- 1School of Basic Medicine, Health Science Center, Yangtze University, Jingzhou, Hubei, China
- 2Editorial Department of Journal of Hubei University of Science and Technology, Xianning, Hubei, China
- 3Department of Abdominal and Pelvic Medical Oncology, Huangshi Central Hospital, Affiliated Hospital of Hubei Polytechnic University, Edong Healthcare Group, Huangshi, Hubei, China
- 4Department of Clinical Laboratory, Huangshi Central Hospital, Affiliated Hospital of Hubei Polytechnic University, Edong Healthcare Group, Huangshi, Hubei, China
N6-methyladenosinen (m6A) methylation is a frequent RNA methylation modification that is regulated by three proteins: “writers”, “erasers”, and “readers”. The m6A modification regulates RNA stability and other mechanisms, including translation, cleavage, and degradation. Interestingly, recent research has linked m6A RNA modification to the occurrence and development of cancers, such as hepatocellular carcinoma and non-small cell lung cancer. This review summarizes the regulatory role of m6A RNA modification in gastric cancer (GC), including targets, the mechanisms of action, and the potential signaling pathways. Our present findings can facilitate our understanding of the significance of m6A RNA modification in GC.
1. Introduction
The most abundant form of RNA modification, N6-methyladenosinen (m6A) methylation, occurs at the N6-position of adenosine by recognizing the motif RRm6ACH ([G/A/U] [G/A]m6AC[U/A/C]) (1–3). The m6A modification regulates mRNA stability, translation, or degradation. m6A methylation in non-coding RNA (ncRNA), not only regulates the miRNA process but also affects the translation or degradation of lncRNA and circRNA (4–12). In addition to m6A modification, other frequent RNA modifications include pseudouridine (Ψ), N4-acetylcytidine (ac4C), N1-methyladenosine (m1A), 5-methylcytidine(m5C), and N7-methylguanosine (m7G) (1, 13, 14). Gene expression, biological rhythms, autophagy, apoptosis, and other biological processes are all regulated by RNA modification (3). RNA modification, like DNA methylation and histone modification, is also a type of epigenetic alteration. Recent studies have shown that epigenetics has a regulatory role in the development of Gastric cancer (GC) (15). GC is the fifth most common malignancy in humans and the third leading cause of cancer-related deaths. Clinically, the late diagnosis and poor prognosis in GC cause difficulties in the treatment. Therefore, it is very important to clarify the pathogenesis of GC. Recent studies have shown that m6A plays a critical role in GC formation. With the continuous emergence of m6A publications, there is an urgent need for a review to summarize its significance. However, we were unable to find relevant reviews in a large number of basic medical research (16–21). We will discuss the current regulatory mechanism of m6A methylation in the GC process, and propose the critical role of m6A modification in the diagnosis, treatment, and prognosis. This will provide us with a profound understanding of the m6A RNA modification as well as potential strategies for future clinical treatment in GC.
2. The m6A modification on RNA
2.1. The mechanism of m6A RNA modification on mRNA
2.1.1. Methylation modification of mRNA by “writers”
m6A RNA modification is a dynamic reversible chemical modification that is regulated by “writers”, “erasers”, and “readers” (Figure 1) (8). The core components of methyltransferase complex (MTC) include methyltransferase like 3 (METTL3), methyltransferase like 14 (METTL14), and Wilms tumor 1-associating protein (WTAP), which is the main factor of “writers” in inducing m6A methylation (9). In the process of MTC-induced mRNA methylation, METTL3 has an S-adenosyl methionine (SAM)-binding sequence. METTL14, as a structural support of RNA binding, binds H3K36me3 and enhances the catalytic ability of METTL3 (3, 4, 22–24). METTL3 and METTL14 form heterodimers that WTAP recruits into specific RNA sequences to methylate mRNA (25–27). Intriguingly, METTL3 acts as a “reader” to recruit eIF3, thereby promoting mRNA translation in the cytoplasm (28). Furthermore, the MTC contains RNA-binding motif protein 15 and 15B (RBM15/15B), Cbl proto-oncogene like 1 (CBLL1/HAKAI), zinc-finger CCCH domain-containing protein 13 (ZC3H13), methyltransferase like 5 (METTL5), tRNA methyltransferase activator subunit 11-2 (TRMT112), and KIAA1429 (25, 29–31). KIAA1429 is the most studied “writer” among them. KIAA1429, also known as vir-like m6A methyltransferase-associated protein (VIRMA), mediates m6A methylation preferentially at the 3’ UTR and near the stop codon (32, 33).
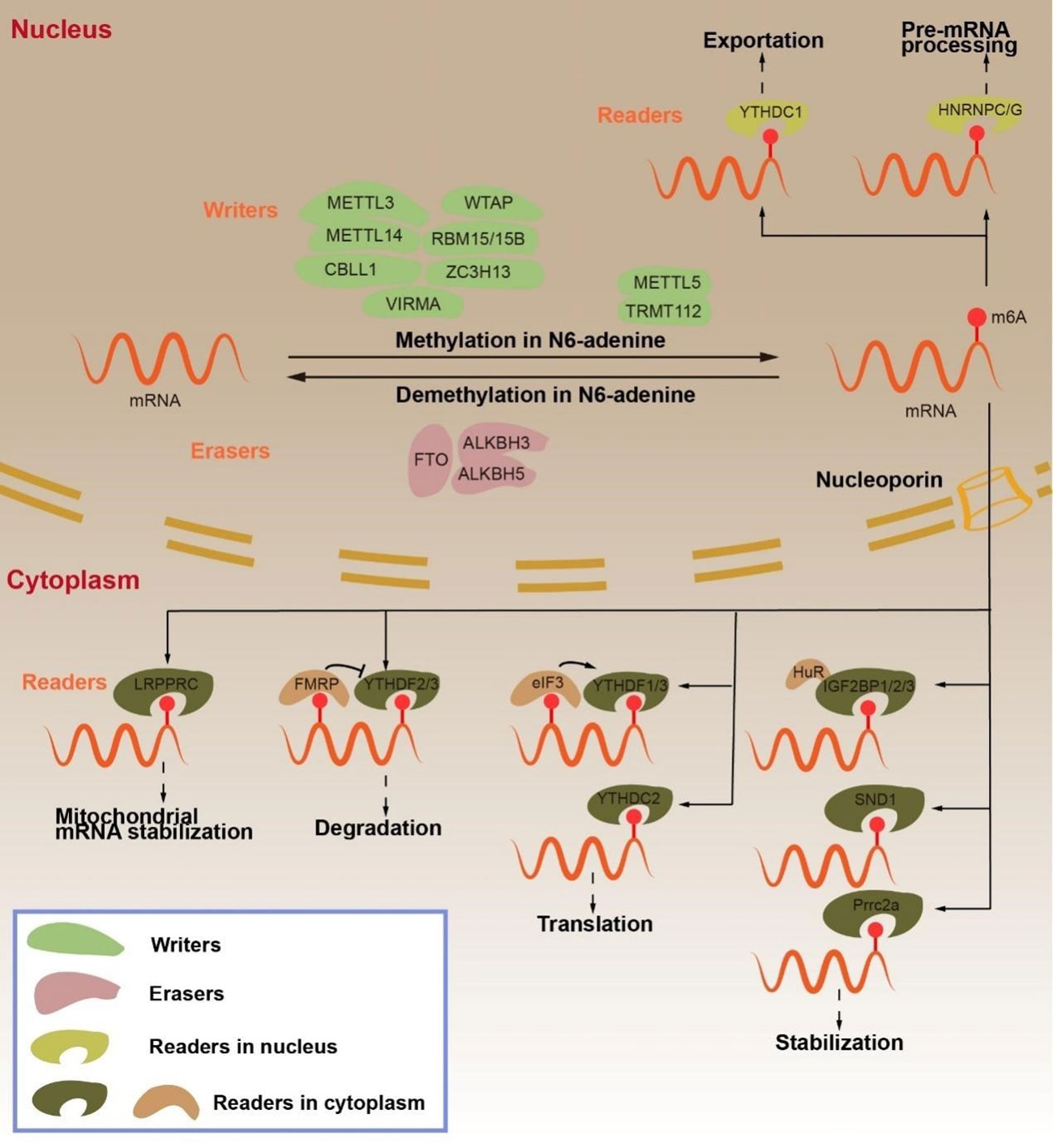
Figure 1 Regulation and function of m6A RNA modifications in mRNA by “writers”, “erasers” and “readers”. The m6A “writers”, including METTL3, METTL14, CBLL1, WTAP, RBM15/15B, ZC3H13 and VIRMA, cause mRNA methylation in N6-adenosine. The m6A “erasers”, including FTO, ALKBH3 and ALKBH5, lead to demethylation in N6-adenosine. The m6A “readers”, include YTHDC1, HNRNPC/G, LRPPRC, YTHDF2/3, FMRP, YTHDF1/3, YTHDC2, eIF3, IGF2BP1/2/3, SND1, Prrc2a. YTHDC1 transports mRNA from the nucleus to the cytoplasm. HNRNPC/G participates in pre-mRNA processing. LRPPRC maintains mitochondrial mRNA stabilization. YTHDF2/3 promotes mRNA degradation, but FMRP inhibits mRNA degradation. YTHDF1/3, eIF3 AND YTHDC2 promote mRNA translation. IGF2BP1/2/3, SND1, Prrc2a enhance mRNA stabilization.
2.1.2. Methylation modification of mRNA by “erasers”
In contrast, the role of “writers” and “erasers” remove m6A modifications from mRNA. Fat mass and obesity-associated (FTO) and AlkB homolog 5 (ALKBH5) are both Fe2+ and 2-oxoglutarate (2OG)-dependent AlkB dioxygenases that mediate demethylation by recognizing the methylation sites on m6A and subsequently regulating the mRNA function (34, 35). The difference between the two is that FTO oxidizes m6A to N6-hydroxymethyladenosine (hm6A) and N6-formyladenosine (f6A) and then hydrolyzes it to adenosine, while ALKBH5 locates the nuclear speck and directly eliminates mRNA m6A methylation on the mRNA (24, 30, 36). ALKBH3 is like a demethylase, but it is preferable to demethylate tRNA (3, 13). “Writers” and “erasers” interact to regulate the abundance of m6A, while “readers”—as special RNA-binding proteins—regulate the stability, splicing, nuclear output, translation, or degradation of m6A-modified mRNA (37, 38).
2.1.3. Methylation modification of mRNA by “readers”
“Readers” are mainly divided into three classes, YT521-B homology (YTH) domain family, heterogeneous nuclear ribonucleoproteins (hnRNPs), and insulin-like growth factor 2 mRNA-binding proteins (IGF2BPs). YTH domain family includes YTHDF1, YTHDF2, YTHDF3, YTHDC1, and YTHDC2 (10). YTHDF1 binds the eukaryotic initiation factor (eIF3) to the m6A site on mRNA and promotes mRNA translation. YTHDF2, on the contrary, recognizes m6A modification sites on the RNA 3’ UTR and recruits the CCR4-NOT deadenylase complex to promote mRNA degradation. YTHDC2 is also associated with RNA hydrolases to promote mRNA degradation (3, 24). YTHDF3 functions as an accelerator, promoting mRNA translation or degradation in collaboration with YTHDF1 or YTHDF2 (24, 34, 39–42). Intriguingly, fragile-X mental retardation protein (FMRP) has been shown to bind to YTHDF2, which inhibits the degradation of mRNA and promotes its stability (43). YTHDC1 binds the nuclear export protein serine/arginine splicing factor 3 (SRSF3) and inhibits SRSF10 in the nucleus, thereby facilitating mRNA splicing (37, 44). HNRNPs are a class of RNA-binding proteins that act as “m6A switches” to regulate RNA abundance. HNRNPA2B1 enhances the stability of m6A-modified mRNA, and both HNRNPC and HNRNPG can regulate pre-mRNA alternative splicing (23, 45–47). The IGF2BPs are the third type of “readers”. Unlike YTHDF2, IGF2BP1-3 promotes mRNA stability and translation by recruiting ELAV-like RNA-binding protein 1 (ELAVL1), which is also known as HuR (23, 38, 48). Furthermore, LRPPRC leucine-rich PPR-motif-containing protein (LRPPRC) can promote mRNA stability in the mitochondria, whereas Staphylococcal Nuclease and Tudor Domain Containing 1 (SND1) and Proline-Rich Coiled-Coil 2A (Prrc2a) such as IGF2BPs promote mRNA stability in m6A modification (24, 34, 49, 50).
2.2. m6A methylation interacts with ncRNA to regulate functions
In addition to mRNA, m6A modification modulated ncRNA splicing, maturation, and other processes (Figure 2) (6, 51).
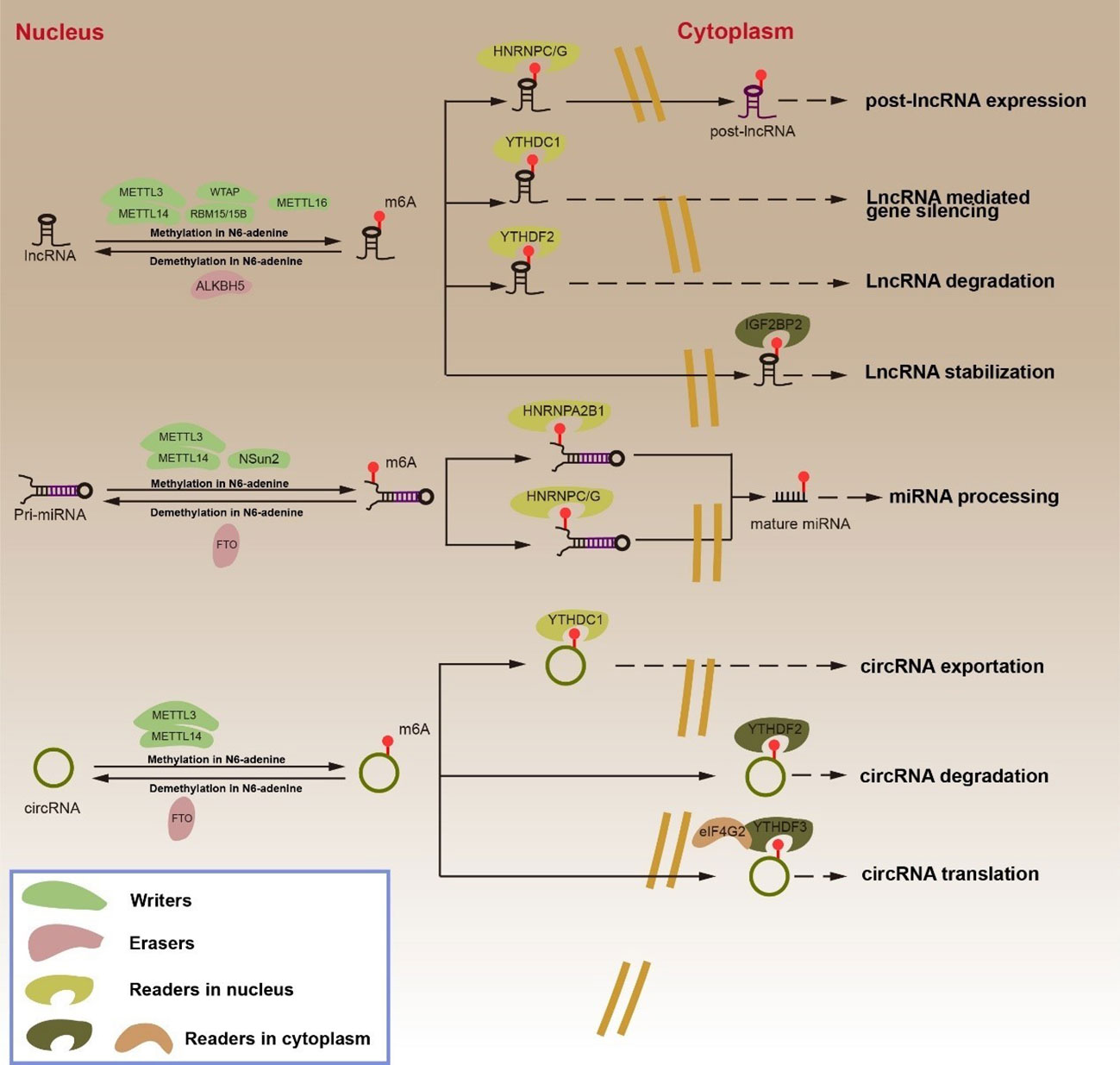
Figure 2 Regulation and function of m6A RNA modifications in ncRNA by “writers”, “erasers” and “readers”. The m6A “writers”, including METTL3, METTL14, WTAP, RBM15/15B and METTL16, cause lncRNA methylation in N6-adenosine. METTL3, METTL14 and NSun2 cause pri-miRNA methylation in N6-adenosine. METTL3 and METTL14 cause circRNA methylation in N6-adenosine. The m6A “erasers”, ALKBH5 lead to lncRNA demethylation in N6-adenosine. FTO result in pri-miRNA and circRNA demethylation in N6-adenosine. The m6A “readers”, HNRNPC/G promotes lncRNA expression. YTHDC1 mediates gene silencing. YTHDF2 leads to lncRNA degradation. IGF2BP2 enhances lncRNA stabilization. HNRNPA2B1 and HNPNPC/G participates in miRNA processing. YTHDC1 transports circRNA from the nucleus to the cytoplasm. YTHDF2 causes circRNA degradation. YTHDF2 and eIF4G2 facilitate circRNA translation.
2.2.1. Regulation of LncRNA by m6A modification
LncRNA can be categorized into 5 categories in accordance with the different positions of its transcription genes: internal lncRNA (lincRNA), internal lncRNA, sense lncRNA, antisense lncRNA, and bidirectional lncRNA. Although lncRNAs lack ORF and cannot be used as templates for protein synthesis, they do play an important regulatory role in RNA transcription and post-transcriptional modification (52, 53). Past studies have demonstrated that m6A modification can regulate the function of lncRNA. On one hand, m6A modification regulates the interaction between lncRNA and specific DNA sites via the RNA-DNA triple helix structure; on the other hand, m6A modification affects the binding of “readers” to lncRNA (54–56). YTHDC1 binds m6A methylated long-noncoding RNA X inactivity-specific transcripts (XIST) and promotes XIST-mediated gene silencing through the modification of WTAP and RBM15/15B (57, 58). LncRNA metastasis-associated lung adenocarcinoma transcript 1 (MALAT1) positively correlates with the methylation modification of METTL3/METTL14. The binding of “m6A switch” HNRNPC to MALAT1 reduced with reducing expression of METTL3 or METTL14. In addition, MALAT1’s binding ability to HNRNPC/G was significantly enhanced following m6A methylation at A2577 of MALAT1. Both HNRNPC and HNRNPG were found to be capable of regulating MALAT1 in alternative splicing and in promoting the expression of post-lncRNA (59–61). METTL16 is a methyltransferase of U6 spliceosome small nuclear RNA (snRNA), but it directly interacts with the MALAT1 triple helix to promote the cleavage of MALAT1 (62). m6A-modified lncRNAs play an important function in different cancers. ALKBH5, for example, promotes tumorigenesis in GC by reversing the methylation of nuclear para-peckle assembly transcript 1 (NEAT1) (63). YTHDF2 promotes the degradation of FOXF1-adjacent noncoding developmental regulatory RNA (FENDRR) in endometrioid endometrial carcinoma (EEC) (64). The co-action of METTL3 and METTL14 with m6A modification promotes the stability of lncRNA LNCAROD in head and neck squamous cell carcinoma (HNSCC). The lncRNA differentiation antagonizing nonprotein coding RNA (DANCR) is essential in cancer development. IGF2BP2 has been demonstrated to recognize the m6A modification site on lncRNA DANCR, which promotes its stability (65).
2.2.2. Regulation of miRNA by m6A modification
MiRNA is a single-stranded non-coding RNA of length 21–25 nucleotides. MiRNA can form the RNA-induced silencing complex (RISC), which binds the 3’ UTR of target mRNA, resulting in translational inhibition or degradation of mRNA (66–68). MiRNA processing can mainly be categorized into two phases. The initial step is for DGCR8 and DROSHA to splice pri-miRNA generated by miRNA gene transcription to form pre-miRNA. After the pre-miRNA is exported from the nucleus to the cytoplasm via export protein 5 (XPO5), DICER further processes the pre-miRNA into mature miRNA (66, 69–71). Intriguingly, the maturation of miRNAs relies on m6A modification (6). It has been reported that the maturation of pri-miRNAs is m6A-dependent (72). METTL3 is a common m6A regulatory protein that associates with DGCR8 in bladder cancer cells to promote the maturation of miR-221/222 (73). METTL3 also promotes the cleavage of pri-miRNA-let-7e and pri-miR-17-92 to form let-7e-5p and miR-18a-5p (74). METTL14 positively regulates the maturation of the tumor suppressors miR-126, miR-375, and miR-19a, as well as inhibiting invasion and migration in several cancers (75–77). METTL3 and METTL14 cooperate to promote m6A RNA modification, but they have been reported to demonstrate opposite effects on the occurrence of the same tumor. On one hand, although METTL3 and METTL14 form a complex to jointly promote m6A RNA modification, METTL3 or METTL14 in this complex can have their direct targets. This target can act as a tumor suppressor or an oncogene, and the abnormal expression of METTL3 or METTL14 biases them toward the regulation of a certain target, thereby exhibiting the opposite effect. In addition, the complex formed by METTL3 and METTL14 promotes m6A RNA modification, and “Readers” further process RNAs in which m6A occurs to determine the state of these RNAs. Therefore, in different types of same cancer, owing to the differences in the expression of different “Readers”, METTL3 or METTL14 can have opposite effects in the same cancer type. In fact, the current understanding of this mechanism is unclear, warranting further research. Similarly, the demethylase FTO may influence miRNA stability by inhibiting the expression of miR-181b-3p and miR-576 by interacting with DGCR8 (78, 79). Various “readers” proteins promote miRNA maturation by recognizing the m6A-modified sites in addition to “writers” in m6A -modified regulatory proteins. During pancreatic cancer progression, NKAP leads to the overmaturation of miR-25 under overexpressed METTL3 m6A modification, thereby inhibiting PHLPP2 and activating the AKT-p70S6K signaling to promote cancer progression (80). Moreover, HNRNPA2B1 can interact with DGCR8 and DROSHA to promote their binding to miRNAs (81–83). HNRNPC binds miR-21 directly to promote miRNA maturation (84). m6A modification, however, can also inhibit miRNA processing (6). Through methylation, NSun2 inhibits the splicing of pri-miR125b2 to pre-miR125b2, which reduces the miR-125 expression (85, 86).
2.2.3. Regulation of circRNAs by m6A modification
CircRNAs belongs to a class of circular non-coding RNAs with a closed circular structure. CircRNAs can be classified into the following 4 types: exonic circRNAs (ecircRNAs), intronic circRNAs (ciRNAs), exon-intron circRNAs (elciRNAs), and those formed by the circularization of viral RNA, tRNA, and shRNA (87). CircRNAs, as competitive endogenous RNAs (ceRNA), can sponge miRNA. CircRNA function can be influenced by m6A modification. Some circRNAs have protein-coding potential, while m6A modification can drive the translation process. Promoter eIF4G2 and YTHDF3, under the enhanced action of methyltransferase METTL3/14, start the translation of circRNA, while demethylase FTO inhibits the translation of circRNA (56, 88). CircCPSF6 is recognized and degraded by YTHDF2 during ALKBH5 demethylation (89). YTHDC1 has been reported to promote the nuclear output of circRNA NOP2/Sun RNA methyltransferase 2 (circNSUN2) (88).
3. The regulatory mechanism of m6A in GC
By regulating protein synthesis and changes in the signaling pathways, the m6A modification induces cancer progression, such as protective autophagy, metastasis, abnormal proliferation, EMT, drug resistance, and abnormal glycolysis in cancer cells. The abnormally expressed m6A regulators can be utilized as cancer therapeutic drug targets to expand cancer diagnosis and treatment options (3, 20, 90, 91). Table 1 summarizes the regulatory effects of m6A methylation on the common pathways to GC.
3.1. Regulation of m6A mRNA modification in GC
3.1.1. The m6A modification promotes oncogene expression and leads to abnormal proliferation in GC
Cancer progression is related to abnormal tumor proliferation, which is regulated by m6A modification. Ras is activated when a growth factor binds to the receptor, which in turn binds GTP to form Ras-GTP. The activated Ras affects multiple downstream proteins, including Ras-GTP, which then recruits and leads to Raf phosphorylation activation. The activated Raf kinase then binds to and activates the downstream MEK and ERK. After the phosphorylation-activated ERK is transferred from the cytoplasm to the nucleus, it can promote the phosphorylation activation of transcription factors such as c-Jun, MYC, and SP1, thereby inducing the expression of cell cycle and proliferation genes (Figure 3) (120, 121). BATF2 is a tumor suppressor gene in GC that inhibits the ERK phosphorylation by binding with P53, while METTL3 methylation inhibits the BATF2 expression (98, 122, 123). In addition, KIAA1429 directly targets and promotes the expression of c-Jun, facilitates the transcription of downstream MMP3, MMP9, and cyclin D1, and causes GC abnormal proliferation (Figure 3) (96, 98). Notably, METTL16 can promote the stability and high expression of cyclin D1 mRNA in this signaling pathway via m6A methylation, but whether cyclin D1 is the direct target of METTL16 requires further research (107). METTL3 promotes the MYC expression by m6A methylation and positively regulates the key components of the MYC pathway MCM5 and MCM6. Intriguingly, silencing HBXIP effectively suppressed the process, although further studies on the downstream targets of the HBXIP/METTL3/MYC axis are needed (1, 99). Similarly, the Wnt/β-catenin signaling pathway regulates abnormal GC proliferation (Figure 3) (124). YTHDF1 can promote the translation of FZD7, a key molecule of the Wnt signaling pathway. FZD7 binds to the Wnt protein on the cell membrane, which promotes downstream β-catenin to enter the nucleus from the cytoplasm. β-catenin is a multifunctional protein that can not only act as an adaptor protein in the cytoplasm to promote cell adhesion but also bind to the transcription factor TCF/LEF in the nucleus to promote the transcription of downstream target genes HMGA2, cyclin D, CDC25A, COX2, Sox9, and VEGFA (101, 125–127). Therefore, YTHDF1 overexpression significantly promotes the activation of the Wnt pathway (101). Interestingly, YAP/TAZ regulates β-catenin in the Wnt pathway. YAP/TAZ can form the β-catenin destruction complex in the cytoplasm, resulting in β-catenin degradation. In the nucleus, YAP binds β-catenin to form a complex that promotes the expression of downstream target genes (128–130). An active Hippo signaling pathway results in MST1/2 and SAV1 phosphorylation activation of LATS1/2. Activated LATS1/2 further phosphorylates YAP/TAZ, preventing it from entering the nucleus, and therefore blocking the regulation of downstream target genes expression when the transcriptional co-activators YAP/TAZ link to TEAD (Figure 3) (105, 110, 131–133). m6A methylation promotes abnormal proliferation in GC by regulating the expression of YAP in the Hippo signaling pathway. METTL3 induces methylation of YAP1 mRNA, and YTHDC2 recognizes m6A methylated YAP mRNA and promotes its translation (105, 110). The expression of METTL3 is positively correlated with the phosphorylation level of AKT. The downregulation of METTL3 resulted in low levels of p70S6K and cyclinD1, both downstream AKT (92). When the METTL3 is silenced, SOCS2 is unable to activate the JAK/STAT signal pathway, thereby inhibiting the proliferation, metastasis, and apoptosis in GC cells (97). Although YTHDF2 degradation of FOXC2 mRNA can inhibit the proliferation of abnormal GC, the upstream regulators in the YTHDF2/FOXC2 pathway have not been explored (111). m6A modification is implicated in several signaling pathways and mediates abnormal proliferation in GC. This finding suggests that m6A-modified mRNA in the regulation of abnormal proliferation is a potential therapeutic target with significant clinical implications.
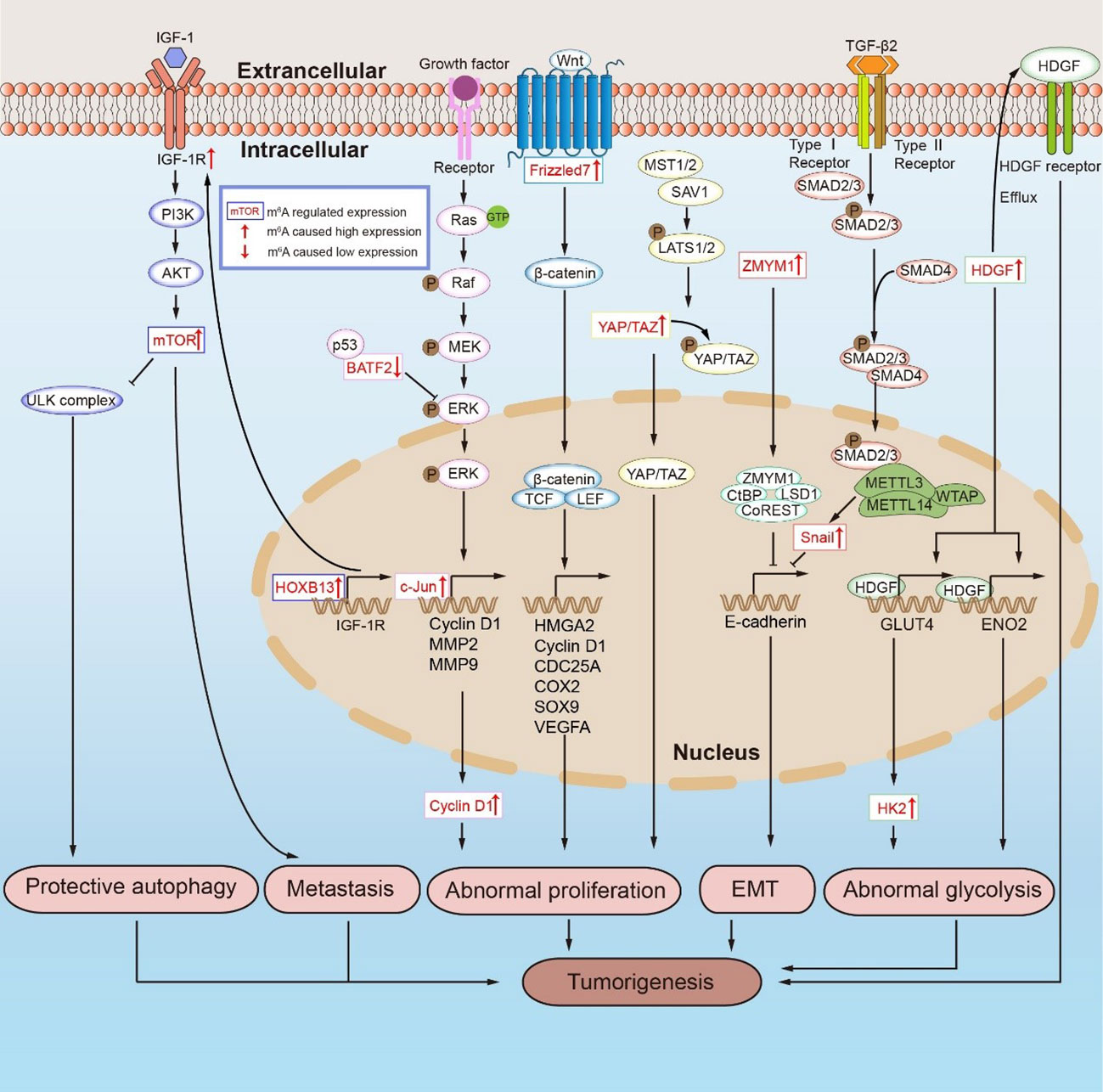
Figure 3 m6A RNA modifications mediate tumorigenesis in GC by regulating mRNA expression. PI3K/AKT/mTOR signaling pathway promotes protective autophagy and metastasis in GC. Ras/Raf/MAPK signaling pathway, Hippo signaling pathway and Wnt/β-catenin signaling pathway promote the abnormal proliferation of GC cells. The upregulation of ZMYM1 inhibits the expression of E-cadherin, which lead to the occurrence of EMT in GC. HDGF, including karyotype HDGF and secreted HDGF, cause abnormal glycolysis and tumorigenesis in GC.
3.1.2. m6A modification promotes the metastasis of GC
The progression of cancer is marked by distant metastasis of tumor cells. The PI3K/AKT/mTOR signaling pathway is often activated during tumorigenesis. This pathway not only regulates cell proliferation, apoptosis, autophagy, and other physiological processes but is also closely related to cancer cell metastasis (134, 135). METTL14 overexpression has been reported in studies to inhibit the PI3K/AKT/mTOR pathway activation and metastasis in GC (Figure 3) (100). METTL3 cooperates with YTHDF1 to promote SPHK2 mRNA translation, while m6A modification promotes GC metastasis via the METTL3/SPHK2/KLF2 axis, suggesting that the regulatory process is related to the poor prognosis in GC (106, 136). USP14 is a member of the USP ubiquitinase subfamily, and its expression in the GC tissues is higher than that in normal tissues. By knocking down USP14, it was found that the decreased expression of USP14 made GC cells sensitive to cisplatin by blocking the Akt or ERK signaling pathways. YTHDF1 could promote the translation of USP14 protein, which then promoted the tumorigenesis and metastasis of GC (109, 137). The PI3K/AKT/mTOR signaling pathway is activated in GC by HOXB13 overexpression, which is upregulated by FTO. In addition to “writers” and “readers”, the regulation of “erasers” in GC is equally significant. Past studies have shown that HOXB13 is highly expressed in GC cells due to increased demethylation of FTO and activation of the PI3K/AKT/mTOR signaling pathway (Figure 3). Further research revealed that HOXB13 enhanced the expression of IGF-1R by acting on the promoter region of IGF-1R. The specific mechanism of HOXB13 controlling the IGF-1R expression warrants further analysis, although it is suggested that the FTO/HOXB13/IGF-1R/PI3K/Akt/mTOR axis should be explored as a potential therapeutic target for GC (104). FTO induces ITGB1 mRNA hypomethylation and promotes its expression in the FTO/ITGB1/p-FAK axis. Since ITGFB1 is a key signaling molecule in the signal transduction between cells and the extracellular matrix, increased ITGFB1 expression promotes metastasis in GC (108, 138, 139). Although METTL3 mediates PI3K/AKT/mTOR pathway activation, its direct target remains unknown. Therefore, positively searching for the downstream effectors of m6A regulatory proteins in the PI3K/AKT/mTOR signaling pathway can facilitate a better understanding of the role of this pathway in GC metastasis.
3.1.3. m6A modification regulates the expression of key enzymes in the Warburg effect to promote abnormal glycolysis
Abnormal glycolysis is one of the hallmarks of further deterioration in GC. Glycolysis is an important energy source for GC deterioration in the metabolic process (140–143). Only HDGF was always regulated by METTL3 in four GC cell lines with an overexpression or knockout of METTL3, while the expression of GLUT4 and ENO2 increased correspondingly in GC cells with a high expression of METTL3 (Figure 3) (93). HDGF is essential in GC metastasis. The karyotype HDGF functions act as a transcriptional regulator to accelerate its expression in the promoter regions of GLUT4 and ENO2. The secreted HDGF promotes tumor angiogenesis by inducing VEGF (93, 144–146). The functional acetylation of H3K27me3 by p300 activates METTL3, which then promotes m6A methylation of HDGF mRNA. On the other hand, IGF2BP3 recognition of the m6A modification sites increases HDGF mRNA stability. HDGF promotes the expression of GLUT and ENO2 in the nucleus and initiates the glycolytic pathway in tumor cells (93). WTAP stabilized HK2 mRNA by binding to the 3’-UTR m6A site of HK2 mRNA and promoted its expression, making WTAP a novel target for GC therapy (21, 147). Moreover, ENO2 participates in the Warburg effect and together promotes aberrant glycolysis in GC under the regulation of m6A modification (93). We discovered that blocking tumorigenesis in the glycolysis process in GC can be employed as a new clinical treatment, and the proteins regulated by WTAP or those involved in m6A modification can be new drug targets.
3.1.4. m6A modification regulates E-cadherin protein expression to affect the EMT process in GC
In GC, EMT is the key step influencing distant metastasis (94). E-cadherin is a cell adhesion molecule that can promote adhesion between cells or between cells and the intercellular matrix. The emergence of EMT is marked by the deletion of the epithelial marker E-cadherin and the synthesis of the mesenchymal markers vimentin, N-cadherin, and smooth muscle actin (αSMA), which mark the occurrence of EMT (148). Past studies have demonstrated that METTL3 modifies ZMYM1 mRNA and promotes its high expression. ZMYM1 recruits the CtBP/LSD1/CoREST complex and inhibits the role of the E-cadherin promoter, resulting in EMT in GC (Figure 3) (94). Snail is a zinc finger protein that inhibits the expression of E-cadherin (149). After modifying the snail protein via the HOXA10/TGF-β/Smad/METTL3/snail signaling pathway, METTL3 inhibited the E-cadherin expression, thereby facilitating GC metastasis (Figure 3) (103). In another study, METTL14 and FTO knockdown activated the Wnt/PI3K-AKT signaling pathways (Figure 3) (95). Wnt/PI3K-AKT pathways promote EMT in GC by inhibiting the expression of E-cadherin (95, 150, 151). However, it remains unclear whether m6A modification inhibits E-cadherin expression through pathways besides the snail pathway and Wnt/PI3K-AKT signaling pathway toward promoting the EMT generation.
3.1.5. m6A modification promotes protective autophagy in GC by PI3K/AKT/mTOR axis
Autophagy is a double-edged sword in tumorigenesis. On one hand, autophagy inhibits tumor growth by eliminating harmful cellular components and preventing DNA damage. Autophagy, on the other hand, can provide nutrition for tumor cells while also promoting cancer progression (152). The protective autophagy of tumor cells diminishes their drug sensitivity, which contributes to cancer progression. Since the PI3K/AKT/mTOR pathway is involved in the mechanism of autophagy, targeted modulation of this pathway has become an important approach for cancer therapy (153). The FTO expression was negatively correlated with disease-free survival in GC, and it not only regulated HOXB13 in the PI3K/AKT/mTOR signaling pathway but was also related to the mTORC1 expression (Figure 3). When the ULK1 complex is activated, it directly phosphorylates Beclin-1 and ATG14L, thereby phosphorylating the downstream substrate VPS34 complex and promoting autophagy. mTORC1 functions in the general pathway by inhibiting the ULK1 complex, which suppresses cytoprotective autophagy and improves tumor drug sensitivity (102, 104, 152, 154–156). In the drug resistance study of GC cells, omeprazole inhibition of FTO could improve the inhibitory effect of 5-Fu, DDP, and TAX on GC cells as well as enhance the ability of mTORC1 to inhibit autophagy and improve the anti-tumor ability (102).
3.1.6. m6A RNA modification participates in GC immune infiltration and apoptosis
m6A modifications were involved in regulating other functions during GC progression. WTAP is an m6A-modified regulatory protein, and its high expression is closely related to m6A methylation. Interestingly, reduced WTAP expression has been linked to T lymphocyte infiltration (157). Apoptosis is a type of programmed cell death helpful to eliminate tumor cells. METTL3 reduces the expression of anti-apoptotic protein Bcl-2 through m6A methylation modification, while the pro-apoptotic protein Bax is relatively increased, which then activates Caspase-3 to promote the apoptotic pathway in GC (Figure 3) (92).
3.2. Modification of ncRNA by m6A in GC
3.2.1. Interaction between lncRNAs and m6A methylation during GC progression
During tumorigenesis, m6A methylation interacts with lncRNAs to regulate mRNA expression. Methyltransferase KIAA1429 inhibits the decay of lncRNA LINC00958, which can target GLUT1 to mediate aerobic glycolysis in GC. Therefore, KIAA1429 accelerates the glycolysis process by modifying LINC00958 with m6A methylation (116). ALKBH5 mediates lncNEAT1 demethylation, which affects the combination of lncNEAT1 and EZH2, thereby promoting GC development (63). METTL14 methylation enhanced LINC01320, which then absorbed miR-495-5p like a sponge. METTL3 promotes the RAB19 expression via the miR-495-5p/RAB19 axis, resulting in GC proliferation, migration, and invasion. However, the LINC01320/miR-495-5p/RAB19 axis in GC has not been thoroughly investigated, and this pathway warrants additional investigation (118). LncRNAs are not only modified by m6A, but they may also promote or inhibit m6A modification. As an oncogene, LINC00470 interacts with METTL3 and mediates the PTEN mRNA degradation in a YTHDF2-dependent manner (Figure 4) (158). LncRNA NRON combined with ALKBH5 mediates Nanog mRNA demethylation of Nanog mRNA and promotes Nanog stability during tumorigenesis (Figure 4) (115). LncRNA ARHGAP5-AS1 is also related to m6A regulatory protein. METTL3 combined with lncRNA ARHGAP5-AS1 to promote ARHGAP5 mRNA translation and induce protective autophagy in GC cells (Figure 4) (112). In general, a high expression of m6A-modified lncRNA has been closely related to poor prognosis for GC, although the specific mechanism of lncRNA and m6A modification remains unclear. This observation emphasizes the need for additional research on m6A-modified lncRNAs.
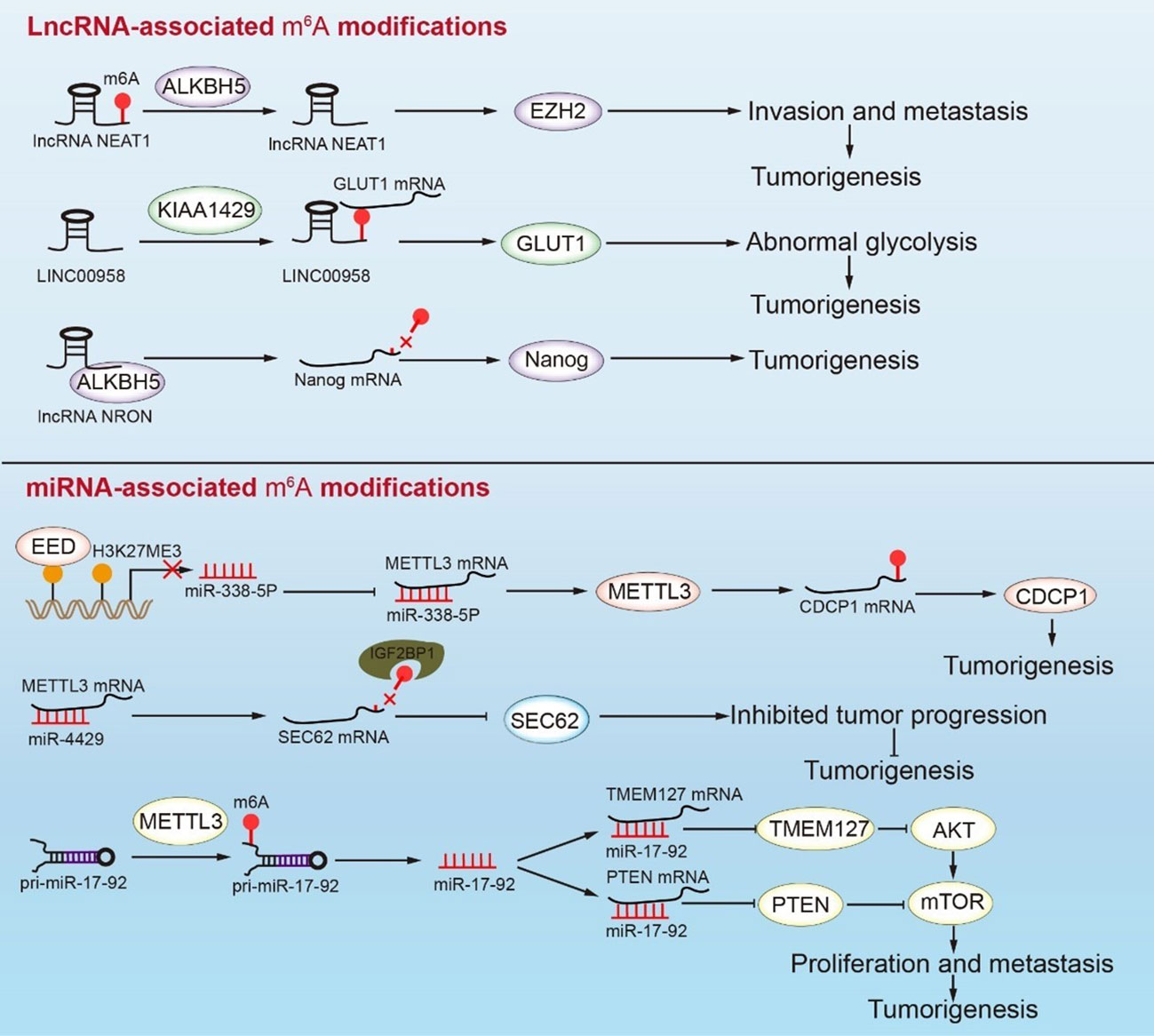
Figure 4 Interactions between m6A RNA modifications and ncRNAs mediate tumorigenesis in GC. The demethylation of NEAT1 enhances the expression of EZH2, which leads to invasion and metastasis in GC. KIAA1429 causes the methylation of LINC00958, which promotes GC abnormal glycolysis by facilitating the expression of GLUT1 mRNA. In addition, NRON binding ALKBH5 causes the demethylation of Nanog mRNA and enhances Nanog expression. The transcriptional repression of miR-338-5p rescues METTL3 mRNA, which enhances the expression of CDCP1 and leads to tumorigenesis. Conversely, miR-4429 inhibits the expression of METTL3, thus decreasing the methylation of SEC62 mRNA, which represses the SEC62 levels and inhibits tumor progression. METTL3 participates in miR-17-92 processing, which regulates the PI3K/AKT signaling pathway to promote the proliferation and metastasis of GC cells.
3.2.2. Interaction between m6A modification and miRNA
The interaction between miRNA and m6A-regulatory protein promotes proliferation, metastasis, and invasion in GC (Figure 4). Mature miR-17-92 inhibits the AKT/mTOR signaling pathway by affecting the expression of PTEN and TMEM127, which limits tumor proliferation and metastasis (114). METTL3 may promote pri-miR-17-92 processing through methylation, which is closely related to the poor prognosis in GC. The miR-660/E2F3 pathway is critical in the development of GC (119). He et al. reported that the m6A-modified tumor suppressor gene miR-660 could inhibit GC proliferation. Furthermore, miRNA is involved in the regulation of m6A-regulatory proteins. Both miR-338-5p and miR-4429 bind to METTL3 mRNA and inhibit its expression. SEC62 has a carcinogenic effect and is abundant in GC cells. It was discovered that METTL3 might interact with SEC62 mRNA via m6A modification, thereby allowing IGFBP1 to recognize and promote the stability of SEC62 mRNA. MiR-4429 inhibits the m6A methylation level of SEC62 mRNA by targeting METTL3, thus preventing GC progression (113). High EED expression in GC patients has been associated with poor prognosis. EED inhibited the splicing of METTL3 mRNA by downregulating the expression of miR-338-5p through H3K27me3. The increased METTL3 directly promoted the methylation modification and expression of CDCP1 mRNA, which, in turn, accelerated the occurrence in GC (117, 159). Some m6A regulatory proteins can precisely target specific miRNA sites, which can be employed as highly precise intervention targets. miRNAs bind to the mRNA of m6A regulatory proteins to regulate the m6A level of downstream signaling factors and provide a biomarker for future research.
3.2.3. Interaction between circRNAs and m6A methylation during GC progression
It is well known that circRNA usually acts as ceRNA to competitively inhibit miRNA function. In GC cells, miR-30c-2-3p can inhibit the proliferation and metastasis of GC. Knocking down MTEEL14 reduced the m6A methylation level of circORC5, and increased the expression of circORC5, thereby reducing the content of miR-30c-2-3p (160). From this, we can know that METTL14 regulates miR-30c-2-3p/AKT1S1 axis through methylation modification of circORC5, thereby inhibiting the deterioration of GC. However, research on the connection of circRNA and m6A regulated is still needed.
4. Future prospects
4.1. The role of m6A modification in diagnosis
At the molecular level, m6A modification affects the stability and degradation of RNA, thereby inducing changes in protein translation and regulating signal pathways. It has been reported that the m6A-regulatory protein with high specificity and sensitivity Our revised content of Q1(2) by reviewer 1is crucial in GC diagnosis. YTHDF1 is highly expressed in high-risk subtypes of GC patients. By constructing a diagnostic-m6A-score (DMS) and analyzing the ROC curve, DMS was found to demonstrate high specificity and sensitivity in the diagnosis of GC (area under the ROC curve [AUC] = 0.986). The AUC, sensitivity, and specificity of YTHDF1 were 0.52, 97.9, and 8.2%, respectively, in the TCGA cohort (161). Moreover, Kaplan–Meier survival analysis revealed that GC patients with a high YTHDF1 expression had a poor overall survival (OS) rate (P = 0.0352) and a higher risk of tumor recurrence. Therefore, detecting the level of m6A-methylated reading protein YTHDF1 in gastric cancer tissues after treatment can serve as an indicator to predict the prognosis of patients (162). Jing et al. (163) discovered that FTO was related to the tumor stage by analyzing the expression of the m6A-regulatory protein gene in the prognosis for GC by utilizing UALCAN and Oncomine web resources. Wang et al. (108) analyzed the prognostic value of 33 m6A RNA-methylation regulators in gastric cancer through univariate and multivariate Cox regression analyses. FTO was considered an independent risk factor for predicting the poor prognosis (HR = 1.60, P = 0.027, 95% CI: 1.10–2.50), with FTO serving as the key prognostic risk factor for OS of gastric cancer patients.
4.2. M6A modification and therapy
The regulation of m6A RNA modification on cancer progression is helpful for the development of targeted therapeutic drugs. Targeted drugs specifically interfere with the growth, proliferation, and diffusion of tumor cells by targeting specific proteins or genes. However, with the widespread use of targeted drugs, tumor cells gradually develop drug resistance (12). By regulating FOXO3-mediated autophagy, m6A methylation regulates the drug resistance to sorafenib in liver cancer (164). After autophagy damage caused by LncRNA ARHGAP5-AS1, METTL3 combined with LncRNA ARHGAP5-AS1 to promote the massive expression of ARHGAP5, resulting in GC drug resistance (112). Everolimus is an mTOR inhibitor that targets PTEN and TMEM127, and the resultant inhibitory effect is more pronounced in cancers with high METTL3 expression, albeit small molecule inhibitors of METTL3 are currently under investigation (114). Furthermore, the FTO inhibitors Rhein, MA2, and R-2HG all exhibit anticancer potential. Omeprazole can reverse the inhibitory effect of FTO on mTORC1 and its downstream signaling factor DDIT3, thereby promoting the regulation of autophagy levels (102). Past studies have demonstrated that the expression level of FTO in HER2-positive breast cancer tissues is higher than that in non-tumor tissues. Among them, FTO promotes the metastasis and invasion of cancer cells through the FTO/miR-181b-3p/ARL5B signaling pathway (78). In GC, FTO promotes GC cell migration and invasion by targeting HOXB13 and ITGB1. Therefore, it presents a new research direction in the area of GC treatment to detect whether FTO is related to the treatment of HER2-positive GC in the two signaling pathways of FTO-HOXB13-PI3K-AKT-mTOR and FTO-ITGB1-p-FAK (104, 108). Microsatellite instability (MSI) is a molecular indicator of defective DNA mismatch repair (MMR). Wang et al. (165) suggested that, in the interaction of m6A modification with lncRNA, the m6A methylation level is closely related to the MSI of GC. Among them, the prognostic effect of MSI-positive GC after chemotherapy was better, and the occurrence of high MSI-H was greater in a group with a high m6A methylation level. Therefore, the interaction between m6A modification and lncRNA is a novel research direction for the treatment of MSI-positive GC (166). When compared with chemotherapy and targeted therapy, immunotherapy not only improves the living environment of patients with advanced cancer but also avoids drug resistance (167). As a result, elucidating the regulatory role of m6A in the diagnosis, treatment, and prognosis of GC can enable GC patients to achieve a longer survival time. Immune cells in the tumor microenvironment (TME) play a key role in tumor progression, immune escape, and their impact on immunotherapeutic response. Immune checkpoint block (ICB) therapy eliminates cancer cells by targeting programmed death molecule 1 and its ligand (PD-1/PD-L1) in cytotoxic T cells and activating the immune system (168). M6A modification is related to PD1/PD-L1 immunotherapy. Through the construction of the m6A-scoring system, it was found that m6A methylation modification was significantly related to tumor response to PD-1/L1 immunotherapy (20). Overexpression of FTO promotes tumor cell growth, while knockdown of FTO significantly increases tumor cells’ response to INF-γ and anti PD-L1 treatment sensitivity. The possible mechanism is that FTO reduces the levels of m6A in PD-1 and prevents YTHDF2-mediated PD-1 RNA decay. In addition, in vitro experiments revealed that blocking YTHDF1 improved the therapeutic effect of anti-PD-L1 in GC cells, and the knockout of m6A demethylase AlKBH5 makes tumor sensitive to anti PD-1 immunotherapy and changes immune cell recruitment (167, 169). In order to improve the efficacy of immunotherapy, it is urgent to understand the tumor microenvironment (TME) and determine the mechanism behind the low response rate of ICB. Knocking down YTHDF1 in DC cells can enhance the cross presentation of tumor antigen and the cross start of CD8+T cells. In TME, with the increase of demethylase FTO and ALKBH5, the proportion of CD4+ positive T cells increased gradually. This observation demonstrated that m6A modification participates in the regulation of TME formation and plays an active role in tumor immunotherapy. However, in the study of the effect of m6A RNA modification on gastric cancer, due to the different m6A regulatory proteins, the effects are also different, albeit the specific regulatory targets required further research. At the epigenetic level, there is further scope for research on the generation, deterioration, or treatment of cancer.
Conclusion
m6A methylation, as an important post-transcriptional modification, has gradually become an important regulatory mechanism in the occurrence and development of cancer. Functionally, m6A RNA modification causes a change in the RNA state, which results in the abnormal expression of various proteins in cancer. We found that m6A modification promotes tumor progression by regulating the activation state of various signaling pathways. By modifying mRNA or interacting with ncRNA, m6A modification regulates the tumorigenesis process of abnormal proliferation, glycolysis, metastasis, invasion, EMT, and protective autophagy in the development of GC. This paper reviews the latest research progress on m6A methylation in GC, in which m6A-modified targets and their signal transduction factors were identified as potential biomarkers for GC diagnosis and prognosis or treatment targets, thereby providing ideas for future research on the regulation of m6A methylation on the tumorigenesis pathway and the development of GC-targeted therapeutic drugs. However, the regulatory pathways and targets of m6A modification in GC are insufficiently explored, warranting additional research.
Author contributions
GL, QF, CL, YP, JG, SL, YH, and HZ: contributed to this article with the design. GL, QF, and YP: literature search. GL, QF, CL, and YH: drafting. GL, QF, CL, YP, YH, and HZ: revision. GL, QF, CL, YP, YH, and HZ: editing. YH, and HZ: final approval. All authors contributed to the article and approved the submitted version.
Funding
Our work was supported by the Key Project of Joint Funds of Hubei Health and Family Planning Commission (Grant No. WJ2018H174).
Acknowledgments
All authors of this manuscript apologize to researchers whose works were not cited in this review article due to space limitations. The authors would like to thank all the reviewers who participated in the review and MJEditor (www.mjeditor.com) for its linguistic assistance during the preparation of this manuscript.
Conflict of interest
The authors declare that the research was conducted in the absence of any commercial or financial relationships that could be construed as a potential conflict of interest.
Publisher’s note
All claims expressed in this article are solely those of the authors and do not necessarily represent those of their affiliated organizations, or those of the publisher, the editors and the reviewers. Any product that may be evaluated in this article, or claim that may be made by its manufacturer, is not guaranteed or endorsed by the publisher.
References
1. Yang Z, Jiang X, Li D, Jiang X. HBXIP promotes gastric cancer via METTL3-mediated MYC mRNA m6A modification. Aging (Albany NY) (2020) 12(24):24967–82. doi: 10.18632/aging.103767
2. Desrosiers R, Friderici K, Rottman F. Identification of methylated nucleosides in messenger RNA from novikoff hepatoma cells. Proc Natl Acad Sci U.S.A. (1974) 71(10):3971–5. doi: 10.1073/pnas.71.10.3971
3. Sun T, Wu R, Ming L. The role of m6A RNA methylation in cancer. BioMed Pharmacother (2019) 112:108613. doi: 10.1016/j.biopha.2019.108613
4. Deng X, Yang Z, Jiang X, Li D, Jiang X. RNA N6-methyladenosine modification in cancers: current status and perspectives. Cell Res (2018) 28(5):507–17. doi: 10.1038/s41422-018-0034-6
5. Helm M, Motorin Y. Detecting RNA modifications in the epitranscriptome: predict and validate. Nat Rev Genet (2017) 18(5):275–91. doi: 10.1038/nrg.2016.169
6. Chen Y, Lin Y, Shu Y, He J, Gao W. Interaction between N(6)-methyladenosine (m(6)A) modification and noncoding RNAs in cancer. Mol Cancer (2020) 19(1):94. doi: 10.1186/s12943-020-01207-4
7. Shi H, Wang X, Lu Z, Zhao BS, Ma H, Hsu PJ, et al. YTHDF3 facilitates translation and decay of N6-methyladenosine-modified RNA. Cell Res (2017) 27(3):315–28. doi: 10.1038/cr.2017.15
8. Roundtree IA, Roundtree IA, Evans ME, Pan T, He C. Dynamic RNA modifications in gene expression regulation. Cell (2017) 169(7):1187–200. doi: 10.1016/j.cell.2017.05.045
9. Aguilo F, Zhang F, Sancho A, Fidalgo M, Di Cecilia S, Vashisht A, et al. Coordination of m(6)A mRNA methylation and gene transcription by ZFP217 regulates pluripotency and reprogramming. Cell Stem Cell (2015) 17(6):689–704. doi: 10.1016/j.stem.2015.09.005
10. Liu J, Li K, Cai J, Zhang M, Zhang X, Xiong X, et al. Landscape and regulation of m(6)A and m(6)Am methylome across human and mouse tissues. Mol Cell (2020) 77(2):426–440.e6. doi: 10.1016/j.molcel.2019.09.032
11. Cao G, Li HB, Yin Z, Flavell RA. Recent advances in dynamic m6A RNA modification. Open Biol (2016) 6(4):160003. doi: 10.1098/rsob.160003
12. Li B, Jiang J, Assaraf YG, Xiao H, Chen ZS, Huang C. Surmounting cancer drug resistance: New insights from the perspective of N(6)-methyladenosine RNA modification. Drug Resist Update (2020) 53:100720. doi: 10.1016/j.drup.2020.100720
13. Wiener D, Schwartz S. The epitranscriptome beyond m(6)A. Nat Rev Genet (2021) 22(2):119–31. doi: 10.1038/s41576-020-00295-8
14. Zhao BS, Roundtree IA, He C. Post-transcriptional gene regulation by mRNA modifications. Nat Rev Mol Cell Biol (2017) 18(1):31–42. doi: 10.1038/nrm.2016.132
15. Röcken C. Molecular classification of gastric cancer. Expert Rev Mol Diagn (2017) 17(3):293–301. doi: 10.1080/14737159.2017.1286985
16. Zhou Z, Lv J, Yu H, Han J, Yang X, Feng D, et al. Mechanism of RNA modification N6-methyladenosine in human cancer. Mol Cancer (2020) 19(1):104. doi: 10.1186/s12943-020-01216-3
17. Tedaldi G, Molinari C, São José C, Barbosa-Matos R, André A, Danesi R, et al. Genetic and epigenetic alterations of CDH1 regulatory regions in hereditary and sporadic gastric cancer. Pharm (Basel) (2021) 14(5):457. doi: 10.3390/ph14050457
18. Luo J, Liu H, Luan S, He C, Li Z. Aberrant regulation of mRNA m6A modification in cancer development. Int J Mol Sci (2018) 19(9):2515. doi: 10.3390/ijms19092515
19. Zhang H, Shi X, Huang T, Zhao X, Chen W, Gu N, et al. Dynamic landscape and evolution of m6A methylation in human. Nucleic Acids Res (2020) 48(11):6251–64. doi: 10.1093/nar/gkaa347
20. Zhang B, Wu Q, Li B, Wang D, Wang L, Zhou YL. m(6)A regulator-mediated methylation modification patterns and tumor microenvironment infiltration characterization in gastric cancer. Mol Cancer (2020) 19(1):53. doi: 10.1186/s12943-020-01170-0
21. Yu H, Zhao K, Zeng H, Li Z, Chen K, Zhang Z, et al. N(6)-methyladenosine (m(6)A) methyltransferase WTAP accelerates the warburg effect of gastric cancer through regulating HK2 stability. BioMed Pharmacother (2021) 133:111075. doi: 10.1016/j.biopha.2020.111075
22. Liu J, Yue Y, Han D, Wang X, Fu Y, Zhang L, et al. A METTL3-METTL14 complex mediates mammalian nuclear RNA N6-adenosine methylation. Nat Chem Biol (2014) 10(2):93–5. doi: 10.1038/nchembio.1432
23. Zhao Y, Shi Y, Shen H, Xie W. m(6)A-binding proteins: the emerging crucial performers in epigenetics. J Hematol Oncol (2020) 13(1):35. doi: 10.1186/s13045-020-00872-8
24. Chen M, Wong CM. The emerging roles of N6-methyladenosine (m6A) deregulation in liver carcinogenesis. Mol Cancer (2020) 19(1):44. doi: 10.1186/s12943-020-01172-y
25. Qu N, Bo X, Li B, Ma L, Wang F, Zheng Q, et al. Role of N6-methyladenosine (m(6)A) methylation regulators in hepatocellular carcinoma. Front Oncol (2021) 11:755206. doi: 10.3389/fonc.2021.755206
26. Wang X, Feng J, Xue Y, Guan Z, Zhang D, Liu Z, et al. Corrigendum: Structural basis of N(6)-adenosine methylation by the METTL3-METTL14 complex. Nature (2017) 542(7640):260. doi: 10.1038/nature21073
27. Ping XL, Sun BF, Wang L, Xiao W, Yang X, Wang WJ, et al. Mammalian WTAP is a regulatory subunit of the RNA N6-methyladenosine methyltransferase. Cell Res (2014) 24(2):177–89. doi: 10.1038/cr.2014.3
28. Lin S, Choe J, Du P, Triboulet R, Gregory RI. The m(6)A methyltransferase METTL3 promotes translation in human cancer cells. Mol Cell (2016) 62(3):335–45. doi: 10.1016/j.molcel.2016.03.021
29. Guo L, Yang H, Zhou C, Shi Y, Huang L, Zhang J, et al. N6-methyladenosine RNA modification in the tumor immune microenvironment: Novel implications for immunotherapy. Front Immunol (2021) 12:773570. doi: 10.3389/fimmu.2021.773570
30. Zhang W, Qian Y, Jia G. The detection and functions of RNA modification m(6)A based on m(6)A writers and erasers. J Biol Chem (2021) 297(2):100973. doi: 10.1016/j.jbc.2021.100973
31. Moindrot B, Cerase A, Coker H, Masui O, Grijzenhout A, Pintacuda G, et al. A pooled shRNA screen identifies Rbm15, spen, and wtap as factors required for xist RNA-mediated silencing. Cell Rep (2015) 12(4):562–72. doi: 10.1016/j.celrep.2015.06.053
32. Schwartz S, Mumbach MR, Jovanovic M, Wang T, Maciag K, Bushkin GG, et al. Perturbation of m6A writers reveals two distinct classes of mRNA methylation at internal and 5' sites. Cell Rep (2014) 8(1):284–96. doi: 10.1016/j.celrep.2014.05.048
33. Jia G, Fu Y, Zhao X, Dai Q, Zheng G, Yang Y, et al. N6-methyladenosine in nuclear RNA is a major substrate of the obesity-associated FTO. Nat Chem Biol (2011) 7(12):885–7. doi: 10.1038/nchembio.687
34. Huo FC, Zhu ZM, Pei DS. N(6) -methyladenosine (m(6) a) RNA modification in human cancer. Cell Prolif (2020) 53(11):e12921. doi: 10.1111/cpr.12921
35. Huang Y, Yan J, Li Q, Li J, Gong S, Zhou H, et al. Meclofenamic acid selectively inhibits FTO demethylation of m6A over ALKBH5. Nucleic Acids Res (2015) 43(1):373–84. doi: 10.1093/nar/gku1276
36. Song H, Feng X, Zhang H, Luo Y, Huang J, Lin M, et al. METTL3 and ALKBH5 oppositely regulate m(6)A modification of TFEB mRNA, which dictates the fate of hypoxia/reoxygenation-treated cardiomyocytes. Autophagy (2019) 15(8):1419–37. doi: 10.1080/15548627.2019.1586246
37. Roundtree IA, Luo GZ, Zhang Z, Wang X, Zhou T, Cui Y, et al. YTHDC1 mediates nuclear export of N(6)-methyladenosine methylated mRNAs. Elife (2017) 6:e31311. doi: 10.7554/eLife.31311
38. Huang H, Weng H, Sun W, Qin X, Shi H, Wu H, et al. Recognition of RNA N(6)-methyladenosine by IGF2BP proteins enhances mRNA stability and translation. Nat Cell Biol (2018) 20(3):285–95. doi: 10.1038/s41556-018-0045-z
39. Zhang N, Zuo Y, Peng Y, Zuo L. Function of N6-methyladenosine modification in tumors. J Oncol (2021) 2021:6461552. doi: 10.1155/2021/6461552
40. Liu S, Li G, Li Q, Zhang Q, Zhuo L, Chen X, et al. The roles and mechanisms of YTH domain-containing proteins in cancer development and progression. Am J Cancer Res (2020) 10(4):1068–84.
41. Hazra D, Chapat C, Graille M. m6A mRNA destiny: Chained to the rhYTHm by the YTH-containing proteins. Genes (Basel) (2019) 10(1):49. doi: 10.3390/genes10010049
42. Wu F, Cheng W, Zhao F, Tang M, Diao Y, Xu R. Association of N6-methyladenosine with viruses and virally induced diseases. Front Biosci (Landmark Ed) (2020) 25:1184–201. doi: 10.2741/4852
43. Edens BM, Vissers C, Su J, Arumugam S, Xu Z, Shi H, et al. FMRP modulates neural differentiation through m(6)A-dependent mRNA nuclear export. Cell Rep (2019) 28(4):845–854.e5. doi: 10.1016/j.celrep.2019.06.072
44. Xiao W, Adhikari S, Dahal U, Chen YS, Hao YJ, Sun BF, et al. Nuclear m(6)A reader YTHDC1 regulates mRNA splicing. Mol Cell (2016) 61(4):507–19. doi: 10.1016/j.molcel.2016.01.012
45. Liu N, Dai Q, Zheng G, He C, Parisien M, Pan T. N(6)-methyladenosine-dependent RNA structural switches regulate RNA-protein interactions. Nature (2015) 518(7540):560–4. doi: 10.1038/nature14234
46. Wang X, Zhao BS, Roundtree IA, Lu Z, Han D, Ma H, et al. N(6)-methyladenosine modulates messenger RNA translation efficiency. Cell (2015) 161(6):1388–99. doi: 10.1016/j.cell.2015.05.014
47. Jiang F, Tang X, Tang C, Hua Z, Ke M, Wang C, et al. HNRNPA2B1 promotes multiple myeloma progression by increasing AKT3 expression via m6A-dependent stabilization of ILF3 mRNA. J Hematol Oncol (2021) 14(1):54. doi: 10.1186/s13045-021-01066-6
48. Samuels TJ, Järvelin AI, Ish-Horowicz D, Davis I. Imp/IGF2BP levels modulate individual neural stem cell growth and division through myc mRNA stability. Elife (2020) 9:e51529. doi: 10.7554/eLife.51529
49. Zhou M, Liu W, Zhang J, Sun N. RNA m(6)A modification in immunocytes and DNA repair: The biological functions and prospects in clinical application. Front Cell Dev Biol (2021) 9:794754. doi: 10.3389/fcell.2021.794754
50. Qin S, Mao Y, Chen X, Xiao J, Qin Y, Zhao L. The functional roles, cross-talk and clinical implications of m6A modification and circRNA in hepatocellular carcinoma. Int J Biol Sci (2021) 17(12):3059–79. doi: 10.7150/ijbs.62767
51. Xiao S, Cao S, Huang Q, Xia L, Deng M, Yang M, et al. The RNA N(6)-methyladenosine modification landscape of human fetal tissues. Nat Cell Biol (2019) 21(5):651–61. doi: 10.1038/s41556-019-0315-4
52. Quinn JJ, Chang HY. Unique features of long non-coding RNA biogenesis and function. Nat Rev Genet (2016) 17(1):47–62. doi: 10.1038/nrg.2015.10
53. Wang H, Meng Q, Ma B. Characterization of the prognostic m6A-related lncRNA signature in gastric cancer. Front Oncol (2021) 11:630260. doi: 10.3389/fonc.2021.630260
54. Rinn JL, Chang HY. Genome regulation by long noncoding RNAs. Annu Rev Biochem (2012) 81:145–66. doi: 10.1146/annurev-biochem-051410-092902
55. Ma S, Chen C, Ji X, Liu J, Zhou Q, Wang G, et al. The interplay between m6A RNA methylation and noncoding RNA in cancer. J Hematol Oncol (2019) 12(1):121. doi: 10.1186/s13045-019-0805-7
56. Yi YC, Chen XY, Zhang J, Zhu JS. Novel insights into the interplay between m(6)A modification and noncoding RNAs in cancer. Mol Cancer (2020) 19(1):121. doi: 10.1186/s12943-020-01233-2
57. Patil DP, Chen CK, Pickering BF, Chow A, Jackson C, Guttman M, et al. m(6)A RNA methylation promotes XIST-mediated transcriptional repression. Nature (2016) 537(7620):369–73. doi: 10.1038/nature19342
58. Yang X, Zhang S, He C, Xue P, Zhang L, He Z, et al. METTL14 suppresses proliferation and metastasis of colorectal cancer by down-regulating oncogenic long non-coding RNA XIST. Mol Cancer (2020) 19(1):46. doi: 10.1186/s12943-020-1146-4
59. Liu P, Zhang B, Chen Z, He Y, Du Y, Liu Y, et al. m(6)A-induced lncRNA MALAT1 aggravates renal fibrogenesis in obstructive nephropathy through the miR-145/FAK pathway. Aging (Albany NY) (2020) 12(6):5280–99. doi: 10.18632/aging.102950
60. Liu N, Dai Q, Zheng G, He C, Parisien M, Pan T, et al. N6-methyladenosine-dependent RNA structural switches regulate RNA–protein interactions. Nature (2015) 518(7540):560–4. doi: 10.1038/nature14234
61. Zhou KI, Parisien M, Dai Q, Liu N, Diatchenko L, Sachleben JR, et al. N(6)-methyladenosine modification in a long noncoding RNA hairpin predisposes its conformation to protein binding. J Mol Biol (2016) 428(5 Pt A):822–33. doi: 10.1016/j.jmb.2015.08.021
62. Ruszkowska A. METTL16, methyltransferase-like protein 16: Current insights into structure and function. Int J Mol Sci (2021) 22(4):2176. doi: 10.3390/ijms22042176
63. Zhang J, Guo S, Piao HY, Wang Y, Wu Y, Meng XY, et al. ALKBH5 promotes invasion and metastasis of gastric cancer by decreasing methylation of the lncRNA NEAT1. J Physiol Biochem (2019) 75(3):379–89. doi: 10.1007/s13105-019-00690-8
64. Shen J, Feng XP, Hu RB, Wang H, Wang YL, Qian JH, et al. N-methyladenosine reader YTHDF2-mediated long noncoding RNA FENDRR degradation promotes cell proliferation in endometrioid endometrial carcinoma. Lab Invest (2021) 101(6):775–84. doi: 10.1038/s41374-021-00543-3
65. Ban Y, Tan P, Cai J, Li J, Hu M, Zhou Y, et al. LNCAROD is stabilized by m6A methylation and promotes cancer progression via forming a ternary complex with HSPA1A and YBX1 in head and neck squamous cell carcinoma. Mol Oncol (2020) 14(6):1282–96. doi: 10.1002/1878-0261.12676
66. Ali Syeda Z, Langden SSS, Munkhzul C, Lee M, Song SJ. Regulatory mechanism of MicroRNA expression in cancer. Int J Mol Sci (2020) 21(5):1723. doi: 10.3390/ijms21051723
67. O'Brien J, Hayder H, Zayed Y, Peng C. Overview of MicroRNA biogenesis, mechanisms of actions, and circulation. Front Endocrinol (Lausanne) (2018) 9:402. doi: 10.3389/fendo.2018.00402
68. Catalanotto C, Cogoni C, Zardo G. MicroRNA in control of gene expression: An overview of nuclear functions. Int J Mol Sci (2016) 17(10):1712. doi: 10.3390/ijms17101712
69. Lee Y, Ahn C, Han J, Choi H, Kim J, Yim J, et al. The nuclear RNase III drosha initiates microRNA processing. Nature (2003) 425(6956):415–9. doi: 10.1038/nature01957
70. Cui Y, Lyu X, Ding L, Ke L, Yang D, Pirouz M, et al. Global miRNA dosage control of embryonic germ layer specification. Nature (2021) 593(7860):602–6. doi: 10.1038/s41586-021-03524-0
71. Rice GM, Shivashankar V, Ma EJ, Baryza JL, Nutiu R. Functional atlas of primary miRNA maturation by the microprocessor. Mol Cell (2020) 80(5):892–902.e4. doi: 10.1016/j.molcel.2020.10.028
72. Alarcón CR, Lee H, Goodarzi H, Halberg N, Tavazoie SF. N6-methyladenosine marks primary microRNAs for processing. Nature (2015) 519(7544):482–5. doi: 10.1038/nature14281
73. Han J, Wang JZ, Yang X, Yu H, Zhou R, Lu HC, et al. METTL3 promote tumor proliferation of bladder cancer by accelerating pri-miR221/222 maturation in m6A-dependent manner. Mol Cancer (2019) 18(1):110. doi: 10.1186/s12943-019-1036-9
74. Chamorro-Jorganes A, Sweaad WK, Katare R, Besnier M, Anwar M, Beazley-Long N, et al. METTL3 regulates angiogenesis by modulating let-7e-5p and miRNA-18a-5p expression in endothelial cells. Arterioscler Thromb Vasc Biol (2021) 41(6):e325–37. doi: 10.1161/ATVBAHA.121.316180
75. Zhang BY, Han L, Tang YF, Zhang GX, Fan XL, Zhang JJ, et al. METTL14 regulates M6A methylation-modified primary miR-19a to promote cardiovascular endothelial cell proliferation and invasion. Eur Rev Med Pharmacol Sci (2020) 24(12):7015–23. doi: 10.26355/eurrev_202006_21694
76. Ma JZ, Yang F, Zhou CC, Liu F, Yuan JH, Wang F, et al. METTL14 suppresses the metastatic potential of hepatocellular carcinoma by modulating N(6) -methyladenosine-dependent primary MicroRNA processing. Hepatology (2017) 65(2):529–43. doi: 10.1002/hep.28885
77. Chen X, Xu M, Xu X, Zeng K, Liu X, Sun L, et al. METTL14 suppresses CRC progression via regulating N6-Methyladenosine-Dependent primary miR-375 processing. Mol Ther (2020) 28(2):599–612. doi: 10.1016/j.ymthe.2019.11.016
78. Xu Y, Ye S, Zhang N, Zheng S, Liu H, Zhou K, et al. The FTO/miR-181b-3p/ARL5B signaling pathway regulates cell migration and invasion in breast cancer. Cancer Commun (Lond) (2020) 40(10):484–500. doi: 10.1002/cac2.12075
79. Zhou G, Yan K, Liu J, Gao L, Jiang X, Fan Y. FTO promotes tumour proliferation in bladder cancer via the FTO/miR-576/CDK6 axis in an m6A-dependent manner. Cell Death Discovery (2021) 7(1):329. doi: 10.1038/s41420-021-00724-5
80. Zhang J, Bai R, Li M, Ye H, Wu C, Wang C, et al. Excessive miR-25-3p maturation via N(6)-methyladenosine stimulated by cigarette smoke promotes pancreatic cancer progression. Nat Commun (2019) 10(1):1858. doi: 10.1038/s41467-019-09712-x
81. Alarcón CR, Goodarzi H, Lee H, Liu X, Tavazoie S, Tavazoie SF. HNRNPA2B1 is a mediator of m(6)A-dependent nuclear RNA processing events. Cell (2015) 162(6):1299–308. doi: 10.1016/j.cell.2015.08.011
82. Pérez-Boza J, Boeckx A, Lion M, Dequiedt F, Struman I. hnRNPA2B1 inhibits the exosomal export of miR-503 in endothelial cells. Cell Mol Life Sci (2020) 77(21):4413–28. doi: 10.1007/s00018-019-03425-6
83. Li C, Qin F, Wang W, Ni Y, Gao M, Guo M, et al. hnRNPA2B1-mediated extracellular vesicles sorting of miR-122-5p potentially promotes lung cancer progression. Int J Mol Sci (2021) 22(23):12866. doi: 10.3390/ijms222312866
84. Park YM, Hwang SJ, Masuda K, Choi KM, Jeong MR, Nam DH, et al. Heterogeneous nuclear ribonucleoprotein C1/C2 controls the metastatic potential of glioblastoma by regulating PDCD4. Mol Cell Biol (2012) 32(20):4237–44. doi: 10.1128/MCB.00443-12
85. Yuan S, Tang H, Xing J, Fan X, Cai X, Li Q, et al. Methylation by NSun2 represses the levels and function of microRNA 125b. Mol Cell Biol (2014) 34(19):3630–41. doi: 10.1128/MCB.00243-14
86. Yang L, Ma Y, Han W, Li W, Cui L, Zhao X, et al. Proteinase-activated receptor 2 promotes cancer cell migration through RNA methylation-mediated repression of miR-125b. J Biol Chem (2015) 290(44):26627–37. doi: 10.1074/jbc.M115.667717
87. Chen I, Chen CY, Chuang TJ. Biogenesis, identification, and function of exonic circular RNAs. Wiley Interdiscip Rev RNA (2015) 6(5):563–79. doi: 10.1002/wrna.1294
88. Yang Y, Fan X, Mao M, Song X, Wu P, Zhang Y, et al. Extensive translation of circular RNAs driven by N(6)-methyladenosine. Cell Res (2017) 27(5):626–41. doi: 10.1038/cr.2017.31
89. Chen Y, Ling Z, Cai X, Xu Y, Lv Z, Man D, et al. Activation of YAP1 by N6-Methyladenosine-Modified circCPSF6 drives malignancy in hepatocellular carcinoma. Cancer Res (2022) 82(4):599–614. doi: 10.1158/0008-5472.CAN-21-1628
90. Deng LJ, Deng WQ, Fan SR, Chen MF, Qi M, Lyu WY, et al. m6A modification: recent advances, anticancer targeted drug discovery and beyond. Mol Cancer (2022) 21(1):52. doi: 10.1186/s12943-022-01510-2
91. Zhi Y, Zhang S, Zi M, Wang Y, Liu Y, Zhang M, et al. Potential applications of N(6) -methyladenosine modification in the prognosis and treatment of cancers via modulating apoptosis, autophagy, and ferroptosis. Wiley Interdiscip Rev RNA (2022) 13(5):e1719. doi: 10.1002/wrna.1719
92. Lin S, Liu J, Jiang W, Wang P, Sun C, Wang X, et al. METTL3 promotes the proliferation and mobility of gastric cancer cells. Open Med (Wars) (2019) 14:25–31. doi: 10.1515/med-2019-0005
93. Wang Q, Chen C, Ding Q, Zhao Y, Wang Z, Chen J, et al. METTL3-mediated m(6)A modification of HDGF mRNA promotes gastric cancer progression and has prognostic significance. Gut (2020) 69(7):1193–205. doi: 10.1136/gutjnl-2019-319639
94. Yue B, Song C, Yang L, Cui R, Cheng X, Zhang Z, et al. METTL3-mediated N6-methyladenosine modification is critical for epithelial-mesenchymal transition and metastasis of gastric cancer. Mol Cancer (2019) 18(1):142. doi: 10.1186/s12943-019-1065-4
95. Zhang C, Zhang M, Ge S, Huang W, Lin X, Gao J, et al. Reduced m6A modification predicts malignant phenotypes and augmented Wnt/PI3K-akt signaling in gastric cancer. Cancer Med (2019) 8(10):4766–81. doi: 10.1002/cam4.2360
96. Miao R, Dai CC, Mei L, Xu J, Sun SW, Xing YL, et al. KIAA1429 regulates cell proliferation by targeting c-jun messenger RNA directly in gastric cancer. J Cell Physiol (2020) 235(10):7420–32. doi: 10.1002/jcp.29645
97. Jiang L, Chen T, Xiong L, Xu JH, Gong AY, Dai B, et al. Knockdown of m6A methyltransferase METTL3 in gastric cancer cells results in suppression of cell proliferation. Oncol Lett (2020) 20(3):2191–8. doi: 10.3892/ol.2020.11794
98. Xie JW, Huang XB, Chen QY, Ma YB, Zhao YJ, Liu LC, et al. m(6)A modification-mediated BATF2 acts as a tumor suppressor in gastric cancer through inhibition of ERK signaling. Mol Cancer (2020) 19(1):114. doi: 10.1186/s12943-020-01223-4
99. Yang DD, Chen ZH, Yu K, Lu JH, Wu QN, Wang Y, et al. METTL3 promotes the progression of gastric cancer via targeting the MYC pathway. Front Oncol (2020) 10:115. doi: 10.3389/fonc.2020.00115
100. Liu X, Xiao M, Zhang L, Li L, Zhu G, Shen E, et al. The m6A methyltransferase METTL14 inhibits the proliferation, migration, and invasion of gastric cancer by regulating the PI3K/AKT/mTOR signaling pathway. J Clin Lab Anal (2021) 35(3):e23655. doi: 10.1002/jcla.23655
101. Pi J, Wang W, Ji M, Wang X, Wei X, Jin J, et al. YTHDF1 promotes gastric carcinogenesis by controlling translation of FZD7. Cancer Res (2021) 81(10):2651–65. doi: 10.1158/0008-5472.CAN-20-0066
102. Feng S, Qiu G, Yang L, Feng L, Fan X, Ren F, et al. Omeprazole improves chemosensitivity of gastric cancer cells by m6A demethylase FTO-mediated activation of mTORC1 and DDIT3 up-regulation. Biosci Rep (2021) 41(1):BSR20200842. doi: 10.1042/BSR20200842
103. Song C, Zhou C. HOXA10 mediates epithelial-mesenchymal transition to promote gastric cancer metastasis partly via modulation of TGFB2/Smad/METTL3 signaling axis. J Exp Clin Cancer Res (2021) 40(1):62. doi: 10.1186/s13046-021-01859-0
104. Guo C, Chu H, Gong Z, Zhang B, Li C, Chen J, et al. HOXB13 promotes gastric cancer cell migration and invasion via IGF-1R upregulation and subsequent activation of PI3K/AKT/mTOR signaling pathway. Life Sci (2021) 278:119522. doi: 10.1016/j.lfs.2021.119522
105. Zhou W, Xian Q, Wang Q, Wu C, Yan H, Li X, et al. m6A methyltransferase 3 promotes the proliferation and migration of gastric cancer cells through the m6A modification of YAP1. J Oncol (2021) 2021:8875424. doi: 10.1155/2021/8875424
106. Huo FC, Zhu ZM, Zhu WT, Du QY, Liang J, Mou J. METTL3-mediated m(6)A methylation of SPHK2 promotes gastric cancer progression by targeting KLF2. Oncogene (2021) 40(16):2968–81. doi: 10.1038/s41388-021-01753-1
107. Wang XK, Zhang YW, Wang CM, Li B, Zhang TZ, Zhou WJ, et al. METTL16 promotes cell proliferation by up-regulating cyclin D1 expression in gastric cancer. J Cell Mol Med (2021) 25(14):6602–17. doi: 10.1111/jcmm.16664
108. Wang D, Qu X, Lu W, Wang Y, Jin Y, Hou K, et al. N(6)-methyladenosine RNA demethylase FTO promotes gastric cancer metastasis by down-regulating the m6A methylation of ITGB1. Front Oncol (2021) 11:681280. doi: 10.3389/fonc.2021.681280
109. Chen XY, Liang R, Yi YC, Fan HN, Chen M, Zhang J, et al. The m(6)A reader YTHDF1 facilitates the tumorigenesis and metastasis of gastric cancer via USP14 translation in an m(6)A-dependent manner. Front Cell Dev Biol (2021) 9:647702. doi: 10.3389/fcell.2021.647702
110. Yuan W, Chen S, Li B, Han X, Meng B, Zou Y, et al. The N6-methyladenosine reader protein YTHDC2 promotes gastric cancer progression via enhancing YAP mRNA translation. Transl Oncol (2022) 16:101308. doi: 10.1016/j.tranon.2021.101308
111. Shen X, Zhao K, Xu L, Cheng G, Zhu J, Gan L, et al. YTHDF2 inhibits gastric cancer cell growth by regulating FOXC2 signaling pathway. Front Genet (2020) 11:592042. doi: 10.3389/fgene.2020.592042
112. Zhu L, Zhu Y, Han S, Chen M, Song P, Dai D, et al. Impaired autophagic degradation of lncRNA ARHGAP5-AS1 promotes chemoresistance in gastric cancer. Cell Death Dis (2019) 10(6):383. doi: 10.1038/s41419-019-1585-2
113. He H, Wu W, Sun Z, Chai L. MiR-4429 prevented gastric cancer progression through targeting METTL3 to inhibit m(6)A-caused stabilization of SEC62. Biochem Biophys Res Commun (2019) 517(4):581–7. doi: 10.1016/j.bbrc.2019.07.058
114. Sun Y, Li S, Yu W, Zhao Z, Gao J, Chen C, et al. N(6)-methyladenosine-dependent pri-miR-17-92 maturation suppresses PTEN/TMEM127 and promotes sensitivity to everolimus in gastric cancer. Cell Death Dis (2020) 11(10):836. doi: 10.1038/s41419-020-03049-w
115. Wang S, Wang Y, Zhang Z, Zhu C, Wang C, Yu F, et al. Long non-coding RNA NRON promotes tumor proliferation by regulating ALKBH5 and nanog in gastric cancer. J Cancer (2021) 12(22):6861–72. doi: 10.7150/jca.60737
116. Yang D, Chang S, Li F, Ma M, Yang J, Lv X, et al. m(6) a transferase KIAA1429-stabilized LINC00958 accelerates gastric cancer aerobic glycolysis through targeting GLUT1. IUBMB Life (2021) 73(11):1325–33. doi: 10.1002/iub.2545
117. Zhang F, Yan Y, Cao X, Zhang J, Li Y, Guo C. Methylation of microRNA-338-5p by EED promotes METTL3-mediated translation of oncogene CDCP1 in gastric cancer. Aging (Albany NY) (2021) 13(8):12224–38. doi: 10.18632/aging.103822
118. Hu N, Ji H. N6-methyladenosine (m6A)-mediated up-regulation of long noncoding RNA LINC01320 promotes the proliferation, migration, and invasion of gastric cancer via miR495-5p/RAB19 axis. Bioengineered (2021) 12(1):4081–91. doi: 10.1080/21655979.2021.1953210
119. He X, Shu Y. RNA N6-methyladenosine modification participates in miR-660/E2F3 axis-mediated inhibition of cell proliferation in gastric cancer. Pathol Res Pract (2019) 215(6):152393. doi: 10.1016/j.prp.2019.03.021
120. McCubrey JA, Steelman LS, Chappell WH, Abrams SL, Wong EW, Chang F, et al. Roles of the Raf/MEK/ERK pathway in cell growth, malignant transformation and drug resistance. Biochim Biophys Acta (2007) 1773(8):1263–84. doi: 10.1016/j.bbamcr.2006.10.001
121. Qian J, Kong X, Deng N, Tan P, Chen H, Wang J, et al. OCT1 is a determinant of synbindin-related ERK signalling with independent prognostic significance in gastric cancer. Gut (2015) 64(1):37–48. doi: 10.1136/gutjnl-2013-306584
122. Meng Q, Xia Y. C-jun, at the crossroad of the signaling network. Protein Cell (2011) 2(11):889–98. doi: 10.1007/s13238-011-1113-3
123. Lee H, Kim WJ, Kang HG, Jang JH, Choi IJ, Chun KH, et al. Upregulation of LAMB1 via ERK/c-jun axis promotes gastric cancer growth and motility. Int J Mol Sci (2021) 22(2):626. doi: 10.3390/ijms22020626
124. Koushyar S, Powell AG, Vincan E, Phesse TJ. Targeting wnt signaling for the treatment of gastric cancer. Int J Mol Sci (2020) 21(11):3927. doi: 10.3390/ijms21113927
125. Shang S, Hua F, Hu ZW. The regulation of β-catenin activity and function in cancer: therapeutic opportunities. Oncotarget (2017) 8(20):33972–89. doi: 10.18632/oncotarget.15687
126. Robinson KF, Narasipura SD, Wallace J, Ritz EM, Al-Harthi L. β-catenin and TCFs/LEF signaling discordantly regulate IL-6 expression in astrocytes. Cell Commun Signal (2020) 18(1):93. doi: 10.1186/s12964-020-00565-2
127. Nojima M, Suzuki H, Toyota M, Watanabe Y, Maruyama R, Sasaki S, et al. Frequent epigenetic inactivation of SFRP genes and constitutive activation of wnt signaling in gastric cancer. Oncogene (2007) 26(32):4699–713. doi: 10.1038/sj.onc.1210259
128. Azzolin L, Panciera T, Soligo S, Enzo E, Bicciato S, Dupont S, et al. YAP/TAZ incorporation in the β-catenin destruction complex orchestrates the wnt response. Cell (2014) 158(1):157–70. doi: 10.1016/j.cell.2014.06.013
129. Li N, Lu N, Xie C. The hippo and wnt signalling pathways: crosstalk during neoplastic progression in gastrointestinal tissue. FEBS J (2019) 286(19):3745–56. doi: 10.1111/febs.15017
130. Qiao Y, Li T, Zheng S, Wang H. The hippo pathway as a drug target in gastric cancer. Cancer Lett (2018) 420:14–25. doi: 10.1016/j.canlet.2018.01.062
131. Pocaterra A, Romani P, Dupont S. YAP/TAZ functions and their regulation at a glance. J Cell Sci (2020) 133(2):jcs230425. doi: 10.1242/jcs.230425
132. Ma S, Meng Z, Chen R, Guan KL. The hippo pathway: Biology and pathophysiology. Annu Rev Biochem (2019) 88:577–604. doi: 10.1146/annurev-biochem-013118-111829
133. Lee Y, Finch-Edmondson M, Cognart H, Zhu B, Song H, Low BC, et al. Common and unique transcription signatures of YAP and TAZ in gastric cancer cells. Cancers (Basel) (2020) 12(12):3667. doi: 10.3390/cancers12123667
134. Aoki M, Fujishita T. Oncogenic roles of the PI3K/AKT/mTOR axis. Curr Top Microbiol Immunol (2017) 407:153–89. doi: 10.1007/82_2017_6
135. Fattahi S, Amjadi-Moheb F, Tabaripour R, Ashrafi GH, Akhavan-Niaki H. PI3K/AKT/mTOR signaling in gastric cancer: Epigenetics and beyond. Life Sci (2020) 262:118513. doi: 10.1016/j.lfs.2020.118513
136. Jia Z, Tang X, Zhang X, Shen J, Sun Y, Qian L. miR-153-3p attenuates the development of gastric cancer by suppressing SphK2. Biochem Genet (2022) 60(5):1748–61. doi: 10.1007/s10528-021-10166-4
137. Fu Y, Ma G, Liu G, Li B, Li H, Hao X, et al. USP14 as a novel prognostic marker promotes cisplatin resistance via Akt/ERK signaling pathways in gastric cancer. Cancer Med (2018) 7(11):5577–88. doi: 10.1002/cam4.1770
138. Wang N, Chang LL. Maspin suppresses cell invasion and migration in gastric cancer through inhibiting EMT and angiogenesis via ITGB1/FAK pathway. Hum Cell (2020) 33(3):663–75. doi: 10.1007/s13577-020-00345-7
139. Zhang L, Yao L, Zhou W, Tian J, Ruan B, Lu Z, et al. miR-497 defect contributes to gastric cancer tumorigenesis and progression via regulating CDC42/ITGB1/FAK/PXN/AKT signaling. Mol Ther Nucleic Acids (2021) 25:567–77. doi: 10.1016/j.omtn.2021.07.025
140. Yang H, Li Y, Hu B. Potential role of mitochondria in gastric cancer detection: Fission and glycolysis. Oncol Lett (2021) 21(6):439. doi: 10.3892/ol.2021.12700
141. Zhang B, Wu J, Cai Y, Luo M, Wang B, Gu Y. AAED1 modulates proliferation and glycolysis in gastric cancer. Oncol Rep (2018) 40(2):1156–64. doi: 10.3892/or.2018.6478
142. Doherty JR, Cleveland JL. Targeting lactate metabolism for cancer therapeutics. J Clin Invest (2013) 123(9):3685–92. doi: 10.1172/JCI69741
143. Shao M, Zhang J, Zhang J, Shi H, Zhang Y, Ji R, et al. SALL4 promotes gastric cancer progression via hexokinase II mediated glycolysis. Cancer Cell Int (2020) 20:188. doi: 10.1186/s12935-020-01275-y
144. Kishima Y, Yamamoto H, Izumoto Y, Yoshida K, Enomoto H, Yamamoto M, et al. Hepatoma-derived growth factor stimulates cell growth after translocation to the nucleus by nuclear localization signals. J Biol Chem (2002) 277(12):10315–22. doi: 10.1074/jbc.M111122200
145. Chen W, Zhou Y, Wu G, Sun P. CCNI2 promotes the progression of human gastric cancer through HDGF. Cancer Cell Int (2021) 21(1):661. doi: 10.1186/s12935-021-02352-6
146. Lee KH, Choi EY, Kim MK, Lee SH, Jang BI, Kim TN, et al. Hepatoma-derived growth factor regulates the bad-mediated apoptotic pathway and induction of vascular endothelial growth factor in stomach cancer cells. Oncol Res (2010) 19(2):67–76. doi: 10.3727/096504010X12864748215043
147. Tan VP, Miyamoto S. HK2/hexokinase-II integrates glycolysis and autophagy to confer cellular protection. Autophagy (2015) 11(6):963–4. doi: 10.1080/15548627.2015.1042195
148. Serrano-Gomez SJ, Maziveyi M, Alahari SK. Regulation of epithelial-mesenchymal transition through epigenetic and post-translational modifications. Mol Cancer (2016) 15:18. doi: 10.1186/s12943-016-0502-x
149. Cano A, Pérez-Moreno MA, Rodrigo I, Locascio A, Blanco MJ, del Barrio MG, et al. The transcription factor snail controls epithelial-mesenchymal transitions by repressing e-cadherin expression. Nat Cell Biol (2000) 2(2):76–83. doi: 10.1038/35000025
150. Bougen-Zhukov N, Nouri Y, Godwin T, Taylor M, Hakkaart C, Single A, et al. Allosteric AKT inhibitors target synthetic lethal vulnerabilities in e-Cadherin-Deficient cells. Cancers (Basel) (2019) 11(9):1359. doi: 10.3390/cancers11091359
151. Wells A, Yates C, Shepard CR. E-cadherin as an indicator of mesenchymal to epithelial reverting transitions during the metastatic seeding of disseminated carcinomas. Clin Exp Metastasis (2008) 25(6):621–8. doi: 10.1007/s10585-008-9167-1
152. Li GM, Li L, Li MQ, Chen X, Su Q, Deng ZJ, et al. DAPK3 inhibits gastric cancer progression via activation of ULK1-dependent autophagy. Cell Death Differ (2021) 28(3):952–67. doi: 10.1038/s41418-020-00627-5
153. Li H, Zhao S, Shen L, Wang P, Liu S, Ma Y, et al. E2F2 inhibition induces autophagy via the PI3K/Akt/mTOR pathway in gastric cancer. Aging (Albany NY) (2021) 13(10):13626–43. doi: 10.18632/aging.202891
154. Saxton RA, Sabatini DM. mTOR signaling in growth, metabolism, and disease. Cell (2017) 168(6):960–76. doi: 10.1016/j.cell.2017.02.004
155. Hosokawa N, Hara T, Kaizuka T, Kishi C, Takamura A, Miura Y, et al. Nutrient-dependent mTORC1 association with the ULK1-Atg13-FIP200 complex required for autophagy. Mol Biol Cell (2009) 20(7):1981–91. doi: 10.1091/mbc.e08-12-1248
156. Lin X, Peng Z, Wang X, Zou J, Chen D, Chen Z, et al. Targeting autophagy potentiates antitumor activity of met-TKIs against met-amplified gastric cancer. Cell Death Dis (2019) 10(2):139. doi: 10.1038/s41419-019-1314-x
157. Li H, Su Q, Li B, Lan L, Wang C, Li W, et al. High expression of WTAP leads to poor prognosis of gastric cancer by influencing tumour-associated T lymphocyte infiltration. J Cell Mol Med (2020) 24(8):4452–65. doi: 10.1111/jcmm.15104
158. Yan J, Huang X, Zhang X, Chen Z, Ye C, Xiang W, et al. LncRNA LINC00470 promotes the degradation of PTEN mRNA to facilitate malignant behavior in gastric cancer cells. Biochem Biophys Res Commun (2020) 521(4):887–93. doi: 10.1016/j.bbrc.2019.11.016
159. Yang F, Jin H, Que B, Chao Y, Zhang H, Ying X, et al. Dynamic m(6)A mRNA methylation reveals the role of METTL3-m(6)A-CDCP1 signaling axis in chemical carcinogenesis. Oncogene (2019) 38(24):4755–72. doi: 10.1038/s41388-019-0755-0
160. Fan HN, Chen ZY, Chen XY, Chen M, Yi YC, Zhu JS, et al. METTL14-mediated m(6)A modification of circORC5 suppresses gastric cancer progression by regulating miR-30c-2-3p/AKT1S1 axis. Mol Cancer (2022) 21(1):51. doi: 10.1186/s12943-022-01521-z
161. Liu T, Yang S, Cheng YP, Kong XL, Du DD, Wang X, et al. The N6-methyladenosine (m6A) methylation gene YTHDF1 reveals a potential diagnostic role for gastric cancer. Cancer Manag Res (2020) 12:11953–64. doi: 10.2147/CMAR.S279370
162. Wu W, Zhang F, Zhao J, He P, Li Y. The N6-methyladenosine:mechanisms, diagnostic value, immunotherapy prospec-ts and challenges in gastric cancer. Exp Cell Res (2022) 415(2):113115. doi: 10.1016/j.yexcr.2022.113115
163. Jing JJ, Zhao X, Li H, Sun LP, Yuan Y. Expression profiles and prognostic roles of m6A writers, erasers and readers in gastric cancer. Future Oncol (2021) 17(20):2605–20. doi: 10.2217/fon-2020-0630
164. Lin Z, Niu Y, Wan A, Chen D, Liang H, Chen X, et al. RNA m(6) a methylation regulates sorafenib resistance in liver cancer through FOXO3-mediated autophagy. EMBO J (2020) 39(12):e103181. doi: 10.15252/embj.2019103181
165. Wang Y, Zhu GQ, Tian D, Zhou CW, Li N, Feng Y, et al. Comprehensive analysis of tumor immune microenvironment and prognosis of m6A-related lncRNAs in gastric cancer. BMC Cancer (2022) 22(1):316. doi: 10.1186/s12885-022-09377-8
166. Meijing Z, Tianhang L, Biao Y. N6-methyladenosine modification patterns and tumor microenvironment immune characteristics associated with clinical prognosis analysis in stomach adenocarcinoma. Front Cell Dev Biol (2022) 10:913307. doi: 10.3389/fcell.2022.913307
167. Xu Y, Huang C. Progress and application of epitranscriptomic m(6)A modification in gastric cancer. RNA Biol (2022) 19(1):885–96. doi: 10.1080/15476286.2022.2096793
168. Zhang X, Su H, Chen H, Li Q, Liu X, Zhang L, et al. RNA Modifications in gastrointestinal cancer: Current status and future perspectives. Biomedicines (2022) 10(8):1918. doi: 10.3390/biomedicines10081918
Keywords: m6A RNA modification, gastric cancer, mRNA, ncRNA, RNA methylation
Citation: Li G, Fu Q, Liu C, Peng Y, Gong J, Li S, Huang Y and Zhang H (2022) The regulatory role of N6-methyladenosine RNA modification in gastric cancer: Molecular mechanisms and potential therapeutic targets. Front. Oncol. 12:1074307. doi: 10.3389/fonc.2022.1074307
Received: 19 October 2022; Accepted: 16 November 2022;
Published: 06 December 2022.
Edited by:
Brian D. Adams, Brain Institute of America, United StatesReviewed by:
Subhayan Sur, Indian Institute of Science Education and Research Kolkata, IndiaChristos K. Kontos, National and Kapodistrian University of Athens, Greece
Costas Koufaris, University of Cyprus, Cyprus
Copyright © 2022 Li, Fu, Liu, Peng, Gong, Li, Huang and Zhang. This is an open-access article distributed under the terms of the Creative Commons Attribution License (CC BY). The use, distribution or reproduction in other forums is permitted, provided the original author(s) and the copyright owner(s) are credited and that the original publication in this journal is cited, in accordance with accepted academic practice. No use, distribution or reproduction is permitted which does not comply with these terms.
*Correspondence: Haiyuan Zhang, aHl6aGFuZ184OEAxNjMuY29t; Yan Huang, NjEwMTgwMjhAcXEuY29t
†These authors have contributed equally to this work