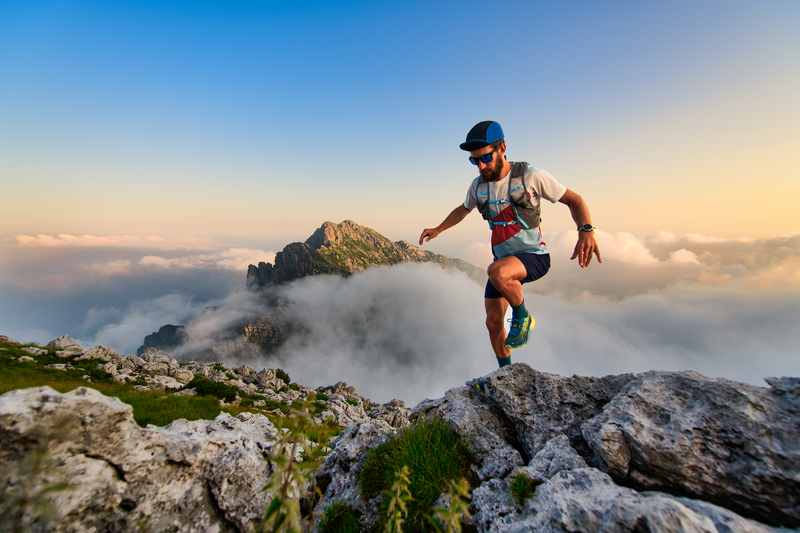
94% of researchers rate our articles as excellent or good
Learn more about the work of our research integrity team to safeguard the quality of each article we publish.
Find out more
REVIEW article
Front. Oncol. , 16 February 2023
Sec. Radiation Oncology
Volume 12 - 2022 | https://doi.org/10.3389/fonc.2022.1085432
This article is part of the Research Topic Integrating Nanotechnology and Nanomedicine into Cancer Radiotherapy View all 4 articles
Radiotherapy remains the major therapeutic intervention for tumor patients. However, the hypoxic tumor microenvironment leads to treatment resistance. Recently, a burgeoning number of nano-radiosensitizers designed to increase the oxygen concentration in tumors were reported. These nano radiosensitizers served as oxygen carriers, oxygen generators, and even sustained oxygen pumps, attracting increased research interest. In this review, we focus on the novel oxygen-enrich nano radiosensitizers, which we call oxygen switches, and highlight their influence in radiotherapy through different strategies. Physical strategies-based oxygen switches carried O2 into the tumor via their high oxygen capacity. The chemical reactions to generate O2 in situ were triggered by chemical strategies-based oxygen switches. Biological strategies-based oxygen switches regulated tumor metabolism, remodeled tumor vasculature, and even introduced microorganisms-mediated photosynthesis for long-lasting hypoxia alleviating. Moreover, the challenges and perspectives of oxygen switches-mediated oxygen-enrich radiotherapy were discussed.
Radiotherapy remains a major treatment for tumor patients (1). It is reported that 50% of tumor patients required radiotherapy (2). Oxygen serves as the fuel to stabilize DNA damage caused by radiation and prevent a DNA self-repair process (3). However, the efficacy of radiotherapy has been severely limited due to the hypoxia status in most solid tumors (4). Hypoxia not only leads to limited treatment efficacy but also causes tumor recurrence and metastasis after radiotherapy. The establishment of the hypoxia microenvironment is an outcome for multiple reasons, including tumor cell proliferation, abnormal vascular distribution, reprogrammed energy metabolism, etc. Oxygen-enriched strategies are necessary for refueling cancer radiotherapy.
To relieve hypoxia in the tumor microenvironment, various oxygen delivery strategies have been tested nowadays. Hyperbaric oxygen (HBO) inhalation cannot meet the need for radiotherapy, because the oxygen level only remained elevated for a short time (5). What is worse, combining erythropoietin treatment and radiotherapy for head and neck tumors resulted in significantly worse prognosis in patients (6). This phenomenon presumably ascribes to that squamous cell carcinoma tumor cells also express the erythropoietin receptor (7). Artificial blood including perfluorocarbon and hemoglobin-based oxygen-carrying solutions have been explored to increase the effectiveness of radiotherapy in rodent tumors (8). But contentious results for different doses and different schedules retarded clinical translation. A more precise and intelligent oxygen delivery strategy is needed.
To further improve the efficiency of radiotherapy, nano oxygen modulators have raised global attention. As oxygen serves as the fuel to fix radiation-induced DNA damage, radiotherapy could be boosted by evaluating oxygen concentration. In this review, a variety of agents introduced for oxygen modulation show magnifying effects for radiotherapy. Thus, we collectively refer to these nano agents as “Oxygen Switch”, which allows precise and high performance in reshaping the hypoxia microenvironment (Figure 1). The physical strategies including hemoglobin-based and perfluorocarbon-based oxygen carriers will be first introduced in this review, followed by a detailed discussion of chemical strategies including in situ H2O2 catalytic decomposition and metallic oxide decomposition. Next, we highlighted novel biological strategies such as in-situ photosynthesis and tumor vasculature remodeling. The mechanism and applications of these “oxygen switches” for radiotherapy enhancement are also covered. Finally, the challenges and perspectives of oxygen switches utilized in radiotherapy are presented.
Figure 1 The wrestling between tumor hypoxia and related alleviating strategies. (A) Tumor hypoxia remains established for multiple reasons, such as tumor cell proliferation, abnormal vascular distribution, reprogrammed energy metabolism, and so on. Thus, physical strategies, chemical strategies, and biological strategies are designed to relieve tumor hypoxia. (B) Oxygen switches increase O2 supply or decrease O2 consumption to combat treatment resistance to radiotherapy.
To relieve the hypoxia in the tumor microenvironment and improve the efficacy of radiotherapy, delivering exogenous oxygen into the tumor site as a radiosensitizer was first explored in the 1930s (9). Physical strategies refer to directly delivering exogenous oxygen via oxygen carriers without chemical or biological reactions. Though physical only relieves hypoxia temporally, it is enough for the radiation process via precise tumor targeting. To date, perfluorocarbons (PFCs) and hemoglobin (Hb) or their derivatives are regarded as excellent oxygen carriers for their high oxygen capacity and favorable biocompatibility, and emerging physical strategies-based oxygen switches are established (Table 1).
PFCs demonstrated an outstanding oxygen affinity due to their fluorine atoms in the carbon skeleton (21). Of the high biocompatibility and chemical stability, PFCs have been widely used in the clinic, such as in organ transplantation and ultrasound imaging. Once the high oxygen solubility of PFCs was found to enable tumor hypoxia alleviation, PFC-based oxygen carriers were developed to enhance radiotherapy (22). Wang et al. developed a tumor-targeted 1H, 1H-perfluorooctylamine-modified hyaluronic acid-coated perfluorocarbon oxygen carrier, O2@PFC@FHA (perfluorooctylamine-modified hyaluronic acid) (10). For the interaction between HA and CD44 and a large amount of oxygen dissolved in the PFC core, O2@PFC@FHA NPs not only improved the tumor targeting but also enabled more oxygen to reach the hypoxic area of the tumor. Moreover, the encapsulation of FHA reduced the leakage of oxygen in circulation and thereby alleviating tumor hypoxia and strengthening radiotherapy.
The platelet inhibition of PFC was neglected and might contribute to increasing red blood cell infiltration into tumors and improving oxygen supply. Zhou et al. screened all the perfluorocarbon compounds and found that perfluorotributylamine (PFTBA) processed the strongest platelet inhibition effect (17). Thus, the two-way O2 delivery system PFTBA@HSA was established, which took advantage of the platelet inhibition effect of PFTBA (Figure 2A). After the release of physical bound O2 (first step), PFTBA inhibited platelet activation and led to an increase in red blood cell (RBC) infiltration, which delivered oxygen to the tumor as the second step. This work presented a simple but effective method to reverse the resistance of tumor hypoxia to radiotherapy.
Figure 2 The fabrication of oxygen switches. (A) Schematic illustration of PFTBA@HSA preparation (17). Copyright© 2021, The Author(s). (B) Schematic illustration of EMs preparation (3). Copyright© 2021, American Chemical Society. (C) Schematic illustration of CAT-SAHA@PLGA preparation (23). Copyright© 2021, The Author(s).
For the prolonged blood circulation time, endogenous biomimetic methods were established (24). Gao et al. developed PFC@PLGA-RBC membrane (RBCM) NPs, in which the PFC core showed efficient loading of oxygen, as well as greatly prolonged blood circulation time because of the coating of RBCM (18). The treatment efficacy during radiotherapy was remarkably enhanced for the greatly relieved tumor hypoxia. Furthermore, Yu et al. reported a nano RBC is fabricated that replaces heme with perfluorodecalin (FDC) and coated with RBCM (20). This method enabled the delivery of FDC because it cannot be emulsified by any FDA-approved emulsifiers.
For the reversible and inherent oxygen-carrying capability of hemoglobin (Hb), a higher Hb level helped improved the response rate of radiotherapy (25). However, free Hb could not be directly administrated for its poor stability, which breaks the redox homeostasis and causes a severe systemic reaction. Thus, physical encapsulation or chemical conjugation is conducted to overcome these shortcomings.
Gao et al. reported that Hb and curcumin formed self-assembled nanoparticles (12). The self-assembly process was driven by hydrophobic forces and contributed to a higher cell absorption rate and lower cytotoxicity than free curcumin. Combined with the radiosensitivity of curcumin and the oxygen delivery of Hb, these nanoparticles effectively enhanced the radiotherapy for hepatocellular in vivo.
Hafnium (Hf), a high-Z radiosensitizer, coordinated with chlorin e6 (Ce6), and Hb was encapsulated to modulate the oxygen balance in the hypoxic TME by Wei et al. (14). The radioluminescence excited by Hf under X-ray irradiation was used to activate Ce6 for ROS generation by radiodynamic therapy (RDT). Such a multifunctional nanoplatform in the combination of oxygen supply and radiotherapy-RDT might provide a new therapeutic option for cancer eradication.
Oxygen can be generated in a large number of chemical reactions in nature. However, producing O2 in vivo by decomposing oxygenated chemicals safely and steadily is not easy. Different kinds of oxygen switches were developed, including an oxygen catalyzer or being decomposed (Table 2).
Early leakage of delivering exogenous O2 into the tumor site remains a challenge for physical strategies. Thus, delivering the precursors of oxygen to the hypoxic area and generating O2 in situ is attractive.
Chen et al. utilized CuO nanoparticles to generate O2 under microwave irradiation in the tumor microenvironment (38). Decorated with MW sensitizer 1-butyl-3-methylimidazolium hexafluorophosphate (IL) and radiosensitizer of Quercetin (Qu), the mesoporous sandwich SiO2@ZrO2 nanoparticles (SiO2@ZrO2 NPs) persistently released oxygen under MW irradiation, which significantly increased the re-oxygenation ability of tumor cells. Due to the reshaping of the tumor microenvironment, a high inhibition rate of 98.62% was witnessed in the in vivo anti-tumor experiment.
Due to the abnormal metabolism and redox homeostasis, a high level of H2O2 is found in the tumor cells compared to normal cells. To generate O2 via H2O2 decomposition, catalase (CAT) and nano-enzyme-loaded oxygen switches were developed to enhance radiotherapy in situ.
CAT generates oxygen in situ but may be degraded in vivo due to the upregulated protease in the tumor. Zai et al. developed highly protease-resistant E. coli membrane vesicles (EMs) to contain CAT and thus relieve tumor hypoxia for a long time (3) (Figure 2B). EMs demonstrated a higher CAT activity than free CAT even in the concentration of 100-fold protease. Combined with immune stimulation features, EMs maintained their hypoxia relief ability for a long time and enhanced radiotherapy.
Song et al. developed a strategy that delivers exogenous H2O2 to the tumor microenvironment and subsequent CAT-triggered H2O2 decomposition (48). CAT and H2O2 were separately encapsulated within stealthy liposomes for a long-lasting effect in tumor re-oxygenation enhancement. Furthermore, the relieved tumor hypoxia enhanced the therapeutic effects of radiotherapy and reversed the immunosuppressive tumor microenvironment. Combined with CTLA4 blockade, the radio-immunotherapy induced effective anti-tumor immune responses to destruct tumors.
Zhang et al. reported a nanoplatform based on poly(N-vinyl caprolactam) (PVCL) nanogels (NGs) co-loaded with gold (Au) and manganese dioxide (MnO2) nanoparticles (NPs) for sensitized radiotherapy (28). MnO2 displayed the CAT-mimic catalytic activity that decomposed H2O2 to form O2 and alleviate tumor hypoxia (Figure 2C). Resulted Mn2+ exerted a Fenton-like reaction to cause intracellular ROS and made the cells more susceptible to radiotherapy. Meanwhile, Au NPs and Mn (II) transformed from MnO2 NPs guided the in vivo radiotherapy through dual mode CT/MR imaging.
Though chemical strategies display a prolonged oxygen modulation capacity than physical strategies, the hypoxic environment reappears once the chemicals are exhausted. Since tumor hypoxia is the outcome of abnormal biological behavior of tumor cells, emerging biological strategies-based oxygen switches may provide exciting opportunities and should be highlighted (Table 3).
To support accelerated cell proliferation, excessive oxygen consumed is one of the critical reasons for tumor hypoxia. Thus, metabolism regulation-based strategies that inhibit tumor aerobic respiration is promising.
As mitochondria refer to the energy house that consumes oxygen to generate energy for tumor growth, mitochondria-targeted interventions are believed to enhance radiotherapy. Gao et al. fabricated a mitochondria-targeted nano-platform via the integration of a self-assembled peptide and a positively charged cyclen (62). The positively charged cyclen anchored to the mitochondria and loaded lonidamine, which served as the energy stripper of cancer cells, inhibited energy metabolism and oxygen consumption. Combined with radiotherapy and endogenous apoptosis pathway, this mitochondria-targeted intervention led to tumor eradication in vivo.
Recently, therapeutic gases were attractive and found to exhibit regulation effects. Nitric oxide (NO), hydrogen sulfide (H2S), and carbon monoxide (CO) were utilized in tumor treatment for their therapeutic capacity. Duo et al. designed an irradiation-triggered NO-release nano-prodrug to improve radiosensitization (62). Through the reaction of sodium nitroprusside and L-glutathione, high content of NO was released and thus inhibited cell respiration and oxygen consumption. Then O2 accumulation improved the therapeutic outcomes under irradiation by generating more ROS in the tumor microenvironment. Besides, H2S was also employed as an oxygen switch to remodel oxygen metabolism by inhibiting cytochrome c oxidase activity in a high-Z metal ion-sensitized radiotherapy (54).
One important reason for tumor hypoxia is the abnormal blood vessel. To obtain nutrients for growth and to metastasize, tumor blood vessels are leaky, tortuous, and saccular (63). Thus, Tumor vascular normalization is a promising method to increase blood perfusion and relieve tumor hypoxia. Wang et al. modified Au NPs with 8-hydroxyquinoline (HQ) to obtain AuHQ, which attenuated the expression of angiopoietin-2, vascular endothelial growth factor 2 (56). Moreover, AuHQ treatment increased pericyte coverage and modulated tumor leakage, which led to increased blood perfusion. Tumor vascular normalization not only alleviated tumor hypoxia but also contributed to an increased AuHQ accumulation. Ultimately, compared to Au NPs, the anti-tumor efficacy of radiotherapy was increased by 38% in the AuHQ group.
Apart from chemical strategies, generating O2 in situ could be achieved by biological strategies via a natural photosynthetic system (64, 65). Qiao et al. engineered RBCM to modify the algal surface and deliver this RBCM-Algae to the tumor to increase tumor oxygenation (58). With red light-induced photosynthesis, RBCM-Algae generated O2 in situ and alleviated tumor hypoxia, and further led to re-oxygenated radiotherapy. Cyanobacteria were also utilized for continuous photosynthetic oxygen evolution in a two-dimensional bismuthene platform with high-Z components, which demonstrated the photosynthetic hypoxia-alleviation capability and radiosensitization performance (59). These works exemplified the construction of microorganism-enabled oxygen switches for radiosensitizer-augmented radiotherapy.
Given the central role of tumor hypoxia in the treatment resistance to radiotherapy, a various of oxygen switches were fabricated based on physical strategies, chemical strategies, and biological strategies. In this review, we summarized the oxygen switches designed for hypoxic-tumor radiotherapy. Physical oxygen switches served as a high-capacity carrier to deliver exogenous O2. Chemical oxygen switches triggered an in-situ reaction to generate O2 in vivo. Biological oxygen switches reshaped the tumor microenvironment by regulating biological behavior or introducing microorganism-mediated photosynthesis. Over the past few years, prosperous designs gained much attention and gratifying results in pre-clinical experiments, but there remained several challenges to be addressed.
The first limitation refers to the efficacy of these oxygen switches. As known, the hypoxic tumor area is quite complex and exhibits steric heterogeneity. On the one hand, deepen understanding of the mechanism of tumor hypoxia and radiosensitization are necessary. On the other hand, oxygen switches have to improve the O2 concentration continuously and accurately. Bio-mimic encapsulation was employed in oxygen switches to avoid early leakage and immune clearance (20). Stimulus-response (near infrared-triggered, irradiation-triggered, focused ultrasound-triggered, etc.) oxygen switch helped to achieve spatiotemporal specificity oxygen generation (62). As the penetration depth of NIR was limited, irradiation-triggered or focused ultrasound-triggered oxygen switch was worth further exploration (66). Moreover, novel in-situ microorganism-mediated photosynthesis could generate O2 continuously. However, it is still a challenge to prolong the survival time of microorganisms.
Secondly, the biosafety and biocompatibility of these oxygen switches are of concern. Unexpected Hb exposure can cause severe side effects including blood clot formation, renal toxicity, and cardiovascular complications (67). The metal oxide may affect intracellular redox homeostasis and induce macromolecule dysfunction. Especially, CAT-mimic nano-enzyme enables activating the matrix metalloproteinases in the tumor tissue, which could lead to inflammation and even tumor metastasis (68). Introducing microorganism into a human may activate the unfavorable immune response and induces local microbiome disturbances (69).
Finally, the coordination of oxygen switches and radiotherapy should be strengthened. The pharmacokinetics of oxygen switches should be tailored to the conduction of radiotherapy. Since radiotherapy is an oxygen-consumed intervention, the amount of oxygen generated from the oxygen switches has to be precisely measured to keep the balance of oxygen concentration and reach the best outcome. Furthermore, the combination of other therapeutic interventions such as photodynamic therapy and RDT may improve the utilization efficiency of oxygen.
Researchers paid much effort to the approach of oxygen-enriched radiotherapy. Nowadays, novel technologies are paving the path to more precise clinical medicine. Single-cell sequencing helped us understand how hypoxia is shaped and the evolutionary landscapes of tumor genomics (70). Nano-robots can follow a specified route and directionality under control, thus leading to a more precise oxygen delivery (71). Genome editing may improve the safety and oxygen-generating capability of microorganisms-mediated photosynthesis (72).
To be concluded, from the first attempt to combat hypoxia for radiotherapy to enhancement, multiple strategies have been developed to increase available oxygen. Though different strategies work on different principles, the efficacy of these oxygen switches has been widely recognized. However, there remain some obstacles before clinical translation. With a more in-depth understanding of tumor hypoxia, we should believe that better radiosensitized oxygen switches will emerge.
XL, HW, ZL, and FT wrote the manuscript. SL, JW, and WG revised the manuscript. All authors contributed to the article and approved the submitted version.
The authors acknowledge the support from the National Natural Science Foundation of China (82172645), Natural Science Foundation of Jiangsu Province (BK20200052, BK20220472), Key project of Nanjing Health Commission (ZKX21013), Bethune Charitable Foundation (05002), and Clinical Trials from the Affiliated Drum Tower Hospital, Medical School of Nanjing University (2021-LCYJ-MS-09 and 2021-LCYJ-PY-17).
The authors declare that the research was conducted in the absence of any commercial or financial relationships that could be construed as a potential conflict of interest.
All claims expressed in this article are solely those of the authors and do not necessarily represent those of their affiliated organizations, or those of the publisher, the editors and the reviewers. Any product that may be evaluated in this article, or claim that may be made by its manufacturer, is not guaranteed or endorsed by the publisher.
PFC, perfluorocarbon; Hb. Perfluorocarbons; RBC, red blood cells; RBCM, red blood cell membrane; Hf, Hafnium; Ce6, chlorin e6; RDT, radiodynamic therapy; CAT, catalase; EMs, E. coli membrane vesicles; MnO2, manganese dioxide; NPs, nanoparticles; NO, nitric oxide; H2S, hydrogen sulfide; CO, carbon monoxide.
2. Barker HE, Paget JTE, Khan AA, Harrington KJ. The tumour microenvironment after radiotherapy: Mechanisms of resistance and recurrence. Nat Rev Cancer. Nat Publishing Group; (2015) 15:409–25. doi: 10.1038/nrc3958
3. Zai W, Kang L, Dong T, Wang H, Yin L, Gan S, et al. E. coli membrane vesicles as a catalase carrier for long-term tumor hypoxia relief to enhance radiotherapy. ACS Nano. (2021) 15:15381–94. doi: 10.1021/acsnano.1c07621
4. Moding EJ, Kastan MB, Kirsch DG. Strategies for optimizing the response of cancer and normal tissues to radiation. Nat Rev Drug Discovery Nat Publishing Group; (2013) 12:526–42. doi: 10.1038/nrd4003
5. Thews O, Vaupel P. Temporal changes in tumor oxygenation and perfusion upon normo- and hyperbaric inspiratory hyperoxia. Strahlentherapie und Onkol. (2016) 192:174–81. doi: 10.1007/s00066-015-0916-1
6. Henke M, Laszig R, Rübe C, Schäfer U, Haase KD, Schilcher B, et al. Erythropoietin to treat head and neck cancer patients with anaemia undergoing radiotherapy: Randomised, double-blind, placebo-controlled trial. Lancet (2003) 362:1255–60. doi: 10.1016/S0140-6736(03)14567-9
7. Arcasoy MO, Amin K, Chou SC, Haroon ZA, Varia M, Raleigh JA. Erythropoietin and erythropoietin receptor expression in head and neck cancer: Relationship to tumor hypoxia. Clin Cancer Res (2005) 11:20–7. doi: 10.1158/1078-0432.20.11.1
8. Yu M, Dai M, Liu Q, Xiu R. Oxygen carriers and cancer chemo- and radiotherapy sensitization: Bench to bedside and back. Cancer Treat Rev (2007) 33:757–61. doi: 10.1016/j.ctrv.2007.08.002
9. Crabtree HG, Cramer W. The action of radium on cancer cells. II. some factors determining the susceptibility of cancer cells to radium. Proc R Soc B Biol Sci (1933) 113:238–50.
10. Wang W, Wang X, Tao F, Hu K, Zhang J, Wu J, et al. Fluorinated hyaluronic acid encapsulated perfluorocarbon nanoparticles as tumor-targeted oxygen carriers to enhance radiotherapy.Macromol Biosci (2022) 21:1–12. doi: 10.1021/acs.molpharmaceut.2c00432
11. Dong Z, Wang C, Gong Y, Zhang Y, Fan Q, Hao Y, et al. Chemical modulation of glucose metabolism with a fluorinated CaCO3Nanoregulator can potentiate radiotherapy by programming antitumor immunity. ACS Nano (2022) 6:6–13. doi: 10.1021/acsnano.2c02688
12. Gao R, Gu Y, Yang Y, He Y, Huang W, Sun T, et al. Robust radiosensitization of hemoglobin-curcumin nanoparticles suppresses hypoxic hepatocellular carcinoma. J Nanobiotechnology. BioMed Central; (2022) 20:1–15. doi: 10.1186/s12951-022-01316-w
13. Duan Z, Luo Q, Gu L, Li X, Zhu H, Gu Z, et al. A co-delivery nanoplatform for a lignan-derived compound and perfluorocarbon tuning IL-25 secretion and the oxygen level in tumor microenvironments for meliorative tumor radiotherapy. Nanoscale. R Soc Chemistry; (2021) 13:13681–92. doi: 10.1039/D1NR03738B
14. Sang W, Xie L, Wang G, Li J, Zhang Z, Li B, et al. Oxygen-enriched metal-phenolic X-ray nanoprocessor for cancer radio-radiodynamic therapy in combination with checkpoint blockade immunotherapy. Adv Sci (2021) 8:1–15. doi: 10.1002/advs.202003338
15. Tang W, Yang Z, He L, Deng L, Fathi P, Zhu S, et al. A hybrid semiconducting organosilica-based O2 nanoeconomizer for on-demand synergistic photothermally boosted radiotherapy. Nat Commun Springer US; (2021) 12. doi: 10.1038/s41467-020-20860-3
16. Xia D, Hang D, Li Y, Jiang W, Zhu J, Ding Y, et al. Au-hemoglobin loaded platelet alleviating tumor hypoxia and enhancing the radiotherapy effect with low-dose x-ray. ACS Nano. (2020) 14:15654–68. doi: 10.1021/acsnano.0c06541
17. Zhou Z, Zhang B, Wang H, Yuan A, Hu Y, Wu J. Two-stage oxygen delivery for enhanced radiotherapy by perfluorocarbon nanoparticles. Theranostics (2018) 8:4898–911. doi: 10.7150/thno.27598
18. Gao M, Liang C, Song X, Chen Q, Jin Q, Wang C, et al. Erythrocyte-membrane-enveloped perfluorocarbon as nanoscale artificial red blood cells to relieve tumor hypoxia and enhance cancer radiotherapy. Adv Mater (2017) 29:1–7. doi: 10.1002/adma.201701429
19. Wang X, Niu X, Sha W, Feng X, Yu L, Zhang Z, et al. An oxidation responsive nano-radiosensitizer increases radiotherapy e ffi cacy by remolding. R Soc Chem (2021) 8:6308–24. doi: 10.1002/advs.202003338
20. Yu P, Han X, Yin L, Hui K, Guo Y, Yuan A, et al. Artificial red blood cells constructed by replacing heme with perfluorodecalin for hypoxia-induced radioresistance. Adv Ther (2019) 2:1–7. doi: 10.1002/adtp.201900031
21. Jin Z, Zhao Q, Yuan S, Jiang W, Hu Y. Strategies of alleviating tumor hypoxia and enhancing tumor therapeutic effect by macromolecular nanomaterials. Macromol Biosci (2021) 21:1–12. doi: 10.1002/mabi.202100092
22. Cheng Y, Cheng H, Jiang C, Qiu X, Wang K, Huan W, et al. Perfluorocarbon nanoparticles enhance reactive oxygen levels and tumour growth inhibition in photodynamic therapy. Nat Commun Nat Publishing Group; (2015) 6:6–13. doi: 10.1038/ncomms9785
23. Meng L, Cheng Y, Tong X, Gan S, Ding Y, Zhang Y, et al. Tumor oxygenation and hypoxia inducible factor-1 functional inhibition via a reactive oxygen species responsive nanoplatform for enhancing radiation therapy and abscopal effects. ACS Nano. (2018) 12:8308–22. doi: 10.1021/acsnano.8b03590
24. Li X, Wang H, Li Z, Li D, Lu X, Ai S, et al. Oxygen tank for synergistic hypoxia relief to enhance mitochondria-targeted photodynamic therapy. Biomater Res BioMed Central; (2022) 26:1–17. doi: 10.1186/s40824-022-00296-0
25. Lavey RS. Clinical trial experience using erythropoietin during radiation therapy. Strahlenther Onkol (1998) 61(18). doi: 10.1002/anie.202200830
26. Hu B, Xiao X, Chen P, Qian J, Yuan G, Ye Y, et al. Enhancing anti-tumor effect of ultrasensitive bimetallic RuCu nanoparticles as radiosensitizers with dual enzyme-like activities. Biomaterials. Elsevier Ltd; (2022) 290:121811. doi: 10.1016/j.biomaterials.2022.121811
27. Liu X, Kifle MT, Xie H, Xu L, Luo M, Li Y, et al. Biomineralized manganese oxide nanoparticles synergistically relieve tumor hypoxia and activate immune response with radiotherapy in non-small cell lung cancer. Nanomaterials (2022) 12:4365–79. doi: 10.3390/nano12183138
28. Zhang C, Tu W, Chen X, Xu B, Li X, Hu C, et al. Intelligent design of polymer nanogels for full-process sensitized radiotherapy and dual-mode computed tomography/magnetic resonance imaging of tumors. Theranostics (2022) 12:3420–37. doi: 10.7150/thno.70346
29. Ni J, Xu H, Zhong Y, Zhou Y, Hu S. Activatable UCL/CT/MR-enhanced: In vivo imaging-guided radiotherapy and photothermal therapy. J Mater Chem B R Soc Chemistry; (2022) 10:549–61. doi: 10.1039/D1TB02006D
30. Wang A, Fang J, Ye S, Mao Q, Zhao Y, Cui C, et al. Assembly transformation jointly driven by the LAP enzyme and GSH boosting theranostic capability for effective tumor therapy. ACS Appl Mater Interfaces. (2021) 13:59787–802. doi: 10.1021/acsami.1c21062
31. Wang Z, Zeng W, Chen Z, Suo W, Quan H, Tan ZJ. An intratumoral injectable nanozyme hydrogel for hypoxia-resistant thermoradiotherapy. Colloids Surfaces B Biointerfaces. Elsevier B.V. (2021) 207:112026. doi: 10.1016/j.colsurfb.2021.112026
32. Li S, Sun W, Luo Y, Gao Y, Jiang X, Yuan C, et al. Hollow PtCo alloy nanospheres as a high-zand oxygen generating nanozyme for radiotherapy enhancement in non-small cell lung cancer. J Mater Chem B R Soc Chemistry; (2021) 9:4643–53. doi: 10.1039/D1TB00486G
33. Wang M, Li H, Huang B, Chen S, Cui R, Sun ZJ, et al. An ultra-stable, oxygen-supply nanoprobe emitting in near-Infrared-II window to guide and enhance radiotherapy by promoting anti-tumor immunity. Adv Healthc Mater (2021) 10:65–84. doi: 10.1002/adhm.202100090
34. He Z, Yan H, Zeng W, Yang K, Rong P. Tumor microenvironment-responsive multifunctional nanoplatform based on MnFe2O4-PEG for enhanced magnetic resonance imaging-guided hypoxic cancer radiotherapy. J Mater Chem B R Soc Chemistry; (2021) 9:1625–37. doi: 10.1039/D0TB02631J
35. Zhang J, Liu Y, Wang X, Du J, Song K, Li B, et al. Nanozyme-incorporated biodegradable bismuth mesoporous radiosensitizer for tumor microenvironment-modulated hypoxic tumor thermoradiotherapy. ACS Appl Mater Interfaces. (2020) 12:57768–81. doi: 10.1021/acsami.0c18853
36. Lin T, Zhang Q, Yuan A, Wang B, Zhang F, Ding Y, et al. Synergy of tumor microenvironment remodeling and autophagy inhibition to sensitize radiation for bladder cancer treatment. Theranostics (2020) 10:7683–96. doi: 10.7150/thno.45358
37. Zhu D, Lyu M, Jiang W, Suo M, Huang Q, Li K. A biomimetic nanozyme/camptothecin hybrid system for synergistically enhanced radiotherapy. J Mater Chem B R Soc Chemistry; (2020) 8:5312–9. doi: 10.1039/D0TB00676A
38. Chen Z, Guo W, Wu Q, Tan L, Ma T, Fu C, et al. Tumor reoxygenation for enhanced combination of radiation therapy and microwave thermal therapy using oxygen generation in situ by CuO nanosuperparticles under microwave irradiation. Theranostics (2020) 10:4659–75. doi: 10.7150/thno.42818
39. Jiang W, Han X, Zhang T, Xie D, Zhang H, Hu Y. An oxygen self-evolving, multistage delivery system for deeply located hypoxic tumor treatment. Adv Healthc Mater (2020) 9:13884–99. doi: 10.1002/adhm.201901303
40. Yang Y, Chen M, Wang B, Wang P, Liu Y, Zhao Y, et al. NIR-II driven plasmon-enhanced catalysis for a timely supply of oxygen to overcome hypoxia-induced radiotherapy tolerance. Angew Chemie - Int Ed (2019) 58:15069–75. doi: 10.1002/anie.201906758
41. Wang Y, Song S, Lu T, Cheng Y, Song Y, Wang S, et al. Oxygen-supplementing mesoporous polydopamine nanosponges with WS2 QDs-embedded for CT/MSOT/MR imaging and thermoradiotherapy of hypoxic cancer. Biomaterials. Elsevier; (2019) 220:119405. doi: 10.1016/j.biomaterials.2019.119405
42. Li Y, Yun KH, Lee H, Goh SH, Suh YG, Choi Y. Porous platinum nanoparticles as a high-z and oxygen generating nanozyme for enhanced radiotherapy in vivo. Biomaterials. Elsevier; (2019) 197:12–9. doi: 10.1016/j.biomaterials.2019.01.004
43. Zhang C, Yan L, Wang X, Dong X, Zhou R, Gu Z, et al. Tumor microenvironment-responsive Cu 2 (OH)PO 4 nanocrystals for selective and controllable radiosentization via the X-ray-Triggered fenton-like reaction. Nano Lett (2019) 19:1749–57. doi: 10.1021/acs.nanolett.8b04763
44. Yao X, Lu S, Feng C, Suo R, Li H, Zhang Y, et al. Tumor oxygenation nanoliposome synergistic hypoxia-inducible-factor-1 inhibitor enhanced iodine-125 seed brachytherapy for esophageal cancer. Biomaterials (2022) 289:121801. doi: 10.1016/j.biomaterials.2022.121801
45. Gong L, Zhang Y, Zhao J, Zhang Y, Tu K, Jiao L, et al. All-In-One biomimetic nanoplatform based on hollow polydopamine nanoparticles for synergistically enhanced radiotherapy of colon cancer. Small (2022) 18. doi: 10.3390/nano12183138
46. He Z, Huang X, Wang C, Li X, Liu Y, Zhou Z, et al. A catalase-like metal-organic framework nanohybrid for O2-evolving synergistic chemoradiotherapy. Angew Chemie - Int Ed (2019) 58:8752–6. doi: 10.1002/anie.201902612
47. Chao Y, Xu L, Liang C, Feng L, Xu J, Dong Z, et al. Combined local immunostimulatory radioisotope therapy and systemic immune checkpoint blockade imparts potent antitumour responses. Nat BioMed Eng. Springer US; (2018) 2:611–21. doi: 10.1038/s41551-018-0262-6
48. Song X, Xu J, Liang C, Chao Y, Jin Q, Wang C, et al. Self-supplied tumor oxygenation through separated liposomal delivery of H2O2 and catalase for enhanced radio-immunotherapy of cancer. Nano Lett (2018) 18:6360–8. doi: 10.1021/acs.nanolett.8b02720
49. Hua Y, Huang JH, Shao ZH, Luo XM, Wang ZY, Liu JQ, et al. Composition-dependent enzyme mimicking activity and radiosensitizing effect of bimetallic clusters to modulate tumor hypoxia for enhanced cancer therapy. Adv Mater (2022) 34:1–9. doi: 10.1002/adma.202203734
50. Zhou X, You M, Wang F, Wang Z, Gao X, Jing C, et al. Multifunctional graphdiyne–cerium oxide nanozymes facilitate MicroRNA delivery and attenuate tumor hypoxia for highly efficient radiotherapy of esophageal cancer. Adv Mater (2021) 33:1–10. doi: 10.1002/adma.202100556
51. Chen Q, Chen J, Yang Z, Xu J, Xu L, Liang C, et al. Nanoparticle-enhanced radiotherapy to trigger robust cancer immunotherapy. Adv Mater (2019) 31:1–12. doi: 10.1002/adma.201802228
52. Zhang Z, Wang L, Ding Y, Wu J, Hu Y, Yuan A. Synergy of hypoxia relief and chromatin remodeling to overcome tumor radiation resistance. Biomater Sci R Soc Chemistry; (2020) 8:4739–49. doi: 10.1039/D0BM00119H
53. Chen Y, Liu S, Gao P, Wei Pan MS, Li N, Tang B. NIR-II light-assisted radiotherapy based on ultrasmall HfO2-embedded porous carbon nanooctahedra for overcoming tumor radioresistance. Mater Today Nano. (2022) 20:100253. doi: 10.1016/j.mtnano.2022.100253
54. Li J, Xie L, Sang W, Li W, Wang G, Yan J, et al. A metal-phenolic nanosensitizer performs hydrogen sulfide-reprogrammed oxygen metabolism for cancer radiotherapy intensification and immunogenicity. Angew Chemie - Int Ed (2022) 61:5312–9. doi: 10.1039/d0tb00676a
55. Duo Y, Liu Q, Zhu D, Zhang B, Luo G, Wang FB, et al. Proof of concept for dual anticancer effects by a novel nanomaterial-mediated cancer cell killing and nano-radiosensitization. Chem Eng J Elsevier B.V. (2022) 429:132328. doi: 10.1016/j.cej.2021.132328
56. Wang X, Niu X, Zhang X, Zhang Z, Gao X, Wang W, et al. Construction of an AuHQ nano-sensitizer for enhanced radiotherapy efficacy through remolding tumor vasculature. J Mater Chem B R Soc Chemistry; (2021) 9:4365–79. doi: 10.1039/D1TB00515D
57. Yang C, Mu G, Zhang Y, Gao Y, Zhang W, Liu J, et al. Supramolecular nitric oxide depot for hypoxic tumor vessel normalization and radiosensitization. Adv Mater (2022) 34. doi: 10.1002/adma.202202625
58. Qiao Y, Yang F, Xie T, Du Z, Zhong D, Qi Y, et al. Engineered algae: A novel oxygen-generating system for effective treatment of hypoxic cancer. Sci Adv (2020) 6:12–9. doi: 10.1126/sciadv.aba5996
59. Chai R, Yu L, Dong C, Yin Y, Wang S, Chen Y, et al. Oxygen-evolving photosynthetic cyanobacteria for 2D bismuthene radiosensitizer-enhanced cancer radiotherapy. Bioact Mater KeAi Commun Co. Ltd; (2022) 17:276–88. doi: 10.1016/j.bioactmat.2022.01.014
60. Jiang J, Wang W, Zheng H, Chen X, Liu X, Xie Q, et al. Nano-enabled photosynthesis in tumours to activate lipid peroxidation for overcoming cancer resistances. Biomaterials. Elsevier Ltd; (2022) 285:121561. doi: 10.1016/j.biomaterials.2022.121561
61. Li W, Zhong D, Hua S, Du Z, Zhou M. Biomineralized biohybrid algae for tumor hypoxia modulation and cascade radio-photodynamic therapy. ACS Appl Mater Interfaces. (2020) 12:44541–53. doi: 10.1021/acsami.0c14400
62. Gao J, Wang Z, Guo Q, Tang H, Wang Z, Yang C, et al. Mitochondrion-targeted supramolecular “nano-boat” simultaneously inhibiting dual energy metabolism for tumor selective and synergistic chemo-radiotherapy. Theranostics (2022) 12:1286–302. doi: 10.7150/thno.67543
63. Jain RK. Normalization of tumor vasculature: An emerging concept in antiangiogenic therapy. Science (2005) 307:58–62. doi: 10.1126/science.1104819
64. Wang H, Guo Y, Gan S, Liu H, Chen Q, Yuan A, et al. Photosynthetic microorganisms-based biophotothermal therapy with enhanced immune response. Small (2021) 17:611–21. doi: 10.1002/smll.202007734
65. Wang H, Liu H, Guo Y, Zai W, Li X, Xiong W, et al. Photosynthetic microorganisms coupled photodynamic therapy for enhanced antitumor immune effect. Bioact Mater KeAi Commun Co. Ltd; (2022) 12:97–106. doi: 10.1016/j.bioactmat.2021.10.028
66. Li H, Kim Y, Jung H, Hyun JY, Shin I. Near-infrared (NIR) fluorescence-emitting small organic molecules for cancer imaging and therapy. Chem Soc Rev R Soc Chem (2022) 307:58–62. doi: 10.1039/D2CS00722C
67. Jansman MMT, Hosta-Rigau L. Recent and prominent examples of nano- and microarchitectures as hemoglobin-based oxygen carriers. Adv Colloid Interface Sci Elsevier B.V (2018) 31:65–84. doi: 10.1016/j.cis.2018.08.006
68. Nishikawa M, Hashida M, Takakura Y. Catalase delivery for inhibiting ROS-mediated tissue injury and tumor metastasis. Adv Drug Delivery Rev [Internet]. Elsevier B.V.; (2009) 61:319–26. doi: 10.1016/j.addr.2009.01.001
69. Wu L, Bao F, Li L, Yin X, Hua Z. Bacterially mediated drug delivery and therapeutics: Strategies and advancements. Adv Drug Delivery Rev Elsevier B.V. (2022) 187:114363. doi: 10.1016/j.addr.2022.114363
70. Bhandari V, Li CH, Bristow RG, Boutros PC. Divergent mutational processes distinguish hypoxic and normoxic tumours. Nat Commun Springer US; (2020) 11:1–10. doi: 10.1038/s41467-019-14052-x
71. Wang J, Soto F, Liu S, Yin Q, Purcell E, Zeng Y, et al. Volbots: Volvox microalgae-based robots for multimode precision imaging and therapy. Adv Funct Mater (2022) 34. doi: 10.1002/adfm.202201800
Keywords: radiotherapy, tumor hypoxia, oxygen carrier, in-situ oxygen generation, photosynthesis
Citation: Li X, Wang H, Li Z, Tao F, Wu J, Guan W and Liu S (2023) Oxygen switches: Refueling for cancer radiotherapy. Front. Oncol. 12:1085432. doi: 10.3389/fonc.2022.1085432
Received: 31 October 2022; Accepted: 28 December 2022;
Published: 16 February 2023.
Edited by:
Shuanghu Yuan, Shandong First Medical University and Shandong Academy of Medical Sciences, ChinaCopyright © 2023 Li, Wang, Li, Tao, Wu, Guan and Liu. This is an open-access article distributed under the terms of the Creative Commons Attribution License (CC BY). The use, distribution or reproduction in other forums is permitted, provided the original author(s) and the copyright owner(s) are credited and that the original publication in this journal is cited, in accordance with accepted academic practice. No use, distribution or reproduction is permitted which does not comply with these terms.
*Correspondence: Jinhui Wu, wuj@nju.edu.cn; Wenxian Guan, medguanwx@163.com; Song Liu, liusong@njglyy.com
†These authors have contributed equally to this work
Disclaimer: All claims expressed in this article are solely those of the authors and do not necessarily represent those of their affiliated organizations, or those of the publisher, the editors and the reviewers. Any product that may be evaluated in this article or claim that may be made by its manufacturer is not guaranteed or endorsed by the publisher.
Research integrity at Frontiers
Learn more about the work of our research integrity team to safeguard the quality of each article we publish.