- 1Department of Oncology, The First Affiliated Hospital of Zhengzhou University, Zhengzhou, China
- 2Department of Cell Biology, Wuhan Institute of Bioengineering, Wuhan, China
Triple negative breast cancer (TNBC) refers to the subtype of breast cancer which is negative for ER, PR, and HER-2 receptors. Tumor-associated macrophages (TAMs) refer to the leukocyte infiltrating tumor, derived from circulating blood mononuclear cells and differentiating into macrophages after exuding tissues. TAMs are divided into typical activated M1 subtype and alternately activated M2 subtype, which have different expressions of receptors, cytokines and chemokines. M1 is characterized by expressing a large amount of inducible nitric oxide synthase and TNF-α, and exert anti-tumor activity by promoting pro-inflammatory and immune responses. M2 usually expresses Arginase 1 and high levels of cytokines, growth factors and proteases to support their carcinogenic function. Recent studies demonstrate that TAMs participate in the process of TNBC from occurrence to metastasis, and might serve as potential biomarkers for prognosis prediction.
Introduction
Triple negative breast cancer (TNBC) refers to the subtype of breast cancer which is negative for ER, PR, and HER-2 receptors, accounting for approximately 12%-17% of invasive breast cancer (1). Compared with other breast cancer subtypes, TNBC has the highest recurrence rate and metastasis rate. Chemotherapy is most common treatment for TNBC currently while drug resistance, non-target characteristics and severe systemic side effects lead to ineffective prognosis for patients with metastatic cases (2, 3). Therefore, it is necessary to discover cutting-edge and effective treatment strategies for TNBC.
In recent years, the treatment strategy based on the interference and remodeling of the tumor microenvironment (TME) has gradually emerged (4). With the deepening of research on TME, it is found that immune cells in TME play a complex and non-negligible role in tumor progression. Among them, tumor-associated macrophages (TAMs) are such a kind of important components (5, 6). TAMs participate in the process of TNBC from occurrence to metastasis, and have potential value in evaluating disease-free survival and overall survival of TNBC (7, 8).
TAMs refer to the leukocyte infiltrating tumor, derived from circulating blood mononuclear cells and differentiating into macrophages after exuding tissues (9–12). Increasing evidence indicates that macrophages are not homogeneous. They can be divided into specific subgroups based on polarization requirements, phenotype and function. TAMs are divided into typical activated M1 subtype and alternately activated M2 subtype, which have different expressions of receptors, cytokines and chemokines (13–15). M1 is characterized by expressing a large amount of inducible nitric oxide synthase and TNF-α, and exert anti-tumor activity by promoting pro-inflammatory and immune responses (5, 16, 17). M2 usually expresses Arginase 1 and high levels of cytokines, growth factors and proteases to support their carcinogenic function (18, 19). In addition, M2 is involved in stimulating tumor angiogenesis, matrix remodeling, tumor cell migration and invasion, and promoting immune suppression (7, 8) (Figure 1).
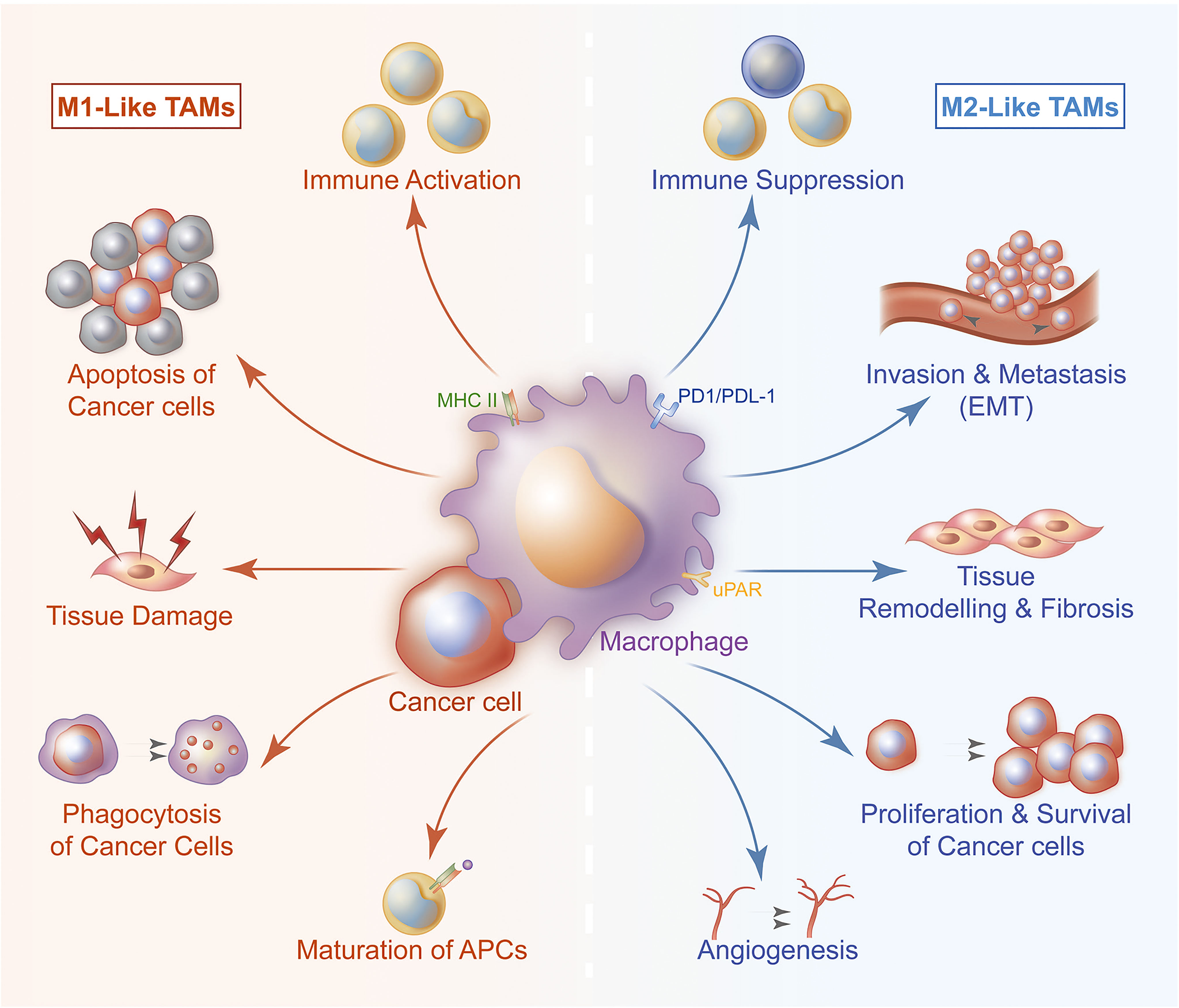
Figure 1 TAMs are divided into typical activated M1 subtype and alternately activated M2 subtype. The two subtypes participate in the process of TNBC from occurrence to metastasis of TNBC. M1 exerts anti-tumor activity by promoting pro-inflammatory and immune responses. M1 can present antigen to immune cell, then these cells are activated and directed to kill tumor cells. M1 can also secrete cytokines to induce tumor cells apoptosis. M2 is involved in stimulating tumor angiogenesis, matrix remodeling, tumor cell migration and invasion, it also plays a significant role in immune suppression and tissue repair.
Polarization and Induction of TAMs
Circulating precursors of macrophages derived from bone marrow are recruited into the tumor microenvironment which are affected by inflammatory mediators and chemokines to participate in the tumor immune response (20, 21). Recruited TAMs can differentiate into macrophages with different phenotypes and functions under diverse activation conditions. Stimulation of Lipopolysaccharide (LPS), IFN-γ, etc. is the classical pathway to activate macrophages (M1 type); Stimulation of anti-inflammatory factors such as IL-10 and TGF-β, is a non-classical pathway to activate macrophages (M2) (Table 1) (21–25).
M1 macrophages are highly effective pro-inflammatory immune effector cells that release superoxide anions and nitrogen free radicals after injury or inflammatory activation (26, 27). It plays a role of extracellular killing and present antigens to T cells triggering anti-tumor effects. IL-1β is a cytokine, with the concentration-dependent anti-tumor effect, secreted by macrophage. LPS can activate macrophages to secrete IL-1β. Cytolysin A (ClyA), secreted by Gram-negative bacteria, have been proven to induce IL-1β secretion, which can enhance the tumoricidal activities (28). M2 macrophages have a negative regulatory effect on tumor immunity by repairing damaged tissues and inhibiting inflammation (29). In the tumor environment, IL-10 and TGF-β can transform macrophages from M1 phenotype into M2 phenotype (15–17, 26, 34) (Figure 1). Not only the specific cytokines or factors can render the macrophage become M2, injury or damage can also make macrophage polarize to M2. In return, M2 secrete Arginase 1 (Arg1), VEGF and TNF-α to repair damage through the CXCL-10/CXCR3 pathway (30).
Accumulated evidence have shown that TAMs in the tumor tissues tend to polarize to M2 type once they affected or interact with tumor extracellular matrix (23, 34). By co-inoculating macrophages RAW264.7 and triple-negative breast cancer cells 4T1 into the mammary ducts of mice, process of TNBC progression from carcinoma in situ to invasive carcinoma was simulated (35–37). It was found that the expression level of IL-12 related to M1 macrophages in the co-inoculation group was significantly lower than that in the macrophage alone group during the process of cell inoculation to tumor cells and breaking through the duct basement membrane (38, 39). The level of TGF-β1, a M2- related cytokine, was significantly increased, accompanied by distant lymph node and lung metastasis. In addition, an increasing level of MMP-8 and VEGF in the peripheral blood of mice was also observed. MMP-8 and VEGF are important M1/M2 polarization inducing factors (37). Therefore, under the induction of tumor cells, M2 polarization of TAMs can be considered as an alternating positive feedback process (Figure 1).
Tumor Immunosuppression and Immune Escape
Macrophages have strong phagocytic ability and antigen presentation ability which play an important role in connecting innate immunity and adaptive immunity (40–45). In TME, TAMs switch from M1 subtype with tumor killing function to M2 subtype with tissue repair function, which greatly weakens tumor killing ability of tumor system (18, 44, 46–49). Transformation from M1 subtype to M2 subtype can limit inherent recognition and phagocytic abilities of macrophages and tumor-killing ability of CD4+ T cells and CD8+ T cells that cooperate with them. It can also activates Treg cells and helper T cells causing tumor immunosuppression (50–58) (Figure 1).
As a regulatory factor which limits killing effect of T cells, PD-1 is widely present in a variety of T cells (59–63). PD-L1 is the receptor of PD-1, which is mostly expressed on the surface of tumor cells and macrophages (61, 64–66). TNBC cells can highly express PD-L1 so that their T cell killing effect in the tumor environment is significantly inhibited (56, 67–70). TAMs can secrete a variety of cytokines in the TNBC environment, which mediate their immunosuppressive and tumor-promoting activities (71–73). Studies have found that TAMs can secrete IFN-γ through JAK/STAT3 and PI3K/AKT signaling pathways, thereby inducing the expression of PD-L1 (74, 75). IL-6 is related to growth of TNBC and prognosis of patients. In the absence of IL-6, expression of PD-L1 is enhanced and the anti-PD-L1 antibody’s inhibitory activity in vivo is more significant (76–79). IL-18 is a pleiotropic cytokine member of the IL-1 family which has pro-inflammatory and anti-inflammatory functions (77, 80). It is produced by a variety of cell types including macrophages. Tumor-derived IL-18 levels are significantly related to the low survival rate of TNBC patients (81–83). TGF-β is a multifunctional cytokine, which participates in the production of Treg in the mouse tumor microenvironment and supports its suppression of effector T cells (18). TGF-β can also increase its inhibitory activity by inducing polarization of TAM to M2 phenotype, and induce the up-regulation of PD-L1 leading to tumor escape (84, 85). In addition, TAMs can also promote the development and activity of PD-1+Treg, and then participate in TNBC tumor immune escape (55, 86). PD-1 was also reported to be expressed on the surface of TAMs and mainly exist as type M2 (56, 87–89). Compare to CD68, a surface marker of M2, CD163 and CD260 are major markers with more specificity to help us identify M2 (Table 1) (90, 91).
TAMs with high expression of PD-1 have reduced phagocytic ability, which reduce anti-tumor immune effect to a certain extent (89). It blocks PD -1/PD-L1 binding and enhances the phagocytic ability of macrophages to inhibit tumor growth and effectively prolong the survival time of tumor-bearing mice (47). Under induction of tumor cells, TAMs become important mediators and regulatory factors for tumor immunosuppression and immune escape.
Promoting Tumor Blood Vessel and Lymphatic Vessel Formation
Tumor angiogenesis is an essential part of TNBC proliferation and metastasis (14, 92, 93). TAMs plays an indispensable role in promoting tumor angiogenesis (93). Hypoxia is a typical characteristic of solid tumors (94). Expression of HIF-1 is up-regulated in TNBC, which activates the HIF-CSF pathway and recruits a large number of macrophages to the tumor area (95, 96). This process is a key step in the recruitment of macrophages in TME. Recruited macrophages can participate in various stages of tumor angiogenesis. For example, matrix metalloproteinases and proteolytic enzymes produced by macrophages can reconstruct the extracellular matrix and provide favorable conditions for the formation of new blood vessels (93). Cytokines secreted by macrophages can provide a connecting framework for new blood vessels (20). Macrophages in TME can promote growth of lymphatic endothelial cells and provide support for tumor lymphatic metastasis. Induced by tumor cells, macrophages overexpress β4 integrin which forces macrophages to aggregate and adhere to the proximal end of lymphatic vessels. At the same time, their own expression of TGF-β1 drives the contraction of lymphatic endothelial cells (97) (Figure 1). Aggregated macrophages undergo lymphatic remodeling by increasing permeability and destroying surrounding tissues to achieve tumor cell metastasis via lymphatic pathways. Macrophages’ tissue function including renewal and remodeling of blood vessels together with lymphatic vasculature, though it improves the aggressiveness of tumors in the tumor environment.
TAMs and TNBC Migration and Invasion
It has been found that TAMs can enhance tumor cell stemness and increase tumor cell invasiveness by participating in epithelial-mesenchymal transition (EMT) (7, 98–100). Matrix metalloproteinases (MMPs), cysteine cathepsin and serine proteases secreted by TAMs can hydrolyze the extracellular matrix, which is conducive to invasion of tumor cells to surroundings (Figure 1). After co-incubating macrophages with different phenotypes with breast cancer cells, it was found that those co-incubated with M1 macrophages appeared as cobblestone-like epithelial-like cells under microscope. Compared with M2 macrophages, co-incubators appear as slenderer mesenchymal-like cells. In addition, E-cadherin in co-incubated with M2 group was significantly higher in co-incubated with M1 macrophages (7, 98, 100–104). It shows that M1 TAMs have the potential to reverse EMT, which can reduce the invasiveness of tumor cells to a certain extent.
TAMs and Prognosis Prediction
High density of TAMs in TNBC is associated with poor prognosis and indicates a higher risk of metastasis (105). TAMs immunohistochemical staining of tumor tissues found that patients with higher pathological grades were often accompanied by higher TAMs level. Compared with patients with low TAMs infiltration, the overall survival and disease-free survival of patients with higher group was significantly shortened (39, 51, 106). CD163 and CD204 are relatively specific markers for M2, the breast cancer infiltration of CD163 positive and CD204 positive TAMs tends to have a poor prognosis as these TAMs are associated with fast proliferation, poor differentiation (31–33). In addition, infiltrating TAMs also have a certain impact on the efficacy of chemotherapy. Common TNBC chemotherapeutic drugs can activate TAMs, and activated TAMs can promote the repair of damaged tumor tissues, thereby inducing chemotherapy tolerance (107, 108). Not only that, TAMs can produce a large amount of IL-10, which can inhibit the production of IL-12 by dendritic cells and limit the immune killing of tumors with CD8+ T cells (39). Further research found that compared with the number of TAMs, the phenotype of TAMs is more suitable for predicting efficacy of TNBC anthracycline chemotherapeutics (109).
TAMs-Based Targeted Therapy
In recent years, the targeted therapy of TAMs mainly focuses on inhibiting TAMs recruitment, TAMs depletion, and reversing the polarization of TAMs (110). Blocking the effect of chemokines is an important method to inhibit the recruitment of TAMs, and the current research targets are mostly CCL2/CCR2 (52, 111). The inhibition of the CCL2/CCR2 axis can reduce the mobilization of bone marrow mononuclear cells, thereby reducing the infiltration of macrophages in the breast (112, 113). Studies have shown that trabectedine and bortezomib can inhibit the recruitment of macrophages by reducing the content of CCL2 in plasma (114). CCL5 can induce the recurrence of breast cancer by recruiting macrophages in residual tumors. CCL5 may become an important target for adjuvant chemotherapy and curbing recurrence of TNBC (39, 115, 116). Cytokines can effectively regulate the polarization direction of TAMs. For instance, when TAMs are exposed to cytokines secreted by CD4+Th1 cells (such as TNF, IL-12, etc.), TAMs tend to be polarized as M1 type. The NF-kB pathway is an important pathway that regulates the transcription of CD4+Th1 cytokines. Activating the NF-kB pathway can promote the polarization of TAMs to M1 type, thereby inhibiting the progress of TNBC (18, 117).
Bisphosphonate-based macrophage apoptosis inducers have been widely used in TAMs depletion (118). Bisphosphonate is easily captured by macrophages through endocytosis. The internalized Bisphosphonate can inhibit the activity of famesyl diphosphate (FPP) synthase and induce macrophage apoptosis by limiting the prenylation of RAS-related proteins. Continuous administration of zoledronic acid in a mouse spontaneous breast cancer model can significantly reduce angiogenesis, reduce the density of TAMs, and improve survival. Many clinical trials have shown that bisphosphonate therapy in post-menopausal women with breast cancer have a significant benefit (119). However, it is not applied to the menopausal women (120).
CSF1 and CCL2 play a key role in the generation of TAM and are related to the growth of TNBC tumors (61, 121, 122). Inhibition of CSF1 in vivo can reduce TAM infiltration and tumor growth and progression. Blocking CSF-1 can affect the osteoclast production of cancer cells in the co-culture system (123). Similarly, inhibiting CCL2 can block tumor stem cell renewal and M2 recruitment, thereby inhibiting the progression of TNBC (124, 125). This indicates that inhibiting CSF1 and CCL2 may be an effective strategy to reduce the accumulation of TAM. The transcription factor NF-κB can regulate the expression of tumor-promoting genes (IL-6 and TNF-α). By activating the activity of NF-κB through the IKKβ pathway, TAM can be re-cultured to the M1 phenotype (126). Therefore, converting M2 to the anti-tumor M1 phenotype may be a potentially effective strategy for cancer patients.
In addition, regulating the expression of PD-1/PD-L1 by regulating various cytokines secreted by TAMs is also a potential therapeutic strategy (56). For example, JAK/STAT3 signal is related to PD-L1 overexpression induced by IFN-γ. Inhibition of STAT3 signal by WP1066 can reduce tumor-related endothelial angiogenesis and invasion, thereby reducing the incidence of brain metastasis (56). TGF-β is related to M2 polarization and PD-L1 overexpression (48). Therefore, the combination of TGF-β inhibitors and anti-PD-1/PD-L1 specific antibodies is reasonable in clinical practice, and related clinical trials are also underway.
Application of nanoparticle targeted drug delivery systems to traditional TAMs is currently a hot research topic (109, 127). Nanoparticles can carry drugs, metal materials, and miRNAs, which can work together to interfere with TAMs through a variety of mechanisms of action. Studies have found that dextran-coated iron oxide nanoparticles can generate reactive oxygen species (ROS) through the Fenton reaction mediated by iron oxide, which mediates the repolarization of TAMs to M1 macrophages, thereby inhibiting breast cancer progression (128). Incorporation chemotherapy with macrophage-related treatment can enhance the antitumor effect by recruiting macrophage to TAM and induce M2 polarize to M1 (22, 129).
Conclusion and Perspectives
TAMs are an important component of the tumor microenvironment and occupy a high proportion of immune cells (45). They participate in whole process of TNBC occurrence, development and metastasis by regulating tumor cell immune evasion, tumor blood vessel and lymphangiogenesis (130). The phenotypic transition of TAMs in TME promotes the tumor immune microenvironment to change from an anti-tumor state to an immunosuppressive state. This dynamic change makes TAMs an important part of regulating tumor behavior and feedback on efficacy evaluation. In view of the important role of TAMs in tumor progression, treatment strategies based on TAMs have emerged. Due to the high heterogeneity of TNBC, targeted therapy for a single TAMs-related pathway often comes to failure. In the future, cooperation macrophage-targeted therapy with conventional chemotherapy, immunotherapy and adjuvant therapy maybe a promising choice for TNBC (Table 2), and multimodal targeted therapy based on TAMs may become a research hotspot (131).
Author Contributions
RQ, RL, XQ, and ZL contributed to the conception of the study and wrote the manuscript. All authors contributed to the article and approved the submitted version.
Conflict of Interest
The authors declare that the research was conducted in the absence of any commercial or financial relationships that could be construed as a potential conflict of interest.
Publisher’s Note
All claims expressed in this article are solely those of the authors and do not necessarily represent those of their affiliated organizations, or those of the publisher, the editors and the reviewers. Any product that may be evaluated in this article, or claim that may be made by its manufacturer, is not guaranteed or endorsed by the publisher.
Abbreviations
TNBC, Triple negative breast cancer; ER, estrogen receptor; PR, progesterone receptor; HER-2, human epidermal growth factor receptor 2; TME, tumor microenvironment; ClyA, Cytolysin A; LPS, Lipopolysaccharide; Arg1, Arginase I; IFN-γ, Interferon-gamma; TAMs, tumor-associated macrophages; TGF-β, Transforming growth factor beta; VEGF, vascular endothelial growth factor; TNF-α, Tumor necrosis factor alpha; IL, Interleukin; iNOS, Inducible nitric oxide synthase; CXCL-10:C-X-C Motif Chemokine Ligand 10 also known as Interferon γ–induced protein-10 (IP-10); CXCR3, C-X-C Motif Chemokine Receptor 3; CSF1, Colony stimulating factor 1; CCL2, Chemokine ligand 2; CCR2, chemokine receptor 2; FPP, famesyl diphosphate; PD-1, Programmed death-1; PD-L1, Programmed death-1 receptor; ROS, reactive oxygen species; EMT, epithelial-mesenchymal transition; MMPs, Matrix metalloproteinases.
References
1. Bergin ART, Loi S. Triple-Negative Breast Cancer: Recent Treatment Advances. F1000Res (2019) 8:F1000 Faculty Rev-1342. doi: 10.12688/f1000research.18888.1
2. Costa RLB, Gradishar WJ. Triple-Negative Breast Cancer: Current Practice and Future Directions. J Oncol Pract (2017) 13(5):301–3. doi: 10.1200/jop.2017.023333
3. André F, Zielinski CC. Optimal Strategies for the Treatment of Metastatic Triple-Negative Breast Cancer With Currently Approved Agents. Ann Oncol (2012) 23:vi46–51. doi: 10.1093/annonc/mds195
4. Mathot L, Stenninger J. Behavior of Seeds and Soil in the Mechanism of Metastasis: A Deeper Understanding. Cancer Sci (2012) 103(4):626–31. doi: 10.1111/j.1349-7006.2011.02195.x
5. Vinogradov S, Warren G, Wei X. Macrophages Associated With Tumors as Potential Targets and Therapeutic Intermediates. Nanomed (Lond) (2014) 9(5):695–707. doi: 10.2217/nnm.14.13
6. Siveen KS, Kuttan G. Role of Macrophages in Tumour Progression. Immunol Lett (2009) 123(2):97–102. doi: 10.1016/j.imlet.2009.02.011
7. Zhang W-j, Wang X-h, Gao S-t, Chen C, Xu X-y, Sun Q, et al. Tumor-Associated Macrophages Correlate With Phenomenon of Epithelial-Mesenchymal Transition and Contribute to Poor Prognosis in Triple-Negative Breast Cancer Patients. J Surg Res (2018) 222:93–101. doi: 10.1016/j.jss.2017.09.035
8. Yuan Z-Y, Luo R-Z, Peng R-J, Wang S-S, Xue C. High Infiltration of Tumor-Associated Macrophages in Triple-Negative Breast Cancer is Associated With a Higher Risk of Distant Metastasis. OncoTargets Ther (2014) 7:1475–80. doi: 10.2147/OTT.S61838
9. Munir MT, Kay MK, Kang MH, Rahman MM, Al-Harrasi A, Choudhury M, et al. Tumor-Associated Macrophages as Multifaceted Regulators of Breast Tumor Growth. Int J Mol Sci (2021) 22(12):6526. doi: 10.3390/ijms22126526
10. Tan Y, Wang M, Zhang Y, Ge S, Zhong F, Xia G, et al. Tumor-Associated Macrophages: A Potential Target for Cancer Therapy. Front Oncol (2021) 11:693517. doi: 10.3389/fonc.2021.693517
11. Li C, Xu X, Wei S, Jiang P, Xue L, Wang J. Tumor-Associated Macrophages: Potential Therapeutic Strategies and Future Prospects in Cancer. J Immunother Cancer (2021) 9(1):e001341. doi: 10.1136/jitc-2020-001341
12. Yan S, Wan G. Tumor-Associated Macrophages in Immunotherapy. FEBS J (2021) 288(21):6174–86. doi: 10.1111/febs.15726
13. Noy R, Pollard JW. Tumor-Associated Macrophages: From Mechanisms to Therapy. Immunity (2014) 41(1):49–61. doi: 10.1016/j.immuni.2014.06.010
14. Biswas SK, Allavena P, Mantovani A. Tumor-Associated Macrophages: Functional Diversity, Clinical Significance, and Open Questions. Semin Immunopathol (2013) 35(5):585–600. doi: 10.1007/s00281-013-0367-7
15. Galdiero MR, Garlanda C, Jaillon S, Marone G, Mantovani A. Tumor Associated Macrophages and Neutrophils in Tumor Progression. J Cell Physiol (2013) 228(7):1404–12. doi: 10.1002/jcp.24260
16. Hu W, Li X, Zhang C, Yang Y, Jiang J, Wu C. Tumor-Associated Macrophages in Cancers. Clin Trans Oncol (2016) 18(3):251–8. doi: 10.1007/s12094-015-1373-0
17. Chanmee T, Ontong P, Konno K, Itano N. Tumor-Associated Macrophages as Major Players in the Tumor Microenvironment. Cancers (2014) 6(3):1670–90. doi: 10.3390/cancers6031670
18. Sawa-Wejksza K, Kandefer-Szerszeń M. Tumor-Associated Macrophages as Target for Antitumor Therapy. Archivum Immunol Ther Experimentalis (2018) 66(2):97–111. doi: 10.1007/s00005-017-0480-8
19. Mantovani A, Allavena P. The Interaction of Anticancer Therapies With Tumor-Associated Macrophages. J Exp Med (2015) 212(4):435–45. doi: 10.1084/jem.20150295
20. Wang J, Li D, Cang H, Guo B. Crosstalk Between Cancer and Immune Cells: Role of Tumor-Associated Macrophages in the Tumor Microenvironment. Cancer Med (2019) 8(10):4709–21. doi: 10.1002/cam4.2327
21. Pathria P, Louis TL, Varner JA. Targeting Tumor-Associated Macrophages in Cancer. Trends Immunol (2019) 40(4):310–27. doi: 10.1016/j.it.2019.02.003
22. Adams S, Wuescher LM, Worth R, Yildirim-Ayan E. Mechano-Immunomodulation: Mechanoresponsive Changes in Macrophage Activity and Polarization. Ann BioMed Eng (2019) 47(11):2213–31. doi: 10.1007/s10439-019-02302-4
23. Zhou K, Cheng T, Zhan J, Peng X, Zhang Y, Wen J, et al. Targeting Tumor-Associated Macrophages in the Tumor Microenvironment. Oncol Lett (2020) 20(5):234. doi: 10.3892/ol.2020.12097.
24. Shu Y, Cheng P. Targeting Tumor-Associated Macrophages for Cancer Immunotherapy. Biochim Biophys Acta Rev Cancer (2020) 1874(2):188434. doi: 10.1016/j.bbcan.2020.188434
25. Salmaninejad A, Valilou SF, Soltani A, Ahmadi S, Abarghan YJ, Rosengren RJ, et al. Tumor-Associated Macrophages: Role in Cancer Development and Therapeutic Implications. Cell Oncol (Dordrecht) (2019) 42(5):591–608. doi: 10.1007/s13402-019-00453-z
26. Pan Y, Yu Y, Wang X, Zhang T. Tumor-Associated Macrophages in Tumor Immunity. Front Immunol (2020) 11:583084. doi: 10.3389/fimmu.2020.583084
27. Malekghasemi S, Majidi J, Baghbanzadeh A, Abdolalizadeh J, Baradaran B, Aghebati-Maleki L. Tumor-Associated Macrophages: Protumoral Macrophages in Inflammatory Tumor Microenvironment. Adv Pharm Bull (2020) 10(4):556–65. doi: 10.34172/apb.2020.066
28. Guan Y, Chen JQ, Li XY, Jiang SN. ClyA Enhances LPS-Induced IL-1beta Secretion in Human Macrophages Through TLR4 and NLRP3 Signaling. J Biol Regul Homeost Agents (2021) 35(2):495–504. doi: 10.23812/20-500-A
29. Wang LX, Zhang SX, Wu HJ, Rong XL, Guo J. M2b Macrophage Polarization and its Roles in Diseases. J Leukoc Biol (2019) 106(2):345–58. doi: 10.1002/JLB.3RU1018-378RR
30. Li LX, Xia YT, Sun XY, Li LR, Yao L, Ali MI, et al. CXCL-10/CXCR3 in Macrophages Regulates Tissue Repair by Controlling the Expression of Arg1, VEGFa and TNFalpha. J Biol Regul Homeost Agents (2020) 34(3):987–99. doi: 10.23812/20-59-A-65.
31. Jamiyan T, Kuroda H, Yamaguchi R, Abe A, Hayashi M. CD68- and CD163-Positive Tumor-Associated Macrophages in Triple Negative Cancer of the Breast. Virchows Arch (2020) 477(6):767–75. doi: 10.1007/s00428-020-02855-z
32. Sousa S, Brion R, Lintunen M, Kronqvist P, Sandholm J, Monkkonen J, et al. Human Breast Cancer Cells Educate Macrophages Toward the M2 Activation Status. Breast Cancer Res (2015) 17:101. doi: 10.1186/s13058-015-0621-0
33. Zhang B, Cao M, He Y, Liu Y, Zhang G, Yang C, et al. Increased Circulating M2-Like Monocytes in Patients With Breast Cancer. Tumour Biol (2017) 39(6):1010428317711571. doi: 10.1177/1010428317711571
34. Laviron M, Boissonnas A. Ontogeny of Tumor-Associated Macrophages. Front Immunol (2019) 10:1799. doi: 10.3389/fimmu.2019.01799
35. Liu Y, Ji X, Kang N, Zhou J, Liang X, Li J, et al. Tumor Necrosis Factor α Inhibition Overcomes Immunosuppressive M2b Macrophage-Induced Bevacizumab Resistance in Triple-Negative Breast Cancer. Cell Death Dis (2020) 11(11):993. doi: 10.1038/s41419-020-03161-x
36. Donzelli S, Sacconi A, Turco C, Gallo E, Milano E, Iosue I, et al. Paracrine Signaling From Breast Cancer Cells Causes Activation of ID4 Expression in Tumor-Associated Macrophages. Cells (2020) 9(2):418. doi: 10.3390/cells9020418
37. Steenbrugge J, Breyne K, Demeyere K, De Wever O, Sanders NN, Van Den Broeck W, et al. Anti-Inflammatory Signaling by Mammary Tumor Cells Mediates Prometastatic Macrophage Polarization in an Innovative Intraductal Mouse Model for Triple-Negative Breast Cancer. J Exp Clin Cancer Res: CR (2018) 37(1):191–1. doi: 10.1186/s13046-018-0860-x
38. Zhuang H, Dai X, Zhang X, Mao Z, Huang H. Sophoridine Suppresses Macrophage-Mediated Immunosuppression Through TLR4/IRF3 Pathway and Subsequently Upregulates CD8(+) T Cytotoxic Function Against Gastric Cancer. Biomed Pharmacother = Biomed Pharmacother (2020) 121:109636. doi: 10.1016/j.biopha.2019.109636
39. Wang J, Chen H, Chen X, Lin H. Expression of Tumor-Related Macrophages and Cytokines After Surgery of Triple-Negative Breast Cancer Patients and its Implications. Med Sci Monit (2016) 22:115–20. doi: 10.12659/msm.895386
40. Lavy M, Gauttier V, Poirier N, Barillé-Nion S, Blanquart C. Specialized Pro-Resolving Mediators Mitigate Cancer-Related Inflammation: Role of Tumor-Associated Macrophages and Therapeutic Opportunities. Front Immunol (2021) 12:702785. doi: 10.3389/fimmu.2021.702785
41. Sami E, Paul BT, Koziol JA, ElShamy WM. The Immunosuppressive Microenvironment in BRCA1-IRIS-Overexpressing TNBC Tumors Is Induced by Bidirectional Interaction With Tumor-Associated Macrophages. Cancer Res (2020) 80(5):1102–17. doi: 10.1158/0008-5472.can-19-2374
42. Yang S, Liu Q, Liao Q. Tumor-Associated Macrophages in Pancreatic Ductal Adenocarcinoma: Origin, Polarization, Function, and Reprogramming. Front Cell Dev Biol (2020) 8:607209. doi: 10.3389/fcell.2020.607209
43. Stopforth RJ, Ward ES. The Role of Antigen Presentation in Tumor-Associated Macrophages. Crit Rev Immunol (2020) 40(3):205–24. doi: 10.1615/CritRevImmunol.2020034910
44. Liang W, Huang X, Carlos CJJ, Lu X. Research Progress of Tumor Microenvironment and Tumor-Associated Macrophages. Clin Trans Oncol (2020) 22(12):2141–52. doi: 10.1007/s12094-020-02367-x
45. Zhou J, Tang Z, Gao S, Li C, Feng Y, Zhou X. Tumor-Associated Macrophages: Recent Insights and Therapies. Front Oncol (2020) 10:188. doi: 10.3389/fonc.2020.00188
46. Roumenina LT, Daugan MV, Noé R, Petitprez F, Vano YA, Sanchez-Salas R, et al. Tumor Cells Hijack Macrophage-Produced Complement C1q to Promote Tumor Growth. Cancer Immunol Res (2019) 7(7):1091–105. doi: 10.1158/2326-6066.cir-18-0891
47. Gordon SR, Maute RL, Dulken BW, Hutter G, George BM, McCracken MN, et al. PD-1 Expression by Tumour-Associated Macrophages Inhibits Phagocytosis and Tumour Immunity. Nature (2017) 545(7655):495–9. doi: 10.1038/nature22396
48. Petty AJ, Yang Y. Tumor-Associated Macrophages: Implications in Cancer Immunotherapy. Immunotherapy (2017) 9(3):289–302. doi: 10.2217/imt-2016-0135
49. Obeid E, Nanda R, Fu YX, Olopade OI. The Role of Tumor-Associated Macrophages in Breast Cancer Progression (Review). Int J Oncol (2013) 43(1):5–12. doi: 10.3892/ijo.2013.1938
50. Mantovani A, Marchesi F, Malesci A, Laghi L, Allavena P. Tumour-Associated Macrophages as Treatment Targets in Oncology. Nat Rev Clin Oncol (2017) 14(7):399–416. doi: 10.1038/nrclinonc.2016.217
51. Kuroda H, Jamiyan T, Yamaguchi R, Kakumoto A, Abe A, Harada O, et al. Tumor Microenvironment in Triple-Negative Breast Cancer: The Correlation of Tumor-Associated Macrophages and Tumor-Infiltrating Lymphocytes. Clin Trans Oncol (2021) 23(12):2513–25. doi: 10.1007/s12094-021-02652-3
52. Fujiwara T, Healey J, Ogura K, Yoshida A, Kondo H, Hata T, et al. Role of Tumor-Associated Macrophages in Sarcomas. Cancers (2021) 13(5):1086. doi: 10.3390/cancers13051086
53. Huang Q, Liang X, Ren T, Huang Y, Zhang H, Yu Y, et al. The Role of Tumor-Associated Macrophages in Osteosarcoma Progression - Therapeutic Implications. Cell Oncol (Dordrecht) (2021) 44(3):525–39. doi: 10.1007/s13402-021-00598-w
54. Lecoultre M, Dutoit V, Walker PR. Phagocytic Function of Tumor-Associated Macrophages as a Key Determinant of Tumor Progression Control: A Review. J Immunother Cancer (2020) 8(2):e001408. doi: 10.1136/jitc-2020-001408
55. Yamaguchi K, Tsuchihashi K, Tsuji K, Kito Y, Tanoue K, Ohmura H, et al. Prominent PD-L1-Positive M2 Macrophage Infiltration in Gastric Cancer With Hyper-Progression After Anti-PD-1 Therapy: A Case Report. Medicine (2021) 100(19):e25773. doi: 10.1097/MD.0000000000025773
56. Santoni M, Romagnoli E, Saladino T, Foghini L, Guarino S, Capponi M, et al. Triple Negative Breast Cancer: Key Role of Tumor-Associated Macrophages in Regulating the Activity of Anti-PD-1/PD-L1 Agents. Biochim Biophys Acta Rev Cancer (2018) 1869(1):78–84. doi: 10.1016/j.bbcan.2017.10.007
57. Fujimura T, Kambayashi Y, Fujisawa Y, Hidaka T, Aiba S. Tumor-Associated Macrophages: Therapeutic Targets for Skin Cancer. Front Oncol (2018) 8:3. doi: 10.3389/fonc.2018.00003
58. Fujimura T, Kakizaki A, Furudate S, Kambayashi Y, Aiba S. Tumor-Associated Macrophages in Skin: How to Treat Their Heterogeneity and Plasticity. J Dermatol Sci (2016) 83(3):167–73. doi: 10.1016/j.jdermsci.2016.05.015
59. Tian Y, Li R, Liu Y, Li M, Song Y, Zheng Y, et al. The Risk of Immune-Related Thyroid Dysfunction Induced by PD-1/PD-L1 Inhibitors in Cancer Patients: An Updated Systematic Review and Meta-Analysis. Front Oncol (2021) 11:667650. doi: 10.3389/fonc.2021.667650
60. Que Y, Wang J, Zhu J, Li N, Huang J, Lu S, et al. Combination Therapy With Anti-PD-1 or PD-1 Antibody Alone in Asian Pediatric Patients With Relapsed or Refractory Cancer. Front Immunol (2021) 12:647733:647733. doi: 10.3389/fimmu.2021.647733
61. Cai H, Zhang Y, Wang J, Gu J. Defects in Macrophage Reprogramming in Cancer Therapy: The Negative Impact of PD-L1/PD-1. Front Immunol (2021) 12:690869. doi: 10.3389/fimmu.2021.690869
62. Wang Q, Bardhan K, Boussiotis VA, Patsoukis N. The PD-1 Interactome. Adv Biol (2021) 25:e2100758. doi: 10.1002/adbi.202100758
63. Pauken KE, Torchia JA, Chaudhri A, Sharpe AH, Freeman GJ. Emerging Concepts in PD-1 Checkpoint Biology. Semin Immunol (2021) 15:101480. doi: 10.1016/j.smim.2021.101480
64. Zhao Y, Liu L, Weng L. Comparisons of Underlying Mechanisms, Clinical Efficacy and Safety Between Anti-PD-1 and Anti-PD-L1 Immunotherapy: The State-Of-the-Art Review and Future Perspectives. Front Pharmacol (2021) 12:714483. doi: 10.3389/fphar.2021.714483
65. Wojtukiewicz MZ, Rek MM, Karpowicz K, Gorska M, Politynska B, Wojtukiewicz AM, et al. Inhibitors of Immune Checkpoints-PD-1, PD-L1, CTLA-4-New Opportunities for Cancer Patients and a New Challenge for Internists and General Practitioners. Cancer Metastasis Rev (2021) 40(3):949–82. doi: 10.1007/s10555-021-09976-0
66. Borst J, Busselaar J, Bosma DMT, Ossendorp F. Mechanism of Action of PD-1 Receptor/Ligand Targeted Cancer Immunotherapy. Eur J Immunol (2021) 51(8):1911–20. doi: 10.1002/eji.202048994
67. Gonzalez-Ericsson PI, Wulfkhule JD, Gallagher RI, Sun X, Axelrod ML, Sheng Q, et al. Tumor-Specific Major Histocompatibility-II Expression Predicts Benefit to Anti-PD-1/L1 Therapy in Patients With HER2-Negative Primary Breast Cancer. Clin Cancer Res (2021) 27(19):5299–306. doi: 10.1158/1078-0432.CCR-21-0607
68. Brautigam K, Kabore-Wolff E, Hussain AF, Polack S, Rody A, Hanker L, et al. Inhibitors of PD-1/PD-L1 and ERK1/2 Impede the Proliferation of Receptor Positive and Triple-Negative Breast Cancer Cell Lines. J Cancer Res Clin Oncol (2021) 147(10):2923–33. doi: 10.1007/s00432-021-03694-4
69. Qiu Y, Yang Y, Yang R, Liu C, Hsu JM, Jiang Z, et al. Activated T Cell-Derived Exosomal PD-1 Attenuates PD-L1-Induced Immune Dysfunction in Triple-Negative Breast Cancer. Oncogene (2021) 40(31):4992–5001. doi: 10.1038/s41388-021-01896-1
70. Bottai G, Raschioni C, Losurdo A, Di Tommaso L, Tinterri C, Torrisi R, et al. An Immune Stratification Reveals a Subset of PD-1/LAG-3 Double-Positive Triple-Negative Breast Cancers. Breast Cancer Res: BCR (2016) 18(1):121. doi: 10.1186/s13058-016-0783-4
71. Wu S, Shi X, Wang J, Wang X, Liu Y, Luo Y, et al. Triple-Negative Breast Cancer: Intact Mismatch Repair and Partial Co-Expression of PD-L1 and LAG-3. Front Immunol (2021) 12:561793. doi: 10.3389/fimmu.2021.561793
72. Li C, Xu L. Single-Cell Transcriptome Analysis Reveals the M2 Macrophages and Exhausted T Cells and Intratumoral Heterogeneity in Triple-Negative Breast Cancer. Anti-cancer Agents Med Chem (2021) 22(2):294–312. doi: 10.2174/1871520621666210618100857
73. Ahmed FS, Gaule P, McGuire J, Patel K, Blenman K, Pusztai L, et al. PD-L1 Protein Expression on Both Tumor Cells and Macrophages are Associated With Response to Neoadjuvant Durvalumab With Chemotherapy in Triple-Negative Breast Cancer. Clin Cancer Res (2020) 26(20):5456–61. doi: 10.1158/1078-0432.ccr-20-1303
74. Parveen S, Siddharth S, Cheung LS, Kumar A, Shen J, Murphy JR, et al. Therapeutic Targeting With DABIL-4 Depletes Myeloid Suppressor Cells in 4T1 Triple-Negative Breast Cancer Model. Mol Oncol (2021) 15(5):1330–44. doi: 10.1002/1878-0261.12938
75. Zhang X, Zeng Y, Qu Q, Zhu J, Liu Z, Ning W, et al. PD-L1 Induced by IFN-γ From Tumor-Associated MacrophagesVia the JAK/STAT3 and PI3K/AKT Signaling Pathways Promoted Progression of Lung Cancer. Int J Clin Oncol (2017) 22(6):1026–33. doi: 10.1007/s10147-017-1161-7
76. Qiao J, Chen Y, Mi Y, Jin H, Wang L, Huang T, et al. Macrophages Confer Resistance to BET Inhibition in Triple-Negative Breast Cancer by Upregulating IKBKE. Biochem Pharmacol (2020) 180:114126. doi: 10.1016/j.bcp.2020.114126
77. Song W, Thakor P, Vesey DA, Gobe GC, Morais C. Conditioned Medium From Stimulated Macrophages Inhibits Growth But Induces an Inflammatory Phenotype in Breast Cancer Cells. Biomed Pharmacother = Biomed Pharmacother (2018) 106:247–54. doi: 10.1016/j.biopha.2018.06.126
78. Morrow RJ, Etemadi N, Yeo B, Ernst M. Challenging a Misnomer? The Role of Inflammatory Pathways in Inflammatory Breast Cancer. Mediators Inflamm (2017) 2017:4754827. doi: 10.1155/2017/4754827
79. Cannon MJ, Ghosh D, Gujja S. Signaling Circuits and Regulation of Immune Suppression by Ovarian Tumor-Associated Macrophages. Vaccines (2015) 3(2):448–66. doi: 10.3390/vaccines3020448
80. Deng XX, Jiao YN, Hao HF, Xue D, Bai CC, Han SY. Taraxacum Mongolicum Extract Inhibited Malignant Phenotype of Triple-Negative Breast Cancer Cells in Tumor-Associated Macrophages Microenvironment Through Suppressing IL-10/STAT3/PD-L1 Signaling Pathways. J Ethnopharmacol (2021) 274:113978. doi: 10.1016/j.jep.2021.113978
81. Dixon KO, Tabaka M, Schramm MA, Xiao S, Tang R, Dionne D, et al. TIM-3 Restrains Anti-Tumour Immunity by Regulating Inflammasome Activation. Nature (2021) 595(7865):101–6. doi: 10.1038/s41586-021-03626-9
82. Li S, Jiang L, Beckmann K, Højen JF, Pessara U, Powers NE, et al. A Novel Anti-Human IL-1R7 Antibody Reduces IL-18-Mediated Inflammatory Signaling. J Biol Chem (2021) 296:100630. doi: 10.1016/j.jbc.2021.100630
83. Kanai T, Uraushihara K, Totsuka T, Okazawa A, Hibi T, Oshima S, et al. Macrophage-Derived IL-18 Targeting for the Treatment of Crohn’s Disease. Curr Drug Targets Inflammation Allergy (2003) 2(2):131–6. doi: 10.2174/1568010033484250
84. Rabinovich GA, Conejo-García JR. Shaping the Immune Landscape in Cancer by Galectin-Driven Regulatory Pathways. J Mol Biol (2016) 428(16):3266–81. doi: 10.1016/j.jmb.2016.03.021
85. Lucas CL, Workman CJ, Beyaz S, LoCascio S, Zhao G, Vignali DA, et al. LAG-3, TGF-β, and Cell-Intrinsic PD-1 Inhibitory Pathways Contribute to CD8 But Not CD4 T-Cell Tolerance Induced by Allogeneic BMT With Anti-CD40L. Blood (2011) 117(20):5532–40. doi: 10.1182/blood-2010-11-318675
86. Koh J, Hur JY, Lee KY, Kim MS, Heo JY, Ku BM, et al. Regulatory (FoxP3(+)) T Cells and TGF-β Predict the Response to Anti-PD-1 Immunotherapy in Patients With non-Small Cell Lung Cancer. Sci Rep (2020) 10(1):18994. doi: 10.1038/s41598-020-76130-1
87. Hensler M, Kasikova L, Fiser K, Rakova J, Skapa P, Laco J, et al. M2-Like Macrophages Dictate Clinically Relevant Immunosuppression in Metastatic Ovarian Cancer. J Immunother Cancer (2020) 8(2):e000979. doi: 10.1136/jitc-2020-000979
88. Jiang LR, Zhang N, Chen ST, He J, Liu YH, Han YQ, et al. PD-1-Positive Tumor-Associated Macrophages Define Poor Clinical Outcomes in Patients With Muscle Invasive Bladder Cancer Through Potential CD68/PD-1 Complex Interactions. Front Oncol (2021) 11:679928. doi: 10.3389/fonc.2021.679928
89. Lu D, Ni Z, Liu X, Feng S, Dong X, Shi X, et al. Beyond T Cells: Understanding the Role of PD-1/PD-L1 in Tumor-Associated Macrophages. J Immunol Res (2019) 2019:1919082. doi: 10.1155/2019/1919082
90. Rebelo SP, Pinto C, Martins TR, Harrer N, Estrada MF, Loza-Alvarez P, et al. 3D-3-Culture: A Tool to Unveil Macrophage Plasticity in the Tumour Microenvironment. Biomaterials (2018) 163:185–97. doi: 10.1016/j.biomaterials.2018.02.030
91. Tedesco S, Bolego C, Toniolo A, Nassi A, Fadini GP, Locati M, et al. Phenotypic Activation and Pharmacological Outcomes of Spontaneously Differentiated Human Monocyte-Derived Macrophages. Immunobiology (2015) 220(5):545–54. doi: 10.1016/j.imbio.2014.12.008
92. Chen Y, Song Y, Du W, Gong L, Chang H, Zou Z. Tumor-Associated Macrophages: An Accomplice in Solid Tumor Progression. J Biomed Sci (2019) 26(1):78. doi: 10.1186/s12929-019-0568-z
93. Fu LQ, Du WL, Cai MH, Yao JY, Zhao YY, Mou XZ. The Roles of Tumor-Associated Macrophages in Tumor Angiogenesis and Metastasis. Cell Immunol (2020) 353:104119. doi: 10.1016/j.cellimm.2020.104119
94. Henze AT, Mazzone M. The Impact of Hypoxia on Tumor-Associated Macrophages. J Clin Invest (2016) 126(10):3672–9. doi: 10.1172/jci84427
95. Capece D, Fischietti M, Verzella D, Gaggiano A, Cicciarelli G, Tessitore A, et al. The Inflammatory Microenvironment in Hepatocellular Carcinoma: A Pivotal Role for Tumor-Associated Macrophages. BioMed Res Int (2013) 2013:187204. doi: 10.1155/2013/187204
96. Szebeni GJ, Vizler C, Kitajka K, Puskas LG. Inflammation and Cancer: Extra- and Intracellular Determinants of Tumor-Associated Macrophages as Tumor Promoters. Mediators Inflamm (2017) 2017:9294018. doi: 10.1155/2017/9294018
97. Evans R, Flores-Borja F, Nassiri S, Miranda E, Lawler K, Grigoriadis A, et al. Integrin-Mediated Macrophage Adhesion Promotes Lymphovascular Dissemination in Breast Cancer. Cell Rep (2019) 27(7):1967–1978.e4. doi: 10.1016/j.celrep.2019.04.076
98. Bao X, Shi R, Zhao T, Wang Y, Anastasov N, Rosemann M, et al. Integrated Analysis of Single-Cell RNA-Seq and Bulk RNA-Seq Unravels Tumour Heterogeneity Plus M2-Like Tumour-Associated Macrophage Infiltration and Aggressiveness in TNBC. Cancer Immunol Immunother: CII (2021) 70(1):189–202. doi: 10.1007/s00262-020-02669-7
99. Cheng Z, Wang L, Wu C, Huang L, Ruan Y, Xue W. Tumor-Derived Exosomes Induced M2 Macrophage Polarization and Promoted the Metastasis of Osteosarcoma Cells Through Tim-3. Arch Med Res (2021) 52(2):200–10. doi: 10.1016/j.arcmed.2020.10.018
100. Song W, Mazzieri R, Yang T, Gobe GC. Translational Significance for Tumor Metastasis of Tumor-Associated Macrophages and Epithelial-Mesenchymal Transition. Front Immunol (2017) 8:1106. doi: 10.3389/fimmu.2017.01106
101. Lin L, Luo X, Wang L, Xu F, He Y, Wang Q, et al. BML-111 Inhibits EMT, Migration and Metastasis of TAMs-Stimulated Triple-Negative Breast Cancer Cells via ILK Pathway. Int Immunopharmacol (2020) 85:106625. doi: 10.1016/j.intimp.2020.106625
102. Vakili-Ghartavol R, Mombeiny R, Salmaninejad A, Sorkhabadi SMR, Faridi-Majidi R, Jaafari MR, et al. Tumor-Associated Macrophages and Epithelial-Mesenchymal Transition in Cancer: Nanotechnology Comes Into View. J Cell Physiol (2018) 233(12):9223–36. doi: 10.1002/jcp.27027
103. Zeng X-Y, Xie H, Yuan J, Jiang X-Y, Yong J-H, Zeng D, et al. M2-Like Tumor-Associated Macrophages-Secreted EGF Promotes Epithelial Ovarian Cancer Metastasis via Activating EGFR-ERK Signaling and Suppressing lncRNA LIMT Expression. Cancer Biol Ther (2019) 20(7):956–66. doi: 10.1080/15384047.2018.1564567
104. Zhang J, Yao H, Song G, Liao X, Xian Y, Li W. Regulation of Epithelial-Mesenchymal Transition by Tumor-Associated Macrophages in Cancer. Am J Trans Res (2015) 7(10):1699–711. doi: 10.1038/1943-8141/AJTR0012104
105. Bottai G, Raschioni C, Székely B, Di Tommaso L, Szász AM, Losurdo A, et al. AXL-Associated Tumor Inflammation as a Poor Prognostic Signature in Chemotherapy-Treated Triple-Negative Breast Cancer Patients. NPJ Breast Cancer (2016) 2:16033. doi: 10.1038/npjbcancer.2016.33
106. Hollmén M, Karaman S, Schwager S, Lisibach A, Christiansen AJ, Maksimow M, et al. G-CSF Regulates Macrophage Phenotype and Associates With Poor Overall Survival in Human Triple-Negative Breast Cancer. Oncoimmunology (2016) 5(3):e1115177. doi: 10.1080/2162402x.2015.1115177
107. Chen M, Miao Y, Qian K, Zhou X, Guo L, Qiu Y, et al. Detachable Liposomes Combined Immunochemotherapy for Enhanced Triple-Negative Breast Cancer Treatment Through Reprogramming of Tumor-Associated Macrophages. Nano Lett (2021) 21(14):6031–41. doi: 10.1021/acs.nanolett.1c01210
108. Ye JH, Wang XH, Shi JJ, Yin X, Chen C, Chen Y, et al. Tumor-Associated Macrophages are Associated With Response to Neoadjuvant Chemotherapy and Poor Outcomes in Patients With Triple-Negative Breast Cancer. J Cancer (2021) 12(10):2886–92. doi: 10.7150/jca.47566
109. Niu M, Valdes S, Naguib YW, Hursting SD, Cui Z. Tumor-Associated Macrophage-Mediated Targeted Therapy of Triple-Negative Breast Cancer. Mol Pharmaceutics (2016) 13(6):1833–42. doi: 10.1021/acs.molpharmaceut.5b00987
110. Cheng N, Bai X, Shu Y, Ahmad O, Shen P. Targeting Tumor-Associated Macrophages as an Antitumor Strategy. Biochem Pharmacol (2021) 183:114354. doi: 10.1016/j.bcp.2020.114354
111. Gambardella V, Castillo J, Tarazona N, Gimeno-Valiente F, Martínez-Ciarpaglini C, Cabeza-Segura M, et al. The Role of Tumor-Associated Macrophages in Gastric Cancer Development and Their Potential as a Therapeutic Target. Cancer Treat Rev (2020) 86:102015. doi: 10.1016/j.ctrv.2020.102015
112. Yin S, Wang N, Riabov V, Mossel DM, Larionova I, Schledzewski K, et al. SI-CLP Inhibits the Growth of Mouse Mammary Adenocarcinoma by Preventing Recruitment of Tumor-Associated Macrophages. Int J Cancer (2020) 146(5):1396–408. doi: 10.1002/ijc.32685
113. Li F, Kitajima S, Kohno S, Yoshida A, Tange S, Sasaki S, et al. Retinoblastoma Inactivation Induces a Protumoral Microenvironment via Enhanced CCL2 Secretion. Cancer Res (2019) 79(15):3903–15. doi: 10.1158/0008-5472.can-18-3604
114. Tang X, Mo C, Wang Y, Wei D, Xiao H. Anti-Tumour Strategies Aiming to Target Tumour-Associated Macrophages. Immunology (2013) 138(2):93–104. doi: 10.1111/imm.12023
115. Frankenberger C, Rabe D, Bainer R, Sankarasharma D, Chada K, Krausz T, et al. Metastasis Suppressors Regulate the Tumor Microenvironment by Blocking Recruitment of Prometastatic Tumor-Associated Macrophages. Cancer Res (2015) 75(19):4063–73. doi: 10.1158/0008-5472.can-14-3394
116. Araujo JM, Gomez AC, Aguilar A, Salgado R, Balko JM, Bravo L, et al. Effect of CCL5 Expression in the Recruitment of Immune Cells in Triple Negative Breast Cancer. Sci Rep (2018) 8(1):4899. doi: 10.1038/s41598-018-23099-7
117. Bauer D, Redmon N, Mazzio E, Soliman KF. Apigenin Inhibits Tnfα/IL-1α-Induced CCL2 Release Through IKBK-Epsilon Signaling in MDA-MB-231 Human Breast Cancer Cells. PloS One (2017) 12(4):e0175558. doi: 10.1371/journal.pone.0175558
118. Rogers TL, Holen I. Tumour Macrophages as Potential Targets of Bisphosphonates. J Trans Med (2011) 9:177–7. doi: 10.1186/1479-5876-9-177
119. Liu Y, Zhao S, Chen W, Hu F, Zhu L, Zhang Q, et al. Bisphosphonate Use and the Risk of Breast Cancer: A Meta-Analysis of Published Literature. Clin Breast Cancer (2012) 12(4):276–81. doi: 10.1016/j.clbc.2012.04.003
120. Early Breast Cancer Trialists’ Collaborative G. Adjuvant Bisphosphonate Treatment in Early Breast Cancer: Meta-Analyses of Individual Patient Data From Randomised Trials. Lancet (2015) 386(10001):1353–61. doi: 10.1016/S0140-6736(15)60908-4
121. Chaturvedi P, Gilkes DM, Takano N, Semenza GL. Hypoxia-Inducible Factor-Dependent Signaling Between Triple-Negative Breast Cancer Cells and Mesenchymal Stem Cells Promotes Macrophage Recruitment. Proc Natl Acad Sci USA (2014) 111(20):E2120–9. doi: 10.1073/pnas.1406655111
122. Wang L, Simons DL, Lu X, Tu TY, Avalos C, Chang AY, et al. Breast Cancer Induces Systemic Immune Changes on Cytokine Signaling in Peripheral Blood Monocytes and Lymphocytes. EBioMedicine (2020) 52:102631. doi: 10.1016/j.ebiom.2020.102631
123. Liverani C, Mercatali L, Spadazzi C, La Manna F, De Vita A, Riva N, et al. CSF-1 Blockade Impairs Breast Cancer Osteoclastogenic Potential in Co-Culture Systems. Bone (2014) 66::214–22. doi: 10.1016/j.bone.2014.06.017
124. Liu Y, Tiruthani K, Wang M, Zhou X, Qiu N, Xiong Y, et al. Tumor-Targeted Gene Therapy With Lipid Nanoparticles Inhibits Tumor-Associated Adipocytes and Remodels the Immunosuppressive Tumor Microenvironment in Triple-Negative Breast Cancer. Nanoscale Horizons (2021) 6(4):319–29. doi: 10.1039/d0nh00588f
125. Hollmén M, Roudnicky F, Karaman S, Detmar M. Characterization of Macrophage–Cancer Cell Crosstalk in Estrogen Receptor Positive and Triple-Negative Breast Cancer. Sci Rep (2015) 5:9188. doi: 10.1038/srep09188
126. Rego SL, Helms RS, Dréau D. Tumor Necrosis Factor-Alpha-Converting Enzyme Activities and Tumor-Associated Macrophages in Breast Cancer. Immunol Res (2014) 58(1):87–100. doi: 10.1007/s12026-013-8434-7
127. Andón FT, Digifico E, Maeda A, Erreni M, Mantovani A, Alonso MJ, et al. Targeting Tumor Associated Macrophages: The New Challenge for Nanomedicine. Semin Immunol (2017) 34:103–13. doi: 10.1016/j.smim.2017.09.004
128. Binnemars-Postma K, Storm G, Prakash J. Nanomedicine Strategies to Target Tumor-Associated Macrophages. Int J Mol Sci (2017) 18(5):979. doi: 10.3390/ijms18050979
129. Zhao Y, Zhang C, Gao L, Yu X, Lai J, Lu D, et al. Chemotherapy-Induced Macrophage Infiltration Into Tumors Enhances Nanographene-Based Photodynamic Therapy. Cancer Res (2017) 77(21):6021–32. doi: 10.1158/0008-5472.CAN-17-1655
130. Oner G, Altintas S, Canturk Z, Tjalma W, Verhoeven Y, Van Berckelaer C, et al. Triple-Negative Breast Cancer-Role of Immunology: A Systemic Review. Breast J (2020) 26(5):995–9. doi: 10.1111/tbj.13696
Keywords: TNBC, TAMs, ER, PR, HER-2
Citation: Qiu X, Zhao T, Luo R, Qiu R and Li Z (2022) Tumor-Associated Macrophages: Key Players in Triple-Negative Breast Cancer. Front. Oncol. 12:772615. doi: 10.3389/fonc.2022.772615
Received: 08 September 2021; Accepted: 24 January 2022;
Published: 14 February 2022.
Edited by:
Mariana Segovia, National Autonomous University of Mexico, MexicoReviewed by:
Pio Conti, University of Studies G. d’Annunzio Chieti and Pescara, ItalyClaudia Angélica Garay-Canales, National Autonomous University of Mexico, Mexico
Copyright © 2022 Qiu, Zhao, Luo, Qiu and Li. This is an open-access article distributed under the terms of the Creative Commons Attribution License (CC BY). The use, distribution or reproduction in other forums is permitted, provided the original author(s) and the copyright owner(s) are credited and that the original publication in this journal is cited, in accordance with accepted academic practice. No use, distribution or reproduction is permitted which does not comply with these terms.
*Correspondence: Zhaoming Li, emhhb21pbmdsaTIwMTNAMTYzLmNvbQ==; Ran Qiu, MzA4NDQ1OTU5MEBxcS5jb20=
†These authors have contributed equally to this work