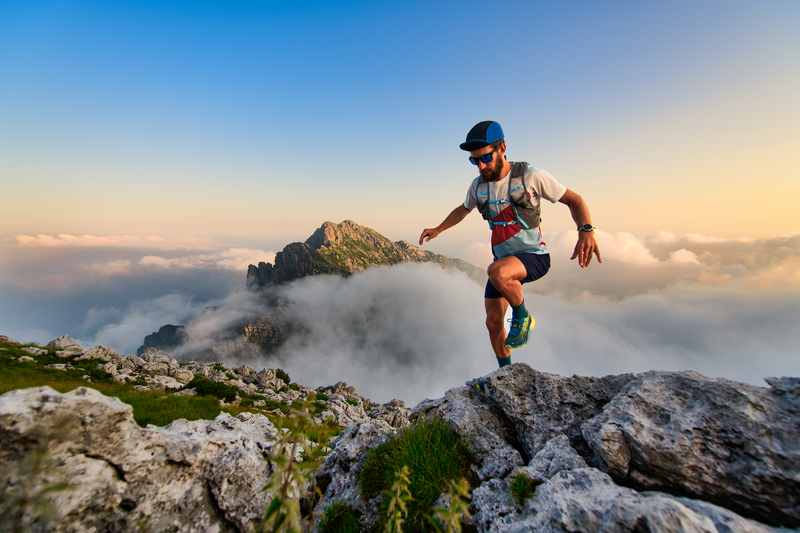
95% of researchers rate our articles as excellent or good
Learn more about the work of our research integrity team to safeguard the quality of each article we publish.
Find out more
REVIEW article
Front. Oncol. , 23 January 2023
Sec. Molecular and Cellular Oncology
Volume 12 - 2022 | https://doi.org/10.3389/fonc.2022.1103446
This article is part of the Research Topic Extracellular Vesicles in Gastrointestinal Cancers View all 5 articles
Extracellular vesicles are fundamentally significant in the communication between cells. Outer Membrane Vesicles(OMVs) are a special kind of EVs produced by Gram-negative bacteria, which are minute exosome-like particles budding from the outer membrane, which have been found to play essential roles in diverse bacterial life events, including regulation of microbial interactions, pathogenesis promotion, stress responses and biofilm formation. Recently, and more researches have explored the substantial potentials of EVs as natural functional nanoparticles in the bioengineering applications in infectious diseases, cardiovascular diseases, autoimmune diseases and neurological diseases, such as antibacterial therapy, cancer drugs and immunoadjuvants, with several candidates in clinical trials showing promising efficacy. However, due to the poor understanding of sources, membrane structures and biogenesis mechanisms of EVs, progress in clinical applications still remains timid. In this review, we summarize the latest findings of EVs, especially in gastrointestinal tract tumours, to provide a comprehensive introduction of EVs in tumorigenesis and therapeutics.
During bacterial growth, extracellular vesicles(EVs)are released from the cell surface after vesiculation of the outer membrane(OM) (1–6). Bacterial EVs were first discovered in Escherichia coli. Since then, numerous Gram-negative bacteria have been found to produce and secrete EVs, such as Pseudomonas aeruginosa, Salmonella typhimurium and Helicobacter pylori. Additionally, Gram-positive bacteria such as Bacillus anthracis, Bacillus subtilis and Staphylococcus aureus can also produce EVs (7) (Table 1 and Figure 1). EVs are spherical particles with an average diameter of 10–300 nm that are formed by budding vesicles originating from the OM that bulges outward and enwraps the surrounding cytoplasm, proteins, DNA and RNA (8). The EV proteome contains proteins not found in parent cells. A multifunctional vaccine platform based on bacterial EVs has received incremental attention in recent years (9). Immune responses can be triggered both innately and adaptively by EVs, which are intrinsically immunostimulatory (10). Moreover, their nanoscale size facilitates their processing by immune cells. EVs can be modified in several ways, such as via genetic manipulation, vector charging and nanoparticle wrapping, to create multifunction platforms that can be used to treat diverse diseases (11). EVs have emerged as useful components for formulating new vaccines. Additionally, EVs can retain and distribute nutrients, virulence factors and toxins while also using host-produced antimicrobial molecules (12). These natural nanostructures have many useful biological functions for immune-linked inhibition (13).
Figure 1 Bacteria are distributed in the whole digestive tract, and different bacteria tend to reside in specific organs.
Pathogenic and non-pathogenic Gram-negative and -positive bacteria release nanosized granules of 20–300 nm in diameter from their OM into the extracellular environment (14). Bacterial EVs play a major role in a wide range of biological processes, including virulence, aclinic gene output, cellular metabolism, bacteriophage infection and cell-to-cell interactions (15). EVs are usually generated via controlled blistering of bacteria. Given the core components and capabilities of EVs, they can be used to treat gastrointestinal neoplasms (16).
Abundant OM proteins(OMPs; i.e., OmpA, OmpC and OmpF), membrane interstitial proteins(AcrA and alkaline phosphatase), misfolded proteins and virulence factors associated with adhesion and invasion of host organs are found in EVs (17) (Figure 2). More than 3500 proteins belonging to various functional classes have been characterised using MS-based proteomic approaches after purification of EVs via ultracentrifugation. According to a global proteomic analysis, EVs lack components of the inner cell membrane and cytoplasm, implying that vesicle formation is a directed process instead of a random process (18). EVs are formed via blistering and swelling and mostly consist of interstitial proteins and OM protein envelope components. Over the past few years, high-throughput proteomic data of EVs collected from pathogens and commensal bacteria have revealed new functional proteins that can be used for biomedical applications (19). Therefore, the integration of proteomic data under various physiological conditions may facilitate the development of EV-based biomimetic materials (20).
Figure 2 Different functional constituents existed in bacteria derived OMVs, e.g., proteins, nucleic acids, lipids, virulence factors and PAMPs.
Bacterial EVs carry lumen- and surface-associated DNA, RNA, purified plasmids, bacteriophage DNA and chromosomal DNA(Figure 2). A study revealed the presence of DNA in EVs by treating bacterial samples with DNase (21). Resistance to luminal DNA was maintained even after finishing the course of treatment (22). Additionally, a few different forms of Escherichia coli(E. coli), Neisseria gonorrhoeae(N. gonorrhoeae), Pseudomonas aeruginosa(P. aeruginosa)and Haemophilus influenzae(H. influenzae)have been reported (22). Genomic DNA has been found in EVs released by the commensal bacterium Enterobacter cloacae (18). Over the past few years, small noncoding RNAs(sRNAs)have been discovered in bacterial EVs (23). These sRNAs have a length of 50–400 nucleotides (24). For instance, Ghosal et al. reported that sRNAs in E. coli MG1655-derived EVs had a length of 15–40 nucleotides and performed numerous functions (25, 26). To escape degradation by RNases and perform biological functions in the related host, bacteria may carry functional sRNAs in EVs. Although functional sRNAs native to bacterial EVs have been identified in various studies via high-throughput RNA sequencing (26), their function remains unclear.
Lipids are one of the key components of the structure of bacterial EVs. Based on the enriched and excluded EV components, some lipid components may be important for EV biogenesis. In Gram-negative bacteria, lipopolysaccharides(LPSs)and phospholipids(PLs)are the most common lipid components (27) (Figure 2). PLs constitute the inner layer of the OM, whereas LPSs are found only on the outer surface. The content of PLs varies among Gram-negative bacteria. Phosphatidylethanolamine(PE), phosphatidylglycerol(PG)and lysophosphate(LY)are the main PLs in E. coli EVs, whereas PG, PE and stearic acid are the major lipid components in other bacterial EVs (28). The three major classes of OM lipids in the myxobacterium Sorangium cellulosum include sphingolipids(SLs), which have long-chain amino alcohol backbone; glyceryl ether lipids(GELs)and bird-containing lipids amino acid lipid (OLs). In group A Streptococcus (GAS) -derived EVs, more than 85 glycerolipids (GLs)are present, including anionic and cationic PLs (29). In Hp-derived EVs, cardiolipin(CL)is a major lipid component. In Gram-negative bacteria, the OM contains both neutral (O-LPS)and negatively charged (A) -LPSO antigenic residues. EVs derived from Gram-negative bacteria contain only A-LPS (17). Gingivalase, a protein highly abundant in P. gingivalis, is eliminated in the A-LPS mutant strain, which highlights the importance of EV packaging. A recent study demonstrated that delivery of P. gingivalis SL via EVs can alter host inflammatory responses. Because EVs derived from Singomonas paucimobilis contain SL, a lipid class that plays an important structural and signalling role in eukaryotes, this bacterium may be used to build a system in which host–bacteria interactions are exploited.
EVs can directly communicate between bacteria and the host by delivering toxins and enhancing bacterial virulence in host cells to benefit pathogenic bacteria (30) (Figure 2). As an example, EstA, a bacterial virulence factor derived from the EVs of P. aeruginosa, induces nitric oxide and pro-inflammatory cytokines during macrophage interaction with host cells (18). As a result of these virulence factors, host cells are dysregulated by proteins released by EVs, thereby affecting proteolysis, ion transport and ion binding (18). EVs derived from H. pylori exhibit Lewis antigens on their surface and can activate the immune system of the host. As a result, EVs directly bind to anti-Lewis antibodies in serum, thereby decreasing the self-defence ability of host cells (31). EVs play an important role in a wide range of biomedical applications because they can deliver various toxins and activate the defence system of the host. Therefore, EVs can be used to develop nano-platforms.
LPS is the most potent and immunostimulatory component of EVs (32). EV-based vaccines with diametrically opposed views were developed by two scientists who discovered LPS. Owing to the risk of inducing systemic reactogenicity when administered to humans(which is why LPS was historically called an endotoxin), LPS poses safety concerns. However, the presence of LPS supports the ability of EVs to stimulate the immune system effectively. Developing EV-based vaccines requires maintaining the balance between reactogenic risk and the ability to stimulate an effective immune response (33).
The lipid A subunit of LPS directly interacts with toll-like receptor (TLR) 4 (32), and changes in its composition significantly affect its binding and recognition, resulting in decreased agonistic or antagonistic effects, dimerisation of TLR4/MD-2 and downstream signalling.
The basic lipid A structure in Gram-negative bacteria is phosphorylated at positions 1 and 40 by a glucosamine disaccharide and Acylated composition at positions 2 and 3 with R-3-hydroxymyristate at positions 20 and 30 (known as lipid IV A). Several enzymes decorate this structure at various positions, especially fatty acids of varying length and saturation, or alter it by adding (or removing)phosphate groups of glucosamine (34). Bacteria use changes in the basic structure of lipid A to respond to various shocks and modulate host–pathogen interactions, thereby evading innate immune detection (34, 35). In E. coli and Shigella, the late acyltransferases HtrB (36)(also known as LpxL) and MsbB (also known as LpxM)transfer lauroyl and myristoyl fatty acids to positions 30 and 20, respectively, resulting in the highest reactivity of lipid A (phosphorylated at positions 1 and 40, acyl chain length of 12–14 carbons and asymmetric distribution) (37). PagP (38) catalyses the addition of a seventh fatty acid chain to hexaacylated lipid A via 2-O-palmitoylation, whereas LpxR (39) or PagL (40) catalyses deacylation. EptA and ArnT add phosphatidylethanolamine or arabinose to 1 and 40 phosphate groups (41), respectively, whereas LpxE degrades one phosphate group. Hydroxylation of LpxO is another widely described example of an enzymatic reaction that targets different lipid A sites (42, 43). Additionally, different enzymes can act on the same site of lipid A molecules, catalysing the substitution of some acyl chains by other amino chains(LpxP can add palm oleyl chains to the same site as HtrB) (44). Depending on the substrate specificity, enzymes have different acyl chains(acyl chains of varying lengths of 2–4 carbons are attached depending on stress conditions or the metabolites present). Therefore, the various types of lipid A provide bacteria with different abilities to activate TLR4, and hence, EVs have different abilities to activate TLR4, with different structures having different agonistic and antagonistic abilities (45).
A majority of genes involved in lipid A biosynthesis are essential for bacterial membrane integrity. Therefore, to alter the acylation state of lipid A with minimal defects in bacterial growth, genes encoding late acyltransferases should be inactivated individually or in combination. For instance, HtrB or MsbB deletion results in the production of EVs with predominantly pentaacylated lipid A, with EVs carrying wild-type lipid A for each. However, these EVs significantly decrease the levels of proinflammatory cytokines. In Salmonella enterica and Francisella tularensis (33, 46, 47), dephosphorylation of lipid A has been examined preclinically by knocking down the enzyme encoded by lpxE or by overexpressing the deacylase encoded by lpxR, which resulted in the cleavage of two fatty acid chains or PagL, which is a 3-O-degradation enzyme. For the development of meningococcal vaccines based on EVs, the endotoxin-reducing activity of lipid A is also essential. The LPS content of EVs is reduced when a detergent-based extraction method(for example, Bexsero)is used. In addition, genetically detoxified LPS can be used to generate meningococcal strains, specifically by knocking out the L1 (48) and L2 (49) genes. These genes produce pentaacylated lipid A, which significantly attenuates endotoxin activity, both preclinically and clinically.
Other PAMPs have also been found in EVs. Stably transfected human embryonic kidney cells expressing only specific TLRs consistent with their native lipoprotein content have been shown to efficiently activate TLR2 via GMMA in Shigella (46), Salmonella (50) and Neisseria meningitidis. Additionally, targeted blockade of TLRs in human PBMCs, alone or in combination, elucidates the relative contribution of TLR2, TLR4 and TLR5 to the ability of Shigella and Salmonella GMMA with modified lipid A to induce pro-inflammatory responses (Figure 2). The contribution of TLR4 to pro-inflammatory responses is significantly reduced to a level where TLR2 activation mainly mediates the response, whereas the relative contribution of PRRs other than TLR2, TLR4 and TLR5 is negligible(<10%). Non-LPS PAMPs can enhance immune responses to vesicles (51) in LPS-deficient EVs in Neisseria. Flagellin and CpG DNA have been found in Salmonella GMMA and Pseudomonas aeruginosa-derived vesicles (52). However, whether they directly affect the response of the host to intact vesicles containing LPS remains unclear. Shigella GMMA preparations, possibly purified with GMMA, have low levels of cytoplasmic proteins(mainly ribosomal proteins) (53) (Figure 3).
Cellular components and nanoparticles can be delivered via EVs. These specific cargoes facilitate bacterial communication, immunomodulatory host–bacteria communication and cell detoxification.
EVs can interact with other microorganisms and hosts in the environment and have specialised communication systems under variable or challenging environmental conditions, including quorum sensing(QS), biofilm formation, nutrient acquisition, antibiotic resistance and competition with or defence against other microorganisms (54). Paracoccus denitrificans- and Vibrio harveii-derived EVs use quorum sensing under activated quinolone signalling(PQS)to interact with other bacteria and host cells, such as C16-HSL and CAI-1 (54). In bacterial communities, EVs share biofilm substrates to improve their ability to absorb nutrients and survive. Plankton- and biofilm-derived EVs in P. aeruginosa differ in quantity, quality, proteolytic activity and antibiotic-binding ability and participate in some activities of biofilms (55). Additionally, EVs derived from antibiotic-resistant strains have been shown to transfer antibiotic resistance genes and proteins to susceptible strains (9). For example, Acinetobacter baumannii-derived EVs contain carbapenem-resistance-related genes that can be horizontally transferred to carbapenem-resistant organisms (56). Furthermore, E. coli produces EVs that contain colistin, which degrades antimicrobial peptides such as melittins (57). The EVs of Moraxella catarrhalis and Staphylococcus aureus carry beta-lactamase, which allows them to escape antibiotics.
Horizontal gene transfer is a primary EV-mediated mechanism. However, only a few studies have examined the gene transfer ability of EVs. There are three steps in the process as follows: DNA is released from EV-encapsulated donor bacteria, EVs are incorporated with recipient bacteria and genetic material is transferred into the cytoplasm of the recipient bacteria. Several instances and related factors have been reported;however, this review does not address them in detail. Further investigation is required to understand the mechanisms underlying the loading of EVs and their attachment to recipient bacteria. In addition, novel mechanisms underlying EV-mediated bacterial communication should be investigated in species with varying lipid compositions (58) (Figure 4).
Figure 4 Three different mechanisms that mediating transferring of OMVs from bacteria to target cells.
Because EVs contain many immune-reactive proteins originating from their parent bacterium, they modulate innate immune responses in the host without direct host cell–bacteria interactions (59). In terms of structure and biological activity, bacteria-derived EVs are similar to those secreted by mammalian cells. EVs are required to alter the integrity of the epithelial barrier to maintain homeostasis in mammalian cells associated with inflammatory and metabolic diseases and induce immunomodulatory activity (60). Furthermore, the gut microbiome and intestinal epithelium interact to maintain barrier integrity. For example, EVs derived from probiotic E. coli and commensal ECOR63 can enhance barrier function (61).
In addition to interacting with host epithelial cells, in which EVs derived from both Gram-negative and -positive bacteria are involved, EVs directly affect immune cells, either by activating or suppressing immune responses. The gastric mucosa of patients with H. pyroli infection has been reported to contain EVs (62). S. typhimurium-derived EVs promote flagellin-mediated caspase-1 activation and IL-1β secretion in an endocytosis-dependent manner (59). EVs derived from H. pyroli, Neisseria, Pseudomonas, Campylobacter and Vibrio induce the secretion of interleukin-8(IL-8), a chemokine produced by tissues and blood cells, in non-phagocytic epithelial cells (63). EVs derived from Gram-positive bacteria such as Lactobacillus plantarum and L. sakei can protect against pathogen infection by stimulating host defence-related genes. The TLR8 and NF-κB signalling pathways in A. actinomycetemcomitans-derived EVs can activate the pro-inflammatory cytokine TNF-α (64).
EVs derived from many pathogenic bacteria contain toxins, degrading enzymes, virulence factors and pro-inflammatory compounds. These components can be used as tools to facilitate the contact of EVs with host cells. The Apx toxin (Shiga toxin)is activated by A. pleuropneumoniae-derived EVs and induces haemolysis and cytolysis. Salmonella typhimurium, however, possesses a special secretion system called the type III secretion system (T3SS)that secretes more than 40 virulence factors (65). EV-related virulence factors are not completely understood;however, it is evident that the survival of Salmonella is reduced without these factors. Although these toxins are found on the outer surface of vesicles, they can often resist proteases (65).
Gram-negative and -positive bacteria secrete EVs with particle sizes of 20–300 nm. EVs contain biologically active proteins, toxins, virulence factors and immunogenic materials that stimulate bacteria–host interactions, through which vaccines are developed (65). The non-replicative nature of EVs makes them relatively safe, and their biological roles are unpredictable under different environmental stresses. Therefore, in vivo biomedical applications of EVs can be more effective.
The human microbiome is an inherent element in the lifelong conservation of fitness and immune system homeostasis. It plays a fundamental role in tumorigenesis, cancer course and disease feedback. Approximately 85% of cases are related to PDAC, with only 24% of patients surviving 1 year after diagnosis, and the 5-year survival rate is approximately 5–7%.
Porphyromonas gingivalis and Aggregatibacter actinomycetemcomitans have been associated with an increased risk of PDAC (66). Porphyromonas gingivalis and Actinobacillus congeners were found to increase the risk of PDAC by 59% and 50%, respectively, in a nested case–control study that involved 361 patients with PDAC and 371 control patients and spanned over a decade (67). Periodontitis plays an important role in the pathogenesis of PC, and the two abovementioned oral microbes are causative agents (66, 68). Microbes from the mouth can migrate and translocate to the pancreas and other organs through the vascular network and/or the digestive tract. When located at a distance, disruption of the immune system and inflammation can accelerate cancer progression (69).
Porphyromonas gingivalis is a major pathogen associated with oral and gastrointestinal cancers (70). It exerts its toxicity through various virulence factors;however, recent studies have revealed a unique protein called PPAD. Gabarrini et al. reported that PPAD homologues are found in other Porphyromonas species, such as P. gulae and P. loveae (71, 72). Several studies have suggested that PPAD plays a role in the tumorigenesis of PC through KRAS mutation and P53 activation. Normally, the tumour suppressor gene P53 causes cell cycle arrest, thus allowing DNA repair in damaged cells (73). If the damage cannot be repaired, the cells become apoptotic targets. Approximately 50% of human cancers have mutations in p53 (74). The KRAS gene is an oncogene that usually has GTPase activity, and mutations in this gene interfere with its activity and may lead to inappropriate cell proliferation and transformation, which are associated with tumorigenesis and a poor prognosis. KRAS mutations are associated with several pathological conditions with an aggressive phenotype and a poor prognosis. PDAC is usually associated with KRAS mutations. Studies on Porphyromonas gingivalis and PPAD have reported that rheumatoid arthritis is associated with both periodontitis and citrullination (75). PPAD can catalyse citrullination, a post-translational modification.
Chronic inflammation and changes in the diversity and function of the gut microbiota are accompanied by changes in immune responses. A major risk factor for colorectal cancer (CRC)is chronic inflammation. TLRs play an important role in inflammatory responses. They can sense microbial constituents, including nucleic acids, LPSs, peptidoglycans and bacterial EVs. EVs can carry or be decorated with these TLR activators. These microbial factors can boost tolerance or activate signalling pathways that result in chronic inflammation. In a survey, we discussed the role of the gut microbiome and EVs derived from gut bacteria in promoting the development of chronic inflammation and colitis-associated CRC. In addition, we discussed the role of TLRs (Figure 3) in mediating the microbiome inflammatory axis and subsequent tumour development.
Acetate, propionate and butyrate are short-chain fatty acids (SCFAs)produced by intestinal bacteria (76). In the human colon, butyrate is mainly synthesised by glycolysis from hydrocarbons in Firmicutes spp., Ruminococcus spp. and sea squirrel families. The inhibitory effects of butyrate on cancer cell proliferation and cell death are attributed to its role in inhibiting histone deacetylase(HDAC) (77). Normal colon cells generate energy by oxidising butyrate through tricarboxylic acid oxidation (TCA cycle)or cytosolic acyl-CoA oxidation. Wahlberg et al. reported that cancer cells can alter their metabolic patterns even when oxygen is present and prefer the glycolytic pathway instead of the oxidative phosphorylation pathway(OXPHOS)to convert glucose into lactate. Glycolytic metabolism can be altered in cancer cells (78). Owing to the Warburg effect, colon cancer cells prefer glucose to butyrate for energy. Consequently, colon cancer cells accumulate high levels of butyrate, which inhibits HDAC (79). The levels of short-chain acyl-CoA dehydrogenase(SCAD), the primary enzyme catalysing mitochondrial butyrate oxidation, are decreased when butyrate directly enters the nucleus and inhibits histone deacetylase 1. As a result, autooxidation of butyrate in cancer cells is reduced, and butyrate accumulates in cancer cells, thereby inhibiting the development of cancer. Therefore, tumour cells are more sensitive to the inhibitors of HDAC than nontransformed ones (80).
Overexpression of microRNA-92a (miR-92a)promotes tumour growth and invasion in CRC by targeting KLF4 and downstream p21, whereas downregulation of miR-92a can result in the apoptosis of CRC cells (81). miR-92a can inhibit phosphatase and tensin homologue (PTEN) (82), which is an anti-oncogene found on chromosome 10q23 and is the most important negative regulator of PI3K signalling. Extracellular stimuli can activate PI3K, which converts phosphatidylinositol-4, 5-bisphosphate (PIP2) to phosphatidylinositol-3, 4, 5-trisphosphate (PIP3), which phosphorylates and activates the Akt pathway (83). To block PI3K signalling, PTEN dephosphorylates PIP3 and forms PIP2, thus blocking the PIP3K signalling cascade (84). Inhibition of miR-92a by butyrate can stimulate apoptosis and prevent the proliferation of colon cancer cells. Butyrate can also reduce the phosphorylation of Akt1 and ERK1/2 by blocking HDAC3 activity, thus inhibiting any subsequent cell movement and preventing the metastasis and invasion of CRC (85).
When miR-92a is overexpressed in CRCs, it targets KLF4 and downstream p21, promoting tumour growth and invasion. Similarly, reduced miR-92a expression can induce apoptosis in CRC. In response to extracellular stimuli (including insulin, growth factors and chemokines), PIP2 is converted to PIP3 by activated PI3K and Akt (protein kinase B)is phosphorylated and activated. Dose-dependent downregulation of miR-92a expression by butyrate via c-Myc may reduce the proliferation of colon cancer cells and stimulate apoptosis. In addition, butyrate inhibits the movement of cells by blocking the activity of HDAC3 and inhibiting the phosphorylation of Akt1 and ERK1/2, which eventually prevents tumour metastasis and invasion.
Tumour size and pathological stage (pTNM)are associated with decreased expression of miR-203 in CRC tissues and cells (86). Epithelial cells undergo epithelial–mesenchymal transition (EMT)during the early stages of cancer. During EMT, the loss of E-cadherin, an important component of adhesion, disrupts cell-to-cell contact. E-cadherin is degraded by Hakai, an E3 ubiquitin ligase that regulates cell adhesion by binding and degrading it. Based on the differentiated TNM staging(I–IV), Hakai expression is upregulated in colorectal adenocarcinoma and adenoma. Overexpression of Hakai in epithelial cells induces cellular transformation and mesenchymal and invasive phenotypes, whereas inhibition of E-cadherin promotes cell proliferation. miR-203 inhibits cell proliferation by directly targeting Hakai and reducing its levels. As part of the Crk-associated substrate (CAS)family, NEDD9 is upregulated in multiple cancer types and participates in the adhesion and migration of cancer cells and tumour invasion. In CRC, NEDD9 promotes EMT via the JNK pathway(c-Jun N-terminal kinase) (87). MiR-203 inhibits the proliferation and colonisation of CRC cells and tumour invasion and induces apoptosis by targeting NEDD9. Butyrate also upregulates miR-203, thereby inhibiting the proliferation and colonisation of CRC cells and tumour invasion and inducing apoptosis (88).
Sulfate-reducing bacteria (SRB)metabolise sulfate and other sulfur-containing compounds, including taurine, in food, releasing hydrogen sulfide (H2S) (89). H2S promotes the growth of CRC cells. H2S levels are higher in the stool of patients with CRC than in the stool of healthy individuals. Additionally, faecal H2S levels are significantly higher among patients with colon tumours who have received sigmoid surgery than among healthy individuals of similar ages, suggesting that patients with colon cancer have a reduced ability to detoxify H2S (90). H2S may be involved in the pathogenesis of inflammatory bowel disease (IBD)and CRC. Several studies have reported the role of H2S in CRC. At physiological concentrations, H2S may promote cancer through inflammation and genotoxicity in addition to inhibiting butyrate oxidation and promoting cell proliferation in vitro. In the colon wall, where epithelial cells are exposed to bacteria and toxins, disulfide bonds are disrupted by H2S, leading to pro-inflammatory effects. A few studies have shown that H2S can prevent inflammation by protecting and rebuilding damaged mucous layers. H2S is produced by novel nonsteroidal anti-inflammatory drugs(NSAIDs). In several cancer cells, compounds that release H2S exhibit potent anticancer effects by inhibiting proliferation and/or inducing apoptosis, including CRC (91). The underlying mechanisms remain unclear but may be related to the inhibition of nuclear factor-κB (NF-κB)signalling by H2S, with an increase in intracellular calcium concentration as the secondary result. Overall, the role of H2S in CRC is controversial and warrants further investigation.
As a key virulence factor found in Fusobacterium nucleatum(Fn), FadA regulates cell adhesion and tumour invasion (92). Comparing specimens from CRC patients with normal tissues, FadA gene expression was significantly increased. pre-FadA has 129 amino acid (AA)residues, which are not secreted, whereas the mature FadA has 111 AA residues (93). As a result of intrinsic FadA and mFadA pre-complexes, Fn can bind to and invade host epithelial cells, which is important for Fn adhesion and cell invasion and results in detachment of CDH5 from cells, thereby increasing endothelial permeability and allowing bacteria to pass through vogue junctions, that FadA binds to host endothelial receptors and vascular endothelial globulin (CDH5) (92). In addition to binding to E-cadherin in vitro, FadA can bind to HEK293 cells, which are not cancerous, and CRC cells other than RKO cells. The E-cadherin receptor participates in Fn adhesion and CRC invasion through FadA. FadAc binds to E-cadherin and inhibits its tumour suppressor activity by phosphorylating and internalising it. As a result, β-catenin regulates the transcription and gene expression of NF-κB and Wnt, promoting the proliferation of CRC cells (94).
Intestinal bacteria such as E. coli and other Gram-negative bacteria produce colistin and cytolethal distending toxins(CDTs), which directly damage DNA in the large intestine (95–97). Biosynthetic processes produce colibactin, a heterogeneous ketone compound and non-ribosomal peptide complex. Certain strains that produce myxomycetin are associated. Eukaryotic cells can become senescent when colibactin damages double-stranded DNA and affects chromosome stability. Bacteria such as E. coli can modify the tumour microenvironment by secreting growth factors (98), leading to cellular ageing. The CDT family of toxins is produced by a wide range of Gram-negative bacteria, including E. coli, Actinomycetes, Shigella and Helicobacter. CDT has three subunits, namely, CdtA, CdtB and CdtC. CdtB is similar to DNAase I in that it causes damage to host DNA (99). In addition to mediating the interaction of toxins with cytoplasmic membranes, the CdtA and CdtC subunits are essential for internalising CdtB, which is part of an essential active subunit. CDT can cause DNA damage, leading to cell cycle arrest and cellular senescence. The presence of CDT plays a critical role in reducing the carcinogenic effects of Campylobacter jejuni. CDTs produced by Campylobacter jejuni damage host cell DNA and promote colorectal tumorigenesis by triggering cell growth and enhancing the nuclear translocation of β-catenin (99).
EVs activate inflammatory responses by activating TLRs (100) (Figure 3). TLRs sense microbial components, such as nucleic acids, LPSs, peptidoglycans and EVs. These TLRs can be decorated on EVs or carried as transmitters by them. As a result of these microbial factors, tolerance may be promoted or signalling pathways leading to chronic inflammation may be activated (100).
Hepatitis A, B and C viral infections cause the majority of hepatitis-related deaths, with HBV infection being the most common (101). Although there is an approved vaccine for HBV infection, it remains unknown whether HCV can be prevented via vaccination (102). HCV infection can be effectively treated with direct-acting antiviral therapy;however, prevention remains the most important challenge (102). In a study, Lactococcus lactis was engineered to produce polyhydroxybutyrate inclusions displaying the core antigen of the hepatitis C virus. Mice that received antigen-presenting particles produced more IFN-c, IL-17A, tumour necrosis factor-alpha and IL-6 and fewer IgG2c antibodies. Administration of Emulsigen with the vaccine produced strong IgG1 antibodies and cytokine responses. Vaccines can be developed against HCV infection using a fusion protein that encodes a truncated form of the core antigen fused with the nonstructural protein 3 (103). Subcutaneous administration of the antigen in combination with purified N. meningitidis-derived EVs in mice can increase the production of IgG antibodies, pro-inflammatory cytokines and granzyme B, suggesting that the combination may be used for developing vaccines against HCV infection (103).
To interact with microbes and maintain a symbiotic relationship, the host requires a well-developed communication system with its symbionts and pathogens. Factors secreted by cells can be degraded by enzymes (e. g. proteases and nucleases). Prokaryotes and eukaryotes package cargoes (e. g. proteins, ribonucleotides and metabolites)in extracellular vesicles (EVs)to prevent extracellular degradation (104). A cell vesicle is a ubiquitous cellular carrier capable of transferring biomolecules and transmitting signals. Subcategories are effectively created when EVs have multiple functions, goods and compositions. The adaptive immune response is initiated by certain eukaryotic cells, such as B cells and dendritic cells, by presenting antigens through EVs. EVs are also used by other cell types, such as neurons, to improve action potentials and stimulate targets, and by adipocytes to process insulin in a paracrine manner. EVs can regulate cancer cell communication as well (105). The DNA, metabolites and proteins found in EVs secreted by normal cells can be used by tumour cells for’educating’non-cancerous cells to promote carcinogenesis through EVs. Using these vesicles, cancer cells can control the local environment and cellular processes, promoting inflammation and cancer development and evasion of the immune system.
In eukaryotes, EVs are enriched with certain classes of biomolecules, indicating the involvement of selective transport pathways. EVs can be used as novel biomarkers or therapeutic delivery systems for certain diseases and for the development of vaccines. EVs participate in various molecular pathways, including TLRs (106) (Table 2). LPS(TLR4), peptidoglycan (TLR2) and nucleic acids (TLR3/7/8/9)are a few biomolecules that interact with TLRs when attached to EVs. Many bacterial EVs bind to TLRs to promote disease or maintain homeostasis;however, the underlying mechanisms remain unclear. The IEC and E. coli C25 strains are symbiotic strains that interact via EVs. EV–IEC interactions upregulate TLR2/4/5 expression and promote IL-8 production, causing mild inflammation and preventing bacterial internalisation into the epithelium. The EVs of commensal E. coli cultures can induce the expression of TLR 1/5/6/7/8/9, IL-10, IL-12A and IL-1 in intestinal epithelial cells (107). Similarly, other commensal microbes interact with immune cells to affect gut homeostasis.
PSA binds to TLR2 on DCs, thus enabling B. fragilis (NTBF)to promote intestinal immunotolerance. In the same fashion, B. fragilis(NTBF)-derived EVs are enriched with PSA, which activates TLR2-mediated increase in IL-10 production in DCs and enhances Treg production to protect against experimental colitis. Bacteroides vulgatus, an intestinal symbiont, releases EVs that activate TLR2 and TLR4 on CD11c+ DCs to promote anti-inflammatory responses (108). It is also possible for the health status of the host to influence how responsive the host cells are to commensal EVs. Specifically, EVs derived from Bacteroides thetaiotaomicron(Bt)stimulate the expression of IL-10 and IL-6 in DCs derived from healthy individuals, which in cell cultures from IBD patients, but EVs were ineffective, suggesting that TLR expression or components of the TLR pathway are altered. Therefore, EVs derived from commensal strains help to regulate homeostasis in the intestine through immunomodulatory antigens (109). However, interactions between pathogen-derived EVs and host cells result in the integration of microbial antigens within host cells, affecting intracellular processes and exacerbating the disease state (110).
IBD and cancer are exacerbated by EVs derived from pathogens (111). Innate immune responses are initiated by epithelial cells during immunosurveillance. P. gingivalis-derived EVs attract strong TLR2 (peptidoglycan)and TLR4 (LPS)responses and activate TLR7/8/9 (nucleic acids) (112) to a minor extent in the epithelium. H. pylori-derived EVs transfer virulence factors (i. e. CagA)that activate the TLR and NF-kB pathways in gastric and B cells, thus increasing the release of pro-inflammatory cytokines and cell proliferation in gastric neoplasm. H. pylori is a well-documented oncogenic bacterium that used EVs to initiate carcinogenesis. Other intestinal microbes may similarly use EVs to influence carcinogenesis. RNA within EVs derived from Aggregatibacter actinomycetemcomitans enhances the pro-inflammatory effects of TNF through TLR8 and NFkB (113). The delivery of DNA and RNA via EVs derived from Staphylococcus aureus can lead to potent IFN-β responses in macrophages via endosomal TLRs. EVs derived from the microbiome of rats with colitis can interact with TLR4 on IECs (114), thus triggering proinflammatory responses from macrophages, especially the release of IL-8. EVs in patients with IBD can induce pro-inflammatory responses, enhance the destruction of the intestinal epithelium and promote macrophage polarisation to M1 and M2 types (113). Peptidoglycan present in EVs can be internalised by IECs via TLR2 and transported to the basic membrane (115). After the peptidoglycan is transferred, EVs communicate with macrophages and induce the secretion of IL-6, which exacerbates inflammation in CRC. Therefore, EVs are very similar to their eukaryotic counterparts and represent an important component of intercellular interactions between microbes and host cells by interacting with TLRs (116).
Cancer immunotherapy (Figure 5) has been performed using bacteria-derived vesicles. Owing to the abundance of PAMPs in bacteria, bacterial EVs are highly immunogenic and can attract and activate immune cells at tumour sites (117). However, intact bacteria pose safety concerns, and hence, bacterial ghosts whose intracellular contents have been removed are a safer alternative. Incorporation of adjuvants or antigens can enhance the efficacy and specificity of hollowed-out bacteria. Compared with their free-form counterparts, antigens incorporated into bacterial ghosts have demonstrated better retention, increased immune responses and improved tumour growth suppression after subcutaneous administration. When subcutaneously injected, EVs enable effective lymphatic drainage and enhance localisation to solid tumours through passive targeting. In addition to natural accumulation at tumour sites, EVs can be used therapeutically against multiple types of cancer because they can use local cancer cells as antigen sources in situ. For example, E. coli-derived EVs administered intravenously can eradicate CT26 and MC38 colorectal cancer, 4T1 metastatic breast cancer and B16BL6 metastatic melanoma (117).
Figure 5 OMV vaccines play important roles in immunotherapy against various gastrointestinal tumours.
Several lines of evidence support the potential capability of entrance of bacterial EVs into tumor microenvironment and modification of immune status. For example, Kim et al. showed that systematically administered bacterial outer membrane vesicles specifically target and accumulate in the tumor tissue, and subsequently induce the production of antitumor cytokines CXCL10 and interferon-γ. This antitumor effect is interferon-γ dependent, as interferon-γ-deficient mice could not induce such outer membrane vesicle-mediated immune response. This work showed remarkable capability of bacterial outer membrane vesicles to effectively induce long-term antitumor immune responses that can fully eradicate established tumors without notable adverse effects.
The combination of immunotherapy and EVs can synergistically enhance anti-tumour efficacy (Figure 6). Because EVs can localise to tumour sites, they can be encapsulated with chemotherapeutic drugs, including doxorubicin and paclitaxel (118, 119). Compared with the use of monotherapy, this combination therapy exhibited significantly greater inhibitory effects on tumour growth. In a study, EVs were coated with polymeric micelles containing tegafur, a fluorouracil prodrug derived from attenuated Salmonella that induces tumour cell apoptosis and sensitises cancer cells to cytotoxic T lymphocytes (120). Indocyanine green is another photosensitiser used in photoimmunotherapy. During laser ablation, a large amount of cancer antigens is released for immune priming after their accumulation in tumours (121). Through thrombosis and erythrocyte extravasation, Salmonella typhimurium-derived EVs sensitise tumours to photothermal therapy even in the absence of photothermal agents (59, 100, 122). To enhance the effectiveness of EVs, radiation therapy can also be used, which is considered a purely external method for destroying tumours. The efficacy of EVs can be enhanced using external tumour-destroying methods such as radiation therapy (123). This type of therapy has been used to eradicate B78 melanoma and NXS2 neuroblastoma in previous studies. Furthermore, loading of EVs onto micromotors is an innovative combination therapy, which causes considerable physical damage and destruction to tumours. Compared with non-propulsion micromotors, EV-loaded micromotors can effectively clear tumours at the primary site and improve the control of tumour growth distally in MC38, CT26 and B16-F10 tumour models (124).
Figure 6 Biological effects that OMVs conferred to tumor cells and key molecules mediating such effects.
The purpose of this section is primarily to explore how insulin can be added to chemotherapy to treat gastrointestinal cancers, analyse and compare the effects of EVs and other methods combining EVs on gastrointestinal tumours and examine the novelty of EVs in treating gastrointestinal tumours in clinical settings.
Chemotherapy is an important treatment method for malignant digestive tract tumours. During chemotherapy, insulin treatment significantly reduces the effects of gastrointestinal tumours, and the efficacy of chemotherapy does not plunge (125). Insulin enhances the ability of tissues to absorb glucose and promotes anabolism in the liver, muscle and adipose tissues (125). In addition to improving metabolism and stimulating the growth and proliferation of normal and tumour cells, insulin can promote RNA and DNA synthesis in cells and tissues and induce tumour cell proliferation (126).
When insulin concentrations are within a certain range, it has no toxic effects on the human body. Studies have shown that insulin (2. 0–15. 0 mU/mL)can enhance the cytotoxic effects of etoposide on oesophageal and pancreatic cancer cells (127). In a study, large, medium and small doses of insulin combined with 5-Fu were found to be significantly more effective than 5-Fu alone in suppressing tumour growth in nude mouse S180 sarcoma and H22 liver cancer xenograft models (127). However, the number of white blood cells did not significantly differ between mice that received combination therapy and mice that receive monotherapy (P > 0. 05). Additionally, no difference was observed between the two groups of mice in terms of the morphology of the pancreas, liver and kidney. Therefore, combination therapy with insulin with chemotherapeutic drugs not only enhances the therapeutic efficacy but also reduces the toxicity of chemotherapeutic drugs (127). Using the abovementioned animal experiment as a basis, several clinical experiments were conducted by the authors. Chemotherapeutic regimens, such as DLF, are commonly used and highly effective for digestive system tumours. A study evaluated the efficacy of chemotherapy in preventing and treating hypoglycaemia;however, no significant differences were observed in chemotherapeutic efficacy between the experimental and control groups. The insulin group had larger or smaller lesions than the control group, depending on the size of the lesions, and the combination of insulin and chemotherapy was ineffective in the treatment of malignant tumours (125–127). In addition, it has an attenuation effect that does not reduce chemotherapy efficacy.
The combination of photothermal therapy and EVs has been successfully used to treat tumours of the digestive system. EVs are effective for specific anticancer therapy because they can target tumours (128). In a study, EVs elicited a photoacoustic signal in tumours immediately after intravenous injection, which increased during 120 minutes, indicating tumour accumulation. Several proteins and LPSs are present on the membrane surface of EVs, which may cause systemic toxicity. Studies involving immunocompetent mice have been conducted to address safety concerns regarding EVs. EVs induce significantly stronger TLR responses than EV and EV (100, 128, 129), suggesting that the latter two types of EVs were derived from a low-endotoxic E. coli strain that is generally well tolerated in a different way (100, 128). According to the results of studies on the use of bioengineered EVs in mice with cancer, the EV platform appears less likely to induce chronic systemic toxicity. The fate of EVs or melanin, which can function as a self-antigen, is an important question. Researchers have found that other EVs are degraded in late endosomes or lysosomes, and NADPH-dependent oxidoreductases degrade intracellular melanin. Because the bacterial membrane covers melanin, the immune system may be unaware of its presence in intact EVs (100, 128). As part of our future research, we will examine whether repeated administration of modified EVs in small and large animals results in systemic toxicity or toxic effects on major organs. In addition, it is important to explore synergies between photothermal therapy mediated by melanin and the immune responses triggered by EVs. Clinical applications require an evaluation of the long-term stability of EVs (100, 128). Previous studies have reported that EVs show clear melanin signals in both the skin and liver adjacent to the tumour. Therefore, the safety implications of EVs in healthy organs surrounding the tumour should be investigated. Photothermal therapy, in which light is irradiated on the tumour, may not necessarily cause toxic effects (100, 128).
An example of biomimetic nanoparticles(NPs)includes NPs fabricated using natural cellular materials for use in biomedicine (100, 128). In addition to retaining the original biological functions of the cellular material, biomimetic NPs can retain the physicochemical properties of synthetic nanoparticles. Consequently, NPs coated with cell membrane-derived components have attracted substantial attention in the biomedical field. This NP-based platform has multiple biomedical applications, especially cancer therapy with EV-coated NPs (100, 128). Biomedical applications of EVs, such as in photothermally active bacterial infections, have shown great promise. A few studies have reported the incorporation of NPs in biomimetic designs. It is encouraging that researchers are exploring non-conventional approaches to coating NPs with cancer cell membranes for biomedical applications. Cancer cell membranes have several unique properties that make them effective, including unlimited replication potential, immune tolerance, resistance to cell death, long circulation time and homologous binding capacity.
During chemotherapy, the most common side effects seen in patients with malignant digestive tract tumours are bone marrow suppression, nausea and vomiting, and more than 60% of patients cannot tolerate or complete the chemotherapeutic regimen as planned because of the high incidence rate of these side effects (130). Therefore, one of the current research directions in tumour treatment is to find a drug that does not reduce the efficacy of chemotherapy but reduces its toxic effects. Compared with immunotherapy, EV-based therapy has fewer side effects and more precise targeting. Cancer cells can be effectively destroyed by deciphering their DNA or RNA sequences at the genetic level instead of killing them superficially and disrupting normal metabolic functions (131).
This review summarises the EVs of digestive tract microorganisms and their application in the treatment of digestive tract diseases. Several challenges limiting the use of EVs remain to be addressed: first, the safety and effectiveness of EVs should be improved;second, the structural integrity of EVs should be maintained; third, EVs should be homogeneous. To improve the safety and efficacy of EVs, genetic engineering can be used to modify them (132). The simultaneous expression of multiple antigens on EVs has a wide range of applications. The production of EVs can also be improved by engineering bacteria (122). Owing to the involvement of LPS in reducing the safety of EVs and inducing immune responses, it is extremely important to prepare EVs without LPS or with low levels of LPS. However, Pulido et al. immunised mice with A. baumannii-derived EVs in the presence or absence of LPS and found that EVs were 75% effective in mice without LPS, whereas the protection rate of EVs with LPS was up to 100%. Therefore, EVs containing LPS may have some protective properties. A gene knockout-based method was used by Lee et al. to prepare low-LPS Salmonella typhimurium-derived EVs (59, 100, 122). After these EVs were fused with OMPA, they substantially increased serum antibody levels in immunised mice. Sodium deoxycholate, a detergent, combined with EDTA is used to extract EVs with low LPS levels. Physical or chemical extraction of EVs may also selectively reduce LPS levels. EDTA- and proteinase K-treated EVs and intact EVs do not have significantly different effects on the expression of tumour-promoting factors, and responses to cancer may rely on the structural integrity of EVs. Nitrogen gas has been reported as a method for rapid preparation of EVs. EVs prepared using this method retain the integrity of bacterial membranes and are rich in membrane proteins. In addition to protecting mice against Pseudomonas aeruginosa infection, these EVs can improve their chances of survival. This nitrogen-based approach may become a novel method for vaccine preparation. EVs vary greatly among individuals. EVs derived from carbapenem-resistant Klebsiella pneumoniae(CRKP)can be modified to ensure homogeneity and improve immune efficacy (133). BN-EV-based vaccines with BSA NPs of controlled size can significantly increase the production of CRKP-specific antibody titres and improve the survival of mice (134).
Immunostimulatory properties of pathogen-associated molecular patterns are attractive for vaccine development;however, the potential risks associated with the introduction of bacterial toxins such as LPS into human patients should be neutralised. Toxic components in bacteria can be isolated more precisely from EVs by developing more efficient separation and purification techniques (99, 100, 135, 136). The development of novel genetic engineering approaches to reduce toxic factors is very promising, and these strategies may eventually lead to the production of EVs that are inherently safe and do not require complex handling. Additionally, repeated EV vaccinations may induce immune responses against the cellular carrier, which can decrease immunity to the intended target antigen (124). The density of the total antigen can be increased through various engineering approaches, and non-essential proteins that are particularly immunogenic can be removed or decreased in concentration. In the future, a multi-antigen drug should be rationally designed using EVs (100, 124). At present, clinically approved artificial serum preparations require the mixing of multiple antigens, which may simplify vaccine production.
Although there is significant room for improvement, great breakthroughs have been made in the development of EV-based platforms for the clinical management of microbial infections and gastrointestinal tumours (100, 124). Providing innovative solutions to address current challenges and consistent, researches on EVs will enable their widespread adoption primarily for clinical purposes.
All authors listed have made a substantial, direct, and intellectual contribution to the work and approved it for publication.
National Key R&D Program of China (2019YFC1315900, 2019YFC1315802), and National Natural Science Foundation of China (82072760, 81902990).
The authors declare that the research was conducted in the absence of any commercial or financial relationships that could be construed as a potential conflict of interest.
All claims expressed in this article are solely those of the authors and do not necessarily represent those of their affiliated organizations, or those of the publisher, the editors and the reviewers. Any product that may be evaluated in this article, or claim that may be made by its manufacturer, is not guaranteed or endorsed by the publisher.
1. Knox KW, Vesk M, Work E. Relation between excreted lipopolysaccharide complexes and surface structures of a lysine-limited culture of escherichia coli. J Bacteriol (1966) 92:1206–17. doi: 10.1128/jb.92.4.1206-1217.1966
2. Johnstone RM, Adam M, Hammond JR, Orr L, Turbide C. Vesicle formation during reticulocyte maturation. association of plasma membrane activities with released vesicles (exosomes). J Biol Chem (1987) 262:9412–20. doi: 10.1016/S0021-9258(18)48095-7
3. Rivera J, Cordero RJ, Nakouzi AS, Frases S, Nicola A, Casadevall A, et al. Bacillus anthracis produces membrane-derived vesicles containing biologically active toxins. Proc Natl Acad Sci U.S.A. (2010) 107:19002–7. doi: 10.1073/pnas.1008843107
4. Valadi H, Ekstrom K, Bossios A, Sjostrand M, Lee JJ, Lotvall JO, et al. Exosome-mediated transfer of mRNAs and microRNAs is a novel mechanism of genetic exchange between cells. Nat Cell Biol (2007) 9:654–9. doi: 10.1038/ncb1596
5. Garcia-del Portillo F, Stein MA, Finlay BB. Release of lipopolysaccharide from intracellular compartments containing salmonella typhimurium to vesicles of the host epithelial cell. Infect Immun (1997) 65:24–34. doi: 10.1128/iai.65.1.24-34.1997
6. Cai W, Kesavan DK, Wan J, Abdelaziz MH, Su Z, Xu H, et al. Bacterial outer membrane vesicles, a potential vaccine candidate in interactions with host cells based. Diagn Pathol (2018) 13:95. doi: 10.1186/s13000-018-0768-y
7. Zhou L, Srisatjaluk R, Justus DE, Doyle RJ. On the origin of membrane vesicles in gram-negative bacteria. FEMS Microbiol Lett (1998) 163:223–8. doi: 10.1111/j.1574-6968.1998.tb13049.x
8. Roier S, Zingl FG, Cakar F, Durakovic S, Kohl P, Eichmann TO, et al. A novel mechanism for the biogenesis of outer membrane vesicles in gram-negative bacteria. Nat Commun (2016) 7:10515. doi: 10.1038/ncomms10515
9. Schwechheimer C, Kuehn MJ. Outer-membrane vesicles from gram-negative bacteria: biogenesis and functions. Nat Rev Microbiol (2015) 13:605–19. doi: 10.1038/nrmicro3525
10. Beveridge TJ. Structures of gram-negative cell walls and their derived membrane vesicles. J Bacteriol (1999) 181:4725–33. doi: 10.1128/jb.181.16.4725-4733.1999
11. Tan K, Li R, Huang X, Liu Q. Outer membrane vesicles: Current status and future direction of these novel vaccine adjuvants. Front Microbiol (2018) 9:783. doi: 10.3389/fmicb.2018.00783
12. Belshe R, Lee MS, Walker RE, Stoddard J, Mendelman PM. Safety, immunogenicity and efficacy of intranasal, live attenuated influenza vaccine. Expert Rev Vaccines (2004) 3:643–54. doi: 10.1586/14760584.3.6.643
13. Dorward DW, Garon CF. DNA Is packaged within membrane-derived vesicles of gram-negative but not gram-positive bacteria. Appl Environ Microbiol (1990) 56:1960–2. doi: 10.1128/aem.56.6.1960-1962.1990
14. Wang M, Xin Y, Cao H, Li W, Hua Y, Webster TJ, et al. Recent advances in mesenchymal stem cell membrane-coated nanoparticles for enhanced drug delivery. Biomater Sci (2021) 9:1088–103. doi: 10.1039/d0bm01164a
15. Jiménez-Jiménez C, Manzano M, Vallet-Regí M. Nanoparticles coated with cell membranes for biomedical applications. Biol (Basel) (2020) 9:406. doi: 10.3390/biology9110406
16. McBroom AJ, Johnson AP, Vemulapalli S, Kuehn MJ. Outer membrane vesicle production by escherichia coli is independent of membrane instability. J Bacteriol (2006) 188:5385–92. doi: 10.1128/jb.00498-06
17. Jan AT. Outer membrane vesicles (OMVs) of gram-negative bacteria: A perspective update. Front Microbiol (2017) 8:1053. doi: 10.3389/fmicb.2017.01053
18. Wang S, Gao J, Wang Z. Outer membrane vesicles for vaccination and targeted drug delivery. Wiley Interdiscip Rev Nanomed Nanobiotechnol (2019) 11:e1523. doi: 10.1002/wnan.1523
19. Guerrero-Mandujano A, Hernández-Cortez C, Ibarra JA, Castro-Escarpulli G. The outer membrane vesicles: Secretion system type zero. Traffic (2017) 18:425–32. doi: 10.1111/tra.12488
20. Klimentova J, Rehulka P, Pavkova I, Kubelkova K, Bavlovic J, Stulik J. Cross-species proteomic comparison of outer membrane vesicles and membranes of francisella tularensis subsp. tularensis versus subsp. holarctica. J Proteome Res (2021) 20:1716–32. doi: 10.1021/acs.jproteome.0c00917
21. Renelli M, Matias V, Lo RY, Beveridge TJ. DNA-Containing membrane vesicles of pseudomonas aeruginosa PAO1 and their genetic transformation potential. Microbiol (Reading) (2004) 150:2161–9. doi: 10.1099/mic.0.26841-0
22. Lee EY, Choi DS, Kim KP, Gho YS. Proteomics in gram-negative bacterial outer membrane vesicles. Mass Spectrom Rev (2008) 27:535–55. doi: 10.1002/mas.20175
23. Ghosal A, Upadhyaya BB, Fritz JV, Heintz-Buschart A, Desai MS, Yusuf D, et al. The extracellular RNA complement of escherichia coli. Microbiologyopen (2015) 4:252–66. doi: 10.1002/mbo3.235
24. Sjöström AE, Sandblad L, Uhlin BE, Wai SN. Membrane vesicle-mediated release of bacterial RNA. Sci Rep (2015) 5:15329. doi: 10.1038/srep15329
25. Blenkiron C, Simonov D, Muthukaruppan A, Tsai P, Dauros P, Green S, et al. Uropathogenic escherichia coli releases extracellular vesicles that are associated with RNA. PLos One (2016) 11:e0160440. doi: 10.1371/journal.pone.0160440
26. Koeppen K, Hampton TH, Jarek M, Scharfe M, Gerber SA, Mielcarz DW, et al. A novel mechanism of host-pathogen interaction through sRNA in bacterial outer membrane vesicles. PLos Pathog (2016) 12:e1005672. doi: 10.1371/journal.ppat.1005672
27. Kohl P, Zingl FG, Eichmann TO, Schild S. Isolation of outer membrane vesicles including their quantitative and qualitative analyses. Methods Mol Biol (2018) 1839:117–34. doi: 10.1007/978-1-4939-8685-9_11
28. Tashiro Y, Inagaki A, Shimizu M, Ichikawa S, Takaya N, Nakajima-Kambe T, et al. Characterization of phospholipids in membrane vesicles derived from pseudomonas aeruginosa. Biosci Biotechnol Biochem (2011) 75:605–7. doi: 10.1271/bbb.100754
29. Yu YJ, Wang XH, Fan GC. Versatile effects of bacterium-released membrane vesicles on mammalian cells and infectious/inflammatory diseases. Acta Pharmacol Sin (2018) 39:514–33. doi: 10.1038/aps.2017.82
30. Strahl H, Errington J. Bacterial membranes: Structure, domains, and function. Annu Rev Microbiol (2017) 71:519–38. doi: 10.1146/annurev-micro-102215-095630
31. Hynes SO, Keenan JI, Ferris JA, Annuk H, Moran AP. Lewis Epitopes on outer membrane vesicles of relevance to helicobacter pylori pathogenesis. Helicobacter (2005) 10:146–56. doi: 10.1111/j.1523-5378.2005.00302.x
32. Poltorak A, He X, Smirnova I, Liu MY, Van Huffel C, Du X, et al. Defective LPS signaling in C3H/HeJ and C57BL/10ScCr mice: mutations in Tlr4 gene. Science (1998) 282:2085–8. doi: 10.1126/science.282.5396.2085
33. Gerke C, Colucci AM, Giannelli C, Sanzone S, Vitali CG, Sollai L, et al. Production of a shigella sonnei vaccine based on generalized modules for membrane antigens (GMMA), 1790GAHB. PLos One (2015) 10:e0134478. doi: 10.1371/journal.pone.0134478
34. Raetz CR, Reynolds CM, Trent MS, Bishop RE. Lipid a modification systems in gram-negative bacteria. Annu Rev Biochem (2007) 76:295–329. doi: 10.1146/annurev.biochem.76.010307.145803
35. Trent MS, Stead CM, Tran AX, Hankins JV. Diversity of endotoxin and its impact on pathogenesis. J Endotoxin Res (2006) 12:205–23. doi: 10.1179/096805106x118825
36. Clementz T, Bednarski JJ, Raetz CR. Function of the htrB high temperature requirement gene of escherichia coli in the acylation of lipid a: HtrB catalyzed incorporation of laurate. J Biol Chem (1996) 271:12095–102. doi: 10.1074/jbc.271.20.12095
37. Clementz T, Zhou Z, Raetz CR. Function of the escherichia coli msbB gene, a multicopy suppressor of htrB knockouts, in the acylation of lipid a. acylation by MsbB follows laurate incorporation by HtrB. J Biol Chem (1997) 272:10353–60. doi: 10.1074/jbc.272.16.10353
38. Bishop RE, Kim SH, El Zoeiby A. Role of lipid a palmitoylation in bacterial pathogenesis. J Endotoxin Res (2005) 11:174–80. doi: 10.1179/096805105x35242
39. Reynolds CM, Ribeiro AA, McGrath SC, Cotter RJ, Raetz CRH, Trent MS. An outer membrane enzyme encoded by salmonella typhimurium lpxR that removes the 3'-acyloxyacyl moiety of lipid a. J Biol Chem (2006) 281:21974–87. doi: 10.1074/jbc.M603527200
40. Kawasaki K, Teramoto M, Tatsui R, Amamoto S. Lipid a 3'-o-deacylation by salmonella outer membrane enzyme LpxR modulates the ability of lipid a to stimulate toll-like receptor 4. Biochem Biophys Res Commun (2012) 428:343–7. doi: 10.1016/j.bbrc.2012.10.054
41. Lee H, Hsu FF, Turk J, Groisman EA. The PmrA-regulated pmrC gene mediates phosphoethanolamine modification of lipid a and polymyxin resistance in salmonella enterica. J Bacteriol (2004) 186:4124–33. doi: 10.1128/jb.186.13.4124-4133.2004
42. Anandan A, Vrielink A. Structure and function of lipid a-modifying enzymes. Ann N Y Acad Sci (2020) 1459:19–37. doi: 10.1111/nyas.14244
43. Kong Q, Six DA, Roland KL, Liu Q, Gu L, Reynolds CM, et al. Salmonella synthesizing 1-dephosphorylated [corrected] lipopolysaccharide exhibits low endotoxic activity while retaining its immunogenicity. J Immunol (2011) 187:412–23. doi: 10.4049/jimmunol.1100339
44. Vorachek-Warren MK, Carty SM, Lin S, Cotter RJ, Raetz CR. An escherichia coli mutant lacking the cold shock-induced palmitoleoyltransferase of lipid a biosynthesis: absence of unsaturated acyl chains and antibiotic hypersensitivity at 12 degrees c. J Biol Chem (2002) 277:14186–93. doi: 10.1074/jbc.M200408200
45. Needham BD, Carroll SM, Giles DK, Georgiou G, Whiteley M, Trent MS. Modulating the innate immune response by combinatorial engineering of endotoxin. Proc Natl Acad Sci U.S.A. (2013) 110:1464–9. doi: 10.1073/pnas.1218080110
46. Rossi O, Pesce I, Giannelli C, Aprea S, Caboni M, Citiulo F, et al. Modulation of endotoxicity of shigella generalized modules for membrane antigens (GMMA) by genetic lipid a modifications: Relative activation of TLR4 and TLR2 pathways in different mutants. J Biol Chem (2014) 289:24922–35. doi: 10.1074/jbc.M114.566570
47. Berlanda Scorza F, Colucci AM, Maggiore L, Sanzone S, Rossi O, Ferlenghi I, et al. High yield production process for shigella outer membrane particles. PLos One (2012) 7:e35616. doi: 10.1371/journal.pone.0035616
48. Keiser PB, Biggs-Cicatelli S, Moran EE, Schmiel DH, Pinto VB, Burden RE, et al. A phase 1 study of a meningococcal native outer membrane vesicle vaccine made from a group b strain with deleted lpxL1 and synX, over-expressed factor h binding protein, two PorAs and stabilized OpcA expression. Vaccine (2011) 29:1413–20. doi: 10.1016/j.vaccine.2010.12.039
49. Keiser PB, Gibbs BT, Coster TS, Moran EE, Stoddard MB, Labrie JE 3rd, et al. A phase 1 study of a group b meningococcal native outer membrane vesicle vaccine made from a strain with deleted lpxL2 and synX and stable expression of opcA. Vaccine (2010) 28:6970–6. doi: 10.1016/j.vaccine.2010.08.048
50. Rossi O, Caboni M, Negrea A, Necchi F, Alfini R, Micoli F, et al. Toll-like receptor activation by generalized modules for membrane antigens from lipid a mutants of salmonella enterica serovars typhimurium and enteritidis. Clin Vaccine Immunol (2016) 23:304–14. doi: 10.1128/cvi.00023-16
51. Fransen F, Boog CJ, van Putten JP, van der Ley P. Agonists of toll-like receptors 3, 4, 7, and 9 are candidates for use as adjuvants in an outer membrane vaccine against neisseria meningitidis serogroup b. Infect Immun (2007) 75:5939–46. doi: 10.1128/iai.00846-07
52. Bauman SJ, Kuehn MJ. Purification of outer membrane vesicles from pseudomonas aeruginosa and their activation of an IL-8 response. Microbes Infect (2006) 8:2400–8. doi: 10.1016/j.micinf.2006.05.001
53. Maggiore L, Yu L, Omasits U, Rossi O, Dougan G, Thomson NR, et al. Quantitative proteomic analysis of shigella flexneri and shigella sonnei generalized modules for membrane antigens (GMMA) reveals highly pure preparations. Int J Med Microbiol (2016) 306:99–108. doi: 10.1016/j.ijmm.2015.12.003
54. Caruana JC, Walper SA. Bacterial membrane vesicles as mediators of microbe - microbe and microbe - host community interactions. Front Microbiol (2020) 11:432. doi: 10.3389/fmicb.2020.00432
55. Schooling SR, Beveridge TJ. Membrane vesicles: An overlooked component of the matrices of biofilms. J Bacteriol (2006) 188:5945–57. doi: 10.1128/jb.00257-06
56. Li M, Zhou H, Yang C, Wu Y, Zhou X, Liu H, et al. Bacterial outer membrane vesicles as a platform for biomedical applications: An update. J Control Release (2020) 323:253–68. doi: 10.1016/j.jconrel.2020.04.031
57. Kulkarni HM, Nagaraj R, Jagannadham MV. Protective role of e. coli outer membrane vesicles against antibiotics. Microbiol Res (2015) 181:1–7. doi: 10.1016/j.micres.2015.07.008
58. Lee J, Lee EY, Kim SH, Kim DK, Park KS, Kim KP, et al. Staphylococcus aureus extracellular vesicles carry biologically active β-lactamase. Antimicrob Agents Chemother (2013) 57:2589–95. doi: 10.1128/aac.00522-12
59. Yang J, Hwang I, Lee E, Shin SJ, Lee EJ, Rhee JH, et al. Bacterial outer membrane vesicle-mediated cytosolic delivery of flagellin triggers host NLRC4 canonical inflammasome signaling. Front Immunol (2020) 11:581165. doi: 10.3389/fimmu.2020.581165
60. Hering NA, Fromm M, Schulzke JD. Determinants of colonic barrier function in inflammatory bowel disease and potential therapeutics. J Physiol (2012) 590:1035–44. doi: 10.1113/jphysiol.2011.224568
61. Alvarez CS, Badia J, Bosch M, Giménez R, Baldomà L. Outer membrane vesicles and soluble factors released by probiotic escherichia coli nissle 1917 and commensal ECOR63 enhance barrier function by regulating expression of tight junction proteins in intestinal epithelial cells. Front Microbiol (2016) 7:1981. doi: 10.3389/fmicb.2016.01981
62. Fiocca R, Necchi V, Sommi P, Ricci V, Telford J, Cover TL, et al. Release of helicobacter pylori vacuolating cytotoxin by both a specific secretion pathway and budding of outer membrane vesicles. uptake of released toxin and vesicles by gastric epithelium. J Pathol (1999) 188:220–6. doi: 10.1002/(sici)1096-9896(199906)188:2<220::Aid-path307>3.0.Co;2-c
63. Parker H, Chitcholtan K, Hampton MB, Keenan JI. Uptake of helicobacter pylori outer membrane vesicles by gastric epithelial cells. Infect Immun (2010) 78:5054–61. doi: 10.1128/iai.00299-10
64. Han EC, Choi SY, Lee Y, Park JW, Hong SH, Lee HJ. Extracellular RNAs in periodontopathogenic outer membrane vesicles promote TNF-α production in human macrophages and cross the blood-brain barrier in mice. FASEB J (2019) 33:13412–22. doi: 10.1096/fj.201901575R
65. Coburn B, Sekirov I, Finlay BB. Type III secretion systems and disease. Clin Microbiol Rev (2007) 20:535–49. doi: 10.1128/cmr.00013-07
66. Fan X, Alekseyenko AV, Wu J, Peters BA, Jacobs EJ, Gapstur SM, et al. Human oral microbiome and prospective risk for pancreatic cancer: a population-based nested case-control study. Gut (2018) 67:120–7. doi: 10.1136/gutjnl-2016-312580
67. Olsen I, Yilmaz Ö. Possible role of porphyromonas gingivalis in orodigestive cancers. J Oral Microbiol (2019) 11:1563410. doi: 10.1080/20002297.2018.1563410
68. Michaud DS, Joshipura K, Giovannucci E, Fuchs CS. A prospective study of periodontal disease and pancreatic cancer in US male health professionals. J Natl Cancer Inst (2007) 99:171–5. doi: 10.1093/jnci/djk021
69. Michaud DS, Izard J. Microbiota, oral microbiome, and pancreatic cancer. Cancer J (2014) 20:203–6. doi: 10.1097/ppo.0000000000000046
70. Ahn J, Segers S, Hayes RB. Periodontal disease, porphyromonas gingivalis serum antibody levels and orodigestive cancer mortality. Carcinogenesis (2012) 33:1055–8. doi: 10.1093/carcin/bgs112
71. Gabarrini G, de Smit M, Westra J, Brouwer E, Vissink A, Zhou K, et al. The peptidylarginine deiminase gene is a conserved feature of porphyromonas gingivalis. Sci Rep (2015) 5:13936. doi: 10.1038/srep13936
72. Goulas T, Mizgalska D, Garcia-Ferrer I, Kantyka T, Guevara T, Szmigielski B, et al. Structure and mechanism of a bacterial host-protein citrullinating virulence factor, porphyromonas gingivalis peptidylarginine deiminase. Sci Rep (2015) 5:11969. doi: 10.1038/srep11969
73. Gabarrini G, Grasso S, van Winkelhoff AJ, van Dijl JM. Gingimaps: Protein localization in the oral pathogen porphyromonas gingivalis. Microbiol Mol Biol Rev (2020) 84:e00032–19. doi: 10.1128/mmbr.00032-19
74. Conlin A, Smith G, Carey FA, Wolf CR, Steele RJ. The prognostic significance of K-ras, p53, and APC mutations in colorectal carcinoma. Gut (2005) 54:1283–6. doi: 10.1136/gut.2005.066514
75. Wu CY, Carpenter ES, Takeuchi KK, Halbrook CJ, Peverley LV, Bien H, et al. PI3K regulation of RAC1 is required for KRAS-induced pancreatic tumorigenesis in mice. Gastroenterology (2014) 147:1405–1416.e1407. doi: 10.1053/j.gastro.2014.08.032
76. van de Wouw M, Boehme M, Lyte JM, Wiley N, Strain C, O'Sullivan O, et al. Short-chain fatty acids: microbial metabolites that alleviate stress-induced brain-gut axis alterations. J Physiol (2018) 596:4923–44. doi: 10.1113/jp276431
77. Medina V, Edmonds B, Young GP, James R, Appleton S, Zalewski PD. Induction of caspase-3 protease activity and apoptosis by butyrate and trichostatin a (inhibitors of histone deacetylase): Dependence on protein synthesis and synergy with a mitochondrial/cytochrome c-dependent pathway. Cancer Res (1997) 57:3697–707.
78. Koppenol WH, Bounds PL, Dang CV. Otto Warburg's contributions to current concepts of cancer metabolism. Nat Rev Cancer (2011) 11:325–37. doi: 10.1038/nrc3038
79. Eslami M, Sadrifar S, Karbalaei M, Keikha M, Kobyliak NM, Yousefi B. Importance of the microbiota inhibitory mechanism on the warburg effect in colorectal cancer cells. J Gastrointest Cancer (2020) 51:738–47. doi: 10.1007/s12029-019-00329-3
80. Dashwood RH, Myzak MC, Ho E. Dietary HDAC inhibitors: time to rethink weak ligands in cancer chemoprevention? Carcinogenesis (2006) 27:344–9. doi: 10.1093/carcin/bgi253
81. Lv H, Zhang Z, Wang Y, Li C, Gong W, Wang X. MicroRNA-92a promotes colorectal cancer cell growth and migration by inhibiting KLF4. Oncol Res (2016) 23:283–90. doi: 10.3727/096504016x14562725373833
82. Tsuchida A, Ohno S, Wu W, Borjigin N, Fujita K, Aoki T, et al. miR-92 is a key oncogenic component of the miR-17-92 cluster in colon cancer. Cancer Sci (2011) 102:2264–71. doi: 10.1111/j.1349-7006.2011.02081.x
83. Milella M, Falcone I, Conciatori F, Cesta Incani U, Del Curatolo A, Inzerilli N, et al. PTEN: Multiple functions in human malignant tumors. Front Oncol (2015) 5:24. doi: 10.3389/fonc.2015.00024
84. Hu S, Liu L, Chang EB, Wang JY, Raufman JP. Butyrate inhibits pro-proliferative miR-92a by diminishing c-myc-induced miR-17-92a cluster transcription in human colon cancer cells. Mol Cancer (2015) 14:180. doi: 10.1186/s12943-015-0450-x
85. Li Q, Ding C, Meng T, Lu W, Liu W, Hao H, et al. Butyrate suppresses motility of colorectal cancer cells via deactivating Akt/ERK signaling in histone deacetylase dependent manner. J Pharmacol Sci (2017) 135:148–55. doi: 10.1016/j.jphs.2017.11.004
86. Chiang Y, Song Y, Wang Z, Chen Y, Yue Z, Xu H, et al. Aberrant expression of miR-203 and its clinical significance in gastric and colorectal cancers. J Gastrointest Surg (2011) 15:63–70. doi: 10.1007/s11605-010-1367-8
87. Castosa R, Martinez-Iglesias O, Roca-Lema D, Casas-Pais A, Diaz-Diaz A, Iglesias P, et al. Hakai overexpression effectively induces tumour progression and metastasis. vivo Sci Rep (2018) 8:3466. doi: 10.1038/s41598-018-21808-w
88. Han R, Sun Q, Wu J, Zheng P, Zhao G. Sodium butyrate upregulates miR-203 expression to exert anti-proliferation effect on colorectal cancer cells. Cell Physiol Biochem (2016) 39:1919–29. doi: 10.1159/000447889
89. Kimura H. Production and physiological effects of hydrogen sulfide. Antioxid Redox Signal (2014) 20:783–93. doi: 10.1089/ars.2013.5309
90. O'Keefe SJ, Li JV, Lahti L, Ou J, Carbonero F, Mohammed K, et al. Fat, fibre and cancer risk in African americans and rural africans. Nat Commun (2015) 6:6342. doi: 10.1038/ncomms7342
91. Ijssennagger N, van der Meer R, van Mil SWC. Sulfide as a mucus barrier-breaker in inflammatory bowel disease? Trends Mol Med (2016) 22:190–9. doi: 10.1016/j.molmed.2016.01.002
92. Rubinstein MR, Wang X, Liu W, Hao Y, Cai G, Han YW. Fusobacterium nucleatum promotes colorectal carcinogenesis by modulating e-cadherin/β-catenin signaling via its FadA adhesin. Cell Host Microbe (2013) 14:195–206. doi: 10.1016/j.chom.2013.07.012
93. Xu M, Yamada M, Li M, Liu H, Chen SG, Han YW. FadA from fusobacterium nucleatum utilizes both secreted and nonsecreted forms for functional oligomerization for attachment and invasion of host cells. J Biol Chem (2007) 282:25000–9. doi: 10.1074/jbc.M611567200
94. Sun CH, Li BB, Wang B, Zhao J, Zhang XY, Li TT, et al. The role of fusobacterium nucleatum in colorectal cancer: From carcinogenesis to clinical management. Chronic Dis Transl Med (2019) 5:178–87. doi: 10.1016/j.cdtm.2019.09.001
95. Ge Z, Schauer DB, Fox JG. In vivo virulence properties of bacterial cytolethal-distending toxin. Cell Microbiol (2008) 10:1599–607. doi: 10.1111/j.1462-5822.2008.01173.x
96. Wu S, Rhee KJ, Albesiano E, Rabizadeh S, Wu X, Yen HR, et al. A human colonic commensal promotes colon tumorigenesis via activation of T helper type 17 T cell responses. Nat Med (2009) 15:1016–22. doi: 10.1038/nm.2015
97. Cuevas-Ramos G, Petit CR, Marcq I, Boury M, Oswald E, Nougayrede JP. Escherichia coli induces DNA damage in vivo and triggers genomic instability in mammalian cells. Proc Natl Acad Sci U.S.A. (2010) 107:11537–42. doi: 10.1073/pnas.1001261107
98. Dalmasso G, Cougnoux A, Delmas J, Darfeuille-Michaud A, Bonnet R. The bacterial genotoxin colibactin promotes colon tumor growth by modifying the tumor microenvironment. Gut Microbes (2014) 5:675–80. doi: 10.4161/19490976.2014.969989
99. He Z, Gharaibeh RZ, Newsome RC, Pope JL, Dougherty MW, Tomkovich S, et al. Campylobacter jejuni promotes colorectal tumorigenesis through the action of cytolethal distending toxin. Gut (2019) 68:289–300. doi: 10.1136/gutjnl-2018-317200
100. Krishnan N, Kubiatowicz LJ, Holay M, Zhou J, Fang RH, Zhang L. Bacterial membrane vesicles for vaccine applications. Adv Drug Delivery Rev (2022) 185:114294. doi: 10.1016/j.addr.2022.114294
101. Jechlinger W, Haller C, Resch S, Hofmann A, Szostak MP, Lubitz W. Comparative immunogenicity of the hepatitis b virus core 149 antigen displayed on the inner and outer membrane of bacterial ghosts. Vaccine (2005) 23:3609–17. doi: 10.1016/j.vaccine.2004.11.078
102. Parlane NA, Grage K, Lee JW, Buddle BM, Denis M, Rehm BH. Production of a particulate hepatitis c vaccine candidate by an engineered lactococcus lactis strain. Appl Environ Microbiol (2011) 77:8516–22. doi: 10.1128/aem.06420-11
103. Hekmat S, Sadat SM, Aslani MM, Mahdavi M, Bolhassani A, Asgar Halvaee F, et al. Truncated Core/NS3 fusion protein of HCV adjuvanted with outer membrane vesicles of neisseria meningitidis serogroup b: Potent inducer of the murine immune system. Iran BioMed J (2019) 23:235–45. doi: 10.29252/.23.4.235
104. Shah MS, DeSantis TZ, Weinmaier T, McMurdie PJ, Cope JL, Altrichter A, et al. Leveraging sequence-based faecal microbial community survey data to identify a composite biomarker for colorectal cancer. Gut (2018) 67:882–91. doi: 10.1136/gutjnl-2016-313189
105. Turley SJ, Inaba K, Garrett WS, Ebersold M, Unternaehrer J, Steinman RM, et al. Transport of peptide-MHC class II complexes in developing dendritic cells. Science (2000) 288:522–7. doi: 10.1126/science.288.5465.522
106. Hornung V, Rothenfusser S, Britsch S, Krug A, Jahrsdorfer B, Giese T, et al. Quantitative expression of toll-like receptor 1-10 mRNA in cellular subsets of human peripheral blood mononuclear cells and sensitivity to CpG oligodeoxynucleotides. J Immunol (2002) 168:4531–7. doi: 10.4049/jimmunol.168.9.4531
107. Schulz O, Diebold SS, Chen M, Naslund TI, Nolte MA, Alexopoulou L, et al. Toll-like receptor 3 promotes cross-priming to virus-infected cells. Nature (2005) 433:887–92. doi: 10.1038/nature03326
108. Edwards AD, Diebold SS, Slack EM, Tomizawa H, Hemmi H, Kaisho T, et al. Toll-like receptor expression in murine DC subsets: lack of TLR7 expression by CD8 alpha+ DC correlates with unresponsiveness to imidazoquinolines. Eur J Immunol (2003) 33:827–33. doi: 10.1002/eji.200323797
109. Browne EP. Regulation of b-cell responses by toll-like receptors. Immunology (2012) 136:370–9. doi: 10.1111/j.1365-2567.2012.03587.x
110. Visintin A, Mazzoni A, Spitzer JH, Wyllie DH, Dower SK, Segal DM. Regulation of toll-like receptors in human monocytes and dendritic cells. J Immunol (2001) 166:249–55. doi: 10.4049/jimmunol.166.1.249
111. Canto E, Ricart E, Monfort D, Gonzalez-Juan D, Balanzo J, Rodriguez-Sanchez JL, et al. TNF alpha production to TLR2 ligands in active IBD patients. Clin Immunol (2006) 119:156–65. doi: 10.1016/j.clim.2005.12.005
112. Schlaepfer E, Speck RF. Anti-HIV activity mediated by natural killer and CD8+ cells after toll-like receptor 7/8 triggering. PLos One (2008) 3:e1999. doi: 10.1371/journal.pone.0001999
113. Stough JMA, Beaudoin AJ, Schloss PD. Coding-complete RNA virus genomes assembled from murine cecal metatranscriptomes. Microbiol Resour Announc (2020) 9:e00018-20. doi: 10.1128/mra.00018-20
114. Takeuchi O, Hoshino K, Kawai T, Sanjo H, Takada H, Ogawa T, et al. Differential roles of TLR2 and TLR4 in recognition of gram-negative and gram-positive bacterial cell wall components. Immunity (1999) 11:443–51. doi: 10.1016/s1074-7613(00)80119-3
115. Hausmann M, Kiessling S, Mestermann S, Webb G, Spottl T, Andus T, et al. Toll-like receptors 2 and 4 are up-regulated during intestinal inflammation. Gastroenterology (2002) 122:1987–2000. doi: 10.1053/gast.2002.33662
116. Round JL, Mazmanian SK. The gut microbiota shapes intestinal immune responses during health and disease. Nat Rev Immunol (2009) 9:313–23. doi: 10.1038/nri2515
117. Chiang CJ, Huang PH. Metabolic engineering of probiotic escherichia coli for cytolytic therapy of tumors. Sci Rep (2021) 11:5853. doi: 10.1038/s41598-021-85372-6
118. Kuerban K, Gao X, Zhang H, Liu J, Dong M, Wu L, et al. Doxorubicin-loaded bacterial outer-membrane vesicles exert enhanced anti-tumor efficacy in non-small-cell lung cancer. Acta Pharm Sin B (2020) 10:1534–48. doi: 10.1016/j.apsb.2020.02.002
119. Guo Q, Li X, Zhou W, Chu Y, Chen Q, Zhang Y, et al. Sequentially triggered bacterial outer membrane vesicles for macrophage metabolism modulation and tumor metastasis suppression. ACS Nano (2021) 15:13826–38. doi: 10.1021/acsnano.1c05613
120. Chen Q, Bai H, Wu W, Huang G, Li Y, Wu M, et al. Bioengineering bacterial vesicle-coated polymeric nanomedicine for enhanced cancer immunotherapy and metastasis prevention. Nano Lett (2020) 20:11–21. doi: 10.1021/acs.nanolett.9b02182
121. Qing S, Lyu C, Zhu L, Pan C, Wang S, Li F, et al. Biomineralized bacterial outer membrane vesicles potentiate safe and efficient tumor microenvironment reprogramming for anticancer therapy. Adv Mater (2020) 32:e2002085. doi: 10.1002/adma.202002085
122. Zhuang Q, Xu J, Deng D, Chao T, Li J, Zhang R, et al. Bacteria-derived membrane vesicles to advance targeted photothermal tumor ablation. Biomaterials (2021) 268:120550. doi: 10.1016/j.biomaterials.2020.120550
123. Patel RB, Ye M, Carlson PM, Jaquish A, Zangl L, Ma B, et al. Development of an In situ cancer vaccine via combinational radiation and bacterial-Membrane-Coated nanoparticles. Adv Mater (2019) 31:e1902626. doi: 10.1002/adma.201902626
124. Zhou J, Karshalev E, Mundaca-Uribe R, Esteban-Fernandez de Avila B, Krishnan N, Xiao C, et al. Physical disruption of solid tumors by immunostimulatory microrobots enhances antitumor immunity. Adv Mater (2021) 33:e2103505. doi: 10.1002/adma.202103505
125. Li TT, Liu H, Yu J, Shi GY, Zhao LY, Li GX. Prognostic and predictive blood biomarkers in gastric cancer and the potential application of circulating tumor cells. World J Gastroenterol (2018) 24:2236–46. doi: 10.3748/wjg.v24.i21.2236
126. Liu X, Lu Y, Xu Y, Hou S, Huang J, Wang B, et al. Exosomal transfer of miR-501 confers doxorubicin resistance and tumorigenesis via targeting of BLID in gastric cancer. Cancer Lett (2019) 459:122–34. doi: 10.1016/j.canlet.2019.05.035
127. Louvet C, Andre T, Tigaud JM, Gamelin E, Douillard JY, Brunet R, et al. Phase II study of oxaliplatin, fluorouracil, and folinic acid in locally advanced or metastatic gastric cancer patients. J Clin Oncol (2002) 20:4543–8. doi: 10.1200/jco.2002.02.021
128. Raposo G, Stoorvogel W. Extracellular vesicles: Exosomes, microvesicles, and friends. J Cell Biol (2013) 200:373–83. doi: 10.1083/jcb.201211138
129. Abreu MT. Toll-like receptor signalling in the intestinal epithelium: How bacterial recognition shapes intestinal function. Nat Rev Immunol (2010) 10:131–44. doi: 10.1038/nri2707
130. Takahashi K, Yan IK, Kogure T, Haga H, Patel T. Extracellular vesicle-mediated transfer of long non-coding RNA ROR modulates chemosensitivity in human hepatocellular cancer. FEBS Open Bio (2014) 4:458–67. doi: 10.1016/j.fob.2014.04.007
131. Ferlay J, Soerjomataram I, Dikshit R, Eser S, Mathers C, Rebelo M, et al. Cancer incidence and mortality worldwide: Sources, methods and major patterns in GLOBOCAN 2012. Int J Cancer (2015) 136:E359–386. doi: 10.1002/ijc.29210
132. Chen Q, Huang G, Wu W, Wang J, Hu J, Mao J, et al. A hybrid eukaryotic-prokaryotic nanoplatform with photothermal modality for enhanced antitumor vaccination. Adv Mater (2020) 32:e1908185. doi: 10.1002/adma.201908185
133. Wang D, Liu C, You S, Zhang K, Li M, Cao Y, et al. Bacterial vesicle-cancer cell hybrid membrane-coated nanoparticles for tumor specific immune activation and photothermal therapy. ACS Appl Mater Interfaces (2020) 12:41138–47. doi: 10.1021/acsami.0c13169
134. Zhou J, Kroll AV, Holay M, Fang RH, Zhang L. Biomimetic nanotechnology toward personalized vaccines. Adv Mater (2020) 32:e1901255. doi: 10.1002/adma.201901255
135. Rocha FG, Ottenberg G, Eure ZG, Davey ME, Gibson FC 3rd. Sphingolipid-containing outer membrane vesicles serve as a delivery vehicle to limit macrophage immune response to porphyromonas gingivalis. Infect Immun (2021) 89:e00614-20. doi: 10.1128/iai.00614-20
Keywords: extracellular vesicles (EVs), cancer, immunotherapy, TLR, LPS, PAMP
Citation: Ge Y, Sun F, Zhao B, Kong F, Li Z and Kong X (2023) Bacteria derived extracellular vesicles in the pathogenesis and treatment of gastrointestinal tumours. Front. Oncol. 12:1103446. doi: 10.3389/fonc.2022.1103446
Received: 20 November 2022; Accepted: 28 December 2022;
Published: 23 January 2023.
Edited by:
Zhangjun Cheng, Southeast University, ChinaReviewed by:
Zhiqiang Liu, Tianjin Medical University, ChinaCopyright © 2023 Ge, Sun, Zhao, Kong, Li and Kong. This is an open-access article distributed under the terms of the Creative Commons Attribution License (CC BY). The use, distribution or reproduction in other forums is permitted, provided the original author(s) and the copyright owner(s) are credited and that the original publication in this journal is cited, in accordance with accepted academic practice. No use, distribution or reproduction is permitted which does not comply with these terms.
*Correspondence: Xiangyu Kong, eGlhbmd5dWtvbmcxODVAaG90bWFpbC5jb20=; Zhaoshen Li, emhhb3NoZW5saS5zbW11LmVkdUBob3RtYWlsLmNvbQ==; Fanyang Kong, a2Z5ODE5NTI4OEAxNjMuY29t
†These authors share first authorship
‡These authors have contributed equally to this work
Disclaimer: All claims expressed in this article are solely those of the authors and do not necessarily represent those of their affiliated organizations, or those of the publisher, the editors and the reviewers. Any product that may be evaluated in this article or claim that may be made by its manufacturer is not guaranteed or endorsed by the publisher.
Research integrity at Frontiers
Learn more about the work of our research integrity team to safeguard the quality of each article we publish.