- 1School of Information Science and Technology, Fudan University, Shanghai, China
- 2Academy for Engineering and Technology, Fudan University, Shanghai, China
- 3Shanghai Fifth People’s Hospital, Fudan University, Shanghai, China
- 4Zhongshan Fudan Joint Innovation Center, Zhongshan, China
Osteosarcoma (OS) is the most common primary malignant bone tumor that mainly affects the pediatric and adolescent population; limb salvage treatment has become one of the most concerned and expected outcomes of OS patients recently. Phototherapy (PT), as a novel, non-invasive, and efficient antitumor therapeutic approach including photodynamic therapy (PDT), photothermal therapy (PTT), and photobiomodulation therapy (PBMT), has been widely applied in superficial skin tumor research and clinical treatment. OS is the typical deep tumor, and its phototherapy research faces great limitations and challenges. Surprisingly, pulse mode LED light can effectively improve tissue penetration and reduce skin damage caused by high light intensity and has great application potential in deep tumor research. In this review, we discussed the research progress and related molecular mechanisms of phototherapy in the treatment of OS, mainly summarized the status quo of blue light PBMT in the scientific research and clinical applications of tumor treatment, and outlooked the application prospect of pulsed blue LED light in the treatment of OS, so as to further improve clinical survival rate and prognosis of OS treatment and explore corresponding cellular mechanisms.
Introduction
Osteosarcoma (OS) is the high-grade malignant bone tumor, mainly occurring in children and adolescents but a second and smaller peak in elderly patients (1). It exhibits a preference to arise in the metaphysis of long bones and the sites of rapid bone growth, such as the distal femur (43%), proximal tibia (23%), proximal humerus (10%), skull, mandible, and other sites (2, 3). Despite the high survival rate of OS (70%∼80%) with traditional therapy nowadays, around 20% after metastasis is still dismal (3, 4). In particular, limb salvage, postoperative quality of life, and inhibition of metastasis are the most important issues in the current treatment of OS (3, 5). Therefore, the exploration of new treatment methods is extremely important.
At present, some new antitumor methods, such as targeted therapy and immunotherapy, continue to play an important role in the treatment of a variety of cancers (6, 7). However, due to the defects of drug resistance and specific treatment, there are still many problems in clinical treatment. On the other hand, phototherapy, a novel, non-invasive, and efficient antitumor therapy, including photodynamic therapy (PDT), photothermal therapy (PTT), and photobiomodulation therapy (PBMT), has gradually become a hot spot in the current scientific research and clinical experiments of OS (8). Currently, the laser and LED are two common light sources in phototherapy. LED devices are gradually widely applied in cancer treatment research fields because of their cheapness, large radiation range, and wearability (9). Therefore, LED phototherapy will develop rapidly in deep cancer treatment in the future.
Current research shows that continuous wave light PBMTs have been gradually applicated in dermatology (acne, eczema, and psoriasis) and skin cancer in vitro and in vivo. For example, Oh et al. (2015) found that blue light had antiproliferative and proapoptotic effects on melanoma cells and reduced tumor growth in mouses (10). Chen et al. (2021) summarized the research status of light therapy for melanoma in the past 20 years and also found that blue light can effectively inhibit the proliferation of melanoma cells (11). Besides, the growth of malignant glioma (12), mouse B-cell lymphoma (13), colon cancer (14), and pancreatic cancer (15) can be effectively inhibited by blue light in animal experiments. However, due to the limited tissue penetration of continuous wave light, there are still limitations in the study of deeper tumors. On the other hand, pulse mode LED light can make up for the defect of low tissue penetration of continuous wave light due to high peak irradiance (16, 17). At the same time, adjusting the pulse mode can effectively ensure the stability of temperature, so as to achieve the inhibitory effect of high light intensity on superficial epidermal tumors such as skin tumors (11). Although some studies have shown that phototherapy can effectively inhibit tumor proliferation in vivo and in vitro, numerous studies have not carried out long-term safety testing, so the safer spectral application mode and subsequent safety testing related research need to be further explored.
In brief, we described the development and current treatments for OS before focusing on research progress from traditional therapies to new therapeutic approaches including targeted therapy, immunotherapy, PTT, PDT, and PBMT that played an irreplaceable role in improving survival rate of clinical for this disease. Finally, we mainly focused on the research status of PBMT of blue LED light in the treatment of OS, looked ahead to the results of existing research in this laboratory, and further discussed the research prospect of blue light and the related mechanism of phototherapy in OS.
Occurrence and metastasis of osteosarcoma
OS is a genetically diverse and karyotypically complex cancer characterized by chromosomal instability, copy number alterations, and chromothripsis, mainly originated from bone with high concentrations of mesenchymal progenitor cells and developed in bone (18, 19). A number of studies identify mesenchymal stem cells (MSCs) or committed osteoblast precursors as the OS cell of origin (20). As research on OS deepens, more etiologies are identified such as several chemical agents (beryllium, methylcholanthrene), viruses (FBJ), and hereditary diseases (Li–Fraumeni syndrome, Rothmund–Thomson syndrome, and Bloom syndrome), while leading to prevalent alterations in tumor-suppressor genes, including TP53 (>90%), RB1 (29%), DLG2 (53%), and ATRX (29%) (21, 22). As mentioned earlier, the tumor-suppressor pathway regulated by p53 and Rb is one of the main factors involved in the etiopathogenesis of OS, mainly regulated in DNA damage and cell-cycle progress (23). Chou and Gorlick (2006) provided a paradigm in the potential pathogenesis of OS, mainly including the main development mechanisms of precursor cells and early OS cells (19, 24).
Recent findings also indicate that OS progression is a multistep and complicated process associated with an intricate tumor microenvironment. The mesenchymal stroma played a critical role in OS growth, maintenance, and micrometastasis, and the interactions between OS cells and multiple factors (cytokines, exosomes, metabolites) in mesenchymal stroma reveal the complexity of OS occurrence (19).
OS cells perform a high propensity to spread and metastasize, which seems to be the most important internal factor for poor prognosis in OS patients (2). The inability to detect the occurrence of OS metastasis in a timely and rapid manner is the main reason for the low cure rate of metastatic patients. Therefore, new detection methods and comprehensive and in-depth understanding of the molecular mechanisms of OS metastasis are urgently needed to develop. Studies have shown that primary OS mainly metastasizes to the lungs (81.2%), and other metastatic sites include bone and lymph nodes (25). Many researchers have conducted sweeping research on OS metastasis. Sheng et al. (2021) reviewed the metastasis process of OS according to the existing research results and provided a comprehensive understanding of cross-regulatory networks in metastasis based on the diverse biological behaviors and associated mechanistic pathways of OS metastasis (3). Bruland et al. (2005) used a sensitive immunomagnetic detection assay that successfully identified micrometastases from bone marrow and peripheral blood in OS patients (26). Guan et al. (2015) developed a specific molecular probe named CXCR4-targeted near-infrared (NIR) fluorescent imaging agent to detect pulmonary micrometastases in OS cells and mouse xenograft models, with the hope to apply in micrometastasis of human OS (27). Wang et al. (2018) found that ssDNA aptamer LP-16, as a promising molecular probe, can achieve significant targeting efficiency for OS lung-metastasis diagnosis (28).
At present, the primary diagnosis and detection of OS micrometastasis are necessary and important to improve the survival of patients. Further, developing and identifying new therapeutic strategies to combat metastasis is also the key to the treatment of OS.
Treatment of osteosarcoma
Traditional treatment of osteosarcoma
Nowadays, despite the development of new therapeutic approaches, the prevailing methods are still surgery, chemotherapy, and radiotherapy. The combination of multiagent chemotherapy with surgical resection or radiation therapy drastically improved the survival rate to become the standard therapy for OS. However, the prognosis of the patients has not improved considerably since then, and limited therapeutic progress of OS treatment has been made for several decades.
Nowadays, the most effective dosage regimen of drugs was still the combination of high-dose methotrexate, doxorubicin, and cisplatin, usually referred to as MAP, similarly to the first agents in the mid-1970s (29). Nevertheless, adding other new chemotherapeutic agents such as ifosfamide and etoposide into the regimen does not improve prognosis effectively (30, 31). Furthermore, problems of drug resistance and side effects, such as cardiac toxicity, nephrotoxicity, and other rare toxicities, have existed subsequently (32). Drug resistance to conventional combination therapies including chemotherapy, as an important obstacle in OS treatment, is closely related to the changes in tumor metabolism (10). Thus, the broader availability of therapeutic techniques and correlative molecular profiling with the biological insights should be further explored in OS treatment.
Molecular targeted therapy of osteosarcoma
Molecular targeted therapy refers to the utilization of drugs or other substances that target specific molecules to prevent the growth and spread of tumor cells. It is one of the promising methods to further improve the survival rate of patients with OS. Sayles et al. (2019) further extended the non-overlapping whole-genome sequencing (WGS) dataset of OS, which indicated the characterizations of OS genomes consistent with those previously found such as notable gene copy number alterations and multiple structural rearrangements, while six OS subclassifications will be divided by the key gene signaling pathways activated, namely, cyclin E/CDK2, MYC/CDK9, CDK4/CDK6/FOXM1, PTEN/AKT1/PI3K/mTOR, AURKB, and VEGFA/VEGFR, mainly regulating biological processes of the cell cycle, proliferation, cell-cycle progression during G1–S, signaling, mitosis, and signaling angiogenesis, respectively (6). Subsequently, Liu et al. (2021) summarized the preclinical and developmental research of targeted anti-angiogenesis therapy for OS in detail and described the relevant biological mechanism more comprehensively, which further determined the research prospects of targeted therapy, although it was still uncertain in clinical trials (33). Among them, multiple tyrosine kinase inhibitors, such as sorafenib, regorafenib, cabozantinib, lenvatinib, pazopanib, and everolimus, have shown certain efficacy in small sample studies and need to be further confirmed (34). Also, although some drugs targeting the cell cycle and DNA repair like palbociclib (CDK4/6), alisertib (AURKA), and dinaciclib (CDK2) have not been evaluated in clinical trials of OS, those drugs have been proved to induce apoptosis and inhibit cell proliferation in vitro or in vivo (35).
On the other hand, molecular targeted therapy models of OS included patient-derived xenograft (PDX) and transgenic models, which played an important role in the study of the biological characteristics and clinical trials of human OS (36, 37). However, a number of drawbacks in targeted therapy based on the concept of precision medicine still remain.
Hence, molecular targeted therapy for OS should be predicated on the selection of biomarkers to increase the possibility that a drug is effective in heterogeneous patient populations, rather than defining therapeutic activity through the direct relationship between the target and the drug (30). Therefore, targeted therapy needs further research to evaluate its potential role as a promising novel therapy in the treatment of OS in the future.
Immunotherapy of osteosarcoma
Studies have shown that OS is a tumor susceptible to immunotherapy, which is largely attributed to the OS cells derived from multipotent mesenchymal stem cells and the changes in the tumor microenvironment (TME) (7, 38). Indeed, more than 100 years ago, surgeon William Coley (1891, 1910) found that the use of a mixture of bacterial toxins to treat patients with bone and soft tissue sarcoma successfully subsided the tumor and first envisaged the immune component of OS treatment (39, 40). In recent years, with the rapid development of immunotherapy, it has been widely used in a variety of malignant tumors. Chen et al. (2021) summarized the current status and breakthroughs of immunotherapy for OS (41). Although the current reports on the clinical immunotherapy of OS are limited, immunotherapy is still considered to be a promising option for the treatment of OS and one of the important approaches to further improve the overall survival rate of patients.
At present, lung metastasis and recurrence of OS are still the main factors limiting the improvement of survival rate. Therefore, activation of associated cells of the TME is one of the therapeutic strategies of OS, tumor-associated macrophages (TAMs), and tumor-infiltrating lymphocytes (TILs) like macrophages and T cells play significant roles in the immune response to OS (42). A variety of immunotherapy methods, such as chimeric antigen receptor T-cell (CAR-T) therapy, immune checkpoint blocking therapy, natural killer (NK) cell infusion therapy, macrophage activation therapy (GcMAF), and oncolytic virus therapy, are constantly explored in the basic research and clinical application of malignant bone tumors. Among them, immune checkpoint inhibitors (ICIS), as a new class of therapeutic drugs, have completely changed the treatment of previously incurable malignant tumors, stimulated interest in this therapeutic method, and opened a new door for the immunotherapy of OS (43).
Besides, although immunotherapy has not yet observed surprising effects in OS clinical treatment, the continuous emergence of newly discovered immune checkpoint blockade targets has created a new dawn for the exploration of OS treatment. Therefore, we believe that unprecedented progress and major breakthroughs will be made in the treatment and understanding of OS in the near future.
Phototherapy of osteosarcoma
In recent decades, the survival rate of OS has reached a platform period. Although the combination of surgery and other treatments has improved the cure rate of OS to around 70%, the bone defect and complex skeletal rebuilding of the affected limb caused by surgery still limit the application (5). Therefore, more promising novel therapeutic approaches to minimally invasive treatment are receiving more and more attention in tumor-related research. Phototherapy is the clinical application of using light to treat specific diseases, which is safe, non-invasive, economical, and easy to handle, including PDT and PTT (8). Finson obtained a Nobel Prize in Physiology or Medicine in 1903 for discovering the therapeutic effect of light on diseases such as smallpox and lupus. Subsequently, the healing properties to wounds and cytotoxic characteristics for tumor cells of light were gradually identified and widely used in minimally invasive cancer therapy until now (44, 45). On the other hand, PBM, also known as low-level light therapy (LLLT), is a non-thermal and non-invasive therapy that uses light with a specific spectrum (390–1100 nm) to regulate cell life activities and produce therapeutic effects (46, 47). At present, PBM has been widely used to reduce inflammation, relieve pain, and promote wound healing (48). Meanwhile, PBM is also applicated in tumor treatment such as melanoma due to its non-invasive advantages, also named PBMT (10, 11). Accordingly, research of the novel therapies such as PDT, PTT, and PBMT for tumor treatment is extremely important. Here, we have comprehensively summarized the main research progress (Figure 1) and related molecular mechanisms (Figure 2) of phototherapy in OS.
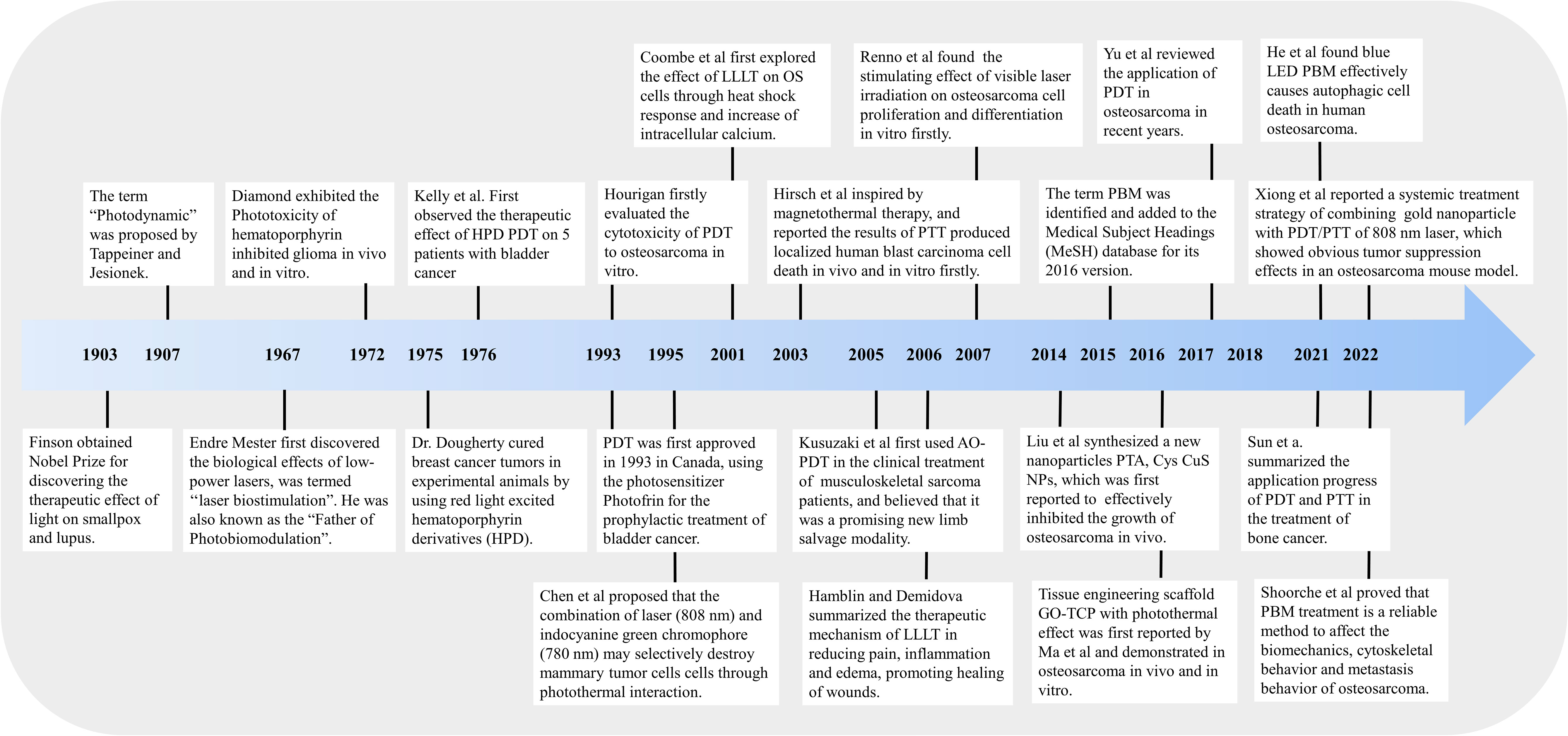
Figure 1 Schematic diagram of research progress of phototherapy (PDT, PTT, and PBMT) in the treatment of osteosarcoma.
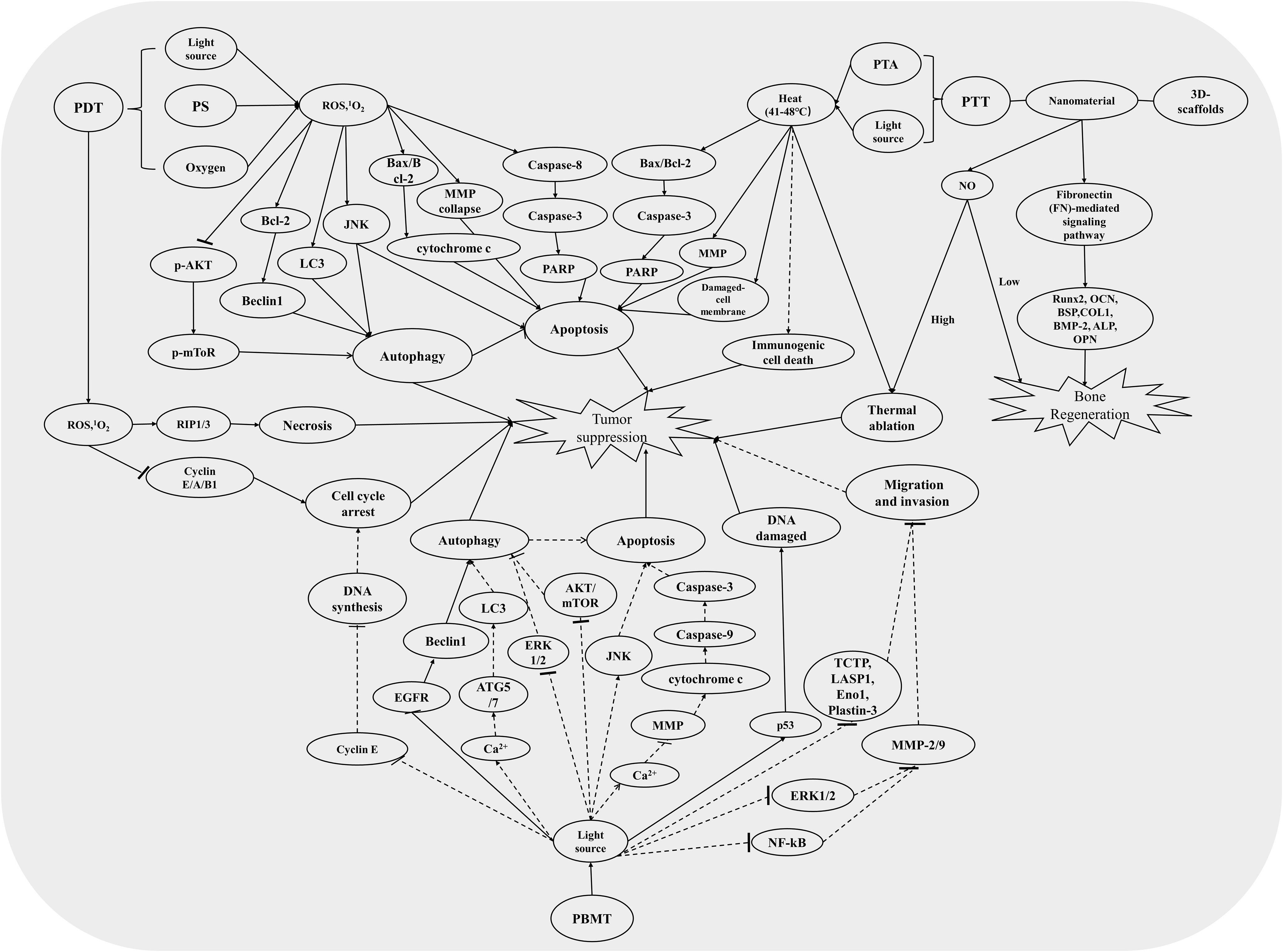
Figure 2 The relevant mechanism pathways involved in PDT, PTT, and PBMT induced an antitumor effect. The solid line represents the pathways that have been studied in the treatment of osteosarcoma, and the dotted line represents the pathways that have been studied in the treatment of other tumors but not in osteosarcoma.
Photodynamic therapy of osteosarcoma
PDT, as a non-invasive treatment, has been widely developed and applied in a variety of cancer treatments because of phototoxicity produced by reactive oxygen species (ROS) to kill tumor cells since first approved for clinical applications using the photosensitizer Photofrin for bladder cancer in Canada in 1993 (49). Three basic factors of PDT, namely, photosensitizer (PS), light source, and oxidative stress (, ROS), were exploited to kill tumor cells. The specific mechanism is that the PSs are activated from the ground state to the excited state under the action of light of a specific wavelength, and the activated PSs are able to directly react with the substrate (such as a cell membrane or molecule) to form free radicals and interact with oxygen to produce ROS (type I reaction) or direct energy transfer to oxygen to form singlet oxygen (1O2), causing cell damage by 1O2 directly (type II reaction) (49, 50). In sum, light energy is converted into chemical energy through appropriate PSs in PDT. The selective photodamage and cytotoxicity are important factors in the treatment of different cancers with PDT (51). Also, Dolmans et al. (2003) summarized three main mechanisms of PDT affecting tumor growth, namely, direct cancer cell killing by ROS, vascular damage, and immune response (49).
PS, as one of the important components of PDT, had been summarized in OS treatment by Yu et al. (2017); they found that although PDT had gradually become a hot spot in cancer treatment research, related research in OS was still insufficient, and PSs were limited to more than a dozen types mainly including acridine orange (50, 52). However, the rapid development of nanoparticles (NPs) has provided a major breakthrough for the PS defects of PDT in cancer treatment, including deeper tumors such as OS. The characteristics of modification and enhanced permeability and retention (EPR) effects of NPs reduce the sedimentation and cytotoxicity of PS in the liver, kidney, and other normal organs and tissues and enhance the application prospect of PDT in the treatment of OS recently (53). At the meantime, Yu et al. (2017) summarized seven feasible improvements in OS PDT, including the further exploration of PSs and light sources, and the combination with other treatment technologies such as targeted therapy and immunotherapy (50). Therefore, further study of PSs will contribute to the prominent development and application of PDT in the treatment of OS.
Furthermore, PS could target cancer cells and destroy them with the excitation of appropriate wavelength and light intensity (54). As early as 1993, Hourigan et al. firstly evaluated the cytotoxicity of PDT to OS in vitro. They found that the cell viability of human osteosarcoma cells (HOS) was inversely proportional to the energy dose (Dose = Irradiance × Time) after incubation with Photofrin at 3 μg/ml for 72 h followed by light exposure (630 nm, 600 mW). The cell viability is less than 50% when the energy dose was greater than 7 J/cm2, effectively inhibiting the proliferation of OS cells (55). Later, Kusuzaki et al. (2005) applied photodynamic therapy using acridine orange (1 μg/ml) (AO-PDT) to the clinical treatment of 10 musculoskeletal sarcomas patients, only one patient had local tumor recurrence, and the limb function in other patients returned to normal without other complications. They believed that AO-PDT had an obvious inhibitory effect on the local recurrence and was a promising novel therapy for patients of musculoskeletal sarcoma (56). Subsequently, a number of PSs such as 5-methylene blue (57, 58), aminolevulinic acid (ALA) (59, 60), HiPorfin (61), 5,15-bis(2-bromo-5-hydroxyphenyl) porphyrin (62), aloe-emodin (60), Foscan and Foslip (63), pyropheophorbide-α methyl ester (MPPa) (64), and zinc phthalocyanine-bovine serum albumin (ZnPc/BSA) nanoparticle (65), were widely applied in scientific research and clinical treatment of OS. Substantial research about light wavelength mainly focused on 600–800 nm; the NIR spectral region can effectively affect the deeper tissues (66). Also, with the rapid improvements of lighting technology, LED gradually became common in OS PDT due to its superior abilities to control light parameters. For example, White et al. (2016) found that the OS MG-63 had the highest cell death rate under 1 mM ALA after illumination with 3 J/cm2 LED light (636 nm) (67). Another study conducted by Tu et al. (2016) also found notable toxicity in MG-63 human osteosarcoma cells at 10 µM aloe-emodin and 4.8 J/cm2 LED light irradiation conditions (430 nm) (60). Also, Yu et al. synthesized ZnPc/BSA nanoparticle as a specific PS to evaluate the antitumor effects of ZnPc/BSA-induced PDT on OS under LED illumination (660 nm, 0.5 W/cm2, 1.8 kJ/cm2) (65).
Besides, ROS plays an extremely important role in PDT on cancer treatments. In Sun’s research, they assessed the ROS level using carboxy-H2DCFDA as an ROS indicator. They found a remarkable increase of ROS in OS cells treated with HiPorfin-PDT and that the expression levels of proteins (Bcl-2, Bax, cleaved-caspase-3, cleaved PARP-1) related to apoptosis and cycle arrest were significantly increased (61). Another study showed that AE-PDT effectively reduced the viability of MG-63 cells and induced autophagy and apoptosis by activating the ROS-JNK signaling pathway (60). Besides, a wealth of studies indicated that the PDT on OS treatment also concentrates on ROS-induced cell death; the relevant pathways, such as apoptosis and necrosis by cell-cycle arrest, vessel injury, autophagy, and immune response, had been concluded (50, 68). We had summarized the mainly molecular mechanism of PDT in the treatment of OS in Figure 2. Nevertheless, although the depth of OS is less than that of glioma and pancreatic cancer, as a deeper tumor (shallower than other deep tumors), due to the coverage of muscle and skin, there will be insufficient light and surface tissue damage in PDT (69). Recently, two-photon photodynamic therapy has been gradually studied and applied in malignant tumors, including osteosarcoma, because of its deeper tissue penetration and oxygen independent characteristics (70, 71). Dobos and coworkers (2019) explored the efficiency of two-photon excited PDT (TPE-PDT) in a 3D osteosarcoma model; results showed a considerable decrease in cell viability after TPE-PDT (72). Nonetheless, there are still limitations like the lack of efficient and safe two-photon PSs and difficulties in practical application of double pulsed laser sources. Research on PDT in OS is still in the tumor model experiments, and its clinical application is still very limited. Thus, more comprehensive and in-depth research should be explored in the field of PDT.
Photothermal therapy of osteosarcoma
PTT is also a minimally invasive, controllable, and highly effective antitumor therapeutic modality (73). In early 1995, Chen et al. firstly found that indocyanine green and 808-nm diode laser combined to produce a photothermal effect could kill breast tumor cells (74). Subsequently, the utilization of PTT for cancer therapy was first reported by Hirsch et al. in 2003, which was inspired by magnetothermal therapy (75). In 2014, Liu et al. synthesized a new nanoparticle PTA, Cys CuS NPs, which was first reported to effectively inhibit the growth of osteosarcoma in vivo (76). Then, a large number of studies have been published on the application of PTT on OS in vivo and in vitro since 2014. Here, we present key findings in Figure 1.
Two key elements in PTT, namely, photothermal agent (PTA) and light source, were exploited to kill tumor cells. At present, four main types of PTAs, namely, metal, carbon, semiconductor, and organic molecule-based materials, were widely investigated in PTT (8). However, sometimes heat would inevitably leak out of the target tissue and damage the surrounding normal tissue due to the limitations of PTAs previously. Therefore, with the dramatic progress of PTAs by modification and nanoformulation, some nanomaterials, as the most promising PTAs with continuous in-depth research nowadays, were gradually used as effective photothermal sensors because of their unique properties and special chemical structures, which could effectively generate heat in the target cancer tissue through the optical absorption, instead of the healthy tissues (73). Additionally, a specific light wavelength, mainly including NIR light, will be absorbed by PTAs to efficiently convert to heat by local irradiation of the tumor regions. As tumor cells are more sensitive to heat-induced cytotoxicity, when the local tumor area temperature increases to the range of 41°C–48°C owing to high photothermal conversion performance, heat induced cell biological processes and caused cancer cell death (77). Recently, PTT with other therapies, such as chemotherapy and immunotherapy, was able to more comprehensively and efficiently improve the treatment efficacy of different cancers (50).
At present, the majority of studies on PTT treatment of OS mainly focus on the synergistic treatment of nanomaterials, modifying substances or other chemotherapy-related drugs (8). Among them, gold nanoparticles (AuNPs) are extensively studied in OS PTT because of the higher NIR absorption coefficient and stability compared with other nanomaterials. Liu et al. (2017) first designed a gold nanoshell-coated betulinic acid liposome (AuNS-BA-Lip) delivery system, which strongly absorbed the 808-nm NIR laser (2 W/cm2, 10 min) and showed the pronounced antitumor effects in 143B cells and corresponding tumor-bearing mice (78). Recently, a systemic treatment strategy of a gold nanoparticle combined with PDT/PTT by 808-nm laser showed obvious tumor suppression effects in an osteosarcoma mouse model (79). Also, Sun et al. (2021) comprehensively summarized the progress of PTT and PDT in bone cancer (8). On the other hand, some nanoparticle-integrated 3D-printing scaffolds with PTT played an important role in OS treatment and NO-associated bone regeneration (80). Therefore, PTT, as a novel and practicable therapeutic approach, will also become a promising diagnosis and therapeutic method for OS.
Also, the molecular mechanisms related to the treatment of OS by PTT have also been studied, although they have not been fully elucidated. Studies have shown that the inhibitory effect of PTT on OS is related to apoptosis (81–83) and thermal ablation pathways (84, 85). Flow cytometry is widely used in apoptosis analysis. Lee and colleagues (2021) demonstrated that apoptotic cells were more than necrotic cells on the PLIN (indocyanine green and diethylenetriamine/nitric oxide adduct-loaded polylactic acid) monoliths after laser irradiation (808 nm, 0.7 W/cm2, 5 min) in OS MG63 cells; the expression levels of related proteins, such as cleaved PARP and cleaved caspase-3, were remarkably increased (81). Dang et al. (2021) had similar results in vitro, and further study in mice found that a special scaffold had a remarkable antitumor effect on OS mice after NIR laser irradiation (808 nm, 0.9 W/cm2, 10 min) and no obvious damage in mice’s other organs (82). Some researchers also discovered that a multifunctional biomaterial system combined with PTT plays a significant role in OS therapy and bone regeneration (64, 85). Moreover, Dong et al. (2020) reported a multifunctional bioceramic platform with carbon aerogel (CA), which plays an efficient therapeutic effect in OS through thermal ablation and promotes bone regeneration via the fibronectin-mediated signaling pathway; the expression levels of a substantial number of osteogenic genes such as ALP, BMP2, OCN, and OPN are dramatically upregulated (84). Here, we summarized the molecular mechanisms of PTT inhibition of OS related to most of the findings, as shown in Figure 2.
Photobiomodulation therapy of osteosarcoma
PBMT is one of the exciting hotspots in the current biomedical research field. Actually, as early as more than 50 years ago, surgeon Endre Mester, known as “father of photobioinduction,” first discovered the biological effects of low-dose laser light and recorded the experiment of the Arndt-Schultz biophysical law of laser light (86). Therefore, this treatment method had been called low-level laser therapy (LLLT). Until 2015, the more precise and comprehensive term PBM was identified and added to the Medical Subject Headings (MeSH) database for its 2016 version (46). Also, in addition to the laser as the light source, other new types of optical devices such as LED and broadband light sources are gradually applied in the field of treatment.
At present, most studies indicate that PBMT plays an important role in the aspects of wound healing (87), anti-inflammatory (88), traumatic brain injury (89), and related clinical treatment applications (48). In addition, PBMT is gradually emerging in oncology applications and its prognosis. A substantial number of studies showed that PBMT had a significant inhibitory effect on melanoma cells under suitable light parameters. At the same time, the related molecular mechanisms have also been deeply studied. Ohara et al. (2002) found that 470-nm LED blue light inhibited the proliferation of melanoma cells associated with cell-cycle arrest (90). Oh et al. (2015) observed that blue light can further induce cell apoptosis and inhibit the proliferation of melanoma B16-F10 cells by activating the mitochondria (10). Chen et al. (2022) summarized the effect of different light parameters on the growth of melanoma and its molecular mechanism; they believed that the inhibitory effect of PBMT is related to the influence of OPN photoacceptors and the activation of mitochondria and some signaling pathways (11). A similar result was evaluated by Shakibaiea et al. (2020), using breast cancer cells (MCF-7) with inhibition of cell proliferation and metabolic activation by 435-nm LED irradiation (91). Besides, the authors also found that radiation with a wavelength of 629 nm induces enhanced metabolic activity including the expression of lactate dehydrogenase A and glutaminase (91). Meanwhile, Kara et al. (2018) showed that LLLT irradiation (1,064 nm, 100 mJ) promoted the proliferation of osteosarcoma cells (Saos2) and lung carcinoma cells (A549) (92). In addition, Zhang et al. (2008) reported that low-power laser irradiation (<50 J/cm2) by He–Ne laser (632.8 nm) promoted Hela cell viability by induced ROS-mediated Src activation (93). Also, Tam et al. (2020) raised the question that normal cells and tumor cells have different responses to LLLT (94). In sum, the controversial results on cancer cells are associated with various factors; wavelength is one of the main influencing factors. A substantial number of studies showed that blue light PBMT played a significant role in inhibiting cancer cell proliferation (90, 91, 95). Therefore, OS, as one of the malignant bone tumors, had been found to have a stimulating effect of infrared laser irradiation as early as 2001 (96). Although it had no significant effect on cell proliferation, heat shock response and the increase of intracellular calcium had been found in OS cells (96). Subsequently, Renno et al. (2006) found that visible light (670 nm) also had a stimulating effect on OS cells (97). Recently, Feng et al. (2019) reported the results on the combination of blue LED irradiation and ATO suppressed cell proliferation, increased apoptosis, inhibited cell migration and invasion, and found that it was related to ROS accumulation and DNA damage-mediated p53 activation (95). Besides, He et al. (2021) found that blue LED PBMT effectively causes autophagic cell death in human OS cells, which was induced by promoting ROS and the EGFR/Beclin-1-mediated signaling pathway (98). Recently, Shoorche et al. (2022) also discovered that red laser irradiation (650 nm, 780 nm) inhibited OS cell migration and provided a quantitative description of cytoskeleton changes through a structure of F−actin analysis (99). Therefore, PBMT has great research value and prospects in the treatment of OS. More key research progresses and related molecular mechanisms on PBMT are shown in Figures 1, 2.
In recent years, increasing evidence has shown that different light parameters, including wavelength, irradiance, and energy densities, have different effects among different tumor types including OS, promoting or inhibiting proliferation (100). However, with the rapid development of LED technology, the preferred light source has been gradually developed in the treatment of a variety of PBMT diseases because of their lower cost, high variability of wavelengths, safety of device, and wearability compared with laser devices (13, 101). Hence, blue LED light PBMT will become one of the most promising approaches for the treatment of OS.
Blue light photobiomodulation therapy in cancer treatment
The biological function of blue light in osteosarcoma and other cancers
Several studies have indicated that light irradiation (LED or laser) can trigger various physiological processes by affecting human skin, which is pretty significant for human health (102, 103). In the current studies, we have found that the exploration of ultraviolet and far-infrared light has been deeply analyzed, and visible light has also been found to have various biological functions (46). Blue light (400–500 nm) LED irradiation is known to have many promising effects and can be used in different treatment modalities. Although the role of blue light irradiation in mammalian cellular molecular processes and its application in phototherapy applications is currently poorly understood, this emerging field is receiving increasing attention.
Blue light triggers signal cascades and corresponding cellular reactions through a molecular photoreceptor or an endogenous photosensitizer to affect some biological systems. Current research indicates that photoreceptors of blue light in plants and bacteria have relatively mature research results. For example, cryptochromes have obvious light-dependent functions, but there are still disputes on cryptochromes as a photoreceptor in mammals (104, 105). Despite the controversy in illustrating relevant photoacceptors (chromophores) of blue light in mammal cells, five different photoacceptors, namely, flavins (106–108), porphyrins (109, 110), nitrosated proteins (111, 112), opsins (OPN) (113–115), and cytochrome c oxidase (CCO) (116–118), have been identified in non-pigmented mammalian cells (keratinocytes and fibroblasts), while previous studies mainly focused on pigmented cells, such as retinal ganglion cells (119, 120). Blue light stimulates OPN to cause structural changes and induce the activity of the downstream transient receptor protein (TRP) channel (TRPV1), then calcium influx activates CaMKII, which affects the gene transcription level in the nucleus and further affects cell promotion and differentiation, vasorelaxation, and barrier homeostasis (113–115). Flavin (460 nm) and porphyrin (410–440 nm) absorb blue light to produce ROS and activate NFκB, TGFβ, Nrf2, and MAPK signaling pathway and further inhibit cell proliferation, induce apoptosis and necrosis, and reduce inflammation (106–110). Nitrosated proteins (420–490 nm) induce the non-enzymatic release of bioactive compounds in the blue spectrum and play an important role in anti-inflammatory effect and cell differentiation (111, 112, 114). The PBM effect of CCO mainly affects the mitochondrial activity through the mitochondrial electron transport chain and then affects the biological mechanism of cells (116, 117). On the other hand, CCO contain porphyrin and also have the function of nitrite reductase to produce NO in mitochondria; it is considered as another possible mechanism of CCO blue light response (114, 118). Moreover, Becker et al. (2016) found that aryl hydrocarbon receptor (AHR) may be a possible target for blue light PBM (121). Moreover, 6-formylindolo[3,2-b] carbazole (FICZ), as a photoinduced ligand of the aryl hydrocarbon receptor (AHR), is a tryptophan photoproduct, which has been widely studied (122). These studies on blue light receptors play an important role in exploring biological mechanisms. Therefore, we briefly summarized the current main research on blue light receptors and their related biological mechanisms as shown in Figure 3.
Considerable research showed that phototherapy based on blue light has produced beneficial consequences such as treatment of neonatal jaundice (123–125), regulation of melatonin and circadian rhythm (126–128), anti-inflammation (129–131), immunomodulation (132), wound healing (133–135), tissue regeneration, and anticancer therapies (103, 114, 133, 136). Furthermore, blue light reduced the follicular colonization of Propionibacterium acnes by activating the endogenous bacterial porphyrin and inhibiting acne development, while possibly interfering with lipogenesis in adipocytes and suppressing sebum formation for acne treatment (137). Blue light irradiation (450 nm) induced the release of nitric oxide (NO) from cytochrome oxidase and other mitochondrial heme proteins but can reverse the effects of NO and improve mitochondrial function, while the absorption of light by hemoglobin can cause local temperature increase, promoting wound healing through coagulation effect (138, 139). Kim et al. (2016) demonstrated that the anti-inflammatory effect of blue light (410 nm) on human epithelial keratinocytes (NHEKs) was associated with NO and S-nitrosylation (140). Also, blue light irradiation (420 nm) affected the proliferation, differentiation, and growth of human dermal fibroblasts and even had dose-related toxic effects (141). Becker et al. (2016) discovered that the proliferation of immortalized human keratinocytes (HaCaT) induced by blue light showed a biphasic dose–response curve, similarly to the PBM conclusion proposed by Hamblin et al. (121). Furthermore, Oh et al. repeatedly found that blue light could even reduce cell viability and induce apoptosis of different types of cancer cells (10, 13, 14). Those findings determined the effect of blue light on the proliferation, differentiation, apoptosis, inflammation, vasorelaxation, and barrier homeostasis of cells, while others describe cytotoxicity (142). Despite controversy over the role of blue light, these results and conclusions still play a significant role in the exploration of the physiological role of blue light, also laying a biological foundation for the clinical application of blue light in phototherapy.
However, if the light parameters are not appropriate, blue light will also be harmful to cells, which is described as cytotoxicity. Relevant studies have shown that blue light with high dose/irradiance may cause damage to keratinocytes, fibroblasts, retinal epithelial cells, skin-derived endothelial cells, and other mammalian cells in addition to pigmented cells (143). Consequently, the biological effects of LED blue light irradiation are dose-dependent; it is necessary to define parameters like wavelength, dose (fluence, energy density), irradiance (intensity, power density), light mode (continuous wave, pulsed wave (peak irradiance, duty cycle)), treatment interval, light source type, and cellular species so as to obtain specifically the cellular responses and better clinical treatment effect of cancers in the future (143).
Application of blue light PBMT in different cancers
Blue light, as a visible light with a wavelength range of 400–500 nm, has been clinically applied successfully comprising neonatal jaundice, psoriasis (Pv), atopic dermatitis (AD), eczema, acne, and other inflammatory skin conditions, although the number of available clinical studies evaluating the efficacy of blue light treatment is still limited (103, 136). Furthermore, recent studies have demonstrated that blue light irradiation inhibits the proliferation of multiple types of cancer cells in vitro and in vivo, including colon cancer cells (14, 144, 145), malignant glioma cells (12), melanoma cells (10, 146, 147), B-cell lymphoma cells (13), fibrosarcoma cells (14), pancreatic cancer cells (15), cutaneous squamous cell carcinoma cells (CSCC), epidermoid carcinoma cells (148, 149), leukemia cells (Kasumi-1) (150), bladder cancer cells (151), colorectal cancer cells (152, 153), breast cancer cells (91), and human OS cells (95, 98). Here, we have briefly summarized the relevant research of blue light PBMT in the field of cancer treatment (Table 1).
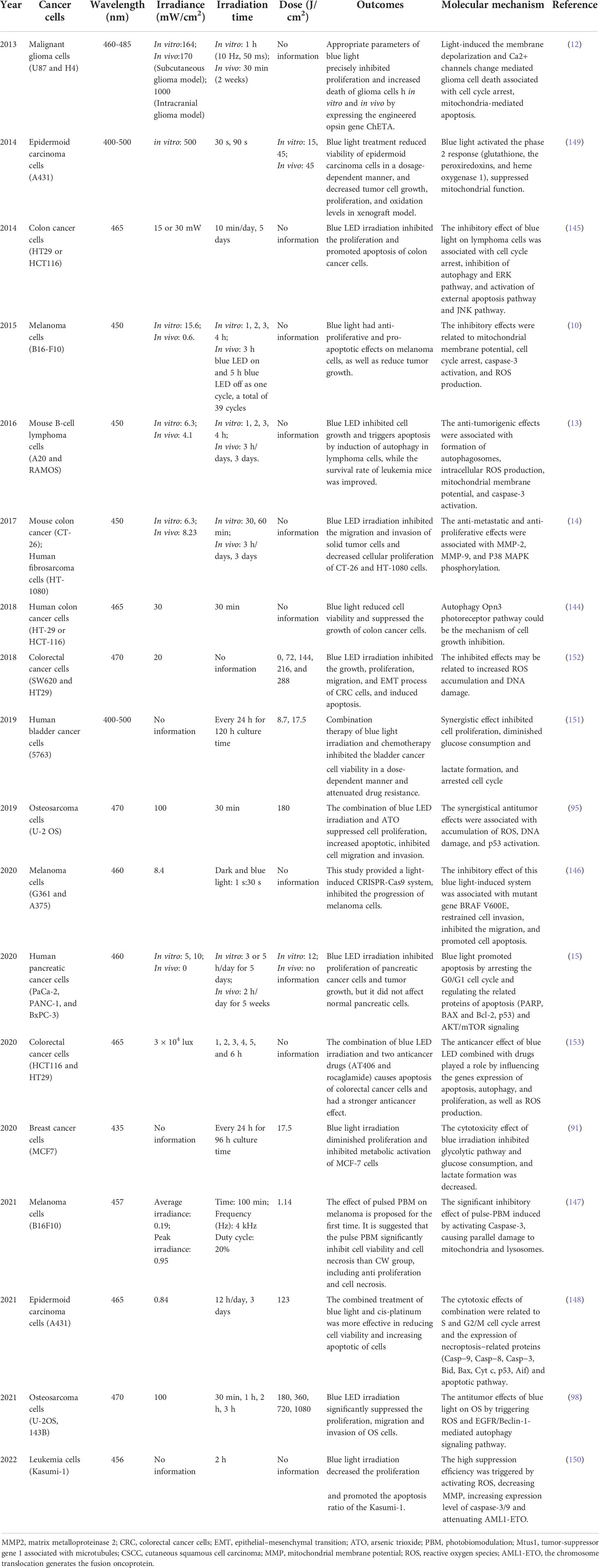
Table 1 Summary of the most relevant experimental studies on cancers treatment by blue light irradiation.
The relationship between blue light and bone metabolism has become the focus of attention in the field of orthopedics recently. Blue light stimulation (480 nm) induced an increase in tartrate-resistant acid phosphatase activity of RAW264.7 cells while promoting osteoclast differentiation (154). Yuan et al. (2017) also found that blue light (470 nm) inhibited the proliferation of bone marrow-derived mesenchymal stem cells and osteogenic differentiation but promoted apoptosis (142). Consequently, we inferred that blue LED light irradiation showed significantly suppressed effects in multiple cancer cell lines including cells associated with bone metabolism. Although the mechanisms leading to cell death were not identical in each cell line analyzed, it was reasonable to believe that blue light irradiation was one of the important and effective anticancer therapies in the clinical treatment of future.
At present, a number of studies and clinical treatments have shown that OS therapy still have some limitations such as multidrug resistance, high cost, and the initial stage of the therapeutic methods (10, 32, 33). Lately, blue LED irradiation-based phototherapy has gradually emerged as a new approach to the treatment of cancers. Although the efficacy of blue light LED therapy in the treatment of OS has not been clarified, some studies have shown that blue light also has an inhibitory effect on OS cells. Feng et al. (2019) observed that the combination treatments of blue LED irradiation (470 nm) and antitumor agent-arsenic trioxide exert synergistical antitumor effects in OS cells, and the synergistic manner suppressed cell proliferation, migration, invasion, and induced apoptosis of OS cells (95). Simultaneously, they proposed relevant biological mechanisms related to ROS accumulation, DNA damage, and p53 activation (95). Additionally, He et al. (2021) evaluated the antitumor effects of LED blue light therapy individually on human OS cells for the first time, and the results showed that blue LED irradiation significantly inhibited the proliferation, migration, and invasion of human OS cells by triggering ROS, inducing cell apoptosis and autophagy pathways, providing a potential approach and strategy for human OS treatment (98). Also, the investigators found that circadian rhythm may be closely related to endogenous control of tumor progression, including pancreatic adenocarcinoma (155), Glasgow osteosarcoma (155), breast cancer (156), and colorectal tumor (157). We know that light is one of the important factors affecting circadian rhythm. Cry1 and Cry2 (cryptochrome circadian clock) are the important core clock genes that achieve tumor suppression by affecting the cell cycle (158). Therefore, we inferred that Cry1 and Cry2 will play an essential role in the process of OS suppression by blue LED light.
Also, this laboratory found that pulsed blue light could significantly inhibit OS cell line MG63, which was related to SOCS3 (suppressor of cytokine signaling 3) protein (not shown). Therefore, we speculated that a difference in the inhibitory mechanism of pulsed light and continuous wave light on OS cells may exist. Also, the phenotype of blue light inhibiting OS cells is consistent with the results we summarized, showing that blue LED irradiation suppressed proliferation in various cancer cells (Table 1).
Although some cell experiments have basically verified that blue light has an inhibitory effect on OS, relevant clinical treatments have not been reported, so the clinical application of blue light LED irradiation for OS still faces great challenges. Hence, it is necessary to further strengthen the research of blue light therapy in the treatment of OS in vivo and in vitro, and the relevant molecular and cellular mechanisms still need to be further explored.
Conclusion
Here, we comprehensively summarized the therapeutic advances of OS, reviewed the current situation of traditional treatment methods of OS, and prospected the research progress of new non-invasive phototherapy on the prognosis of OS. Among them, blue photobiomodulation therapy (PBMT) has been paid more attention to in cancer treatment because of its simple operation and effective results currently. A substantial number of studies demonstrated that blue light effectively inhibited tumor proliferation. Although blue continuous wave light PBMT in different cancer cell lines and superficial skin tumors had made some research progress, it still faces great limitations in deep tumor treatment. Inhibition of tumor growth by blue LED light may also be related to the effects of SOCS3 and CRY1/2, which needs further verification. In sum, in-depth studies of a series of treatment methods and related tumor killing mechanisms are expected to break the status quo, stating that the survival rate of osteosarcoma has reached the platform in recent decades and further improved the cure rate and prognosis of patients with OS.
Author contributions
ML and YL contributed to the design of the review and checked and revised the manuscript. JY collected information and wrote the main manuscript. QF and HJ edited the manuscript. ML and YL served as the authors responsible for contact and ensuring communication. All authors contributed to the article and approved the submitted version.
Funding
This work was supported by grants from the National Natural Science Foundation of China (No. 81671376).
Conflict of interest
The authors declare that the research was conducted in the absence of any commercial or financial relationships that could be construed as a potential conflict of interest.
Publisher’s note
All claims expressed in this article are solely those of the authors and do not necessarily represent those of their affiliated organizations, or those of the publisher, the editors and the reviewers. Any product that may be evaluated in this article, or claim that may be made by its manufacturer, is not guaranteed or endorsed by the publisher.
References
1. Mirabello L, Troisi RJ, Savage SA. Osteosarcoma incidence and survival rates from 1973 to 2004 data from the surveillance, epidemiology, and end results program. Cancer (2009) 115(7):1531–43. doi: 10.1002/cncr.24121
2. Bielack SS, Kempf-Bielack B, Delling G, Exner GU, Flege S, Helmke K, et al. Prognostic factors in high-grade osteosarcoma of the extremities or trunk: An analysis of 1,702 patients treated on neoadjuvant cooperative osteosarcoma study group protocols. J Clin Oncol (2002) 20(3):776–90. doi: 10.1200/JCO.2002.20.3.776
3. Sheng G, Gao Y, Yang Y, Wu H. Osteosarcoma and metastasis. Front Oncol (2021) 11:780264. doi: 10.3389/fonc.2021.780264
4. Anderson ME. Update on survival in osteosarcoma. Orthop Clin North Am (2016) 47(1):283–+. doi: 10.1016/j.ocl.2015.08.022
5. Tiwari A. Current concepts in surgical treatment of osteosarcoma. J Clin Orthop Trauma (2012) 3(1):4–9. doi: 10.1016/j.jcot.2012.04.004
6. Sayles LC, Breese MR, Koehne AL, Leung SG, Lee AG, Liu HY, et al. Genome-informed targeted therapy for osteosarcoma. Cancer Discovery (2019) 9(1):46–63. doi: 10.1158/2159-8290.CD-17-1152
7. Wedekind MF, Wagner LM, Cripe TP. Immunotherapy for osteosarcoma: Where do we go from here? Pediatr Blood Cancer (2018) 65(9):e27227. doi: 10.1002/pbc.27227
8. Sun JC, Xing F, Braun J, Traub F, Rommens PM, Xiang Z, et al. Progress of phototherapy applications in the treatment of bone cancer. Int J Mol Sci (2021) 22(21):11354. doi: 10.3390/ijms222111354
9. Salehpour F, Mahmoudi J, Kamari F, Sadigh-Eteghad S, Rasta SH, Hamblin MR. Brain photobiomodulation therapy: A narrative review. Mol Neurobiol (2018) 55(8):6601–36. doi: 10.1007/s12035-017-0852-4
10. Oh PS, Na KS, Hwang H, Jeong HS, Lim S, Sohn MH, et al. Effect of blue light emitting diodes on melanoma cells: Involvement of apoptotic signaling. J Photochem Photobiol B (2015) 142:197–203. doi: 10.1016/j.jphotobiol.2014.12.006
11. Chen Z, Huang S, Liu M. The review of the light parameters and mechanisms of photobiomodulation on melanoma cells. Photodermatol Photoimmunol Photomed (2022) 38(1):3–11. doi: 10.1111/phpp.12715
12. Yang F, Tu J, Pan JQ, Luo HL, Liu YH, Wan J, et al. Light-controlled inhibition of malignant glioma by opsin gene transfer. Cell Death Dis (2013) 4:e893. doi: 10.1038/cddis.2013.425
13. Oh PS, Hwang H, Jeong HS, Kwon J, Kim HS, Kim M, et al. Blue light emitting diode induces apoptosis in lymphoid cells by stimulating autophagy. Int J Biochem Cell Biol (2016) 70:13–22. doi: 10.1016/j.biocel.2015.11.004
14. Oh PS, Kim HS, Kim EM, Hwang H, Ryu HH, Lim S, et al. Inhibitory effect of blue light emitting diode on migration and invasion of cancer cells. J Cell Physiol (2017) 232(12):3444–53. doi: 10.1002/jcp.25805
15. Kim YM, Ko SH, Shin YI, Kim Y, Kim T, Jung J, et al. Light-emitting diode irradiation induces AKT/mTOR-mediated apoptosis in human pancreatic cancer cells and xenograft mouse model. J Cell Physiol (2020) 236(2):1362–74. doi: 10.1002/jcp.29943
16. Barbora A, Bohar O, Sivan AA, Magory E, Nause A, Minnes R. Higher pulse frequency of near-infrared laser irradiation increases penetration depth for novel biomedical applications. PloS One (2021) 16(1):e0245350. doi: 10.1371/journal.pone.0245350
17. Hashmi JT, Huang YY, Sharma SK, Kurup DB, De Taboada L, Carroll JD, et al. Effect of pulsing in low-level light therapy. Lasers Surg Med (2010) 42(6):450–66. doi: 10.1002/lsm.20950
18. Smida J, Xu HG, Zhang YP, Baumhoer D, Ribi S, Kovac M, et al. Genome-wide analysis of somatic copy number alterations and chromosomal breakages in osteosarcoma. Int J Cancer (2017) 141(4):816–28. doi: 10.1002/ijc.30778
19. Cortini M, Avnet S, Baldini N. Mesenchymal stroma: Role in osteosarcoma progression. Cancer Lett (2017) 405:90–9. doi: 10.1016/j.canlet.2017.07.024
20. Mutsaers AJ, Walkley CR. Cells of origin in osteosarcoma: mesenchymal stem cells or osteoblast committed cells? Bone (2014) 62:56–63. doi: 10.1016/j.bone.2014.02.003
21. Fuchs B, Pritchard DJ. Etiology of osteosarcoma. Clin Orthop Relat Res (2002) 397):40–52. doi: 10.1097/00003086-200204000-00007
22. Chen X, Bahrami A, Pappo A, Easton J, Dalton J, Hedlund E, et al. Recurrent somatic structural variations contribute to tumorigenesis in pediatric osteosarcoma. Cell Rep (2014) 7(1):104–12. doi: 10.1016/j.celrep.2014.03.003
23. Li H, Han X, Yang S, Wang Y, Dong Y, Tang T. FOXP1 drives osteosarcoma development by repressing P21 and RB transcription downstream of P53. Oncogene (2021) 40(15):2785–802. doi: 10.1038/s41388-021-01742-4
24. Chou AJ, Gorlick R. Chemotherapy resistance in osteosarcoma: Current challenges and future directions. Expert Rev Anticancer Ther (2006) 6(7):1075–85. doi: 10.1586/14737140.6.7.1075
25. Kager L, Zoubek A, Potschger U, Kastner U, Flege S, Kempf-Bielack B, et al. Primary metastatic osteosarcoma: Presentation and outcome of patients treated on neoadjuvant cooperative osteosarcoma study group protocols. J Clin Oncol (2003) 21(10):2011–8. doi: 10.1200/JCO.2003.08.132
26. Bruland OH. H.; saeter, g.; smeland, s.; fodstad, o. hematogenous micrometastases in osteosarcoma patients. Hum Cancer Biol (2005) 11(13):4666–73. doi: 10.1158/1078-0432.CCR-05-0165
27. Guan G, Lu Y, Zhu X, Liu L, Chen J, Ma Q, et al. CXCR4-targeted near-infrared imaging allows detection of orthotopic and metastatic human osteosarcoma in a mouse model. Sci Rep (2015) 5:15244. doi: 10.1038/srep15244
28. Wang L, Li P, Xiao X, Li J, Li J, Yang HH, et al. Generating lung-metastatic osteosarcoma targeting aptamers for in vivo and clinical tissue imaging. Talanta (2018) 188:66–73. doi: 10.1016/j.talanta.2018.05.011
29. Isakoff MS, Bielack SS, Meltzer P, Gorlick R. Osteosarcoma: Current treatment and a collaborative pathway to success. J Clin Oncol (2015) 33(27):3029–35. doi: 10.1200/JCO.2014.59.4895
30. Gill J, Gorlick R. Advancing therapy for osteosarcoma. Nat Rev Clin Oncol (2021) 18(10):609–24. doi: 10.1038/s41571-021-00519-8
31. Goorin AM, Harris MB, Bernstein M, Ferguson W, Devidas M, Siegal GP, et al. Phase II/III trial of etoposide and high-dose ifosfamide in newly diagnosed metastatic osteosarcoma: A pediatric oncology group trial. J Clin Oncol (2002) 20(2):426–33. doi: 10.1200/jco.20.2.426
32. Janeway KA, Grier HE. Sequelae of osteosarcoma medical therapy: A review of rare acute toxicities and late effects. Lancet Oncol (2010) 11(7):670–8. doi: 10.1016/s1470-2045(10)70062-0
33. Liu Y, Huang N, Liao S, Rothzerg E, Yao F, Li Y, et al. Current research progress in targeted anti-angiogenesis therapy for osteosarcoma. Cell Prolif (2021) 54(9):e13102. doi: 10.1111/cpr.13102
34. Greenfield EM, Collier CD, Getty PJ. Receptor tyrosine kinases in osteosarcoma: 2019 update. In: Kleinerman ES, Gorlick R, editors. Current advances in the science of osteosarcoma: Research perspectives: Tumor biology, organ microenvironment, potential new therapeutic targets, and canine models, 2nd edition. advances in experimental medicine and biology (2022) Cham: Springer (2020) 1258 p. 141–55. doi: 10.1007/978-3-030-43085-6_9
35. Zhou Y, Shen JK, Yu Z, Hornicek FJ, Kan Q, Duan Z. Expression and therapeutic implications of cyclin-dependent kinase 4 (CDK4) in osteosarcoma. Biochim Biophys Acta Mol Basis Dis (2018) 1864(5 Pt A):1573–82. doi: 10.1016/j.bbadis.2018.02.004
36. Walkley CR, Qudsi R, Sankaran VG, Perry JA, Gostissa M, Roth SI, et al. Conditional mouse osteosarcoma, dependent on p53 loss and potentiated by loss of Rb, mimics the human disease. Genes Dev (2008) 22(12):1662–76. doi: 10.1101/gad.1656808
37. Rokita JL, Rathi KS, Cardenas MF, Upton KA, Jayaseelan J, Cross KL, et al. Genomic profiling of childhood tumor patient-derived xenograft models to enable rational clinical trial design. Cell Rep (2019) 29(6):1675–+. doi: 10.1016/j.celrep.2019.09.071
38. Lin PP, Pandey MK, Jin FH, Raymond AK, Akiyama H, Lozano G. Targeted mutation of p53 and Rb in mesenchymal cells of the limb bud produces sarcomas in mice. Carcinogenesis (2009) 30(10):1789–95. doi: 10.1093/carcin/bgp180
39. Coley WB. Contribution to the nowledge of sarcoma. Ann Surg (1891) 14:199–220. doi: 10.1097/00000658-189112000-00015
40. Coley WB. The treatment of inoperable sarcoma by bacterial toxins (the mixed toxins of the streptococcus erysipelas and the bacillus prodigiosus). Proc R Soc Med (1910) 3:1–48. doi: 10.1177/003591571000301601
41. Chen C, Xie L, Ren T, Huang Y, Xu J, Guo W. Immunotherapy for osteosarcoma: Fundamental mechanism, rationale, and recent breakthroughs. Cancer Lett (2021) 500:1–10. doi: 10.1016/j.canlet.2020.12.024
42. Corre I, Verrecchia F, Crenn V, Redini F, Trichet V. The osteosarcoma microenvironment: A complex but targetable ecosystem. Cells-Basel (2020) 9(4):976. doi: 10.3390/cells9040976
43. Roberts SS, Chou AJ, Cheung NK. Immunotherapy of childhood sarcomas. Front Oncol (2015) 5:181. doi: 10.3389/fonc.2015.00181
44. Allison RR, Sibata CH. Oncologic photodynamic therapy photosensitizers: A clinical review. Photodiagnosis Photodyn Ther (2010) 7(2):61–75. doi: 10.1016/j.pdpdt.2010.02.001
45. Allison RR, Mota HC, Sibata CH. Clinical PD/PDT in north America: An historical review. Photodiagnosis Photodyn Ther (2004) 1(4):263–77. doi: 10.1016/s1572-1000(04)00084-5
46. Anders JJ, Lanzafame RJ, Arany PR. Low-level light/laser therapy versus photobiomodulation therapy. Photomed Laser Surg (2015) 33(4):183–4. doi: 10.1089/pho.2015.9848
47. Hamblin MR. Photobiomodulation, photomedicine, and laser surgery: A new leap forward into the light for the 21(st) century. Photomed Laser Surg (2018) 36(8):395–6. doi: 10.1089/pho.2018.29011.mrh
48. Hamblin MR. Mechanisms and applications of the anti-inflammatory effects of photobiomodulation. AIMS Biophys (2017) 4(3):337–61. doi: 10.3934/biophy.2017.3.337
49. Felsher DW. Cancer revoked: oncogenes as therapeutic targets. Nat Rev Cancer (2003) 3(5):375–80. doi: 10.1038/nrc1070
50. Yu W, Zhu J, Wang YT, Wang JJ, Fang WJ, Xia KS, et al. A review and outlook in the treatment of osteosarcoma and other deep tumors with photodynamic therapy: from basic to deep. Oncotarget (2017) 8(24):39833–48. doi: 10.18632/oncotarget.16243
51. Agostinis P, Berg K, Cengel KA, Foster TH, Girotti AW, Gollnick SO, et al. Photodynamic therapy of cancer: An update. CA Cancer J Clin (2011) 61(4):250–81. doi: 10.3322/caac.20114
52. Fotia C, Avnet S, Kusuzaki K, Roncuzzi L, Baldini N. Acridine orange is an effective anti-cancer drug that affects mitochondrial function in osteosarcoma cells. Curr Pharm Des (2015) 21(28):4088–94. doi: 10.2174/1381612821666150918144953
53. Yu W, Ye MZ, Zhu J, Wang YT, Liang CZ, Tang JB, et al. Zinc phthalocyanine encapsulated in polymer micelles as a potent photosensitizer for the photodynamic therapy of osteosarcoma. Nanomedicine-Nanotechnol Biol Med (2018) 14(4):1099–110. doi: 10.1016/j.nano.2018.02.005
54. Allison RR. Photodynamic therapy: oncologic horizons. Future Oncol (2014) 10(1):123–42. doi: 10.2217/fon.13.176
55. Hourigan AJ, Kells AF, Schwartz HS. IN-VITRO PHOTODYNAMIC THERAPY OF MUSCULOSKELETAL NEOPLASMS. J Orth Res (1993) 11(5):633–7. doi: 10.1002/jor.1100110504
56. Kusuzaki K, Murata H, Matsubara T, Miyazaki S, Okamura A, Seto M, et al. Clinical trial of photodynamic therapy using acridine orange with/without low dose radiation as new limb salvage modality in musculoskeletal sarcomas. Anticancer Res (2005) 25(2B):1225–35.
57. Guan J, Lai X, Wang X, Leung AW, Zhang H, Xu C. Photodynamic action of methylene blue in osteosarcoma cells in vitro. Photodiagnosis Photodyn Ther (2014) 11(1):13–9. doi: 10.1016/j.pdpdt.2013.09.003
58. Elfeky SA, Elsayed A, Moawad M, Ahmed WA. Hydroxyapatite nanocomposite as a potential agent in osteosarcoma PDT. Photodiagnosis Photodyn Ther (2020) 32:102056. doi: 10.1016/j.pdpdt.2020.102056
59. Coupienne I, Fettweis G, Piette J. RIP3 expression induces a death profile change in U2OS osteosarcoma cells after 5-ALA-PDT. Lasers Surg Med (2011) 43(7):557–64. doi: 10.1002/lsm.21088
60. Tu P, Huang Q, Ou Y, Du X, Li K, Tao Y, et al. Aloe-emodin-mediated photodynamic therapy induces autophagy and apoptosis in human osteosarcoma cell line MG63 through the ROS/JNK signaling pathway. Oncol Rep (2016) 35(6):3209–15. doi: 10.3892/or.2016.4703
61. Sun M, Zhou C, Zeng H, Puebla-Osorio N, Damiani E, Chen J, et al. Hiporfin-mediated photodynamic therapy in preclinical treatment of osteosarcoma. Photochem Photobiol (2015) 91(3):533–44. doi: 10.1111/php.12424
62. de Miguel GC, Abrantes AM, Laranjo M, Grizotto AYK, Camporeze B, Pereira JA, et al. A new therapeutic proposal for inoperable osteosarcoma: Photodynamic therapy. Photodiagnosis Photodyn Ther (2018) 21:79–85. doi: 10.1016/j.pdpdt.2017.11.009
63. Meier D, Botter SM, Campanile C, Robl B, Grafe S, Pellegrini G, et al. Foscan and foslip based photodynamic therapy in osteosarcoma in vitro and in intratibial mouse models. Int J Cancer (2017) 140(7):1680–92. doi: 10.1002/ijc.30572
64. Chen YY, Yin H, Tao Y, Zhong SH, Yu HY, Li JX, et al. Antitumor effects and mechanisms of pyropheophorbide−α methyl ester−mediated photodynamic therapy on the human osteosarcoma cell line MG−63. Int J Mol Med (2020) 45(4):971–82. doi: 10.3892/IJMM.2020.4494
65. Yu W, Wang Y, Zhu J, Jin L, Liu B, Xia K, et al. Autophagy inhibitor enhance ZnPc/BSA nanoparticle induced photodynamic therapy by suppressing PD-L1 expression in osteosarcoma immunotherapy. Biomaterials (2019) 192:128–39. doi: 10.1016/j.biomaterials.2018.11.019
66. Juzeniene A, Nielsen KP, Moan J. Biophysical aspects of photodynamic therapy. J Environ Pathol Toxicol Oncol (2006) 25(1-2):7–28. doi: 10.1615/JEnvironPatholToxicolOncol.v25.i1-2.20
67. White B, Rossi V, Baugher PJ. Aminolevulinic acid-mediated photodynamic therapy causes cell death in MG-63 human osteosarcoma cells. Photomed Laser Surg (2016) 34(9):400–5. doi: 10.1089/pho.2016.4091
68. Xu C, Wang M, Guo W, Sun W, Liu Y. Curcumin in osteosarcoma therapy: Combining with immunotherapy, chemotherapeutics, bone tissue engineering materials and potential synergism with photodynamic therapy. Front Oncol (2021) 11:672490. doi: 10.3389/fonc.2021.672490
69. Gao W, Wang Z, Lv L, Yin D, Chen D, Han Z, et al. Photodynamic therapy induced enhancement of tumor vasculature permeability using an upconversion nanoconstruct for improved intratumoral nanoparticle delivery in deep tissues. Theranostics (2016) 6(8):1131–44. doi: 10.7150/thno.15262
70. Wilson BC, Patterson MS. The physics, biophysics and technology of photodynamic therapy. Phys Med Biol (2008) 53(9):R61–109. doi: 10.1088/0031-9155/53/9/R01
71. Bolze F, Jenni S, Sour A, Heitz V. Molecular photosensitisers for two-photon photodynamic therapy. Chem Commun (Camb) (2017) 53(96):12857–77. doi: 10.1039/c7cc06133a
72. Dobos A, Steiger W, Theiner D, Gruber P, Lunzer M, Van Hoorick J, et al. Screening of two-photon activated photodynamic therapy sensitizers using a 3D osteosarcoma model. Analyst (2019) 144(9):3056–63. doi: 10.1039/c9an00068b
73. Doughty ACV, Hoover AR, Layton E, Murray CK, Howard EW, Chen WR. Nanomaterial applications in photothermal therapy for cancer. Mater (Basel) (2019) 12(5):779. doi: 10.3390/ma12050779
74. Chen WR, Adams RL, Heaton S, Dickey DT, Bartels KE, Nordquist RE. Chromophore-enhannced laser-tumor tissue photothermal interaction using an 808 nm diode laser. Cancer Lett (1995) 88(1):15–9. doi: 10.1016/0304-3835(94)03609-m
75. Hirsch LR, Stafford RJ, Bankson JA, Sershen SR, Rivera B, Price RE, et al. Nanoshell-mediated near-infrared thermal therapy of tumors under magnetic resonance guidance. P Natl Acad Sci USA (2003) 100(23):13549–54. doi: 10.1073/pnas.2232479100
76. Liu X, Li B, Fu F, Xu K, Zou R, Wang Q, et al. Facile synthesis of biocompatible cysteine-coated CuS nanoparticles with high photothermal conversion efficiency for cancer therapy. Dalton T (2014) 43(30):11709–15. doi: 10.1039/c4dt00424h
77. Jaque D, Martinez Maestro L, del Rosal B, Haro-Gonzalez P, Benayas A, Plaza JL, et al. Nanoparticles for photothermal therapies. Nanoscale (2014) 6(16):9494–530. doi: 10.1039/c4nr00708e
78. Liu Y, Zhang X, Liu Z, Wang L, Luo L, Wang M, et al. Gold nanoshell-based betulinic acid liposomes for synergistic chemo-photothermal therapy. Nanomed (2017) 13(6):1891–900. doi: 10.1016/j.nano.2017.03.012
79. Xiong S, Xiong G, Li Z, Jiang Q, Yin J, Yin T, et al. Gold nanoparticle-based nanoprobes with enhanced tumor targeting and photothermal/photodynamic response for therapy of osteosarcoma. Nanotechnology (2021) 32(15):155102. doi: 10.1088/1361-6528/abd816
80. Ma H, Jiang C, Zhai D, Luo Y, Chen Y, Lv F, et al. A bifunctional biomaterial with photothermal effect for Tumor therapy and bone regeneration. Adv Funct Mater (2016) 26(8):1197–208. doi: 10.1002/adfm.201504142
81. Lee J-H, Uyama H, Kwon O-K, Kim Y-J. Nitric oxide and reactive oxygen species-releasing polylactic acid monolith for enhanced photothermal therapy of osteosarcoma. J Ind Eng Chem (2021) 94:498–506. doi: 10.1016/j.jiec.2020.11.026
82. Dang W, Jin Y, Yi K, Ju E, Zhuo C, Wei H, et al. Hemin particles-functionalized 3D printed scaffolds for combined photothermal and chemotherapy of osteosarcoma. Chem Eng J (2021) 422:129919. doi: 10.1016/j.cej.2021.129919
83. Yin X, Ran S, Cheng H, Zhang M, Sun W, Wan Y, et al. Polydopamine-modified ZIF-8 nanoparticles as a drug carrier for combined chemo-photothermal osteosarcoma therapy. Colloids Surf B Biointerfaces (2022) 216:112507. doi: 10.1016/j.colsurfb.2022.112507
84. Dong S, Zhang YN, Wan J, Cui R, Yu X, Zhao G, et al. A novel multifunctional carbon aerogel-coated platform for osteosarcoma therapy and enhanced bone regeneration. J Mater Chem B (2020) 8(3):368–79. doi: 10.1039/c9tb02383f
85. Yang Q, Yin H, Xu T, Zhu D, Yin J, Chen Y, et al. Engineering 2D mesoporous Silica@MXene-integrated 3D-printing scaffolds for combinatory osteosarcoma therapy and NO-augmented bone regeneration. Small (2020) 16(14):e1906814. doi: 10.1002/smll.201906814
86. Mester A, Mester A. The history of photobiomodulation: Endre mester (1903-1984). Photomed Laser Surg (2017) 35(8):393–4. doi: 10.1089/pho.2017.4332
87. Gupta A, Keshri GK, Yadav A, Gola S, Chauhan S, Salhan AK, et al. Superpulsed (Ga-as, 904 nm) low-level laser therapy (LLLT) attenuates inflammatory response and enhances healing of burn wounds. J Biophotonics (2015) 8(6):489–501. doi: 10.1002/jbio.201400058
88. Martins DF, Turnes BL, Cidral-Filho FJ, Bobinski F, Rosas RF, Danielski LG, et al. Light-emitting diode therapy reduces persistent inflammatory pain: Role of interleukin 10 and antioxidant enzymes. Neuroscience (2016) 324:485–95. doi: 10.1016/j.neuroscience.2016.03.035
89. Thunshelle C, Hamblin MR. Transcranial low-level laser (Light) therapy for brain injury. Photomed Laser Surg (2016) 34(12):587–98. doi: 10.1089/pho.2015.4051
90. OMKYKOW H. Blue light inhibits the growth of B16 melanoma cells. Japanese J Cancer Res Gann (2002) 93(5):551–8. doi: 10.1111/j.1349-7006.2002.tb01290.x
91. Shakibaie M, Vaezjalali M, Rafii-Tabar H, Sasanpour P. Phototherapy alters the oncogenic metabolic activity of breast cancer cells. Photodiagnosis Photodyn Ther (2020) 30:101695. doi: 10.1016/j.pdpdt.2020.101695
92. Kara C, Selamet H, Gokmenoglu C, Kara N. Low level laser therapy induces increased viability and proliferation in isolated cancer cells. Cell Prolif (2018) 51(2):e12417. doi: 10.1111/cpr.12417
93. Zhang J, Xing D, Gao X. Low-power laser irradiation activates src tyrosine kinase through reactive oxygen species-mediated signaling pathway. J Cell Physiol (2008) 217(2):518–28. doi: 10.1002/jcp.21529
94. Tam SY, Tam VCW, Ramkumar S, Khaw ML, Law HKW, Lee SWY. Review on the cellular mechanisms of low-level laser therapy use in oncology. Front Oncol (2020) 10:1255. doi: 10.3389/fonc.2020.01255
95. Feng C, Gong R, Zheng Q, Yan G, He M, Lei H, et al. Synergistic anti-tumor effects of arsenic trioxide and blue LED irradiation on human osteosarcoma. Int J Biol Sci (2019) 15(2):386–94. doi: 10.7150/ijbs.28356
96. Coombe AR, Ho CT, Darendeliler MA, Hunter N, Philips JR, Chapple CC, et al. The effects of low level laser irradiation on osteoblastic cells. Clin Orthod Res (2001) 4(1):3–14. doi: 10.1034/j.1600-0544.2001.040102.x
97. Renno ACM, McDonnell PA, Parizotto NA, Laakso EL. The effects of laser irradiation on osteoblast and osteosarcoma cell proliferation and differentiation in vitro. Photomed Laser Surg (2007) 25(4):275–80. doi: 10.1089/pho.2007.2055
98. He M, Yan G, Wang Y, Gong R, Lei H, Yu S, et al. Blue LED causes autophagic cell death in human osteosarcoma by increasing ROS generation and dephosphorylating EGFR. J Cell Mol Med (2021) 25(11):4962–73. doi: 10.1111/jcmm.16412
99. Barati Shoorche A, Mohammadkarim A, Jadidi M, Bahraminasab M. Photobiomodulation therapy affects the elastic modulus, cytoskeletal rearrangement and migration capability of human osteosarcoma cells. Lasers Med Sci (2022) 37:2855–63. doi: 10.1007/s10103-022-03554-8
100. Ates GB, Ak A, Garipcan B, Yuksel S, Gulsoy M (2015). Controversial effects of low level laser irradiation on the proliferation of human osteoblasts, in: Conference on Mechanisms for Low-Light Therapy X, San Francisco, CA. 9309:930907 doi: 10.1117/12.2076345
101. Heiskanen V, Hamblin MR. Photobiomodulation: lasersvs.light emitting diodes? Photochem Photobiol Sci (2018) 17(8):1003–17. doi: 10.1039/c8pp00176f
102. Sutherland JC. Biological effects of polychromatic light¶. Photochem Photobiol (2002) 76(2):167–70. doi: 10.1562/0031-8655(2002)076<0164:Beopl>2.0.Co;2
103. Garza ZCF, Born M, Hilbers PAJ, van Riel NAW, Liebmann J. Visible blue light therapy: Molecular mechanisms and therapeutic opportunities. Curr Med Chem (2018) 25(40):5564–77. doi: 10.2174/0929867324666170727112206
104. Cashmore AR, Jarillo JA, Wu YJ, Liu DM. Cryptochromes: Blue light receptors for plants and animals. Science (1999) 284(5415):760–5. doi: 10.1126/science.284.5415.760
105. Losi A, Gardner KH, Moglich A. Blue-light receptors for optogenetics. Chem Rev (2018) 118(21):10659–709. doi: 10.1021/acs.chemrev.8b00163
106. Yang MY, Chang CJ, Chen LY. Blue light induced reactive oxygen species from flavin mononucleotide and flavin adenine dinucleotide on lethality of HeLa cells. J Photochem Photobiol B-Biol (2017) 173:325–32. doi: 10.1016/j.jphotobiol.2017.06.014
107. Cardoso DR, Scurachio RS, Santos WG, Homem-de-Mello P, Skibsted LH. Riboflavin-photosensitized oxidation is enhanced by conjugation in unsaturated lipids. J Agr Food Chem (2013) 61(9):2268–75. doi: 10.1021/jf305280x
108. Massey V. The chemical and biological versatility of riboflavin. Biochem Soc Trans (2000) 28:283–96. doi: 10.1042/0300-5127:0280283
109. Mueller-Enoch D. Blue light mediated photoreduction of the flavoprotein NADPH-cytochrome P450 reductase: A Forster-type energy transfer. Z Naturforsch Sect C J Biosci (1997) 52(9-10):605–14. doi: 10.1515/znc-1997-9-1007
110. Lewis JB, Wataha JC, Messer RL, Caughman GB, Yamamoto T, Hsu SD. Blue light differentially alters cellular redox properties. J BioMed Mater Res B Appl Biomater (2005) 72(2):223–9. doi: 10.1002/jbm.b.30126
111. Oplander C, Deck A, Volkmar CM, Kirsch M, Liebmann J, Born M, et al. Mechanism and biological relevance of blue-light (420-453 nm)-induced onenzymatic nitric oxide generation from photolabile nitric oxide derivates in human skin in vitro and in vivo. Free Radic Biol Med (2013) 65:1363–77. doi: 10.1016/j.freeradbiomed.2013.09.022
112. Vladimirov Y, Borisenko G, Boriskina N, Kazarinov K, Osipov A. NO-hemoglobin may be a light-sensitive source of nitric oxide both in solution and in red blood cells. J Photochem Photobiol B-Biol (2000) 59(1-3):115–22. doi: 10.1016/s1011-1344(00)00148-2
113. Haltaufderhyde K, Ozdeslik RN, Wicks NL, Najera JA, Oancea E. Opsin expression in human epidermal skin. Photochem Photobiol (2015) 91(1):117–23. doi: 10.1111/php.12354
114. Serrage H, Heiskanen V, Palin WM, Cooper PR, Milward MR, Hadis M, et al. Under the spotlight: Mechanisms of photobiomodulation concentrating on blue and green light. Photochem Photobiol Sci (2019) 18(8):1877–909. doi: 10.1039/c9pp00089e
115. Yoo JA, Yu E, Park SH, Oh SW, Kwon K, Park SJ, et al. Blue light irradiation induces human keratinocyte cell damage via transient receptor potential vanilloid 1 (TRPV1) regulation. Oxid Med Cell Longev (2020) 2020:8871745. doi: 10.1155/2020/8871745
116. Karu T. Primary and secondary mechanisms of action of visible to near-IR radiation on cells. J Photochem Photobiol B-Biol (1999) 49(1):1–17. doi: 10.1016/s1011-1344(98)00219-x
117. Dungel P, Mittermayr R, Haindl S, Osipov A, Wagner C, Redl H, et al. Illumination with blue light reactivates respiratory activity of mitochondria inhibited by nitric oxide, but not by glycerol trinitrate. Arch Biochem Biophys (2008) 471(2):109–15. doi: 10.1016/j.abb.2008.01.009
118. Veerman ECI, Vanleeuwen JW, Vanbuuren KJH, Vangelder BF. THE REACTION OF CYTOCHROME-AA3 WITH (PORPHYRIN) CYTOCHROME-c AS STUDIED BY PULSE-RADIOLYSIS. Biochim Biophys Acta (1982) 680(2):134–41. doi: 10.1016/0005-2728(82)90004-4
119. Shen Y, Xie C, Gu Y, Li X, Tong J. Illumination from light-emitting diodes (LEDs) disrupts pathological cytokines expression and activates relevant signal pathways in primary human retinal pigment epithelial cells. Exp Eye Res (2016) 145:456–67. doi: 10.1016/j.exer.2015.09.016
120. Knels L, Valtink M, Roehlecke C, Lupp A, de la Vega J, Mehner M, et al. Blue light stress in retinal neuronal (R28) cells is dependent on wavelength range and irradiance. Eur J Neurosci (2011) 34(4):548–58. doi: 10.1111/j.1460-9568.2011.07790.x
121. Becker A, Klapczynski A, Kuch N, Arpino F, Simon-Keller K, de la Torre C, et al. Gene expression profiling reveals aryl hydrocarbon receptor as a possible target for photobiomodulation when using blue light. Sci Rep (2016) 6:33847. doi: 10.1038/srep33847
122. Zhang C, Creech KL, Zuercher WJ, Willson TM. Gram-scale synthesis of FICZ, a photoreactive endogenous ligand of the aryl hydrocarbon receptor. Sci Rep (2019) 9(1):9982. doi: 10.1038/s41598-019-46374-7
123. Mreihil K, Mcdonagh A, Nakstad B, Hansen T. Early isomerization of bilirubin in phototherapy of neonatal jaundice. Pediatr Res (2010) 67(6):656–9. doi: 10.1203/PDR.0b013e3181dcedc0
124. Yu BX, Hu FQ. Exploration of the pathogenic factors of neonatal jaundice and the clinical effect of blue phototherapy. Am J Transl Res (2021) 13(6):6802–6.
125. Mreihil K, Nakstad B, Stensvold HJ, Benth JS, Hansen TWR, Norwegian NPSG, et al. Uniform national guidelines do not prevent wide variations in the clinical application of phototherapy for neonatal jaundice. Acta Paediatr (2018) 107(4):620–7. doi: 10.1111/apa.14142
126. Smilowska K, van Wamelen DJ, Schoutens AMC, Meinders MJ, Bloem BR. Blue light therapy glasses in parkinson's disease: Patients' experience. Parkinsons Dis (2019) 2019:1906271. doi: 10.1155/2019/1906271
127. Wever RA, Polasek J, Wildgruber CM. Bright light affects human circadian rhythms. Pflugers Archiv Eur J Physiol (1983) 396:85–7. doi: 10.1007/BF00584704
128. Glickman G, Byrne B, Pineda C, Hauck WW, Brainard GC. Light therapy for seasonal affective disorder with blue narrow-band light-emitting diodes (LEDs). Biol Psychiatry (2006) 59(6):502–7. doi: 10.1016/j.biopsych.2005.07.006
129. Jalili A. Chromophore gel-assisted phototherapy: Anovel and promising photobiomodulation therapy for facial inflammatory skin diseases and skin aging. J Fur Asthetische Chirurgie (2019) 12:S1–5. doi: 10.1007/s12631-018-0121-z
130. Papageorgiou P, Katsambas A, Chu A. Phototherapy with blue (415 nm) and red (660 nm) light in the treatment of acne vulgaris. Brit J Dermatol (2000) 142(5):973–8. doi: 10.1046/j.1365-2133.2000.03481.x
131. Becker D, Langer E, Seemann M, Seemann G, Fell I, Saloga J, et al. Clinical efficacy of blue light full body irradiation as treatment option for severe atopic dermatitis. PloS One (2011) 6(6):e20566. doi: 10.1371/journal.pone.0020566
132. Carvalho-Costa TM, Mendes MT, da Silva MV, Rodrigues V, Thedei G, Oliveira CJF, et al. Light-emitting diode at 460 +/- 20 nm increases the production of IL-12 and IL-6 in murine dendritic cells. Photomed Laser Surg (2017) 35(10):560–6. doi: 10.1089/pho.2016.4244
133. Rossi F, Magni G, Tatini F, Banchelli M, Cherchi F, Rossi M, et al. Photobiomodulation of human fibroblasts and keratinocytes with blue light: Implications in wound healing. Biomedicines (2021) 9(1):1–14. doi: 10.3390/biomedicines9010041
134. Tatini F, Magni G, de Siena G, Pini R, Bacci S, Gasperini S, et al. Blue light induced modulation in the early phase of wound healing, in: Conference on Medical Laser Applications and Laser-Tissue Interactions IX, Munich, GERMANY (2019) 11079:110790S. doi: 10.1117/12.2527017.
135. Masson-Meyers DS, Bumah VV, Enwemeka CS. Blue light does not impair wound healing in vitro. J Photochem Photobiol B-Biol (2016) 160:53–60. doi: 10.1016/j.jphotobiol.2016.04.007
136. Sadowska M, Narbutt J, Lesiak A. Blue light in dermatology. Life (Basel) (2021) 11(7):1–13. doi: 10.3390/life11070670
137. Masson-Meyers DS, Bumah VV, Castel C, Castel D, Enwemeka CS. Pulsed 450 nm blue light significantly inactivates propionibacterium acnes more than continuous wave blue light. J Photochem Photobiol B-Biol (2020) 202:111719. doi: 10.1016/j.jphotobiol.2019.111719
138. Dungel P, Mittermayr R, Haindl S, Osipov A, Wagner C, Redl H, et al. Illumination with blue light reactivates respiratory activity of mitochondria inhibited by nitric oxide, but not by glycerol trinitrate. Arch Biochem Biophys (2008) 471(2):109–15. doi: 10.1016/j.abb.2008.01.009
139. Passarella S, Karu T. Absorption of monochromatic and narrow band radiation in the visible and near IR by both mitochondrial and non-mitochondrial photoacceptors results in photobiomodulation. J Photochem Photobiol B (2014) 140:344–58. doi: 10.1016/j.jphotobiol.2014.07.021
140. Kim HJ, Choi MS, Bae IH, Jung JY, Son ED, Lee TR, et al. Short wavelength visible light suppresses innate immunity-related responses by modulating protein s-nitrosylation in keratinocytes. J Invest Dermatol (2016) 136(3):727–31. doi: 10.1016/j.jid.2015.12.004
141. Taflinski L, Demir E, Kauczok J, Fuchs PC, Born M, Suschek CV, et al. Blue light inhibits transforming growth factor-beta1-induced myofibroblast differentiation of human dermal fibroblasts. Exp Dermatol (2014) 23(4):240–6. doi: 10.1111/exd.12353
142. Yuan Y, Yan G, Gong R, Zhang L, Liu T, Feng C, et al. Effects of blue light emitting diode irradiation on the proliferation, apoptosis and differentiation of bone marrow-derived mesenchymal stem cells. Cell Physiol Biochem (2017) 43(1):237–46. doi: 10.1159/000480344
143. Liebmann J, Born M, Kolb-Bachofen V. Blue-light irradiation regulates proliferation and differentiation in human skin cells. J Invest Dermatol (2010) 130(1):259–69. doi: 10.1038/jid.2009.194
144. Yoshimoto T, Morine Y, Takasu C, Feng R, Ikemoto T, Yoshikawa K, et al. Blue light-emitting diodes induce autophagy in colon cancer cells by opsin 3. Ann Gastroenterol Surg (2018) 2(2):154–61. doi: 10.1002/ags3.12055
145. Matsumoto N, Yoshikawa K, Shimada M, Kurita N, Sato H, Iwata T, et al. Effect of light irradiation by light emitting diode on colon cancer cells. Anticancer Res (2014) 34(9):4709–16. doi: 10.1245/s10434-014-3557-1
146. Wu X, Huang H, Yu B, Zhang J. A blue light-inducible CRISPR-Cas9 system for inhibiting progression of melanoma cells. Front Mol Biosci (2020) 7:606593. doi: 10.3389/fmolb.2020.606593
147. Chen Z, Qin H, Lin S, Lu Z, Fan X, Liu X, et al. Comparative transcriptome analysis of gene expression patterns on B16F10 melanoma cells under photobiomodulation of different light modes. J Photochem Photobiol B (2021) 216:112127. doi: 10.1016/j.jphotobiol.2021.112127
148. Tartaglione M, Zabaleta M, Lazzarini R, Piva F, Busilacchi E, Poloni A, et al. Apoptotic mechanism activated by blue light and cisplatinum in cutaneous squamous cell carcinoma cells. Int J Mol Med (2021) 47(4):48. doi: 10.3892/ijmm.2021.4881
149. Patel A, Rotenberg S, Messer R, Wataha J, Ogbureke K, McCloud V, et al. Blue light activates phase 2 response proteins and slows growth of A431 epidermoid carcinoma xenografts. Anticancer Res (2014) 34(11):6305–13.
150. Zhuang J, Xia L, Zou Z, Yin J. Blue light induces ROS mediated apoptosis and degradation of AML1-ETO oncoprotein in kasumi-1 cells. Med Oncol (2022) 39(5):52. doi: 10.1007/s12032-022-01650-x
151. Shakibaie M, Vaezjalali M, Rafii-Tabar H, Sasanpour P. Synergistic effect of phototherapy and chemotherapy on bladder cancer cells. J Photochem Photobiol B (2019) 193:148–54. doi: 10.1016/j.jphotobiol.2019.02.004
152. Yan G, Zhang L, Feng C, Gong R, Idiiatullina E, Huang Q, et al. Blue light emitting diodes irradiation causes cell death in colorectal cancer by inducing ROS production and DNA damage. Int J Biochem Cell Biol (2018) 103:81–8. doi: 10.1016/j.biocel.2018.08.006
153. Li C, Zhu G, Cui Z, Zhang J, Zhang S, Wei Y. The strong inhibitory effect of combining anti-cancer drugs AT406 and rocaglamide with blue LED irradiation on colorectal cancer cells. Photodiagnosis Photodyn Ther (2020) 30:101797. doi: 10.1016/j.pdpdt.2020.101797
154. Notomi T, Kuno M, Hiyama A, Ezura Y, Honma M, Ishizuka T, et al. Membrane depolarization regulates intracellular RANKL transport in non-excitable osteoblasts. Bone (2015) 81:306–14. doi: 10.1016/j.bone.2015.07.031
155. Filipski E, King VM, Li XM, Granda TG, Mormont MC, Liu XH, et al. Host circadian clock as a control point in tumor progression. J Natl Cancer Inst (2002) 94(9):690–7. doi: 10.1093/jnci/94.9.690
156. Lesicka M, Jablonska E, Wieczorek E, Seroczynska B, Siekierzycka A, Skokowski J, et al. Altered circadian genes expression in breast cancer tissue according to the clinical characteristics. PloS One (2018) 13(6):e0199622. doi: 10.1371/journal.pone.0199622
157. Huisman SA, Oklejewicz M, Ahmadi AR, Tamanini F, Ijzermans JNM, van der Horst GTJ, et al. Colorectal liver metastases with a disrupted circadian rhythm phase shift the peripheral clock in liver and kidney. Int J Cancer (2015) 136(5):1024–32. doi: 10.1002/ijc.29089
Keywords: osteosarcoma, phototherapy, photobiomodulation therapy, cellular mechanism, blue light
Citation: Yang J, Fu Q, Jiang H, Li Y and Liu M (2022) Progress of phototherapy for osteosarcoma and application prospect of blue light photobiomodulation therapy. Front. Oncol. 12:1022973. doi: 10.3389/fonc.2022.1022973
Received: 19 August 2022; Accepted: 20 September 2022;
Published: 13 October 2022.
Edited by:
Bertrand Liagre, Université de Limoges, FranceReviewed by:
Mohammad H. Ghazimoradi, Tarbiat Modares University, IranEnrico Lucarelli, Rizzoli Orthopedic Institute (IRCCS), Italy
Copyright © 2022 Yang, Fu, Jiang, Li and Liu. This is an open-access article distributed under the terms of the Creative Commons Attribution License (CC BY). The use, distribution or reproduction in other forums is permitted, provided the original author(s) and the copyright owner(s) are credited and that the original publication in this journal is cited, in accordance with accepted academic practice. No use, distribution or reproduction is permitted which does not comply with these terms.
*Correspondence: Yinghua Li, emh1bGloYW5AZnVkYW4uZWR1LmNu; Muqing Liu, bXFsaXVAZnVkYW4uZWR1LmNu