- 1Mechanobiology Lab, Department of Mechanical Engineering, Massachusetts Institute of Technology, Cambridge, MA, United States
- 2Biomicrofluidics Lab, Department of Mechanical Engineering, Korea Advanced Institute of Science and Technology, Daejeon, South Korea
Emerging microfluidic disease models have amply demonstrated their value in many fields of cancer research. These in vitro technologies recapitulate key aspects of metastatic cancer, including the process of tumor cell arrest and extravasation at the site of the metastatic tumor. To date, extensive efforts have been made to capture key features of the microvasculature to reconstitute the pre-metastatic niche and investigate dynamic extravasation behaviors using microfluidic systems. In this mini-review, we highlight recent microfluidic vascular models of tumor cell extravasation and explore how this approach contributes to development of in vitro disease models to enhance understanding of metastasis in vivo.
Introduction
Despite advances in cancer treatment such as surgical resection and adjuvant therapy, cancer metastasis still accounts for more than 90% of cancer patients’ deaths (1). Cancer metastasis often begins when tumor cells undergo an epithelial-to-mesenchymal transition (EMT), in which they acquire higher motility and invasiveness and break away from the primary tumor (2). Then, these tumor cells invade surrounding tissues, intravasate to the nearby microvasculature as either single cells or cell clusters, and become circulating tumor cells (CTCs), often aggregating with platelets or monocytes, enabling them to withstand fluid shear stress or anoikis (3) and avoid attack by circulating NK cells. Surviving CTCs can then adhere to the endothelium and extravasate into the distant secondary organ and subsequently progress into metastatic tumors (Figure 1). In the secondary organ, factors including the pre-metastatic microenvironment (e.g., resident stromal cells) determines the extravasation sites and plays an important role in metastasis progression (4). Therefore, it is important to explore how the interactions between CTCs and the microenvironment in the secondary organ affect cancer cell extravasation.
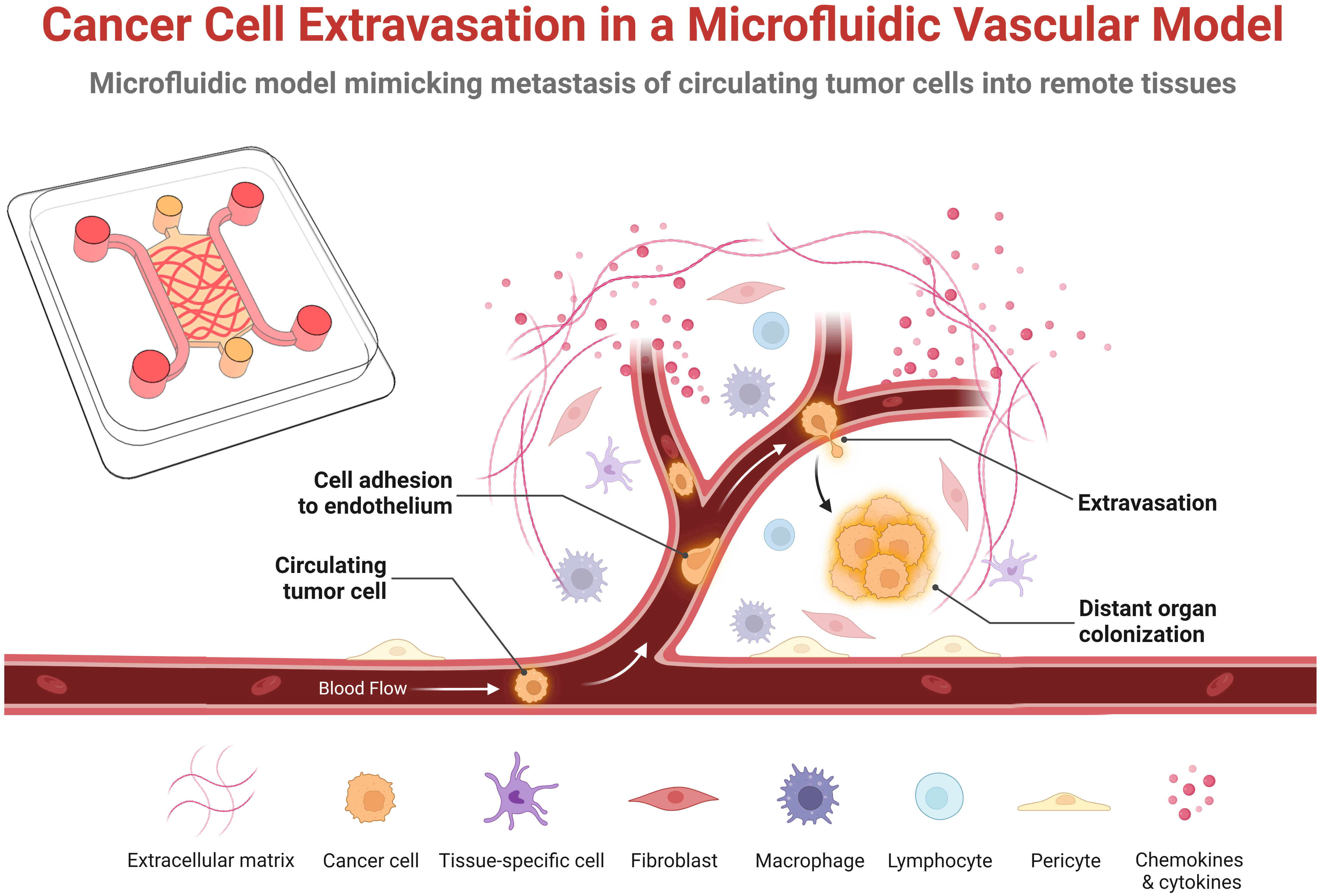
Figure 1 Schematic representation of the key steps of cancer cell extravasation within a microfluidic vascular model. Circulating tumor cells originate from the primary tumor, enter the bloodstream that transports them to a distance organ or tissue where they adhere or become physically trapped in the microvasculature. Some fraction of these survive in the vessel and undergo trans-endothelial migration by extending protrusions across the endothelial monolayer, adhering to the basal membrane and extracellular matrix, and pulling or pushing itself across the endothelial monolayer into the underlying tissue. In the extravascular space, the extravasated tumor cells then either proliferate, die, or enters into a dormant state. This schematic was created with BioRender.com.
Preclinical animal models such as patient-derived xenograft (PDX) mouse models have been established to study cancer stemness and stroma-tumor interactions, predict potential biomarkers, and identify anti-cancer therapeutic strategies (5). PDX models preserve blood vessels and stroma conserved in the surgical tissue, which reflects the unique features of primary tumor and enables a more accurate evaluation of anti-metastatic treatments. Although PDX models have led to enormous advances in anti-cancer therapeutic research, certain inherent challenges are still difficult to address. A major hurdle is the use of immunodeficient mice to overcome transplant rejection, which hampers the studies of the complex roles of the immune system involved in metastasis (6). The applicable types and grades of cancers are limited for successful establishment of PDX models, and the human stroma of xenografts may be replaced by murine components due to a series of tumor passaging, resulting in loss of the characteristics of the original cancer tissue. In addition, access to high-resolution imaging technologies to visualize tumor cell activity in PDX models is another challenge (7), despite the vast capabilities of intravital imaging (8). Finally, a lack of control over various important steps of the metastatic cascade, the presence of non-human cells, and limitations to the use of genetic modifications all point to the need of alternative models.
Microfluidic-based cancer models, also called tumor-on-a-chip models, have emerged as an intermediate and complementary technology positioned between animal models and in vitro two-dimensional (2D) models (9–11). A microfluidic three-dimensional (3D) system is defined as a miniaturized cell culture platform engineered to model the functional units of human tissue/organs (12, 13). This advanced technology recapitulates many features of the complex human tumor/host microenvironments by controlling biophysical and biochemical factors in on-demand manners, which enables the reconstitution of key biological processes and disease states (14–16). Numerous reviews have summarized the advances in tumor-on-a-chip models (17–23). Here, we focus on processes occurring in the metastatic site utilizing microfluidic vascular models (Table 1).
Microfluidic vascular models to study tumor cell extravasation
One approach used in modeling the process of extravasation in vitro utilizes microfluidic vascular models (57). There are three representative types of on-chip endothelial models, namely, a self-assembled microvascular network (MVN), an endothelial-lined channel, and a planar endothelial barrier (58). In summary, the self-assembled MVN (59) or endothelial-lined channel (60) can be created via the proliferation and maturation of vascular cells that are embedded within the 3D extracellular matrix (ECM) or seeded on the gel-liquid interface, respectively. In these designs, on-chip implant of microliter-scale hydrogel is realized with microstructures such as hydrophilic surface rail, sacrificial gel, micro-posts, or channel height differences (61–64). The planar endothelial barrier is typically formed by vascular cell proliferation onto a microporous polymer membrane flanked by microchannels. (65). Utilizing the membrane requires the examination of whether several parameters including pore size, pore density, and thickness hinder the transport of molecules and cells while maintaining tissue barrier function with a sufficient contact area. To overcome these challenges, other semi-permeable materials such as dried ECM film or electrospun nanofibers have been integrated into the microfluidic system (66, 67). Notably, the engineered vascular models have different characteristics as follows. For instance, the 3D MVN has the smallest vessel diameters (~ 15-50 μm) compared to those of others, recapitulating in vivo microvasculature (68, 69). Given the importance of ECM in tumor extravasation and colonization, the presence of ECM that encapsulates MVNs can provide the experimental opportunity for studying the protease activity of tumor cells during ECM invasion and adaption of tumor cells at the secondary microenvironment. The planar endothelial barrier model allows the acquisition of conditioned medium in extravascular region and the measurement of transepithelial electrical resistance (TEER) across the endothelium, which are limited in the MVN model.
Cancer cells are seeded in these lumenized channels or vasculature where they interact with the host microenvironment, become physically lodged in the small vessels or adhere to the endothelium, and extravasate across the endothelial monolayer (70). Because of its high versatility and robust reproducibility, microfluidic vascular models have been used for pre-clinical assays, such as studies of anti-metastatic drug responses and immunotherapy. Previous reviews have summarized tumor extravasation studies using microfluidic vascular models (57, 71–75), thus we only highlight here the recently published works.
Adhesion molecules in tumor cell extravasation
Cell-cell and cell matrix adhesion plays a central role in the arrest and transmigration of tumor cells. Earlier studies in 3D, self-assembled MVNs have investigated the role of various integrins during extravasation, identifying the importance of the α3β1 and α6β1 subunits, implicating laminin in the endothelial basement membrane as a key factor in extravasation (42). Other adhesion molecules have been identified in the initial adhesion of a tumor cell to the endothelium. For example, in a series of studies, the role of the glycocalyx, a common surface coating of glycoproteins and proteoglycans, has been studied (76). The endothelial glycocalyx, including hyaluronic acid (HA), heparan sulfate, and chondroitin sulfate, has traditionally been considered as a repulsive barrier that prevents cell adhesion (77). Recently, an in vitro microfluidic vascular model identified a mechanism in which tumor cells prepared an adhesive vascular niche for subsequent tumor cell adhesion by depositing tumor HA on the apical surface of the endothelium (29). Notably, tumor cells arrested on such an adhesive vascular niche through their CD44v binding to the deposited HA. Genetic and pharmacological inhibition of CD44 abrogated such adhesion. These results suggested that the cancer glycocalyx may be a promising target for therapeutic intervention for metastasis.
Beside glycocalyx, focal adhesion signaling pathway is also important for tumor cell arrest and extravasation (78). Gilardi et al. investigated the cyclin-dependent kinase 5 (Cdk5)/Talin 1 (Tln1)/focal adhesion kinase (FAK) axis in breast cancer and fibrosarcoma extravasation using a three-gel channel microfluidic device, where the microvasculature was in the center and stromal cells are seeded in the left and right gel channels (27). Knocking down Tln1, FAK, or Cdk5 in tumor cells reduced vascular adhesion and trans-endothelium migration. Particularly, inhibitor experiments showed that FAK S732 phosphorylation was required for tumor cell extravasation, which may be explained by phosphorylated-S732 dependent pERK expression in nuclei.
Physical factors in tumor cell extravasation
Throughout the process of metastasis, tumor cells are subjected to a variety of physical stressors such as fluid shear stress (FSS) (79), and their progress is influenced by mechanical factors such as ECM fiber orientation and substrate stiffness (80, 81). To investigate the effects of fluid flows on cancer extravasation, breast cancer cells have been perfused into an in vitro microfluidic MVN model, controlling the intravascular and transmural (trans-endothelial) pressure differences to produce physiological luminal, transmural, and interstitial flows (31). These breast cancer cells extravasated in a single cell manner. When applied with luminal flow (up to ~500 μm/s), cancer cell extravasation efficiencies were significantly increased compared to static control, independent of transmural flow rate. Notably, transmural flow increased both the speed of tumor cell trans-endothelial migration and migration in the surrounding matrix. The directionality of tumor cells under flows was also assessed: intravascular adherent tumor cells traveled predominantly in the direction of luminal flow, whereas extravasated tumor cells showed random migration patterns. This work suggests the importance of applying physiological luminal flow when studying extravasation in vitro.
Another microfluidic platform constructed by a sequential edge-guided patterning method was developed to study tumor cell intravasation and extravasation (52). In this study, colon cancer cells, SW620, were perfused into MVNs and cultured under a flow that was driven by a hydrostatic pressure differential between medium channels. Unlike the breast cancer cells mentioned above (31), SW620 formed micro-tumors attached to the endothelium and extravasated from the vessels. Future work on the mechanism is still under investigation. Different from the self-organized MVNs mentioned above, the Goetz group used a human umbilical vein EC (HUVEC)-coated channel to mimic vasculature and investigated endothelial remodeling by FSS. They found that flow upregulated VEGFR signaling in ECs and that blocking VEGFR signaling reduced EC remodeling and tumor cell extravasation (82).
Besides FSS, substrate stiffness, as another biophysical cue, was recently investigated for its role in regulating cancer cell extravasation (32). Breast cancer cell lines, MCF7 and MDA-MB-231, were precultured on fibronectin-coated polydimethylsiloxane (PDMS) substrates of different stiffness and then added to an EC monolayer in a microfluidic device to evaluate their extravasation rate. Tumor cells precultured on the stiff substrate had the highest extravasation rate probably due to the elevated MMP9 expression in tumor cells. The same group, later utilized the same EC monolayer system to evaluate the extravasation rate of breast cancer cells treated with an EGFR-targeting anti-cancer drug, Cetuximab. The reduced extravasation rate with Cetuximab treatment was associated with altered cell physical features with decreased actin, vinculin, and myosin II expression (47).
Tissue-specific models for tumor cell extravasation study
Cancers typically exhibit organ-specificity in their metastatic cascades, known as “organotropism”, in which cancers tend to predominantly spread to a distant organ or show sequential organ-specific colonization (83). Organ-specific metastasis is likely regulated by multiple factors, including circulation patterns, tumor-intrinsic factors, metabolic changes, immune landscapes of target organs, and the interaction between tumor cells and the secondary organ microenvironment (84). Although the underlying mechanisms of non-random distributions of metastatic cancers have been not fully understood, clinical evidence has indicated clear patterns of organ-specific metastasis (85). Given the complexity involved in organ-specific metastasis, 3D microfluidic cell culture systems have advantages in recapitulating various features of the host microenvironment by employing organ- or tissue-specific cell types in a miniaturized 3D assay compared to conventional systems. As a representative study, a 3D in vitro blood-brain barrier (BBB) model was used to investigate the mechanisms brain-specificity in multiple cancer types (24). This study confirmed previous studies in mice that identified ST6GALNAC5 as an important factor in brain selectivity (86). It also showed that, in addition, astrocytes in brain parenchyma directly promoted cancer cell transmigration through C-C motif chemokine ligand 2 (CCL2) secretion (24). The BBB model was composed of induced pluripotent stem cell (iPSC)-derived ECs (iPSC-ECs), brain pericytes, and astrocytes, reconstituting key morphological and functional characteristics of the brain capillaries (i.e. cellular organization, expression of junctional and transporter proteins, vascular permeability, and deposition of the basement membrane and glycocalyx). Another group recently developed a blood-brain niche system using a two-channel microfluidic device separated by a porous membrane (54). The bottom of the device was filled with human astrocytes in collagen gel to recapitulate a brain microenvironment. ECs were seeded as a monolayer on the membrane to serve as a barrier. MDA-231-BR tumor cells were perfused into the vessel and extravasated by the attraction of astrocytes themselves or astrocytes-conditioned medium. The secretome analysis of ECs and astrocytes identified that Dickkopf-related protein 1 (Dkk-1) was elevated in astrocytes when stimulated with MDA-231-BR. Treating tumor cells with Dkk-1 upregulated FGF-13, PLCB1, and MYC gene expression, which are key components in Ras, PI3K-MAPK, and Wnt signaling pathways. Neutralizing Dkk-1 and knocking down FGF-13 in tumor cells were able to reduce the extravasation rate. This work identified DKK-1 function in the brain metastatic niche using a vascularized microfluidic device. Recently, an omentum-on-a-chip model was developed by including ECs, adipocytes, mesothelial cells, and tumor cells to mimic tumor cell invasion from the peritoneal cavity to the sub-peritoneal space (87). Although this model was not used to study metastasis from the vasculature, it could also be used for tissue specific tumor cell extravasation studies since it contained a perfusable MVN.
Immune cell function in tumor cell extravasation
During metastasis, tumor cells encounter numerous types of immune cells, i.e. platelets, monocytes, neutrophils, NK cells, and lymphocytes (88). The immune cells are considered cooperative or antagonistic for the cancer progression depending on the nature of their complex interactions with microenvironmental factors and tumor cells (89). Previous studies have demonstrated the function of neutrophils (33), monocytes (35), and macrophages (34) in tumor cell extravasation using the microfluidic vascular models. Recently, another report explored the contribution of neutrophils and platelets in tumor cell extravasation (36). Neutrophils and platelets were co-cultured on the MDA-MB-231 monolayer for 24 hours before being perfused into the MVNs, of which the platelets promoted tumor cell mesenchymal phenotypes. Co-perfusing neutrophils and platelets with tumor cells increased the tumor cell extravasation rate. Treating the system with a clinically-approved integrin β3-targeting antiplatelet drug impaired platelet aggregation and activation, decreased mesenchymal marker expression in tumor cells, downregulated phosphorylation of VE-cadherin in ECs, lowered the permeability of the MVNs, all contributing to a reduction in tumor cell extravasation rates. This work demonstrated the capability of utilizing a microfluidic vascular platform to study immune cell and clinical drug functions in metastasis.
Extravasation of tumor cell clusters and other recent studies
It has been found that clusters of circulating tumor cells (CTCs) have a greater propensity to cause metastases than single cells (3). Several studies have therefore included perfused tumor cell clusters, in addition to single CTCs, into microfluidic vasculature to mimic circulating tumor cluster transportation in vivo (59, 90). Recently, a two-step seeding method was developed to generate perfusable MVNs with physiological-like small diameters, where tumor cell clusters (containing 2-4 cells) were trapped and tracked to monitor extravasation (91). Another vessel-on-a-chip platform was developed to study CTC and endothelium interactions (43). Prostate cancer (PC3) single cells or clusters were perfused through an EC-coated channel with a diameter over 200 µm and extravasated under HGF stimulation. Tumor cells extravasated without visibly disrupting the integrity of the endothelium, but tumor clusters destroyed the integrity of the vessels in a brutal and faster extravasation manner.
3D MVNs were established in microfluidics to compare the extravasation rate of lung cancer cells with different EMT phenotypes (92). A549 holoclone cells with an epithelial and stem-like phenotype, and A549 paraclone cells with a mesenchymal phenotype, were perfused into MVNs and cultured for 24 hours. Paraclones extravasated across the vessels whereby holoclones remained inside the microvasculature. Another vessel-on-a-chip model was developed to demonstrate breast cancer cell MDA-MB-231 extravasation in the presence of a chemoattractant, CXCL12 (53). The mold of this device was laser-cut and the gel channel and medium channel were separated with a porous membrane. Comparing the migration rate of cancer cells with or without an EC monolayer towards CXCL12 over 24 hours, the authors discovered that MDA-MB-231 cells required at least 10 hours to cross the endothelium. The underlying mechanisms were left for future studies.
An iPSC-derived vessel-on-a-chip model was employed to explore cancer-vascular interactions during cancer cell extravasation (41). The implanted iPSC-ECs formed a single lumen structure allowing intravascular perfusion of tumor cells. Within hours of co-culture, the perfused tumor cells adhered to the endothelium and extravasated out of the vessel. MDA-MB-231 breast cancer cells upregulated endothelial ICAM-1 expression through paracrine signaling. In contrast, extravasating cancer cells reduced endothelial collagen IV deposition via cell-cell physical interactions. In addition, inflammatory cytokines such as MMP-3, IL-6, and IL-8 were significantly upregulated in cancer-vascular co-culture conditions, resulting in increased vascular permeability and correspondingly increased trans-endothelial cancer cell migration, which were consequently inhibited by the addition of therapeutic inhibitors of these cytokines.
Challenges, future perspectives, and conclusions
Since the concept of the organ-on-a-chip has been introduced, numerous studies have demonstrated that microfluidic vascular models could closely mimic key steps of metastasis. Despite many promising contributions of these models, there are still several challenges to overcome.
First, there remain many challenges in tissue engineering of cancer. Although several efforts have been made to robustly generate perfusable vasculature in vitro (91, 93, 94), micro-vessels with small diameters, high perfusability, specific tissue-type, and high physiological/pathological relevance are needed. This could be address through the use of primary cells from patients to generate an improved pathological model for personalized medicine. Most studies generated vasculature using model ECs and stromal cells (e.g., HUVECs and normal lung FBs), usually lacking the pathology-associated features of patient cells. Whether and to what extent cancer in one location affects the vasculature in a remote metastatic site, however, remains an open question. In addition, most studies use cancer cell lines. Although these have been shown in many studies to behave in a similar manner to patient cancer cells, some cell lines lose their original cancer characteristics along multiple passaging. Thus, primary tumor cells, especially CTCs/clusters, along with patient-derived vascular cells are recommended to establish better models to mimic metastasis. The use of patient-iPSC-derived cells is a viable alternative for personalized models providing accurate prediction and treatment of diseases. Another challenge of current microfluidic (and other in vitro) systems is the choice of culture medium. Most studies used media that favor endothelium survival, but this may compromise physiological/pathological relevance. More efforts are needed to create a new medium that better mimics whole blood.
Second, challenges also arise in the basic engineering of the models. External equipment often connects to microfluidics to apply mechanical stimulations, such as FSS. Such equipment could be a barrier for researchers lacking engineering experience. Continued efforts are needed to develop actuators and new microfluidic systems with high accessibility and compatibility. While miniaturized microfluidic systems have the advantage of requiring only a small number of cells, conventional biological assays often demand a large number of cells. Thus, highly-sensitive assays need to be incorporated into current platforms. Multi-organ microfluidic systems combining two or more organotypic modules are being developed. Currently, modules are connected through a simple microchannel/tubing, which impedes the ability to independently regulate individual modules’ functions. A precisely controlled system should be developed to mimic each organotypic microenvironment while connected through tubing, or better yet, living vasculature.
Regarding experimental techniques, some new directions could be explored in the future. The studies of tumor cell extravasation heavily rely on imaging-based assays, requiring cell labeling, immunostaining, etc. Integrating real-time detection systems into the microfluidic assays could enable continuous tracking of cell dynamics and microenvironmental changes during metastasis. Other imaging-related probes could be integrated to study these dynamic changes, such as chemical or genetic biosensors (calcium sensor, pH sensor, cell membrane tension sensor, kinase phosphorylation FRET sensor) in real time (95). Other imaging techniques could also be utilized, such as super-resolution imaging, traction force microscopy, optical tweezers, and atomic force microscopy (AFM), although some challenges have to be solved first (95). For example, a new open-top design will be needed to allow the AFM probe to access vessels or tumor cells. Besides imaging, other assays could also be used, such as Luminex ELISA for secretome analysis, Seahorse XF for real-time metabolic analysis, and next-generation sequencing for gene expression analysis.
One barrier to using RNA sequencing is how to collect extravasated cells and separate them from the non-extravasated ones. Microneedle systems might be able to extract single extravasated tumor cells from matrix, but the throughput would be low. Another strategy is to ablate endothelium on demand using chemical inducible cell death tools (96). For example, ECs could be engineered to express the inducible cell death system and further form a perfusable vasculature. After tumor cells are perfused into the vasculature and extravasation has occurred, then the non-extravasated tumor cells could be eliminated from vasculature by a high intravascular flow. Next, the chemical agent to specifically induce EC death could be introduced, dead cell debris removed by washing, and the extravasated tumor cells remaining in the stroma collected by matrix digestion and sorted. These tumor cells could be used for both bulk and single-cell RNA-seq to study the chances during extravasation.
As for new tissue type platforms, liver is among the most highly metastasized tissues, but there are only a few liver tissue-specific microfluidic vascular models. One recent study generated liver-specific endothelium using liver sinusoidal ECs in a membrane based microfluidic device, and demonstrated that transforming growth factor β1 (TGFβ1) in the breast cancer-derived extracellular vesicles (EVs) increased cancer cell adhesion to liver endothelium (55). However, more work is still needed to study tumor cell extravasation in the liver microenvironment. Similarly, although some in vitro studies focus on tumor cell interaction with lymphatic vessels, none address the potential for tumor cell extravasation from lymphatic vessels.
Finally, there needs to be a balance between new technology development and studies of the underlying biology of tumor cell extravasation. More collaborations are needed between the developers of the technology and the oncologists and cancer biologists probing the mechanisms of metastasis, for example, how tumor-resident intracellular bacteria, which promote CTC survival (97), would regulate tumor cell extravasation.
In summary, microfluidic vascular models have emerged that recapitulate many aspects of in vivo cancer metastasis. These engineering efforts have and continue to show enormous value in elucidating the basic mechanisms of metastasis and are pointing the way toward personalized medicine. As these approaches gain wider acceptance, they will also help to reduce, and perhaps someday replace, animal experiments. The future is bright, but there remains much to do.
Author contributions
All authors conceptualized, wrote and reviewed the manuscript under supervision by RK and JJ. All authors contributed to the article and approved the submitted version.
Funding
This work was supported by the US National Cancer Institute (U01 CA214381 and U54 CA261694) and by a grant of the Korea Health Technology R&D Project through the Korea Health Industry Development Institute (KHIDI), funded by the Ministry of Health and Welfare, Republic of Korea (no. HI20C0589).
Conflict of interest
RK is the co-founder of and holds a significant financial interest in AIM Biotech, a company that produces microfluidic devices. RK also receives research support from Amgen, Novartis, Boehringer Ingelheim, GSK, AbbVie and Roche.
The remaining authors declare that the research was conducted in the absence of any commercial or financial relationships that could be construed as a potential conflict of interest.
Publisher’s note
All claims expressed in this article are solely those of the authors and do not necessarily represent those of their affiliated organizations, or those of the publisher, the editors and the reviewers. Any product that may be evaluated in this article, or claim that may be made by its manufacturer, is not guaranteed or endorsed by the publisher.
References
1. Valastyan S, Weinberg RA. Tumor metastasis: molecular insights and evolving paradigms. Cell (2011) 147:275–92. doi: 10.1016/j.cell.2011.09.024
2. Massagué J, Obenauf AC. Metastatic colonization by circulating tumour cells. J.N. (2016) 529:298–306. doi: 10.1038/nature17038
3. Macaraniag C, Luan Q, Zhou J, Papautsky I. Microfluidic techniques for isolation, formation, and characterization of circulating tumor cells and clusters. APL Bioeng (2022) 6:031501. doi: 10.1063/5.0093806
4. Hanahan D, Weinberg RA. Hallmarks of cancer: the next generation. Cell (2011) 144:646–74. doi: 10.1016/j.cell.2011.02.013
5. Tentler JJ, Tan AC, Weekes CD, Jimeno A, Leong S, Pitts TM, et al. Patient-derived tumour xenografts as models for oncology drug development. Nat Rev Clin Oncol (2012) 9:338–50. doi: 10.1038/nrclinonc.2012.61
6. Olson B, Li Y, Lin Y, Liu ET, Patnaik A. Mouse models for cancer immunotherapy research. Cancer Discovery (2018) 8:1358–65. doi: 10.1158/2159-8290.CD-18-0044
7. Byrne AT, Alferez DG, Amant F, Annibali D, Arribas J, Biankin AV, et al. Interrogating open issues in cancer precision medicine with patient-derived xenografts. Nat Rev Cancer (2017) 17:254–68. doi: 10.1038/nrc.2016.140
8. Bakker GJ, Weischer S, Ferrer Ortas J, Heidelin J, Andresen V, Beutler M, et al. Intravital deep-tumor single-beam 3-photon, 4-photon, and harmonic microscopy. Elife (2022) 11:e63776. doi: 10.7554/eLife.63776
9. Bhatia SN, Ingber DE. Microfluidic organs-on-chips. Nat Biotechnol (2014) 32:760–72. doi: 10.1038/nbt.2989
10. Park SE, Georgescu A, Huh D. Organoids-on-a-chip. Science (2019) 364:960–5. doi: 10.1126/science.aaw7894
11. Nam U, Kim S, Park J, Jeon JS. Lipopolysaccharide-induced vascular inflammation model on microfluidic chip. Micromachines (2020) 11:747. doi: 10.3390/mi11080747
12. Zhang S, Wan Z, Kamm RD. Vascularized organoids on a chip: strategies for engineering organoids with functional vasculature. Lab Chip (2021) 21:473–88. doi: 10.1039/D0LC01186J
13. Ayuso JM, Virumbrales-Munoz M, Lang JM, Beebe DJ. A role for microfluidic systems in precision medicine. Nat Commun (2022) 13:3086. doi: 10.1038/s41467-022-30384-7
14. Lee C, Kim S, Hugonnet H, Lee M, Park W, Jeon JS, et al. Label-free three-dimensional observations and quantitative characterisation of on-chip vasculogenesis using optical diffraction tomography. Lab Chip (2021) 21:494–501. doi: 10.1039/D0LC01061H
15. Roth A, Berlin M-W. Human microphysiological systems for drug development. Science (2021) 373:1304–6. doi: 10.1126/science.abc3734
16. Yoon N, Kim S, Sung HK, Dang TQ, Jeon JS, Sweeney G. Use of 2-dimensional cell monolayers and 3-dimensional microvascular networks on microfluidic devices shows that iron increases transendothelial adiponectin flux via inducing ROS production. Biochim Biophys Acta (BBA)-General Subj (2021) 1865:129796. doi: 10.1016/j.bbagen.2020.129796
17. Wang X, Sun Q, Pei J. Microfluidic-based 3D engineered microvascular networks and their applications in vascularized microtumor models. Micromachines (Basel) (2018) 9:493. doi: 10.3390/mi9100493
18. Aazmi A, Zhou H, Li Y, Yu M, Xu X, Wu Y, et al. Engineered vasculature for organ-on-a-chip systems. Engineering. (2021) 9:131–147. doi: 10.1016/j.eng.2021.06.020
19. Liu X, Fang J, Huang S, Wu X, Xie X, Wang J, et al. Tumor-on-a-chip: from bioinspired design to biomedical application. Microsyst Nanoeng (2021) 7:50. doi: 10.1038/s41378-021-00277-8
20. Shirure VS, Hughes CCW, George SC. Engineering vascularized organoid-on-a-Chip models. Annu Rev BioMed Eng (2021) 23:141–67. doi: 10.1146/annurev-bioeng-090120-094330
21. Wu Y, Zhou Y, Qin X, Liu Y. From cell spheroids to vascularized cancer organoids: Microfluidic tumor-on-a-chip models for preclinical drug evaluations. Biomicrofluidics (2021) 15:061503. doi: 10.1063/5.0062697
22. Jung S, Jo H, Hyung S, Jeon NL. Advances in 3D vascularized tumor-on-a-Chip technology. Adv Exp Med Biol (2022) 1379:231–56. doi: 10.1007/978-3-031-04039-9_9
23. Mehta P, Rahman Z, Ten Dijke P, Boukany PE. Microfluidics meets 3D cancer cell migration. Trends Cancer (2022) 8:683–97. doi: 10.1016/j.trecan.2022.03.006
24. Hajal C, Shin Y, Li L, Serrano JC, Jacks T, Kamm R.D.J.S.A. The CCL2-CCR2 astrocyte-cancer cell axis in tumor extravasation at the brain. Science Advances (2021) 7 doi: 10.1126/sciadv.abg8139
25. Xu Z, Li E, Guo Z, Yu R, Hao H, Xu Y, et al. Design and construction of a multi-organ microfluidic chip mimicking the in vivo microenvironment of lung cancer metastasis. ACS Appl Mater Interfaces (2016) 8:25840–7. doi: 10.1021/acsami.6b08746
26. Liu W, Song J, Du X, Zhou Y, Li Y, Li R, et al. AKR1B10 (Aldo-keto reductase family 1 B10) promotes brain metastasis of lung cancer cells in a multi-organ microfluidic chip model. Acta Biomater (2019) 91:195–208. doi: 10.1016/j.actbio.2019.04.053
27. Gilardi M, Bersini S, Valtorta S, Proietto M, Crippa M, Boussommier-Calleja A, et al. The driving role of the Cdk5/Tln1/FAK(S732) axis in cancer cell extravasation dissected by human vascularized microfluidic models. Biomaterials (2021) 276:120975. doi: 10.1016/j.biomaterials.2021.120975
28. Fröse J, Chen MB, Hebron KE, Reinhardt F, Hajal C, Zijlstra A, et al. Epithelial-mesenchymal transition induces podocalyxin to promote extravasation via ezrin signaling. Cell Reports (2018) 24:962–72. doi: 10.1016/j.celrep.2018.06.092
29. Offeddu GS, Hajal C, Foley CR, Wan Z, Ibrahim L, Coughlin MF, et al. The cancer glycocalyx mediates intravascular adhesion and extravasation during metastatic dissemination. Commun Biol (2021) 4:255. doi: 10.1038/s42003-021-01774-2
30. Song J, Miermont A, Lim CT, Kamm RD. A 3D microvascular network model to study the impact of hypoxia on the extravasation potential of breast cell lines. Sci Rep (2018) 8:17949. doi: 10.1038/s41598-018-36381-5
31. Hajal C, Ibrahim L, Serrano JC, Offeddu GS, Kamm RD. The effects of luminal and trans-endothelial fluid flows on the extravasation and tissue invasion of tumor cells in a 3D in vitro microvascular platform. Biomaterials (2021) 265:120470. doi: 10.1016/j.biomaterials.2020.120470
32. Azadi S, Tafazzoli Shadpour M, Warkiani ME. Characterizing the effect of substrate stiffness on the extravasation potential of breast cancer cells using a 3D microfluidic model. Biotechnol Bioeng (2021) 118:823–35. doi: 10.1002/bit.27612
33. Chen MB, Hajal C, Benjamin DC, Yu C, Azizgolshani H, Hynes RO, et al. Inflamed neutrophils sequestered at entrapped tumor cells via chemotactic confinement promote tumor cell extravasation. Proc Natl Acad Sci U.S.A. (2018) 115:7022–7. doi: 10.1073/pnas.1715932115
34. Kim H, Chung H, Kim J, Choi DH, Shin Y, Kang YG, et al. Macrophages-triggered sequential remodeling of endothelium-interstitial matrix to form pre-metastatic niche in microfluidic tumor microenvironment. Adv Sci (Weinh) (2019) 6:1900195. doi: 10.1002/advs.201900195
35. Boussommier-Calleja A, Atiyas Y, Haase K, Headley M, Lewis C, Kamm RD. The effects of monocytes on tumor cell extravasation in a 3D vascularized microfluidic model. Biomaterials (2019) 198:180–93. doi: 10.1016/j.biomaterials.2018.03.005
36. Crippa M, Bersini S, Gilardi M, Arrigoni C, Gamba S, Falanga A, et al. A microphysiological early metastatic niche on a chip reveals how heterotypic cell interactions and inhibition of integrin subunit β 3 impact breast cancer cell extravasation. J.L.O.A.C (2021) 21:1061–72. doi: 10.1039/D0LC01011A
37. Spiegel A, Brooks MW, Houshyar S, Reinhardt F, Ardolino M, Fessler E, et al. Neutrophils suppress intraluminal NK cell–mediated tumor cell clearance and enhance extravasation of disseminated carcinoma cells neutrophil-mediated tumor cell survival and extravasation. Cancer Discovery (2016) 6:630–49. doi: 10.1158/2159-8290.CD-15-1157
38. Marturano-Kruik A, Nava MM, Yeager K, Chramiec A, Hao L, Robinson S, et al. Human bone perivascular niche-on-a-chip for studying metastatic colonization. Proc Natl Acad Sci U.S.A. (2018) 115:1256–61. doi: 10.1073/pnas.1714282115
39. Kwak TJ, Lee E. Rapid multilayer microfabrication for modeling organotropic metastasis in breast cancer. Biofabrication (2020) 13:015002. doi: 10.1088/1758-5090/abbd28
40. Jeon JS, Bersini S, Gilardi M, Dubini G, Charest JL, Moretti M, et al. Human 3D vascularized organotypic microfluidic assays to study breast cancer cell extravasation. Proc Natl Acad Sci U.S.A. (2015) 112:214–9. doi: 10.1073/pnas.1417115112
41. Humayun M, Ayuso JM, Brenneke RA, Virumbrales-Munoz M, Lugo-Cintron K, Kerr S, et al. Elucidating cancer-vascular paracrine signaling using a human organotypic breast cancer cell extravasation model. Biomaterials (2021) 270:120640. doi: 10.1016/j.biomaterials.2020.120640
42. Chen MB, Lamar JM, Li R, Hynes RO, Kamm RD. Elucidation of the roles of tumor integrin β1 in the extravasation stage of the metastasis CascadeRole of tumor integrin β1 in extravasation. Cancer Research (2016) 76:2513–24. doi: 10.1158/0008-5472.CAN-15-1325
43. Wu Y, Zhou Y, Paul R, Qin X, Islam K, Liu Y. Adaptable microfluidic vessel-on-a-Chip platform for investigating tumor metastatic transport in bloodstream. Anal Chem (2022) 94:12159–66. doi: 10.1021/acs.analchem.2c02556
44. Xu H, Li Z, Yu Y, Sizdahkhani S, Ho WS, Yin F, et al. A dynamic in vivo-like organotypic blood-brain barrier model to probe metastatic brain tumors. Sci Rep (2016) 6:36670. doi: 10.1038/srep36670
45. Roberts SA, Waziri AE, Agrawal N. Development of a single-cell migration and extravasation platform through selective surface modification. Anal Chem (2016) 88:2770–6. doi: 10.1021/acs.analchem.5b04391
46. Beyer S, Blocki A, Cheung MCY, Wan ZHY, Mehrjou B, Kamm RD. Lectin staining of microvascular glycocalyx in microfluidic cancer cell extravasation assays. Life (Basel) (2021) 11:179. doi: 10.3390/life11030179
47. Azadi S, Mohammadi E, Tafazzoli-Shadpour M, Tabatabaei M. Effects of chemically EGFR targeting on non-targeted physical cell behaviors in 2D and 3D microfluidic cultures of invasive and non-invasive breast cancer cell lines. Biochem Biophys Res Commun (2022) 622:1–7. doi: 10.1016/j.bbrc.2022.07.013
48. Bertulli C, Gerigk M, Piano N, Liu Y, Zhang D, Muller T, et al. Image-assisted microvessel-on-a-Chip platform for studying cancer cell transendothelial migration dynamics. Sci Rep (2018) 8:12480. doi: 10.1038/s41598-018-30776-0
49. Mei X, Middleton K, Shim D, Wan Q, Xu L, Ma YV, et al. Microfluidic platform for studying osteocyte mechanoregulation of breast cancer bone metastasis. Integr Biol (Camb) (2019) 11:119–29. doi: 10.1093/intbio/zyz008
50. Brett ME, Bomberger HE, Doak GR, Price MA, Mccarthy JB, Wood DK. In vitro elucidation of the role of pericellular matrix in metastatic extravasation and invasion of breast carcinoma cells. Integr Biol (Camb) (2018) 10:242–52. doi: 10.1039/C7IB00173H
51. Mollica H, Palomba R, Primavera R, Decuzzi P. Two-channel compartmentalized microfluidic chip for real-time monitoring of the metastatic cascade. ACS Biomater Sci Eng (2019) 5:4834–43. doi: 10.1021/acsbiomaterials.9b00697
52. Yu J, Lee S, Song J, Lee SR, Kim S, Choi H, et al. Perfusable micro-vascularized 3D tissue array for high-throughput vascular phenotypic screening. Nano Converg (2022) 9:16. doi: 10.1186/s40580-022-00306-w
53. Asaumi Y, Sasaki N. Photolithography-free vessel-on-a-chip to simulate tumor cell extravasation. Sensors Materials (2021) 33:241–50. doi: 10.18494/SAM.2021.3073
54. Oliver CR, Westerhof TM, Yang BA, Merrill NM, Yates JA, Russell L, et al. Characterization of secretory cues that promote brain metastasis using a microfluidic blood brain niche (BBN) device. Cancer Res (2022) 82:3189–9. doi: 10.1158/1538-7445.AM2022-3189
55. Kim J, Lee C, Kim I, Ro J, Kim J, Min Y, et al. Three-dimensional human liver-chip emulating premetastatic niche formation by breast cancer-derived extracellular vesicles. ACS Nano (2020) 14:14971–88. doi: 10.1021/acsnano.0c04778
56. Choi B, Choi J-W, Jin H, Sim H-R, Park J-H, Park T-E, et al. Condensed ECM-based nanofilms on highly permeable PET membranes for robust cell-to-cell communications with improved optical clarity. J.B (2021) 13:045020. doi: 10.1088/1758-5090/ac23ad
57. Coughlin MF, Kamm RD. The use of microfluidic platforms to probe the mechanism of cancer cell extravasation. Adv Healthc Mater (2020) 9:e1901410. doi: 10.1002/adhm.201901410
58. Kim S, Kim W, Lim S, Jeon JS. Vasculature-On-A-Chip for In vitro disease models. Bioengineering (Basel) (2017) 4:8. doi: 10.3390/bioengineering4010008
59. Chen MB, Whisler JA, Frose J, Yu C, Shin Y, Kamm RD. On-chip human microvasculature assay for visualization and quantification of tumor cell extravasation dynamics. Nat Protoc (2017) 12:865–80. doi: 10.1038/nprot.2017.018
60. Shin Y, Han S, Jeon JS, Yamamoto K, Zervantonakis IK, Sudo R, et al. Microfluidic assay for simultaneous culture of multiple cell types on surfaces or within hydrogels. Nature Protocols (2012) 7:1247–59. doi: 10.1038/nprot.2012.051
61. Lee S, Lim J, Yu J, Ahn J, Lee Y, Jeon NL. Engineering tumor vasculature on an injection-molded plastic array 3D culture (IMPACT) platform. Lab Chip (2019) 19:2071–80. doi: 10.1039/C9LC00148D
62. Ayuso JM, Rehman S, Virumbrales-Munoz M, Mcminn PH, Geiger P, Fitzgerald C, et al. Microfluidic tumor-on-a-chip model to evaluate the role of tumor environmental stress on NK cell exhaustion. Science Advances (2021) 7. doi: 10.1126/sciadv.abc2331
63. Kim S, Park J, Kim J, Jeon JS. Microfluidic tumor vasculature model to recapitulate an endothelial immune barrier expressing FasL. ACS Biomaterials Sci Eng (2021) 7:1230–41. doi: 10.1021/acsbiomaterials.0c01542
64. Park J, Kim S, Hong J, Jeon JS. Enabling perfusion through multicellular tumor spheroid promoting lumenization in vascularized cancer model. Lab Chip (2022). doi: 10.1039/D2LC00597B
65. Ahn SI, Sei YJ, Park H-J, Kim J, Ryu Y, Choi JJ, et al. Microengineered human blood–brain barrier platform for understanding nanoparticle transport mechanisms. Nature Communications (2020) 11:1–12. doi: 10.1038/s41467-019-13896-7
66. Mondrinos MJ, Yi Y-S, Wu N-K, Ding X, Huh D. Native extracellular matrix-derived semipermeable, optically transparent, and inexpensive membrane inserts for microfluidic cell culture. Lab Chip (2017) 17:3146–58. doi: 10.1039/c7lc00317j
67. Park SM, Kim H, Song KH, Eom S, Park H, Doh J, et al. Ultra-thin, aligned, free-standing nanofiber membranes to recapitulate multi-layered blood vessel/tissue interface for leukocyte infiltration study. J.B. (2018) 169:22–34. doi: 10.1016/j.biomaterials.2018.03.053
68. Offeddu GS, Haase K, Gillrie MR, Li R, Morozova O, Hickman D, et al. An on-chip model of protein paracellular and transcellular permeability in the microcirculation. J.B. (2019) 212:115–25. doi: 10.1016/j.biomaterials.2019.05.022
69. Bittner KR, Jiménez JM, Peyton SR. Vascularized biomaterials to study cancer metastasis. Advanced Healthcare Materials (2020) 9:1901459. doi: 10.1002/adhm.201901459
70. Sontheimer-Phelps A, Hassell BA, Ingber DE. Modelling cancer in microfluidic human organs-on-chips. Nat Rev Cancer (2019) 19:65–81. doi: 10.1038/s41568-018-0104-6
71. Ma YHV, Middleton K, You LD, Sun Y. A review of microfluidic approaches for investigating cancer extravasation during metastasis. Microsystems Nanoengineering (2018) 4:1–13. doi: 10.1038/micronano.2017.104
72. Mondadori C, Crippa M, Moretti M, Candrian C, Lopa S, Arrigoni C. Advanced microfluidic models of cancer and immune cell extravasation: A systematic review of the literature. Front Bioeng Biotechnol (2020) 8:907. doi: 10.3389/fbioe.2020.00907
73. Mansoorifar A, Gordon R, Bergan R, Bertassoni LE. Bone-on-a-chip: microfluidic technologies and microphysiologic models of bone tissue. Adv Funct Mater (2021) 31:2006796. doi: 10.1002/adfm.202006796
74. Parlato S, Grisanti G, Sinibaldi G, Peruzzi G, Casciola CM, Gabriele L. Tumor-on-a-chip platforms to study cancer-immune system crosstalk in the era of immunotherapy. Lab Chip (2021) 21:234–53. doi: 10.1039/D0LC00799D
75. Zhang X, Karim M, Hasan MM, Hooper J, Wahab R, Roy S, et al. Cancer-on-a-Chip: Models for studying metastasis. Cancers (Basel) (2022) 14:648. doi: 10.3390/cancers14030648
76. Mitchell MJ, King MR. Physical biology in cancer. 3. the role of cell glycocalyx in vascular transport of circulating tumor cells. Am J Physiol Cell Physiol (2014) 306:C89–97. doi: 10.1152/ajpcell.00285.2013
77. Tarbell JM, Cancel LM. The glycocalyx and its significance in human medicine. J Intern Med (2016) 280:97–113. doi: 10.1111/joim.12465
78. Eke I, Cordes N. Focal adhesion signaling and therapy resistance in cancer. Semin Cancer Biol (2015) 31:65–75. doi: 10.1016/j.semcancer.2014.07.009
79. Yankaskas CL, Bera K, Stoletov K, Serra SA, Carrillo-Garcia J, Tuntithavornwat S, et al. The fluid shear stress sensor TRPM7 regulates tumor cell intravasation. Sci Adv (2021) 7:eabh3457. doi: 10.1126/sciadv.abh3457
80. Han W, Chen S, Yuan W, Fan Q, Tian J, Wang X, et al. Oriented collagen fibers direct tumor cell intravasation. Proc Natl Acad Sci U.S.A. (2016) 113:11208–13. doi: 10.1073/pnas.1610347113
81. Stowers RS, Shcherbina A, Israeli J, Gruber JJ, Chang J, Nam S, et al. Matrix stiffness induces a tumorigenic phenotype in mammary epithelium through changes in chromatin accessibility. Nat BioMed Eng (2019) 3:1009–19. doi: 10.1038/s41551-019-0420-5
82. Follain G, Osmani N, Gensbittel V, Asokan N, Larnicol A, Mercier L, et al. Impairing flow-mediated endothelial remodeling reduces extravasation of tumor cells. Sci Rep (2021) 11:13144. doi: 10.1038/s41598-021-92515-2
83. Obenauf AC, Massagué J. Surviving at a distance: organ-specific metastasis. Trends in Cancer (2015) 1:76–91. doi: 10.1016/j.trecan.2015.07.009
84. Gao Y, Bado I, Wang H, Zhang W, Rosen JM, Zhang XH. Metastasis organotropism: Redefining the congenial soil. Dev Cell (2019) 49:375–91. doi: 10.1016/j.devcel.2019.04.012
85. Nguyen DX, Bos PD, Massagué J. Metastasis: from dissemination to organ-specific colonization. Nature Reviews Cancer (2009) 9:274–84. doi: 10.1038/nrc2622
86. Bos PD, Zhang XH, Nadal C, Shu W, Gomis RR, Nguyen DX, et al. Genes that mediate breast cancer metastasis to the brain. Nature (2009) 459:1005–9. doi: 10.1038/nature08021
87. Ibrahim LI, Hajal C, Offeddu GS, Gillrie MR, Kamm RD. Omentum-on-a-chip: A multicellular, vascularized microfluidic model of the human peritoneum for the study of ovarian cancer metastases. Biomaterials (2022) 288:121728. doi: 10.1016/j.biomaterials.2022.121728
88. Kitamura T, Qian BZ, Pollard JW. Immune cell promotion of metastasis. Nat Rev Immunol (2015) 15:73–86. doi: 10.1038/nri3789
89. Ho WJ, Wood LD. Opposing roles of the immune system in tumors. Science (2021) 373:1306–7. doi: 10.1126/science.abl5376
90. Chen MB, Whisler JA, Jeon JS, Kamm RD. Mechanisms of tumor cell extravasation in an in vitro microvascular network platform. Integr Biol (Camb) (2013) 5:1262–71. doi: 10.1039/c3ib40149a
91. Wan Z, Zhong AX, Zhang S, Pavlou G, Coughlin MF, Shelton SE, et al. A robust method for perfusable microvascular network formation In vitro. Small Methods (2022) 6:e2200143. doi: 10.1002/smtd.202200143
92. Zeinali S, Rechberger K, Dubey C, Gashi R, Marti T, Dorn P, et al. 130P metastasis-on-chip: 3D human microvasculature network to study extravasation dynamics of lung cancer in vitro. Ann Oncol (2022) 33:S92. doi: 10.1016/j.annonc.2022.02.159
93. Wan Z, Zhang S, Zhong AX, Shelton SE, Campisi M, Sundararaman SK, et al. A robust vasculogenic microfluidic model using human immortalized endothelial cells and Thy1 positive fibroblasts. Biomaterials (2021) 276:121032. doi: 10.1016/j.biomaterials.2021.121032
94. Zhang S, Wan Z, Pavlou G, Zhong AX, Xu L, Kamm RD. Interstitial flow promotes the formation of functional microvascular networks In vitro through upregulation of matrix metalloproteinase-2. Advanced Funct Materials (2022) 32:2206767. doi: 10.1002/adfm.202206767
95. Wan Z, Shaheen S, Chau A, Zeng Y, Liu W. Imaging: Gear up for mechano-immunology. Cell Immunol (2020) 350:103926. doi: 10.1016/j.cellimm.2019.103926
96. Song HG, Lammers A, Sundaram S, Rubio L, Chen AX, Li L, et al. Transient support from fibroblasts is sufficient to drive functional vascularization in engineered tissues. Adv Funct Mater (2020) 30:2003777. doi: 10.1002/adfm.202003777
Keywords: cancer extravasation, microfluidic chip, preclinical disease model, in vitro vascular model, pre-metastatic microenvironment
Citation: Kim S, Wan Z, Jeon JS and Kamm RD (2022) Microfluidic vascular models of tumor cell extravasation. Front. Oncol. 12:1052192. doi: 10.3389/fonc.2022.1052192
Received: 23 September 2022; Accepted: 26 October 2022;
Published: 11 November 2022.
Edited by:
Yunfei Wang, Tianjin Medical University General Hospital, ChinaReviewed by:
Tae-Eun Park, Ulsan National Institute of Science and Technology, South KoreaCopyright © 2022 Kim, Wan, Jeon and Kamm. This is an open-access article distributed under the terms of the Creative Commons Attribution License (CC BY). The use, distribution or reproduction in other forums is permitted, provided the original author(s) and the copyright owner(s) are credited and that the original publication in this journal is cited, in accordance with accepted academic practice. No use, distribution or reproduction is permitted which does not comply with these terms.
*Correspondence: Jessie S. Jeon, anNqZW9uQGthaXN0LmFjLmty; Roger D. Kamm, cmRrYW1tQG1pdC5lZHU=
†These authors have contributed equally to this work