at room temperature for 24 h and frozen at −20°C as they have proven to be stable for almost 6 months (80).
Invasion into the anterior segment was traditionally considered an absolute indication for eye enucleation, based on the possible increased risk of systemic dissemination via the heavily vascularized structures of that part of the eye. However, many patients respond to repeated intra-cameral injection of chemotherapy, usually in combination with IVi chemotherapy, and many such treated eyes could be saved. However, because of the rarity of anterior segment invasion, experience is still based on a limited number of cases. As in the case of IVi chemotherapy, melphalan and more recently topotecan have been used for intracameral injection (2, 87).
Periocular injection of chemotherapy has the advantage of circumventing the blood-retinal barrier, reaching the tumor through transscleral passage without the need to puncture the globe. Nonetheless, orbital and choroidal blood flow, tissue binding, and tight junctions between RPE cells hinder free drug transscleral diffusion.
Carboplatin has been the most intensively investigated drug and although it resulted in a substantial increase in vitreous concentrations in preclinical models, it was abandoned by most groups because of its local and profound hematopoietic toxicity if given in combination with systemic topotecan (88–90). Due to the physicochemical properties of topotecan, it diffuses across the sclera less efficiently than carboplatin and therefore reaches the vitreous after distribution from the bloodstream, as shown in non-tumor-bearing rabbits (91). Nonetheless, a phase I study was opened to evaluate a novel sustained-release episcleral plaque loaded with topotecan called “Chemoplaque” (92).
Patients with leptomeningeal retinoblastoma are seldom cured with current therapy. In these cases, intrathecal (IT) or intraventricular (IVt) administration of chemotherapy may provide a means of attaining pharmacologically active levels in the CSF. These routes ensure direct delivery of chemotherapy to the CSF and thus are particularly advantageous for drugs whose free penetration is restricted by the blood–brain barrier.
Intrathecal methotrexate and cytarabine were used to treat retinoblastoma in the 1980s but in vitro results showed low or modest cyototoxicity in retinoblastoma cell lines (31, 93). More recently, IT/IVt topotecan has been introduced. Moreover, administration of topotecan is feasible at a maximum dose of 0.4 mg with manageable toxicity and clinical responses in patients with leukemia (94). In retinoblastoma, IVt topotecan and cytarabine in combination with IV or OAC chemotherapy have been effective in a few cases (95). A current Latin American GALOP group-led study is testing the use of IT topotecan in the adjuvant setting (96, 97); more such studies are necessary to determine the role of IT topotecan in retinoblastoma. Other putative candidates for intrathecal use include romidepsin, etoposide, and teniposide (98).
New drugs effective for both intraocular and disseminated retinoblastoma are urgently needed; these may include novel drugs and repurposed agents, i.e., those already used in the clinic for other cancers or diseases but still without an indication for retinoblastoma. Assessing response to treatment in the preclinical setting is a challenge due to the limited availability of models that recapitulate clinical observations. Similarly, assessing clinical response in the setting of intraocular disease is difficult because of the lack of consensus for response criteria. The RECIST response criteria were recently proposed to address this issue, but they require MRI, which is expensive and unable to assess small tumors, and ultrasound, the use of which is not standardized worldwide and not reproducible in retinoblastoma (99). Current approaches to developing new treatments include drug discovery using innovative technology to identify deregulated pathways in specific tumors, against which targeted agents may be designed, and drug repurposing, a faster and cheaper strategy.
The discovery of new drugs with potential for translation to the retinoblastoma clinic should be rationally designed to prioritize the most promising candidates. The fundamental starting point in the selection process is comprehensive pharmacological characterization to determine the capacity of each agent to induce selective cytotoxicity in tumor cells using commercial or primary cell lines derived from intraocular tumors or sites of dissemination (100–105) (Figure 1). Such evaluation may consist of high-throughput screens (HTS) in which large libraries of hundreds of compounds are rapidly tested, though few HTS screens have been reported, likely due to challenges in establishing and maintaining retinoblastoma cell lines. Preferably several cell lines should be tested to account for the diversity in drug response observed in patients. Animal models bearing tumors xenografted from intraocular or metastatic patient retinoblastomas could provide a strategic tool to evaluate drug performance before translation to humans (106, 107) (Figure 1).
Figure 1 Workflow for tumor sampling and genomic and pharmacological characterization. Samples obtained from enucleated eyes with retinoblastoma, cerebrospinal fluid, and/or bone marrow are subjected to multi-omic analysis to identify deregulated pathways that may be subjected to targeted agents. Also, these samples may be used to establish tumor cell lines and patient-derived xenografts in immunocompromised animals to evaluate tumor invasiveness and pharmacological sensitivity to novel agents, repositioned drugs, or combination therapies.
One HTS study identified cardenolides as active against retinoblastoma (108). Based on this finding, one patient was reported cured from recurrent intraocular disease after OAC delivery of the cardenolide digoxin, but a cataract developed as a consequence (109). Nonetheless, OAC may not be the optimal route for digoxin due to low intravitreal levels and systemic exposure related to cardiotoxicity; IVi may be preferable. In rabbits, IVi of digoxin resulted in levels well above the IC50 and plasma concentrations far below the concentrations related to cardiac toxicity in humans; however, the injected dose was toxic to the retina (110, 111).
Subsequently, almost 200 compounds were found to be cytotoxic to primary cell lines derived from intraocular and CSF-disseminated retinoblastomas, including drugs already used in pediatric oncology and new hits in preliminary phases of drug development (98). A selection process for prioritizing drugs for subsequent studies before clinical translation based on their pharmacokinetics, biopharmaceutical properties, safety profiles, and the intent of treatment was proposed. Further, FDA-approved agents that have been investigated in Phase I/II studies in pediatrics or clinically used in this age group may be more rapidly translated into the clinic as repurposed drugs. However, an alternative option would be to identify tumor targets and then select or develop direct treatments. In all cases, one of the first steps in the decision process is to consider the properties of a given drug (solubility, metabolism, and ability to cross the blood-ocular or blood–brain barrier) in light of potential delivery routes and corresponding clinical uses (OAC and IVi for intraocular disease and IT/IVt to target tumor cells that have spread to the CNS) (Table 1).
According to this approach, promising agents in early phases of drug development, namely, inhibitors of Bcl-2 proteins, the proteasome, bromodomain and extra-terminal motif proteins (BET), NF-κB, histone deacetylase, kinesin spindle protein, STAT3, and survivin, were identified (Figure 2). Similarly, functional genomics and transcriptomic analysis of retinoblastoma tumors showed the DNA repair proteins RAD51 and BRCA1 to be deregulated, leading to evaluation of the combination of topotecan, a DNA-damaging drug, with a specific small molecule inhibitor of RAD51, which resulted in synergistic in vitro and in vivo antitumor effects (112). A triple combination with the BCL-2 anti-apoptotic inhibitor navitoclax was proposed to lower the dose of the synergistic combination and avoid tumor cell resistance.
Figure 2 Drug discovery in retinoblastoma. Left: Pathways shown to be deregulated in retinoblastoma and drugs targeting them that may represent future candidates for translation into the clinic. These include agents that disrupt the interaction between MDM2/MDM4 and p53 (e.g., nutlin); proteasome, and histone deacetylase (HDAC) inhibitors; bromodomain extra-terminal (BET) proteins inhibitors, which may be selectively active against tumors with MYCN amplification; and a genetically modified adenovirus that selectively replicates in cells with high levels of free E2F transcription factor resulting from a dysfunctional RB1 pathway. Right: Proposed immunotherapy approaches in retinoblastoma, namely, anti-GD2 and anti-NcGM3 monoclonal antibodies, anti-GD2CART cells, and inhibitors of immune checkpoints as PD-1/PD-L1 and B7H3 (omburtamab). In the presence of anti-GD2 monoclonal antibodies, retinoblastoma cells may be targeted by the immune system, as described in neuroblastoma, via a response including granulocyte- and natural killer-mediated antibody-dependent cellular cytotoxicity (ADCC) and complement- and macrophage-mediated cytotoxicity.
One member of the ubiquitin-proteasome system shown to be critical for RB1−/− retinoblastoma is the cullin-RING E3 ligase Skp2 (113, 114). Translation of these results into a novel pharmacological development is limited by the low potency of Skp2 inhibitors. An alternative approach to disrupt ubiquitylation is to inhibit activation of the cullin component using pevonedistat, which is in more advanced stages of drug development in models of neuroblastoma (115–117). IVi pevonedistat, selected as the delivery route because of the low permeability of the drug across the blood–brain barrier, resulted in antitumor effects in in vitro and in vivo preclinical models including MYCN amplified retinoblastomas without evidence of ocular toxicity.
A recent analysis of single-cell sequencing data identified molecular pathways that are deregulated in retinoblastoma and could be targeted as novel treatment strategies (118). Among the most deregulated was the MDM2/MDM4-p53 pathway (119). Although the gene TP53 is almost always unaltered in retinoblastoma, the negative regulator MDM2 and its homolog MDM4 may be overexpressed, blocking the transcriptional activity and stimulating degradation of the p53 protein (120). Therefore, novel drugs such as nutlin-3, which inhibits the interaction between MDM2 and MDM4 with p53, have been pursued as a strategy to restore p53-mediated apoptosis in tumor cells (121, 122) (Figure 2). Nonetheless, contradictory results regarding the incidence of MDM2/MDM4 overexpression in patients with retinoblastoma, along with the toxicity of nutlin-3a and poor bioavailability in ocular tissues, prevented its translation into the clinic despite promising results in genetically engineered and xenograft models (120, 122, 123). New classes of small molecule MDM2/MDM4 inhibitors are being evaluated in Phase I/II studies in combination with broad-spectrum and targeted therapies; one of these is a trial in pediatric cancer including retinoblastoma (121, 124). Another option is targeting another p53 negative regulator, HDM2, a small molecule inhibitor which have been shown to cause cell death in retinoblastoma cells (125).
Other pathways that were among the most significantly deregulated in the above single-cell sequencing study included MYC and GABA receptor signaling, both activated, prompting further investigation of inhibitors of MYC signaling, such as MYC/MAX interaction inhibitors and BET inhibitors, and repurposing of calcium and potassium channel blockers (118) (Figure 2). Expression of members of the retinoid X receptor family was also found to be altered, supporting vitamin D and retinoic acid, the ligands of these receptor, as candidate retinoblastoma therapeutics. Finally, the NF-κB, IGF1R, and STAT3 pathways were also deregulated; agents that target these pathways have already been proposed (98).
Epigenetic alterations have also been investigated as alternative targetable pathways for retinoblastoma treatment. A multi-omic study showed that epigenetic changes, including upregulation of SYK, cooperate with RB1 loss to support retinoblastoma tumor progression (126). The SYK inhibitor BAY-61-3606 led to apoptosis in retinoblastoma cell lines and resulted in antitumor effects after subconjunctival administration in orthotopic xenografts. In contrast, the SYK inhibitor fostamatinib, an orally available prodrug that has recently been approved for the treatment of adult patients with chronic immune thrombocytopenia, and the active metabolite R406, had no antitumor effects in tumor-bearing animals when administered via several local routes and in multiple formulations (127–129). This lack of antitumor activity calls into question the clinical relevance of targeting epigenetic deregulation of SYK.
Continued efforts to identify alterations in signaling and regulatory pathways driving retinoblastoma development and progression promises to contribute to the development of novel targeted agents and bring new combination therapies to the clinic.
Retinoblastoma widely expresses the ganglioside GD2 even in pre-treated chemo-refractory cases with MYCN amplification (105, 130, 131) (Figure 2), suggesting that anti-GD2 monoclonal antibodies may be effective. These antibodies are approved for the treatment of neuroblastoma (132, 133), but there is little clinical experience with their use in retinoblastoma and its niche therapeutic effect (if any) needs to be ascertained.
The major toxicity of anti-GD2 antibodies is related to peripheral nerve toxicity, limiting their use as conservative therapy for retinoblastoma and for the treatment of extraocular disease as the major site of failure in these cases is the CNS. Nonetheless, the radio conjugated anti-GD2 murine antibody 131I-3F8 was shown to be well-tolerated in a Phase I study when delivered from an Ommaya reservoir for control of CNS metastasis (134). Other gangliosides such as NcGM3 are also highly expressed in retinoblastoma and may represent potential immunotherapy targets, as shown in a child with trilateral retinoblastoma who was treated with an anti-idiotypic vaccine targeting NcGM3 (135).
GD2 along with the neural cell adhesion glycoprotein CD171, which is also highly expressed on the surface of retinoblastoma cells, is also a target for recently designed CART cells. Sequential administration of these two types of CART cells was cytotoxic to a panel of retinoblastoma cell lines (136). Subsequently, others developed local immunotherapy formulations also based on anti-GD2 CART cells, including an injectable hydrogel to prolong their localization at the site of injection. After IVi administration, the formulation resulted in complete tumor response and no recurrence or ocular toxicity in orthotopic xenograft models (137, 138) (Figure 2).
Though the eye is traditionally considered an “immune-privileged” organ (139), recent transcriptomic data on initially enucleated eyes revealed an immune signature specific to a retinoblastoma subtype characterized by a putative “late cone” precursor (subtype 1 retinoblastoma) (140). These cases, compared with those originating from earlier cone precursors which may show an immunosuppressive signature as in other neuroectodermal tumors (subtype II retinoblastoma), have relatively high infiltration of immune cells in the tumor microenvironment. Moreover, PD-1 and PD-L1 expression were observed in 20–40% of cases, depending on whether the eye was primarily or secondarily enucleated (141–143), while CTLA-4 expression followed a similar pattern. Some retinoblastomas also express B7H3 (144), another immune checkpoint target for which omburtamab has been developed for clinical use as radio-immunotherapy to treat other neural and mesenchymal tumors (Figure 2). These findings support the investigation of immune checkpoint inhibitors for retinoblastoma, but such studies have not yet been undertaken.
The eye presents a favorable environment for intraocular injection of therapeutic viral vectors. IVi administration of an adenoviral vector carrying a suicidal gene followed by ganciclovir therapy was tested in a Phase I study, which reported that one of eight patients treated achieved tumor control (145). However, the advent of effective IVi chemotherapy limited its subsequent development. Other groups carried out preclinical development of conditional replicating oncolytic adenovirus. One of these vectors was clinically translated as VCN-01, a genetically modified adenovirus designed to selectively replicate in cells with high levels of free E2F-1, which results from RB1 pathway dysfunction (66, 146). Preclinical studies showed high antitumor activity in a set of patient-derived cell lines and xenografts with little or no dissemination to other organs and no replication in healthy retinas after IVi. The experience was translated to the clinic and a Phase I study is currently open with initial results revealing antitumor activity in one of the treated patients (66).
Recent studies suggest that biological subtypes of retinoblastoma may differ in their ability to metastasize. The more aggressive genetic subtype 2 is more prevalent among patients with unilateral disease, who may be at higher risk of tumor dissemination (140). However, our capacity to noninvasively identify patients at higher risk for developing metastatic disease or those with MYCN-amplified tumors who are not candidates for eye salvage is still limited (147). A promising method of noninvasive tumor monitoring is the measurement of circulating tumor DNA (ctDNA) in liquid biopsy samples. Liquid biopsy studies have shown that patients who subsequently developed metastatic relapse had higher levels of ctDNA (148, 149). In these patients, ctDNA levels could indicate higher tumor burden, and continued ctDNA detection after enucleation would predict impending extraocular relapse. Another major site of tumor dissemination is the CSF, but yet it has not been sampled for liquid biopsy study in retinoblastoma.
Retinoblastoma has been selected as a priority tumor by the WHO for its Global Initiative for Childhood Cancer. Despite being highly curable at early stages, it may be fatal if left untreated. Eye-globe salvage treatments have substantially evolved over the last few decades, making intraocular retinoblastoma the most curable of all pediatric cancers in high-income countries. The development of local drug delivery methods that maximize chemotherapy exposure in the retinal, subretinal, and vitreous spaces, namely OAC and a safety-enhanced technique for IVi injection, have resulted in an unprecedented rate of eye globe and vision preservation. Importantly, these new local treatments result in very high concentrations of chemotherapy in the retina and optic nerve as shown in preclinical models, preventing dissemination to the CNS. So far, after more than a decade of continuous use at major clinical centers around the world and after more than 200 articles published in the field, IVi and OAC have been proven safe without increasing the risk of metastatic dissemination. By eliminating the use of EBRT and systemic chemotherapy, these treatments have improved long-term survival by reducing the incidence of treatment-associated severe toxicities, the risk of secondary malignancies, and related deaths.
In contrast to this exceptional improvement in treatment outcomes, children with disseminated retinoblastoma have few therapeutic options, generally limited to high-dose chemotherapy, stem cell transplant, and local radiotherapy. Even worse is the scenario for patients with metastasis in the CNS, as these patients seldom survive even with intensive therapies. Thus, newer treatments and improved methods of targeting drug delivery to the CNS may improve outcomes. Examples of CNS-targeted routes include IT and IVt injection, which ensure direct delivery of chemotherapy to the CSF, circumventing the blood–brain barrier. OAC may also be useful in patients with orbital retinoblastoma with massive optic nerve and chiasmatic tumor involvement because of maximal local exposure to chemotherapy as shown in animal models. Future clinical assessments are necessary to determine the role of local chemotherapy delivery in disseminated retinoblastoma.
In all cases of intraocular and extraocular disease, there is a need for new therapies that are more effective and carry less risk of toxicity. New treatment modalities, namely, targeted therapies, immunotherapy, and oncolytic viruses are emerging as possible non-chemotherapeutic options. These novel treatments may further reduce the use of cytotoxic agents, potentially leading to even higher ocular preservation rates, reduced toxicities, and prevention of tumor dissemination.
Identifying high-risk features associated with tumor progression and metastasis by histopathological analysis of the enucleated eye is critical for selecting appropriate management. These approaches may soon be supplemented by ctDNA analysis, which may be an early and noninvasive prognostic biomarker of treatment response and risk of occult extraocular dissemination. In addition, ctDNA may be helpful in noninvasive genomic profiling, especially to identify patients with the subtype 2 molecular signature who have an increased risk of extraocular relapse.
In summary, retinoblastoma treatment has evolved over the last century, resulting in a striking change in the treatment paradigm of this ocular tumor. Advances in the knowledge of its tumor biology and drug response and the development of new routes of drug delivery promise to lead to additional new, more effective, and less toxic therapies in retinoblastoma.
Conceptualization, initial idea: PS, GC, and DHA. Manuscript preparation: PS, JHF, MBC, GC, AMC, and DHA. Supervision and manuscript review: PS, GC, and DHA. All authors listed have made a substantial, direct, and intellectual contribution to the work and approved it for publication.
This work was funded by the Fund for Ophthalmic Knowledge, NY, USA. The funders had no role in the decision to publish or preparation of the manuscript.
GC has received speaker honoraria from Ymabs, Tecnopharma, and Bayer.
The remaining authors declare that the research was conducted in the absence of any commercial or financial relationships that could be construed as a potential conflict of interest.
All claims expressed in this article are solely those of the authors and do not necessarily represent those of their affiliated organizations, or those of the publisher, the editors and the reviewers. Any product that may be evaluated in this article, or claim that may be made by its manufacturer, is not guaranteed or endorsed by the publisher.
1. Group GRS, Fabian ID, Abdallah E, Abdullahi SU, Abdulqader RA, Boubacar SA, et al. Global Retinoblastoma Presentation and Analysis by National Income Level. JAMA Oncol (2020) 6:685–95. doi: 10.1001/JAMAONCOL.2019.6716
2. Munier FL, Beck-Popovic M, Chantada GL, Cobrinik D, Kivelä TT, Lohmann D, et al. Conservative Management of Retinoblastoma: Challenging Orthodoxy Without Compromising the State of Metastatic Grace. “Alive, With Good Vision and No Comorbidity”. Prog Retin Eye Res (2019) 73:100764. doi: 10.1016/j.preteyeres.2019.05.005
3. Eng C, Li FP, Abramson DH, Ellsworth RM, Wong FL, Goldman MB, et al. Mortality From Second Tumors Among Long-Term Survivors of Retinoblastoma. J Natl Cancer Inst (1993) 85:1121–8. doi: 10.1093/jnci/85.14.1121
4. Shields CL, Bas Z, Tadepalli S, Dalvin LA, Rao R, Schwendeman R, et al. Long-Term (20-Year) Real-World Outcomes of Intravenous Chemotherapy (Chemoreduction) for Retinoblastoma in 964 Eyes of 554 Patients at a Single Centre. Br J Ophthalmol (2020) 104:1548–55. doi: 10.1136/bjophthalmol-2019-315572
5. Rodriguez-Galindo C, Wilson MW, Haik BG, Merchant TE, Billups CA, Shah N, et al. Treatment of Intraocular Retinoblastoma With Vincristine and Carboplatin. J Clin Oncol (2003) 21:2019–25. doi: 10.1200/JCO.2003.09.103
6. Abramson DH, Ellsworth RM, Tretter P, Adams K, Kitchin FD. Simultaneous Bilateral Radiation for Advanced Bilateral Retinoblastoma. Arch Ophthalmol (1981) 99:1763–6. doi: 10.1001/archopht.1981.03930020637003
7. Luna-Fineman S, Chantada G, Alejos A, Amador G, Barnoya M, Castellanos ME, et al. Delayed Enucleation With Neoadjuvant Chemotherapy in Advanced Intraocular Unilateral Retinoblastoma: AHOPCA II, a Prospective, Multi-Institutional Protocol in Central America. J Clin Oncol (2019) 37:2875–82. doi: 10.1200/JCO.18.00141
8. Wong JR, Morton LM, Tucker MA, Abramson DH, Seddon JM, Sampson JN, et al. Risk of Subsequent Malignant Neoplasms in Long-Term Hereditary Retinoblastoma Survivors After Chemotherapy and Radiotherapy. J Clin Oncol (2014) 32:3284–90. doi: 10.1200/JCO.2013.54.7844
9. Gombos DS, Hungerford J, Abramson DH, Kingston J, Chantada G, Dunkel IJ, et al. Secondary Acute Myelogenous Leukemia in Patients With Retinoblastoma. Is Chemotherapy a Factor? Ophthalmology (2007) 114:1378–83. doi: 10.1016/j.ophtha.2007.03.074
10. Chantada GL, Fandiño AC, Schvartzman E, Raslawski E, Schaiquevich P, Manzitti J. Impact of Chemoreduction for Conservative Therapy for Retinoblastoma in Argentina. Pediatr Blood Cancer (2014) 61:821–6. doi: 10.1002/pbc.24857
11. Qaddoumi I, Bass JK, Wu J, Billups CA, Wozniak AW, Merchant TE, et al. Carboplatin-Associated Ototoxicity in Children With Retinoblastoma. J Clin Oncol (2012) 30:1034–41. doi: 10.1200/JCO.2011.36.9744
12. Soliman SE, D’Silva CN, Dimaras H, Dzneladze I, Chan H, Gallie BL. Clinical and Genetic Associations for Carboplatin-Related Ototoxicity in Children Treated for Retinoblastoma: A Retrospective Noncomparative Single-Institute Experience. Pediatr Blood Cancer (2018) 65(5):e26931. doi: 10.1002/pbc.26931
13. Francis JH, Levin AM, Zabor EC, Gobin YP, Abramson DH. Ten-Year Experience With Ophthalmic Artery Chemosurgery: Ocular and Recurrence-Free Survival. PloS One (2018) 13:e0197081. doi: 10.1371/JOURNAL.PONE.0197081
14. Abramson DH, Marr BP, Brodie SE, Dunkel I, Palioura S, Gobin YP. Ophthalmic Artery Chemosurgery for Less Advanced Intraocular Retinoblastoma: Five Year Review. PloS One (2012) 7:e34120. doi: 10.1371/JOURNAL.PONE.0034120
15. Abramson DH, Dunkel IJ, Brodie SE, Kim JW, Gobin YP. A Phase I/II Study of Direct Intraarterial (Ophthalmic Artery) Chemotherapy With Melphalan for Intraocular Retinoblastoma. Initial Results. Ophthalmology (2008) 115(8):1398–404. doi: 10.1016/j.ophtha.2007.12.014
16. Abramson DH, Dunkel IJ, Brodie SE, Marr B, Gobin YP. Bilateral Superselective Ophthalmic Artery Chemotherapy for Bilateral Retinoblastoma: Tandem Therapy. Arch Ophthalmol (2010) 128:370–2. doi: 10.1001/archophthalmol.2010.7
17. Abramson DH, Fabius AWM, Francis JH, Marr BP, Dunkel IJ, Brodie SE, et al. Ophthalmic Artery Chemosurgery for Eyes With Advanced Retinoblastoma. Ophthalmic Genet (2017) 38:16–21. doi: 10.1080/13816810.2016.1244695
18. Abramson DH, Fabius AWM, Issa R, Francis JH, Marr BP, Dunkel IJ, et al. Advanced Unilateral Retinoblastoma: The Impact of Ophthalmic Artery Chemosurgery on Enucleation Rate and Patient Survival at MSKCC. PloS One (2015) 10(12):e0145436. doi: 10.1371/journal.pone.0145436
19. Francis JH, Roosipu N, Levin AM, Brodie SE, Dunkel IJ, Gobin YP, et al. Current Treatment of Bilateral Retinoblastoma: The Impact of Intraarterial and Intravitreous Chemotherapy. Neoplasia (United States) (2018) 20:757–63. doi: 10.1016/j.neo.2018.05.007
20. Munier FL, Mosimann P, Puccinelli F, Gaillard MC, Stathopoulos C, Houghton S, et al. First-Line Intra-Arterial Versus Intravenous Chemotherapy in Unilateral Sporadic Group D Retinoblastoma: Evidence of Better Visual Outcomes, Ocular Survival and Shorter Time to Success With Intra-Arterial Delivery From Retrospective Review of 20years of T. Br J Ophthalmol (2017) 101:1086–93. doi: 10.1136/bjophthalmol-2016-309298
21. Munier FL, Soliman S, Moulin AP, Gaillard MC, Balmer A, Beck-Popovic M. Profiling Safety of Intravitreal Injections for Retinoblastoma Using an Anti-Reflux Procedure and Sterilisation of the Needle Track. Br J Ophthalmol (2012) 96:1084–7. doi: 10.1136/bjophthalmol-2011-301016
22. Abramson DH, Ji X, Francis JH, Catalanotti F, Brodie SE, Habib L. Intravitreal Chemotherapy in Retinoblastoma: Expanded Use Beyond Intravitreal Seeds. Br J Ophthalmol (2019) 103:488–93. doi: 10.1136/bjophthalmol-2018-312037
23. Francis JH, Brodie SE, Marr B, Zabor EC, Mondesire-Crump I, Abramson DH. Efficacy and Toxicity of Intravitreous Chemotherapy for Retinoblastoma: Four-Year Experience. Ophthalmology (2017) 124:488–95. doi: 10.1016/j.ophtha.2016.12.015
24. Kaneko A, Suzuki S. Eye-Preservation Treatment of Retinoblastoma With Vitreous Seeding. Jpn J Clin Oncol (2003) 33:601–7. doi: 10.1093/jjco/hyg113
25. Abramson DH. Retinoblastoma in the 20th Century: Past Success and Future Challenges. The Weisenfeld Lecture. Investig Ophthalmol Vis Sci (2005) 46:2684–91. doi: 10.1167/iovs.04-1462
26. Kingston JE, Hungerford JL, Plowman PN. Chemotherapy in Metastatic Retinoblastoma. Ophthalmic Genet (1987) 8:69–72. doi: 10.3109/13816818709028519
27. Kingston JE, Hungerford JL, Madreperla SA, Plowman PN. Results of Combined Chemotherapy and Radiotherapy for Advanced Intraocular Retinoblastoma. Arch Ophthalmol (1996) 114:1339–43. doi: 10.1001/archopht.1996.01100140539004
28. Laurie NA, Gray JK, Zhang J, Leggas M, Relling M, Egorin M, et al. Topotecan Combination Chemotherapy in Two New Rodent Models of Retinoblastoma. Clin Cancer Res (2005) 11:7569–78. doi: 10.1158/1078-0432.CCR-05-0849
29. Fabius AWM, Wijsard M van H, van Leeuwen FE, Moll AC. Subsequent Malignant Neoplasms in Retinoblastoma Survivors. Cancers (2021) 13:1200. doi: 10.3390/CANCERS13061200
30. Habib LA, Francis JH, Fabius AWM, Gobin PY, Dunkel IJ, Abramson DH. Second Primary Malignancies in Retinoblastoma Patients Treated With Intra-Arterial Chemotherapy: The First 10 Years. Br J Ophthalmol (2018) 102:272–5. doi: 10.1136/BJOPHTHALMOL-2017-310328
31. Inomata M, Kaneko A. Chemosensitivity Profiles of Primary and Cultured Human Retinoblastoma Cells in a Human Tumor Clonogenic Assay. Jpn J Cancer Res (1987) 78:858–68.
32. Suzuki S, Yamane T, Mohri M, Kaneko A. Selective Ophthalmic Arterial Injection Therapy for Intraocular Retinoblastoma: The Long-Term Prognosis. Ophthalmology (2011) 118:2081–7. doi: 10.1016/j.ophtha.2011.03.013
33. Francis JH. Recent Advances in Retinoblastoma Treatment. Francis JH, Abramson DH, editors. Cham: Springer International Publishing (2015). doi: 10.1007/978-3-319-19467-7
34. Francis JH, Levin AM, Zabor EC, Gobin YP, Abramson DH. Ten-Year Experience With Ophthalmic Artery Chemosurgery: Ocular and Recurrence-Free Survival. PloS One (2018) 13:e0197081. doi: 10.1371/journal.pone.0197081
35. Requejo F, Marelli J, Ruiz Johnson A, Sampor C, Chantada G. The Technique of Superselective Ophthalmic Artery Chemotherapy for Retinoblastoma: The Garrahan Hospital Experience. Interv Neuroradiol (2018) 24:93–9. doi: 10.1177/1591019917738962
36. Venturi C, Bracco S, Cerase A, Cioni S, Galluzzi P, Gennari P, et al. Superselective Ophthalmic Artery Infusion of Melphalan for Intraocular Retinoblastoma: Preliminary Results From 140 Treatments. Acta Ophthalmol (2013) 91:335–42. doi: 10.1111/J.1755-3768.2011.02296.X
37. Oporto JI, Zúñiga P, Ossandón D, Zanolli M, Pérez V, López JP, et al. Intra-Arterial Chemotherapy for Retinoblastoma Treatment in Chile: Experience and Results 2013-2020. Arch Soc Esp Oftalmol (2021) 96:288–92. doi: 10.1016/J.OFTALE.2020.10.003
38. Batu Oto B, Sarıcı AM, Kızılkılıç O. Superselective Intra-Arterial Chemotherapy Treatment for Retinoblastoma: Clinical Experience From a Tertiary Referral Centre. Can J Ophthalmol (2020) 55:406–12. doi: 10.1016/J.JCJO.2020.04.009
39. Stenzel E, Göricke S, Temming P, Biewald E, Zülow S, Göbel J, et al. Feasibility of Intra-Arterial Chemotherapy for Retinoblastoma: Experiences in a Large Single Center Cohort Study. Neuroradiol (2019) 61:351–7. doi: 10.1007/S00234-019-02153-9
40. Fischer C, Petriccione M, Vitolano S, Guarini E, Davis ME, Dunkel IJ. The Effect of Ophthalmic Artery Chemosurgery on Immune Function in Retinoblastoma Patients: A Single Institution Retrospective Analysis. J Pediatr Hematol Oncol (2017) 39:555–9. doi: 10.1097/MPH.0000000000000968
41. Akella SS, Francis JH, Knezevic A, Ostrovnaya I, Gobin YP, Friedman D, et al. Growth Patterns of Survivors of Retinoblastoma Treated With Ophthalmic Artery Chemosurgery. PloS One (2018) 13(5):e0197052. doi: 10.1371/journal.pone.0197052
42. Abramson DH, Daniels AB, Marr BP, Francis JH, Brodie SE, Dunkel IJ, et al. Intra-Arterial Chemotherapy (Ophthalmic Artery Chemosurgery) for Group D Retinoblastoma. PloS One (2016) 11(1):e0146582. doi: 10.1371/journal.pone.0146582
43. Abramson DH, Gobin YP, Dunkel IJ, Francis JH. Successful Treatment of Massive Choroidal Invasion in Retinoblastoma With Intra-Arterial Chemotherapy (Ophthalmic Artery Chemosurgery). Ophthalmol Retin (2021) 5:936–9. doi: 10.1016/j.oret.2020.12.018
44. Rodriguez A, Zugbi S, Requejo F, Deu A, Sampor C, Sgroi M, et al. Combined High-Dose Intra-Arterial and Intrathecal Chemotherapy for the Treatment of a Case of Extraocular Retinoblastoma. Pediatr Blood Cancer (2018) 65(12):e27385. doi: 10.1002/pbc.27385
45. Yannuzzi NA, Francis JH, Marr BP, Belinsky I, Dunkel IJ, Gobin YP, et al. Enucleation vs Ophthalmic Artery Chemosurgery for Advanced Intraocular Retinoblastoma a Retrospective Analysis. JAMA Ophthalmol (2015) 133:1062–6. doi: 10.1001/jamaophthalmol.2015.2243
46. Abdelhakim AH, Francis JH, Marr BP, Gobin YP, Abramson DH, Brodie SE. Retinal Reattachment and ERG Recovery After Ophthalmic Artery Chemosurgery for Advanced Retinoblastoma in Eyes With Minimal Baseline Retinal Function. Br J Ophthalmol (2017) 101:623–8. doi: 10.1136/bjophthalmol-2016-308591
47. Schaiquevich P, Buitrago E, Ceciliano A, Fandino AC, Asprea M, Sierre S, et al. Pharmacokinetic Analysis of Topotecan After Superselective Ophthalmic Artery Infusion and Periocular Administration in a Porcine Model. Retina (2012) 32:387–95. doi: 10.1097/IAE.0b013e31821e9f8a
48. Taich P, Requejo F, Asprea M, Sgroi M, Gobin P, Abramson DH, et al. Topotecan Delivery to the Optic Nerve After Ophthalmic Artery Chemosurgery. PloS One (2016) 11:151343. doi: 10.1371/JOURNAL.PONE.0151343
49. Houghton PJ, Cheshire PJ, Hallman JD, Lutz L, Friedman HS, Danks MK, et al. Efficacy of Topoisomerase I Inhibitors, Topotecan and Irinotecan, Administered at Low Dose Levels in Protracted Schedules to Mice Bearing Xenografts of Human Tumors. Cancer Chemother Pharmacol (1995) 36:393–403. doi: 10.1007/BF00686188
50. Panetta JC, Schaiquevich P, Santana VM, Stewart CF. Using Pharmacokinetic and Pharmacodynamic Modeling and Simulation to Evaluate Importance of Schedule in Topotecan Therapy for Pediatric Neuroblastoma. Clin Cancer Res (2008) 14:318–25. doi: 10.1158/1078-0432.CCR-07-1243
51. Daniels AB, Froehler MT, Kaczmarek JV, Bogan CM, Santapuram PR, Pierce JM, et al. Efficacy, Toxicity, and Pharmacokinetics of Intra-Arterial Chemotherapy Versus Intravenous Chemotherapy for Retinoblastoma in Animal Models and Patients. Transl Vis Sci Technol (2021) 10:10–0. doi: 10.1167/tvst.10.11.10
52. Daniels AB, Froehler MT, Pierce JM, Nunnally AH, Calcutt MW, Bridges TM, et al. Pharmacokinetics, Tissue Localization, Toxicity, and Treatment Efficacy in the First Small Animal (Rabbit) Model of Intra-Arterial Chemotherapy for Retinoblastoma. Invest Ophthalmol Vis Sci (2018) 59:446–54. doi: 10.1167/IOVS.17-22302
53. Schaiquevich P, Buitrago E, Taich P, Torbidoni A, Ceciliano A, Fandino A, et al. Pharmacokinetic Analysis of Melphalan After Superselective Ophthalmic Artery Infusion in Preclinical Models and Retinoblastoma Patients. Investig Ophthalmol Vis Sci (2012) 53:4205–12. doi: 10.1167/iovs.12-9501
54. Dunkel IJ, Shi W, Salvaggio K, Marr BP, Brodie SE, Gobin YP, et al. Risk Factors for Severe Neutropenia Following Intra-Arterial Chemotherapy for Intra-Ocular Retinoblastoma. PloS One (2014) 9:e108692. doi: 10.1371/journal.pone.0108692
55. Taich P, Ceciliano A, Buitrago E, Sampor C, Fandino A, Villasante F, et al. Clinical Pharmacokinetics of Intra-Arterial Melphalan and Topotecan Combination in Patients With Retinoblastoma. Ophthalmology (2014) 121:889–97. doi: 10.1016/j.ophtha.2013.10.045
56. Santana VM, Zamboni WC, Kirstein MN, Tan M, Liu T, Gajjar A, et al. A Pilot Study of Protracted Topotecan Dosing Using a Pharmacokinetically Guided Dosing Approach in Children With Solid Tumors. Clin Cancer Res (2003) 9:633–40.
57. Munier FL, Beck-Popovic M, Balmer A, Gaillard MC, Bovey E, Binaghi S. Occurrence of Sectoral Choroidal Occlusive Vasculopathy and Retinal Arteriolar Embolization After Superselective Ophthalmic Artery Chemotherapy for Advanced Intraocular Retinoblastoma. Retina (2011) 31:566–73. doi: 10.1097/IAE.0b013e318203c101
58. Shields CL, Bianciotto CG, Jabbour P, Griffin GC, Ramasubramanian A, Rosenwasser R, et al. Intra-Arterial Chemotherapy for Retinoblastoma: Report No. 2, Treatment Complications. Arch Ophthalmol (Chicago Ill 1960) (2011) 129:1407–15. doi: 10.1001/ARCHOPHTHALMOL.2011.151
59. Scharoun JH, Han JH, Gobin YP. Anesthesia for Ophthalmic Artery Chemosurgery. Anesthesiology (2017) 126:165–72. doi: 10.1097/ALN.0000000000001381
60. De La Huerta I, Seider MI, Hetts SW, Damato BE. Delayed Cerebral Infarction Following Intra-Arterial Chemotherapy for Retinoblastoma. JAMA Ophthalmol (2016) 134:712–4. doi: 10.1001/JAMAOPHTHALMOL.2016.0025
61. Torbidoni AV, Laurent VE, Sampor C, Ottaviani D, Vazquez V, Gabri MR, et al. Association of Cone-Rod Homeobox Transcription Factor Messenger RNA With Pediatric Metastatic Retinoblastoma. JAMA Ophthalmol (2015) 133(7):805–12. doi: 10.1001/JAMAOPHTHALMOL.2015.0900
62. Abramson DH, Gobin YP, Francis JH. Orbital Retinoblastoma Treated With Intra-Arterial Chemotherapy. Ophthalmology (2021) 128:1437. doi: 10.1016/j.ophtha.2021.03.018
63. Grigorovski N, Macedo C, Sampor C, Mattosinho C, Teixeira L, Fandiño A, et al. Multimodality Treatment Including Intra-Arterial Chemotherapy for Disseminated Retinoblastoma. In: International Society for Genetic Eye Diseases & Retinoblastoma 2021 Meeting. Lausanne. (2021) p. 162.
64. Bosaleh A, Sampor C, Solernou V, Fandiño A, Domínguez J, De Dávila MTG, et al. Outcome of Children With Retinoblastoma and Isolated Choroidal Invasion. Arch Ophthalmol (2012) 130:724–9. doi: 10.1001/archophthalmol.2012.567
65. Francis JH, Abramson DH, Ji X, Shields CL, Teixeira LF, Schefler AC, et al. Risk of Extraocular Extension in Eyes With Retinoblastoma Receiving Intravitreous Chemotherapy. JAMA Ophthalmol (2017) 135:1426–9. doi: 10.1001/jamaophthalmol.2017.4600
66. Pascual-Pasto G, Bazan-Peregrino M, Olaciregui NG, Restrepo-Perdomo CA, Mato-Berciano A, Ottaviani D, et al. Therapeutic Targeting of the RB1 Pathway in Retinoblastoma With the Oncolytic Adenovirus VCN-01. Sci Transl Med (2019) 11(476):eaat9321. doi: 10.1126/scitranslmed.aat9321
67. Winter U, Nicolas M, Sgroi M, Sampor C, Torbidoni A, Fandiño A, et al. Assessment of Retinoblastoma RNA Reflux After Intravitreal Injection of Melphalan. Br J Ophthalmol (2018) 102:415–8. doi: 10.1136/bjophthalmol-2017-310574
68. Francis JH, Schaiquevich P, Buitrago E, Del Sole MJ, Zapata G, Croxatto JO, et al. Local and Systemic Toxicity of Intravitreal Melphalan for Vitreous Seeding in Retinoblastoma: A Preclinical and Clinical Study. Ophthalmology (2014) 121:1810–7. doi: 10.1016/j.ophtha.2014.03.028
69. Francis JH, Abramson DH, Gaillard MC, Marr BP, Beck-Popovic M, Munier FL. The Classification of Vitreous Seeds in Retinoblastoma and Response to Intravitreal Melphalan. Ophthalmology (2015) 122:1173–9. doi: 10.1016/j.ophtha.2015.01.017
70. Francis JH, Marr BP, Abramson DH. Classification of Vitreous Seeds in Retinoblastoma Correlations With Patient, Tumor, and Treatment Characteristics. Ophthalmology (2016) 123:1601–5. doi: 10.1016/j.ophtha.2016.02.036
71. Munier FL. Classification and Management of Seeds in Retinoblastomaellsworth Lecture Ghent August 24th 2013. Ophthalmic Genet (2014) 35:193–207. doi: 10.3109/13816810.2014.973045
72. Nadelmann J, Francis JH, Brodie SE, Muca E, Abramson DH. Is Intravitreal Topotecan Toxic to Retinal Function? Br J Ophthalmol (2021) 105:1016–8. doi: 10.1136/bjophthalmol-2020-316588
73. Smith SJ, Pulido JS, Salomão DR, Smith BD, Mohney B. Combined Intravitreal and Subconjunctival Carboplatin for Retinoblastoma With Vitreous Seeds. Br J Ophthalmol (2012) 96:1073–7. doi: 10.1136/bjophthalmol-2011-300829
74. Buitrago E, Winter U, Williams G, Asprea M, Chantada G, Schaiquevich P. Pharmacokinetics of Melphalan After Intravitreal Injection in a Rabbit Model. J Ocul Pharmacol Ther (2016) 32:230–5. doi: 10.1089/jop.2015.0088
75. Bogan CM, Kaczmarek JV, Pierce JM, Chen SC, Boyd KL, Calcutt MW, et al. Evaluation of Intravitreal Topotecan Dose Levels, Toxicity and Efficacy for Retinoblastoma Vitreous Seeds: A Preclinical and Clinical Study. Br J Ophthalmol (2022) 106:288–96. doi: 10.1136/bjophthalmol-2020-318529
76. Hsieh T, Liao A, Francis JH, Lavery JA, Mauguen A, Brodie SE, et al. Comparison of Efficacy and Toxicity of Intravitreal Melphalan Formulations for Retinoblastoma. PloS One (2020) 15:e0235016. doi: 10.1371/journal.pone.0235016
77. Liao A, Hsieh T, Francis JH, Lavery JA, Mauguen A, Brodie SE, et al. Toxicity and Efficacy of Intravitreal Melphalan for Retinoblastoma: 25 µg Versus 30 µg. Retina (2021) 41:208–12. doi: 10.1097/IAE.0000000000002782
78. Bogan CM, Pierce JM, Doss SD, Tao YK, Chen S, Chiann, Boyd KL, et al. Intravitreal Melphalan Hydrochloride vs Propylene Glycol-Free Melphalan for Retinoblastoma Vitreous Seeds: Efficacy, Toxicity and Stability in Rabbits Models and Patients. Exp Eye Res (2021) 204:e108439. doi: 10.1016/j.exer.2021.108439
79. Süsskind D, Hagemann U, Schrader M, Januschowski K, Schnichels S, Aisenbrey S. Toxic Effects of Melphalan, Topotecan and Carboplatin on Retinal Pigment Epithelial Cells. Acta Ophthalmol (2016) 94:471–8. doi: 10.1111/aos.12990
80. Buitrago E, Lagomarsino E, Mato G, Schaiquevich P. Stability of Melphalan Solution for Intravitreal Injection for Retinoblastoma. JAMA Ophthalmol (2014) 132:1372–3. doi: 10.1001/jamaophthalmol.2014.2324
81. Singh R, Chen J, Miller T, Bergren M, Mallik R. Solution Stability of Captisol-Stabilized Melphalan (Evomela) Versus Propylene Glycol-Based Melphalan Hydrochloride Injection. Pharm Dev Technol (2018) 23:1024–9. doi: 10.1080/10837450.2016.1265557
82. Dalvin LA, Kumari M, Essuman VA, Shohelly Shipa S, Ancona-Lezama D, Lucio-Alvarez JA, et al. Primary Intra-Arterial Chemotherapy for Retinoblastoma in the Intravitreal Chemotherapy Era: Five Years of Experience. Ocul Oncol Pathol (2019) 5:139–46. doi: 10.1159/000491580
83. Kiratli H, Koç I, Öztürk E, Varan A, Akyüz C. Comparison of Intravitreal Melphalan With and Without Topotecan in the Management of Vitreous Disease in Retinoblastoma. Jpn J Ophthalmol (2020) 64:351–8. doi: 10.1007/s10384-020-00743-2
84. Rao R, Honavar SG, Mulay K, Reddy VAP. Eye Salvage in Diffuse Anterior Retinoblastoma Using Systemic Chemotherapy With Periocular and Intravitreal Topotecan. J AAPOS (2018) 22:235–7.e2. doi: 10.1016/j.jaapos.2017.11.013
85. Tubergen DG, Stewart CF, Pratt CB, Zamboni WC, Winick N, Santana VM, et al. Phase I Trial and Pharmacokinetic (PK) and Pharmacodynamics (PD) Study of Topotecan Using a Five-Day Course in Children With Refractory Solid Tumors: A Pediatric Oncology Group Study. J Pediatr Hematol Oncol (1996) 18:352–61. doi: 10.1097/00043426-199611000-00004
86. Del Sole MJ, Clausse M, Nejamkin P, Cancela MB, Del Río M, Zugbi S, et al. Ocular and Systemic Safety of Repeated Intravitreal High Doses of Topotecan in Rabbits: Implications in Retinoblastoma Treatment. In: In International Society for Genetic Eye Diseases & Retinoblastoma 2021 Meeting. Lausanne, Switzerland. (2021). p. 134–5.
87. Munier FL, Moulin A, Gaillard MC, Bongiovanni M, Decembrini S, Houghton S, et al. Intracameral Chemotherapy for Globe Salvage in Retinoblastoma With Secondary Anterior Chamber Invasion. Ophthalmology (2018) 125:615–7. doi: 10.1016/j.ophtha.2017.11.010
88. Brennan RC, Qaddoumi I, Mao S, Wu J, Billups CA, Stewart CF, et al. Ocular Salvage and Vision Preservation Using a Topotecan-Based Regimen for Advanced Intraocular Retinoblastoma. J Clin Oncol (2017) 35:72–7. doi: 10.1200/JCO.2016.69.2996
89. Abramson DH, Frank CM, Dunkel IJ. A Phase I/II Study of Subconjunctival Carboplatin for Intraocular Retinoblastoma. Ophthalmology (1999) 106:1947–50. doi: 10.1016/S0161-6420(99)90406-2
90. Kang KB, Francis JH, Abramson DH. What Do We Know About Intraocular Carboplatin? J Ocul Pharmacol Ther (2014) 30:688–90. doi: 10.1089/jop.2014.0051
91. Carcaboso AM, Bramuglia GF, Chantada GL, Fandiño AC, Chiappetta DA, De Davila MTG, et al. Topotecan Vitreous Levels After Periocular or Intravenous Delivery in Rabbits: An Alternative for Retinoblastoma Chemotherapy. Investig Ophthalmol Vis Sci (2007) 48:3761–7. doi: 10.1167/iovs.06-1152
92. National Library of Medicine. Topotecan Episcleral Plaque for Treatment of Retinoblastoma - Full Text View (2020). ClinicalTrials.gov. Available at: https://clinicaltrials.gov/ct2/show/NCT04428879 (Accessed October 26, 2021).
93. Schvartzman E, Chantada G, Fandiño A, de Dávila MT, Raslawski E, Manzitti J. Results of a Stage-Based Protocol for the Treatment of Retinoblastoma. J Clin Oncol (1996) 14:1532–6. doi: 10.1200/JCO.1996.14.5.1532
94. Potter SLP, Berg S, Ingle AM, Krailo M, Adamson PC, Blaney SM. Phase 2 Clinical Trial of Intrathecal Topotecan in Children With Refractory Leptomeningeal Leukemia: A Children’s Oncology Group Trial (P9962). Pediatr Blood Cancer (2012) 58:362–5. doi: 10.1002/pbc.23317
95. Dimaras H, Hon E, Budning A, Doyle JJ, Halliday W, Gallie BL, et al. Retinoblastoma CSF Metastasis Cured by Multimodality Chemotherapy Without Radiation. Ophthalmic Genet (2009) 30:121–6. doi: 10.1080/13816810902988780
96. Sampor C, Chantada G. Treatment Protocol for Non-Metastatic Unilateral Retinoblastoma (Rbgalop2) (2018). Available at: https://clinicaltrials.gov/ct2/show/NCT03475121 (Accessed October 27, 2021).
97. Sampor C, Correa Llanos MG, Oller A, Fandiño A, Schaiquevich P, Aschero R, et al. Intrathecal Topotecan as Part of Retinoblastoma Treatment. Pediatr Blood Cancer (2019) S74:e27989. doi: 10.1002/pbc.27989
98. Cancela MB, Zugbi S, Winter U, Martinez AL, Sampor C, Sgroi M, et al. A Decision Process for Drug Discovery in Retinoblastoma. Investig New Drugs (2020) 39:426–41. doi: 10.1007/S10637-020-01030-0
99. Berry JL, Munier FL, Gallie BL, Polski A, Shah S, Shields CL, et al. Response Criteria for Intraocular Retinoblastoma: RB-RECIST. Pediatr Blood Cancer (2021) 68(5):e28964. doi: 10.1002/pbc.28964
100. Schwermer M, Hiber M, Dreesmann S, Rieb A, Theißen J, Herold T, et al. Comprehensive Characterization of RB1 Mutant and MYCN Amplified Retinoblastoma Cell Lines. Exp Cell Res (2019) 375:92–9. doi: 10.1016/J.YEXCR.2018.12.018
101. Bond WS, Akinfenwa PY, Perlaky L, Hurwitz MY, Hurwitz RL, Chévez-Barrios P. Tumorspheres But Not Adherent Cells Derived From Retinoblastoma Tumors Are of Malignant Origin. PloS One (2013) 8:e63519. doi: 10.1371/JOURNAL.PONE.0063519
102. Winter U, Winter U, Ganiewich D, Ganiewich D, Ottaviani D, Zugbi S, et al. Genomic and Transcriptomic Tumor Heterogeneity in Bilateral Retinoblastoma. JAMA Ophthalmol (2020) 138:569–74. doi: 10.1001/jamaophthalmol.2020.0427
103. Inomata M, Kaneko A, Saijo N, Tokura S. Culture of Retinoblastoma Cells From Clinical Specimens: Growth-Promoting Effect of 2-Mercaptoethanol. J Cancer Res Clin Oncol (1994) 120:149–55. doi: 10.1007/BF01202193
104. Ravishankar H, Mangani AS, Shankar MB, Joshi M, Devasena T, Parameswaran S, et al. Characterization of NCC-RbC-51, an RB Cell Line Isolated From a Metastatic Site. Histochem Cell Biol (2020) 153:101–9. doi: 10.1007/s00418-019-01832-1
105. Zugbi S, Ganiewich D, Bhattacharyya A, Aschero R, Ottaviani D, Sampor C, et al. Clinical, Genomic, and Pharmacological Study of MYCN-Amplified RB1 Wild-Type Metastatic Retinoblastoma. Cancers (Basel) (2020) 12:1–20. doi: 10.3390/cancers12092714
106. Pascual-Pasto G, Olaciregui NG, Vila-Ubach M, Paco S, Monterrubio C, Rodriguez E, et al. Preclinical Platform of Retinoblastoma Xenografts Recapitulating Human Disease and Molecular Markers of Dissemination. Cancer Lett (2016) 380:10–9. doi: 10.1016/j.canlet.2016.06.012
107. Bond WS, Wadhwa L, Perlaky L, Penland RL, Hurwitz MY, Hurwitz RL, et al. Establishment and Propagation of Human Retinoblastoma Tumors in Immune Deficient Mice. J Vis Exp (2011) (54):2644. doi: 10.3791/2644
108. Antczak C, Kloepping C, Radu C, Genski T, Müller-Kuhrt L, Siems K, et al. Revisiting Old Drugs as Novel Agents for Retinoblastoma: In Vitro and In Vivo Antitumor Activity of Cardenolides. Investig Ophthalmol Vis Sci (2009) 50:3065–73. doi: 10.1167/iovs.08-3158
109. Patel M, Paulus YM, Gobin YP, Djaballah H, Marr B, Dunkel IJ, et al. Intra-Arterial and Oral Digoxin Therapy for Retinoblastoma. Ophthalmic Genet (2011) 32:147–50. doi: 10.3109/13816810.2010.544530
110. Khodabande A, Ghassemi F, Asadi Amoli F, Riazi-Esfahani H, Mahmoudzadeh R, Mehrpour M, et al. Ocular Safety of Repeated Intravitreal Injections of Carboplatin and Digoxin: A Preclinical Study on the Healthy Rabbits. Pharmacol Res Perspect (2021) 9:814. doi: 10.1002/prp2.814
111. Winter U, Buitrago E, Mena HA, Del Sole MJ, Laurent V, Negrotto S, et al. Pharmacokinetics, Safety, and Efficacy of Intravitreal Digoxin in Preclinical Models for Retinoblastoma. Investig Ophthalmol Vis Sci (2015) 56:4382–93. doi: 10.1167/iovs.14-16239
112. Aubry A, Pearson JD, Huang K, Livne-bar I, Ahmad M, Jagadeesan M, et al. Functional Genomics Identifies New Synergistic Therapies for Retinoblastoma. Oncogene (2020) 39:5338–57. doi: 10.1038/s41388-020-1372-7
113. Wang H, Bauzon F, Ji P, Xu X, Sun D, Locker J, et al. Skp2 Is Required for Survival of Aberrantly Proliferating Rb1-Deficient Cells and for Tumorigenesis in Rb1+/mice. Nat Genet (2010) 42:83–8. doi: 10.1038/ng.498
114. Xu XL, Singh HP, Wang L, Qi DL, Poulos BK, Abramson DH, et al. Rb Suppresses Human Cone-Precursor-Derived Retinoblastoma Tumours. Nature (2014) 514:385–8. doi: 10.1038/nature13813
115. Aubry A, Yu T, Bremner R. Preclinical Studies Reveal MLN4924 Is a Promising New Retinoblastoma Therapy. Cell Death Discov (2020) 6:1–12. doi: 10.1038/s41420-020-0237-8
116. Foster JH, Barbieri E, Zhang L, Scorsone KA, Moreno-smith M, Zage P, et al. The Anti-Tumor Activity of the Nedd8 Inhibitor Pevonedistat in Neuroblastoma. Int J Mol Sci (2021) 22(12):6565. doi: 10.3390/ijms22126565
117. Pavicic E. Pevonedistat With VXLD Chemotherapy for Adolescent/Young Adults With Relapsed/Refractory ALL or Lymphoblastic NHL - Full Text View - ClinicalTrials.Gov. Available at: https://clinicaltrials.gov/ct2/show/NCT03349281?term=pevonedistat&age=0&draw=2&rank=3 (Accessed October 28, 2021).
118. Collin J, Queen R, Zerti D, Steel DH, Bowen C, Parulekar M, et al. Dissecting the Transcriptional and Chromatin Accessibility Heterogeneity of Proliferating Cone Precursors in Human Retinoblastoma Tumors by Single Cell Sequencing—Opening Pathways to New Therapeutic Strategies? Invest Ophthalmol Vis Sci (2021) 62:18–8. doi: 10.1167/IOVS.62.6.18
119. Corson TW, Gallie BL. One Hit, Two Hits, Three Hits, More? Genomic Changes in the Development of Retinoblastoma. Genes Chromosom Cancer (2007) 46:617–34. doi: 10.1002/gcc.20457
120. Laurie NA, Donovan SL, Shih C-S, Zhang J, Mills N, Fuller C, et al. Inactivation of the P53 Pathway in Retinoblastoma. Nature (2006) 444:61–6. doi: 10.1038/nature05194
121. Burgess A, Chia KM, Haupt S, Thomas D, Haupt Y, Lim E. Clinical Overview of MDM2/X-Targeted Therapies. Front Oncol (2016) 6:7. doi: 10.3389/fonc.2016.00007
122. Brennan RC, Federico S, Bradley C, Zhang J, Flores-Otero J, Wilson M, et al. Targeting the P53 Pathway in Retinoblastoma With Subconjunctival Nutlin-3a. Cancer Res (2011) 71:4205–13. doi: 10.1158/0008-5472.CAN-11-0058
123. Guo Y, Pajovic S, Gallie BL. Expression of P14arf, MDM2, and MDM4 in Human Retinoblastoma. Biochem Biophys Res Commun (2008) 375:1–5. doi: 10.1016/j.bbrc.2008.07.055
124. Shulman DS. Phase 1 Study of the Dual MDM2/MDMX Inhibitor ALRN-6924 in Pediatric Cancer - Full Text View - ClinicalTrials.Gov. Available at: https://clinicaltrials.gov/ct2/show/NCT03654716?term=MDM2&age=0&draw=2&rank=1 (Accessed October 27, 2021).
125. Elison JR, Cobrinik D, Claros N, Abramson DH, Lee TC. Small Molecule Inhibition of HDM2 Leads to P53-Mediated Cell Death in Retinoblastoma Cells. Arch Ophthalmol (2006) 124:1269–75. doi: 10.1001/ARCHOPHT.124.9.1269
126. Zhang J, Benavente CA, McEvoy J, Flores-Otero J, Ding L, Chen X, et al. A Novel Retinoblastoma Therapy From Genomic and Epigenetic Analyses. Nature (2012) 481:329–34. doi: 10.1038/nature10733
127. Newland A, McDonald V. Fostamatinib: A Review of Its Clinical Efficacy and Safety in the Management of Chronic Adult Immune Thrombocytopenia. (2020) 12(18):1325–40. doi: 10.2217/IMT-2020-0215
128. Pritchard EM, Stewart E, Zhu F, Bradley C, Griffiths L, Yang L, et al. Pharmacokinetics and Efficacy of the Spleen Tyrosine Kinase Inhibitor R406 After Ocular Delivery for Retinoblastoma. Pharm Res (2014) 31:3060–72. doi: 10.1007/S11095-014-1399-Y
129. U.S. Food and Drug Administration website. Rigel Pharmaceuticals, Inc TAVALISSE™ (Fostamatinib Disodium Hexahydrate) . Available at: https://www.accessdata.fda.gov/drugsatfda_docs/label/2018/209299lbl.pdf (Accessed October 27, 2021).
130. Laurent VE, Otero LL, Vazquez V, Camarero S, Gabri MR, Labraga M, et al. Optimization of Molecular Detection of GD2 Synthase mRNA in Retinoblastoma. Mol Med Rep (2010) 3:253–9. doi: 10.3892/mmr-00000248
131. Chantada GL, Rossi J, Casco F, Fandiño A, Scopinaro M, De Dávila MTG, et al. An Aggressive Bone Marrow Evaluation Including Immunocytology With GD2 for Advanced Retinoblastoma. J Pediatr Hematol Oncol (2006) 28:369–73. doi: 10.1097/00043426-200606000-00009
132. Yu AL, Gilman AL, Ozkaynak MF, London WB, Kreissman SG, Chen HX, et al. Anti-GD2 Antibody With GM-CSF, Interleukin-2, and Isotretinoin for Neuroblastoma. N Engl J Med (2010) 363:1324–34. doi: 10.1056/nejmoa0911123
133. Mora J. Dinutuximab for the Treatment of Pediatric Patients With High-Risk Neuroblastoma. Expert Rev Clin Pharmacol (2016) 9:647–53. doi: 10.1586/17512433.2016.1160775
134. Kramer K, Humm JL, Souweidane MM, Zanzonico PB, Dunkel IJ, Gerald WL, et al. Phase I Study of Targeted Radioimmunotherapy for Leptomeningeal Cancers Using Intra-Ommaya 131-I-3f8. J Clin Oncol (2007) 25:5465–70. doi: 10.1200/JCO.2007.11.1807
135. Cacciavillano W, Sampor C, Venier C, Gabri MR, de Dávila MTG, Galluzzo ML, et al. Chantada GL. A Phase I Study of the Anti-Idiotype Vaccine Racotumomab in Neuroblastoma and Other Pediatric Refractory Malignancies. Pediatr Blood Cancer (2015) 62:2120–4. doi: 10.1002/pbc.25631
136. Andersch L, Radke J, Klaus A, Schwiebert S, Winkler A, Schumann E, et al. CD171- and GD2-Specific CAR-T Cells Potently Target Retinoblastoma Cells in Preclinical In Vitro Testing. BMC Cancer (2019) 19:1–17. doi: 10.1186/s12885-019-6131-1
137. Wang K, Chen Y, Ahn S, Zheng M, Landoni E, Dotti G, et al. GD2-Specific CAR T Cells Encapsulated in an Injectable Hydrogel Control Retinoblastoma and Preserve Vision. Nat Cancer (2020) 1:990–7. doi: 10.1038/s43018-020-00119-y
138. Sujjitjoon J, Sayour E, Tsao ST, Uiprasertkul M, Sanpakit K, Buaboonnam J, et al. GD2-Specific Chimeric Antigen Receptor-Modified T Cells Targeting Retinoblastoma – Assessing Tumor and T Cell Interaction. Transl Oncol (2021) 14:100971. doi: 10.1016/j.tranon.2020.100971
139. Gery I, Caspi RR. Tolerance Induction in Relation to the Eye. Front Immunol (2018) 9:2304. doi: 10.3389/fimmu.2018.02304
140. Liu J, Ottaviani D, Sefta M, Desbrousses C, Chapeaublanc E, Aschero R, et al. A High-Risk Retinoblastoma Subtype With Stemness Features, Dedifferentiated Cone States and Neuronal/Ganglion Cell Gene Expression. Nat Commun (2021) 12:1–20. doi: 10.1038/s41467-021-25792-0
141. Singh L, Singh MK, Rizvi MA, Bakhshi S, Meel R, Lomi N, et al. Clinical Relevance of the Comparative Expression of Immune Checkpoint Markers With the Clinicopathological Findings in Patients With Primary and Chemoreduced Retinoblastoma. Cancer Immunol Immunother (2020) 69:1087–99. doi: 10.1007/s00262-020-02529-4
142. Miracco C, Toti P, Gelmi MC, Aversa S, Baldino G, Galluzzi P, et al. Retinoblastoma Is Characterized by a Cold, CD8+ Cell Poor, PD-L1– Microenvironment, Which Turns Into Hot, CD8+ Cell Rich, PD-L1+ After Chemotherapy. Invest Ophthalmol Vis Sci (2021) 62(2):6. doi: 10.1167/IOVS.62.2.6
143. Singh L, Kashyap S, Pushker N, Bakhshi S, Sen S, Rizvi MA. Expression Pattern of Immune Checkpoints Programmed Death (PD-1) and Programmed Death-Ligand (PD-L1) in Retinoblastoma and Its Prognostic Significance. Ann Oncol (2017) 28:xi11. doi: 10.1093/annonc/mdx711.017
144. Ganesan B, Parameswaran S, Sharma A, Krishnakumar S. Clinical Relevance of B7H3 Expression in Retinoblastoma. Sci Rep (2020) 10:1–10. doi: 10.1038/s41598-020-67101-7
145. Hurwitz RL, Chintagumpala M, Mieler WF, Paysse E, Boniuk M, Hurwitz MY, et al. 819. Gene Therapy for Retinoblastoma Using AdV/TK Followed by Ganciclovir: Report of a Clinical Trial. Mol Ther (2003) 7:S316. doi: 10.1016/s1525-0016(16)41261-x
146. Song X, Zhou Y, Jia R, Xu X, Wang H, Hu J, et al. Inhibition of Retinoblastoma In Vitro and In Vivo With Conditionally Replicating Oncolytic Adenovirus H101. Investig Ophthalmol Vis Sci (2010) 51:2626–35. doi: 10.1167/iovs.09-3516
147. Jansen RW, De Jong MC, Kooi IE, Sirin S, Göricke S, Brisse HJ, et al. MR Imaging Features of Retinoblastoma: Association With Gene Expression Profiles. Radiology (2018) 288:506–15. doi: 10.1148/radiol.2018172000
148. Abramson DH, Mandelker D, Francis JH, Dunkel IJ, Brannon AR, Benayed R, et al. Retrospective Evaluation of Somatic Alterations in Cell-Free DNA From Blood in Retinoblastoma. Ophthalmol Sci (2021) 1:100015. doi: 10.1016/j.xops.2021.100015
Keywords: innovative treatments, intra-arterial chemotherapy, intravitreal injections, retinoblastoma, pharmacology, chemotherapy, adenovirus
Citation: Schaiquevich P, Francis JH, Cancela MB, Carcaboso AM, Chantada GL and Abramson DH (2022) Treatment of Retinoblastoma: What Is the Latest and What Is the Future. Front. Oncol. 12:822330. doi: 10.3389/fonc.2022.822330
Received: 25 November 2021; Accepted: 24 February 2022;
Published: 01 April 2022.
Edited by:
Timothy W. Corson, Indiana University Bloomington, United StatesReviewed by:
Dong Hyun Jo, Seoul National University Hospital, South KoreaCopyright © 2022 Schaiquevich, Francis, Cancela, Carcaboso, Chantada and Abramson. This is an open-access article distributed under the terms of the Creative Commons Attribution License (CC BY). The use, distribution or reproduction in other forums is permitted, provided the original author(s) and the copyright owner(s) are credited and that the original publication in this journal is cited, in accordance with accepted academic practice. No use, distribution or reproduction is permitted which does not comply with these terms.
*Correspondence: David H. Abramson, YWJyYW1zb2RAbXNrY2Mub3Jn
†These authors share senior authorship
Disclaimer: All claims expressed in this article are solely those of the authors and do not necessarily represent those of their affiliated organizations, or those of the publisher, the editors and the reviewers. Any product that may be evaluated in this article or claim that may be made by its manufacturer is not guaranteed or endorsed by the publisher.
Research integrity at Frontiers
Learn more about the work of our research integrity team to safeguard the quality of each article we publish.