- 1Department of Lifelong Sport, School of Sports Education, Hokusho University, Ebetsu, Japan
- 2Department of Molecular Biology, Hokkaido University Graduate School of Medicine, Sapporo, Japan
- 3Department of Cardiovascular Medicine, Faculty of Medical Sciences, Kyushu University, Fukuoka, Japan
- 4Division of Cardiovascular Medicine, Research Institute of Angiocardiology, Faculty of Medical Sciences, Kyushu University, Fukuoka, Japan
- 5Institute of Health Science Innovation for Medical Care, Hokkaido University Hospital, Sapporo, Japan
- 6Institute for Genetic Medicine, Hokkaido University, Sapporo, Japan
Accumulating clinical data have demonstrated a clear positive association between cancer and cardiac disorders, particularly chronic heart failure (CHF). These two diseases can be mutual drivers of each other, and hence frequently co-occur in patients. The immune system is the core mechanism that eliminates transformed cells from our bodies. However, immune cells often play distinct or even conflicting roles in cancer and CHF. Moreover, CHF alters the properties of immune cells, particularly those of regulatory T cells. Our previous study showed that the oxidative phosphorylation capacity of peripheral blood mononuclear cells is impaired in CHF, leading to the increased production of reactive oxygen species. Therefore, the co-occurrence of cancer and CHF becomes a serious problem, affecting the treatment of both diseases, and consequently negatively affecting patient survival rates. To date, few methods have been identified that effectively treat both diseases at the same time. Mitochondria activity may change in immune cells during their activation and exhaustion, and in CHF. Mitochondria activity is also largely affected in myocardia in CHF. We here focus on the mitochondrial abnormalities of immune cells in cancer and CHF, and discuss possible ways to treat cancer and CHF at the same time by targeting mitochondrial abnormalities. Many cancer cells are inevitably produced daily in our bodies, mostly owing to enzymatic nucleotide errors of DNA replication and repair. Therefore, the possibility of ways to prevent cancer by preventing the onset of heart failure will also be discussed.
Introduction
Cancer and cardiac disorders, including chronic heart failure (CHF), represent two major causes of morbidity and mortality in developed countries (1, 2). Epidemiological studies have shown that the risk of developing cancer in patients with CHF is approx. four times greater than in those without CHF (3–6). Conversely, cancer patients can be at increased risk of cardiac disease due to deterioration of their lifestyle behaviors (e.g., inactivity and an unbalanced diet) (7), and also due to treatment toxicity, as many anticancer drugs are known to cause cardiotoxic side effects (8–11). Therefore, cancer and cardiovascular diseases can be mutual disease drivers, and hence co-occur frequently in patients (Figure 1). Moreover, immune cells, particularly regulatory T (Treg) cells, play distinct or even conflicting roles in cancer and CHF (12, 13). Hence, the co-occurrence of cancer and cardiovascular disease is a serious problem, affecting the treatment of both diseases, and consequently negatively affecting survival rates (14, 15). To date, however, treatments exist only for each disease. Mitochondria are central to ATP production by oxidative phosphorylation (OXPHOS) and to metabolism. To address above problems, we here focus on the mitochondrial abnormalities of immune cells during CHF and cancer, and discuss possible methods to treat cancer and CHF at the same time by targeting these mitochondrial abnormalities; and, moreover, discuss possible ways to prevent cancer by preventing the onset of CHF.
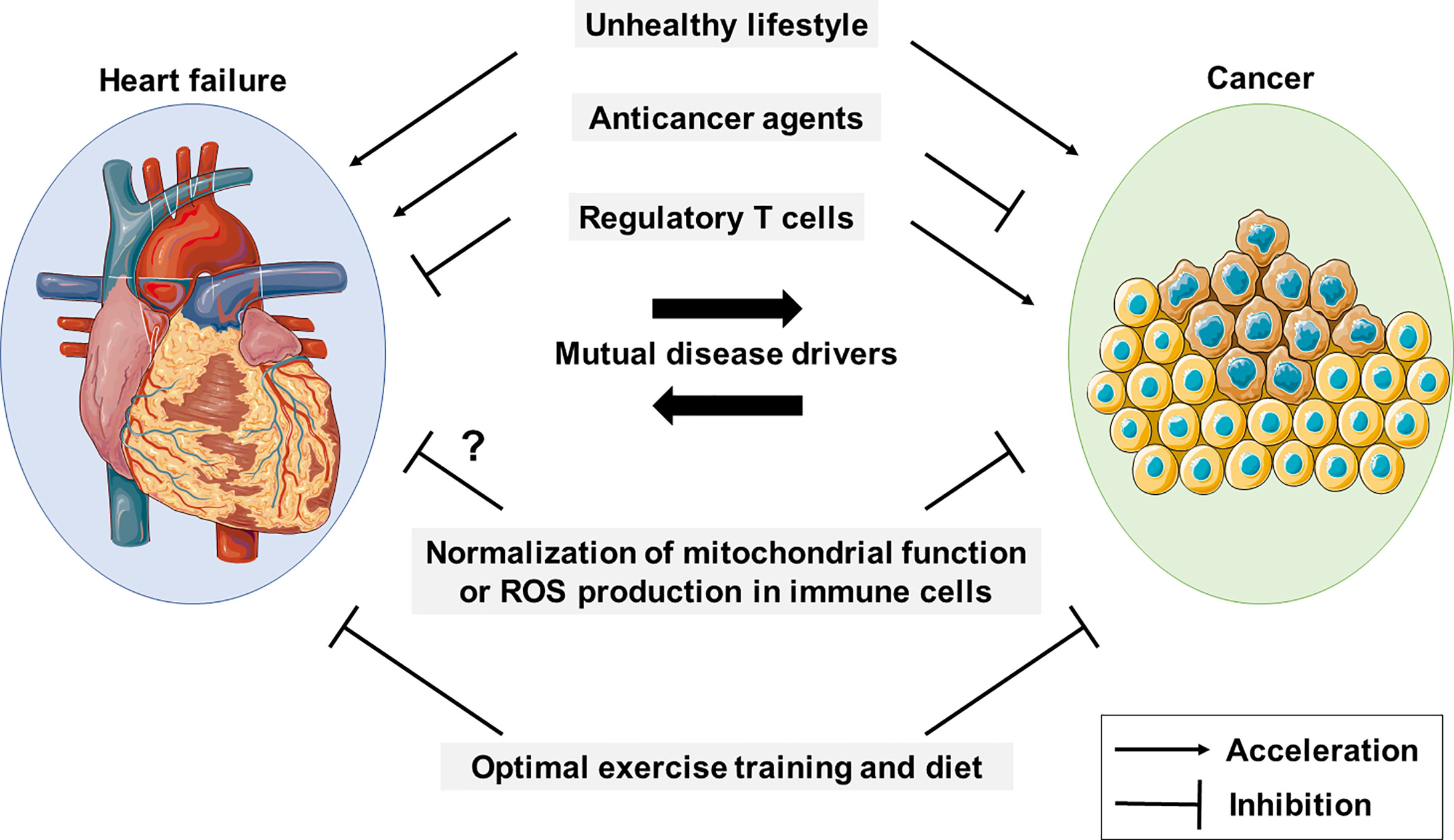
Figure 1 Cancer and HF mutually promote each other. An unhealthy lifestyle contributes to the development of cancer and CHF, and they are mutual disease drivers. Anticancer drugs and regulatory T cells appear to have conflicting roles in cancer and HF. Mitochondrial function and reactive oxygen species (ROS) production in immune cells are potential therapeutic targets in both diseases.
Immune system mediates the crosstalk between cancer and CHF
T-cell dysfunction, particularly of tumor-infiltrating lymphocytes (TILs), is highly detrimental to antitumor immunity and immunotherapy (16). Recently, Koelwyn et al. reported that the adjusted relative risk of death from breast cancer is increased by approx. 60% in the presence of a cardiovascular event (17). They also demonstrated by using mouse models that myocardial infarction (MI), which leads to HF, accelerates breast cancer development (17). Molecularly, it was shown that MI epigenetically reprogrammed Ly6Chi monocytes, which are macrophage precursors in the bone marrow reservoir, to an immunosuppressive state, and increased their circulation and infiltration into tumors, whereas their depletion abrogated tumor growth (17). Moreover, tumors of MI mice had fewer T lymphocytes than control mice, in which Treg cells are predominant. These changes that occur in MI mice may be beneficial to the heart, but they all promote tumor growth and survival (17). Therefore, certain populations of immune cells clearly play a central role in cross-disease communication between cancer and CHF.
CHF affects mitochondrial OXPHOS of immune cells
Mitochondrial OXPHOS plays a central role in lymphocyte activity (18). Mitochondria are also fundamental to the development and fate determination of peripheral lymphocytes (19, 20). Suppressed glycolysis and OXPHOS were shown to be early drivers of CD8+ T-cell exhaustion (21). Moreover, TILs are constantly exposed to tumor antigens, and may also experience metabolic stress, which is thought to occur frequently in the tumor microenvironment. A recent report demonstrated that continuous antigen stimulation together with hypoxia impairs the mitochondrial functions of T cells, and hence promotes terminal T-cell exhaustion (22). Molecularly, it was shown that continuous antigen stimulation upregulates B lymphocyte–induced maturation protein 1, and represses peroxisome proliferator-activated receptor gamma coactivator-1 (PGC-1), resulting in the suppression of mitochondrial biogenesis and T-cell functions (23).
Our group has found that mitochondrial respiratory capacity of peripheral blood mononuclear cells (PBMCs), which are predominantly lymphocytes, declines with the progression of CHF, with class III (i.e., moderate to severe CHF) patients by New York Heart Association (NYHA) criteria having 10-24% lower mitochondrial respiratory capacity than NYHA class I/II (i.e., mild CHF) patients, in which mitochondrial ROS production of PBMCs was increased by 13-24% in patients with NYHA class III compared to those with NYHA class I/II (24). Such changes were observed even in the early stages of HF, and were closely associated with the severity of CHF. We moreover found that the capacity of complex II, but not complex I, of the mitochondrial OXPHOS of PBMCs was specifically decreased in CHF (24). It has been reported in monkeys that there is a close association among the mitochondrial OXPHOS activities of circulating monocytes, cardiac cells, and skeletal muscle cells (25).Therefore, ROS levels in PBMCs can be a marker indicating the onset and the severity of HF. As PBMCs mostly consist of unprimed lymphocytes, it awaits to be clarified whether activated lymphocytes are also affected in CHF patients.
Activating mitochondria of immune cells improves tumor immune therapies
Mitochondrial function of CD8+ T cells in lung cancer patients can be a marker for determining the efficacy of anti-PD-1 immune checkpoint inhibition therapy (26). Scharping et al. have shown that restoration of mitochondrial activity and T-cell function by reversing the loss of PGC-1α in tumor-specific T cells resulted in increased antitumor immune responses (23). Yu et al. demonstrated that administering nicotinamide riboside (NR), a precursor of nicotinamide adenine dinucleotide, may be able to restore mitochondrial activity, prevent T-cell exhaustion, and sustain the antitumor responses of T cells in tumor-bearing mice (27). NR supplementation was moreover found to facilitate antitumor immune activity, when used in conjunction with the anti-PD-1 antibody (27). Vardhana et al. demonstrated that N-acetylcysteine (NAC), which is known to increase glutathione synthesis and neutralize ROS, reverses the metabolic defects of exhausted T cells, and promotes their antitumor immune activity, to act synergistically with anti-PD-L1 immunotherapy in lymphoma and melanoma (28). Therefore, activating immune cell mitochondria may improve the efficacy of immune checkpoint inhibition-based tumor immunotherapy. However, it should be noted that the administration of molecules such as NR or NAC may also activate cancer cells to more malignant states, and it is hence unclear whether they will be effective in the treatment of patients. It is also well documented that the reinvigoration of T cells, once they are deeply exhausted, might be very difficult (29). Another way to improve the efficacy of cancer immunotherapy would be to enhance the new production of T cells, and diversify the T-cell receptor repertoire, as has been demonstrated with radiation (30), but this might also be difficult in patients with CHF because of their poor health condition.
Future perspectives
When cancer and CHF coexist, the treatment of either disease alone is inadequate. Normalization of mitochondrial activity and the function of immune cells, which are frequently impaired in CHF, is a rational strategy to improve cancer therapeutics. For example, identification of a molecular basis for the downregulation of mitochondrial respiratory capacity in the PBMCs of CHF patients, which we have shown previously (24), and if such a mechanism occurs specifically in PBMCs but not in tumor cells, improving mitochondrial respiratory capacity in PBMCs may be promising for the treatment of cancer in patients who also have CHF. Such a strategy targeting immune cells’ mitochondria may also enhance tumor growth suppression in cancer treatment by immune checkpoint inhibitors, although cardiac assessment with a careful follow-up is necessary because immune checkpoint inhibitors are known to have a cardiotoxicity with low incidence rate (<1%) with single use of them (31). Furthermore, activation of immune cells is beneficial for chemotherapy (32), and thus, mitochondria-targeted treatment strategy may help chemotherapy improve outcomes of cancer patients with or without CHF, although robust clinical evidence is still lacking.
Lifestyle habits, such as a proper diet and daily exercise are important preventive measures of cancer and CHF. Regarding the molecular bases, skeletal muscles secrete various myokines, which have positive effects on mitochondria in different organs and tissues, and may also promote immunity (33–35). Proper exercise by patients can also suppress tumor growth and promote anti-tumor immunity, and may improve the therapeutic effects of immune-checkpoint inhibitors, whereas the types of myokines and immune cells therein involved have been shown to differ depending on the types of cancer (36–38). On the other hand, muscle dysfunction occurs not only in CHF (35, 39), but is also a widespread phenomenon of cancer patients regardless of cancer type or stage (40). Therefore, the identification of the singular point (41) before which exercise can be effective in the treatment of cancer and CHF, along with identification of effective exercise regimens and the related drugs, will be the major challenge of medicine in the future.
Author contributions
ST, SK, HH, TY, and HS wrote the manuscript. All authors contributed to the article and approved the submitted version.
Funding
This work was supported in part by Grants-in-Aid for Scientific Research (grant no. JP17H04758 to ST, and 21H03360 to SK, and 21H02675 to HS), and Grants-in-Aid for Challenging Exploratory Research (grant no. 19K22791 to ST) from the Japan Society for the Promotion of Science, grants from the Akiyama Life Science Foundation (to ST), the Suhara Memorial Foundation (to ST), and the Japan Foundation for Applied Enzymology (to ST).
Acknowledgments
The authors thank H.A. Popiel for her critical reading of the manuscript.
Conflict of interest
The authors declare that the research was conducted in the absence of any commercial or financial relationships that could be construed as a potential conflict of interest.
Publisher’s note
All claims expressed in this article are solely those of the authors and do not necessarily represent those of their affiliated organizations, or those of the publisher, the editors and the reviewers. Any product that may be evaluated in this article, or claim that may be made by its manufacturer, is not guaranteed or endorsed by the publisher.
References
1. Christ M, Stork S, Dorr M, Heppner HJ, Muller C, Wachter R, et al. Heart failure epidemiology 2000-2013: Insights from the German federal health monitoring system. Eur J Heart Fail (2016) 18:1009–18. doi: 10.1002/ejhf.567
2. Siegel RL, Miller KD, Jemal A. Cancer statistic. CA Cancer J Clin (2016) 66:7–30. doi: 10.3322/caac.21332
3. Hasin T, Gerber Y, Mcnallan SM, Weston SA, Kushwaha SS, Nelson TJ, et al. Patients with heart failure have an increased risk of incident cancer. J Am Coll Cardiol (2013) 62:881–6. doi: 10.1016/j.jacc.2013.04.088
4. Banke A, Schou M, Videbaek L, Moller JE, Torp-Pedersen C, Gustafsson F, et al. Incidence of cancer in patients with chronic heart failure: a long-term follow-up study. Eur J Heart Fail (2016) 18:260–6. doi: 10.1002/ejhf.472
5. Hasin T, Gerber Y, Weston SA, Jiang R, Killian JM, Manemann SM, et al. Heart failure after myocardial infarction is associated with increased risk of cancer. J Am Coll Cardiol (2016) 68:265–71. doi: 10.1016/j.jacc.2016.04.053
6. Sakamoto M, Hasegawa T, Asakura M, Kanzaki H, Takahama H, Amaki M, et al. Does the pathophysiology of heart failure prime the incidence of cancer? Hypertens Res (2017) 40:831–6. doi: 10.1038/hr.2017.45
7. Hooning MJ, Botma A, Aleman BM, Baaijens MH, Bartelink H, Klijn JG, et al. Long-term risk of cardiovascular disease in 10-year survivors of breast cancer. J Natl Cancer Inst. (2007) 99:365–75. doi: 10.1093/jnci/djk064
8. Yeh ET, Bickford CL. Cardiovascular complications of cancer therapy: incidence, pathogenesis, diagnosis, and management. J Am Coll Cardiol (2009) 53:2231–47. doi: 10.1016/j.jacc.2009.02.050
9. Obata Y, Ishimori N, Saito A, Kinugawa S, Yokota T, Takada S, et al. Activation of invariant natural killer T cells by alpha-galactosylceramide ameliorates doxorubicin-induced cardiotoxicity in mice. Eur J Prev Cardiol (2020) 27:2358–61. doi: 10.1177/2047487319901208
10. Furihata T, Maekawa S, Takada S, Kakutani N, Nambu H, Shirakawa R, et al. Premedication with pioglitazone prevents doxorubicin-induced left ventricular dysfunction in mice. BMC Pharmacol Toxicol (2021) 22:27. doi: 10.1186/s40360-021-00495-w
11. Kadowaki H, Akazawa H, Ishida J, Komuro I. Mechanisms and management of immune checkpoint inhibitor-related cardiac adverse events. JMA J (2021) 4:91–8. doi: 10.31662/jmaj.2021-0001
12. Togashi Y, Shitara K, Nishikawa H. Regulatory T cells in cancer immunosuppression - implications for anticancer therapy. Nat Rev Clin Oncol (2019) 16:356–71. doi: 10.1038/s41571-019-0175-7
13. Lu Y, Xia N, Cheng X. Regulatory T cells in chronic heart failure. Front Immunol (2021) 12:732794. doi: 10.3389/fimmu.2021.732794
14. Ameri P, Canepa M, Anker MS, Belenkov Y, Bergler-Klein J, Cohen-Solal A, et al. Cancer diagnosis in patients with heart failure: epidemiology, clinical implications and gaps in knowledge. Eur J Heart Fail (2018) 20:879–87. doi: 10.1002/ejhf.1165
15. Rohrmann S, Witassek F, Erne P, Rickli H, Radovanovic D. Treatment of patients with myocardial infarction depends on history of cancer. Eur Heart J Acute. Cardiovasc Care (2018) 7:639–45. doi: 10.1177/2048872617729636
16. Thommen DS, Schumacher TN. T Cell dysfunction in cancer. Cancer Cell (2018) 33:547–62. doi: 10.1016/j.ccell.2018.03.012
17. Koelwyn GJ, Newman AAC, Afonso MS, Van Solingen C, Corr EM, Brown EJ, et al. Myocardial infarction accelerates breast cancer via innate immune reprogramming. Nat Med (2020) 26:1452–8. doi: 10.1038/s41591-020-0964-7
18. Kramer PA, Ravi S, Chacko B, Johnson MS, Darley-Usmar VM. A review of the mitochondrial and glycolytic metabolism in human platelets and leukocytes: implications for their use as bioenergetic biomarkers. Redox Biol (2014) 2:206–10. doi: 10.1016/j.redox.2013.12.026
19. Buck MD, O’sullivan D, Klein Geltink RI, Curtis JD, Chang CH, Sanin DE, et al. Mitochondrial dynamics controls T cell fate through metabolic programming. Cell (2016) 166:63–76. doi: 10.1016/j.cell.2016.05.035
20. Liu X, Peng G. Mitochondria orchestrate T cell fate and function. Nat Immunol (2021) 22:276–8. doi: 10.1038/s41590-020-00861-6
21. Bengsch B, Johnson AL, Kurachi M, Odorizzi PM, Pauken KE, Attanasio J, et al. Bioenergetic insufficiencies due to metabolic alterations regulated by the inhibitory receptor pd-1 are an early driver of cd8(+) t cell exhaustion. Immunity (2016) 45:358–73.
22. Scharping NE, Rivadeneira DB, Menk AV, Vignali PDA, Ford BR, Rittenhouse NL, et al. Mitochondrial stress induced by continuous stimulation under hypoxia rapidly drives T cell exhaustion. Nat Immunol (2021) 22:205–15. doi: 10.1038/s41590-020-00834-9
23. Scharping NE, Menk AV, Moreci RS, Whetstone RD, Dadey RE, Watkins SC, et al. The tumor microenvironment represses T cell mitochondrial biogenesis to drive intratumoral T cell metabolic insufficiency and dysfunction. Immunity (2016) 45:374–88. doi: 10.1016/j.immuni.2016.07.009
24. Shirakawa R, Yokota T, Nakajima T, Takada S, Yamane M, Furihata T, et al. Mitochondrial reactive oxygen species generation in blood cells is associated with disease severity and exercise intolerance in heart failure patients. Sci Rep (2019) 9:14709. doi: 10.1038/s41598-019-51298-3
25. Tyrrell DJ, Bharadwaj MS, Jorgensen MJ, Register TC, Molina AJ. Blood cell respirometry is associated with skeletal and cardiac muscle bioenergetics: Implications for a minimally invasive biomarker of mitochondrial health. Redox Biol (2016) 10:65–77. doi: 10.1016/j.redox.2016.09.009
26. Hatae R, Chamoto K, Kim YH, Sonomura K, Taneishi K, Kawaguchi S, Yoshida H, et al. Combination of host immune metabolic biomarkers for the PD-1 blockade cancer immunotherapy. JCI Insight (2020) 5:e133501. doi: 10.1172/jci.insight.133501
27. Yu YR, Imrichova H, Wang H, Chao T, Xiao Z, Gao M, et al. Disturbed mitochondrial dynamics in CD8(+) TILs reinforce T cell exhaustion. Nat Immunol (2020) 21:1540–51. doi: 10.1038/s41590-020-0793-3
28. Vardhana SA, Hwee MA, Berisa M, Wells DK, Yost KE, King B, et al. Impaired mitochondrial oxidative phosphorylation limits the self-renewal of T cells exposed to persistent antigen. Nat Immunol (2020) 21:1022–33. doi: 10.1038/s41590-020-0725-2
29. Sade-Feldman M, Yizhak K, Bjorgaard SL, Ray JP, De Boer CG, Jenkins RW, et al. Defining T cell states associated with response to checkpoint immunotherapy in melanoma. Cell (2019) 176:404. doi: 10.1016/j.cell.2018.12.034
30. Twyman-Saint Victor C, Rech AJ, Maity A, Rengan R, Pauken KE, Stelekati E, et al. Radiation and dual checkpoint blockade activate non-redundant immune mechanisms in cancer. Nature (2015) 520:373–7. doi: 10.1038/nature14292
31. Nishi M, Wang P, Hwang PM. Cardiotoxicity of cancer treatments: Focus on anthracycline cardiomyopathy. Arterioscler Thromb Vasc Biol (2021) 41:2648–60. doi: 10.1161/ATVBAHA.121.316697
32. Iida N, Dzutsev. A, Stewart CA, Smith L, Bouladoux N, Weingarten RA, et al. Commensal bacteria control cancer response to therapy by modulating the tumor microenvironment. Science (2013) 342:967–70. doi: 10.1126/science.1240527
33. Moreira JBN, Wohlwend M, Wisloff U. Exercise and cardiac health: physiological and molecular insights. Nat Metab (2020) 2:829–39. doi: 10.1038/s42255-020-0262-1
34. Murphy RM, Watt MJ, Febbraio MA. Metabolic communication during exercise. Nat Metab (2020) 2:805–16. doi: 10.1038/s42255-020-0258-x
35. Takada S, Sabe H, Kinugawa S. Abnormalities of skeletal muscle, adipocyte tissue, and lipid metabolism in heart failure: Practical therapeutic targets. Front Cardiovasc Med (2020) 7:79. doi: 10.3389/fcvm.2020.00079
36. Pedersen L, Idorn M, Olofsson GH, Lauenborg B, Nookaew I, Hansen RH, et al. Voluntary running suppresses tumor growth through epinephrine- and IL-6-Dependent NK cell mobilization and redistribution. Cell Metab (2016) 23:554–62. doi: 10.1016/j.cmet.2016.01.011
37. Gomes-Santos IL, Amoozgar Z, Kumar AS, Ho WW, Roh K, Talele NP, et al. Exercise training improves tumor control by increasing CD8(+) T-cell infiltration via CXCR3 signaling and sensitizes breast cancer to immune checkpoint blockade. Cancer Immunol Res (2021) 9:765–78. doi: 10.1158/2326-6066.CIR-20-0499
38. Kurz E, Hirsch CA, Dalton T, Shadaloey SA, Khodadadi-Jamayran A, Miller G, et al. Exercise-induced engagement of the IL-15/IL-15Ralpha axis promotes anti-tumor immunity in pancreatic cancer. Cancer Cell (2022) 40:720–737.e725. doi: 10.1016/j.ccell.2022.05.006
39. Takada S, Sabe H, Kinugawa S. Treatments for skeletal muscle abnormalities in heart failure: sodium-glucose transporter 2 and ketone bodies. Am J Physiol Heart Circ Physiol (2022) 322:H117–28. doi: 10.1152/ajpheart.00100.2021
40. Christensen JF, Jones LW, Andersen JL, Daugaard G, Rorth M, Hojman P. Muscle dysfunction in cancer patients. Ann Oncol (2014) 25:947–58. doi: 10.1093/annonc/mdt551
Keywords: immune-checkpoint inhibition, mitochondrial oxidative phosphorylation, disease prevention, myokine, PBMC, exercise, diet, reactive oxygen species
Citation: Takada S, Kinugawa S, Handa H, Yokota T and Sabe H (2022) Cross-disease communication between cancer and heart failure provides a rational approach to prevention and treatment of both diseases. Front. Oncol. 12:1006322. doi: 10.3389/fonc.2022.1006322
Received: 29 July 2022; Accepted: 29 September 2022;
Published: 31 October 2022.
Edited by:
Reo Hamaguchi, Juntendo University, JapanReviewed by:
Marius Sudol, Icahn School of Medicine at Mount Sinai, United StatesAntonis Koromilas, McGill University, Canada
Copyright © 2022 Takada, Kinugawa, Handa, Yokota and Sabe. This is an open-access article distributed under the terms of the Creative Commons Attribution License (CC BY). The use, distribution or reproduction in other forums is permitted, provided the original author(s) and the copyright owner(s) are credited and that the original publication in this journal is cited, in accordance with accepted academic practice. No use, distribution or reproduction is permitted which does not comply with these terms.
*Correspondence: Shingo Takada, cy10YWthZGFAaG9rdXNoby11LmFjLmpw; cy10YWthZGFAaG90bWFpbC5jby5qcA==; Shintaro Kinugawa, a2ludWdhd2Euc2hpbnRhcm8uNzg2QG0ua3l1c2h1LXUuYWMuanA=; Hisataka Sabe, c2FiZWhAbWVkLmhva3VkYWkuYWMuanA=; c2FiZWhAaWdtLmhva3VkYWkuYWMuanA=