- 1Department of Pediatrics, Tongji Medical College, Union Hospital, Huazhong University of Science and Technology, Wuhan, China
- 2Department of Health and Nursing, Nanfang College of Sun Yat-sen University, Guangzhou, China
Chimeric antigen receptor T cell (CAR-T cell) therapy has shown impressive success in the treatment of hematological malignancies, but the systemic toxicity and complex manufacturing process of current autologous CAR-T cell therapy hinder its broader applications. Universal CAR-T cells have been developed to simplify the production process through isolation and editing of allogeneic T cells from healthy persons, but the allogeneic CAR-T cells have recently encountered safety concerns, and clinical trials have been halted by the FDA. Thus, there is an urgent need to seek new ways to overcome the barriers of current CAR-T cell therapy. In-vivo CAR-T cells induced by nanocarriers loaded with CAR-genes and gene-editing tools have shown efficiency for regressing leukemia and reducing systemic toxicity in a mouse model. The in-situ programming of autologous T-cells avoids the safety concerns of allogeneic T cells, and the manufacture of nanocarriers can be easily standardized. Therefore, the in-vivo induced CAR-T cells can potentially overcome the abovementioned limitations of current CAR-T cell therapy. Here, we provide a review on CAR structures, gene-editing tools, and gene delivery techniques applied in immunotherapy to help design and develop new in-vivo induced CAR-T cells.
Introduction
Chimeric antigen receptor T cell (CAR-T cell) therapy is a new cell immunotherapy technique that incorporates synthetic receptors into T cells that recognize and kill tumor cells with a cognate targeting ligand (1, 2). CAR-T cell therapy has demonstrated unprecedented response rates in patients with B cell lymphoma since the first approval of CD19-targeted CAR-T cells in the USA (1, 3–5). However, along with the remarkable achievements of CAR-T cell therapy, many systemic toxicities, such as cytokine release syndrome (CRS) and neurotoxicity, have also been frequently reported (2, 6–8). Additionally, the complex manufacturing process of CAR-T cells limits the broader applications of this therapeutic method as a standard clinical treatment (2, 9–11). Therefore, there is an exigent need to develop a new paradigm of CAR-T cells to overcome these barriers and allow this therapeutic method to benefit more patients. To simplify the complex manufacturing process of CAR-T cells, universal allogeneic CAR-T cells from healthy persons have been tested in clinical trials (12–15). Universal CAR-T cells can be off-the-shelf and then infused into patients like usual medicines, without needing to wait for the isolation of autologous T cells from patients (12, 16); however, last year’s death case during the clinical trial of UCARTCS1A from Cellectis raised safety concerns about allogeneic CAR-T cells. The FDA also recently halted all clinical trials on universal CAR-T cells from Allogene due to safety concerns (17). Thus, we need new strategies to overcome the associated toxicity and simplify the manufacturing process of current CAR-T cell therapy. In-vivo CAR-T cells induced by nanocarriers loaded with CAR genes and gene-editing tools have shown promising effects for regressing leukemia (18–20). The in-situ programming of autologous CAR-T cells can enhance the targeted killing of tumor cells and reduce systemic toxicity such as CRS and neurotoxicity. Additionally, the nanocarriers can be easily manufactured in a standardized method (21) In-vivo induced CAR-T cells provide a potential solution to overcome the barriers of current CAR-T cell therapy. Thus, here, we review CAR structure design, gene-editing tools, and gene delivery systems and the future trend of immune cell therapy.
CAR Structure and Evolution
The structure of the chimeric antigen receptor (CAR) has a modular design consisting of an antigen-binding domain, a hinge, a transmembrane domain, and an intracellular signaling domain (Figure 1A). The antigen-binding domain is usually a single-chain variable fragment (scFv) molecule derived from a monoclonal antibody that can bind to antigens on the surface of malignant cancer cells (4, 22–24). The transmembrane domain is responsible for anchoring the CAR onto the T cell membrane. The intracellular signaling domain generally contains a T cell activation domain derived from the CD3ζ chain of the T cell receptor as well as co-stimulatory domains often comprised of an immunoreceptor tyrosine-based activation motif containing regions of CD28 or 4-1BB (also known as CD137 and TNFRSF9) (25–29). Variations in each component of the CAR structure enable fine-tuning of the functionality and antitumor activity of the resultant CAR-T cell product. Various CAR structures have been designed to improve the safety and efficacy of CAR-T cell therapy. Once the designed CAR genes are integrated into T cells, the scFv on the surface of T cells specifically recognizes tumor-associated antigens and binds CAR-T cells with tumor cells. After that, the intracellular signal domains of CAR-T cells are activated and cause CAR-T cells to proliferate and secrete cytokines that kill tumor cells (30–32).
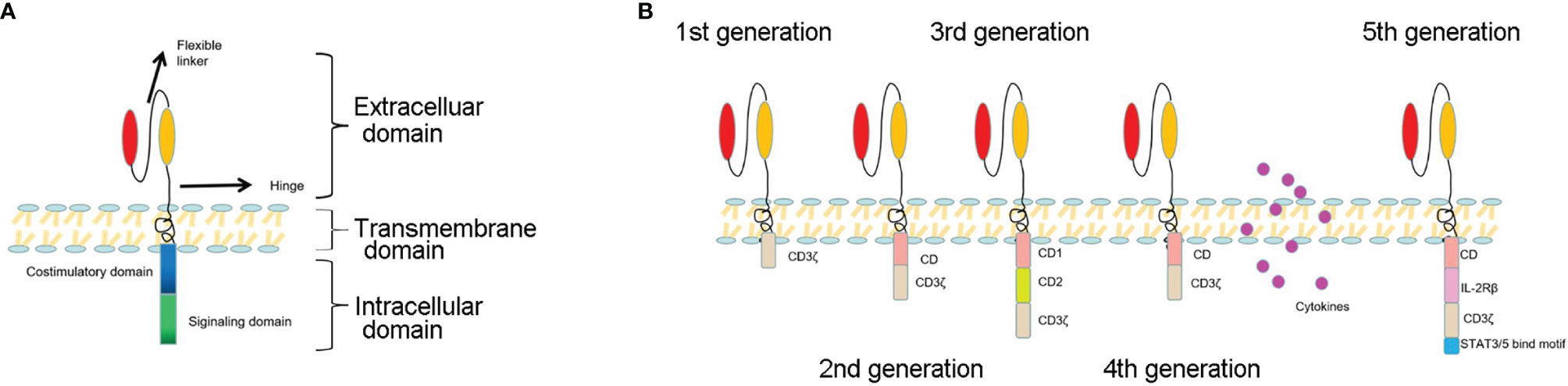
Figure 1 (A) The basic structure of a CAR: extracellular domain, transmembrane domain, and intracellular domain. (B) The development of the five generations of CARs. The first generation only contained the CD3ζ chain functional energy domain9; the second generation contained CD3ζ+ a costimulatory molecular domain (CD28, 4-1BB, etc.); the third generation contained CD3ζ and two costimulatory molecular domains; the fourth generation included suicide gene editing, immune factor modification, and other integrated and refined regulatory tools; the fifth generation included simultaneous activation of TCR, costimulatory domain, and cytokine triple signaling.
There have been five generations of CAR structures since the first clinical application of CAR-T cells by Carl June at the University of Pennsylvania and hematologist David Porter at the Children’s Hospital in Philadelphia in 2011 (33–35). The first-generation CAR contained an intracellular stimulation region and an extracellular scFv. This generation of CAR-T cells could not continuously proliferate due to the lack of costimulatory molecules (Figure 1B) (34). The second-generation CAR added a costimulatory molecule, such as CD28, or 4-1BB (CD137) to enhance the proliferation and reduce the toxicity of CAR-T cells (36). Yescarta™ (Tisagenlecleucel) and Kymriah™ (axicabtagene ciloleucel) are second-generation CAR-T cells that contain CD28 and 4-1BB, respectively (36). The third-generation CAR includes two costimulatory molecules, such as CD27, CD28, tumor necrosis factor superfamily 4 (OX40, also known as CD134), CD137 (4-1BB), or CD244 (37, 38). The fourth-generation CAR is called TRUCKs (T cells redirected for antigen-unrestricted cytokine-initiated killing), which combines the direct antitumor capacities of CAR-T cells with the immune modulating function of the delivered cytokine (34, 39). TRUCKs have entered early-phase clinical trials using a panel of cytokines, including IL-7, IL-12, IL-15, IL-18, IL-23, and their combinations. The fifth generation integrates an additional membrane receptor that controls the activation of CAR-T cells in an antigen-dependent manner (38, 40).
In addition to adding new functional molecules into the CAR structure, many studies have chosen alternative tumor-targeted sites for new CAR structures. CD30 shows very strong expression on malignant cells in Hodgkin’s lymphoma, rather than on healthy lymphocytes and hematopoietic stem/progenitor cells (HSPCs). CD30 CAR-T cell therapy has shown superior results in the treatment of CD30+ malignant tumors, while healthy activated lymphocytes and HSPC were unaffected (41). CD20 is a 33–37-kDa non-glycosylated transmembrane phosphoprotein that helps develop and differentiate B cells (42). CD20 is highly expressed in late pre-B cells and mature B cells, but it is not expressed on the surface of HSPCs (43). CD20 CAR T-cell therapy which has shown promise in the treatment of B-cell non-Hodgkin lymphoma is now being considered for patients with relapsed or refractory CD20-positive chronic lymphocytic leukemia. Lym-1 targets the conformational epitopes of human leukocyte antigen D-associated antigens (HLA-DRs) on the surface of human B-cell lymphoma. The binding affinity of Lym-1 with malignant B cells is higher than that of normal B cells (44). Lym-1 CAR-T cells have exhibited potent antitumor effects against B-cell lymphoma. Some alternative targeting sites combine with CD19 to form dual-target CAR T cells. For example, CD37 combined with CD19 was incorporated into one CAR to generate a dual-specific CAR T cell capable of recognizing CD19 and CD37 alone or together (45). CD79b is also a complementary targeting site for CD19. CD19 and CD79 dual-specific CAR-T cells prevented the escape of B-cell lymphoma from a single CD19 CAR-T cell (46, 47). Some alternative targeting sites have co-targeting functions that act on tumor cells and tumor microenvironments. For instance, CD123 was expressed in both Hodgkin lymphoma cells and tumor-associated macrophages so that anti-CD123 CAR-T cells could co-target these two kinds of cells and kill them simultaneously (48). The CAR structure is continually evolving to improve the efficacy of current CAR-T cell therapy (32, 49).
Barriers to Current CAR-T Cell Therapy
Five CAR-T cell products have been approved by the FDA from 2017 to 2021, as listed in Table 1. KYMRIAH™ (Tisagenlecleucel) is the first approved CAR-T cell therapy for adult patients with certain types of B-cell lymphoma (50). Three approved CAR-T cell products, YESCARTA™ (Axicabtagene ciloleucel), TECARTUS™ (brexucabtagene autoleucel), and BREYANZI® (lisocabtagene maraleucel), are also approved for the treatment of B cell lymphoma (51–53). The fifth CAR-T cell product, ABECMA® (idecabtagene vicleucel), is used for multiple myeloma therapy (54). Beyond the five approved CAR-T cell products, a large pipeline of CAR-T cells is being studied in clinical trials (55–57), but current CAR-T therapy has several barriers, such as associated toxicity, immunosuppressive tumor microenvironments, and complex manufacturing processes, which hamper the more widespread implementation of CAR-T therapy (58–60).
The major toxicities associated with current CAR-T therapy include cytokine release syndrome (CRS), immune effector cell-associated neurotoxicity syndrome (ICANS), and on-target/off-tumor toxicity (61–63). CRS is caused by the generation of massive inflammatory cytokines, such as IL-6, IL-10, IL-2, and TNFα, after CAR-T cell treatment. CRS often causes fever, hypotension, hypoxia, organ dysfunction, and even life-threatening adverse reactions (8, 64, 65). The occurrence of severe or life-threatening CRS can reach 25%. ICANS is another common toxicity associated with CAR-T cell therapy and is characterized by neurological abnormalities with aftereffects, usually within 1 week of CAT-cell treatment. The frequent adverse effects caused by ICANS include toxic encephalopathy with aphasia, confusion, and word-finding difficulty (66–68). On-target/off-tumor toxicity is due to the non-special expression of targeting proteins on both normal and malignant cells (69, 70). For instance, when administrating CD19 CAR-T cell in patients with malignant B cells, the on-target/off-tumor effect will lead to B cell aplasia and result in hypogammaglobulinemia due to the eradication of CD19+ B cell progenitors by CD19 CAR T cells (71, 72).
The immunosuppressive tumor microenvironment (MVT) inhibits the activation of CAR-T cells and accelerates the exhaustion of T cells (70, 73). Unfavorable factors in immunosuppressive MVT include hypoxia, various immunosuppressive cells, and the sustained expression of co-inhibitory receptors (74, 75). Hypoxia is defined as a shortage of oxygen in the tumor MVT. Immunosuppressive cells in the tumor MVT contain regulatory T cells (Tregs), tumor-associated macrophages (TAMs), and myeloid-derived suppressor cells (MDSCs) (74, 76).
The current manufacturing process of CAR-T cells is a highly complex endeavor, including T cell collection, genetic modification and expansion, and infusion back into patients (77, 78). These multistep technologies and logistics are rife with risks (10). Additionally, the long-term and individualized manufacturing processes pose great challenges for building up standard operating procedures (79). The costly and technology-intensive manufacturing processes of current CAR-T cells make them out of reach for many cancer patients in need of this novel therapy.
Gene-Editing Tools in CAR-T Cell Therapy
The gene-editing tools frequently applied to CAR-T cell therapy include zinc-finger nucleases (ZFN), transcription activator-like effector nucleases (TALEN), and clustered regularly interspaced short palindromic repeats-associated 9 (CRISPR-Cas9) technology (80–82). ZFN is the first broadly applied gene-editing tool that includes zinc fingers, a large multimeric protein, wherein each individual finger targets three to four base-pair sequences within genomic DNA (83, 84). Multimeric zinc finger proteins are able to link with the FokI endonuclease to create a ZFN that can cleave site-specific double-stranded DNA and lead to homologous recombination (HR) or non-homologous end-joining (NHEJ) (85). ZFN can achieve effective and specific gene-editing, but it is time-consuming to optimize the targeting protein molecules. TALEN are composed of several TAL units that can recognize base pairs of DNA and link to an endonuclease to generate the site-specific cleavage of DNAs (86, 87). TALEN are more economical than ZFN but still require a long time to optimize the system. CRISPR-Cas9 technology is the most popular gene-editing tool due to its simplicity and efficiency. The CRISPR-Cas9 complex was initially identified as an immune system for cleaving foreign viral DNA in Streptococcus pyogenes (88). These CRISPR complexes are first transcribed into RNAs (crRNAs), including bacterial CRISPR sequences, viral sequences (protospacers), and intervening sequences (PAMs) (89). These crRNAs are then complexed with the Cas endonuclease. Once the Cas–crRNA complex recognizes a homologous protospacer and PAM sequence, the Cas endonuclease cleaves the double-stranded DNA, followed by an automatic DNA repair process (88). A short-guide RNA (sgRNA) was introduced into the CRISPR-Cas9 system as the crRNA, making CRISPR-Cas9 an efficient, specific, and simple gene-editing tool (90). The development of gene-editing technology has allowed the precise surgical gene-editing of CAR-T cells to generate exhaustion-resistant T cells via removing the PD1 of T cells (91). CRISPR-Cas9 was also used to deplete endogenous antigens, such as CD33 and CD7, in normal cells to reduce the on-target off/tumor toxicity of redirected T cells (92, 93). The CRISPR-Cas9 system has been used in many CAR-T clinical trials involving more than twenty-one target antigens (Figure 2) (94–97). CD19 and BCMA account for nearly one-half of the CAR-T clinical trials on these target antigens. To use the CRISPR-Cas9 system more widely to edit CAR-T cells, efficient delivery methods must be developed.
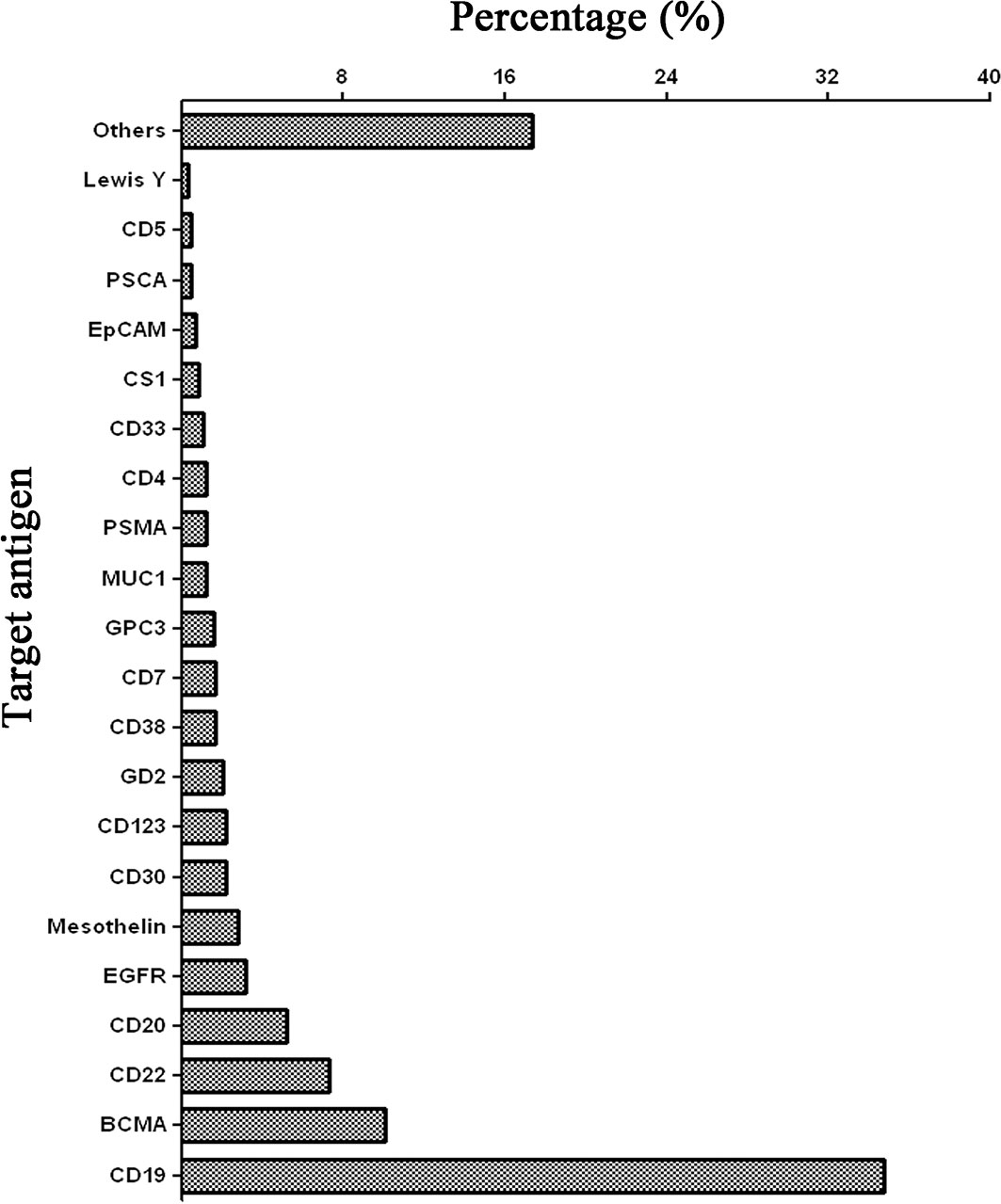
Figure 2 Target antigens of CAR-T cell therapy using CRISPR-Cas9 gene-editing technology registered in ClinicalTrials.gov until June 2021. The data only include clinical trials that are registered in USA.
Gene Delivery Systems
Plenty of delivery systems have been used to deliver gene therapy products including the gene-editing tools and CAR genes (Figure 3). Viral vectors have the highest transfection efficiency and have been widely used to deliver genes in various applications (96), but they suffer from the immunogenicity and cellular toxicity. Adenovirus-associated viruses (AVV) have a lower risk of toxicity than other viral vectors such as lentivirus, and adenovirus due to insertional mutagenesis (98). However, the AAV vector has a smaller packaging size (~5.0 kb) than other viral vectors (99). Non-viral delivery systems for gene delivery can be classified into either physical or chemical techniques. Physical techniques include electroporation, needle injection, laser irradiation, and gene guns. Electroporation is one of the most widespread application methods, which induces pore formation on cell membranes and the transient permeability of genes using electric pulses (100–102). Physical techniques have attractive effects on gene delivery due to their low immunogenicity, but they cannot target internal organs. Chemical techniques that mainly use nano-delivery systems include cationic lipids or polymer-based nanoparticles, golden nanoparticles, silica nanoparticles and quantum dots, carbon nanotubes, exosomes, ferritin, and cell membranes. Lipid-based nanoparticles are one of the most attractive non-viral vectors for gene delivery as several formulations of these carriers have been approved to use in the clinic (103–105). Especially, lipid-based nanoparticles have recently been successfully used to deliver SARS-CoV-2 mRNA vaccines (106). Lipid nanoparticles have also been used to deliver the CRISPR/Cas9 system to achieve in-vivo genome editing at clinically relevant levels (107, 108). Polymer-based nanoparticles are another system suitable for gene delivery applications. Positively charged polymers can form stable polyplexes with genes that disrupt cell membranes and enable endosomal escape (109, 110). The limitation of polymer-based nanoparticles is their toxicity and immunogenicity caused by the interaction of their positively charged surfaces with negatively charged cell membranes and proteins in blood circulation (111, 112). Exosomes are naturally secreted extracellular vesicles with nanometer sizes that are being extensively investigated as gene delivery vectors due to their natural biocompatibility and minimal immune clearance (113, 114); however, more efforts are required to overcome the difficulties in production, isolation, and purification (115). Cell membranes derived from platelets and red blood cells are biomimetic vectors used for gene delivery that have natural biocompatibility and targeting, but their transfection efficiencies need to be improved (116–118). Each of the other chemical nano-vectors has unique characteristics that determine their effects on gene delivery. Some have shown potential efficiency for the treatment of many diseases, but optimal delivery systems are still unrealized for clinical use.
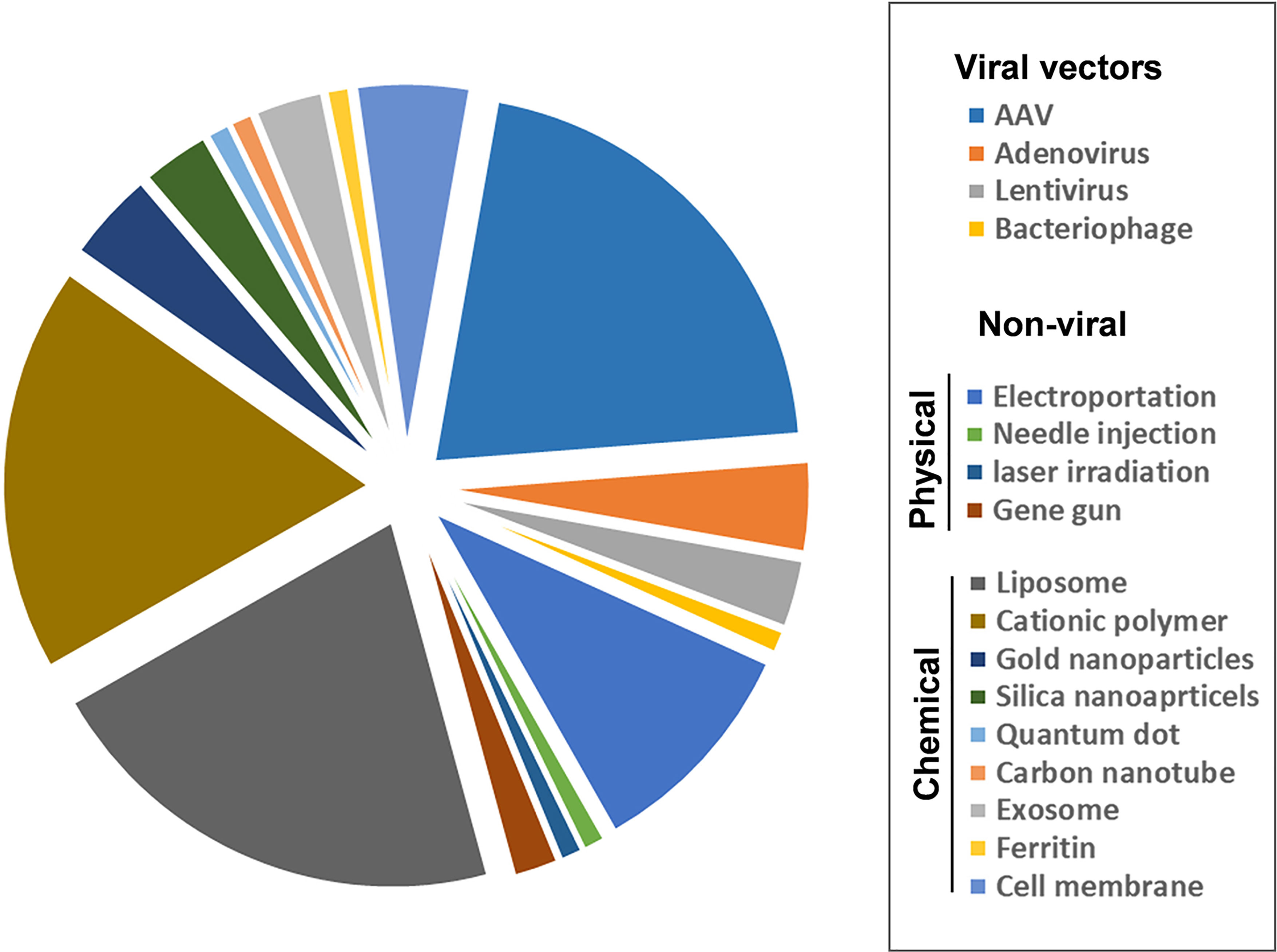
Figure 3 Representation of viral and non-viral nano-delivery systems classified as viral vectors, non-viral (physical and chemical). AAV, adeno-associated virus. The total number of papers is 18,968 obtained from PubMed, and Microsoft Excel was used to obtain the pie graph. The keywords are the name of vectors and gene delivery or CAR gene.
In-Vivo CAR-T Cell Induction
The current manufacturing process of CAR-T cells requires dedicated equipment and significant technical expertise and is also labor-intensive and time-consuming. (10, 119, 120). It limits the broader worldwide applications of this technology and drives up the price of CAR-therapy, making it out of reach of many patients (121). To simplify the production process, universal CAR-T cells from allogeneic healthy persons were tested in clinical trials, but, the FDA recently halted all clinical trials on the universal CAR-T cells from Allogene due to safety concerns of allogeneic CAR-T cells. There is an urgent need to develop a safe and simple production process for CAR-T cells. In-vivo programming of CAR-T cells by nanoparticles is an elegant and novel approach to simplify and standardize the complex manufacturing process of ex-vivo CAR-T cells (122). Additionally, the in-situ induction of CAR-T cells effectively reduces the systemic toxicity of CRS and ICANS. Recently, in-vivo induced CAR-T cells were accomplished through the nano-delivery of CAR structures or gene-editing tools by the team of Matthias Stephan from the Fred Hutchinson Cancer Research Center (Seattle, USA) (18, 20). They accomplished the stable and transient expression of targeting CAR protein in T cells via the infusion of nanoparticles loaded with CAR-DNA and CAR-mRNA, respectively. In these two works, the core of the nano-delivery systems was composed of a cationic polymer, poly(β-amino ester), assembled with a second-generation CAR structure targeted to CD19. The exterior of the nano-delivery system was composed of polyglutamic acid (PGA) conjugated with an anti-CD3 antibody. The polymer nanoparticles carrying CD19-specific CAR genes quickly and specifically edited T-cells in vivo and brought about comparable antitumor efficacies to conventional laboratory-manufactured CAR T-cells without inducing systemic toxicity. In addition to the polymer nanoparticles, viral vectors such as lentiviruses and AAV have also been tested for the in-vivo generation of CAR-T cells. Christian J. Buchholz and his colleagues first reported that lentiviruses encapsulated with a second-generation anti-CD19 CAR gene induced in-situ CAR T cells in immunodeficient NOD-scid-IL2Rcnull (NSG) mice and showed antitumor activity (123, 124). They also exhibited cytokine release syndrome that is notorious in clinical practice. In their study, CAR-positive NK and NKT cells were unexpectedly detected, which were likely caused by the non-specificity of the lentiviral vector. To overcome the non-specificity of the viral vector, Samuel K Lai et al. developed a bispecific binder to redirect the lentiviral vector to T cells for the in-vivo specific engineering of CAR-T cells (125). They observed the antitumor activity from the in-vivo CAR-T cells engineered by lentivirus, but a relatively low number of CAR-expressing T cells. They considered this to be proof of a valuable and unverified theory of the superior performance and self-renewal capacity of in-vivo CAR-T cells compared with that of ex-vivo CAR-T cells. However, the toxicity of the in-vivo CAR-T cells engineered by the bispecific binder-redirected lentivirus was not included in this work. Among the viral vectors, AVV has a lower risk of toxicity. Xilin Wu et al. recently reported that AAV encoding a third-generation CAR gene could sufficiently reprogram immune effector cells to generate in-vivo CAR T cells (126). In this work, they showed a strong proof of concept of AAV-induced in-vivo CAR-T cells, but the authors were concerned about the non-specificity of the AAV carrying the CAR gene. Except for the non-specificity of the viral vector, a universal safety concern of viral vectors is the random insertion of genes in the chromosol. Precise and rapid gene editor tools such as CRISPR have been widely used to generate ex-vivo CAR-T cells. There are many studies on in-vivo gene-editing using CRISPR, but there are still no reports on the application of CRISPR to generate in-vivo CAR-T cells. The future applications of combing gene-editing tools and CAR genes will accelerate the clinical adoption of in-vivo CAR-T cells.
The nano-delivery of designed CAR-structures and gene-editing tools can induce the in-vivo formation of CAR-T cells with multiple functions to overcome the barriers of current CAR-T cells, such as associated CRS and ICANS toxicities, immunosuppressive microenvironment, and complex manufacturing processes (Figure 4). Systemic toxicities can be reduced through tumor in-situ editing and the expansion of T cells (18, 62). The incorporation of special cytokine genes into a CAR structure enables CAR-T cells to secrete cytokines, flushing the immunosuppressive microenvironment and making it suitable for the survival and proliferation of T cells (127–129). Loading gene-editing tools with CAR structures into nanoparticles can knock out the genes of immune checkpoint blockades to reverse T-cell exhaustion (130–132). More importantly, this approach resolves the difficulty of process standardization and scale-up of the manufacture of ex-vivo CAR-T cells (133). The final gene-editor nanoparticles can be conveniently produced, stored, and delivered as usual medicines (Figure 5). These studies are just the beginning of the period of in-vivo induced CAR-T cells. Their clinical applications still require more efforts to monitor the in-vivo editing and expansion status of T-cells.
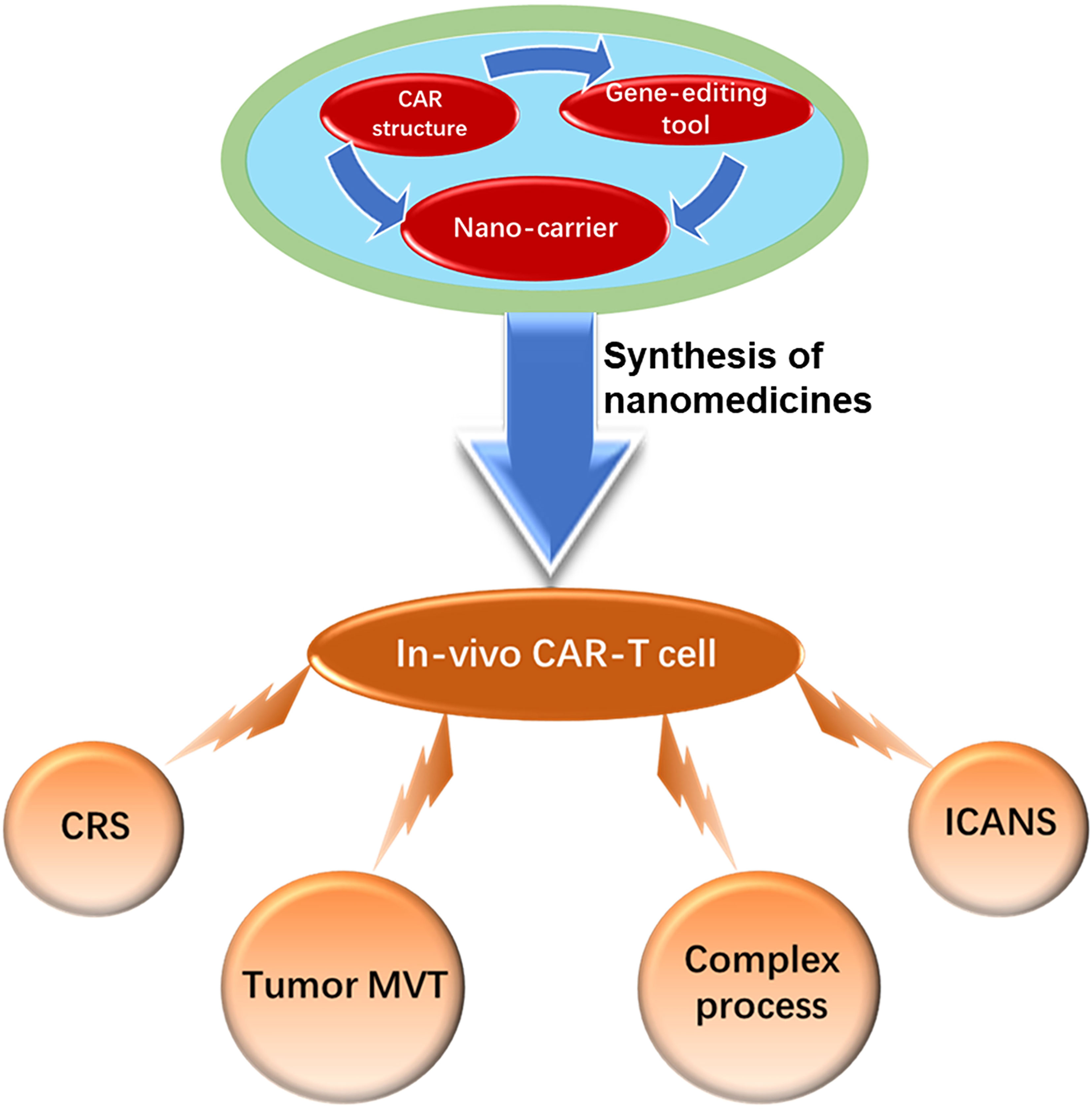
Figure 4 Overcoming the barriers of cytokine release syndrome (CRS), immune effector cell-associated neurotoxicity syndrome (ICANS), tumor microenvironment (MVT), and complex process through in-vivo CAR-T cell induced by nanomedicines composed of nano-carrier loaded with the CAR structure or a gene-editing tool.
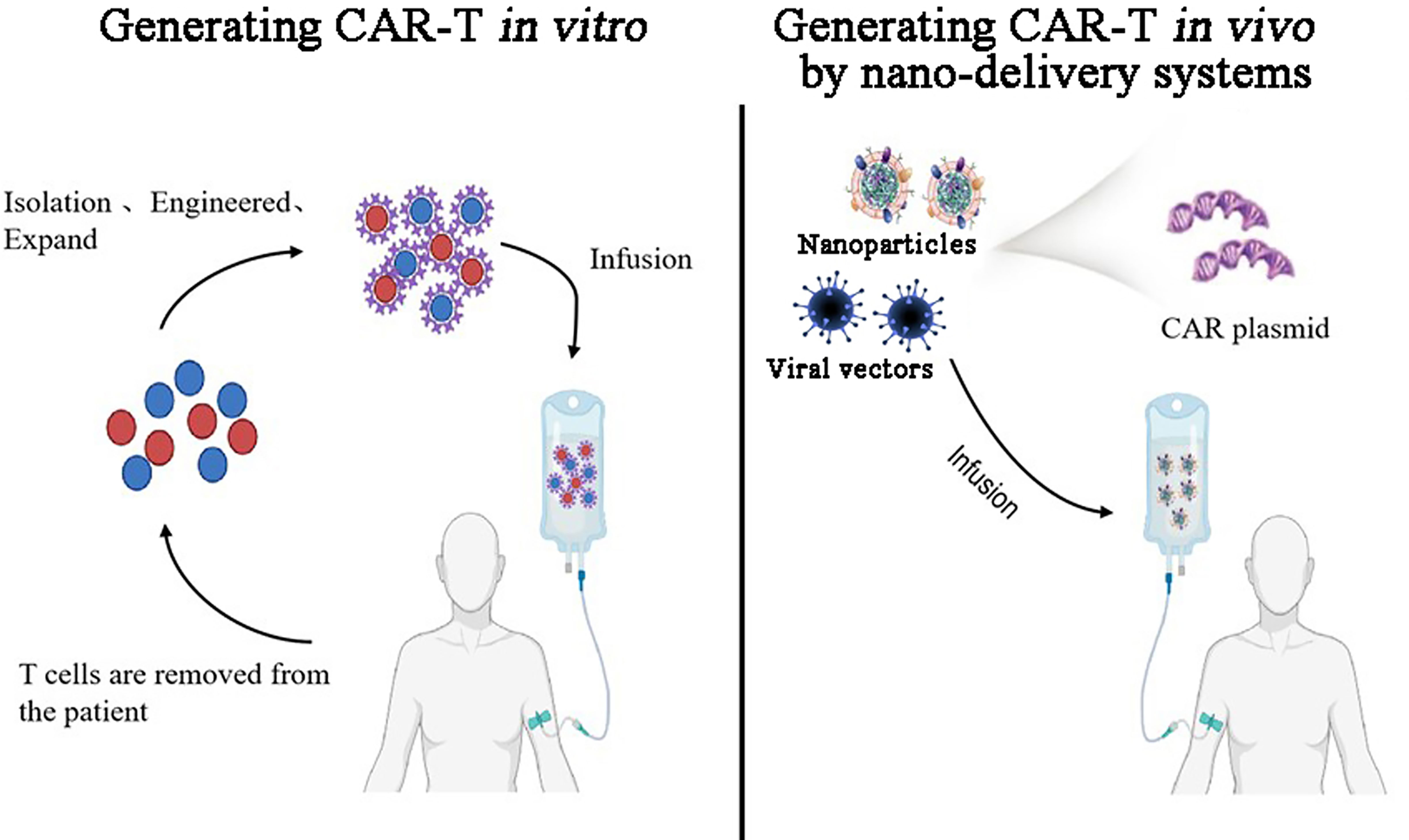
Figure 5 Comparison of generating CAR-T in-vitro and generating CAR-T-in-vivo by nano-delivery systems: In-vitro CAR T cells are first isolated from the patient, proliferated in-vitro, and then genetically engineered to screen the successfully edited CAR T cells, which are amplified to a certain number of infusions into the patient. In-vivo induced CAR T cells use nanotechnology to encapsulate CAR-expressing plasmids into nano-delivery systems including polymer nanoparticles and viral vectors such as lentivirus and AAV, which are then targeted to tumor regions in-vivo to edit T cells in-situ at tumor sites to kill tumors.
Conclusion and Future Prospects
Enormous achievements have been made in CAR-T cell therapy in the last decade, and five CAR-T cell products are available in the clinic. However, current CAR-T cell therapy also has some barriers that need to be overcome such as CRS and ICANS toxicity and expensive and complex manufacturing procedures. The in-vivo induced CAR-T cells by nanoparticles loaded with CAR genes and gene-editing tools have shown potential breakthroughs to overcome the abovementioned barriers of current CAR-T cell therapy. Although very few studies have reported nanoparticle-induced in-vivo CAR-T cells, robust preclinical data have predicted the future of cellular therapy through nano-delivery approaches. The field of in-vivo induced CAR-T cell therapy is still in its infancy with many challenges for the translation of this approach into clinical practice. A systematic summary of the nano-delivery systems for inducing in-vivo CAR-T cells can guide the design of the nanoparticles and their cargo to optimize their efficacy (134–136). In summary, in-vivo induced CAR-T cells are expected to replace current CAR-T cell therapy and become the standard immune-cell therapy for cancers.
Author Contributions
TX, LC, CZ, YZ, ZH, and XW performed the discussion. TX and ZH drew the figures. TX, ZH, and XW conceived and wrote the manuscript. All authors contributed to the article and approved the submitted version.
Funding
This work was supported by the foundation (2019020701011504) from Wuhan Municipal Science and Technology Bureau in China.
Conflict of Interest
The authors declare that the research was conducted in the absence of any commercial or financial relationships that could be construed as a potential conflict of interest.
Publisher’s Note
All claims expressed in this article are solely those of the authors and do not necessarily represent those of their affiliated organizations, or those of the publisher, the editors and the reviewers. Any product that may be evaluated in this article, or claim that may be made by its manufacturer, is not guaranteed or endorsed by the publisher.
References
1. Ramos CA, Heslop HE, Brenner MK. CAR-T Cell Therapy for Lymphoma. Annu Rev Med (2016) 67:165–83. doi: 10.1146/annurev-med-051914-021702
2. Sterner RC, Sterner RM. CAR-T Cell Therapy: Current Limitations and Potential Strategies. Blood Cancer J (2021) 11:69. doi: 10.1038/s41408-021-00459-7
3. June CH, O'connor RS, Kawalekar OU, Ghassemi S, Milone MC. CAR T Cell Immunotherapy for Human Cancer. Science (2018) 359:1361–5. doi: 10.1126/science.aar6711
4. Mohanty R, Chowdhury CR, Arega S, Sen P, Ganguly P, Ganguly N. CAR T Cell Therapy: A New Era for Cancer Treatment (Review). Oncol Rep (2019) 42:2183–95. doi: 10.3892/or.2019.7335
5. Fiorenza S, Turtle CJ. CAR-T Cell Therapy for Acute Myeloid Leukemia: Preclinical Rationale, Current Clinical Progress, and Barriers to Success. BioDrugs (2021) 35:281–302. doi: 10.1007/s40259-021-00477-8
6. Kersten MJ, Spanjaart AM, Thieblemont C. CD19-Directed CAR T-Cell Therapy in B-Cell NHL. Curr Opin Oncol (2020) 32:408–17. doi: 10.1097/CCO.0000000000000668
7. Qualls D, Salles G. Optimizing CAR T Cell Therapy in Lymphoma. Hematol Oncol (2021) 39(Suppl;1):104–12. doi: 10.1002/hon.2844
8. Sheth VS, Gauthier J. Taming the Beast: CRS and ICANS After CAR T-Cell Therapy for ALL. Bone Marrow Transplant (2021) 56:552–66. doi: 10.1038/s41409-020-01134-4
9. Xu J, Melenhorst JJ, Fraietta JA. Toward Precision Manufacturing of Immunogene T-Cell Therapies. Cytotherapy (2018) 20:623–38. doi: 10.1016/j.jcyt.2017.12.007
10. Nawaz W, Xu S, Li Y, Huang B, Wu X, Wu Z. Nanotechnology and Immunoengineering: How Nanotechnology can Boost CAR-T Therapy. Acta Biomater (2020) 109:21–36. doi: 10.1016/j.actbio.2020.04.015
11. Gong N, Sheppard NC, Billingsley MM, June CH, Mitchell MJ. Nanomaterials for T-Cell Cancer Immunotherapy. Nat Nanotechnol (2021) 16:25–36. doi: 10.1038/s41565-020-00822-y
12. Zhao J, Lin Q, Song Y, Liu D. Universal CARs, Universal T Cells, and Universal CAR T Cells. J Hematol Oncol (2018) 11:132. doi: 10.1186/s13045-018-0677-2
13. Perez C, Gruber I, Arber C. Off-the-Shelf Allogeneic T Cell Therapies for Cancer: Opportunities and Challenges Using Naturally Occurring "Universal" Donor T Cells. Front Immunol (2020) 11:583716. doi: 10.3389/fimmu.2020.583716
14. Townsend MH, Bennion K, Robison RA, O'neill KL. Paving the Way Towards Universal Treatment With Allogenic T Cells. Immunol Res (2020) 68:63–70. doi: 10.1007/s12026-020-09119-7
15. Wagner DL, Fritsche E, Pulsipher MA, Ahmed N, Hamieh M, Hegde M, et al. Immunogenicity of CAR T Cells in Cancer Therapy. Nat Rev Clin Oncol (2021) 18:379–93. doi: 10.1038/s41571-021-00476-2
16. Graham C, Jozwik A, Pepper A, Benjamin R. Allogeneic CAR-T Cells: More Than Ease of Access? Cells (2018) 7(10):155. doi: 10.3390/cells7100155
17. Locke FL, Malik S, Tees MT, Neelapu SS, Popplewell L, Abramson JS, et al. First-In-Human Data of ALLO-501A, an Allogeneic Chimeric Antigen Receptor (CAR) T-Cell Therapy and ALLO-647 in Relapsed/Refractory Large B-Cell Lymphoma (R/R LBCL): ALPHA2 Study. J Clin Oncol (2021) 39:2529–9. doi: 10.1200/JCO.2021.39.15_suppl.2529
18. Smith TT, Stephan SB, Moffett HF, Mcknight LE, Ji W, Reiman D, et al. In Situ Programming of Leukaemia-Specific T Cells Using Synthetic DNA Nanocarriers. Nat Nanotechnol (2017) 12:813–20. doi: 10.1038/nnano.2017.57
19. Agarwal S, Hanauer JDS, Frank AM, Riechert V, Thalheimer FB, Buchholz CJ. In Vivo Generation of CAR T Cells Selectively in Human CD4(+) Lymphocytes. Mol Ther (2020) 28:1783–94. doi: 10.1016/j.ymthe.2020.05.005
20. Parayath NN, Stephan SB, Koehne AL, Nelson PS, Stephan MT. In Vitro-Transcribed Antigen Receptor mRNA Nanocarriers for Transient Expression in Circulating T Cells In Vivo. Nat Commun (2020) 11:6080. doi: 10.1038/s41467-020-19486-2
21. Mhaidly R, Verhoeyen E. The Future: In Vivo CAR T Cell Gene Therapy. Mol Ther (2019) 27:707–9. doi: 10.1016/j.ymthe.2019.03.012
22. Zhang C, Liu J, Zhong JF, Zhang X. Engineering CAR-T Cells. Biomark Res (2017) 5:22. doi: 10.1186/s40364-017-0102-y
23. Chandran SS, Klebanoff CA. T Cell Receptor-Based Cancer Immunotherapy: Emerging Efficacy and Pathways of Resistance. Immunol Rev (2019) 290:127–47. doi: 10.1111/imr.12772
24. Huang R, Li X, He Y, Zhu W, Gao L, Liu Y, et al. Recent Advances in CAR-T Cell Engineering. J Hematol Oncol (2020) 13:86. doi: 10.1186/s13045-020-00910-5
25. Muhammad N, Mao Q, Xia H. CAR T-Cells for Cancer Therapy. Biotechnol Genet Eng Rev (2017) 33:190–226. doi: 10.1080/02648725.2018.1430465
26. Schwarzbich MA, Witzens-Harig M. Cellular Immunotherapy in B-Cell Malignancy. Oncol Res Treat (2017) 40:674–81. doi: 10.1159/000481946
27. Zenere G, Olwenyi OA, Byrareddy SN, Braun SE. Optimizing Intracellular Signaling Domains for CAR NK Cells in HIV Immunotherapy: A Comprehensive Review. Drug Discov Today (2019) 24:983–91. doi: 10.1016/j.drudis.2019.02.002
28. Jayaraman J, Mellody MP, Hou AJ, Desai RP, Fung AW, Pham AHT, et al. CAR-T Design: Elements and Their Synergistic Function. EBioMedicine (2020) 58:102931. doi: 10.1016/j.ebiom.2020.102931
29. Zhang Q, Ping J, Huang Z, Zhang X, Zhou J, Wang G, et al. CAR-T Cell Therapy in Cancer: Tribulations and Road Ahead. J Immunol Res (2020) 2020:1924379. doi: 10.1155/2020/1924379
30. Wang Z, Wu Z, Liu Y, Han W. New Development in CAR-T Cell Therapy. J Hematol Oncol (2017) 10:53. doi: 10.1186/s13045-017-0423-1
31. Brudno JN, Kochenderfer JN. Recent Advances in CAR T-Cell Toxicity: Mechanisms, Manifestations and Management. Blood Rev (2019) 34:45–55. doi: 10.1016/j.blre.2018.11.002
32. Larson RC, Maus MV. Recent Advances and Discoveries in the Mechanisms and Functions of CAR T Cells. Nat Rev Cancer (2021) 21:145–61. doi: 10.1038/s41568-020-00323-z
33. Porter DL, Levine BL, Kalos M, Bagg A, June CH. Chimeric Antigen Receptor-Modified T Cells in Chronic Lymphoid Leukemia. N Engl J Med (2011) 365:725–33. doi: 10.1056/NEJMoa1103849
34. Tokarew N, Ogonek J, Endres S, Von Bergwelt-Baildon M, Kobold S. Teaching an Old Dog New Tricks: Next-Generation CAR T Cells. Br J Cancer (2019) 120:26–37. doi: 10.1038/s41416-018-0325-1
35. Moreno-Cortes E, Forero-Forero JV, Lengerke-Diaz PA, Castro JE. Chimeric Antigen Receptor T Cell Therapy in Oncology - Pipeline at a Glance: Analysis of the ClinicalTrials.gov Database. Crit Rev Oncol Hematol (2021) 159:103239. doi: 10.1016/j.critrevonc.2021.103239
36. Kim DW, Cho JY. Recent Advances in Allogeneic CAR-T Cells. Biomolecules (2020) 10(2):263. doi: 10.3390/biom10020263
37. Weinkove R, George P, Dasyam N, Mclellan AD. Selecting Costimulatory Domains for Chimeric Antigen Receptors: Functional and Clinical Considerations. Clin Transl Immunol (2019) 8:e1049. doi: 10.1002/cti2.1049
38. Albinger N, Hartmann J, Ullrich E. Current Status and Perspective of CAR-T and CAR-NK Cell Therapy Trials in Germany. Gene Ther (2021) 28(9):513–27. doi: 10.1038/s41434-021-00246-w
39. Dummy. From CARs to TRUCKs and Beyond: Safely En Route to Adoptive T-Cell Therapy for Cancer. EBioMedicine (2016) 14:1–2. doi: 10.1016/j.ebiom.2016.11.037
40. Dragon AC, Zimmermann K, Nerreter T, Sandfort D, Lahrberg J, Klöß S, et al. CAR-T Cells and TRUCKs That Recognize an EBNA-3C-Derived Epitope Presented on HLA-B*35 Control Epstein-Barr Virus-Associated Lymphoproliferation. J Immunother Cancer (2020) 8(2):e000736. doi: 10.1136/jitc-2020-000736
41. Hombach AA, Görgens A, Chmielewski M, Murke F, Kimpel J, Giebel B, et al. Superior Therapeutic Index in Lymphoma Therapy: CD30(+) CD34(+) Hematopoietic Stem Cells Resist a Chimeric Antigen Receptor T-Cell Attack. Mol Ther (2016) 24:1423–34. doi: 10.1038/mt.2016.82
42. Sentman ML, Murad JM, Cook WJ, Wu MR, Reder J, Baumeister SH, et al. Mechanisms of Acute Toxicity in NKG2D Chimeric Antigen Receptor T Cell-Treated Mice. J Immunol (2016) 197:4674–85. doi: 10.4049/jimmunol.1600769
43. Teeling JL, Mackus WJ, Wiegman LJ, Van Den Brakel JH, Beers SA, French RR, et al. The Biological Activity of Human CD20 Monoclonal Antibodies Is Linked to Unique Epitopes on CD20. J Immunol (2006) 177:362–71. doi: 10.4049/jimmunol.177.1.362
44. Rose LM, Deng CT, Scott SL, Xiong CY, Lamborn KR, Gumerlock PH, et al. Critical Lym-1 Binding Residues on Polymorphic HLA-DR Molecules. Mol Immunol (1999) 36:789–97. doi: 10.1016/S0161-5890(99)00083-8
45. Scarfò I, Ormhøj M, Frigault MJ, Castano AP, Lorrey S, Bouffard AA, et al. Anti-CD37 Chimeric Antigen Receptor T Cells are Active Against B- and T-Cell Lymphomas. Blood (2018) 132:1495–506. doi: 10.1182/blood-2018-04-842708
46. Köksal H, Dillard P, Josefsson SE, Maggadottir SM, Pollmann S, Fåne A, et al. Preclinical Development of CD37CAR T-Cell Therapy for Treatment of B-Cell Lymphoma. Blood Adv (2019) 3:1230–43. doi: 10.1182/bloodadvances.2018029678
47. Ormhøj M, Scarfò I, Cabral ML, Bailey SR, Lorrey SJ, Bouffard AA, et al. Chimeric Antigen Receptor T Cells Targeting CD79b Show Efficacy in Lymphoma With or Without Cotargeting CD19. Clin Cancer Res (2019) 25:7046–57. doi: 10.1158/1078-0432.CCR-19-1337
48. Ruella M, Klichinsky M, Kenderian SS, Shestova O, Ziober A, Kraft DO, et al. Overcoming the Immunosuppressive Tumor Microenvironment of Hodgkin Lymphoma Using Chimeric Antigen Receptor T Cells. Cancer Discov (2017) 7:1154–67. doi: 10.1158/2159-8290.CD-16-0850
49. Roselli E, Faramand R, Davila ML. Insight Into Next-Generation CAR Therapeutics: Designing CAR T Cells to Improve Clinical Outcomes. J Clin Invest (2021) 131(2):e142030. doi: 10.1172/JCI142030
50. Prasad V. Immunotherapy: Tisagenlecleucel - the First Approved CAR-T-Cell Therapy: Implications for Payers and Policy Makers. Nat Rev Clin Oncol (2018) 15:11–2. doi: 10.1038/nrclinonc.2017.156
51. Roberts ZJ, Better M, Bot A, Roberts MR, Ribas A. Axicabtagene Ciloleucel, a First-in-Class CAR T Cell Therapy for Aggressive NHL. Leuk Lymphoma (2018) 59:1785–96. doi: 10.1080/10428194.2017.1387905
52. Abramson JS. Anti-CD19 CAR T-Cell Therapy for B-Cell Non-Hodgkin Lymphoma. Transfus Med Rev (2020) 34:29–33. doi: 10.1016/j.tmrv.2019.08.003
53. Anderson MK, Torosyan A, Halford Z. Brexucabtagene Autoleucel: A Novel Chimeric Antigen Receptor T-Cell Therapy for the Treatment of Mantle Cell Lymphoma. Ann Pharmacother (2021) 2:10600280211026338. doi: 10.1177/10600280211026338
54. Mikkilineni L, Kochenderfer JN. CAR T Cell Therapies for Patients With Multiple Myeloma. Nat Rev Clin Oncol (2021) 18:71–84. doi: 10.1038/s41571-020-0427-6
55. Pettitt D, Arshad Z, Smith J, Stanic T, Holländer G, Brindley D. CAR-T Cells: A Systematic Review and Mixed Methods Analysis of the Clinical Trial Landscape. Mol Ther (2018) 26:342–53. doi: 10.1016/j.ymthe.2017.10.019
56. Vitale C, Strati P. CAR T-Cell Therapy for B-Cell Non-Hodgkin Lymphoma and Chronic Lymphocytic Leukemia: Clinical Trials and Real-World Experiences. Front Oncol (2020) 10:849. doi: 10.3389/fonc.2020.00849
57. Wei J, Guo Y, Wang Y, Wu Z, Bo J, Zhang B, et al. Clinical Development of CAR T Cell Therapy in China: 2020 Update. Cell Mol Immunol (2021) 18:792–804. doi: 10.1038/s41423-020-00555-x
58. Marple AH, Bonifant CL, Shah NN. Improving CAR T-Cells: The Next Generation. Semin Hematol (2020) 57:115–21. doi: 10.1053/j.seminhematol.2020.07.002
59. Rafiq S, Hackett CS, Brentjens RJ. Engineering Strategies to Overcome the Current Roadblocks in CAR T Cell Therapy. Nat Rev Clin Oncol (2020) 17:147–67. doi: 10.1038/s41571-019-0297-y
60. Schubert ML, Schmitt M, Wang L, Ramos CA, Jordan K, Müller-Tidow C, et al. Side-Effect Management of Chimeric Antigen Receptor (CAR) T-Cell Therapy. Ann Oncol (2021) 32:34–48. doi: 10.1016/j.annonc.2020.10.478
61. Anderson JK, Mehta A. A Review of Chimeric Antigen Receptor T-Cells in Lymphoma. Expert Rev Hematol (2019) 12:551–61. doi: 10.1080/17474086.2019.1629901
62. Neelapu SS. Managing the Toxicities of CAR T-Cell Therapy. Hematol Oncol (2019) 37(Suppl 1):48–52. doi: 10.1002/hon.2595
63. Gust J, Ponce R, Liles WC, Garden GA, Turtle CJ. Cytokines in CAR T Cell-Associated Neurotoxicity. Front Immunol (2020) 11:577027. doi: 10.3389/fimmu.2020.577027
64. Norelli M, Camisa B, Barbiera G, Falcone L, Purevdorj A, Genua M, et al. Monocyte-Derived IL-1 and IL-6 Are Differentially Required for Cytokine-Release Syndrome and Neurotoxicity Due to CAR T Cells. Nat Med (2018) 24:739–48. doi: 10.1038/s41591-018-0036-4
65. Ying Z, Huang XF, Xiang X, Liu Y, Kang X, Song Y, et al. A Safe and Potent Anti-CD19 CAR T Cell Therapy. Nat Med (2019) 25:947–53. doi: 10.1038/s41591-019-0421-7
66. Freyer CW, Porter DL. Cytokine Release Syndrome and Neurotoxicity Following CAR T-Cell Therapy for Hematologic Malignancies. J Allergy Clin Immunol (2020) 146:940–8. doi: 10.1016/j.jaci.2020.07.025
67. Kennedy LB, Salama AKS. A Review of Cancer Immunotherapy Toxicity. CA Cancer J Clin (2020) 70:86–104. doi: 10.3322/caac.21596
68. Tallantyre EC, Evans NA, Parry-Jones J, Morgan MPG, Jones CH, Ingram W. Neurological Updates: Neurological Complications of CAR-T Therapy. J Neurol (2021) 268:1544–54. doi: 10.1007/s00415-020-10237-3
69. Yu S, Yi M, Qin S, Wu K. Next Generation Chimeric Antigen Receptor T Cells: Safety Strategies to Overcome Toxicity. Mol Cancer (2019) 18:125. doi: 10.1186/s12943-019-1057-4
70. Castellarin M, Sands C, Da T, Scholler J, Graham K, Buza E, et al. A Rational Mouse Model to Detect on-Target, Off-Tumor CAR T Cell Toxicity. JCI Insight (2020) 5(14):e136012. doi: 10.1172/jci.insight.136012
71. Parker KR, Migliorini D, Perkey E, Yost KE, Bhaduri A, Bagga P, et al. Single-Cell Analyses Identify Brain Mural Cells Expressing CD19 as Potential Off-Tumor Targets for CAR-T Immunotherapies. Cell (2020) 183:126–42.e117. doi: 10.1016/j.cell.2020.08.022
72. Derlin T, Schultze-Florey C, Werner RA, Möhn N, Skripuletz T, David S, et al. (18)F-FDG PET/CT of Off-Target Lymphoid Organs in CD19-Targeting Chimeric Antigen Receptor T-Cell Therapy for Relapsed or Refractory Diffuse Large B-Cell Lymphoma. Ann Nucl Med (2021) 35:132–8. doi: 10.1007/s12149-020-01544-w
73. Hou AJ, Chen LC, Chen YY. Navigating CAR-T Cells Through the Solid-Tumour Microenvironment. Nat Rev Drug Discov (2021) 20:531–50. doi: 10.1038/s41573-021-00189-2
74. Martinez M, Moon EK. CAR T Cells for Solid Tumors: New Strategies for Finding, Infiltrating, and Surviving in the Tumor Microenvironment. Front Immunol (2019) 10:128. doi: 10.3389/fimmu.2019.00128
75. Wagner J, Wickman E, Derenzo C, Gottschalk S. CAR T Cell Therapy for Solid Tumors: Bright Future or Dark Reality? Mol Ther (2020) 28:2320–39. doi: 10.1016/j.ymthe.2020.09.015
76. Newick K, O'brien S, Moon E, Albelda SM. CAR T Cell Therapy for Solid Tumors. Annu Rev Med (2017) 68:139–52. doi: 10.1146/annurev-med-062315-120245
77. Vormittag P, Gunn R, Ghorashian S, Veraitch FS. A Guide to Manufacturing CAR T Cell Therapies. Curr Opin Biotechnol (2018) 53:164–81. doi: 10.1016/j.copbio.2018.01.025
78. Stock S, Schmitt M, Sellner L. Optimizing Manufacturing Protocols of Chimeric Antigen Receptor T Cells for Improved Anticancer Immunotherapy. Int J Mol Sci (2019) 20(24):6223. doi: 10.3390/ijms20246223
79. Roddie C, O'reilly M, Dias Alves Pinto J, Vispute K, Lowdell M. Manufacturing Chimeric Antigen Receptor T Cells: Issues and Challenges. Cytotherapy (2019) 21:327–40. doi: 10.1016/j.jcyt.2018.11.009
80. Jeon S, Lim JM, Lee HG, Shin SE, Kang NK, Park YI, et al. Current Status and Perspectives of Genome Editing Technology for Microalgae. Biotechnol Biofuels (2017) 10:267. doi: 10.1186/s13068-017-0957-z
81. Bak RO, Gomez-Ospina N, Porteus MH. Gene Editing on Center Stage. Trends Genet (2018) 34:600–11. doi: 10.1016/j.tig.2018.05.004
82. Li H, Yang Y, Hong W, Huang M, Wu M, Zhao X. Applications of Genome Editing Technology in the Targeted Therapy of Human Diseases: Mechanisms, Advances and Prospects. Signal Transduct Target Ther (2020) 5:1. doi: 10.1038/s41392-019-0089-y
83. Urnov FD, Miller JC, Lee YL, Beausejour CM, Rock JM, Augustus S, et al. Highly Efficient Endogenous Human Gene Correction Using Designed Zinc-Finger Nucleases. Nature (2005) 435:646–51. doi: 10.1038/nature03556
84. Chandrasegaran S. Recent Advances in the Use of ZFN-Mediated Gene Editing for Human Gene Therapy. Cell Gene Ther Insights (2017) 3:33–41. doi: 10.18609/cgti.2017.005
85. Porteus MH, Baltimore D. Chimeric Nucleases Stimulate Gene Targeting in Human Cells. Science (2003) 300:763. doi: 10.1126/science.1078395
86. Boch J, Scholze H, Schornack S, Landgraf A, Hahn S, Kay S, et al. Breaking the Code of DNA Binding Specificity of TAL-Type III Effectors. Science (2009) 326:1509–12. doi: 10.1126/science.1178811
87. Miller JC, Tan S, Qiao G, Barlow KA, Wang J, Xia DF, et al. A TALE Nuclease Architecture for Efficient Genome Editing. Nat Biotechnol (2011) 29:143–8. doi: 10.1038/nbt.1755
88. Wiedenheft B, Sternberg SH, Doudna JA. RNA-Guided Genetic Silencing Systems in Bacteria and Archaea. Nature (2012) 482:331–8. doi: 10.1038/nature10886
89. Barrangou R, Horvath P. A Decade of Discovery: CRISPR Functions and Applications. Nat Microbiol (2017) 2:17092. doi: 10.1038/nmicrobiol.2017.92
90. Mali P, Yang L, Esvelt KM, Aach J, Guell M, Dicarlo JE, et al. RNA-Guided Human Genome Engineering via Cas9. Science (2013) 339:823–6. doi: 10.1126/science.1232033
91. Ren J, Zhang X, Liu X, Fang C, Jiang S, June CH, et al. A Versatile System for Rapid Multiplex Genome-Edited CAR T Cell Generation. Oncotarget (2017) 8:17002–11. doi: 10.18632/oncotarget.15218
92. Gomes-Silva D, Srinivasan M, Sharma S, Lee CM, Wagner DL, Davis TH, et al. CD7-Edited T Cells Expressing a CD7-Specific CAR for the Therapy of T-Cell Malignancies. Blood (2017) 130:285–96. doi: 10.1182/blood-2017-01-761320
93. Borot F, Wang H, Ma Y, Jafarov T, Raza A, Ali AM, et al. Gene-Edited Stem Cells Enable CD33-Directed Immune Therapy for Myeloid Malignancies. Proc Natl Acad Sci USA (2019) 116:11978–87. doi: 10.1073/pnas.1819992116
94. Liu X, Zhang Y, Cheng C, Cheng AW, Zhang X, Li N, et al. CRISPR-Cas9-Mediated Multiplex Gene Editing in CAR-T Cells. Cell Res (2017) 27:154–7. doi: 10.1038/cr.2016.142
95. Li C, Mei H, Hu Y. Applications and Explorations of CRISPR/Cas9 in CAR T-Cell Therapy. Brief Funct Genomics (2020) 19:175–82. doi: 10.1093/bfgp/elz042
96. Hu KJ, Yin ETS, Hu YX, Huang H. Combination of CRISPR/Cas9 System and CAR-T Cell Therapy: A New Era for Refractory and Relapsed Hematological Malignancies. Curr Med Sci (2021) 41:420–30. doi: 10.1007/s11596-021-2391-5
97. Razeghian E, Nasution MKM, Rahman HS, Gardanova ZR, Abdelbasset WK, Aravindhan S, et al. A Deep Insight Into CRISPR/Cas9 Application in CAR-T Cell-Based Tumor Immunotherapies. Stem Cell Res Ther (2021) 12:428. doi: 10.1186/s13287-021-02510-7
98. David RM, Doherty AT. Viral Vectors: The Road to Reducing Genotoxicity. Toxicol Sci (2017) 155:315–25. doi: 10.1093/toxsci/kfw220
99. Wang D, Tai PWL, Gao G. Adeno-Associated Virus Vector as a Platform for Gene Therapy Delivery. Nat Rev Drug Discov (2019) 18:358–78. doi: 10.1038/s41573-019-0012-9
100. Monjezi R, Miskey C, Gogishvili T, Schleef M, Schmeer M, Einsele H, et al. Enhanced CAR T-Cell Engineering Using non-Viral Sleeping Beauty Transposition From Minicircle Vectors. Leukemia (2017) 31:186–94. doi: 10.1038/leu.2016.180
101. Shi J, Ma Y, Zhu J, Chen Y, Sun Y, Yao Y, et al. A Review on Electroporation-Based Intracellular Delivery. Molecules (2018) 23(11):3044. doi: 10.3390/molecules23113044
102. Zhang Z, Qiu S, Zhang X, Chen W. Optimized DNA Electroporation for Primary Human T Cell Engineering. BMC Biotechnol (2018) 18:4. doi: 10.1186/s12896-018-0419-0
103. Chen S, Wang Y, Nie T, Bao C, Wang C, Xu T, et al. An Artificial Molecular Shuttle Operates in Lipid Bilayers for Ion Transport. J Am Chem Soc (2018) 140:17992–8. doi: 10.1021/jacs.8b09580
104. Rajpoot K. Solid Lipid Nanoparticles: A Promising Nanomaterial in Drug Delivery. Curr Pharm Des (2019) 25:3943–59. doi: 10.2174/1381612825666190903155321
105. Eygeris Y, Patel S, Jozic A, Sahay G. Deconvoluting Lipid Nanoparticle Structure for Messenger RNA Delivery. Nano Lett (2020) 20:4543–9. doi: 10.1021/acs.nanolett.0c01386
106. Polack FP, Thomas SJ, Kitchin N, Absalon J, Gurtman A, Lockhart S, et al. Safety and Efficacy of the BNT162b2 mRNA Covid-19 Vaccine. N Engl J Med (2020) 383:2603–15. doi: 10.1056/NEJMoa2034577
107. Finn JD, Smith AR, Patel MC, Shaw L, Youniss MR, Van Heteren J, et al. A Single Administration of CRISPR/Cas9 Lipid Nanoparticles Achieves Robust and Persistent In Vivo Genome Editing. Cell Rep (2018) 22:2227–35. doi: 10.1016/j.celrep.2018.02.014
108. Wei T, Cheng Q, Min YL, Olson EN, Siegwart DJ. Systemic Nanoparticle Delivery of CRISPR-Cas9 Ribonucleoproteins for Effective Tissue Specific Genome Editing. Nat Commun (2020) 11:3232. doi: 10.1038/s41467-020-17029-3
109. Meneksedag-Erol D, Tang T, Uludağ H. Probing the Effect of miRNA on siRNA-PEI Polyplexes. J Phys Chem B (2015) 119:5475–86. doi: 10.1021/acs.jpcb.5b00415
110. Ita K. Polyplexes for Gene and Nucleic Acid Delivery: Progress and Bottlenecks. Eur J Pharm Sci (2020) 150:105358. doi: 10.1016/j.ejps.2020.105358
111. Kazemi Oskuee R, Dabbaghi M, Gholami L, Taheri-Bojd S, Balali-Mood M, Mousavi SH, et al. Investigating the Influence of Polyplex Size on Toxicity Properties of Polyethylenimine Mediated Gene Delivery. Life Sci (2018) 197:101–8. doi: 10.1016/j.lfs.2018.02.008
112. Hayat SMG, Farahani N, Safdarian E, Roointan A, Sahebkar A. Gene Delivery Using Lipoplexes and Polyplexes: Principles, Limitations and Solutions. Crit Rev Eukaryot Gene Expr (2019) 29:29–36. doi: 10.1615/CritRevEukaryotGeneExpr.2018025132
113. Gao D, Jiang L. Exosomes in Cancer Therapy: A Novel Experimental Strategy. Am J Cancer Res (2018) 8:2165–75.
114. Dutta A. Exosomes-Based Cell-Free Cancer Therapy: A Novel Strategy for Targeted Therapy. Immunol Med (2021) 44:116–23. doi: 10.1080/25785826.2020.1818482
115. Koritzinsky EH, Street JM, Star RA, Yuen PS. Quantification of Exosomes. J Cell Physiol (2017) 232:1587–90. doi: 10.1002/jcp.25387
116. Wu M, Le W, Mei T, Wang Y, Chen B, Liu Z, et al. Cell Membrane Camouflaged Nanoparticles: A New Biomimetic Platform for Cancer Photothermal Therapy. Int J Nanomed (2019) 14:4431–48. doi: 10.2147/IJN.S200284
117. Spanjers JM, Städler B. Cell Membrane Coated Particles. Adv Biosyst (2020) 4:e2000174. doi: 10.1002/adbi.202000174
118. Wang H, Liu Y, He R, Xu D, Zang J, Weeranoppanant N, et al. Cell Membrane Biomimetic Nanoparticles for Inflammation and Cancer Targeting in Drug Delivery. Biomater Sci (2020) 8:552–68. doi: 10.1039/C9BM01392J
119. Smith TA. CAR-T Cell Expansion in a Xuri Cell Expansion System W25. Methods Mol Biol (2020) 2086:151–63. doi: 10.1007/978-1-0716-0146-4_11
120. Geethakumari PR, Ramasamy DP, Dholaria B, Berdeja J, Kansagra A. Balancing Quality, Cost, and Access During Delivery of Newer Cellular and Immunotherapy Treatments. Curr Hematol Malig Rep (2021) 16(4):345–56. doi: 10.1007/s11899-021-00635-3
121. Jayaraman K. Cut-Price CAR-T Cell Therapies Top India's Biotech Agenda. Nat Biotechnol (2019) 37:1388–9. doi: 10.1038/s41587-019-0346-1
122. Lin JK, Muffly LS, Spinner MA, Barnes JI, Owens DK, Goldhaber-Fiebert JD. Cost Effectiveness of Chimeric Antigen Receptor T-Cell Therapy in Multiply Relapsed or Refractory Adult Large B-Cell Lymphoma. J Clin Oncol (2019) 37:2105–19. doi: 10.1200/JCO.18.02079
123. Pfeiffer A, Thalheimer FB, Hartmann S, Frank AM, Bender RR, Danisch S, et al. In Vivo Generation of Human CD19-CAR T Cells Results in B-Cell Depletion and Signs of Cytokine Release Syndrome. EMBO Mol Med (2018) 10(11):e9158. doi: 10.15252/emmm.201809158
124. Agarwal S, Weidner T, Thalheimer FB, Buchholz CJ. In Vivo Generated Human CAR T Cells Eradicate Tumor Cells. Oncoimmunology (2019) 8:e1671761. doi: 10.1080/2162402X.2019.1671761
125. Huckaby JT, Landoni E, Jacobs TM, Savoldo B, Dotti G, Lai SK. Bispecific Binder Redirected Lentiviral Vector Enables In Vivo Engineering of CAR-T Cells. J Immunother Cancer (2021) 9(9):e002737. doi: 10.1136/jitc-2021-002737
126. Nawaz W, Huang B, Xu S, Li Y, Zhu L, Yiqiao H, et al. AAV-Mediated In Vivo CAR Gene Therapy for Targeting Human T-Cell Leukemia. Blood Cancer J (2021) 11:119. doi: 10.1038/s41408-021-00508-1
127. Santoiemma PP, Powell DJ Jr. Tumor Infiltrating Lymphocytes in Ovarian Cancer. Cancer Biol Ther (2015) 16:807–20. doi: 10.1080/15384047.2015.1040960
128. Siegler EL, Wang P. Preclinical Models in Chimeric Antigen Receptor-Engineered T-Cell Therapy. Hum Gene Ther (2018) 29:534–46. doi: 10.1089/hum.2017.243
129. Agliardi G, Liuzzi AR, Hotblack A, De Feo D, Núñez N, Stowe CL, et al. Intratumoral IL-12 Delivery Empowers CAR-T Cell Immunotherapy in a Pre-Clinical Model of Glioblastoma. Nat Commun (2021) 12:444. doi: 10.1038/s41467-020-20599-x
130. Eyquem J, Mansilla-Soto J, Giavridis T, van der Stegen SJ, Hamieh M, Cunanan KM, et al. Targeting a CAR to the TRAC Locus With CRISPR/Cas9 Enhances Tumour Rejection. Nature (2017) 543:113–7. doi: 10.1038/nature21405
131. Mollanoori H, Shahraki H, Rahmati Y, Teimourian S. CRISPR/Cas9 and CAR-T Cell, Collaboration of Two Revolutionary Technologies in Cancer Immunotherapy, an Instruction for Successful Cancer Treatment. Hum Immunol (2018) 79:876–82. doi: 10.1016/j.humimm.2018.09.007
132. Salas-Mckee J, Kong W, Gladney WL, Jadlowsky JK, Plesa G, Davis MM, et al. CRISPR/Cas9-Based Genome Editing in the Era of CAR T Cell Immunotherapy. Hum Vaccin Immunother (2019) 15:1126–32. doi: 10.1080/21645515.2019.1571893
133. Dai X, Mei Y, Cai D, Han W. Standardizing CAR-T Therapy: Getting it Scaled Up. Biotechnol Adv (2019) 37:239–45. doi: 10.1016/j.biotechadv.2018.12.002
134. Ye B, Stary CM, Li X, Gao Q, Kang C, Xiong X. Engineering Chimeric Antigen Receptor-T Cells for Cancer Treatment. Mol Cancer (2018) 17:32. doi: 10.1186/s12943-018-0814-0
135. Ho SWT, Tan P. Dissection of Gastric Cancer Heterogeneity for Precision Oncology. Cancer Sci (2019) 110:3405–14. doi: 10.1111/cas.14191
Keywords: CAR-T cells, barriers, in-situ editing, gene-editing tool, nano-delivery
Citation: Xin T, Cheng L, Zhou C, Zhao Y, Hu Z and Wu X (2022) In-Vivo Induced CAR-T Cell for the Potential Breakthrough to Overcome the Barriers of Current CAR-T Cell Therapy. Front. Oncol. 12:809754. doi: 10.3389/fonc.2022.809754
Received: 05 November 2021; Accepted: 03 January 2022;
Published: 10 February 2022.
Edited by:
Yuanzeng Min, University of Science and Technology of China, ChinaReviewed by:
Yozo Nakazawa, Shinshu University School of Medicine, JapanYiran Zheng, Soochow University, China
Copyright © 2022 Xin, Cheng, Zhou, Zhao, Hu and Wu. This is an open-access article distributed under the terms of the Creative Commons Attribution License (CC BY). The use, distribution or reproduction in other forums is permitted, provided the original author(s) and the copyright owner(s) are credited and that the original publication in this journal is cited, in accordance with accepted academic practice. No use, distribution or reproduction is permitted which does not comply with these terms.
*Correspondence: Xiaoyan Wu, eHd1QGh1c3QuZWR1LmNu; Zhenhua Hu, aHV6aGhAbmZ1LmVkdS5jbg==