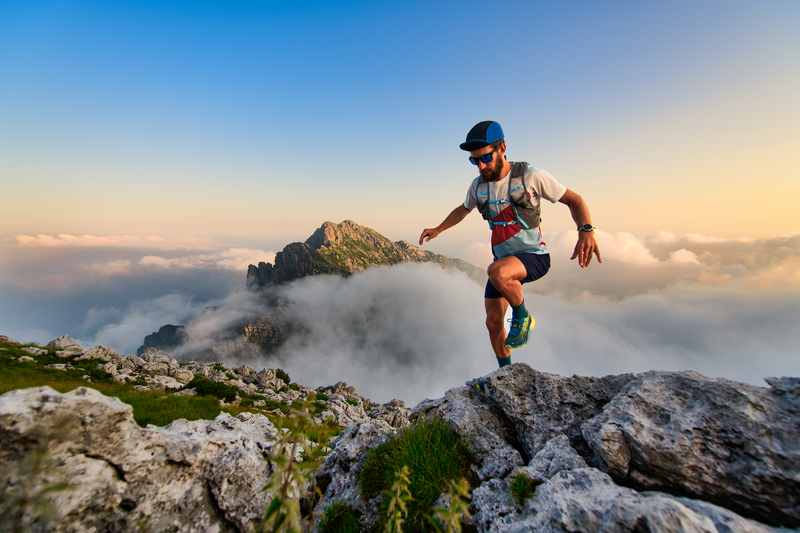
95% of researchers rate our articles as excellent or good
Learn more about the work of our research integrity team to safeguard the quality of each article we publish.
Find out more
REVIEW article
Front. Oncol. , 08 February 2022
Sec. Hematologic Malignancies
Volume 12 - 2022 | https://doi.org/10.3389/fonc.2022.794021
This article is part of the Research Topic Advancing Science for Clinical Care in MDS View all 6 articles
Clonal hematopoiesis (CH), defined as the clonal expansion of mutated hematopoietic stem and progenitor cells (HSPCs), is a common aging process. CH is a risk factor for the development of hematologic malignancies, most commonly myeloid neoplasms (MNs) including acute myeloid leukemia (AML), myelodysplastic syndrome (MDS), and myeloproliferative neoplasm (MPN). Recent work has elucidated how the development and cellular fitness of CH is shaped by aging, environmental exposures, and the germline (inherited) genetic background of an individual. This in turn has provided valuable insights into the pathogenesis of MNs including MDS. Here, in this review, we discuss the genetic origins of CH, the environmental stressors that influence CH, and the implications of CH on health outcomes including MDS. Since MNs have shared risk factors and underlying biology, most of our discussion regarding the implications of CH surrounds MN in general rather than focusing specifically on MDS. We conclude with future directions and areas of investigation including how intervention studies of CH might inform future therapeutic approaches to MN including MDS.
With every round of mitotic division, DNA damage and inefficient repair occur, resulting in the accumulation of somatic mutations in aging hematopoietic stem and progenitor cells (HSPCs) over time. The process of acquired mutations leading to clonal expansion of HSPCs is referred to as CH. The most common biochemical mutational event giving rise to CH is spontaneous deamination of methylated cytosine at CpG dinucleotides resulting in generation of thymine (1). CH is a normal process of aging (2–7). Indeed, the acquisition of aging-related mutations is not limited to stem cells of the hematopoietic system. Age-related somatic mutations are in fact pervasive across human tissues (8–12). Differences in the rate of somatic mutational acquisition across human tissues correlates with the rate of stem cell division and may in part explain variation in cancer risk among human tissues (10, 13). The patterns of aging-related somatic mutagenesis are conserved even across mammalian species with the mutation rate inversely correlated with species lifespan. This suggests that beyond risk of cancer, somatic mutation rate may be a determinant of lifespan (14). Mathematical reconstruction of HSPC lineage histories suggests that CH mutations may arise as early as childhood (even in utero) with some mutations undergoing a gradual expansion throughout life (11, 15).
Our ability to detect CH mutations and the observed prevalence of CH is dependent on the sensitivity of sequencing as determined by sequencing depth. For example, analysis of whole exome sequencing data sequenced at a depth of under 100-fold coverage generally reports age‐dependent single nucleotide variants (SNVs) or small insertions/deletions (indels) in approximately 6% of subjects over 65 years of age (2, 3, 14). In contrast, next-generation sequencing (NGS) technologies with advanced error-correction methods such as unique molecular indices, can accurately detect mutations below 0.5% variant allele fraction (VAF) when performed at a high depth (16). Using approaches such as these, the prevalence of CH mutations in the blood of adults over 50 appears closer to 100% (17). Sequencing technologies such as duplex consensus sequencing (9) and bottleneck sequencing (15) may enable the detection of individually mutated stem cells that have not undergone clonal expansion. The VAF used to define a clonally expanded stem cell is not well-defined and depends on assumptions regarding the number of stem cells actively contributing to white blood cell production. Studies tracking the clonal contributions of cells labelled directly in vivo have suggested that long-term homeostatic hematopoiesis is driven by many thousands of cells (18–20). However, recent work using single-cell-derived HSPCs suggests that the number of hematopoietic stem cells that are actively making white blood cells at any one time to be in the range of 50,000–200,000 (21).
The mutations driving CH confer a survival advantage over wild-type cells that results in the clonal expansion of the mutated cell. Thus, there is overlap in the genetic mutations driving CH and early drivers of hematologic cancer. CH driven by SNVs or indels are most commonly drivers of MNs. This includes epigenetic modifiers (DNMT3A, TET2, ASXL1, IDH1, IDH2), splicing factors (SF3B1, SRSF2, U2AF1), genes involved in the DNA damage response (TP53, PPM1D, CHEK2), and JAK2. Murine models have been useful in understanding the role that mutations in these genes play in conferring a fitness advantage. Mutations in the epigenetic regulators such as DNMT3A and TET2 have been shown to confer an advantage by enhancing self-renewal of stem and progenitor cells and inhibiting their differentiation (22, 23). Mutations in other genes involved in the DNA damage response may enhance cell survival under specific cellular stressors (5, 24, 25).
Copy number events (amplifications, deletions, or copy neutral loss of heterozygosity) are also known to drive CH. Analysis of genome-wide single nucleotide polymorphism (SNP) array data initially suggested that large >2 Mb copy number events in autosomal chromosomes were observed rarely, in roughly 2% of individuals aged 70–75 (2). Computational tools that incorporate long-range phase information have since improved the sensitivity to detect copy number-driven CH almost 10-fold (26). Somatic loss of chromosome Y (LOY) in males is the most common copy number event driving CH, occurring in over 25% men over the age of 60 (6). Interestingly, many of the large copy number events driving CH overlap with those driving lymphoid malignancies (e.g., trisomy 12, deletion 13q, deletion 11q, among others). This likely reflects the importance of copy number events as early drivers in lymphoid malignancies (27). Other classes of mutational events such as medium-sized (>50 bp) copy number events, structural events and noncoding mutations have relevance in hematologic cancer and may also drive CH, yet are not well characterized. With the decreasing cost of high-depth whole genome sequencing and other technologies better positioned to detect these classes of events, the mutational spectrum of the events driving CH will likely become better defined.
Germline (inherited) genetic factors appear important in determining both mutational acquisition and expansion of CH. Both common and rare germline CH susceptibility loci have been identified (19–21). Some genetic loci appear to be associated with CH driven by multiple driver genes (e.g., variants within the TERT and CHEK2 loci are associated with CH driven by alterations in DNMTA, JAK2, and copy number events including LOY). Others, however, show heterogeneity in the strength of the association across driver genes. For example, germline TCL1A variants are associated with DNMT3A-mutant CH but not JAK2-mutant CH (28). While diverse, many loci converge upon key pathways regulating the cell cycle, DNA damage sensing, and the apoptotic process. Another theme is the high overlap between CH susceptibility genes and known cancer susceptibility genes (29). Other loci appear to be the objects of positive or negative clonal selection, i.e., CH events occurring in cis achieve a strong selective advantage by duplicating or removing inherited alleles. This was observed within the setting of healthy individuals in the UK Biobank where rare inherited variants were associated strongly with the acquisition of deletions or loss of heterozygosity in cis (26). Somatic reversion or compensation for pathogenic germline alleles through CH has also been observed in the setting of inherited bone marrow failure syndromes (30, 31).
Studies of genetic susceptibility to LOY have shed light into the mechanism driving the long-established association between LOY and increased cancer susceptibility (32, 33), including MDS (34). Using data from the UK Biobank study, Thompson et al. identified 156 autosomal germline determinates of LOY (6). Genetic susceptibility to LOY was associated with risk of multiple types of cancer not only in men but also in women. Interestingly, LOY genetic susceptibility variants were also associated with age at natural menopause in women. Taken together, this suggests that LOY in men may represent a biomarker of genomic instability occurring in multiple tissue types thus supporting a “common-soil” hypothesis linking LOY to cancer risk. Future studies of genetic susceptibility to CH will provide further insights into the mechanisms linking CH to a diverse array of health outcomes.
Characterization of CH in individuals harboring MN-susceptibility genes has helped define the mechanisms driving genetic predisposition to MN. Two such examples include severe congenital neutropenia (SCN) and Shwachman-Diamond syndrome (SDS). SDS is a rare recessive disorder caused by bi-allelic mutations of SBDS that leads to impaired ribosome assembly, bone marrow failure, and MN early in life. CH due to mutations in TP53 have been shown to occur much more frequently among children with SDS (48%–76%) compared with aged-matched controls (<1%) although the frequency of other common CH mutations (DNMT3A, TET2, ASXL1) was comparable. The most common molecular event demarcating the transition of TP53 CH to MN in SDS was bi-allelic TP53 inactivation and distinguished high from low-risk patients with CH. This suggests the presence of specific stressors in SDS that strongly select for HSPCs bearing TP53 mutations and the importance of bi-allelic loss of TP53 in determining malignant progression (35, 36). Individuals with SCN also have a unique molecular profile of CH with 40% of individuals having truncating mutations in the cytoplasmic domain of granulocyte colony-stimulating factor receptor (CSF3R) compared with 0% of age-matched controls (35). Treatment with granulocyte colony-stimulating factor (G-CSF) is the standard of care for SCN, and expression of the truncated G-CSF receptor confers a sustained increased signal in response to G-CSF (37). These observations suggest that high levels of endogenous G-CSF provide a powerful selective pressure on CSF3R-mutated HSPCs among individuals with SCN. Thus, studies of CH in patients with rare, pathogenic MN susceptibility syndromes provide a unique window into the convergence of genetic and environmental factors dictating CH selection and malignant progression.
We are in the infancy of understanding how environmental factors shape the risk of MDS. Indeed, beyond established associations between MDS and benzene exposure, cigarette smoking, and cytotoxic therapy, little is known regarding environmental risk factors. Problems with the diagnosis and classification of MDS have hampered the conduct of large-scale studies. However, recent work regarding the impact of environmental factors on CH have yielded important insights into how environmental factors might shape the development of MDS. Multiple observational studies have shown that CH is enriched among those exposed to cigarette smoke (2, 3, 5) and oncologic therapy (5, 22, 23). The strength of these associations varies by gene. For example, ASXL1-mutant CH is more strongly enriched among smokers compared with DNMT3A-mutant CH (5, 38), and CH mutations in genes belonging to the cell-cycle/DNA-damage response (DDR) pathway (39) are more common following exposure to cytotoxic therapy or radiation. Specific classes of cytotoxic therapy may confer a higher selective advantage on DDR-mutant HSPCS including platinum, radionuclide therapy, and topoisomerase I/II inhibitors. Chemotherapy-induced DNA damage in HSPCs triggers activation of apoptotic pathways resulting in cell death. HSPCs with mutations in genes regulating cell-cycle arrest and apoptosis including TP53 and PPM1D carry a competitive advantage (38, 40) as HSPCs bearing these mutations result in decreased cell death following DNA damage (24, 25, 39). This suggests that different hematopoietic stressors promote the expansion of specific types of mutant clones. Indeed, this gene-specific selective pressure was confirmed previously by us through longitudinal blood sampling of solid tumor patients receiving cytotoxic therapy (5).
Conflicting epidemiologic findings exist regarding the association between chronic inflammation and risk of MN. Some studies have shown a modestly elevated risk of MN among those with a history of autoimmune diseases (41–43) while others report no significant association (44). Studies of the relationship between CH and inflammation may help address this question. Experimental data in murine model systems suggests that inflammation drives competitive advantage of CH clones. TET2-deficent and TET2-mutant HSCs show a strong proliferative advantage when exposed to exogenous TNF-α (45) and LPS-induced (46) inflammatory stress. Similarly, DNMT3A-mutant HSCs outcompete wild-type cells during infection. The frequency of CH has been reported to be elevated in individuals with some chronic inflammatory conditions [e.g., ulcerative colitis (47), HIV (48)] but not others (e.g., rheumatoid arthritis). Interpretation of these associations is limited by several factors. First, because these studies are cross-sectional, causality is difficult to determine (i.e., whether CH induces or is promoted by these conditions). Second, there are many possible confounders of these associations including related exposures such as medication effects. Serial sequencing of CH in longitudinal patient cohorts will provide further clarity regarding whether inflammatory conditions promote CH in humans.
The vast majority of individuals with CH will never develop MN. However, in a subset, clonal selection and the acquisition of additional genetic aberrations will demarcate the transition to malignancy (5, 18). Individuals with multiple mutations, mutations with high clonal burden (high VAF), mutations in TP53, the spliceosome pathway, and IDH1/2 are high-risk features (4–6). However, mutational features alone provide only modest predictive ability for risk of MN even when combined with blood count parameters and other clinical data (4, 5). Other factors likely play a major modifying role in the risk of developing MN. This could include clonal composition, epigenetic changes, or environmental factors among others.
Clonal cytopenia of undetermined significance (CCUS) is a condition in which individuals with CH also have unexplained low blood counts (cytopenia). Individuals with CCUS have a high probability of progression to hematologic malignancy, particularly MN, compared with individuals with cytopenias but no evidence of CH (5- and 10-year cumulative probability of progression: 82% vs. 9% and 95% vs. 9%, respectively) (49). Similar to CH, individuals with CCUS bearing only isolated DNMT3A mutations have a lower risk of progression to MN whereas individuals harboring TET2, ASXL1, TP53, and spliceosome mutations have higher risks of MN (50).
Characterization of hematopoietic stem cell population dynamics is critical in developing interventional approaches. CH driver mutations exert varying degrees of fitness advantage on HSPCs which partially explains variation in VAF between CH mutations (51, 52). In line with data that higher VAF CH is associated with an increased risk of MN, high-fitness variants (that confer faster CH growth) are associated with a higher risk of MN (51, 53). Longitudinal studies in healthy individuals have shown that while most clones display stable exponential growth over time, there is considerable variability with respect to accelerated and decelerated growth depending on the specific mutation and age of the individual (53). The clonal fitness and the risk of malignant progression may be shaped by not only driver mutations but also perhaps modified by co-occurring passenger mutations (14). Phylogenetic reconstruction suggests that in many cases, the CH mutations that eventually lead to MN may arise early in life, even in utero (54, 55). Thus, further characterization of the lifelong clonal dynamics that demarcate high-risk features for progression will be critical to informing MN prevention strategies.
Beyond hematologic malignancies, CH is associated with an ever increasing list of diseases and health outcomes including cardiovascular disease (24–26), chronic obstructive pulmonary disease (27), infection risk including risk of severe COVID-19 (28, 29), autoimmune diseases (30), and HIV (31). We are in the early stages of understanding the mechanisms underlying this diverse list of disease associations. For example, multiple murine models and human data suggest that CH-induced changes in the hematopoietic stem cell inflammatory milieu may casually contribute to cardiovascular disease risk (26, 32, 33). However, a recent study suggests that HSC proliferation and resultant CH is elevated in mice with atherosclerosis, an observation supported in a small patient cohort (34). A combination of both functional data and longitudinal human studies will be critical in defining causality and the mechanisms driving these associations.
While there are no established treatments for CH, surveillance and interventional strategies are an emerging area of research. Therapeutic intervention for patients with solid tumors with CH represents a possible avenue. Therapy-related MN (t-MN) is defined by the development of MN following exposure to cytotoxic chemotherapy and represents 10%–20% of MN (56). Among adult patients who develop t-MN following oncologic therapy, CH mutations (that develop into the dominant MN clone) can often be detected prior to therapy (5). Thus, assessment of CH prior to and during therapy could be used to identify patients at high risk of MN. For patients considering adjuvant therapy who have “high-risk” CH, consideration of dose reduction or avoidance of therapies known to be highly leukemogenic could be considered (5). This might be of particular relevance for individuals bearing CH mutations in TP53 which are known to be promoted by oncologic therapy. However, this would need to be carefully weighed against the survival benefits of adjuvant therapy. Formal evaluation in specific clinical scenarios would be required prior to implementation.
Elimination of CH through use of a targeted therapy is an attractive goal. In patients with malignancies in which a dominant genetic alteration drives tumor, single agent-targeted therapies are highly effective. Examples include c-KIT mutations in gastrointestinal stromal tumors (GIST) and the BCR-ABL translocation in chronic myelogenous leukemia (CML). Thus, the use of targeted therapies in the setting of CH where the vast majority of patients harbor few mutations might be expected to show superior efficacy compared with MN. However, given the low rate of progression to MN, any adverse effects of treatment aimed at clone elimination must be weighed against the benefit. In this regard, focus on patients at the highest risk such as individuals with CCUS or patients with CH and hereditary predisposition syndromes to MN are of particular interest. Clinical trials utilizing IDH inhibitors in individuals with IDH-mutant CCUS are underway (NCT05030441, NCT05102370). While attractive, IDH-mutant CH is rare and far less common than CH-associated mutations in DNMT3A, TET2, or ASXL1. As additional targeted therapies are shown to be efficacious and safe in MN, more therapeutic options for CCUS and other high-risk forms of CH will emerge. However, most mutations driving CH exert their effects through loss of function of the corresponding gene rather than gain of function and thus will be more difficult to directly target.
A key safety outcome of therapeutic studies in CH, particularly targeted therapy, will be the long-term impact of suppressing specific CH clones with regard to the risk of emergence of other CH clones of malignant potential. The mechanisms of resistance to targeted therapies are diverse and yet to be completely characterized. Broad themes include the acquisition of secondary mutations in the targeted gene that influence drug binding and the emergence or selection of clones in alternative pathways. The extent to which long-term treatment with targeted therapies might suppress/eliminate specific CH mutations at the risk of selection of more pathogenic clones is not defined. Nevertheless, application of targeted therapies to the premalignant setting will further elucidate clonal dynamics and mechanisms of resistance.
Characterization of the causes and consequences of clonal hematopoiesis will continue to provide insights into the pathogenesis of MN, including MDS. We are in the early stages of developing approaches for surveillance and intervention strategies for individuals with high-risk forms of CH. The successes (and failures) of these initial studies will provide additional avenues for optimizing MDS therapy.
IC, BW, and KB jointly wrote the manuscript. All authors listed have made a substantial, direct, and intellectual contribution to the work and approved it for publication.
KB is supported by grants from the Damon Runyon Cancer Research Foundation, the American Society of Hematology, the EvansMDS fund, and the National Cancer Institute (5K08CA241318-02).
KB receives research funding from Bristol Myers Squibb and Servier.
The remaining authors declare that the research was conducted in the absence of any commercial or financial relationships that could be construed as a potential conflict of interest.
All claims expressed in this article are solely those of the authors and do not necessarily represent those of their affiliated organizations, or those of the publisher, the editors and the reviewers. Any product that may be evaluated in this article, or claim that may be made by its manufacturer, is not guaranteed or endorsed by the publisher.
1. Blokzijl F, de Ligt J, Jager M, Sasselli V, Roerink S, Sasaki N, et al. Tissue-Specific Mutation Accumulation in Human Adult Stem Cells During Life. Nature (2016) 538:260–4. doi: 10.1038/nature19768
2. Jacobs KB, Yeager M, Zhou W, Wacholder S, Wang Z, Rodriguez-Santiago B, et al. Detectable Clonal Mosaicism and Its Relationship to Aging and Cancer. Nat Genet (2012) 44:651–8. doi: 10.1038/ng.2270
3. Jaiswal S, Fontanillas P, Flannick J, Manning A, Grauman PV, Mar BG, et al. Age-Related Clonal Hematopoiesis Associated With Adverse Outcomes. N Engl J Med (2014) 371:2488–98. doi: 10.1056/NEJMoa1408617
4. Genovese G, Kähler AK, Handsaker RE, Lindberg J, Rose SA, Bakhoum SF, et al. Clonal Hematopoiesis and Blood-Cancer Risk Inferred From Blood DNA Sequence. N Engl J Med (2014) 371:2477–87. doi: 10.1056/NEJMoa1409405
5. Bolton KL, Ptashkin RN, Gao T, Braunstein L, Devlin SM, Kelly D, et al. Cancer Therapy Shapes the Fitness Landscape of Clonal Hematopoiesis. Nat Genet (2020) 52:1219–26. doi: 10.1038/s41588-020-00710-0
6. Thompson DJ, Genovese G, Halvardson J, Ulirsch JC, Wright DJ, Terao C, et al. Genetic Predisposition to Mosaic Y Chromosome Loss in Blood. Nature (2019) 575:652–7. doi: 10.1038/s41586-019-1765-3
7. Zink F, Stacey SN, Norddahl GL, Frigge ML, Magnusson OT, Jonsdottir I, et al. Clonal Hematopoiesis, With and Without Candidate Driver Mutations, Is Common in the Elderly. Blood (2017) 130:742–52. doi: 10.1182/blood-2017-02-769869
8. Lee-Six H, Olafsson S, Ellis P, Osborne RJ, Sanders MA, Moore L, et al. The Landscape of Somatic Mutation in Normal Colorectal Epithelial Cells. Nature (2019) 574:532–7. doi: 10.1038/s41586-019-1672-7
9. Salk JJ, Loubet-Senear K, Maritschnegg E, Valentine CC, Williams LN, Higgins JE, et al. Ultra-Sensitive TP53 Sequencing for Cancer Detection Reveals Progressive Clonal Selection in Normal Tissue Over a Century of Human Lifespan. Cell Rep (2019) 28:132–44.e3. doi: 10.1016/j.celrep.2019.05.109
10. Martincorena I, Raine KM, Gerstung M, Dawson KJ, Haase K, Van Loo P, et al. Universal Patterns of Selection in Cancer and Somatic Tissues. Cell (2017) 171:1029–41.e21. doi: 10.1016/j.cell.2017.09.042
11. Martincorena I, Fowler JC, Wabik A, Lawson ARJ, Abascal F, Hall MWJ, et al. Somatic Mutant Clones Colonize the Human Esophagus With Age. Science (2018) 362(6417):911–7. doi: 10.1126/science.aau3879
12. Zhu M, Lu T, Jia Y, Luo X, Gopal P, Li L, et al. Somatic Mutations Increase Hepatic Clonal Fitness and Regeneration in Chronic Liver Disease. Cell (2019) 177:608–21.e12. doi: 10.1016/j.cell.2019.03.026
13. Tomasetti C, Vogelstein B. Variation in Cancer Risk Among Tissues can be Explained by the Number of Stem Cell Divisions. Science (2015) 347:78–81. doi: 10.1126/science.1260825
14. Cagan A, Baez-Ortega A, Brzozowska N, Abascal F, Coorens THH, Sanders MA, et al. Somatic Mutation Rates Scale With Lifespan Across Mammals. bioRxiv (2021) 2021.08.19.456982. doi: 10.1101/2021.08.19.456982.
15. Hoang ML, Kinde I, Tomasetti C, McMahon KW, Rosenquist TA, Grollman AP, et al. Genome-Wide Quantification of Rare Somatic Mutations in Normal Human Tissues Using Massively Parallel Sequencing. Proc Natl Acad Sci (2016) 113:9846–51. doi: 10.1073/pnas.1607794113
16. Kivioja T, Vähärautio A, Karlsson K, Bonke M, Enge M, Linnarsson S, et al. Counting Absolute Numbers of Molecules Using Unique Molecular Identifiers. Nat Methods (2012) 9:72–4. doi: 10.1038/nmeth.1778
17. Young AL, Challen GA, Birmann BM, Druley TE. Clonal Haematopoiesis Harbouring AML-Associated Mutations Is Ubiquitous in Healthy Adults. Nat Commun (2016) 7:12484. doi: 10.1038/ncomms12484
18. Sun J, Ramos A, Chapman B, Johnnidis JB, Le L, Ho Y-J, et al. Clonal Dynamics of Native Haematopoiesis. Nature (2014) 514:322–7. doi: 10.1038/nature13824
19. Busch K, Klapproth K, Barile M, Flossdorf M, Holland-Letz T, Schlenner SM, et al. Fundamental Properties of Unperturbed Haematopoiesis From Stem Cells. Vivo Nat (2015) 518:542–6. doi: 10.1038/nature14242
20. Sawai CM, Babovic S, Upadhaya S, Knapp DJHF, Lavin Y, Lau CM, et al. Hematopoietic Stem Cells Are the Major Source of Multilineage Hematopoiesis in Adult Animals. Immunity (2016) 45:597–609. doi: 10.1016/j.immuni.2016.08.007
21. Lee-Six H, Øbro NF, Shepherd MS, Grossmann S, Dawson K, Belmonte M, et al. Population Dynamics of Normal Human Blood Inferred From Somatic Mutations. Nature (2018) 561:473–8. doi: 10.1038/s41586-018-0497-0
22. Challen GA, Sun D, Jeong M, Luo M, Jelinek J, Berg JS, et al. Dnmt3a Is Essential for Hematopoietic Stem Cell Differentiation. Nat Genet (2012) 44:23–31. doi: 10.1038/ng.1009
23. Jeong M, Park HJ, Celik H, Ostrander EL, Reyes JM, Guzman A, et al. Loss of Dnmt3a Immortalizes Hematopoietic Stem Cells In Vivo. Cell Rep (2018) 23:1–10. doi: 10.1016/j.celrep.2018.03.025
24. Hsu JI, Dayaram T, Tovy A, De Braekeleer E, Jeong M, Wang F, et al. PPM1D Mutations Drive Clonal Hematopoiesis in Response to Cytotoxic Chemotherapy. Cell Stem Cell (2018) 23:700–13.e6. doi: 10.1016/j.stem.2018.10.004
25. Wong TN, Miller CA, Jotte MRM, Bagegni N, Baty JD, Schmidt AP, et al. Cellular Stressors Contribute to the Expansion of Hematopoietic Clones of Varying Leukemic Potential. Nat Commun (2018) 9:455. doi: 10.1038/s41467-018-02858-0
26. Loh P-R, Genovese G, Handsaker RE, Finucane HK, Reshef YA, Palamara PF, et al. Insights Into Clonal Haematopoiesis From 8,342 Mosaic Chromosomal Alterations. Nature (2018) 559:350–5. doi: 10.1038/s41586-018-0321-x
27. Landau DA, Tausch E, Taylor-Weiner AN, Stewart C, Reiter JG, Bahlo J, et al. Mutations Driving CLL and Their Evolution in Progression and Relapse. Nature (2015) 526:525–30. doi: 10.1038/nature15395
28. Bick AG, Weinstock JS, Nandakumar SK, Fulco CP, Bao EL, Zekavat SM, et al. Inherited Causes of Clonal Haematopoiesis in 97,691 Whole Genomes. Nature (2020) 586:763–8. doi: 10.1038/s41586-020-2819-2
29. Silver AJ, Bick AG, Savona MR. Germline Risk of Clonal Haematopoiesis. Nat Rev Genet (2021) 22:603–17. doi: 10.1038/s41576-021-00356-6
30. Pasca S, Gondek LP. Clonal Hematopoiesis and Bone Marrow Failure Syndromes. Best Pract Res Clin Haematol (2021) 34:101273. doi: 10.1016/j.beha.2021.101273
31. Tsai FD, Lindsley RC. Clonal Hematopoiesis in the Inherited Bone Marrow Failure Syndromes. Blood (2020) 136:1615–22. doi: 10.1182/blood.2019000990
32. Forsberg LA, Rasi C, Malmqvist N, Davies H, Pasupulati S, Pakalapati G, et al. Mosaic Loss of Chromosome Y in Peripheral Blood Is Associated With Shorter Survival and Higher Risk of Cancer. Nat Genet (2014) 46:624–8. doi: 10.1038/ng.2966
33. Loftfield E, Zhou W, Yeager M, Chanock SJ, Freedman ND, Machiela MJ. Mosaic Y Loss Is Moderately Associated With Solid Tumor Risk. Cancer Res (2019) 79:461–6. doi: 10.1158/0008-5472.CAN-18-2566
34. Wong AK, Fang B, Zhang L, Guo X, Lee S, Schreck R. Loss of the Y Chromosome: An Age-Related or Clonal Phenomenon in Acute Myelogenous Leukemia/Myelodysplastic Syndrome? Arch Pathol Lab Med (2008) 132:1329–32. doi: 10.5858/2008-132-1329-LOTYCA
35. Xia J, Miller CA, Baty J, Ramesh A, Jotte MRM, Fulton RS, et al. Somatic Mutations and Clonal Hematopoiesis in Congenital Neutropenia. Blood (2018) 131:408–16. doi: 10.1182/blood-2017-08-801985
36. Kennedy AL, Myers KC, Bowman J, Gibson CJ, Camarda ND, Furutani E, et al. Distinct Genetic Pathways Define Pre-Malignant Versus Compensatory Clonal Hematopoiesis in Shwachman-Diamond Syndrome. Nat Commun (2021) 12:1334. doi: 10.1038/s41467-021-21588-4
37. Hermans MH, Antonissen C, Ward AC, Mayen AE, Ploemacher RE, Touw IP. Sustained Receptor Activation and Hyperproliferation in Response to Granulocyte Colony-Stimulating Factor (G-CSF) in Mice With a Severe Congenital Neutropenia/Acute Myeloid Leukemia-Derived Mutation in the G-CSF Receptor Gene. J Exp Med (1999) 189:683–92. doi: 10.1084/jem.189.4.683
38. Dawoud AAZ, Tapper WJ, Cross NCP. Clonal Myelopoiesis in the UK Biobank Cohort: ASXL1 Mutations Are Strongly Associated With Smoking. Leukemia (2020) 34:2660–72. doi: 10.1038/s41375-020-0896-8
39. Wong TN, Ramsingh G, Young AL, Miller CA, Touma W, Welch JS, et al. The Role of TP53 Mutations in the Origin and Evolution of Therapy-Related AML. Nature (2015) 518:552–5. doi: 10.1038/nature13968
40. Coombs CC, Zehir A, Devlin SM, Kishtagari A, Syed A, Jonsson P, et al. Therapy-Related Clonal Hematopoiesis in Patients With Non-Hematologic Cancers Is Common and Associated With Adverse Clinical Outcomes. Cell Stem Cell (2017) 21:374–82.e4. doi: 10.1016/j.stem.2017.07.010
41. Anderson LA, Pfeiffer RM, Landgren O, Gadalla S, Berndt SI, Engels EA. Risks of Myeloid Malignancies in Patients With Autoimmune Conditions. Br J Cancer (2009) 100:822–8. doi: 10.1038/sj.bjc.6604935
42. Kristinsson SY, Björkholm M, Hultcrantz M, Derolf ÅR, Landgren O, Goldin LR. Chronic Immune Stimulation Might Act as a Trigger for the Development of Acute Myeloid Leukemia or Myelodysplastic Syndromes. J Clin Oncol Off J Am Soc Clin Oncol (2011) 29:2897–903. doi: 10.1200/JCO.2011.34.8540
43. Johnson KJ, Blair CM, Fink JM, Cerhan JR, Roesler MA, Hirsch BA, et al. Medical Conditions and Risk of Adult Myeloid Leukemia. Cancer Causes Control CCC (2012) 23:1083–9. doi: 10.1007/s10552-012-9977-y
44. Østgård LSG, Nørgaard M, Pedersen L, Østgård RD, Medeiros BC, Overgaard UM, et al. Autoimmune Diseases, Infections, Use of Antibiotics and the Risk of Acute Myeloid Leukaemia: A National Population-Based Case-Control Study. Br J Haematol (2018) 181:205–14. doi: 10.1111/bjh.15163
45. Abegunde SO, Buckstein R, Wells RA, Rauh MJ. An Inflammatory Environment Containing Tnfα Favors Tet2-Mutant Clonal Hematopoiesis. Exp Hematol (2018) 59:60–5. doi: 10.1016/j.exphem.2017.11.002
46. Cai Z, Kotzin JJ, Ramdas B, Chen S, Nelanuthala S, Palam LR, et al. Inhibition of Inflammatory Signaling in Tet2 Mutant Preleukemic Cells Mitigates Stress-Induced Abnormalities and Clonal Hematopoiesis. Cell Stem Cell (2018) 23:833–49.e5. doi: 10.1016/j.stem.2018.10.013
47. Zhang CRC, Nix D, Gregory M, Ciorba MA, Ostrander EL, Newberry RD, et al. Inflammatory Cytokines Promote Clonal Hematopoiesis With Specific Mutations in Ulcerative Colitis Patients. Exp Hematol (2019) 80:36–41.e3. doi: 10.1016/j.exphem.2019.11.008
48. Dharan NJ, Yeh P, Bloch M, Yeung MM, Baker D, Guinto J, et al. HIV Is Associated With an Increased Risk of Age-Related Clonal Hematopoiesis Among Older Adults. Nat Med (2021) 27:1006–11. doi: 10.1038/s41591-021-01357-y
49. Malcovati L, Gallì A, Travaglino E, Ambaglio I, Rizzo E, Molteni E, et al. Clinical Significance of Somatic Mutation in Unexplained Blood Cytopenia. Blood (2017) 129:3371–8. doi: 10.1182/blood-2017-01-763425
50. Gallì A, Todisco G, Catamo E, Sala C, Elena C, Pozzi S, et al. Relationship Between Clone Metrics and Clinical Outcome in Clonal Cytopenia. Blood (2021) 138:965–76. doi: 10.1182/blood.2021011323
51. Watson CJ, Papula AL, Poon GYP, Wong WH, Young AL, Druley TE, et al. The Evolutionary Dynamics and Fitness Landscape of Clonal Hematopoiesis. Science (2020) 367:1449–54. doi: 10.1126/science.aay9333
52. Skead K, Ang Houle A, Abelson S, Agbessi M, Bruat V, Lin B, et al. Interacting Evolutionary Pressures Drive Mutation Dynamics and Health Outcomes in Aging Blood. Nat Commun (2021) 12:4921. doi: 10.1038/s41467-021-25172-8
53. Fabre MA, Almeida JG, Fiorillo E, Mitchell E, Damaskou A, Rak J, et al. The Longitudinal Dynamics and Natural History of Clonal Haematopoiesis. bioRxiv (2021) 2021.8.12.455048. doi: 10.1101/2021.08.12.455048.
54. Van Egeren D, Escabi J, Nguyen M, Liu S, Reilly CR, Patel S, et al. Reconstructing the Lineage Histories and Differentiation Trajectories of Individual Cancer Cells in Myeloproliferative Neoplasms. Cell Stem Cell (2021) 28:514–23.e9. doi: 10.1016/j.stem.2021.02.001
55. Williams N, Lee J, Moore L, Baxter EJ, Hewinson J, Dawson KJ, et al. Phylogenetic Reconstruction of Myeloproliferative Neoplasm Reveals Very Early Origins and Lifelong Evolution. bioRxiv (2020) 2020.11.09.374710. doi: 10.1101/2020.11.09.374710
Keywords: clonal hematopoiesis (CH), myeloid neoplasm, genetic predisposition, environmental risk, myelodysplastic syndrome (MDS)
Citation: Chan ICC, Wiley BJ and Bolton KL (2022) What Clonal Hematopoiesis Can Teach Us About MDS. Front. Oncol. 12:794021. doi: 10.3389/fonc.2022.794021
Received: 13 October 2021; Accepted: 11 January 2022;
Published: 08 February 2022.
Edited by:
Amy DeZern, Johns Hopkins Medicine, United StatesReviewed by:
Lukasz Gondek, Johns Hopkins University, United StatesCopyright © 2022 Chan, Wiley and Bolton. This is an open-access article distributed under the terms of the Creative Commons Attribution License (CC BY). The use, distribution or reproduction in other forums is permitted, provided the original author(s) and the copyright owner(s) are credited and that the original publication in this journal is cited, in accordance with accepted academic practice. No use, distribution or reproduction is permitted which does not comply with these terms.
*Correspondence: Kelly L. Bolton, Ym9sdG9uQHd1c3RsLmVkdQ==
†These authors have contributed equally to this work
Disclaimer: All claims expressed in this article are solely those of the authors and do not necessarily represent those of their affiliated organizations, or those of the publisher, the editors and the reviewers. Any product that may be evaluated in this article or claim that may be made by its manufacturer is not guaranteed or endorsed by the publisher.
Research integrity at Frontiers
Learn more about the work of our research integrity team to safeguard the quality of each article we publish.