- 1Department of Medical Research, Department of Obstetrics and Gynecology, Department of Gastroenterology, and Chinese Medicine Research and Development Center, China Medical University Hospital, Taichung, Taiwan
- 2Graduate Institute of Biomedical Sciences, Center for Tumor Biology, School of Medicine, China Medical University, Taichung, Taiwan
- 3School of Chinese Medicine, China Medical University, Taichung, Taiwan
- 4Division of General Surgery, Department of Surgery, National Cheng Kung University Hospital, College of Medicine, National Cheng Kung University, Tainan, Taiwan
- 5Institute of Clinical Medicine, College of Medicine, National Chen Kung University, Tainan, Taiwan
- 6Department of Nursing, Asia University, Taichung, Taiwan
Pancreatic ductal adenocarcinoma (PDAC) is one of the most fatal types of solid tumors, associated with a high prevalence of cachexia (~80%). PDAC-derived cachexia (PDAC-CC) is a systemic disease involving the complex interplay between the tumor and multiple organs. The endocrine organ–like tumor (EOLT) hypothesis may explain the systemic crosstalk underlying the deleterious homeostatic shifts that occur in PDAC-CC. Several studies have reported a markedly heterogeneous collection of cachectic mediators, signaling mechanisms, and metabolic pathways, including exocrine pancreatic insufficiency, hormonal disturbance, pro-inflammatory cytokine storm, digestive and tumor-derived factors, and PDAC progression. The complexities of PDAC-CC necessitate a careful review of recent literature summarizing cachectic mediators, corresponding metabolic functions, and the collateral impacts on wasting organs. The EOLT hypothesis suggests that metabolites, genetic instability, and epigenetic changes (microRNAs) are involved in cachexia development. Both tumors and host tissues can secrete multiple cachectic factors (beyond only inflammatory mediators). Some regulatory molecules, metabolites, and microRNAs are tissue-specific, resulting in insufficient energy production to support tumor/cachexia development. Due to these complexities, changes in a single factor can trigger bi-directional feedback circuits that exacerbate PDAC and result in the development of irreversible cachexia. We provide an integrated review based on 267 papers and 20 clinical trials from PubMed and ClinicalTrials.gov database proposed under the EOLT hypothesis that may provide a fundamental understanding of cachexia development and response to current treatments.
1 Introduction
For Pancreatic ductal adenocarcinoma (PDAC) is currently the fourth most common cause of cancer-related deaths worldwide and is projected to become the second most common cause of cancer-related deaths by 2030 (1). Due to its aggressiveness and poor prognosis, mortality remains alarmingly high among patients diagnosed with PDAC. Approximately 80%–85% of PDAC patients are diagnosed at advanced stages with unresectable or metastatic tumors, resulting in a 5-year survival rate below 10% (2). During early-stage PDAC, surgical resection is currently the only curative option, although chemotherapy and radiation therapy are also used as primary treatment options, with or without surgery. However, single-agent chemotherapies are rarely effective in PDAC (3). In general, chemotherapy regimens are not universally effective in PDAC and are associated with significant adverse effects, including the development of PDAC-derived cachexia (PDAC-CC), and cachexia occurs in 32%~71% of patients within 12 to 48 weeks of chemotherapy initiation (4).
Patients with PDAC experience a high prevalence (up to 80%) of cachexia, often with early onset (45% of PDAC patients present with cachexia at the time of diagnosis (5)), which may account for up to 30% of mortality (6). Cachexia is defined as the progressive loss of muscle mass and function (6, 7) and is a catabolic multi-organ syndrome characterized by non-volitional weight loss (muscle or adipocyte loss), adipopenia, fatigue, weakness, loss of appetite, and early satiety (8, 9). When muscle mass loss, it enhances chemo-toxicities and insensitivities, contributing to poor overall survival (10).
In general, tumors demand a high energy supply and can promote the wasting of peripheral tissues via hyper-catabolism. Tumors compete with other organs/tissues for energy and nutrients, resulting in elevated resting energy expenditure and inducing a negative energy balance. Energy utilization in tumors also results in increased proteolysis and lipolysis combined with decreased lipogenesis and protein synthesis (8, 9, 11, 12). These metabolic reprogramming effects, combined with poor appetite, lead to rapid weight loss among PDAC patients and can contribute to deterioration in the overall quality of life (QoL) and overall survival (OS) (7, 13–15). The complex, multifactorial nature of the metabolic disruptions in cachexia makes effective treatment challenging. The current lack of consensus regarding how to define cachexia and a scarcity of strong evidence produced by robust, rigorous, and mechanistic studies have limited the development of effective treatments (16). In addition, most cachexia studies focus on symptoms associated with individual organs (such as tumor, muscle, or adipocyte tissues) without considering consider systemic interactions. In this review, we provide an up-to-date overview of current cachexia research in PDAC to provide insight regarding the cachexia mediators that act in different organs and explore whether the endocrine organ–like tumor (EOLT) hypothesis of PDAC can explain the development of systemic complications.
2 Cachexia criteria and stages
Cancer-derived cachexia (CC) is a multifactorial syndrome involving various metabolic changes in several tissues and organs (8, 9, 12, 17–19). Although patients with pancreatic cancer show a wide range of nutritional alterations, the primary symptom is progressive weight loss due to the loss of skeletal muscle mass, with or without the accompanying depletion of adipose tissue (6, 19–23). Other PDAC-CC-related clinical manifestations include inflammation (24–26), anorexia (27, 28) and metabolic reprogramming (9, 29, 30) etc.,
Numerous studies also focus on exploring new PDAC-CC cachectic mediators, corresponding metabolic functions, and the collateral impacts on wasting organs. A systematic review also suggested a network of cytokines (interleukin [IL]-6, tumor necrosis factor-alpha [TNFα], and IL-8) that may be associated with cachexia development (31). Sah et al. (19) suggested that PDAC-CC can be categorized by three distinct metabolic phases: Phase 1 represents the earliest metabolic change, characterized by new-onset hyperglycemia; Phase 2 is associated with a greater than 5% reduction in body weight with pre-cachectic symptoms (appetite loss and impaired glucose metabolism), suggesting the initiation of cachexia; and Phase 3 is associated with dramatic reductions in all monitored metabolites, lipids, subcutaneous fat, and muscle, except fasting glucose.
Traditionally, a Body Mass Index (BMI) < 18.5 kg/m2 was accepted as a marker of being cachectic. However, sarcopenic obesity can be observed in CC, suggesting that weight loss might not be a defining factor (32). According to the most common consensus, published by Fearon et al. (33), the current standard diagnostic criterion for cachexia is represented by percentage of weight loss, BMI values and metabolic changes (29, 33, 34). Simply, CC were classified into three stages: pre-cachexia, cachexia, and refractory cachexia (Table 1). This classification currently did not fully applicable in clinics but is rather to be considered as a proposal under evaluation. Additional parameters (Table 2) have been developed to improve diagnosis, such as food intake measures, albumin levels, anorexia assessment, markers of systemic inflammation (CRP >10 mg/L), muscle mass measurements, the Skeletal Muscle Index (SMI), bioelectrical impedance analysis (BIA), the Fat-Free Mass Index and cachexia index (CXI). Although these diagnostic measurements did not include in the latest consensus, they suggested that several effective parameters could more accurately identify cachexia. A recent systematic review by Paval et al. described the between-study inconsistencies in grouping criteria as a major hindrance to the conduct of meta-analyses for cachexia (31). Refined CC-criteria is critical for evaluating the response to cachexia/antitumor therapy. Because early-onset PDAC-CC can present concomitant with the detection of the primary tumor burden, but cachexia can continue even after the tumors have been surgically removed or effectively treated (15). Patients received either preoperative surgery or chemotherapy/chemoradiation; unintended weight loss coupled with muscle wasting can often be observed, contributing to poor outcomes in PDAC (10, 13, 15, 29). There is no effective strategy to mitigate refractory PDAC-CC. Therefore, the early and precise identification of PDAC-CC is needed to estimate prognosis and prevent progression to the refractory cachexia. More practical, longitudinal definitions of cachexia remain necessary that consider all aspects of the cachexia phenotype.
3 EOLT hypothesis in PDAC-CC
The EOLT hypothesis was proposed to explain how tumor tissues drive disease progression, including CC (31). The EOLT hypothesis states that the tumor acts as an endocrine organ, resulting in dynamic bi-directional communications between the tumor microenvironment (TME) and various organs, leading to the regulation of macroenvironmental changes.
PDAC-CC results in systemic wasting and involves multiple organ dysfunction (Figure 1), accompanied by symptoms including poor appetite, fatigue, depression, muscle wasting, fat wasting, malabsorption, and constipation (Table 3). Tumors secrete cachexia-inducing factors and stimulate host–tumor interactions involve cancer-organ metabolic reprogramming and interorgan signal crosstalk in tumor progression and cachexia development (21, 32). For example, tumor-derived cytokines induce systemic inflammation, stimulating the release of neuropeptides that lead to poor appetite, and the resulting anorexia exacerbates tissue wasting (6, 14, 26). With cachectic environment, adipose and muscle tissues can act like paracrine/endocrine organs in response to cachectic factors, providing reciprocal regulation of energy expenditure and cachexia process (8, 9, 17, 33). Cachexia is a wasting disease that represents metabolic disruptions, mainly catabolisms, driven by systemic inflammation and is characterized by skeletal-muscle proteolysis, adipose tissue lipolysis and hepatic gluconeogenesis (Figure 1) (20, 22, 24, 34). These inter-organ interactions affect metabolisms in the formation of feedback loops. Thus, PDAC-CC can be characterized by two interacting dimensions:
1. Systemic metabolic changes, often associated with KRAS mutations (genetic instability).
2. Pro-cachectic mediators and microRNAs (miRNAs) exacerbated in metabolic disruptions.
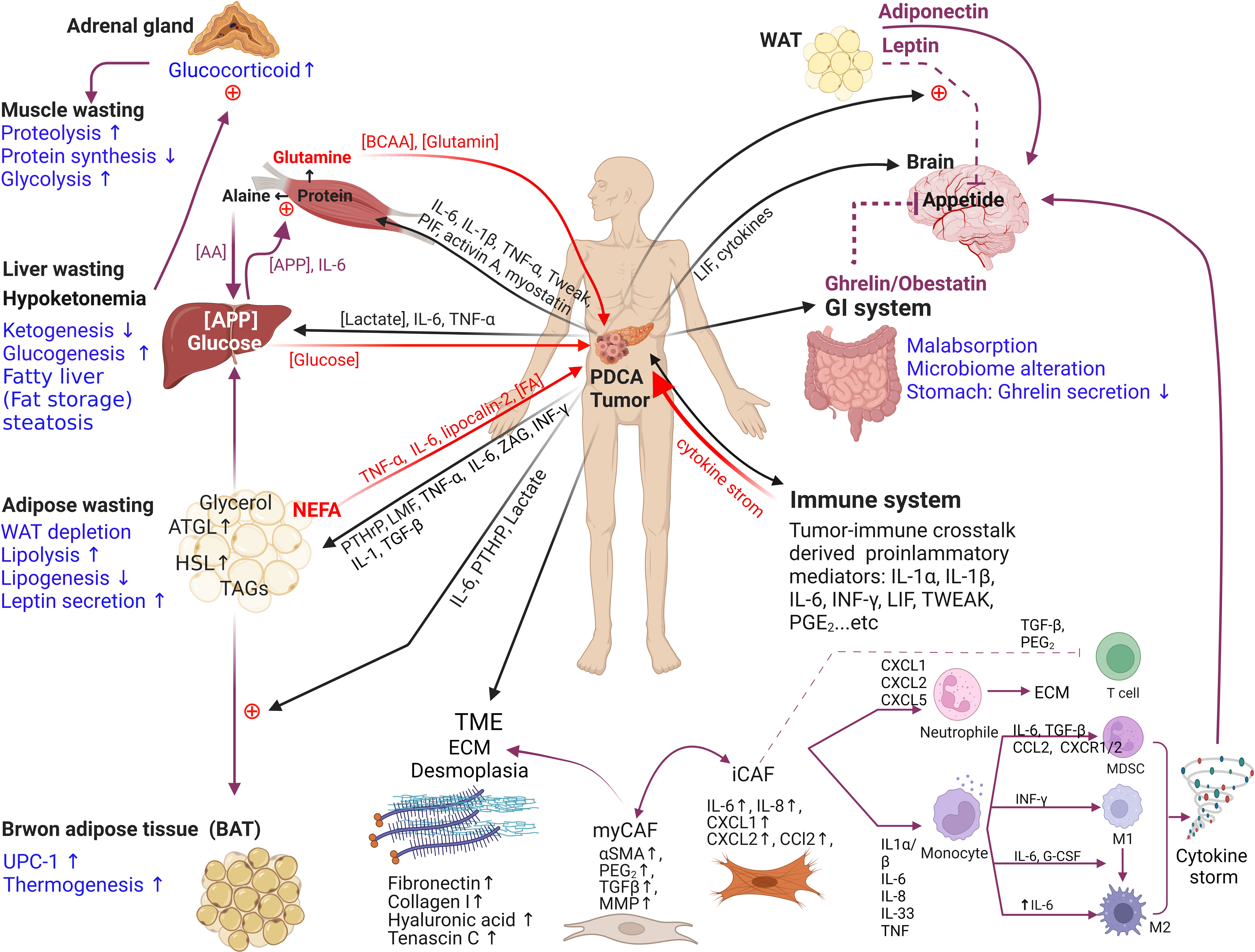
Figure 1 The endocrine organ–like tumor (EOLT) hypothesis for multifactorial cachexia syndrome. EOLT states that solid tumors create multiple endocrine/paracrine organs which differs from the ‘‘seed and soil’’ hypothesis. Tumor-organ crosstalk and interorgan signal crosstalk did not rely on reshaped tumors only. Mostly influenced by different cachectic regulators, such as tumor-derived factors, pro-inflammatory immune mediators (ie. IL-6, IL-1α, IL-1β, TNFα, IFN-γ, ZAG, PIF, activin A, LIF, TWEAK, PGE2), and hormones (including glucocorticoids and PTHrP). These cachectic mediators act as paracrine/autocrine manners, trigger positive feedback to other organs and form a bidirectional circuit (black arrow means mediators derived from tumor; red arrow means mediators derived from peripheral tissues/organs; purple arrow means influence between peripheral tissues/organs). When the communication between tumor and organs exists, metabolic reprogramming (mark in blue: glycolysis↑, proteolysis↑, lipolysis↑ and gluconeogenesis↑) produces bidirectional positive feedback to other organs in cachexia. For example, inflammatory cytokines increase lipolysis in white adipose tissue (WAT), releasing free fatty acids (FAs) that further fuel tumor growth and promote muscle wasting (18–21, 61, 62). Adipocyte also can secrete adipokines (e.g., leptin, adiponectin, and lipocalin-2), IL-6, and TNFα which release via extracellular vesicles (EVs) into the circulation to influence the TME or mediate the appetite (61, 63, 64). Similarly, muscle wasting regulates by hormones, adipocyte-derived mediators and tumor-derived factors (65). Cachexia is a wasting disease that represents metabolic disruptions driven by systemic inflammation and is characterized by the depletion of adipose tissue and skeletal muscle Interleukin, IL; tumor necrosis factor-alpha, TNFα; interferon-gamma, IFN-γ; zinc alpha 2-glycoprotein, ZAG; proteolysis-inducing factor, PIF; leukemia inhibitory factor, LIF; TNF-related weak inducer of apoptosis, TWEAK; prostaglandin E2, PGE2; tumor-derived parathyroid hormone–related protein, PTHrP; amino acid, AA; acute phase protein, APP; triglycerides, TAG; brown adipose tissue, BAT; white adipose tissue, WAT; uncoupling protein 1, UCP1; extracellular matrix, ECM; branched-chain amino acids, BCAAs; chemokine (C-X-C motif) ligand, CXCL; matrix metalloproteinases, MMPs; α-smooth muscle, α-SMA; tumor microenvironment, TME.
The high prevalence of cachexia in PDAC is associated with distinct metabolic effects mediated by tumor created environments, including KRAS mutations, pro-cachexia mediators, and alteration in pancreas and liver. The present review summarizes the current understanding of PDAC-CC according to the EOLT hypothesis.
3.1 Metabolic alterations and high energy demands in tumors
PDACs are characterized by high energy demands within a nutrient-deprived microenvironment. Aggressive PDAC is characterized by increased glycolysis and glutamine metabolism, closely associated with downstream anabolic pathways in the tumor’s hypoxic desmoplastic environment (8, 9, 27, 29, 63). The deprivation of glucose and glutamine and lactic acidosis promote glycolytic and glutaminolysis activity (61, 82, 83). Metabolic alterations are hallmarks of PDAC and PDAC-CC, particularly the dysregulation of glucose and glutamine metabolism (8, 9, 14, 19, 29, 62). However, PDACs under different oxygen and nutrition conditions show distinct and heterogeneous metabolite profiles associated with aerobic glycolysis (the Warburg effect), OXPHOS (oxidative phosphorylation; also known as the reverse Warburg effect), lipid dependence, autophagy, and glutaminolysis (Figure 2). Metabolic alterations are positively correlated with high-grade pancreatic intraepithelial neoplasia (PanIN-3). However, early-onset cachexia also develops independent of PDAC, occasionally presenting in the pre-diagnostic PDAC stage (4). Cachexia is a metabolic disorder involving several nutrient scavenging pathways, including autophagy, micropinocytosis, glycolysis, lipid oxidation, and micropinocytosis (Figure 2: upper panel).
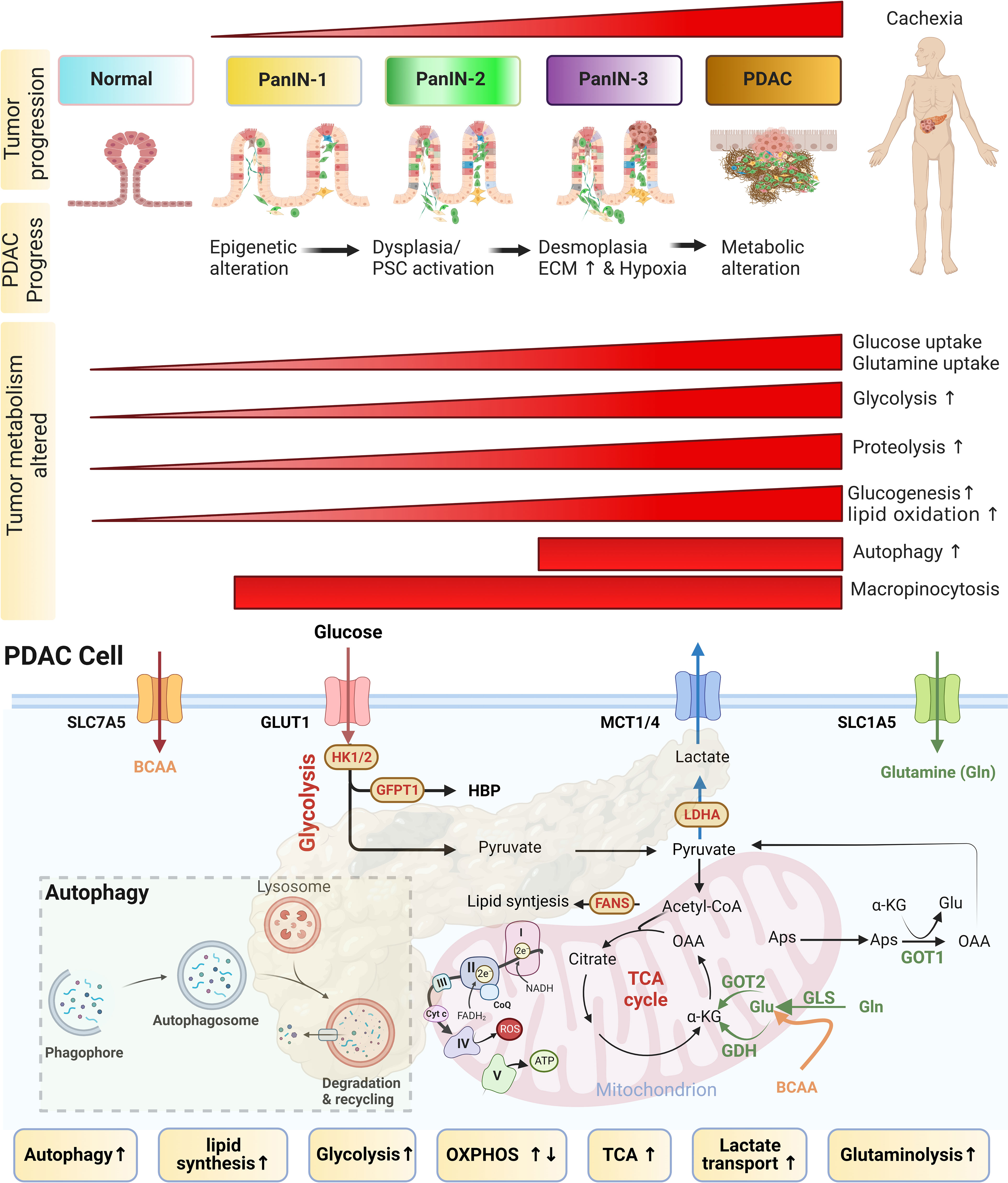
Figure 2 Metabolism alterations in PDAC-CC. The upper panel shows that pancreatic ductal adenocarcinoma (PDAC)-derived cachexia (PDAC-CC) arises from the multi-stage progression of precursor lesions, known as pancreatic intraepithelial neoplasia (PanIN). PanINs are characterized by a continuum of increasingly stroma features (from low-grade dysplasia developing to high-grade desmoplasia). A desmoplastic response induced a fibro-inflammatory microenvironment, stimulating an aberrant metabolic response that is associated with cachexia. During early-stage PDAC, histology can be used to identify several distinct types of precursor lesions. The most common types are microscopic PanIN, low-grade (PanIN-1 and PanIN-2), and high-grade (PanIN-3). The progression to PanIN and to PDAC is associated with cachexia development. Multiple metabolic alterations follow the progression of cachexia, resulting in the reprogramming of glucose, amino acid, and lipid pathways. Metabolic alterations include nutrient scavenging pathway), such as glycolysis glutaminolysis, autophagy, proteolysis, lipid oxidation, and micropinocytosis (Most of them were upregulated during the development of cachexia). However, early-onset cachexia can arise independent of the PDAC stage, occurring in the pre-diagnostic PDAC stage. More than one-third of cancer patients were malnourished before chemotherapy, implying that the cachexia occurred early and followed a poor response to chemotherapy. Interestingly, some of cachexia occurs after the chemotherapy. The lower panel: The metabolic alterations, including increase glycolysis, glutaminolysis, lactate transport and autophagy … etc, in PDAC cell associated with PDAC-CC, primarily due to promote the expression in key enzymes (HK1/2, GFPT1, and LDHA) and transporters (GLUT1, MCT1/4, SLC7A5, and SLC1A5). The metabolic shift from the tricarboxylic acid (TCA) cycle and oxidative phosphorylation (OXPHOS) to aerobic glycolysis is tightly regulated. HK1/2, hexokinase; GFPT1, glutamine fructose 6-phosphate amidotransferase 1; LDHA, lactate dehydrogenase A; GLUT1, Glucose transporter 1; MCT1/4, monocarboxylate transporter 1/4; SLC7A5 (LAT1), neutral amino acid antiporter; SLC1A5, glutamine transporter.
PDAC survives and thrives in relatively hypoxic and nutrient-poor niches, driven by [1] reprogramming intracellular nutrient metabolism, including glucose, amino acids, and lipids; [2] scavenging and recycling nutrients; and [3] promoting metabolic crosstalk (Figures 2: lower panel and Figure 3) (8, 9, 62). PDAC-CC exacerbates metabolic reprogramming, promoting the deterioration of muscle and adipose tissue (Figure 3), further supporting the energy and nutrient needs of the tumor tissue.
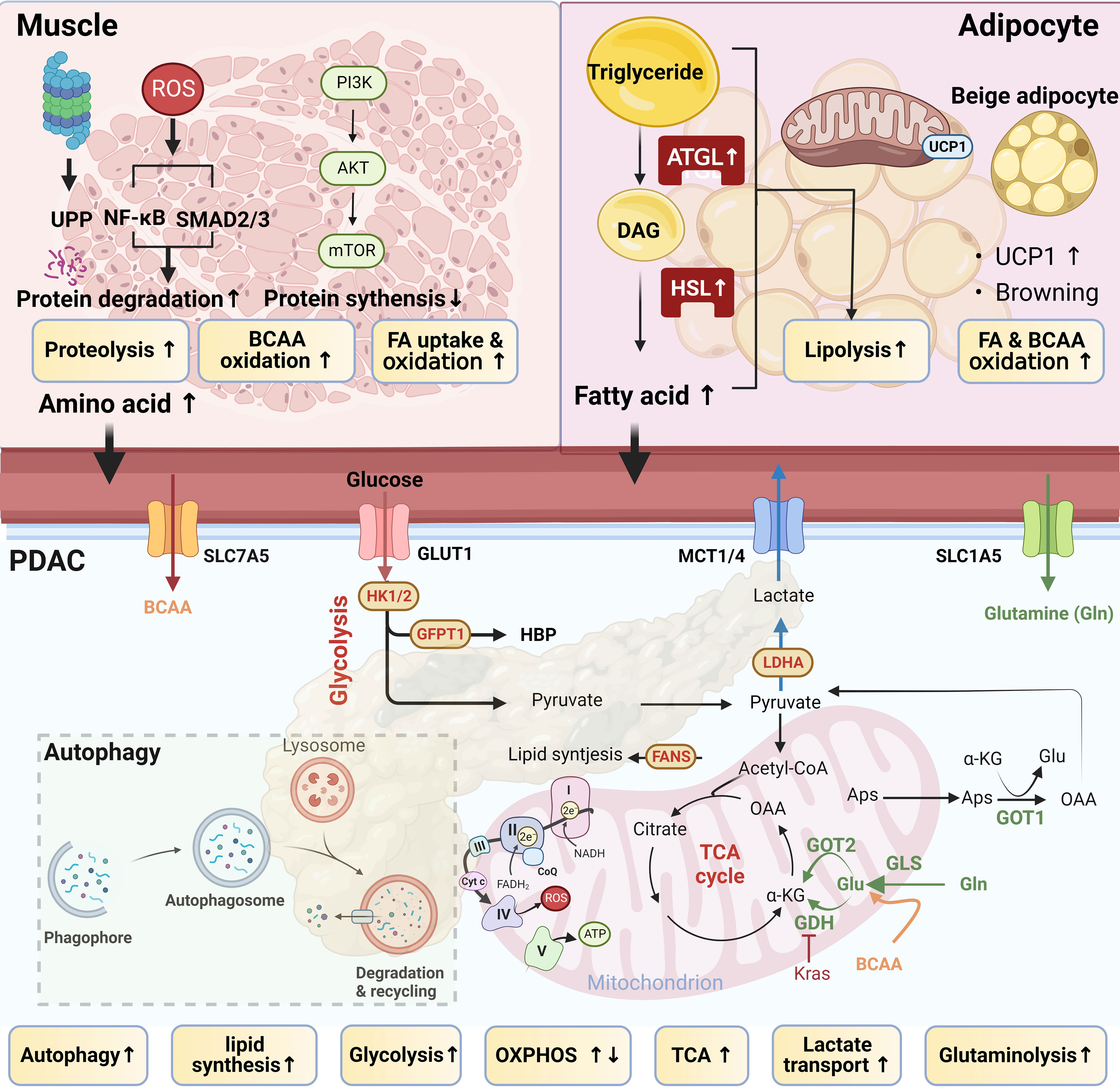
Figure 3 Tumor–muscle–adipocyte crosstalk. Pancreatic ductal adenocarcinoma (PDAC)-derived cachexia occurs due to a feedback circuit among tumor, adipocyte, and muscle tissues. In PDAC, tumor‐derived factors, including interleukin (IL)‐1, IL-6, IL-8, proteolysis-inducing factor (PIF), lipid mobilization factor (LMF), and tumor necrosis factor‐α (TNFα), enhance proteolysis, lipolysis, and the catabolic state of muscle and adipocytes, leading to adipose and muscle wasting. Tumor cell–triggered metabolic reprogramming in muscle and adipocytes releases metabolic products, such as branched-chain amino acids (BCAA) and free fatty acids (FFAs), to fuel tumor growth. Lipolysis and proteolysis are the two main processes causing adipose and muscle wasting in cachexia.
3.1.1 Glucose
Glycolytic flux can result in changes in the pentose phosphate pathway (PPP), the hexosamine biosynthesis pathway (HBP), serine biosynthesis, and the tricarboxylic acid (TCA) cycle, promoting CC development (64). Rate-limiting glycolytic enzymes, such as hexokinase 1/2 (HK1/2), phosphofructokinase 1, and lactate dehydrogenase A (LDHA), are upregulated to facilitate the Warburg effect, resulting in glycolytic flux and the production of lactate from glucose in PDAC-CC (63, 64). The upregulation and translocation of glucose transporters (GLUT1, encoded by SLC21A) in tumor tissues facilitate glucose uptake for aerobic glycolysis. Increased glycolytic flux in response to host–tumor interactions in cachexia results in the production of high lactate levels, leading to lactic acidosis. To address lactic acidosis, PDACs robustly express monocarboxylate transporters (MCT1 and MCT4, encoded by SLC16A1 and SLC16A3, respectively) to coordinate glucose utilization and lactate mobilization (65, 84). Other glucose metabolism pathways are also altered in PDAC, such as the upregulation of the rate-limiting enzyme of the HBP, glutamine: fructose-6-phosphate amidotransferase-1 (GFPT1) (64). Many other mediators regulate glucose metabolism in pancreatic cancer cells. Under hypoxic conditions, hypoxia-inducible factor-1 (HIF-1) can promote glycolysis and upregulate the expression of HBP-related enzymes, such as GFPT2, an isoform of the HBP rate-limiting enzyme GFPT1 (63).
In a model of PDAC-CC, athymic mice injected with high-glycolytic MiaPaCa2 cells showed evidence of cachexia, such as weight loss, fat depletion, and muscle proteolysis (66), suggesting that glycolysis may be involved in PDAC-CC development. Glycolysis was associated with inefficient inter-organ substrate shuttles, as assessed by the lactate-to-pyruvate utilization ratio, LDH activity, and MCT1 expression, which was correlated with cachexia-related weight loss (63). The upregulation of GLUT1 and MCT1/4 promotes glucose utilization and improves the lactate-to-pyruvate utilization ratio in tumor tissue (63, 65). Paradoxically, OXPHOS, also known as the reverse Warburg effect, occurs in muscle, resulting in an increased lactate-to-pyruvate production ratio, providing a potential lactate supply for tumor use and supporting tumor progression and consequent atrophy (85). Additionally, the tumor secretes interferon-gamma (IFN-γ), which mediates the development of insulin resistance via reduced glucose and fatty acid [FA] uptake, leading to enhanced lipolysis in WAT (86). Inefficient inter-organ substrate shuttles are regarded as hallmarks of EOLT (Figure 3).
Aerobic glycolysis occurs more commonly in tumor tissues than OXPHOS, which requires a sufficient oxygen supply, although OXPHOS is more efficient for ATP generation (30, 87). Nutrient depletion forces tumors to adapt by inducing nutrient scavenging mechanisms to support cancer progression, which can lead to CC (8, 9, 88). OXPHOS occurs in the mitochondria and is sensitive to stress conditions, as the respiratory complexes in stressed mitochondria produce high levels of reactive oxygen species (ROS) (62). Autophagy is a stress response induced by ROS to remove damaged mitochondria that overproduce ROS, promoting mitochondrial metabolism (89) (Figure 2: lower panel). In cachectic patients, increased OXPHOS and dysfunctional autophagy are associated with increased muscle wasting (Figure 3) (90). Autophagy is an important proteolysis pathway activated during PDAC-CC and muscle wasting (91, 92).
Tumors supported by an adequate blood supply can perform aerobic metabolism and tend to exhibit the reverse Warburg effect. In tumors, aerobic metabolisms may utilize intermediates, such as lactate, to fuel the TCA cycle (Figure 2: lower panel), decreasing their dependence on glucose. The low uptake of glucose and the enhanced uptake of intermediate metabolites by tumors under aerobic conditions could protect these tumors from competing with hypoxic tumor regions (such as desmoplastic tumors) for glucose. In addition to OXPHOS, tumors able to perform aerobic metabolism can also utilize glutaminolysis as an alternative energy production pathway requiring activated mitochondrial metabolism (93). Glutamine is the most abundant and versatile nonessential amino acid (NEAA), found in both the blood and the cell cytoplasm, and can be used by both the glutamine-dependent pyruvate cycle and the TCA cycle (30, 94). In contrast, hypovascularization and desmoplasia often occur in PDAC; studies also found that HIF-1α (hypoxia-inducible factor-1α) stabilization promotes glycolytic enzymes to shift the metabolism by repressing OXPHOS (95, 96). Supposing that if the function of glycolysis is weakened, OXPHOS and glutamine-based processes will serve as alternative energy generation mechanisms in glucose-limited tumors (87) (Figure 2: lower panel).
3.1.2 Amino acids
Altered amino acid (AA) metabolism is a frequent feature in CC. Branched-chain amino acids (BCAA: leucine, isoleucine, and valine) act as important carbon sources and are useful for FA biosynthesis. High BCAA levels in plasma are associated with early PDAC and are often derived from increased protein breakdown in muscle and other body tissues (Figure 3) (97, 98). The utilization of BCAAs by PDAC can result in plasma BCAA depletion during late-stage PDAC. Similar observations have been reported for glutamate, in which the plasma levels of glutamate and the glutamine/glutamate (Q/E) ratio are significantly reduced in cachectic patients and animal models compared with their healthy counterparts (83, 99). Glutamine metabolism is a primary source of nitrogen and carbon, contributing to macromolecular synthesis and redox balance (83). Glutaminase 1 (GLS1) converts glutamine to glutamate, after which glutamate dehydrogenase (GDH) catalyzes the conversion from glutamate to α-ketoglutarate (α-KG). However, GDH is repressed in PDAC, and cytoplasmic aspartate transaminase (GOT1) is upregulated (83) (Figure 2: lower panel). Cachexia is associated with more aggressive forms of PDAC, which may reflect the increased access of tumor cells to nutrients derived from protein breakdown and systemic changes in glucose metabolism (97, 100). Higher circulating BCAA levels may arise from the impaired catabolism of AAs that are commonly found in muscle (Figure 3). Muscle wasting is characterized by decreased muscle mass, increased proteolysis, and reduced protein synthesis, changes which are mediated by the proteasome, nuclear factor kappa B (NF-κB), and the mammalian target of rapamycin (mTOR) pathways. The phosphoinositol 3-kinase (PI3K)/AKT/mTOR pathway is a nutrient-sensing mechanism stimulated by decreased glucose availability in the muscle. A higher mTOR activity induced by KRAS mutation in PDAC which is positively correlated to higher circulating BCAA levels (12). mTOR activation is responsible for the uptake of BCAA in tumor tissue. Circulating BCAA also can affect subcutaneous adipocyte AA dysmetabolism. Both NF-κB and AKT/mTOR signaling are involved in proteolysis. NF-κB regulates the ATP-dependent ubiquitin–proteasome proteolytic pathway, including muscle-specific E3 ubiquitin ligases (such as muscle atrophy F box protein [MAFbx/atrogin-1] and muscle RING finger–containing protein 1 [MuRF1]), which promote proteolysis and contribute to muscle atrophy (22). Cachexia is the end result of convergent metabolic adaptations induced by tumors to satisfy their metabolic requirements.
3.1.3 Lipids
In addition to glucose and amino acid metabolism, metabolic alterations in cachexia can include lipid metabolism. Approximately 93% of triacylglycerol FAs used by tumors are synthesized de novo by the mitochondria and cytosolic acetyl coenzyme A (CoA). Enzymes that participate in de novo FA and cholesterol synthesis are upregulated in PDAC, such as FA synthase (FASN) and 3-hydroxy-3-methylglutaryl coenzyme A reductase (HMGCR). Under pancreatic inflammatory conditions, wasting adipocytes release FAs into the plasma, increasing plasma concentrations of saturated (SFAs), monounsaturated (MUFAs), and polyunsaturated FAs (PUFAs) (101). SFAs and MUFAs promote PDAC progression (102). However, a study performing transcriptomics and metabolomics suggested that lipase and a panel of FAs were significantly decreased in PDAC, and the presence of two SFAs (palmitate and stearate) inhibited tumor cell proliferation (103). Therefore, the roles played by FAs in PDAC appear complicated and remain unclear. PDAC patients present with distinct phenotypes associated with cachexia development, such as adipose tissue loss prior to skeletal muscle wasting or the loss of adipose tissue alone (104). A recent report indicated that soft tissue changes are initiated in PDAC before skeletal muscle loss (19), and the significant loss of visceral adipose tissue has been observed in PDAC-CC (18). In a retrospective cohort study, PDAC-CC was associated with the accumulation of oleic acid in plasma, resulting from malnutritional compensatory mechanisms triggered by the lack of oleic acid uptake into tissue (105). In a pre-cachexia model, increased FA oxidation occurs before muscle mass reduction, suggesting that FA may serve as a dominant energy source in PDAC-CC (18, 106). Adipose tissue lipolysis contributes to circulating FAs and subsequent FA uptake and lipid accumulation in the muscle and tumor tissue, leading to eventual metabolic derangement and muscle wasting after a period of metabolic adaptation. Several lipolytic enzymes are elevated, such as adipose triglyceride lipase (ATGL) and hormone-sensitive lipase (HSL), further suggesting the occurrence of enhanced lipolysis. Increased ATGL and HSL activity correlate with tumor growth and WAT loss in cachexia (107) (Figure 3). Tumor progression is also associated with the shift from WAT to BAT, known as adipose tissue browning. BAT is a metabolic hallmark mediated by uncoupling protein 1 (UCP-1). In KPC and KrasLSLG12D/+Trp53f/f mice, adipose tissue browning is associated with increased UCP-1 expression and occurs prior to the onset of fat wasting, consistent with clinical observations (108). A few studies have suggested that fat loss is an early and precipitating event prior to muscle loss in PDAC-CC, even in the absence of muscle wasting (18, 19, 21, 33, 104, 105, 107). Clinical studies suggested that fat loss may serve as a driving force for cachexia mortality, emphasizing the important roles of adipocytes in PDAC-CC and supporting the need to monitor adipose in patients with CC (20, 21).
Tumors hijack organ and tissue function, causing muscle and adipocyte wasting. Enhanced glycolysis in tumors and the upregulation of lipolysis and proteolysis in wasting tissue counterbalance the reductions in muscle and fat under cachectic conditions (Figure 3). Wasting muscle and adipocytes are among the convergent metabolic adaptations induced by tumors to satisfy their metabolic requirements. Patients with PDAC and PDAC-CC exhibit distinct and heterogeneous metabolic changes. Tumor, muscle, and adipocyte tissues all act as endocrine organs involved in the regulation of metabolic homeostasis, consistent with the EOLT hypothesis. In addition to metabolic alterations, bi-directional feedback occurs between tumor tissues and other organs, driven by the oncogenes and mediators (8, 12, 21, 32, 62).
3.2 Genetic instability–driven cachexic phenotypes and experimental models
In PDAC, tumors become genetically unstable (Figure 4), and mutations in four oncogenes are common in PDAC: KRAS (>95%), p16/CDNK2A (> 90%), TP53 (~70%), and SMAD4 (55%) (8, 9, 62, 100). The hyperactivation of oncogenes (e.g., KRAS) and the downregulation of tumor suppressor genes (e.g., TP53 and CDKN2A) promote tumor progression through the activation of various signal transduction pathways, including Wnt/Notch, c-Jun N-terminal kinase (JNK), PI3K, KRAS, and transforming growth factor (TGF)-β. A series of genetic and molecular events initiated by early oncogenic mutations in PanINs and later mutations in PDAC have been associated with metabolic alterations (109, 110). PDAC-CC is initiated by a metabolic shift in fuel utilization, in which glycolysis, proteolysis, and lipolysis increase and lipogenesis and protein synthesis decrease (Figure 4). During the pre-cachectic stage, patients experience these metabolic alterations as loss of appetite and impaired glucose metabolism before PDAC diagnosis or significant weight loss is apparent (Figure 2) (19, 67, 105). Early inflammatory signals may trigger the initial muscle and adipocyte wasting signaling cascades (9, 18, 21, 24).
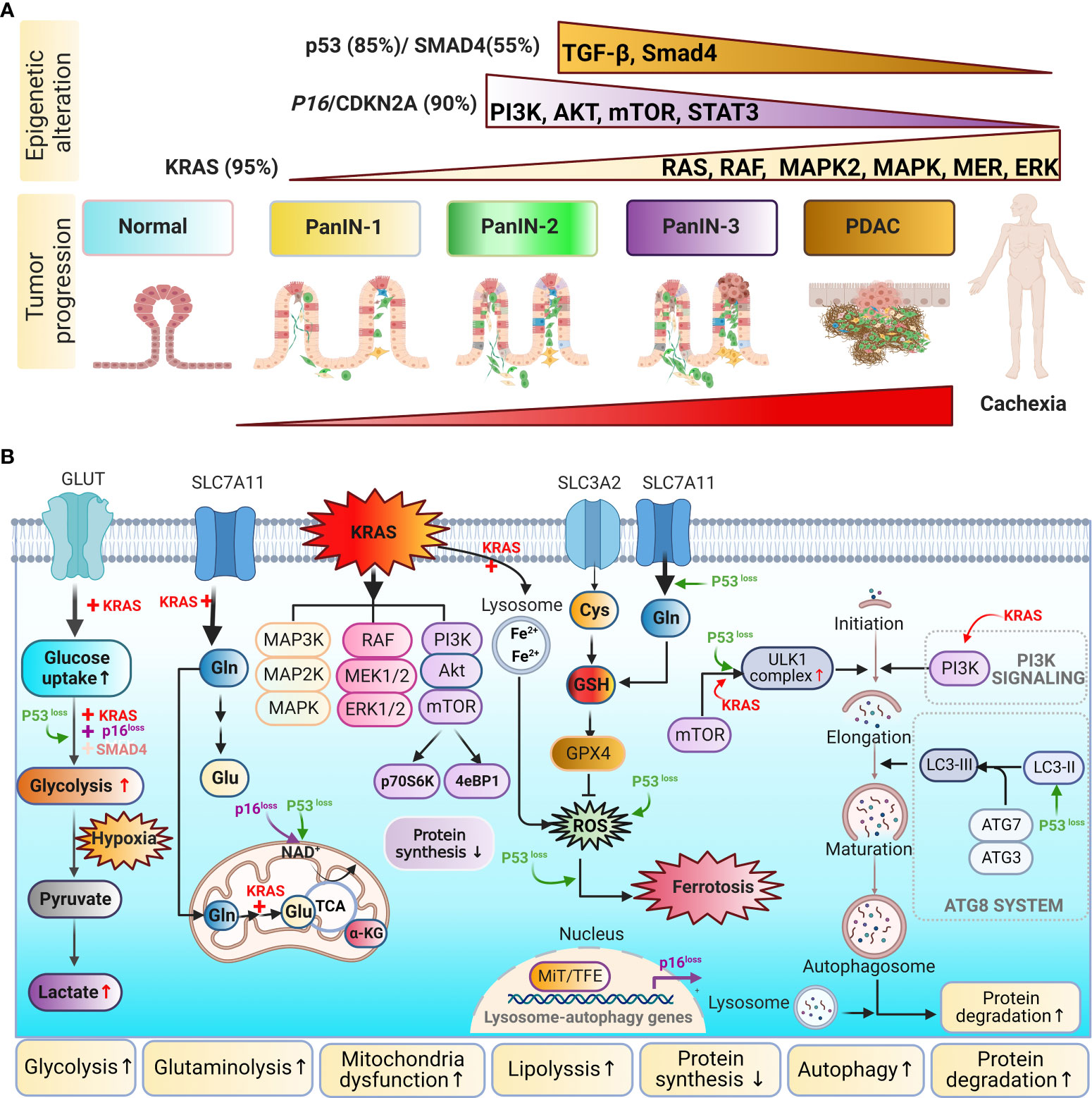
Figure 4 Metabolic remolding is influenced by genetic instability in PDAC. (A) Genetic mutations: PDAC is affected by high frequencies of aberrations and mutations in KRAS, P16/CDKN2A, TP53, and SMAD4. KRAS is involved in the RAF/mitogen-associated protein kinase pathway and the phosphoinositol 3-kinase (PI3K) pathway. P16/CDKN2 mediates the PI3K/AKT/mTOR pathway. TP53 influences the transforming growth factor-beta (TGF-β)/Smad4 pathway. (B) Mutated forms of KRAS, TP53, P16/CDKN2, and SMAD4 promote glucose (Glc) uptake and enhance glycolytic flux, including the production of lactate (Lac). KRAS and TP53 can both reprogram glutamine (Gln) metabolism to balance cellular redox homeostasis. Pancreatic cancer induces metabolic shifts, including increased glycolysis, lipogenesis, glutaminolysis, and autophagy, which are related to cachexia.
KRAS is the most prevalently mutated oncogene, and KRAS mutations are considered to be dominant driver mutations in PDAC. Mutant KRAS regulates components of the mitogen-activated protein kinase (MAPK) and PI3K pathways to reprogram intracellular metabolism, including increasing glycolysis, by altering the levels of GLUT1 (111), HBP, and PPP (64, 112). Direct downstream effector cascades affected by KRAS mutations include the RAF–MAPK kinase (MEK)–extracellular signal–regulated kinase (ERK) and PI3K–AKT–mTOR pathways. The RAF–MEK–ERK pathway is considered among the most critical. The KRASG12D mutation is frequently observed in pancreatic cells and promotes glycolysis via the upregulation of the MEK–ERK–HIF-1α axis. Elevated HIF-1α results in a feedforward loop between the insulin growth factor (IGF)-1 receptor, HIF-1α, and caveolin-1 to facilitate tumor progression and glycolysis (113).
Besides promoting high levels of glycolysis, KRAS upregulates glutaminolysis, allowing glutamine to be used as an additional carbon source for the TCA cycle (114). NEAAs, such as alanine, are alternative carbon sources that can support altered energy metabolism in PDAC (115). KRAS mutations increase glycolysis and the metabolism of amino acids, such as alanine and glutamine, activating downstream catabolic pathways, including proteolysis and lipolysis. Genetic mutations promote the recycling of wasting tissues to fuel cancer growth.
KRAS mutations also promote the generation of inflammatory cytokines, which shape the PDAC TME, including IL-6, IL-8, C–X–C motif ligand (CXCL)1, CXCL2, and CXCL5 (116, 117). Some cytokines/chemokines act in both autocrine and paracrine manners to support tumorigenesis and tumor angiogenesis (116).
KRAS activation leads to the loss of p16, accelerating NADH oxidation and supporting increased glycolysis through the production of NAD+ to support tumor growth (118). However, PDAC cells lack nutrient sensors, and mTOR complex 1 (mTORC1), a nutrient-sensing mechanism, is bypassed in PDAC. Bardeesy et al. proposed that autophagy is driven by the elevated expression of the microphthalmia transcription factor (MiTF) family members MiTF, TFE3, and TFEB in PDAC (119). The loss of SMAD4 is another frequent event associated with PDAC progression, identified in approximately 50% of PDAC cases. SMAD4 is a central component in the transforming growth factor (TGF-β) signaling cascade, and SMAD4 loss enhances glycolysis by altering the expression of the glycolysis enzyme phosphoglycerate kinase (PGK) (120). The loss of TP53 alters metabolism in PDAC by inhibiting mitochondrial respiration and simultaneously stimulating glycolysis. A recent study demonstrated that TP53 rewires glucose and glutamine metabolism in malignant PDAC by preventing the nuclear translocation of glyceraldehyde 3-phosphate dehydrogenase (GAPDH) and stabilizing its function (121). Loss of TP53 can reduce the expression of fructose-2,6-bisphosphate to promote the glycolysis cycle (122). Therefore, KRAS, P16, P53, and SMAD4 have counterintuitive effects that promote tumorigenesis, further highlighting the complexity of interactions between genes and metabolisms in cancer progression and cachexia development.
These metabolic changes are consistently observed in numerous preclinical animal models of PDAC cachexia (Table 4). Commonly used animal models of PDAC-CC include [1] intraperitoneal (IP) injections of PDAC cells, which localize to the pancreas; [2] orthotopic models of PDAC, in which cancer cells are injected directly into the pancreas; [3] patient-derived xenograft (PDX) models, in which a portion of a resected human pancreatic tumors are surgically attached to the mouse pancreas; and [4] genetically engineered mouse models (GEMMs) of PDAC. Up to 85% of PDAC patients suffer from CC, and approximately 30% of PDAC patients succumb to cachexia rather than tumor burden (137, 138). PDX and murine allograft models have been applied to study cachexia, resulting in the identification of Toll-like receptors 7/9 (TLR7/9), MyD88, and TGF-β as mediators of cachexia in PDAC (129, 137, 139, 140). Most PDAC-CC studies focus primarily on weight loss, muscle wasting, and the analysis of mRNA markers. A comparison analysis of subcutaneous, IP, and orthotopic PDAC animal models indicated that the implantation site is crucial when attempting to study PDAC-CC (137). Both IP and orthotopic implantation models develop more severe cachexia symptoms (such as muscle wasting, anorexia, and a decrease in locomotive activity) than the subcutaneous implantation model. The orthotopic animal model is histologically similar to PDAC patients, mimicking the TME associated with intact tumors, suggesting that the TME may be involved in cachexia induction. Studies in PDAC animal models have demonstrated that tumor-associated macrophages mediate muscle wasting via the activation of signal transducer and activator of transcription (STAT)3 signaling (134, 141).
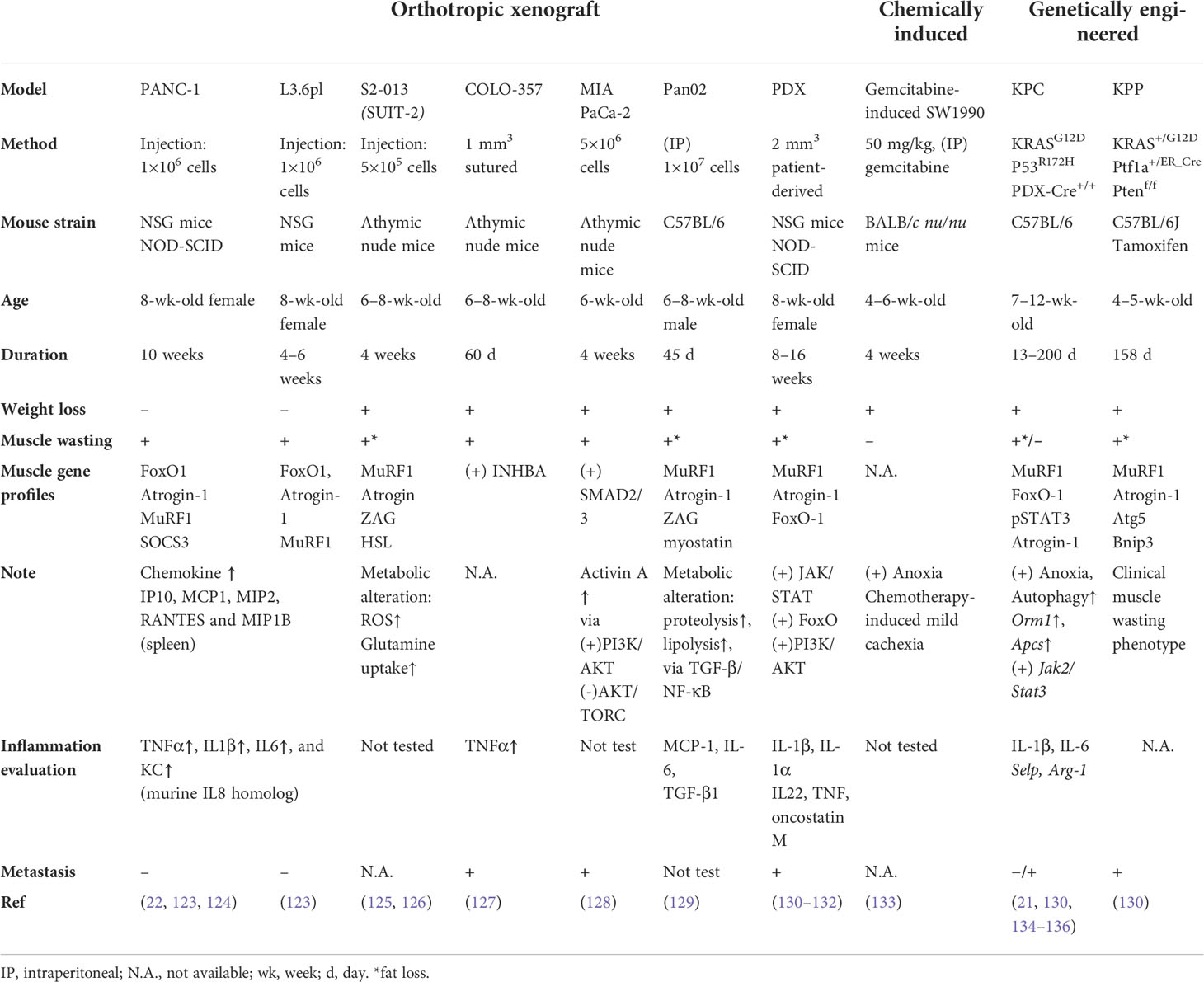
Table 4 Genetically engineered mouse models (GEMMs) of pancreatic cancer-derived cachexia phenotypes.
Most preclinical studies of CC use C-26 (colon cancer) and LLC (lung cancer) mouse models. However, these two models are associated with limitations (1): a limited interval between the onset of CC symptoms and animal death leaves only a small therapeutic window, and (2) translatability to humans may be limited, as the gene expression profiles in these mice did not correlate with those in human cancer tissue biopsies (130). However, GEMMs offer slower cachexia progression and early development than other cancer models, and PDAC-CC animal models are more translatable to humans than models using other cancer types. Therefore, animal models of PDAC-CC are clinically relevant. Preclinical PDAC murine models may be useful for understanding cachexia progression and evaluating therapeutic options for mitigating PDAC-CC. Establishing a model able to fully mimic the human condition remains necessary. Animal models can contribute to improving our understanding of the mechanisms driving tissue wasting for translation into new anti-cachexia therapies.
3.3 Pro-cachectic mediators and microRNAs
3.3.1 Pro-cachectic mediators
Endocrine organs and cells synthesize biologically active compounds that are released directly into the circulation and interact with other cells. Cachexia-associated inflammation is influenced by numerous bioactive molecules, such as TNFα, IL-1, IL-6, and IL-8 (Table 5 and Figure 5). Cachexia-affected organs can act as autocrine or paracrine organs, releasing factors into the bloodstream to promote systemic crosstalk. These cytokines have multifactorial effects, triggering a hypercatabolic feedforward loop between the tumor, adipose tissue, and muscle mediated by the NF-κB and Janus kinase (JAK)/STAT pathways (29, 174, 175) (Figure 5). NF-κB and JAK/STAT activation enhance lipolysis, downregulate lipogenesis, and stimulate the catabolism of lean body mass (12).
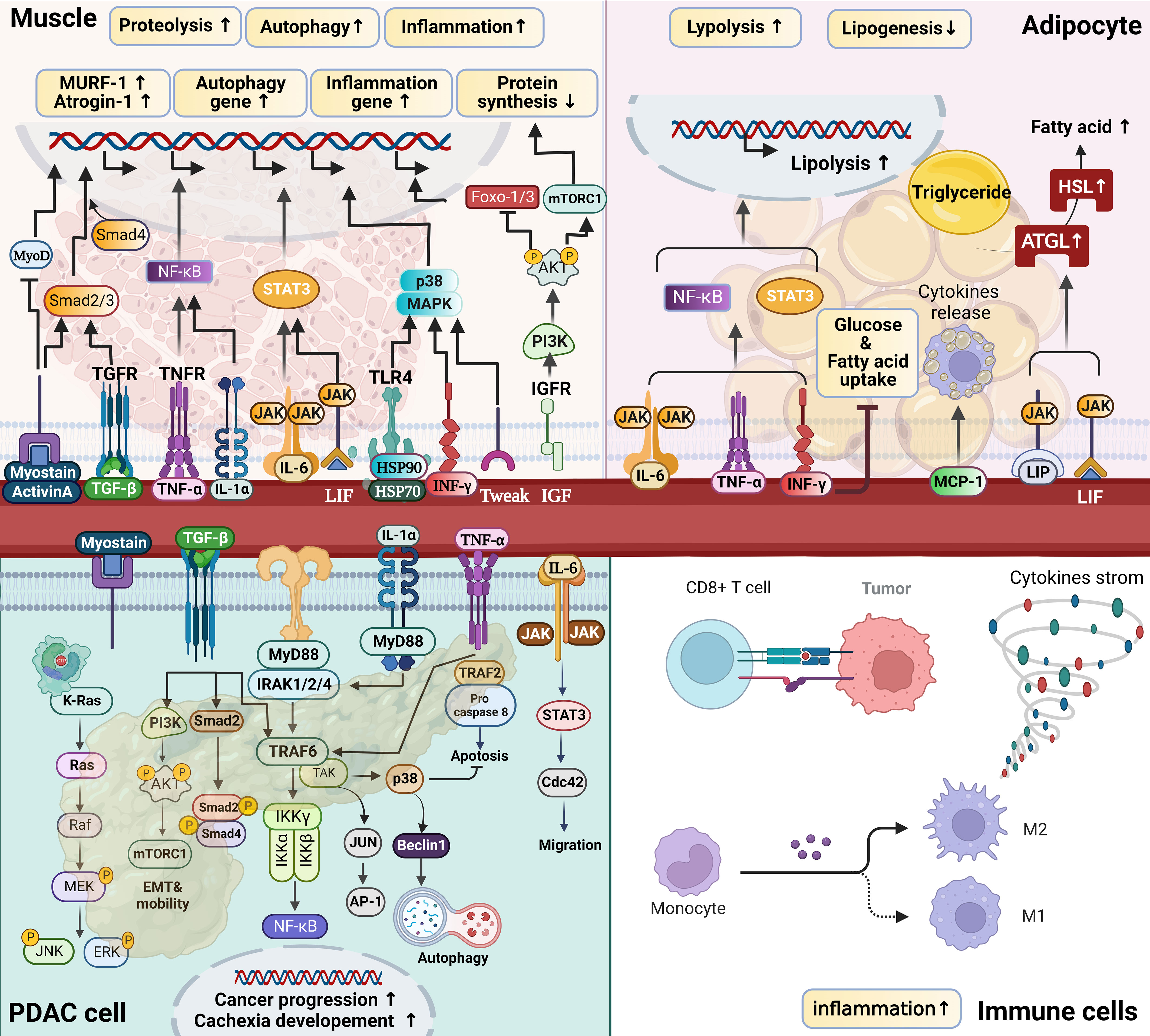
Figure 5 Pro-cachectic mediators of catabolism in PDAC-CC. Cachexia signals induce tissue catabolism by modulating gene expression profiles related to protein synthesis and degradation in muscle, lipid depletion, and tumor progression, primarily via the nuclear factor kappa B (NF-κB) and Janus kinase (JAK)–signal transducer and activator of transcription (STAT) pathways. In tumors, multiple receptors, including the Toll-like/IL1 receptors (TIRs), tumor necrosis factor (TNF), transforming growth factor-beta (TGF-β), and interleukin receptors (IL-6R being the best-studied), utilize overlapping and distinct signal transduction mechanisms to affect cellular outcomes, including increased cytokine production, proliferation, survival, migration, autophagy, and resistance to chemotherapy and immune surveillance. In addition to cytokines in tumors, circulating cytokines affect muscle and adipocytes, resulting in various metabolic alterations. For example, myostatin/activin A binds to type II receptors (ActRIIB), leading to Smad2/3 phosphorylation and the recruitment of Smad4, which results in muscle wasting. Simultaneously, myostatin/activin A signaling inhibits AKT activity and suppresses FoxOs phosphorylation, activating the ubiquitin–proteasome and autophagy–lysosome systems. IL6 binds to receptors to activate JAK/STAT3 signaling, increasing protein degradation. TNFα and IL1 signaling activates the IκB kinase (IKK)–NF-κB axis to initiate proteasome-mediated protein degradation. Higher levels of tumoral and stromal IL-1β expression result in a feedback circuit that attributes to cancer progression and cachexia development.
A salient feature that distinguishes PDAC from other KRAS-mutant cancers is an extensive fibro-inflammatory stroma, which accounts for 80%–85% of the tumor bulk. These stromal cells are recruited and reprogrammed by PDAC cells during cancer progression and cachexia development. Secreted factors (Table 5) enable these cells to communicate with PDAC, creating a dynamic feedback circuit associated with intrinsic KRAS signaling in PDAC cells (Figure 5).
Various circulating pro-inflammatory cytokines have been implicated in PDAC-CC, including IL-6, IL-1, IL-8, TGF-β, and TNFα …etc (detailed in Table 5) (12, 21, 29, 123). These cytokines likely derive from various sources and result in systemic effects (123, 176). Oncogenic RAS drives the expression of multiple inflammatory cytokines, including IL-1 and IL-6 (21, 146). Inflammatory cytokines released by tumor cells may be prominent cachexia-associated factors that regulate autocrine and paracrine function, promoting tumor progression and cachexia development. For example, IL-6 plays autocrine roles in supporting tumorigenesis in vivo and induces weight loss and inflammation in cachexia via a paracrine manner (21, 68, 152). Other cytokines, such as IL-1, IL-8, TNFα, and INF-γ, have also been associated with weight loss and poor survival in PDAC (148, 177, 178). TNF-α and IL-1 can induce anorexia, producing both hypercatabolic and anorexigenic effects (Figures 1 and 5). Circulating IL-1β promotes NF-κB activation in the hypothalamus, enhancing glucocorticoid production and resulting in catabolic effects in both muscle and adipose tissue. TGF-β1 can induce proteolysis through the E3 ligase atrogin-1 in animal models. TGF-β inhibition improved muscle wasting in the KPC model (129). Activin A and myostatin belong to the TGF-β superfamily and are associated with muscle wasting through the activation of the Smad2/3 pathway, which decreases AKT–mTOR-mediated protein synthesis and enhances ubiquitin ligase–mediated proteolysis (18, 128). These cytokines drive diverse catabolic processes across multiple cells and organs, forming a catabolic feedforward loop (Figure 1).
In muscle tissue, the JAK–STAT and NF-κB pathways are the dominant catabolic pathways activated by circulating IL-6 and TNFα in muscle wasting (174). IL-6 induces NF-κB activation, which can also upregulate ubiquitin-mediated proteasomal degradation in wasting (179). Proteolysis is a prerequisite for muscle wasting, and both lipolysis and adipopenia may occur prior to muscle loss. Lipolysis results in increased circulating FFAs, triggering the secretion of Atrogin-1 and MuRF1, which induce muscle atrophy.
In adipocytes, lipolysis plays a substantial role in increasing the catabolism of stored fat. ATGL and HSL act to reduce fat to its component FAs, leading to the loss of body mass. Secreted IL-6 can trigger browning by inducing UCP-1 expression in adipocytes (18, 180). The catabolic effects of IL-6 on WAT in vitro are mediated through the JAK/STAT3 and NF-κB pathways (21, 181).
3.3.2 Epigenetics modulation (miRNAs)
Genetic instability and epigenetic changes are both involved in pancreatic oncogenesis and cachexia development (Table 6). Recently, miRNAs, small non-coding RNAs 19–25 nucleotides in length, have been identified in an increasing number of biological processes, including KRAS signaling and the JAK–STAT, PI3K–AKT, notch, and TGF-β signaling pathways (Figure 6A). These influences contribute to the control of several cancer-related processes in PDAC, such as tumor growth, apoptosis, metastasis, drug resistance, and the immune response. In addition to roles in oncogenesis and tumorigenesis, aberrant miRNA expression may affect cytokine production or directly alter metabolic processes, resulting in a metabolism remodeling that facilitates PDAC progression and cachexia development (see Figure 6 and Table 6).
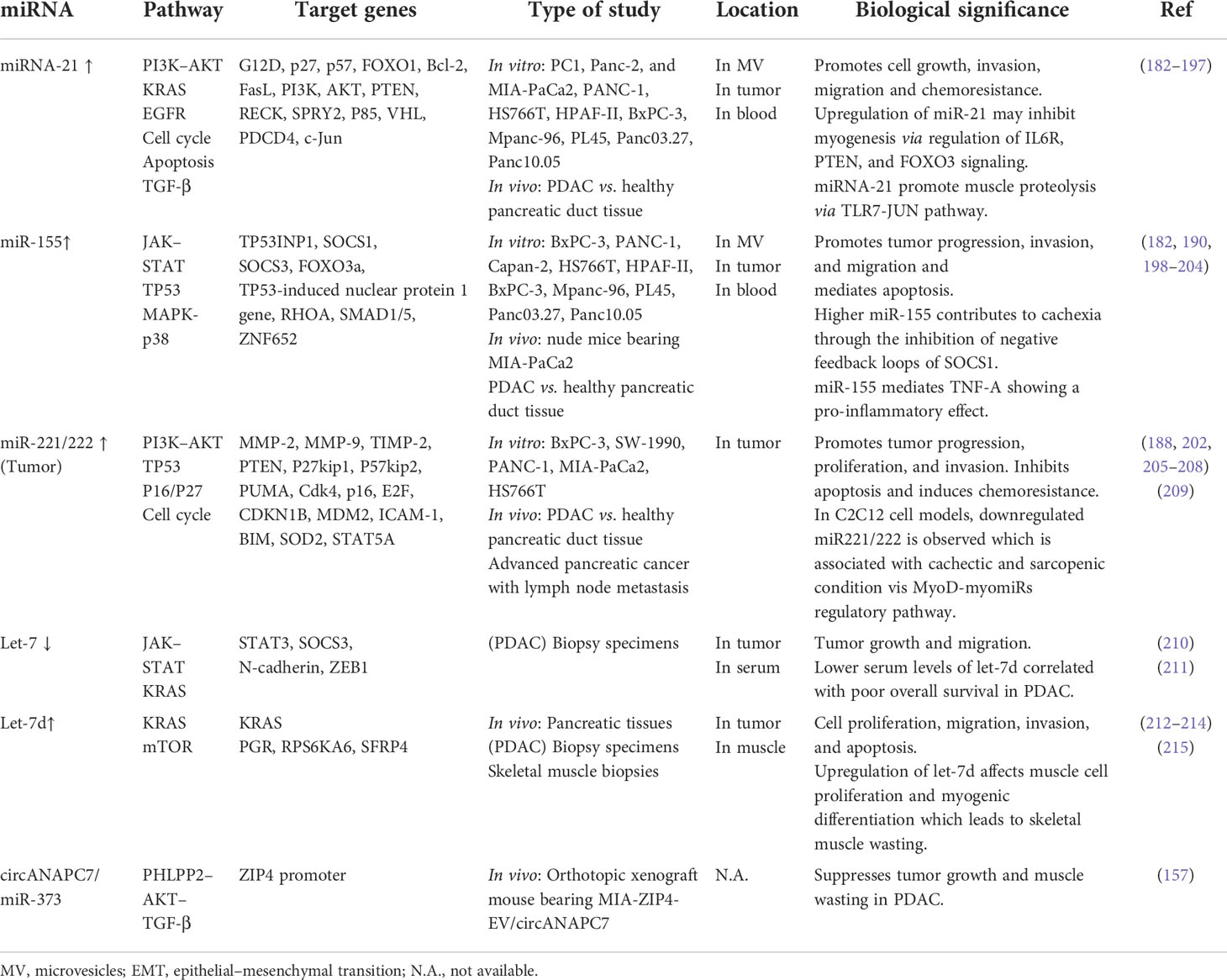
Table 6 MicroRNA (miR) expression levels and functions in pancreatic ductal adenocarcinoma (PDAC) and PDAC cachexia.
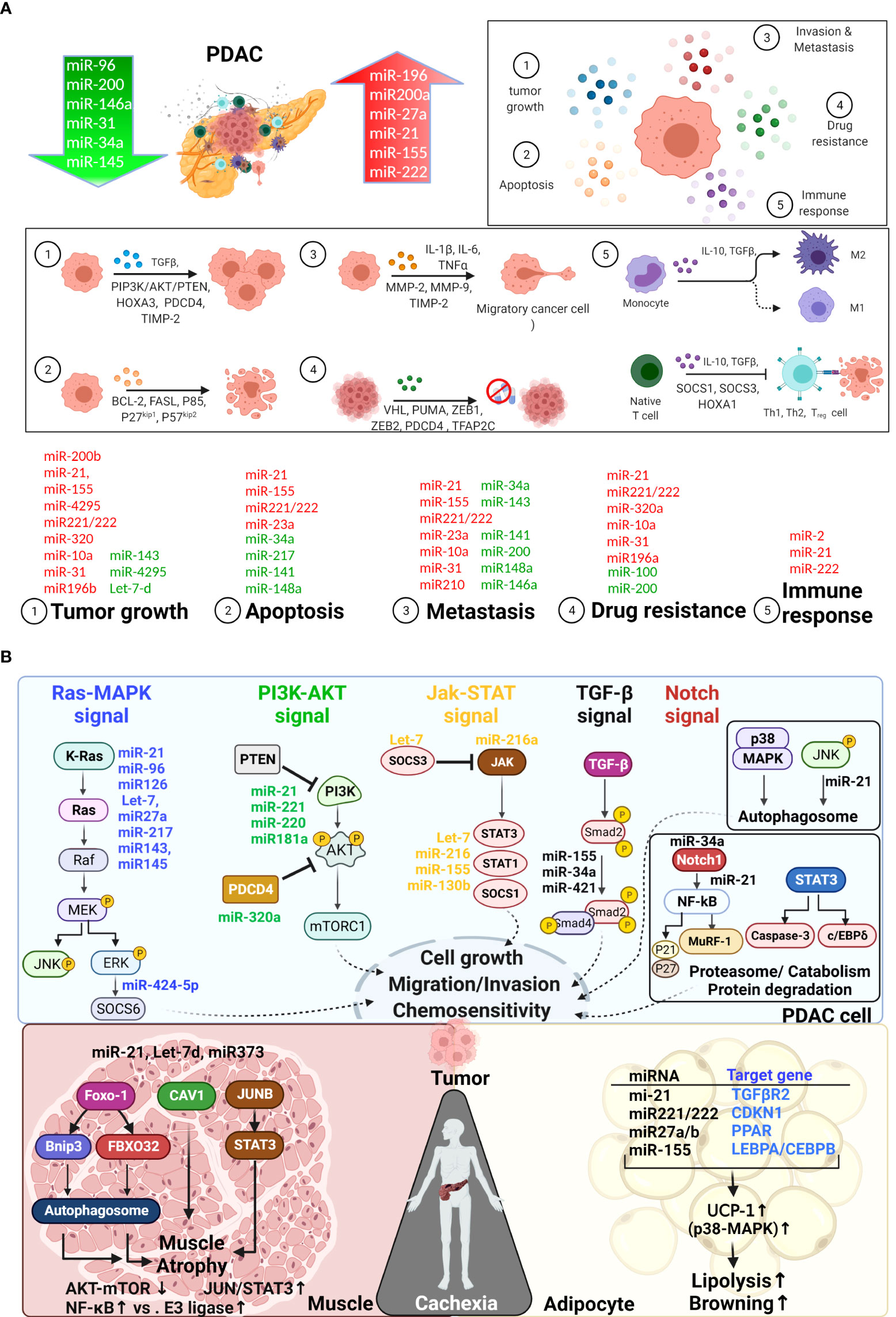
Figure 6 Mechanistic role of miRNAs in PDAC cachexia. (A) Downregulated microRNAs (miRNAs) are indicated in green, and upregulated miRNAs are indicated in red. Based on the literature, miRNAs participate in the regulation of PDAC progression and metastasis, overcoming host immune responses, and the development of chemoresistance. (B). miRNAs associated with PDAC-CC are primarily involved in KRAS-MAPK, PI3K-AKT, JAK-STAT, and TGF-β, NF-κB and p38-MAPK signaling pathway … etc. miRNAs can be detected in tumor and serum and mediate crosstalk in the tumor microenvironment between tumor, muscle, and adipocytes, which are associated with the development of PDAC-CC. Some microRNAs are tissue specific. For example, miR-21 (TLR7-JUN), miR-155, let-7d and miR373 are specific contributed to muscle wasting in PDAC-CC (see Table 6 in detail). The most common genes, such as IL-6R, FOXO1, PDK4, and ZIP14, had been associated with muscle wasting in cachexia. In adipocytes, specific microRNAs may mediate the transcription factors C/EBPβ/δ, C/EBPα, and PPARγ, resulting in adipogenesis.
Recent studies have detected miRNAs in serum, plasma, tissue, and tumors (Table 6). Studies indicates that miRNAs are commonly found in various EVs, such as exosomes, apoptotic bodies, microvesicles (MV), and lipoproteins, allowing them to target cells and contribute to intercellular signaling through endocrine, paracrine, and autocrine pathways (182, 183, 198, 199, 215–217). Losses in muscle proteins and fat mass are the most important signatures of cachexia and can result in the generation of microvesicles containing miRNAs (see Figure 6B: bottom panel). Most studies have identified miRNAs expressed in tumor cells; however, some miRNAs are tissue‐specific or tissue‐enriched, involved in either the active or passive stimulation of metabolic changes and inflammatory responses (Table 6). In PDAC-CC, miR-21, miR221/222, miR27a and miR155 are commonly correlated with muscle and adipocyte wasting through the transcription E3 ubiquitin ligases (mediated by FoxO3 or FoxO1 in muscle), JUN–STAT3, or TGF-β; (Figure 6B: bottom panel). Given the roles of miRNAs in gene expression and the regulation of inflammatory responses and metabolic reprogramming, additional study of miRNAs remains necessary. An ongoing observational trial (NCT05275075) aims to analyze the miRNA profiles in patients with PDAC-CC. The causal roles of miRNAs and molecular mechanisms in cachexia remain under debate and require further discussion and study; however, therapeutic approaches for modifying multiple targets have been suggested (218, 219). The study of miRNAs could also contribute to the development of diagnostic or prognostic biomarkers and new targets for cachexia prevention or treatment.
4 Conclusion
The high prevalence of PDAC-CC may be associated with the unique genetic background (KRAS mutations) and modulators in PDAC, which mainly exacerbate metabolic disruptions, leading to cachexia development. Systemic metabolic alterations mediated by pro-cachectic factors, systemic inflammation, and epigenetic changes, highlighting that PDAC is a systemic disease rather than a single-organ defect. PDAC can induce metabolic disruptions in organs beyond the pancreas. We applied the EOLT hypothesis (33) to emphasize the systemic effects of PDAC, leading to tissue wasting in PDAC-CC. Currently, no FDA-approved agent is able to treat cachexia, although potential treatments are listed in Table 7. However, further studies remain necessary to generate foundational knowledge for the development of additional therapies or understanding the molecular mechanisms of PDAC-CC.
Author contributions
Y-CY and AA drafted manuscript. L-MC, W-CCe, J-CY, H-CL and W-CCa participated in discussion, literature review, supported, and edited manuscript and W-LM initiated and supported the concept. Y-SS and W-LM edited, and final approved the manuscript. All authors contributed to the article and approved the submitted version.
Funding
This work was supported by Ministry of Sciences and Technology, Taiwan (MOST 107-2314-B-039-011; MOST 108-2320-B-039-017; MOST 108-2314-B-039-043-MY3; MOST 108-2314-B-039-052, MOST 111-2320-B-039-011, MOST 111-2314-B-039-062-MY3, MOST 110-2314-B-039-046), and National Health Research Institute, Taiwan (NHRI-EX109-10705BI, NHRI-EX111-11110BI), and China Medical University/Hospital (DMR-109-019, CMU109-MF-26, CMU111-MF-91, CMU111-MF-41, DMR-111-118, DMR-111-238, DMR-110-025, and DMR-111-204) for Dr. Wen Lung Ma, and MOST 110-2314-B-006-106 and MOST 111-2811-B-006-017 for Yan Shen Shan.
Acknowledgments
Authors of this manuscript comply with the ethical guidelines for authorship. All figures created with BioRender.com.
Conflict of interest
The authors declare that the research was conducted in the absence of any commercial or financial relationships that could be construed as a potential conflict of interest.
Publisher’s note
All claims expressed in this article are solely those of the authors and do not necessarily represent those of their affiliated organizations, or those of the publisher, the editors and the reviewers. Any product that may be evaluated in this article, or claim that may be made by its manufacturer, is not guaranteed or endorsed by the publisher.
Glossary
References
1. Rahib L, Smith BD, Aizenberg R, Rosenzweig AB, Fleshman JM, Matrisian LM. Projecting cancer incidence and deaths to 2030: The unexpected burden of thyroid, liver, and pancreas cancers in the united states. Cancer Res (2014) 74(11):2913–21. doi: 10.1158/0008-5472.Can-14-0155
2. Rawla P, Sunkara T, Gaduputi V. Epidemiology of pancreatic cancer: Global trends, etiology and risk factors. World J Oncol (2019) 10(1):10–27. doi: 10.14740/wjon1166
3. Chakrabarti S, Kamgar M, Mahipal A. Systemic therapy of metastatic pancreatic adenocarcinoma: Current status, challenges, and opportunities. Cancers (Basel) (2022) 14(11):2588. doi: 10.3390/cancers14112588
4. Mitsunaga S, Kasamatsu E, Machii K. Incidence and frequency of cancer cachexia during chemotherapy for advanced pancreatic ductal adenocarcinoma. Supportive Care Cancer (2020) 28(11):5271–9. doi: 10.1007/s00520-020-05346-8
5. Hendifar AE, Chang JI, Huang BZ, Tuli R, Wu BU. Cachexia, and not obesity, prior to pancreatic cancer diagnosis worsens survival and is negated by chemotherapy. J Gastrointest Oncol (2018) 9(1):17–23. doi: 10.21037/jgo.2017.11.10
6. Hendifar AE, Petzel MQB, Zimmers TA, Denlinger CS, Matrisian LM, Picozzi VJ, et al. Pancreas cancer-associated weight loss. oncologist (2019) 24(5):691–701. doi: 10.1634/theoncologist.2018-0266
7. Bachmann J, Heiligensetzer M, Krakowski-Roosen H, Büchler MW, Friess H, Martignoni ME. Cachexia worsens prognosis in patients with resectable pancreatic cancer. J gastrointestinal Surg Off J Soc Surg Alimentary Tract (2008) 12(7):1193–201. doi: 10.1007/s11605-008-0505-z
8. Kordes M, Larsson L, Engstrand L, Löhr JM. Pancreatic cancer cachexia: Three dimensions of a complex syndrome. Br J Cancer (2021) 124(10):1623–36. doi: 10.1038/s41416-021-01301-4
9. Poulia KA, Sarantis P, Antoniadou D, Koustas E, Papadimitropoulou A, Papavassiliou AG, et al. Pancreatic cancer and cachexia-metabolic mechanisms and novel insights. Nutrients (2020) 12(6):1543. doi: 10.3390/nu12061543
10. Naumann P, Eberlein J, Farnia B, Hackert T, Debus J, Combs SE. Continued weight loss and sarcopenia predict poor outcomes in locally advanced pancreatic cancer treated with chemoradiation. Cancers (Basel) (2019) 11(5):709. doi: 10.3390/cancers11050709
11. Baracos VE, Martin L, Korc M, Guttridge DC, Fearon KCH. Cancer-associated cachexia. Nat Rev Dis Primers (2018) 4(1):17105. doi: 10.1038/nrdp.2017.105
12. Siddiqui JA, Pothuraju R, Jain M, Batra SK, Nasser MW. Advances in cancer cachexia: Intersection between affected organs, mediators, and pharmacological interventions. Biochim Biophys Acta Rev Cancer (2020) 1873(2):188359. doi: 10.1016/j.bbcan.2020.188359
13. Bachmann J, Büchler MW, Friess H, Martignoni ME. Cachexia in patients with chronic pancreatitis and pancreatic cancer: Impact on survival and outcome. Nutr Cancer (2013) 65(6):827–33. doi: 10.1080/01635581.2013.804580
14. Bachmann J, Ketterer K, Marsch C, Fechtner K, Krakowski-Roosen H, Büchler MW, et al. Pancreatic cancer related cachexia: Influence on metabolism and correlation to weight loss and pulmonary function. BMC Cancer (2009) 9:255. doi: 10.1186/1471-2407-9-255
15. Pausch T, Hartwig W, Hinz U, Swolana T, Bundy BD, Hackert T, et al. Cachexia but not obesity worsens the postoperative outcome after pancreatoduodenectomy in pancreatic cancer. Surgery (2012) 152(3 Suppl 1):S81–8. doi: 10.1016/j.surg.2012.05.028
16. Maccio A, Sanna E, Neri M, Oppi S, Madeddu C. Cachexia as evidence of the mechanisms of resistance and tolerance during the evolution of cancer disease. Int J Mol Sci (2021) 22(6):2890. doi: 10.3390/ijms22062890
17. Wyart E, Bindels LB, Mina E, Menga A, Stanga S, Porporato PE. Cachexia, a systemic disease beyond muscle atrophy. Int J Mol Sci (2020) 21(22):8592. doi: 10.3390/ijms21228592
18. Xu PC, You M, Yu S-Y, Luan Y, Eldani M, Caffrey TC, et al. Visceral adipose tissue remodeling in pancreatic ductal adenocarcinoma cachexia: The role of activin a signaling. Sci Rep (2022) 12(1):1659. doi: 10.1038/s41598-022-05660-7
19. Sah RP, Sharma A, Nagpal S, Patlolla SH, Sharma A, Kandlakunta H, et al. Phases of metabolic and soft tissue changes in months preceding a diagnosis of pancreatic ductal adenocarcinoma. Gastroenterology (2019) 156(6):1742–52. doi: 10.1053/j.gastro.2019.01.039
20. Sun X, Feng X, Wu X, Lu Y, Chen K, Ye Y. Fat wasting is damaging: Role of adipose tissue in cancer-associated cachexia. Front Cell Dev Biol (2020) 8:33. doi: 10.3389/fcell.2020.00033
21. Rupert JE, Narasimhan A, Jengelley DHA, Jiang Y, Liu J, Au E, et al. Tumor-derived IL-6 and trans-signaling among tumor, fat, and muscle mediate pancreatic cancer cachexia. J Exp Med (2021) 218(6):e20190450. doi: 10.1084/jem.20190450
22. Yoo W, Choi H, Son YH, Lee J, Jo S, Jung D, et al. Pancreatic cancer induces muscle wasting by promoting the release of pancreatic adenocarcinoma upregulated factor. Exp Mol Med (2021) 53(3):432–45. doi: 10.1038/s12276-021-00582-2
23. Danai LV, Babic A, Rosenthal MH, Dennstedt EA, Muir A, Lien EC, et al. Altered exocrine function can drive adipose wasting in early pancreatic cancer. Nature (2018) 558(7711):600–4. doi: 10.1038/s41586-018-0235-7
24. Hildebrandt W, Keck J, Schmich S, Bonaterra GA, Wilhelm B, Schwarzbach H, et al. Inflammation and wasting of skeletal muscles in Kras-p53-Mutant mice with intraepithelial neoplasia and pancreatic cancer-when does cachexia start? Cells (2022) 11(10):1607. doi: 10.3390/cells11101607
25. Falconer JS, Fearon KC, Plester CE, Ross JA, Carter DC. Cytokines, the acute-phase response, and resting energy expenditure in cachectic patients with pancreatic cancer. Ann Surg (1994) 219(4):325–31. doi: 10.1097/00000658-199404000-00001
26. Bye A, Wesseltoft-Rao N, Iversen PO, Skjegstad G, Holven KB, Ulven S, et al. Alterations in inflammatory biomarkers and energy intake in cancer cachexia: A prospective study in patients with inoperable pancreatic cancer. Med Oncol (2016) 33(6):54. doi: 10.1007/s12032-016-0768-2
27. Wigmore SJ, Plester CE, Ross JA, Fearon KC. Contribution of anorexia and hypermetabolism to weight loss in anicteric patients with pancreatic cancer. Br J Surg (1997) 84(2):196–7. doi: 10.1046/j.1365-2168.1997.02525.x
28. Ronga I, Gallucci F, Riccardi F, Uomo G. Anorexia-cachexia syndrome in pancreatic cancer: Recent advances and new pharmacological approach. Adv Med Sci (2014) 59(1):1–6. doi: 10.1016/j.advms.2013.11.001
29. Fearon KC, Glass DJ, Guttridge DC. Cancer cachexia: Mediators, signaling, and metabolic pathways. Cell Metab (2012) 16(2):153–66. doi: 10.1016/j.cmet.2012.06.011
30. DeBerardinis RJ, Lum JJ, Hatzivassiliou G, Thompson CB. The biology of cancer: Metabolic reprogramming fuels cell growth and proliferation. Cell Metab (2008) 7(1):11–20. doi: 10.1016/j.cmet.2007.10.002
31. Lee YM, Chang WC, Ma WL. Hypothesis: Solid tumours behave as systemic metabolic dictators. J Cell Mol Med (2016) 20(6):1076–85. doi: 10.1111/jcmm.12794
32. Argilés JM, Stemmler B, López-Soriano FJ, Busquets S. Inter-tissue communication in cancer cachexia. Nat Rev Endocrinol (2018) 15(1):9–20. doi: 10.1038/s41574-018-0123-0
33. Park J, Euhus DM, Scherer PE. Paracrine and endocrine effects of adipose tissue on cancer development and progression. Endocr Rev (2011) 32(4):550–70. doi: 10.1210/er.2010-0030
34. Yang J, Zhang Z, Zhang Y, Ni X, Zhang G, Cui X, et al. Zip4 promotes muscle wasting and cachexia in mice with orthotopic pancreatic tumors by stimulating Rab27b-regulated release of extracellular vesicles from cancer cells. Gastroenterology (2019) 156(3):722–34.e6. doi: 10.1053/j.gastro.2018.10.026
35. WHO Expert Consultation. WHO Expert Consultation Appropriate body-mass index for Asian populations and its implications for policy and intervention strategies. Lancet (2004) 363(9403):157–63. doi: 10.1016/s0140-6736(03)15268-3
36. Bozzetti F, Mariani L. Defining and classifying cancer cachexia: A proposal by the scrinio working group. JPEN J Parenter Enteral Nutr (2009) 33(4):361–7. doi: 10.1177/0148607108325076
37. Fearon KC, Voss AC, Hustead DS. Definition of cancer cachexia: Effect of weight loss, reduced food intake, and systemic inflammation on functional status and prognosis. Am J Clin Nutr (2006) 83(6):1345–50. doi: 10.1093/ajcn/83.6.1345
38. Fearon K, Strasser F, Anker SD, Bosaeus I, Bruera E, Fainsinger RL, et al. Definition and classification of cancer cachexia: An international consensus. Lancet Oncol (2011) 12(5):489–95. doi: 10.1016/S1470-2045(10)70218-7
39. Douglas E, McMillan DC. Towards a simple objective framework for the investigation and treatment of cancer cachexia: The Glasgow prognostic score. Cancer Treat Rev (2014) 40(6):685–91. doi: 10.1016/j.ctrv.2013.11.007
40. Argilés JM, López-Soriano FJ, Toledo M, Betancourt A, Serpe R, Busquets S. The cachexia score (Casco): A new tool for staging cachectic cancer patients. J cachexia sarcopenia Muscle (2011) 2(2):87–93. doi: 10.1007/s13539-011-0027-5
41. Evans WJ, Morley JE, Argilés J, Bales C, Baracos V, Guttridge D, et al. Cachexia: A new definition. Clin Nutr (Edinburgh Scotland) (2008) 27(6):793–9. doi: 10.1016/j.clnu.2008.06.013
42. Zhou T, Wang B, Liu H, Yang K, Thapa S, Zhang H, et al. Development and validation of a clinically applicable score to classify cachexia stages in advanced cancer patients. J cachexia sarcopenia Muscle (2018) 9(2):306–14. doi: 10.1002/jcsm.12275
43. Jafri SH, Previgliano C, Khandelwal K, Shi R. Cachexia index in advanced non-Small-Cell lung cancer patients. Clin Med Insights Oncol (2015) 9:87–93. doi: 10.4137/cmo.S30891
44. Goh MJ, Kang W, Jeong WK, Sinn DH, Gwak G-Y, Paik Y-H, et al. Prognostic significance of cachexia index in patients with advanced hepatocellular carcinoma treated with systemic chemotherapy. Sci Rep (2022) 12(1):7647. doi: 10.1038/s41598-022-11736-1
45. Martin L, Muscaritoli M, Bourdel-Marchasson I, Kubrak C, Laird B, Gagnon B, et al. Diagnostic criteria for cancer cachexia: Reduced food intake and inflammation predict weight loss and survival in an international, multi-cohort analysis. J cachexia sarcopenia Muscle (2021) 12(5):1189–202. doi: 10.1002/jcsm.12756
46. Ottery FD. Definition of standardized nutritional assessment and interventional pathways in oncology. Nutr (Burbank Los Angeles County Calif) (1996) 12(1 Suppl):S15–9. doi: 10.1016/0899-9007(96)90011-8
47. Wheelwright SJ, Hopkinson JB, Darlington AS, Fitzsimmons DF, Fayers P, Balstad TR, et al. Development of the eortc qlq-Cax24, a questionnaire for cancer patients with cachexia. J Pain Symptom Manage (2017) 53(2):232–42. doi: 10.1016/j.jpainsymman.2016.09.010
48. Gioulbasanis I, Georgoulias P, Vlachostergios PJ, Baracos V, Ghosh S, Giannousi Z, et al. Mini nutritional assessment (Mna) and biochemical markers of cachexia in metastatic lung cancer patients: Interrelations and associations with prognosis. Lung Cancer (2011) 74(3):516–20. doi: 10.1016/j.lungcan.2011.05.009
49. LeBlanc TW, Samsa GP, Wolf SP, Locke SC, Cella DF, Abernethy AP. Validation and real-world assessment of the functional assessment of anorexia-cachexia therapy (Faact) scale in patients with advanced non-small cell lung cancer and the cancer anorexia-cachexia syndrome (Cacs). Supportive Care Cancer (2015) 23(8):2341–7. doi: 10.1007/s00520-015-2606-z
50. Proctor MJ, Morrison DS, Talwar D, Balmer SM, O'Reilly DS, Foulis AK, et al. An inflammation-based prognostic score (Mgps) predicts cancer survival independent of tumour site: A Glasgow inflammation outcome study. Br J Cancer (2011) 104(4):726–34. doi: 10.1038/sj.bjc.6606087
51. Martin L, Senesse P, Gioulbasanis I, Antoun S, Bozzetti F, Deans C, et al. Diagnostic criteria for the classification of cancer-associated weight loss. J Clin Oncol (2015) 33(1):90–9. doi: 10.1200/jco.2014.56.1894
52. Blauwhoff-Buskermolen S, Langius JAE, Becker A, Verheul HMW, de van der Schueren MAE. The influence of different muscle mass measurements on the diagnosis of cancer cachexia. J cachexia sarcopenia Muscle (2017) 8(4):615–22. doi: 10.1002/jcsm.12200
53. Walowski CO, Braun W, Maisch MJ, Jensen B, Peine S, Norman K, et al. Reference values for skeletal muscle mass - current concepts and methodological considerations. Nutrients (2020) 12(3):755. doi: 10.3390/nu12030755
54. McGovern J, Dolan RD, Horgan PG, Laird BJ, McMillan DC. Computed tomography-defined low skeletal muscle index and density in cancer patients: Observations from a systematic review. J cachexia sarcopenia Muscle (2021) 12(6):1408–17. doi: 10.1002/jcsm.12831
55. Laskey MA. Dual-energy X-ray absorptiometry and body composition. Nutr (Burbank Los Angeles County Calif) (1996) 12(1):45–51. doi: 10.1016/0899-9007(95)00017-8
56. Tuca A, Jimenez-Fonseca P, Gascón P. Clinical evaluation and optimal management of cancer cachexia. Crit Rev Oncol Hematol (2013) 88(3):625–36. doi: 10.1016/j.critrevonc.2013.07.015
57. Strasser F, Müller-Käser I, Dietrich D. Evaluating cognitive, emotional, and physical fatigue domains in daily practice by single-item questions in patients with advanced cancer: A cross-sectional pragmatic study. J Pain Symptom Manage (2009) 38(4):505–14. doi: 10.1016/j.jpainsymman.2008.12.009
58. Walsh D, Mahmoud F, Barna B. Assessment of nutritional status and prognosis in advanced cancer: Interleukin-6, c-reactive protein, and the prognostic and inflammatory nutritional index. Support Care Cancer (2003) 11(1):60–2. doi: 10.1007/s00520-002-0390-z
59. Buzby GP, Mullen JL, Matthews DC, Hobbs CL, Rosato EF. Prognostic nutritional index in gastrointestinal surgery. Am J Surg (1980) 139(1):160–7. doi: 10.1016/0002-9610(80)90246-9
60. Purcell SA, Elliott SA, Baracos VE, Chu QS, Prado CM. Key determinants of energy expenditure in cancer and implications for clinical practice. Eur J Clin Nutr (2016) 70(11):1230–8. doi: 10.1038/ejcn.2016.96
61. Cameron ME, Yakovenko A, Trevino JG. Glucose and lactate transport in pancreatic cancer: Glycolytic metabolism revisited. J Oncol (2018) 2018:6214838. doi: 10.1155/2018/6214838
62. Qin C, Yang G, Yang J, Ren B, Wang H, Chen G, et al. Metabolism of pancreatic cancer: Paving the way to better anticancer strategies. Mol Cancer (2020) 19(1):50. doi: 10.1186/s12943-020-01169-7
63. Guillaumond F, Leca J, Olivares O, Lavaut MN, Vidal N, Berthezène P, et al. Strengthened glycolysis under hypoxia supports tumor symbiosis and hexosamine biosynthesis in pancreatic adenocarcinoma. Proc Natl Acad Sci United States America (2013) 110(10):3919–24. doi: 10.1073/pnas.1219555110
64. Ying H, Kimmelman AC, Lyssiotis CA, Hua S, Chu GC, Fletcher-Sananikone E, et al. Oncogenic Kras maintains pancreatic tumors through regulation of anabolic glucose metabolism. Cell (2012) 149(3):656–70. doi: 10.1016/j.cell.2012.01.058
65. Baek G, Tse YF, Hu Z, Cox D, Buboltz N, McCue P, et al. MCT4 defines a glycolytic subtype of pancreatic cancer with poor prognosis and unique metabolic dependencies. Cell Rep (2014) 9(6):2233–49. doi: 10.1016/j.celrep.2014.11.025
66. Wang F, Liu H, Hu L, Liu Y, Duan Y, Cui R, et al. The warburg effect in human pancreatic cancer cells triggers cachexia in athymic mice carrying the cancer cells. BMC Cancer (2018) 18(1):360. doi: 10.1186/s12885-018-4271-3
67. Moses AG, Maingay J, Sangster K, Fearon KC, Ross JA. Pro-inflammatory cytokine release by peripheral blood mononuclear cells from patients with advanced pancreatic cancer: Relationship to acute phase response and survival. Oncol Rep (2009) 21(4):1091–5. doi: 10.3892/or_00000328
68. Martignoni ME, Kunze P, Hildebrandt W, Künzli B, Berberat P, Giese T, et al. Role of mononuclear cells and inflammatory cytokines in pancreatic cancer-related cachexia. Clin Cancer Res an Off J Am Assoc Cancer Res (2005) 11(16):5802–8. doi: 10.1158/1078-0432.Ccr-05-0185
69. Burfeind KG, Zhu X, Norgard MA, Levasseur PR, Huisman C, Buenafe AC, et al. Circulating myeloid cells invade the central nervous system to mediate cachexia during pancreatic cancer. Elife (2020) 9:e54095. doi: 10.7554/eLife.54095
70. Oliff A, Defeo-Jones D, Boyer M, Martinez D, Kiefer D, Vuocolo G, et al. Tumors secreting human TNF/Cachectin induce cachexia in mice. Cell (1987) 50(4):555–63. doi: 10.1016/0092-8674(87)90028-6
71. Olson B, Zhu X, Norgard MA, Levasseur PR, Butler JT, Buenafe A, et al. Lipocalin 2 mediates appetite suppression during pancreatic cancer cachexia. Nat Commun (2021) 12(1):2057. doi: 10.1038/s41467-021-22361-3
72. Peixoto da Silva S, Santos JMO, Costa ESMP, Gil da Costa RM, Medeiros R. Cancer cachexia and its pathophysiology: Links with sarcopenia, anorexia and asthenia. J cachexia sarcopenia Muscle (2020) 11(3):619–35. doi: 10.1002/jcsm.12528
73. O'Riordain MG, Falconer JS, Maingay J, Fearon KC, Ross JA. Peripheral blood cells from weight-losing cancer patients control the hepatic acute phase response by a primarily interleukin-6 dependent mechanism. Int J Oncol (1999) 15(4):823–7. doi: 10.3892/ijo.15.4.823
74. Ubachs J, Ziemons J, Soons Z, Aarnoutse R, van Dijk DPJ, Penders J, et al. Gut microbiota and short-chain fatty acid alterations in cachectic cancer patients. J cachexia sarcopenia Muscle (2021) 12(6):2007–21. doi: 10.1002/jcsm.12804
75. Talbert EE, Lewis HL, Farren MR, Ramsey ML, Chakedis JM, Rajasekera P, et al. Circulating monocyte chemoattractant protein-1 (Mcp-1) is associated with cachexia in treatment-naïve pancreatic cancer patients. J cachexia sarcopenia Muscle (2018) 9(2):358–68. doi: 10.1002/jcsm.12251
76. Moshayedi N, Yang J, Lagishetty V, Jacobs J, Placencio-Hickok V, Osipov A, et al. Fecal microbiome composition in pancreatic cancer cachexia and response to nutrition support. J Clin Oncol (2021) 39(15_suppl):4129. doi: 10.1200/JCO.2021.39.15_suppl.4129
77. Chandra V, McAllister F. Therapeutic potential of microbial modulation in pancreatic cancer. Gut (2021) 70(8):1419–25. doi: 10.1136/gutjnl-2019-319807
78. Wei MY, Shi S, Liang C, Meng QC, Hua J, Zhang YY, et al. The microbiota and microbiome in pancreatic cancer: More influential than expected. Mol Cancer (2019) 18(1):97. doi: 10.1186/s12943-019-1008-0
79. Riquelme E, Zhang Y, Zhang L, Montiel M, Zoltan M, Dong W, et al. Tumor microbiome diversity and composition influence pancreatic cancer outcomes. Cell (2019) 178(4):795–806.e12. doi: 10.1016/j.cell.2019.07.008
80. Martignoni ME, Dimitriu C, Bachmann J, Krakowski-Rosen H, Ketterer K, Kinscherf R, et al. Liver macrophages contribute to pancreatic cancer-related cachexia. Oncol Rep (2009) 21(2):363–9. doi: 10.3892/or_00000231
81. Hou Y-C, Wang C-J, Chao Y-J, Chen H-Y, Wang H-C, Tung H-L, et al. Elevated serum interleukin-8 level correlates with cancer-related cachexia and sarcopenia: An indicator for pancreatic cancer outcomes. J Clin Med (2018) 7(12):502. doi: 10.3390/jcm7120502
82. Yan L, Raj P, Yao W, Ying H. Glucose metabolism in pancreatic cancer. Cancers (2019) 11(10):1460. doi: 10.3390/cancers11101460
83. Son J, Lyssiotis CA, Ying H, Wang X, Hua S, Ligorio M, et al. Glutamine supports pancreatic cancer growth through a Kras-regulated metabolic pathway. Nature (2013) 496(7443):101–5. doi: 10.1038/nature12040
84. Kong SC, Nøhr-Nielsen A, Zeeberg K, Reshkin SJ, Hoffmann EK, Novak I, et al. Monocarboxylate transporters MCT1 and MCT4 regulate migration and invasion of pancreatic ductal adenocarcinoma cells. Pancreas (2016) 45(7):1036–47. doi: 10.1097/mpa.0000000000000571
85. Visavadiya NP, Rossiter HB, Khamoui AV. Distinct glycolytic pathway regulation in liver, tumour and skeletal muscle of mice with cancer cachexia. Cell Biochem Funct (2021) 39(6):802–12. doi: 10.1002/cbf.3652
86. Han X, Raun SH, Carlsson M, Sjøberg KA, Henriquez-Olguín C, Ali M, et al. Cancer causes metabolic perturbations associated with reduced insulin-stimulated glucose uptake in peripheral tissues and impaired muscle microvascular perfusion. Metabolism (2020) 105:154169. doi: 10.1016/j.metabol.2020.154169
87. Yu M, Zhou Q, Zhou Y, Fu Z, Tan L, Ye X, et al. Metabolic phenotypes in pancreatic cancer. PloS One (2015) 10(2):e0115153. doi: 10.1371/journal.pone.0115153
88. Tan CR, Yaffee PM, Jamil LH, Lo SK, Nissen N, Pandol SJ, et al. Pancreatic cancer cachexia: A review of mechanisms and therapeutics. Front Physiol (2014) 5:88. doi: 10.3389/fphys.2014.00088
89. Ferro F, Servais S, Besson P, Roger S, Dumas JF, Brisson L. Autophagy and mitophagy in cancer metabolic remodelling. Semin Cell Dev Biol (2020) 98:129–38. doi: 10.1016/j.semcdb.2019.05.029
90. de Castro GS, Simoes E, Lima J, Ortiz-Silva M, Festuccia WT, Tokeshi F, et al. Human cachexia induces changes in mitochondria, autophagy and apoptosis in the skeletal muscle. Cancers (Basel) (2019) 11(9):1264. doi: 10.3390/cancers11091264
91. Reyes-Castellanos G, Abdel Hadi N, Carrier A. Autophagy contributes to metabolic reprogramming and therapeutic resistance in pancreatic tumors. Cells (2022) 11(3):426. doi: 10.3390/cells11030426
92. Kinsey CG, Camolotto SA, Boespflug AM, Guillen KP, Foth M, Truong A, et al. Protective autophagy elicited by Raf→Mek→Erk inhibition suggests a treatment strategy for Ras-driven cancers. Nat Med (2019) 25(4):620–7. doi: 10.1038/s41591-019-0367-9
93. Yoshida GJ. Metabolic reprogramming: The emerging concept and associated therapeutic strategies. J Exp Clin Cancer Res (2015) 34:111. doi: 10.1186/s13046-015-0221-y
94. Blum R, Kloog Y. Metabolism addiction in pancreatic cancer. Cell Death Dis (2014) 5(2):e1065. doi: 10.1038/cddis.2014.38
95. Slot IG, Schols AM, de Theije CC, Snepvangers FJ, Gosker HR. Alterations in skeletal muscle oxidative phenotype in mice exposed to 3 weeks of normobaric hypoxia. J Cell Physiol (2016) 231(2):377–92. doi: 10.1002/jcp.25083
96. Hao X, Ren Y, Feng M, Wang Q, Wang Y. Metabolic reprogramming due to hypoxia in pancreatic cancer: Implications for tumor formation, immunity, and more. Biomedicine Pharmacotherapy (2021) 141:111798. doi: 10.1016/j.biopha.2021.111798
97. Mayers JR, Wu C, Clish CB, Kraft P, Torrence ME, Fiske BP, et al. Elevation of circulating branched-chain amino acids is an early event in human pancreatic adenocarcinoma development. Nat Med (2014) 20(10):1193–8. doi: 10.1038/nm.3686
98. Lee JH, Cho YR, Kim JH, Kim J, Nam HY, Kim SW, et al. Branched-chain amino acids sustain pancreatic cancer growth by regulating lipid metabolism. Exp Mol Med (2019) 51(11):1–11. doi: 10.1038/s12276-019-0350-z
99. Winnard PT Jr., Bharti SK, Sharma RK, Krishnamachary B, Mironchik Y, Penet M-F, et al. Brain metabolites in cholinergic and glutamatergic pathways are altered by pancreatic cancer cachexia. J cachexia sarcopenia Muscle (2020) 11(6):1487–500. doi: 10.1002/jcsm.12621
100. Perera RM, Bardeesy N. Pancreatic cancer metabolism: Breaking it down to build it back up. Cancer Discovery (2015) 5(12):1247–61. doi: 10.1158/2159-8290.Cd-15-0671
101. Zeman M, Macášek J, Burda M, Tvrzická E, Vecka M, Krechler T, et al. Chronic pancreatitis and the composition of plasma phosphatidylcholine fatty acids. Prostaglandins Leukot Essent Fatty Acids (2016) 108:38–44. doi: 10.1016/j.plefa.2016.03.012
102. Yu M, Liu H, Duan Y, Zhang D, Li S, Wang F. Four types of fatty acids exert differential impact on pancreatic cancer growth. Cancer Lett (2015) 360(2):187–94. doi: 10.1016/j.canlet.2015.02.002
103. Zhang G, He P, Tan H, Budhu A, Gaedcke J, Ghadimi BM, et al. Integration of metabolomics and transcriptomics revealed a fatty acid network exerting growth inhibitory effects in human pancreatic cancer. Clin Cancer Res an Off J Am Assoc Cancer Res (2013) 19(18):4983–93. doi: 10.1158/1078-0432.Ccr-13-0209
104. Kays JK, Shahda S, Stanley M, Bell TM, O'Neill BH, Kohli MD, et al. Three cachexia phenotypes and the impact of fat-only loss on survival in folfirinox therapy for pancreatic cancer. J cachexia sarcopenia Muscle (2018) 9(4):673–84. doi: 10.1002/jcsm.12307
105. Gumpper-Fedus K, Hart PA, Belury MA, Crowe O, Cole RM, Pita Grisanti V, et al. Altered plasma fatty acid abundance is associated with cachexia in treatment-naïve pancreatic cancer. Cells (2022) 11(5):910. doi: 10.3390/cells11050910
106. Lee J-S, Oh S-J, Choi H-J, Kang JH, Lee S-H, Ha JS, et al. ATP production relies on fatty acid oxidation rather than glycolysis in pancreatic ductal adenocarcinoma. Cancers (2020) 12(9):2477. doi: 10.3390/cancers12092477
107. Das SK, Eder S, Schauer S, Diwoky C, Temmel H, Guertl B, et al. Adipose triglyceride lipase contributes to cancer-associated cachexia. Science (2011) 333(6039):233–8. doi: 10.1126/science.1198973
108. Mitsunaga S, Ikeda M, Shimizu S, Ohno I, Furuse J, Inagaki M, et al. Serum levels of IL-6 and IL-1β can predict the efficacy of gemcitabine in patients with advanced pancreatic cancer. Br J Cancer (2013) 108(10):2063–9. doi: 10.1038/bjc.2013.174
109. McDonald OG, Li X, Saunders T, Tryggvadottir R, Mentch SJ, Warmoes MO, et al. Epigenomic reprogramming during pancreatic cancer progression links anabolic glucose metabolism to distant metastasis. Nat Genet (2017) 49(3):367–76. doi: 10.1038/ng.3753
110. Carrer A, Trefely S, Zhao S, Campbell SL, Norgard RJ, Schultz KC, et al. Acetyl-CoA metabolism supports multistep pancreatic tumorigenesis. Cancer Discovery (2019) 9(3):416–35. doi: 10.1158/2159-8290.Cd-18-0567
111. Gaglio D, Metallo CM, Gameiro PA, Hiller K, Danna LS, Balestrieri C, et al. Oncogenic K-Ras decouples glucose and glutamine metabolism to support cancer cell growth. Mol Syst Biol (2011) 7:523. doi: 10.1038/msb.2011.56
112. Santana-Codina N, Roeth AA, Zhang Y, Yang A, Mashadova O, Asara JM, et al. Oncogenic Kras supports pancreatic cancer through regulation of nucleotide synthesis. Nat Commun (2018) 9(1):4945. doi: 10.1038/s41467-018-07472-8
113. Yang J, Wang F, Chen X, Qiu S, Cui L, Hu L. Β-Pentagalloyl-Glucose sabotages pancreatic cancer cells and ameliorates cachexia in tumor-bearing mice. Am J Chin Med (2019) 47(3):675–89. doi: 10.1142/s0192415x19500356
114. Altman BJ, Stine ZE, Dang CV. From Krebs to clinic: Glutamine metabolism to cancer therapy. Nat Rev Cancer (2016) 16(10):619–34. doi: 10.1038/nrc.2016.71
115. Sousa CM, Biancur DE, Wang X, Halbrook CJ, Sherman MH, Zhang L, et al. Pancreatic stellate cells support tumour metabolism through autophagic alanine secretion. Nature (2016) 536(7617):479–83. doi: 10.1038/nature19084
116. Ancrile B, Lim KH, Counter CM. Oncogenic Ras-induced secretion of IL6 is required for tumorigenesis. Genes Dev (2007) 21(14):1714–9. doi: 10.1101/gad.1549407
117. O'Hayer KM, Brady DC, Counter CM. ELR+ CXC chemokines and oncogenic Ras-mediated tumorigenesis. Carcinogenesis (2009) 30(11):1841–7. doi: 10.1093/carcin/bgp198
118. Ju HQ, Ying H, Tian T, Ling J, Fu J, Lu Y, et al. Mutant Kras- and p16-regulated NOX4 activation overcomes metabolic checkpoints in development of pancreatic ductal adenocarcinoma. Nat Commun (2017) 8:14437. doi: 10.1038/ncomms14437
119. Perera RM, Stoykova S, Nicolay BN, Ross KN, Fitamant J, Boukhali M, et al. Transcriptional control of autophagy-lysosome function drives pancreatic cancer metabolism. Nature (2015) 524(7565):361–5. doi: 10.1038/nature14587
120. Liang C, Shi S, Qin Y, Meng Q, Hua J, Hu Q, et al. Localisation of PGK1 determines metabolic phenotype to balance metastasis and proliferation in patients with SMAD4-negative pancreatic cancer. Gut (2020) 69(5):888–900. doi: 10.1136/gutjnl-2018-317163
121. Butera G, Pacchiana R, Mullappilly N, Margiotta M, Bruno S, Conti P, et al. Mutant p53 prevents gapdh nuclear translocation in pancreatic cancer cells favoring glycolysis and 2-deoxyglucose sensitivity. Biochim Biophys Acta Mol Cell Res (2018) 1865(12):1914–23. doi: 10.1016/j.bbamcr.2018.10.005
122. Bensaad K, Tsuruta A, Selak MA, Vidal MN, Nakano K, Bartrons R, et al. TIGAR, a p53-inducible regulator of glycolysis and apoptosis. Cell (2006) 126(1):107–20. doi: 10.1016/j.cell.2006.05.036
123. Delitto D, Judge SM, Delitto AE, Nosacka RL, Rocha FG, DiVita BB, et al. Human pancreatic cancer xenografts recapitulate key aspects of cancer cachexia. Oncotarget (2017) 8(1):1177–89. doi: 10.18632/oncotarget.13593
124. Winnard PT Jr., Bharti SK, Penet MF, Marik R, Mironchik Y, Wildes F, et al. Detection of pancreatic cancer-induced cachexia using a fluorescent myoblast reporter system and analysis of metabolite abundance. Cancer Res (2016) 76(6):1441–50. doi: 10.1158/0008-5472.can-15-1740
125. Shukla SK, Dasgupta A, Mehla K, Gunda V, Vernucci E, Souchek J, et al. Silibinin-mediated metabolic reprogramming attenuates pancreatic cancer-induced cachexia and tumor growth. Oncotarget (2015) 6(38):41146–61. doi: 10.18632/oncotarget.5843
126. Shukla SK, Gebregiworgis T, Purohit V, Chaika NV, Gunda V, Radhakrishnan P, et al. Metabolic reprogramming induced by ketone bodies diminishes pancreatic cancer cachexia. Cancer Metab (2014) 2:18–. doi: 10.1186/2049-3002-2-18
127. Jones-Bolin S, Ruggeri B. Orthotopic model of human pancreatic ductal adenocarcinoma and cancer cachexia in nude mice. Curr Protoc Pharmacol (2007), 14.3.1–14.3.21. doi: 10.1002/0471141755.ph1403s37
128. Togashi Y, Kogita A, Sakamoto H, Hayashi H, Terashima M, de Velasco MA, et al. Activin signal promotes cancer progression and is involved in cachexia in a subset of pancreatic cancer. Cancer Lett (2015) 356(2 Pt B):819–27. doi: 10.1016/j.canlet.2014.10.037
129. Greco SH, Tomkötter L, Vahle AK, Rokosh R, Avanzi A, Mahmood SK, et al. TGF-β blockade reduces mortality and metabolic changes in a validated murine model of pancreatic cancer cachexia. PloS One (2015) 10(7):e0132786. doi: 10.1371/journal.pone.0132786
130. Talbert EE, Cuitiño MC, Ladner KJ, Rajasekerea PV, Siebert M, Shakya R, et al. Modeling human cancer-induced cachexia. Cell Rep (2019) 28(6):1612–22.e4. doi: 10.1016/j.celrep.2019.07.016
131. Nosacka RL, Delitto AE, Delitto D, Patel R, Judge SM, Trevino JG, et al. Distinct cachexia profiles in response to human pancreatic tumours in mouse limb and respiratory muscle. J cachexia sarcopenia Muscle (2020) 11(3):820–37. doi: 10.1002/jcsm.12550
132. Delitto D, Pham K, Vlada AC, Sarosi GA, Thomas RM, Behrns KE, et al. Patient-derived xenograft models for pancreatic adenocarcinoma demonstrate retention of tumor morphology through incorporation of murine stromal elements. Am J Pathol (2015) 185(5):1297–303. doi: 10.1016/j.ajpath.2015.01.016
133. Jiang SM, Wu JH, Jia L. Intervention of mirtazapine on gemcitabine-induced mild cachexia in nude mice with pancreatic carcinoma xenografts. World J Gastroenterol (2012) 18(22):2867–71. doi: 10.3748/wjg.v18.i22.2867
134. Shukla SK, Markov SD, Attri KS, Vernucci E, King RJ, Dasgupta A, et al. Macrophages potentiate STAT3 signaling in skeletal muscles and regulate pancreatic cancer cachexia. Cancer Lett (2020) 484:29–39. doi: 10.1016/j.canlet.2020.04.017
135. Michaelis KA, Zhu X, Burfeind KG, Krasnow SM, Levasseur PR, Morgan TK, et al. Establishment and characterization of a novel murine model of pancreatic cancer cachexia. J cachexia sarcopenia Muscle (2017) 8(5):824–38. doi: 10.1002/jcsm.12225
136. Gilabert M, Calvo E, Airoldi A, Hamidi T, Moutardier V, Turrini O, et al. Pancreatic cancer-induced cachexia is Jak2-dependent in mice. J Cell Physiol (2014) 229(10):1437–43. doi: 10.1002/jcp.24580
137. Henderson SE, Makhijani N, Mace TA. Pancreatic cancer-induced cachexia and relevant mouse models. Pancreas (2018) 47(8):937–45. doi: 10.1097/mpa.0000000000001124
138. Wigmore SJ, Plester CE, Richardson RA, Fearon KC. Changes in nutritional status associated with unresectable pancreatic cancer. Br J Cancer (1997) 75(1):106–9. doi: 10.1038/bjc.1997.17
139. Yakovenko A, Cameron M, Trevino JG. Molecular therapeutic strategies targeting pancreatic cancer induced cachexia. World J Gastrointest Surg (2018) 10(9):95–106. doi: 10.4240/wjgs.v10.i9.95
140. Zhu X, Burfeind KG, Michaelis KA, Braun TP, Olson B, Pelz KR, et al. MyD88 signalling is critical in the development of pancreatic cancer cachexia. J cachexia sarcopenia Muscle (2019) 10(2):378–90. doi: 10.1002/jcsm.12377
141. Markov SD, Gonzalez D, Mehla K. Preclinical models for studying the impact of macrophages on cancer cachexia. Curr Protoc Pharmacol (2020) 91(1):e80. doi: 10.1002/cpph.80
142. Niu J, Li Z, Peng B, Chiao PJ. Identification of an autoregulatory feedback pathway involving interleukin-1alpha in induction of constitutive NF-kappaB activation in pancreatic cancer cells. J Biol Chem (2004) 279(16):16452–62. doi: 10.1074/jbc.M309789200
143. Biffi G, Oni TE, Spielman B, Hao Y, Elyada E, Park Y, et al. IL1-induced JAK/STAT signaling is antagonized by TGFβ to shape CAF heterogeneity in pancreatic ductal adenocarcinoma. Cancer Discovery (2019) 9(2):282–301. doi: 10.1158/2159-8290.Cd-18-0710
144. Tjomsland V, Spångeus A, Välilä J, Sandström P, Borch K, Druid H, et al. Interleukin 1α sustains the expression of inflammatory factors in human pancreatic cancer microenvironment by targeting cancer-associated fibroblasts. Neoplasia (2011) 13(8):664–75. doi: 10.1593/neo.11332
145. Zhao X, Fan W, Xu Z, Chen H, He Y, Yang G, et al. Inhibiting tumor necrosis factor-alpha diminishes desmoplasia and inflammation to overcome chemoresistance in pancreatic ductal adenocarcinoma. Oncotarget (2016) 7(49):81110–22. doi: 10.18632/oncotarget.13212
146. Zhang D, Li L, Jiang H, Li Q, Wang-Gillam A, Yu J, et al. Tumor-stroma IL1β-Irak4 feedforward circuitry drives tumor fibrosis, chemoresistance, and poor prognosis in pancreatic cancer. Cancer Res (2018) 78(7):1700–12. doi: 10.1158/0008-5472.CAN-17-1366
147. Fogelman DR, Morris J, Xiao L, Hassan M, Vadhan S, Overman M, et al. A predictive model of inflammatory markers and patient-reported symptoms for cachexia in newly diagnosed pancreatic cancer patients. Support Care Cancer (2017) 25(6):1809–17. doi: 10.1007/s00520-016-3553-z
148. Zhang D, Zhou Y, Wu L, Wang S, Zheng H, Yu B, et al. Association of IL-6 gene polymorphisms with cachexia susceptibility and survival time of patients with pancreatic cancer. Ann Clin Lab Sci (2008) 38(2):113–9.
149. Okada S, Okusaka T, Ishii H, Kyogoku A, Yoshimori M, Kajimura N, et al. Elevated serum interleukin-6 levels in patients with pancreatic cancer. Jpn J Clin Oncol (1998) 28(1):12–5. doi: 10.1093/jjco/28.1.12
150. Ebrahimi B, Tucker SL, Li D, Abbruzzese JL, Kurzrock R. Cytokines in pancreatic carcinoma: Correlation with phenotypic characteristics and prognosis. Cancer (2004) 101(12):2727–36. doi: 10.1002/cncr.20672
151. Holmer R, Goumas FA, Waetzig GH, Rose-John S, Kalthoff H. Interleukin-6: A villain in the drama of pancreatic cancer development and progression. Hepatobiliary Pancreat Dis Int (2014) 13(4):371–80. doi: 10.1016/s1499-3872(14)60259-9
152. Ramsey ML, Talbert E, Ahn D, Bekaii-Saab T, Badi N, Bloomston PM, et al. Circulating interleukin-6 is associated with disease progression, but not cachexia in pancreatic cancer. Pancreatology (2019) 19(1):80–7. doi: 10.1016/j.pan.2018.11.002
153. Daas SI, Rizeq BR, Nasrallah GK. Adipose tissue dysfunction in cancer cachexia. J Cell Physiol (2018) 234(1):13–22. doi: 10.1002/jcp.26811
154. Vaes RDW, van Dijk DPJ, Welbers TTJ, Blok MJ, Aberle MR, Heij L, et al. Generation and initial characterization of novel tumour organoid models to study human pancreatic cancer-induced cachexia. J cachexia sarcopenia Muscle (2020) 11(6):1509–24. doi: 10.1002/jcsm.12627
155. Callaway CS, Delitto AE, Patel R, Nosacka RL, D'Lugos AC, Delitto D, et al. IL-8 released from human pancreatic cancer and tumor-associated stromal cells signals through a CXCR2-ERK1/2 axis to induce muscle atrophy. Cancers (Basel) (2019) 11(12):1863. doi: 10.3390/cancers11121863
156. Karayiannakis AJ, Syrigos KN, Polychronidis A, Pitiakoudis M, Bounovas A, Simopoulos K. Serum levels of tumor necrosis factor-alpha and nutritional status in pancreatic cancer patients. Anticancer Res (2001) 21(2b):1355–8.
157. Shi X, Yang J, Liu M, Zhang Y, Zhou Z, Luo W, et al. Circular RNA ANAPC7 inhibits tumor growth and muscle wasting Via PHLPP2-AKT-TGF-β signaling axis in pancreatic cancer. Gastroenterology (2022) 162(7):2004–17.e2. doi: 10.1053/j.gastro.2022.02.017
158. Matthys P, Dijkmans R, Proost P, Van Damme J, Heremans H, Sobis H, et al. Severe cachexia in mice inoculated with interferon-Gamma-Producing tumor cells. Int J Cancer (1991) 49(1):77–82. doi: 10.1002/ijc.2910490115
159. Qian L, Yu S, Yin C, Zhu B, Chen Z, Meng Z, et al. Plasma IFN-γ-Inducible chemokines CXCL9 and CXCL10 correlate with survival and chemotherapeutic efficacy in advanced pancreatic ductal adenocarcinoma. Pancreatology (2019) 19(2):340–5. doi: 10.1016/j.pan.2019.01.015
160. Shakri AR, Zhong TJ, Ma W, Coker C, Kim S, Calluori S, et al. Upregulation of ZIP14 and altered zinc homeostasis in muscles in pancreatic cancer cachexia. Cancers (2019) 12(1):3. doi: 10.3390/cancers12010003
161. Mancinelli G, Torres C, Krett N, Bauer J, Castellanos K, McKinney R, et al. Role of stromal activin a in human pancreatic cancer and metastasis in mice. Sci Rep (2021) 11(1):7986. doi: 10.1038/s41598-021-87213-y
162. Zhong X, Pons M, Poirier C, Jiang Y, Liu J, Sandusky GE, et al. The systemic activin response to pancreatic cancer: Implications for effective cancer cachexia therapy. J cachexia sarcopenia Muscle (2019) 10(5):1083–101. doi: 10.1002/jcsm.12461
163. Zhong X, Narasimhan A, Silverman LM, Young AR, Shahda S, Liu S, et al. Sex specificity of pancreatic cancer cachexia phenotypes, mechanisms, and treatment in mice and humans: Role of activin. J cachexia sarcopenia Muscle (2022) 13(4):2146–61. doi: 10.1002/jcsm.12998
164. Petruzzelli M, Schweiger M, Schreiber R, Campos-Olivas R, Tsoli M, Allen J, et al. A switch from white to brown fat increases energy expenditure in cancer-associated cachexia. Cell Metab (2014) 20(3):433–47. doi: 10.1016/j.cmet.2014.06.011
165. Molfino A, Belli R, Imbimbo G, Carletti R, Amabile MI, Tambaro F, et al. Evaluation of browning markers in subcutaneous adipose tissue of newly diagnosed gastrointestinal cancer patients with and without cachexia. Cancers (Basel) (2022) 14(8):1948. doi: 10.3390/cancers14081948
166. Islam-Ali B, Khan S, Price SA, Tisdale MJ. Modulation of adipocyte G-protein expression in cancer cachexia by a lipid-mobilizing factor (LMF). Br J Cancer (2001) 85(5):758–63. doi: 10.1054/bjoc.2001.1992
167. Hu L, Xu X, Li Q, Chen X, Yuan X, Qiu S, et al. Caveolin-1 increases glycolysis in pancreatic cancer cells and triggers cachectic states. FASEB J (2021) 35(8):e21826. doi: 10.1096/fj.202100121RRR
168. Shimada M, Andoh A, Araki Y, Fujiyama Y, Bamba T. Ligation of the Fas antigen stimulates chemokine secretion in pancreatic cancer cell line PANC-1. J Gastroenterol Hepatol (2001) 16(9):1060–7. doi: 10.1046/j.1440-1746.2001.02583.x
169. Zhang G, Liu Z, Ding H, Zhou Y, Doan HA, Sin KWT, et al. Tumor induces muscle wasting in mice through releasing extracellular Hsp70 and Hsp90. Nat Commun (2017) 8(1):589. doi: 10.1038/s41467-017-00726-x
170. Zambirinis CP, Ochi A, Barilla R, Greco S, Deutsch M, Miller G. Induction of trif- or MyD88-dependent pathways perturbs cell cycle regulation in pancreatic cancer. Cell Cycle (2013) 12(8):1153–4. doi: 10.4161/cc.24488
171. Beatty GL, Werba G, Lyssiotis CA, Simeone DM. The biological underpinnings of therapeutic resistance in pancreatic cancer. Genes Dev (2021) 35(13-14):940–62. doi: 10.1101/gad.348523.121
172. Martin A, Castells J, Allibert V, Emerit A, Zolotoff C, Cardot-Ruffino V, et al. Hypothalamic–Pituitary–Adrenal axis activation and glucocorticoid-responsive gene expression in skeletal muscle and liver of apc mice. J cachexia sarcopenia Muscle (2022) 13(3):1686–703. doi: 10.1002/jcsm.12939
173. Hong N, Yoon HJ, Lee YH, Kim HR, Lee BW, Rhee Y, et al. Serum pthrp predicts weight loss in cancer patients independent of hypercalcemia, inflammation, and tumor burden. J Clin Endocrinol Metab (2016) 101(3):1207–14. doi: 10.1210/jc.2015-3785
174. Bonetto A, Aydogdu T, Jin X, Zhang Z, Zhan R, Puzis L, et al. JAK/STAT3 pathway inhibition blocks skeletal muscle wasting downstream of IL-6 and in experimental cancer cachexia. Am J Physiol Endocrinol Metab (2012) 303(3):E410–21. doi: 10.1152/ajpendo.00039.2012
175. Zhou W, Jiang ZW, Tian J, Jiang J, Li N, Li JS. Role of NF-kappaB and cytokine in experimental cancer cachexia. World J Gastroenterol (2003) 9(7):1567–70. doi: 10.3748/wjg.v9.i7.1567
176. Gerber MH, Underwood PW, Judge SM, Delitto D, Delitto AE, Nosacka RL, et al. Local and systemic cytokine profiling for pancreatic ductal adenocarcinoma to study cancer cachexia in an era of precision medicine. Int J Mol Sci (2018) 19(12):3836. doi: 10.3390/ijms19123836
177. Egberts JH, Cloosters V, Noack A, Schniewind B, Thon L, Klose S, et al. Anti-tumor necrosis factor therapy inhibits pancreatic tumor growth and metastasis. Cancer Res (2008) 68(5):1443–50. doi: 10.1158/0008-5472.Can-07-5704
178. Balentine CJ, Enriquez J, Fisher W, Hodges S, Bansal V, Sansgiry S, et al. Intra-abdominal fat predicts survival in pancreatic cancer. J gastrointestinal Surg Off J Soc Surg Alimentary Tract (2010) 14(11):1832–7. doi: 10.1007/s11605-010-1297-5
179. Pettersen K, Andersen S, Degen S, Tadini V, Grosjean J, Hatakeyama S, et al. Cancer cachexia associates with a systemic autophagy-inducing activity mimicked by cancer cell-derived IL-6 trans-signaling. Sci Rep (2017) 7(1):2046. doi: 10.1038/s41598-017-02088-2
180. Han J, Meng Q, Shen L, Wu G. Interleukin-6 induces fat loss in cancer cachexia by promoting white adipose tissue lipolysis and browning. Lipids Health Dis (2018) 17(1):14. doi: 10.1186/s12944-018-0657-0
181. Arora G, Gupta A, Guo T, Gandhi A, Laine A, Williams D, et al. Jak inhibitors suppress cancer cachexia-associated anorexia and adipose wasting in mice. JCSM Rapid Commun (2020) 3(2):115–28. doi: 10.1002/rco2.24
182. Lee EJ, Gusev Y, Jiang J, Nuovo GJ, Lerner MR, Frankel WL, et al. Expression profiling identifies MicroRNA signature in pancreatic cancer. Int J Cancer (2007) 120(5):1046–54. doi: 10.1002/ijc.22394
183. He WA, Calore F, Londhe P, Canella A, Guttridge DC, Croce CM. Microvesicles containing miRNAs promote muscle cell death in cancer cachexia Via TLR7. Proc Natl Acad Sci United States America (2014) 111(12):4525–9. doi: 10.1073/pnas.1402714111
184. Ali S, Almhanna K, Chen W, Philip PA, Sarkar FH. Differentially expressed miRNAs in the plasma may provide a molecular signature for aggressive pancreatic cancer. Am J Transl Res (2010) 3(1):28–47.
185. Calatayud D, Dehlendorff C, Boisen MK, Hasselby JP, Schultz NA, Werner J, et al. Tissue MicroRNA profiles as diagnostic and prognostic biomarkers in patients with resectable pancreatic ductal adenocarcinoma and periampullary cancers. biomark Res (2017) 5:8. doi: 10.1186/s40364-017-0087-6
186. du Rieu MC, Torrisani J, Selves J, Al Saati T, Souque A, Dufresne M, et al. MicroRNA-21 is induced early in pancreatic ductal adenocarcinoma precursor lesions. Clin Chem (2010) 56(4):603–12. doi: 10.1373/clinchem.2009.137364
187. Dong J, Zhao YP, Zhou L, Zhang TP, Chen G. Bcl-2 upregulation induced by miR21 Via a direct interaction is associated with apoptosis and chemoresistance in MIA PaCa-2 pancreatic cancer cells. Arch Med Res (2011) 42(1):8–14. doi: 10.1016/j.arcmed.2011.01.006
188. Park J-K, Lee EJ, Esau C, Schmittgen TD. Antisense inhibition of MicroRNA-21 or -221 arrests cell cycle, induces apoptosis, and sensitizes the effects of gemcitabine in pancreatic adenocarcinoma. Pancreas (2009) 38(7):e190–e9. doi: 10.1097/MPA.0b013e3181ba82e1
189. Paik WH, Kim HR, Park JK, Song BJ, Lee SH, Hwang JH. Chemosensitivity induced by down-regulation of MicroRNA-21 in gemcitabine-resistant pancreatic cancer cells by indole-3-Carbinol. Anticancer Res (2013) 33(4):1473–81.
190. Wang P, Zhuang L, Zhang J, Fan J, Luo J, Chen H, et al. The serum miR21 level serves as a predictor for the chemosensitivity of advanced pancreatic cancer, and miR21 expression confers chemoresistance by targeting FasL. Mol Oncol (2013) 7(3):334–45. doi: 10.1016/j.molonc.2012.10.011
191. Talotta F, Cimmino A, Matarazzo MR, Casalino L, De Vita G, D'Esposito M, et al. An autoregulatory loop mediated by miR21 and PDCD4 controls the AP-1 activity in ras transformation. Oncogene (2009) 28(1):73–84. doi: 10.1038/onc.2008.370
192. Ying H, Elpek KG, Vinjamoori A, Zimmerman SM, Chu GC, Yan H, et al. PTEN is a major tumor suppressor in pancreatic ductal adenocarcinoma and regulates an NF-κB-Cytokine network. Cancer Discovery (2011) 1(2):158–69. doi: 10.1158/2159-8290.cd-11-0031
193. Giovannetti E, Funel N, Peters GJ, Del Chiaro M, Erozenci LA, Vasile E, et al. MicroRNA-21 in pancreatic cancer: Correlation with clinical outcome and pharmacologic aspects underlying its role in the modulation of gemcitabine activity. Cancer Res (2010) 70(11):4528–38. doi: 10.1158/0008-5472.can-09-4467
194. Sun J, Jiang Z, Li Y, Wang K, Chen X, Liu G. Downregulation of miR21 inhibits the malignant phenotype of pancreatic cancer cells by targeting vhl. OncoTargets Ther (2019) 12:7215–26. doi: 10.2147/ott.s211535
195. Mortoglou M, Miralles F, Arisan ED, Dart A, Jurcevic S, Lange S, et al. MicroRNA-21 regulates stemness in pancreatic ductal adenocarcinoma cells. Int J Mol Sci (2022) 23(3):1275. doi: 10.3390/ijms23031275
196. Fabbri M, Paone A, Calore F, Galli R, Gaudio E, Santhanam R, et al. MicroRNAs bind to toll-like receptors to induce prometastatic inflammatory response. Proc Natl Acad Sci United States America (2012) 109(31):E2110–6. doi: 10.1073/pnas.1209414109
197. Borja-Gonzalez M, Casas-Martinez JC, McDonagh B, Goljanek-Whysall K. Inflamma-miR21 negatively regulates myogenesis during ageing. Antioxidants (Basel) (2020) 9(4). doi: 10.3390/antiox9040345
198. Yonemori K, Kurahara H, Maemura K, Natsugoe S. MicroRNA in pancreatic cancer. J Hum Genet (2017) 62(1):33–40. doi: 10.1038/jhg.2016.59
199. Gebert LFR, MacRae IJ. Regulation of MicroRNA function in animals. Nat Rev Mol Cell Biol (2019) 20(1):21–37. doi: 10.1038/s41580-018-0045-7
200. Yehia R, Schaalan M, Abdallah DM, Saad AS, Sarhan N, Saleh S. Impact of TNF-α gene polymorphisms on pancreatic and non-small cell lung cancer-induced cachexia in adult Egyptian patients: A focus on pathogenic trajectories. Front Oncol (2021) 11:783231. doi: 10.3389/fonc.2021.783231
201. Gironella M, Seux M, Xie MJ, Cano C, Tomasini R, Gommeaux J, et al. Tumor protein 53-induced nuclear protein 1 expression is repressed by miR155, and its restoration inhibits pancreatic tumor development. Proc Natl Acad Sci United States America (2007) 104(41):16170–5. doi: 10.1073/pnas.0703942104
202. Nishiwada S, Sho M, Banwait JK, Yamamura K, Akahori T, Nakamura K, et al. A MicroRNA signature identifies pancreatic ductal adenocarcinoma patients at risk for lymph node metastases. Gastroenterology (2020) 159(2):562–74. doi: 10.1053/j.gastro.2020.04.057
203. Huang C, Li H, Wu W, Jiang T, Qiu Z. Regulation of miR155 affects pancreatic cancer cell invasiveness and migration by modulating the STAT3 signaling pathway through SOCS1. Oncol Rep (2013) 30(3):1223–30. doi: 10.3892/or.2013.2576
204. Pang W, Su J, Wang Y, Feng H, Dai X, Yuan Y, et al. Pancreatic cancer-secreted miR155 implicates in the conversion from normal fibroblasts to cancer-associated fibroblasts. Cancer Sci (2015) 106(10):1362–9. doi: 10.1111/cas.12747
205. Basu A, Alder H, Khiyami A, Leahy P, Croce CM, Haldar S. MicroRNA-375 and MicroRNA-221: Potential noncoding RNAs associated with antiproliferative activity of benzyl isothiocyanate in pancreatic cancer. Genes Cancer (2011) 2(2):108–19. doi: 10.1177/1947601911409212
206. Su A, He S, Tian B, Hu W, Zhang Z. MicroRNA-221 mediates the effects of PDGF-BB on migration, proliferation, and the epithelial-mesenchymal transition in pancreatic cancer cells. PloS One (2013) 8(8):e71309. doi: 10.1371/journal.pone.0071309
207. Xu Q, Li P, Chen X, Zong L, Jiang Z, Nan L, et al. miR221/222 induces pancreatic cancer progression through the regulation of matrix metalloproteinases. Oncotarget (2015) 6(16):14153–64. doi: 10.18632/oncotarget.3686
208. Sarkar S, Dubaybo H, Ali S, Goncalves P, Kollepara SL, Sethi S, et al. Down-regulation of miR221 inhibits proliferation of pancreatic cancer cells through up-regulation of PTEN, P27(Kip1), P57(Kip2), and PUMA. Am J Cancer Res (2013) 3(5):465–77.
209. Tan SB, Li J, Chen X, Zhang W, Zhang D, Zhang C, et al. Small molecule inhibitor of myogenic MicroRNAs leads to a discovery of miR221/222-myoD-myomiRs regulatory pathway. Chem Biol (2014) 21(10):1265–70. doi: 10.1016/j.chembiol.2014.06.011
210. Patel K, Kollory A, Takashima A, Sarkar S, Faller DV, Ghosh SK. MicroRNA let-7 downregulates STAT3 phosphorylation in pancreatic cancer cells by increasing SOCS3 expression. Cancer Lett (2014) 347(1):54–64. doi: 10.1016/j.canlet.2014.01.020
211. Tesfaye AA, Azmi AS, Philip PA. miRNA and gene expression in pancreatic ductal adenocarcinoma. Am J Pathol (2019) 189(1):58–70. doi: 10.1016/j.ajpath.2018.10.005
212. Jiao LR, Frampton AE, Jacob J, Pellegrino L, Krell J, Giamas G, et al. MicroRNAs targeting oncogenes are down-regulated in pancreatic malignant transformation from benign tumors. PloS One (2012) 7(2):e32068. doi: 10.1371/journal.pone.0032068
213. Narasimhan A, Ghosh S, Stretch C, Greiner R, Bathe OF, Baracos V, et al. Small rnaome profiling from human skeletal muscle: Novel miRNAs and their targets associated with cancer cachexia. J cachexia sarcopenia Muscle (2017) 8(3):405–16. doi: 10.1002/jcsm.12168
214. Freire PP, Fernandez GJ, Cury SS, de Moraes D, Oliveira JS, de Oliveira G, et al. The pathway to cancer cachexia: MicroRNA-regulated networks in muscle wasting based on integrative meta-analysis. Int J Mol Sci (2019) 20(8):1962. doi: 10.3390/ijms20081962
215. Suzuki T, Springer J. MicroRNAs in muscle wasting. J cachexia sarcopenia Muscle (2018) 9(7):1209–12. doi: 10.1002/jcsm.12384
216. Soares RJ, Cagnin S, Chemello F, Silvestrin M, Musaro A, De Pitta C, et al. Involvement of MicroRNAs in the regulation of muscle wasting during catabolic conditions. J Biol Chem (2014) 289(32):21909–25. doi: 10.1074/jbc.M114.561845
217. Camargo RG, Quintas Teixeira Ribeiro H, Geraldo MV, Matos-Neto E, Neves RX, Carnevali LC Jr., et al. Cancer cachexia and MicroRNAs. Mediators Inflammation (2015) 2015:367561. doi: 10.1155/2015/367561
218. Kottorou A, Dimitrakopoulos F-I, Tsezou A. Non-coding RNAs in cancer-associated cachexia: Clinical implications and future perspectives. Trans Oncol (2021) 14(7):101101. doi: 10.1016/j.tranon.2021.101101
219. Li X, Du L, Liu Q, Lu Z. MicroRNAs: Novel players in the diagnosis and treatment of cancer cachexia (Review). Exp Ther Med (2022) 24(1):446. doi: 10.3892/etm.2022.11373
220. Del Fabbro E. Combination therapy in cachexia. Ann Palliat Med (2019) 8(1):59–66. doi: 10.21037/apm.2018.08.05
221. Monaco C, Nanchahal J, Taylor P, Feldmann M. Anti-TNF therapy: Past, present and future. Int Immunol (2014) 27(1):55–62. doi: 10.1093/intimm/dxu102
222. Gullett NP, Hebbar G, Ziegler TR. Update on clinical trials of growth factors and anabolic steroids in cachexia and wasting. Am J Clin Nutr (2010) 91(4):1143S–7S. doi: 10.3945/ajcn.2010.28608E
223. Gueta I, Altman A, Shoenfeld Y. The effect of blocking TNF-alpha in patients with cancer-related cachexia and anorexia. Harefuah (2010) 149(8):512–4.
224. Wu C, Fernandez SA, Criswell T, Chidiac TA, Guttridge D, Villalona-Calero M, et al. Disrupting cytokine signaling in pancreatic cancer: A phase I/II study of etanercept in combination with gemcitabine in patients with advanced disease. Pancreas (2013) 42(5):813–8. doi: 10.1097/MPA.0b013e318279b87f
225. Jatoi A, Ritter HL, Dueck A, Nguyen PL, Nikcevich DA, Luyun RF, et al. A placebo-controlled, double-blind trial of infliximab for cancer-associated weight loss in elderly and/or poor performance non-small cell lung cancer patients (N01C9). Lung Cancer (2010) 68(2):234–9. doi: 10.1016/j.lungcan.2009.06.020
226. Wiedenmann B, Malfertheiner P, Friess H, Ritch P, Arseneau J, Mantovani G, et al. A multicenter, phase II study of infliximab plus gemcitabine in pancreatic cancer cachexia. J Support Oncol (2008) 6(1):18–25.
227. Golan T, Geva R, Richards D, Madhusudan S, Lin BK, Wang HT, et al. Ly2495655, an antimyostatin antibody, in pancreatic cancer: A randomized, phase 2 trial. J cachexia sarcopenia Muscle (2018) 9(5):871–9. doi: 10.1002/jcsm.12331
228. Woodhouse L, Gandhi R, Warden SJ, Poiraudeau S, Myers SL, Benson CT, et al. A phase 2 randomized study investigating the efficacy and safety of myostatin antibody Ly2495655 versus placebo in patients undergoing elective total hip arthroplasty. J Frailty Aging (2016) 5(1):62–70. doi: 10.14283/jfa.2016.81
229. Hirata H, Tetsumoto S, Kijima T, Kida H, Kumagai T, Takahashi R, et al. Favorable responses to tocilizumab in two patients with cancer-related cachexia. J Pain Symptom Manage (2013) 46(2):e9–e13. doi: 10.1016/j.jpainsymman.2013.01.009
230. Ando K, Takahashi F, Kato M, Kaneko N, Doi T, Ohe Y, et al. Tocilizumab, a proposed therapy for the cachexia of Interleukin6-expressing lung cancer. PloS One (2014) 9(7):e102436. doi: 10.1371/journal.pone.0102436
231. Prado BL, Qian Y. Anti-cytokines in the treatment of cancer cachexia. Ann Palliat Med (2019) 8(1):67–79. doi: 10.21037/apm.2018.07.06
232. Hurwitz HI, Uppal N, Wagner SA, Bendell JC, Beck JT, III SMW, et al. Randomized, double-blind, phase II study of ruxolitinib or placebo in combination with capecitabine in patients with metastatic pancreatic cancer for whom therapy with gemcitabine has failed. J Clin Oncol (2015) 33(34):4039–47. doi: 10.1200/jco.2015.61.4578
233. Hurwitz H, Van Cutsem E, Bendell J, Hidalgo M, Li CP, Salvo MG, et al. Ruxolitinib + capecitabine in Advanced/Metastatic pancreatic cancer after disease Progression/Intolerance to first-line therapy: Janus 1 and 2 randomized phase III studies. Invest New Drugs (2018) 36(4):683–95. doi: 10.1007/s10637-018-0580-2
234. Schlingensiepen KH, Jaschinski F, Lang SA, Moser C, Geissler EK, Schlitt HJ, et al. Transforming growth factor-beta 2 gene silencing with trabedersen (AP 12009) in pancreatic cancer. Cancer Sci (2011) 102(6):1193–200. doi: 10.1111/j.1349-7006.2011.01917.x
235. Bogdahn U, Hau P, Stockhammer G, Venkataramana NK, Mahapatra AK, Suri A, et al. Targeted therapy for high-grade glioma with the TGF-β2 inhibitor trabedersen: Results of a randomized and controlled phase IIb study. Neuro Oncol (2011) 13(1):132–42. doi: 10.1093/neuonc/noq142
236. Johnen H, Lin S, Kuffner T, Brown DA, Tsai VW-W, Bauskin AR, et al. Tumor-induced anorexia and weight loss are mediated by the TGF-β superfamily cytokine MIC-1. Nat Med (2007) 13(11):1333–40. doi: 10.1038/nm1677
237. Rooks DS, Laurent D, Praestgaard J, Rasmussen S, Bartlett M, Tankó LB. Effect of bimagrumab on thigh muscle volume and composition in men with casting-induced atrophy. J cachexia sarcopenia Muscle (2017) 8(5):727–34. doi: 10.1002/jcsm.12205
238. Rooks D, Petricoul O, Praestgaard J, Bartlett M, Laurent D, Roubenoff R. Safety and pharmacokinetics of bimagrumab in healthy older and obese adults with body composition changes in the older cohort. J cachexia sarcopenia Muscle (2020) 11(6):1525–34. doi: 10.1002/jcsm.12639
239. Hamauchi S, Furuse J, Takano T, Munemoto Y, Furuya K, Baba H, et al. A multicenter, open-label, single-arm study of anamorelin (ONO-7643) in advanced gastrointestinal cancer patients with cancer cachexia. Cancer (2019) 125(23):4294–302. doi: 10.1002/cncr.32406
240. Wakabayashi H, Arai H, Inui A. The regulatory approval of anamorelin for treatment of cachexia in patients with non-small cell lung cancer, gastric cancer, pancreatic cancer, and colorectal cancer in Japan: Facts and numbers. J cachexia sarcopenia Muscle (2021) 12(1):14–6. doi: 10.1002/jcsm.12675
241. Katakami N, Uchino J, Yokoyama T, Naito T, Kondo M, Yamada K, et al. Anamorelin (ONO-7643) for the treatment of patients with non-small cell lung cancer and cachexia: Results from a randomized, double-blind, placebo-controlled, multicenter study of Japanese patients (Ono-7643-04). Cancer (2018) 124(3):606–16. doi: 10.1002/cncr.31128
242. Garcia JM, Friend J, Allen S. Therapeutic potential of anamorelin, a novel, oral ghrelin mimetic, in patients with cancer-related cachexia: A multicenter, randomized, double-blind, crossover, pilot study. Support Care Cancer (2013) 21(1):129–37. doi: 10.1007/s00520-012-1500-1
243. Temel JS, Abernethy AP, Currow DC, Friend J, Duus EM, Yan Y, et al. Anamorelin in patients with non-Small-Cell lung cancer and cachexia (Romana 1 and romana 2): Results from two randomised, double-blind, phase 3 trials. Lancet Oncol (2016) 17(4):519–31. doi: 10.1016/s1470-2045(15)00558-6
244. Liu Z, Xiong J, Gao S, Zhu MX, Sun K, Li M, et al. Ameliorating cancer cachexia by inhibiting cancer cell release of Hsp70 and Hsp90 with omeprazole. J cachexia sarcopenia Muscle (2022) 13(1):636–47. doi: 10.1002/jcsm.12851
245. Chi KH, Chiou TJ, Li CP, Chen SY, Chao Y. Ms-20, a chemotherapeutical adjuvant, reduces chemo-associated fatigue and appetite loss in cancer patients. Nutr Cancer (2014) 66(7):1211–9. doi: 10.1080/01635581.2014.951731
246. Stewart Coats AJ, Ho GF, Prabhash K, von Haehling S, Tilson J, Brown R, et al. Espindolol for the treatment and prevention of cachexia in patients with stage III/IV non-small cell lung cancer or colorectal cancer: A randomized, double-blind, placebo-controlled, international multicentre phase II study (the act-one trial). J cachexia sarcopenia Muscle (2016) 7(3):355–65. doi: 10.1002/jcsm.12126
247. Calore F, Londhe P, Fadda P, Nigita G, Casadei L, Marceca GP, et al. The TLR7/8/9 antagonist IMO-8503 inhibits cancer-induced cachexia. Cancer Res (2018) 78(23):6680–90. doi: 10.1158/0008-5472.Can-17-3878
248. Michaelis KA, Norgard MA, Zhu X, Levasseur PR, Sivagnanam S, Liudahl SM, et al. The TLR7/8 agonist R848 remodels tumor and host responses to promote survival in pancreatic cancer. Nat Commun (2019) 10(1):4682. doi: 10.1038/s41467-019-12657-w
249. Llovera M, García-Martínez C, Costelli P, Agell N, Carbó N, López-Soriano FJ, et al. Muscle hypercatabolism during cancer cachexia is not reversed by the glucocorticoid receptor antagonist Ru38486. Cancer Lett (1996) 99(1):7–14. doi: 10.1016/0304-3835(95)04026-9
250. Rivadeneira DE, Naama HA, McCarter MD, Fujita J, Evoy D, Mackrell P, et al. Glucocorticoid blockade does not abrogate tumor-induced cachexia. Nutr Cancer (1999) 35(2):202–6. doi: 10.1207/s15327914nc352_16
251. May PE, Barber A, D'Olimpio JT, Hourihane A, Abumrad NN. Reversal of cancer-related wasting using oral supplementation with a combination of beta-Hydroxy-Beta-Methylbutyrate, arginine, and glutamine. Am J Surg (2002) 183(4):471–9. doi: 10.1016/s0002-9610(02)00823-1
252. Berk L, James J, Schwartz A, Hug E, Mahadevan A, Samuels M, et al. A randomized, double-blind, placebo-controlled trial of a beta-hydroxyl beta-methyl butyrate, glutamine, and arginine mixture for the treatment of cancer cachexia (RTOG 0122). Support Care Cancer (2008) 16(10):1179–88. doi: 10.1007/s00520-008-0403-7
253. Soares JDP, Howell SL, Teixeira FJ, Pimentel GD. Dietary amino acids and immunonutrition supplementation in cancer-induced skeletal muscle mass depletion: A mini-review. Curr Pharm Des (2020) 26(9):970–8. doi: 10.2174/1381612826666200218100420
254. Morrison WL, Gibson JN, Rennie MJ. Skeletal muscle and whole body protein turnover in cardiac cachexia: Influence of branched-chain amino acid administration. Eur J Clin Invest (1988) 18(6):648–54. doi: 10.1111/j.1365-2362.1988.tb01282.x
255. Laviano A, Muscaritoli M, Cascino A, Preziosa I, Inui A, Mantovani G, et al. Branched-chain amino acids: The best compromise to achieve anabolism? Curr Opin Clin Nutr Metab Care (2005) 8(4):408–14. doi: 10.1097/01.mco.0000172581.79266.19
256. Tayek JA, Bistrian BR, Hehir DJ, Martin R, Moldawer LL, Blackburn GL. Improved protein kinetics and albumin synthesis by branched chain amino acid-enriched total parenteral nutrition in cancer cachexia. a prospective randomized crossover trial. Cancer (1986) 58(1):147–57. doi: 10.1002/1097-0142(19860701)58:1<147::aid-cncr2820580126>3.0.co;2-i
257. Espina S, Sanz-Paris A, Gonzalez-Irazabal Y, Pérez-Matute P, Andrade F, Garcia-Rodriguez B, et al. Randomized clinical trial: Effects of Β-Hydroxy-Β-Methylbutyrate (Hmb)-enriched vs. hmb-free oral nutritional supplementation in malnourished cirrhotic patients. Nutrients (2022) 14(11):2344. doi: 10.3390/nu14112344
258. Solheim TS, Laird BJA, Balstad TR, Stene GB, Bye A, Johns N, et al. A randomized phase II feasibility trial of a multimodal intervention for the management of cachexia in lung and pancreatic cancer. J cachexia sarcopenia Muscle (2017) 8(5):778–88. doi: 10.1002/jcsm.12201
259. Newell M, Mazurak V, Postovit LM, Field CJ. N-3 long-chain polyunsaturated fatty acids, eicosapentaenoic and docosahexaenoic acid, and the role of supplementation during cancer treatment: A scoping review of current clinical evidence. Cancers (Basel) (2021) 13(6):1206. doi: 10.3390/cancers13061206
260. Baracos VE, Mazurak VC, Ma DW. N-3 polyunsaturated fatty acids throughout the cancer trajectory: Influence on disease incidence, progression, response to therapy and cancer-associated cachexia. Nutr Res Rev (2004) 17(2):177–92. doi: 10.1079/nrr200488
261. Werner K, Küllenberg de Gaudry D, Taylor LA, Keck T, Unger C, Hopt UT, et al. Dietary supplementation with n-3-Fatty acids in patients with pancreatic cancer and cachexia: Marine phospholipids versus fish oil - a randomized controlled double-blind trial. Lipids Health Dis (2017) 16(1):104. doi: 10.1186/s12944-017-0495-5
262. Schmidt N, Møller G, Bæksgaard L, Østerlind K, Stark KD, Lauritzen L, et al. Fish oil supplementation in cancer patients. capsules or nutritional drink supplements? a controlled study of compliance. Clin Nutr ESPEN (2020) 35:63–8. doi: 10.1016/j.clnesp.2019.12.004
263. Torres C, Diaz AM, Principe DR, Grippo PJ. The complexity of omega-3 fatty acid modulation of signaling pathways related to pancreatic cancer. Curr Med Chem (2018) 25(22):2608–23. doi: 10.2174/0929867324666170616111225
264. Nabavi SF, Bilotto S, Russo GL, Orhan IE, Habtemariam S, Daglia M, et al. Omega-3 polyunsaturated fatty acids and cancer: Lessons learned from clinical trials. Cancer Metastasis Rev (2015) 34(3):359–80. doi: 10.1007/s10555-015-9572-2
265. Gorjao R, dos Santos CMM, Serdan TDA, Diniz VLS, Alba-Loureiro TC, Cury-Boaventura MF, et al. New insights on the regulation of cancer cachexia by n-3 polyunsaturated fatty acids. Pharmacol Ther (2019) 196:117–34. doi: 10.1016/j.pharmthera.2018.12.001
266. Landers A, Muircroft W, Brown H. Pancreatic enzyme replacement therapy (Pert) for malabsorption in patients with metastatic pancreatic cancer. BMJ Support Palliat Care (2016) 6(1):75–9. doi: 10.1136/bmjspcare-2014-000694
Keywords: pancreatic ductal adenocarcinoma (PDAC), cachexia, muscle wasting, tissue wasting, endocrine organ-like tumour (EOLT)
Citation: Yu Y-C, Ahmed A, Lai H-C, Cheng W-C, Yang J-C, Chang W-C, Chen L-M, Shan Y-S and Ma W-L (2022) Review of the endocrine organ–like tumor hypothesis of cancer cachexia in pancreatic ductal adenocarcinoma. Front. Oncol. 12:1057930. doi: 10.3389/fonc.2022.1057930
Received: 30 September 2022; Accepted: 26 October 2022;
Published: 17 November 2022.
Edited by:
Emilio Francesco Giunta, Università degli Studi della Campania Luigi Vanvitelli, ItalyReviewed by:
Shiyu Song, Nanjing University, ChinaAditi Arun Narsale, San Diego Biomedical Research Institute, United States
Copyright © 2022 Yu, Ahmed, Lai, Cheng, Yang, Chang, Chen, Shan and Ma. This is an open-access article distributed under the terms of the Creative Commons Attribution License (CC BY). The use, distribution or reproduction in other forums is permitted, provided the original author(s) and the copyright owner(s) are credited and that the original publication in this journal is cited, in accordance with accepted academic practice. No use, distribution or reproduction is permitted which does not comply with these terms.
*Correspondence: Wen-Lung Ma, bWF2ZXJpY2tAbWFpbC5jbXUuZWR1LnR3; Yan-Shen Shan, eXNzaGFuQG1haWwubmNrdS5lZHUudHc=