- Department of Oncology, Shanghai Pulmonary Hospital and Thoracic Cancer Institute, School of Medicine, Tongji University, Shanghai, China
One of the most frequent distant metastases of lung cancer occurs in the brain. The average natural survival duration for patients with lung cancer who have brain metastases is about 1 to 2 months. Knowledge about brain metastases is currently restricted since they are more difficult to acquire than other metastases. This review begins with an analysis of the immune microenvironment of brain metastases; focuses primarily on the functions of microglia, astrocytes, neurons, and tumor-infiltrating lymphocytes in the microenvironment of brain metastases; and offers an atlas of the immune microenvironment of brain metastases involving significant cells. In an effort to give researchers new research ideas, the study also briefly covers how immunotherapy for non-small cell lung cancer with brain metastases is currently faring.
1 Introduction
Lung cancer is the leading cause of cancer-related deaths worldwide. The majority of lung cancers (85%) are non-small cell lung cancers (NSCLCs), which are typically found at an advanced stage and have a dismal 5-year overall survival (OS) of 15%–21% (1). The greatest worry in advanced NSCLC is metastasis, which is typically defined as a process in which malignant cells migrate from the initial location to distant sites and indicates considerable mortality and worse quality of life (2). The metastasis of NSCLC has complex organ-tropism mechanisms, mainly involving the organs of the brain, liver, and bone (3). Due to the challenges in obtaining the tissue, the field of brain metastasis (BM) is by far the least explored of these sites. BM affects 15%–43% of lung cancer patients, with an OS of about 1–2 months for untreated individuals (4, 5). Systemic therapy such as chemotherapy and topical medications such as surgical resection, whole-brain radiation therapy, and stereotactic radiosurgery (SRS) are routinely performed, with limited improvement of OS for patients (6). Immunotherapy provides insight into this dilemma.
The landscape of advanced NSCLC has changed dramatically as a result of the findings from clinical trials like KEYNOTE-024 (7) and KEYNOTE-189 (8). However, the fact that the efficacy of therapeutic agents against BM was discouraging excluded the enrollment of patients with BM in immune checkpoint inhibitor (ICI) clinical trials, resulting in the blank of NSCLC with BM in the ICI field. The impermeability of the blood–brain barrier (BBB) and the complicated location of brain metastases restrict the use of treatment medicines and put a cloud over BM patients (9). The BBB, a structure peculiar to the central nervous system (CNS) and made up of astrocytes, pericytes, and endothelial cells with tight connections, protects the CNS from circulating toxins and inflammation. The BBB has historically been assumed to be difficult for therapeutic medicines with large molecular sizes and limited solubility to pass, making them impossible to exhibit the anti-tumor effect of these regimens in the CNS (10). However, more ICIs have recently shown promise in the routine care of BM patients, pointing to the possibility of a remote interaction between tumor cells and the distinct tumor immune microenvironment (TIME) (11).
In this review, we will summarize the complex TIME in the brain parenchyma and the evolving scenario of the application of ICIs in BM patients to construct a comprehensive understanding of BM.
2 TIME and clinical practice
2.1 TIME
2.1.1 Microglia/BMDMs is the main immune cells in the CNS
The CNS’s principal resident immune cell and the first line of innate immunity, the microglia, is an essential part of the CNS (12). Microglia, which are referred to as the “third element of the nervous system”, are only produced from progenitors found in the yolk sac and developed during the embryonic stage (13). Presenting throughout the CNS, mainly the gray matter (14), these myeloid lineage cells are estimated to constitute up to 5%–10% of the cellular composition (15–17).
The CNS’s homeostasis and the growth of the brain are primarily supported by microglia. Evidence from time-lapse recording suggested that microglia might scan the brain parenchyma during neuronal activation and play a role in synapse modulation (18, 19). In addition, microglia participate in a variety of physiological activities, including the removal of cellular waste and neurogenesis (20, 21). Microglia typically undergo a transformation toward an active phenotype in response to pathogens and cancerous cells as part of their defensive function, allowing them to monitor the microenvironment and keep an eye out for any potential disturbances (22). Pro- and anti-inflammatory cytokines and chemokines are usually released to modulate the scenario of infection or metastasis foci as well (23).
However, bone marrow-derived macrophages (BMDMs) are frequently recruited in the TIME of BM in addition to microglia (24). It is challenging to distinguish between microglia and BMDMs in part because there are few experimental methods and biomarkers available. The advancement of technology gives us opportunities to learn more in this area that have never existed before. Microglia is a differentiated cell that can self-renew (25), while BMDMs replenish through peripheral monocytosis (24). Under physiological settings, microglia appear in a ramified resting status, but when activated, they transform into hypertrophic and finally ameboid active forms (14). In contrast to microglia, BMDMs exhibit a roundish morphology while quiescent and turn stretched and elongated in M2 phenotypes (26), demonstrating the distinction. Microglia and BMDMs shared certain similar markers, such as CD11b, ionized calcium-binding adaptor molecule 1, CD68, and CX3C chemokine receptor 1, even though distinct ontogenesis gave the two different physical characteristics (27–30). The distinction between microglia and BMDMs is the subject of more widespread debate. Among the inflammatory of T. gondii, the analysis of mouse brain revealed that microglia were the CD11b+/CD45low population, compared with the CD11b+/CD45high for macrophage (31). More stable markers are urgently required due to the volatility of CD45 expression after the injury or disease. Transmembrane protein 119, which was not expressed in BMDMs, was discovered by Bennett et al. using a fluorescence-activated cell sorter in conjunction with RNA sequencing to identify microglia as a stable and reliable marker (32). Using Ccr2RFP/+ mice, in which monocytes infiltrating the brain are marked with a red fluorescent protein (RFP) and presenting with the result that RFP+ monocytes were negative for sialic acid-binding immunoglobulin-like lectin H (Siglec-H), Konishi et al. confirmed that Siglec-H was unique to microglia. Siglec-H, a transmembrane lectin, has also been discovered as a marker for microglia from developmental to mature stages by transcriptome analysis and immunohistochemistry (33, 34). All microglia expressed green fluorescent protein (GFP), while only approximately 5% of CNS-associated macrophages did, according to research by Buttgereit et al. using Sall1 reporter mice (Sall1GFP/+). This finding demonstrated that Sall1 was confined to microglia (35). Other markers have also demonstrated the capacity to differentiate between microglia and BMDMs such as CD49D/integrin subunit alpha 4, a marker exclusive to tumor-infiltrating BMDMs (24). However, it should be highlighted that knowledge of microglia and BMDMs is still in its infancy, necessitating a deeper examination of functional analysis in light of the complex situation.
Microglia/BMDMs are crucial to the CNS processes of metastasis, dormancy, and relapse. Clarifying the relationship between microglia/BMDMs and cancer cells is essential. BBB protects BM by acting as a guard to maintain homeostasis. Numerous microglia have been seen in the perivascular region, suggesting that they may be involved in the BBB’s regulation (36). By using the purinergic receptor P2Y, G-protein coupled, 12 (P2RY12) inhibitor clopidogrel, and P2RY12−/− mice, Lou et al. confirmed that microglia could contribute to the resealing of BBB and keep its integrity in a P2RY12-dependent manner (37). Claudin-5 is initially expressed by microglia in order to make contact with endothelial cells and preserve the BBB’s integrity. Astrocytic end-feet are engulfed by activated microglia, which also promote persistent inflammation and BBB damage (38). Other pro-inflammatory cytokines including tumor necrosis factorα (TNF-α), interleukin-1β (IL-1β), and interleukin-6 (IL-6) are also used by microglia to maintain the integrity of the BBB (39).
As the most prevalent tissue-resident macrophage, microglia/BMDMs contribute to the preservation of the BBB as well as the destruction of cancer cells and the growth of BM. The polarization status of M1 microglia is characterized by secreting pro-inflammatory cytokines such as IL-1β, TNF-α, inducible nitric oxide synthase, and reactive oxygen species. M2-type microglia promote tumor growth and are activated by interleukin-4, interleukin-10, and transforming growth factor-β (40). The cytokines secreted by the different states of microglia/BMDMs played the opposite effect. This field needs to be further explored as well.
2.1.2 Astrocyte play a bidirectional role in patients with BM
The largest and most prevalent cell type is the astrocyte, a star-shaped neuroglia that has five times as many cells as neurons. Recent research has demonstrated that the function of nearby neurons is matched by the astrocytes, which occur in intraregional heterogeneity (41, 42). Astrocytes perform a number of crucial tasks, such as ion homeostasis, neurotransmitter recovery, synapse formation, and BBB modulation, due to their diverse physiological activities and ubiquitous nature. Astrocytes cause morphological and transcriptomic alterations and turn into reactive astrocytes (RAs) in response to a stimuli, such as a disease or injury (43). RAs are traditionally characterized by high glial fibrillary acidic protein (44). Technology advancements have made it possible to define the status of RAs using cutting-edge methods like transcriptome analysis, and studies reveal that complex functional alterations occur in RAs across various disease models (45).
At the beginning of metastasis, astrocytes had the ability to cause Fas-dependent death in brain-tropic cells (46). However, it should be noted that RAs succumb to tumor progression because of the paracrine cytokine communication loops between them and cancer cells, which create a pro-tumorigenic environment. Seike et al. carried out co-culture studies based on the aforementioned theory, confirming the reciprocal connection between lung cancer cells and astrocytes. The researchers discovered that substances targeting tumor cells, such as macrophage migration inhibitory factor, interleukin-8, and plasminogen activator inhibitor-1, might activate RAs. Additionally, RAs may emit cytokines like IL-6, TNF-α, and IL-1β that may encourage the growth of cancer cells like PC-9, QG56, and EBC-1, which are generated from human lung cancer (47). The melanoma-to-brain metastasis evidenced the bidirectional signaling in BM. The RAs reprogrammed by melanoma cells expressed interleukin-23 to enhance the invasiveness of cancer cells, and cancer cell-derived matrix metalloproteinase-2 affected the status of astrocytes (48). In patient-derived xenografts of melanoma, polyunsaturated fatty acids, such as amino acid and mead acid, were found to be an RA-derived component to triggered proliferator-activated receptor signaling, hence boosting the growth of the tumor as well (49). Further analysis labeled a subpopulation of RAs with signal transducer and activator of transcription 3 (STAT3), providing the significant role of these RAs in BM. Additionally, microglia and macrophages could interact with RAs with activated STAT3 to create growth-promoting substances and prevent CD8+ T-cell activation (50).
The direct contact between RAs and brain-tropic cells and the pervasive communication through secreted substances play a part in the TIME of BM. Gap junction intercellular communication (GJIC) serves as a possible target and is crucial for preserving tissue homeostasis. Through the GJIC, calcium (Ca2+), an ion involved in homeostasis, is exchanged between RAs (51). Numerous gliotransmitters are said to be released as a result of the Ca2+ signaling. Due to the fact that it causes DNA damage, it is thought to be harmful to cancer cells (52). Lin et al. conducted co-culture experiments and found that the communication of Ca2+ between RAs and tumor cells process the ability to determine the fate of BM. The study suggested that direct physical contact rather than the factors secreted by RAs play a role in the chemoresistance of tumor cells. The proliferation and chemoresistance of melanoma to brain metastasis cells were aided by RAs’ capacity to remove Ca2+ from tumor cells (53). Additionally, recent studies proved that protocadherin 7 (PCDH7) expressed by breast and lung cancer cells could promote the carcinoma–astrocyte gap junction mediated by connexin 43 (Cx43). The gap junction, which sent the second messenger cGAMP from the cancer towards the astrocytes, may be substantially hampered by PCDH7 downregulation. Activating the STING pathway by cGAMP enables astrocytes to express inflammatory factors such as TNF-α and interferon-α. Consequently, STAT1 and NF-κB pathways are activated in metastatic cells to support their proliferation and chemoresistance (54). All the above evidence suggested that GJIC between RAs and malignant cells possess the ability to regulate and rebuild the TIME of BM.
As a result of a number of discoveries, it is clear that RAs play a crucial role in BM by interacting in a variety of ways and serving as a necessary part of the CNS. Malignant brain-tropic cells have taken control of RAs, making them no longer passive spectators. Research is still needed to fully understand the intricate structure of the BM microenvironment.
2.1.3 Neuron is hijacked by tumor cells in BM
As highly specialized cells, the role of neurons in the CNS structure and function has been appreciated for a long time (55, 56). Neuron is the most important cell that processes high plasticity; it exerts functions such as action understanding, empathy, imitation, intention understanding, and language development in the CNS (57–59). Although extensive study has been done on the examination of neurons, it is still unclear how much function neurons have in BM.
Classical electrochemical communication is frequently mediated by neural chemicals released by neurons, such as neurotransmitters and neuropeptides (60). However, as revealed by recent studies, cancer-infected neurons operate as both a hub for information transmission and a driver of tumor growth and development (61, 62). The study by Deshpande et al. focused on the tumor–neuron interaction at the initial state of metastasis. By co-culturing neurons with breast and lung cancer cells, they tried to mimic the potential interaction between brain-seeking tumor cells and neurons and survey the induction of neurotransmitter and synaptic signaling. It was shown that neurons could cause the overexpression of neurotransmitters in tumors by observing the activation of genes for classical neurotransmitter receptors and neuronal synaptic mediators in tumors (63). The interaction between tumor and neurons allows one to target cancer in a neuron-dependent way. Cordero et al. engineered human neural stem cells LM008, which could continuously secrete functional antibodies against HER2 (anti-HER2Ab). By inducing similar effects to trastuzumab in HER2+ overexpressing breast cancer brain metastases (BCBM) models, the LM008 showed the ability to inhibit the proliferation of BCBM in the PI3K-Akt signaling pathway (64).
Additionally, a study of resected breast to brain metastasis (B2BM) specimens revealed a GABAergic phenotype comparable to neurons, which suggested that tumor cells escape their genetic restraints and are prepared for the microenvironment in the BM (65). As more is learned, the direct synapses between neurons and cancer cells aroused curiosity among scientists. According to Zeng et al., B2BM can construct pseudo-tripartite synapses with glutamatergic neurons, using the glutamate released by neurons to stimulate colony development via GluN2B-mediated N-methyl-D-aspartate receptors (NMDARs) (66). They demonstrated that NMDARs, a receptor involved in the transmission of nerve impulses, were upregulated on the cell membranes of B2BM as well (66). Additionally, as presented by Venkataramani et al., glutamatergic synapse also exerted its function in gliomas. Contrary to B2BM, malignant cell activity is promoted by the connections between presynaptic neurons and postsynaptic glioma cells via glutamate-mediated AMPA receptors (67). According to the studies mentioned above, BM may act like neurons to create connections in the CNS that will support the growth of that region. The findings show a bidirectional link between them and suggest that these signaling axes could one day lead to promising treatment options for BM.
2.1.4 Tumor-infiltrating lymphocytes are the atypical cells in the BM
Lymphocytes, which develop from bone marrow stem cells, often serve as barriers against the invasion of various immune system disorders in the periphery (68). Regarding the CNS, the distinctive BBB structure restricts lymphocyte permeation and patrolling to, in the majority of cases, prevent additional damage to the CNS parenchyma (69).
However, with the impairment of the BBB’s integrity resulting from the malignant cell, lymphocytes could be recruited to the CNS and play bidirectional functions, which refers to restricting the metastasis of cancer cells or causing an inflammation in the brain parenchyma (70, 71). Fully understanding the complete scenario of CNS after the influx of lymphocytes caused by BM may yield an even more pronounced effect on developing new therapeutic regimens (72).
The presentation of TILs in the BM is sophisticated, in which the percentage and composition of tumor-infiltrating lymphocyte (TIL) subtypes vary a lot (73). Berghoff et al. conducted immunohistochemistry for biomarkers in the 116 BM specimens (61 from NSCLC BM). The findings demonstrated that the majority of samples exhibited infiltration of CD8+ TILs and dense CD3+ TILs, which suggested a potential successful TIME for immunotherapy; 26.2% of NSCLC specimens also had PD1+ TIL infiltration (74). TIL comparisons between BM and primary tumors revealed more data. In order to assess the expression of programmed death-ligand 1 (PD-L1), the profile of important T-cell subsets, activation and proliferation indicators, and coinhibitory receptors in lung and brain metastases, Lu et al. employed multiplexed quantitative immunofluorescence. In comparison to the main tumor, BM tissue displayed decreased levels of granzyme B, a marker for the efficiency of T cells, as well as CD3+, CD4+, CD8+, and FOXP3+ TILs. In addition, BM showed decreased amounts of T-cell immunoglobulin and mucin domain-containing protein 3, programmed death 1 (PD-1), and lymphocyte-activation gene 3 in CD3+ T cells (75).
2.1.5 Others
Other BM cells presented in TIME of BM include oligodendrocyte, fibroblast, pericytes, and endothelial cells. All the above components play a unique role in BM’s development. Further analysis is needed. The scenario of TIME in BM is generalized in Figure 1.
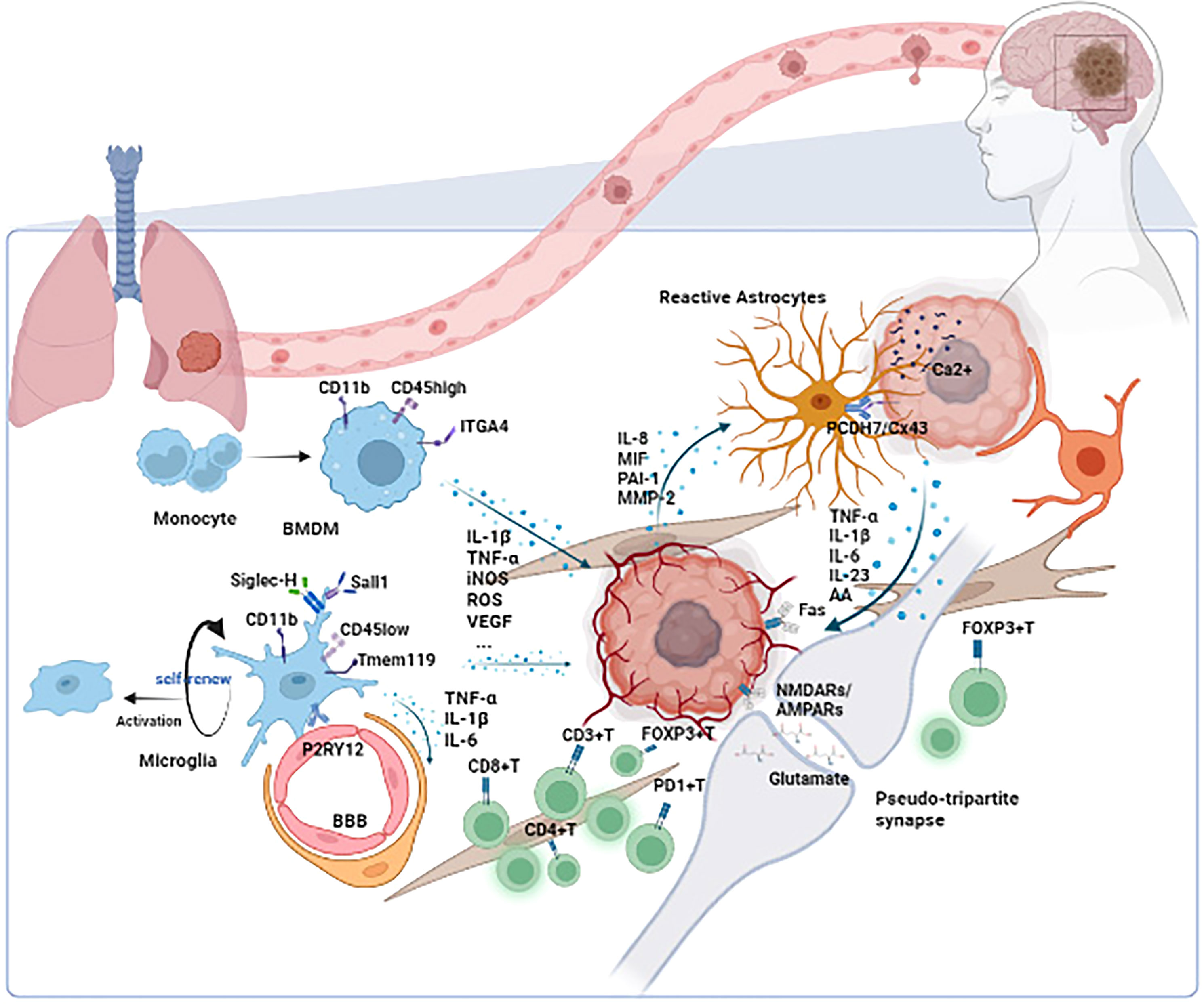
Figure 1 The scenario of TIME in BM. Microglia/BMDMs are the main macrophages in TIME of BM. Depending on the status of microglia/BMDMs, different cytokines such as IL-1β, TNF-α, and VEGF are secreted to influence the development of tumors. Astrocytes play their role through the paracrine cytokine signaling loops and direct contacts such as the PCDH7/Cx43 interaction. The tumor could also utilize the synapse between neuron and tumor to promote its development. Other cells such as lymphocytes, oligodendrocytes, fibroblasts, pericytes, and endothelial cells also function in TIME. TIME, tumor immune microenvironment; BM, brain metastasis; ITGA4, integrin subunit alpha 4; BMDM, bone marrow-derived macrophage; Siglec-H, sialic acid-binding immunoglobulin-like lectin H; Tmem 119, transmembrane protein 119; P2RY12, purinergic receptor P2Y, G-protein coupled, 12; BBB, blood–brain barrier; IL-1β, interleukin 1β; TNF-α, tumor necrosis factor α; iNOS, inducible nitric oxide synthase; ROS, reactive oxygen species; VEGF, vascular endothelial growth factor; IL-6, interleukin 6; IL-8, interleukin 8; MIF, migration inhibitory factor; PAI-1, plasminogen activator inhibitor-1; MMP-2, matrix metalloproteinase-2; PCDH7, protocadherin 7; Cx43, connexin 43; IL-23, interleukin 23; AA, amino acid; NMDARs, N-methyl-D-aspartate receptors; AMPARs, AMPA receptors.
2.2 Scenario of efficacy of ICIs in NSCLC with BM
2.2.1 ICI monotherapy
KEYNOTE-024 was a phase III randomized clinical trial (RCT) that showed the significant superiority of pembrolizumab in advanced NSCLC with PD-L1 expression of at least 50% (7). The median OS (mOS) and median progression-free survival (mPFS) in pembrolizumab were 26.3 months and 7.7 months, compared with 13.4 months and 5.5 months in chemotherapy, respectively (76). However, in the subgroup analysis of brain metastasis patients, which includes 28 (9.2%) patients, the benefit of pembrolizumab was not significant (7). Moreover, Mansfield et al. conducted a pooled analysis of KEYNOTE-001, KEYNOTE-010, KEYNOTE-024, and KEYNOTE-042, which explored the outcomes of pembrolizumab in PD-L1-positive [tumor proportion score (TPS) ≥ 1%] NSCLC with BM (77). Among the 293 (9.2%) patients with BM, pembrolizumab showed a better OS and PFS in both TPS ≥ 1% [OS: hazard ratio (HR) 0.83 95% confidence interval (CI) 0.62–1.10; PFS: HR 0.96 95% CI 0.73–1.25) and TPS ≥ 50% (OS: HR 0.67 95% CI 0.44–1.02; PFS: HR 0.70 95% CI 0.47–1.03) subgroups, while the difference was not significant as well. Another analysis exploring the efficacy of pembrolizumab in patients with BM was conducted in a cohort of melanoma and NSCLC (11). This prospective study enrolled 37 (88.1%) NSCLC patients with PD-L1 expression of at least 1%, of whom the mPFS was 1.9 months (95% CI 1.8–3.7), and the mOS was 9.9 months (95% CI 7.5–29.8) (78).
Nivolumab, another anti-PD-1 antibody, yields similar results to pembrolizumab. In the subgroup analysis of CheckMate-078, 72 (14.3%) patients presented with BM. The nivolumab group containing 45 NSCLC experienced longer OS (HR 0.82 95% CI 0.62–1.10) (79). CheckMate-017, as well as CheckMate-057, explored the efficacy of nivolumab in patients with treated advanced NSCLC. With the success of both trials, nivolumab was approved by Food and Drug Administration and recommended by the National Comprehensive Cancer Network (80, 81). However, due to the limited number of patients with BM included in both trials, further analysis may conduct a large bias. Until recently, a pooled study of CheckMate-017, CheckMate-057, and CheckMate-063 that compared nivolumab with docetaxel showed that NSCLC with BM benefited more from nivolumab (mOS: 8.4 versus 6.2 months HR not mentioned) (82). The EMPOWER-Lung 1 trial examined the efficacy and safety of cemiplimab in untreated advanced NSCLC with PD-L1 at least 50% and enrolled 68 (12.1%) patients with treated BM. The analysis of mPFS (HR 0.45 95% CI 0.22–0.92) and mOS (HR 0.17 95% CI 0.04–0.76) showed that patients benefited significantly from cemiplimab, which showed more promising results to some degree (83).
The efficacy of atezolizumab in NSCLC with BM had been evaluated in OAK and FIR trials. OAK was a phase III RCT that explored the role of atezolizumab in previously treated advanced NSCLC and further demonstrated the potential benefit. A total of 85 patients were included in the subgroup analysis of BM, with 38 (8.9%) receiving atezolizumab, who yielded longer OS (20.1 vs. 11.9 months HR 0.54 95% CI 0.31–0.94) (84). FIR was a phase II trial evaluating atezolizumab, including 13 (9.4%) NSCLC presented with BM. The results showed that the objective response rate (ORR) was 23%, mPFS was 4.3 months, and mOS was 6.8 months in BM patients (85). The specific information of trials mentioned above and other retrospective studies (86–89) are presented in Table 1.
All the trials above suggest that although advanced NSCLC benefits significantly from ICI monotherapy, the improvement of survival benefit to BM patients from ICI monotherapy is still finite, presented with the mPFS varying from 1.7 months to 4.9 months, and mOS varying from 20.1 months to 5.8 months. Further RCTs are needed to explore the efficacy of ICI monotherapy in NSCLC with BM.
2.2.2 ICI combined with chemotherapy
Due to the slow onset of the anti-tumor immune response and the limited efficacy of ICI monotherapy in NSCLC patients with BM, the synergy effects between ICIs and chemotherapy aroused the interest of scientists. Many trials revealed that chemotherapy helps improve the efficacy of immunotherapy for NSCLC patients with BMs.
KEYNOTE-189 revealed that advanced non-squamous NSCLC benefits from adding pembrolizumab to chemotherapy in first-line treatment, with an mPFS and mOS of 9.0 months and 22 months, respectively (93). As for the BM subgroup, 73 (67.6%) patients were in the ICI-based combination group, while 35 (32.4%) were in the chemotherapy group, and significant prolonged PFS (6.9 vs. 4.7 months HR 0.42 95% CI 0.27–0.67) and OS (19.2 vs. 7.5 months HR 0.41 95% CI 0.24–0.67) were observed, which suggested the promising future for the combination of ICIs and chemotherapy (111). The subgroup analysis of BM patients in KEYNOTE-407 was not reported, which explored similar treatment regimes in advanced squamous NSCLC (112). Because of the limited data on the efficacy of pembrolizumab–chemotherapy for NSCLC with BM, Powell et al. conducted a pooled analysis based on KEYNOTE-021, KEYNOTE-189, and KEYNOTE-407. A total of 171 NSCLC patients with BM were enrolled in this study, and the results were similar to KEYNOTE-189, which showed that platinum-doublet chemotherapy with the addition of pembrolizumab not only prolonged the PFS (6.9 vs. 4.1 months, HR 0.44, 95% CI 0.31–0.62) but also improved the OS (18.8 vs. 7.6 months, HR 0.48, 95% CI 0.32–0.70) (95).
Several domestic regimes such as camrelizumab, sintilimab, toripalimab, and tislelizumab achieve promising results nowadays. Seventeen patients with BM were enrolled in CameL, a trial comparing the efficacy of camrelizumab plus chemotherapy with chemotherapy alone in previously untreated non-squamous NSCLC, and the results showed that the ICI combination group benefits more in PFS (HR 0.14, 95% CI 0.01–0.88) (96). ORIENT-11 obtained similar results as well; advanced non-squamous NSCLC patients with BM (58/397) benefited from sintilimab plus pemetrexed-platinum more with a prolonged PFS (HR 0.49, 95% CI 0.26–0.92) and OS (HR 0.57, 95% CI 0.28–1.16), though the difference of OS was not significant (97). However, for the squamous NSCLC, the subgroup analysis of BM patients was not reported in both clinical trials (CameL-sq and ORIENT-12) (113, 114). As for toripalimab and tislelizumab, results for the untreated NSCLC with BM were not reported yet. A phase II study exploring the toripalimab in EGFR-TKI treated patients is presented in Table 1 (99).
Atezo-Brain was a single-arm phase II study reporting the efficacy of atezolizumab, an anti-PD-L1 antibody, in untreated non-squamous NSCLC patients with BM. A total of 40 patients were included, and the systemic PFS and intracranial PFS (iPFS) were 8.9 months (95% CI 6.7–12.9) and 7.1 months (95% CI 4.6–11.2), respectively (100). The detailed information of trials is presented in Table 1.
2.2.3 ICI combined with radiotherapy
As the standard localized therapy for BM patients, radiotherapy could relieve nervous symptoms quickly; thus, the combination of radiotherapy and ICI possibly form the best alliance in clinical practice. A phase II trial enrolling 22 (84.6%) NSCLC with BM evaluated the efficacy and safety of the combination of nivolumab and stereotactic brain radiosurgery. The results showed that upfront SRS during nivolumab in NSCLC was tolerated, and the median iPFS and systematic PFS were 5 months and 2.9 months, respectively (101). Singh et al. conducted a retrospective study assessing the local tumor response and survival outcomes in advanced NSCLC between the group of ICIs plus SRS versus chemotherapy plus SRS. Although the OS and the safety between the two groups showed no significant difference (10 vs. 11.6 months, p = 0.23), ICIs plus SRS presented with a more significant lesion shrank (90% vs. 47.8%, p = 0.001) among the subgroup of patients with brain lesions larger than 500 mm3 (102). Minniti et al. conducted a similar study on NSCLC and melanoma patients receiving postoperative SRS with or without ICIs. The addition of ICIs to SRS significantly prolonged the OS and the PFS. Except for the outcomes above, this study focused on controlling leptomeningeal disease (LMD) and found that the combination decreased the incidence of LMD (103). Compared with SRS, stereotactic radiotherapy (SRT) showed its advantage in reducing the occurrence of radiation necrosis. Two similar retrospective analyses compared SRT plus ICIs versus SRT alone, and the results showed that the combination group improved the OS and local control with a safe profile (104, 105). The detailed information of trials is presented in Table 1.
2.2.4 Others
Except for the therapies mentioned above, others, such as multiple ICIs combination and anti-angiogenic agent combination, have also been thought to produce synergistic effects to NSCLC patients with BM. A post-hoc analysis focusing on the efficacy of ICI therapy in patients with BM of CheckMate-227 revealed that double-ICI regimens, nivolumab plus ipilimumab, worked well, resulting in prolonged OS (18.8 vs. 13.7 months, HR 0.57, 95% CI 0.38-0.85) compared with chemotherapy (106). Another trial exploring the dual-ICI therapy was CheckMate-9LA. Different from CheckMate-227, CheckMate-9LA compared the efficacy of nivolumab plus ipilimumab and two-cycle chemotherapy with four-cycle chemotherapy in advanced NSCLC (108). The results showed that ICIs–chemotherapy has an advantage over chemotherapy alone in the BM subgroup, presenting with a longer OS (19.3 vs. 6.8 months, HR 0.43, 95% CI 0.27–0.67) and PFS (10.6 vs. 4.1 months, HR 0.40, 95% CI 0.25–0.64) (109). As for the combination of anti-angiogenic regimes and ICIs, Chu et al. conducted a phase Ib study exploring the efficacy and safety of sintilimab plus anlotinib in advanced NSCLC. The trial enrolled 4 (18.2%) patients with BM, and 3/4 BM patients achieved partial response (PR) (110). The promising results above suggested the underlying value of multimodality ICI-based therapy in NSCLC with BM. However, it should be noted that there is a long way to go in this area. The detailed information of these trials is presented in Table 1.
3 Discussion and prospects
Patients with BM from lung cancer have poor prognoses and few therapy choices. Immunotherapy has entered a new era, and ICIs have ushered in a new era of treatment for this subgroup. We will be better able to comprehend the mechanism underlying the effectiveness of immunotherapy when we fully mine the TIME features of BM. The primary elements of the brain immune microenvironment are microglia and BMDMS, which have both direct and indirect impacts on the growth of brain metastases through a variety of cytokines. Microglia/BMDMS perform cytotoxic action, phagocytosis, and antigen presentation as part of their immunological function. Future research on this topic will concentrate on understanding how to switch microglia/BMDMS from the tumor-promoting M2 phase to the tumor-suppressing M1 phase. Changes in the functional status of astrocytes, which are the stromal cells of the central nervous system, have varying impacts on the microenvironment of brain metastases. According to studies, reactive astrocytes help create a fibrotic tumor brain microenvironment that is unfavorable to the effects of immunotherapy (115). Additionally, one of the significant elements influencing the effectiveness of immunotherapy is the quantity and activity of lymphocytes in brain metastases, and efficient T cells contribute to this improvement (116).
We also outlined the ongoing clinical trials on NSCLC with BM from the viewpoints of a single medicine, immune combined radiation, immune combined chemotherapy, and dual immune combination based on the existing clinical research. The efficacy and safety of ICIs in patients with BM are similar to those in the general population, according to an increasing body of study data. However, it is also important to keep in mind that most studies only assess patients with BM as a small subgroup; further research in this area is still required.
Author contributions
MX: Manuscript writing. CS: Conceptualization and manuscript revision. All authors contributed to the article and approved the submitted version.
Funding
This work was supported by the Shanghai Hospital Development Center (grant number: SHDC12020110), the Clinical Research Foundation of ShangHai Pulmonary Hospital (grant number: FKLY20013), and the Excellent Academic Leader of Shanghai “Science and Technology Innovation Action Plan” (grant number: 22XD1402500).
Conflict of interest
The authors declare that the research was conducted in the absence of any commercial or financial relationships that could be construed as a potential conflict of interest.
Publisher’s note
All claims expressed in this article are solely those of the authors and do not necessarily represent those of their affiliated organizations, or those of the publisher, the editors and the reviewers. Any product that may be evaluated in this article, or claim that may be made by its manufacturer, is not guaranteed or endorsed by the publisher.
References
1. Siegel RL, Miller KD, Fuchs HE, Jemal A. Cancer statistics, 2021. CA Cancer J Clin (2021) 71(1):7-33. doi: 10.3322/caac.21654
2. Geiger TR, Peeper DS. Metastasis mechanisms. Biochim Biophys Acta (2009) 1796(2):293–308. doi: 10.1016/j.bbcan.2009.07.006
3. Tamura T, Kurishima K, Nakazawa K, Kagohashi K, Ishikawa H, Satoh H, et al. Specific organ metastases and survival in metastatic non-small-cell lung cancer. Mol Clin Oncol (2015) 3(1):217–21. doi: 10.3892/mco.2014.410
4. Markesbery WR, Brooks WH, Gupta GD, et al. Treatment for patients with cerebral metastases. Arch Neurol (1978) 35(11):754–6. doi: 10.1001/archneur.1978.00500350058012
5. Wood SL, Pernemalm M, Crosbie PA, Whetton AD. The role of the tumor-microenvironment in lung cancer-metastasis and its relationship to potential therapeutic targets. Cancer Treat Rev (2014) 40(4):558–66. doi: 10.1016/j.ctrv.2013.10.001
6. Chamberlain MC, Baik CS, Gadi VK, Bhatia S, Chow LQM. Systemic therapy of brain metastases: non-small cell lung cancer, breast cancer, and melanoma. Neuro Oncol (2017) 19(1):1-24. doi: 10.1093/neuonc/now197
7. Reck M, Rodríguez-Abreu D, Robinson AG, Hui R, Csőszi T, Fülöp A, et al. Pembrolizumab versus chemotherapy for PD-L1-Positive non-Small-Cell lung cancer. N Engl J Med (2016) 375(19):1823–33. doi: 10.1056/NEJMoa1606774
8. Gandhi L, Rodríguez-Abreu D, Gadgeel S, Esteban E, Felip E, De Angelis F, et al. Pembrolizumab plus chemotherapy in metastatic non-Small-Cell lung cancer. N Engl J Med (2018) 378(22):2078–92. doi: 10.1056/NEJMoa1801005
9. Mo F, Pellerino A, Soffietti R, Rudà R. Blood-brain barrier in brain tumors: Biology and clinical relevance. Int J Mol Sci (2021) 22(23):12654. doi: 10.3390/ijms222312654
10. Pardridge WM. Drug transport across the blood-brain barrier. J Cereb Blood Flow Metab (2012) 32(11):1959–72. doi: 10.1038/jcbfm.2012.126
11. Goldberg SB, Gettinger SN, Mahajan A, Chiang AC, Herbst RS, Sznol M, et al. Pembrolizumab for patients with melanoma or non-small-cell lung cancer and untreated brain metastases: early analysis of a non-randomised, open-label, phase 2 trial. Lancet Oncol (2016) 17(7):976–83. doi: 10.1016/S1470-2045(16)30053-5
12. Lehnardt S. Innate immunity and neuroinflammation in the CNS: the role of microglia in toll-like receptor-mediated neuronal injury. Glia (2010) 58(3):253–63. doi: 10.1002/glia.20928
13. Rio-Hortega P. THE MICROGLIA. Lancet (1939) 233(6036):1023–6. doi: 10.1016/S0140-6736(00)60571-8
15. Gutmann DH, McLellan MD, Hussain I, Wallis JW, Fulton LL, Fulton RS, et al. Somatic neurofibromatosis type 1 (NF1) inactivation characterizes NF1-associated pilocytic astrocytoma. Genome Res (2013) 23(3):431–9. doi: 10.1101/gr.142604.112
16. Morantz RA, Wood GW, Foster M, Clark M, Gollahon K. Macrophages in experimental and human brain tumors. part 1: Studies of the macrophage content of experimental rat brain tumors of varying immunogenicity. J Neurosurg (1979) 50(3):298–304. doi: 10.3171/jns.1979.50.3.0298
17. Aguzzi A, Barres BA, Bennett ML. Microglia: scapegoat, saboteur, or something else? Science (2013) 339(6116):156–61. doi: 10.1126/science.1227901
18. Nimmerjahn A, Kirchhoff F, Helmchen F. Resting microglial cells are highly dynamic surveillants of brain parenchyma in vivo. Science (2005) 308(5726):1314–8. doi: 10.1126/science.1110647
19. Tremblay M-È, Lowery RL, Majewska AK. Microglial interactions with synapses are modulated by visual experience. PloS Biol (2010) 8(11):e1000527. doi: 10.1371/journal.pbio.1000527
20. Stephan AH, Barres BA, Stevens B. The complement system: an unexpected role in synaptic pruning during development and disease. Annu Rev Neurosci (2012) 35:369–89. doi: 10.1146/annurev-neuro-061010-113810
21. Marín-Teva JL, Dusart I, Colin C, Gervais A, van Rooijen N, Mallat M, et al. Microglia promote the death of developing purkinje cells. Neuron (2004) 41(4):535–47. doi: 10.1016/S0896-6273(04)00069-8
22. Lynch MA. The multifaceted profile of activated microglia. Mol Neurobiol (2009) 40(2):139–56. doi: 10.1007/s12035-009-8077-9
23. Lee YB, Nagai A, Kim SU. Cytokines, chemokines, and cytokine receptors in human microglia. J Neurosci Res (2002) 69(1):94-103. doi: 10.1002/jnr.10253
24. Bowman RL, Klemm F, Akkari L, Pyonteck SM, Sevenich L, Quail DF, et al. Macrophage ontogeny underlies differences in tumor-specific education in brain malignancies. Cell Rep (2016) 17(9):2445–59. doi: 10.1016/j.celrep.2016.10.052
25. Rossi F, Lewis C. Microglia's heretical self-renewal. Nat Neurosci (2018) 21(4):455–6. doi: 10.1038/s41593-018-0123-3
26. Rostam HM, Reynolds PM, Alexander MR, Gadegaard N, Ghaemmaghami AM. Image based machine learning for identification of macrophage subsets. Sci Rep (2017) 7(1):3521. doi: 10.1038/s41598-017-03780-z
27. Jung S, Aliberti J, Graemmel P, Sunshine MJ, Kreutzberg GW, Sher A, et al. Analysis of fractalkine receptor CX(3)CR1 function by targeted deletion and green fluorescent protein reporter gene insertion. Mol Cell Biol (2000) 20(11):4106–14. doi: 10.1128/MCB.20.11.4106-4114.2000
28. Zeiner PS, Preusse C, Golebiewska A, Zinke J, Iriondo A, Muller A, et al. Distribution and prognostic impact of microglia/macrophage subpopulations in gliomas. Brain Pathol (2019) 29(4):513–29. doi: 10.1111/bpa.12690
29. Ohsawa K, Imai Y, Sasaki Y, Kohsaka S. Microglia/macrophage-specific protein Iba1 binds to fimbrin and enhances its actin-bundling activity. J Neurochem (2004) 88(4):844–56. doi: 10.1046/j.1471-4159.2003.02213.x
30. Hopperton KE, Mohammad D, Trépanier MO, Giuliano V, Bazinet RP. Markers of microglia in post-mortem brain samples from patients with alzheimer's disease: a systematic review. Mol Psychiatry (2018) 23(2):177–98. doi: 10.1038/mp.2017.246
31. Suzuki Y, Claflin J, Wang X, Lengi A, Kikuchi T. Microglia and macrophages as innate producers of interferon-gamma in the brain following infection with toxoplasma gondii. Int J Parasitol (2005) 35(1):83–90. doi: 10.1016/j.ijpara.2004.10.020
32. Bennett ML, Bennett FC, Liddelow SA, Ajami B, Zamanian JL, Fernhoff NB, et al. New tools for studying microglia in the mouse and human CNS. Proc Natl Acad Sci U.S.A. (2016) 113(12):E1738–46. doi: 10.1073/pnas.1525528113
33. Chiu IM, Morimoto ETA, Goodarzi H, Liao JT, O'Keeffe S, Phatnani HP, et al. A neurodegeneration-specific gene-expression signature of acutely isolated microglia from an amyotrophic lateral sclerosis mouse model. Cell Rep (2013) 4(2):385–401. doi: 10.1016/j.celrep.2013.06.018
34. Konishi H, Kobayashi M, Kunisawa T, Imai K, Sayo A, Malissen B, et al. Siglec-h is a microglia-specific marker that discriminates microglia from CNS-associated macrophages and CNS-infiltrating monocytes. Glia (2017) 65(12):1927–43. doi: 10.1002/glia.23204
35. Buttgereit A, Lelios I, Yu X, Vrohlings M, Krakoski NR, Gautier EL, et al. Sall1 is a transcriptional regulator defining microglia identity and function. Nat Immunol (2016) 17(12):1397–406. doi: 10.1038/ni.3585
36. Anthony IC, Ramage SN, Carnie FW, Simmonds P, Bell JE. Does drug abuse alter microglial phenotype and cell turnover in the context of advancing HIV infection? [J]. Neuropathol Appl Neurobiol (2005) 31(3):325–38. doi: 10.1111/j.1365-2990.2005.00648.x
37. Lou N, Takano T, Pei Y, Xavier AL, Goldman SA, Nedergaard M. Purinergic receptor P2RY12-dependent microglial closure of the injured blood-brain barrier. Proc Natl Acad Sci U.S.A. (2016) 113(4):1074–9. doi: 10.1073/pnas.1520398113
38. Haruwaka K, Ikegami A, Tachibana Y, Ohno N, Konishi H, Hashimoto A, et al. Dual microglia effects on blood brain barrier permeability induced by systemic inflammation. Nat Commun (2019) 10(1):5816. doi: 10.1038/s41467-019-13812-z
39. Ronaldson PT, Davis TP. Regulation of blood-brain barrier integrity by microglia in health and disease: A therapeutic opportunity. J Cereb Blood Flow Metab (2020) 40(1_suppl):S6-S24. doi: 10.1177/0271678X20951995
40. Wu S-Y, Watabe K. The roles of microglia/macrophages in tumor progression of brain cancer and metastatic disease. Front Biosci (Landmark Ed) (2017) 22(10):1805–29. doi: 10.2741/4573
41. Russo FB, Freitas BC, Pignatari GC, Fernandes IR, Sebat J, Muotri AR, et al. Modeling the interplay between neurons and astrocytes in autism using human induced pluripotent stem cells. Biol Psychiatry (2018) 83(7):569–78. doi: 10.1016/j.biopsych.2017.09.021
42. Farmer WT, Murai K. Resolving astrocyte heterogeneity in the CNS. Front Cell Neurosci (2017) 11:300. doi: 10.3389/fncel.2017.00300
43. Khakh BS, Deneen B. The emerging nature of astrocyte diversity. Annu Rev Neurosci (2019) 42:187–207. doi: 10.1146/annurev-neuro-070918-050443
44. Brenner M, Kisseberth WC, Su Y, Besnard F, Messing A. GFAP promoter directs astrocyte-specific expression in transgenic mice. J Neurosci (1994) 14(3 Pt 1):1030–7. doi: 10.1523/JNEUROSCI.14-03-01030.1994
45. Escartin C, Galea E, Lakatos A, O'Callaghan JP, Petzold GC, Serrano-Pozo A, et al. Reactive astrocyte nomenclature, definitions, and future directions. Nat Neurosci (2021) 24(3):312–25. doi: 10.1038/s41593-020-00783-4
46. Valiente M, Obenauf AC, Jin X, Chen Q, Zhang XHF, Lee DJ, et al. Serpins promote cancer cell survival and vascular co-option in brain metastasis. Cell (2014) 156(5):1002–16. doi: 10.1016/j.cell.2014.01.040
47. Seike T, Fujita K, Yamakawa Y, Kido MA, Takiguchi S, Teramoto N, et al. Interaction between lung cancer cells and astrocytes via specific inflammatory cytokines in the microenvironment of brain metastasis. Clin Exp Metastasis (2011) 28(1):13–25. doi: 10.1007/s10585-010-9354-8
48. Klein A, Schwartz H, Sagi-Assif O, Meshel T, Izraely S, Ben Menachem S, et al. Astrocytes facilitate melanoma brain metastasis via secretion of IL-23. J Pathol (2015) 236(1):116–27. doi: 10.1002/path.4509
49. Zou Y, Watters A, Cheng N, Perry CE, Xu K, Alicea GM, et al. Polyunsaturated fatty acids from astrocytes activate PPARγ signaling in cancer cells to promote brain metastasis. Cancer Discovery (2019) 9(12):1720–35. doi: 10.1158/2159-8290.CD-19-0270
50. Priego N, Zhu L, Monteiro C, Mulders M, Wasilewski D, Bindeman W, et al. STAT3 labels a subpopulation of reactive astrocytes required for brain metastasis. Nat Med (2018) 24(7):1024–35. doi: 10.1038/s41591-018-0044-4
51. Giaume C, Koulakoff A, Roux L, Holcman D, Rouach N. Astroglial networks: a step further in neuroglial and gliovascular interactions. Nat Rev Neurosci (2010) 11(2):87–99. doi: 10.1038/nrn2757
52. Roderick HL, Cook SJ. Ca2+ signalling checkpoints in cancer: remodelling Ca2+ for cancer cell proliferation and survival. Nat Rev Cancer (2008) 8(5):361–75. doi: 10.1038/nrc2374
53. Lin Q, Balasubramanian K, Fan D, Kim S-J, Guo L, Wang H, et al. Reactive astrocytes protect melanoma cells from chemotherapy by sequestering intracellular calcium through gap junction communication channels. Neoplasia (2010) 12(9):748–54. doi: 10.1593/neo.10602
54. Chen Q, Boire A, Jin X, Valiente M, Er EE, Lopez-Soto A, et al. Carcinoma-astrocyte gap junctions promote brain metastasis by cGAMP transfer. Nature (2016) 533(7604):493–8. doi: 10.1038/nature18268
55. Sidiropoulou K, Pissadaki EK, Poirazi P. Inside the brain of a neuron. EMBO Rep (2006) 7(9):886–92. doi: 10.1038/sj.embor.7400789
56. Pfisterer U, Khodosevich K. Neuronal survival in the brain: neuron type-specific mechanisms. Cell Death Dis (2017) 8(3):e2643. doi: 10.1038/cddis.2017.64
57. Rizzolatti G, Craighero L. The mirror-neuron system. Annu Rev Neurosci (2004) 27:169–92. doi: 10.1146/annurev.neuro.27.070203.144230
58. Gallese V, Keysers C, Rizzolatti G. A unifying view of the basis of social cognition. Trends Cognit Sci (2004) 8(9):396–403. doi: 10.1016/j.tics.2004.07.002
59. Rizzolatti G. The mirror neuron system and its function in humans. Anat Embryol (Berl) (2005) 210(5-6):419–21. doi: 10.1007/s00429-005-0039-z
60. Lovinger DM. Communication networks in the brain: neurons, receptors, neurotransmitters, and alcohol. Alcohol Res Health (2008) 31(3):196–214.
61. Venkatesh H, Monje M. Neuronal activity in ontogeny and oncology. Trends Cancer (2017) 3(2):89-112. doi: 10.1016/j.trecan.2016.12.008
62. Schulz M, Salamero-Boix A, Niesel K, Alekseeva T, Sevenich L. Microenvironmental regulation of tumor progression and therapeutic response in brain metastasis. Front Immunol (2019) 10:1713. doi: 10.3389/fimmu.2019.01713
63. Deshpande K, Martirosian V, Nakamura BN, Iyer M, Julian A, Eisenbarth R, et al. Neuronal exposure induces neurotransmitter signaling and synaptic mediators in tumors early in brain metastasis. Neuro Oncol (2021) 24(6):914–24. doi: 10.1093/neuonc/noab290
64. Cordero A, Ramsey MD, Kanojia D, Fares J, Petrosyan E, Schwartz CW, et al. Combination of tucatinib and neural stem cells secreting anti-HER2 antibody prolongs survival of mice with metastatic brain cancer. Proc Natl Acad Sci U.S.A. (2022) 119(1):e2112491119. doi: 10.1073/pnas.2112491119
65. Neman J, Termini J, Wilczynski S, Vaidehi N, Choy C, Kowolik CM, et al. Human breast cancer metastases to the brain display GABAergic properties in the neural niche. Proc Natl Acad Sci U.S.A. (2014) 111(3):984–9. doi: 10.1073/pnas.1322098111
66. Zeng Q, Michael IP, Zhang P, Saghafinia S, Knott G, Jiao W, et al. Synaptic proximity enables NMDAR signalling to promote brain metastasis. Nature (2019) 573(7775):526–31. doi: 10.1038/s41586-019-1576-6
67. Venkataramani V, Tanev DI, Strahle C, Studier-Fischer A, Fankhauser L, Kessler T, et al. Glutamatergic synaptic input to glioma cells drives brain tumour progression. Nature (2019) 573(7775):532–8. doi: 10.1038/s41586-019-1564-x
68. Cronkite DA, Strutt TM. The regulation of inflammation by innate and adaptive lymphocytes. J Immunol Res (2018) 2018:1467538. doi: 10.1155/2018/1467538
69. Obermeier B, Daneman R, Ransohoff RM. Development, maintenance and disruption of the blood-brain barrier. Nat Med (2013) 19(12):1584–96. doi: 10.1038/nm.3407
70. Galea I. The blood-brain barrier in systemic infection and inflammation. Cell Mol Immunol (2021) 18(11):2489–501. doi: 10.1038/s41423-021-00757-x
71. Arvanitis CD, Ferraro GB, Jain RK. The blood-brain barrier and blood-tumour barrier in brain tumours and metastases. Nat Rev Cancer (2020) 20(1):26–41. doi: 10.1038/s41568-019-0205-x
72. Quail DF, Joyce JA. The microenvironmental landscape of brain tumors. Cancer Cell (2017) 31(3):326–41. doi: 10.1016/j.ccell.2017.02.009
73. El Rassy E, Botticella A, Kattan J, Le Péchoux C, Besse B, Hendriks L. Non-small cell lung cancer brain metastases and the immune system: From brain metastases development to treatment. Cancer Treat Rev (2018) 68:69–79. doi: 10.1016/j.ctrv.2018.05.015
74. Berghoff AS, Fuchs E, Ricken G, Mlecnik B, Bindea G, Spanberger T, et al. Density of tumor-infiltrating lymphocytes correlates with extent of brain edema and overall survival time in patients with brain metastases. Oncoimmunology (2016) 5(1):e1057388. doi: 10.1080/2162402X.2015.1057388
75. Lu BY, Gupta R, Aguirre-Ducler A, Gianino N, Wyatt H, Ribeiro M, et al. Spatially resolved analysis of the T cell immune contexture in lung cancer-associated brain metastases. J Immunother Cancer (2021) 9(10):e002684. doi: 10.1136/jitc-2021-002684
76. Reck M, Rodríguez-Abreu D, Robinson AG, Hui R, Csőszi T, Fülöp A, et al. Five-year outcomes with pembrolizumab versus chemotherapy for metastatic non-Small-Cell lung cancer with PD-L1 tumor proportion score ≥ 50. J Clin Oncol (2021) 39(21):2339–49. doi: 10.1200/JCO.21.00174
77. Mansfield AS, Herbst RS, de Castro G, Hui R, Peled N, Kim D-W, et al. Outcomes with pembrolizumab monotherapy in patients with programmed death-ligand 1-positive NSCLC with brain metastases: Pooled analysis of KEYNOTE-001, 010, 024, and 042 [J]. JTO Clin Res Rep (2021) 2(8):100205. doi: 10.1016/j.jtocrr.2021.100205
78. Goldberg SB, Schalper KA, Gettinger SN, Mahajan A, Herbst RS, Chiang AC, et al. Pembrolizumab for management of patients with NSCLC and brain metastases: long-term results and biomarker analysis from a non-randomised, open-label, phase 2 trial. Lancet Oncol (2020) 21(5):655–63. doi: 10.1016/S1470-2045(20)30111-X
79. Wu Y-L, Lu S, Cheng Y, Zhou C, Wang J, Mok T, et al. Nivolumab versus docetaxel in a predominantly Chinese patient population with previously treated advanced NSCLC: CheckMate 078 randomized phase III clinical trial. J Thorac Oncol (2019) 14(5):867–75. doi: 10.1016/j.jtho.2019.01.006
80. Brahmer J, Reckamp KL, Baas P, Crinò L, Eberhardt WEE, Poddubskaya E, et al. Nivolumab versus docetaxel in advanced squamous-cell non-Small-Cell lung cancer. N Engl J Med (2015) 373(2):123–35. doi: 10.1056/NEJMoa1504627
81. Borghaei H, Paz-Ares L, Horn L, Spigel DR, Steins M, Ready NE, et al. Nivolumab versus docetaxel in advanced nonsquamous non-Small-Cell lung cancer. N Engl J Med (2015) 373(17):1627–39. doi: 10.1056/NEJMoa1507643
82. Goldman JW, Crino L, Vokes EE, Holgado E, Reckamp K, Pluzanski A, et al. P2.36: Nivolumab (nivo) in patients (pts) With Advanced (adv) NSCLC and Central Nervous system (CNS) metastases (mets): Track: Immunotherapy. J Thorac Oncol (2016) 11(10):S238–9. doi: 10.1016/j.jtho.2016.08.107
83. Sezer A, Kilickap S, Gümüş M, Bondarenko I, Özgüroğlu M, Gogishvili M, et al. Cemiplimab monotherapy for first-line treatment of advanced non-small-cell lung cancer with PD-L1 of at least 50%: a multicentre, open-label, global, phase 3, randomised, controlled trial. Lancet (2021) 397(10274):592–604. doi: 10.1016/S0140-6736(21)00228-2
84. Rittmeyer A, Barlesi F, Waterkamp D, Park K, Ciardiello F, von Pawel J, et al. Atezolizumab versus docetaxel in patients with previously treated non-small-cell lung cancer (OAK): a phase 3, open-label, multicentre randomised controlled trial. Lancet (2017) 389(10066):255–65. doi: 10.1016/S0140-6736(16)32517-X
85. Spigel DR, Chaft JE, Gettinger S, Chao BH, Dirix L, Schmid P, et al. FIR: Efficacy, safety, and biomarker analysis of a phase II open-label study of atezolizumab in PD-L1-Selected patients with NSCLC. J Thorac Oncol (2018) 13(11):1733–42. doi: 10.1016/j.jtho.2018.05.004
86. Dudnik E, Moskovitz M, Daher S, Shamai S, Hanovich E, Grubstein A, et al. Effectiveness and safety of nivolumab in advanced non-small cell lung cancer: The real-life data. Lung Cancer (2018) 126:217–23. doi: 10.1016/j.lungcan.2017.11.015
87. Cortinovis D, Chiari R, Catino A, Grossi F, De Marinis F, Sperandi F, et al. Italian Cohort of the nivolumab EAP in squamous NSCLC: Efficacy and safety in patients with CNS metastases. Anticancer Res (2019) 39(8):4265–71. doi: 10.21873/anticanres.13590
88. Crinò L, Bronte G, Bidoli P, Cravero P, Minenza E, Cortesi E, et al. Nivolumab and brain metastases in patients with advanced non-squamous non-small cell lung cancer. Lung Cancer (2019) 129:35–40. doi: 10.1016/j.lungcan.2018.12.025
89. Hendriks LEL, Henon C, Auclin E, Mezquita L, Ferrara R, Audigier-Valette C, et al. Outcome of patients with non-small cell lung cancer and brain metastases treated with checkpoint inhibitors. J Thorac Oncol (2019) 14(7):1244–54. doi: 10.1016/j.jtho.2019.02.009
90. Reck M, Rodríguez-Abreu D, Robinson AG, Hochmair MJ, Gadgeel S, Esteban E, et al. Updated analysis of KEYNOTE-024: Pembrolizumab versus platinum-based chemotherapy for advanced non-Small-Cell lung cancer with PD-L1 tumor proportion score of 50% or greater. J Clin Oncol (2019) 37(7):537–46. doi: 10.1016/j.jtho.2021.01.141
91. Rizvi NA, Mazières J, Planchard D, Esteban E, Felip E, Dómine M, et al. Activity and safety of nivolumab, an anti-PD-1 immune checkpoint inhibitor, for patients with advanced, refractory squamous non-small-cell lung cancer (CheckMate 063): a phase 2, single-arm trial. Lancet Oncol (2015) 16(3):257–65. doi: 10.1016/S1470-2045(15)70054-9
92. Borghaei H, Gettinger S, Vokes EE, Tafreshi A, Gümüş M, Mazières J, et al. Five-year outcomes from the randomized, phase III trials CheckMate 017 and 057: Nivolumab versus docetaxel in previously treated non-Small-Cell lung cancer. J Clin Oncol (2021) 39(7):723–33. doi: 10.1200/JCO.20.01605
93. Gray J, Rodríguez-Abreu D, Powell SF, Tafreshi A, Paz-Ares L, Kopp H-G, et al. FP13.02 pembrolizumab + pemetrexed-platinum vs pemetrexed-platinum for metastatic NSCLC: 4-year follow-up from KEYNOTE-189. J Thorac Oncol (2021) 16(3):S224. doi: 10.1016/j.jtho.2021.06.020
94. Garassino MC, Gadgeel S, Esteban E, Zhou J, Lin L, Feng J, et al. Abstract CT043: Outcomes among patients (pts) with metastatic nonsquamous NSCLC with liver metastases or brain metastases treated with pembrolizumab (pembro) plus pemetrexed-platinum: Results from the KEYNOTE-189 study. Cancer Res (2019) 79(13_Supplement):CT043–3. doi: 10.1158/1538-7445.AM2019-CT043
95. Powell SF, Rodríguez-Abreu D, Langer CJ, Fang J, Yu Q, Han B, et al. Outcomes with pembrolizumab plus platinum-based chemotherapy for patients with NSCLC and stable brain metastases: Pooled analysis of KEYNOTE-021, -189, and -407. J Thorac Oncol (2021) 16(11):1883–92. doi: 10.1016/j.jtho.2021.06.020
96. Zhou C, Chen G, Huang Y, Jiang T, Cheng Y, Chen G, et al. Camrelizumab plus carboplatin and pemetrexed versus chemotherapy alone in chemotherapy-naive patients with advanced non-squamous non-small-cell lung cancer (CameL): a randomised, open-label, multicentre, phase 3 trial. Lancet Respir Med (2021) 9(3):305–14. doi: 10.1016/S2213-2600(20)30365-9
97. Yang Y, Sun J, Wang Z, Wang Z, Liu L, Chen G, et al. Updated overall survival data and predictive biomarkers of sintilimab plus pemetrexed and platinum as first-line treatment for locally advanced or metastatic nonsquamous NSCLC in the phase 3 ORIENT-11 study. J Thorac Oncol (2021) 16(12):2109–20. doi: 10.1016/j.jtho.2021.07.015
98. Yang Y, Wang Z, Fang J, Zhao Y, Zhou J, Fan Y, et al. Efficacy and safety of sintilimab plus pemetrexed and platinum as first-line treatment for locally advanced or metastatic nonsquamous NSCLC: a randomized, double-blind, phase 3 study (Oncology pRogram by InnovENT anti-PD-1-11). J Thorac Oncol (2020) 15(10):1636–46. doi: 10.1016/j.jtho.2020.07.014
99. Jiang T, Wang P, Zhang J, Castro RL, Estival A, Mosquera J, et al. Toripalimab plus chemotherapy as second-line treatment in previously EGFR-TKI treated patients with EGFR-mutant-advanced NSCLC: a multicenter phase-II trial. Signal Transduct Target Ther (2021) 6(1):355. doi: 10.1038/s41392-021-00751-9
100. Nadal E, Massuti B, Huidobro G, Panet-Raymond V, Pavic M, Owen SP, et al. OA09.02 atezo-brain: Single arm phase II study of atezolizumab plus chemotherapy in stage IV NSCLC with untreated brain metastases. J Thorac Oncol (2021) 16(10):S863. doi: 10.1200/JCO.2021.39.15_suppl.2023
101. Wong P, Florescu M, Plourde M-E, Chiang VL. A phase II trial combining nivolumab and stereotactic brain radiosurgery for treatment of brain metastases in patients with NSCLC. J Clin Oncol (2021) 39(15_suppl):2023–3. doi: 10.1200/JCO.2021.39.15_suppl.2023
102. Singh C, Qian JM, Yu JB, Giraffa M, Russo I, Cicone F, et al. Local tumor response and survival outcomes after combined stereotactic radiosurgery and immunotherapy in non-small cell lung cancer with brain metastases. J Neurosurg (2019) 132(2):512–7. doi: 10.1136/jitc-2021-003730
103. Minniti G, Lanzetta G, Capone L, Osti MF, Di Franco R, Caini S, et al. Leptomeningeal disease and brain control after postoperative stereotactic radiosurgery with or without immunotherapy for resected brain metastases. J Immunother Cancer (2021) 9(12):1750–64. doi: 10.1136/jitc-2021-003730
104. Scoccianti S, Olmetto E, Pinzi V, Yadav P, Leal T, Baschnagel AM, et al. Immunotherapy in association with stereotactic radiotherapy for non-small cell lung cancer brain metastases: results from a multicentric retrospective study on behalf of AIRO. Neuro Oncol (2021) 23(10):1750–64. doi: 10.1093/neuonc/noab129
105. Enright TL, Witt JS, Burr AR, Provencio M, Burgers S, Carcereny E, et al. Combined immunotherapy and stereotactic radiotherapy improves neurologic outcomes in patients with non-small-cell lung cancer brain metastases. Clin Lung Cancer (2021) 22(2):110–9. doi: 10.1016/j.cllc.2020.10.014
106. Borghaei H, Pluzanski A, Caro RB, Schenker M, Zurawski B, Menezes J, et al. Abstract CT221: Nivolumab (NIVO) + ipilimumab (IPI) as first-line (1L) treatment for patients with advanced non-small cell lung cancer (NSCLC) with brain metastases: Results from CheckMate 227. Cancer Res (2020) 80(16_Supplement):CT221–1. doi: 10.1158/1538-7445.AM2020-CT221
107. Paz-Ares LG, Ramalingam SS, Ciuleanu T-E, Schenker M, Zurawski B, Menezes J, et al. First-line nivolumab plus ipilimumab in advanced NSCLC: 4-year outcomes from the randomized, open-label, phase 3 CheckMate 227 part 1 trial. J Thorac Oncol (2022) 17(2):289–308. doi: 10.1016/j.jtho.2021.09.010
108. Paz-Ares L, Ciuleanu T-E, Cobo M, Zhang B, Zhang W, Shi C, et al. First-line nivolumab plus ipilimumab combined with two cycles of chemotherapy in patients with non-small-cell lung cancer (CheckMate 9LA): an international, randomised, open-label, phase 3 trial [J]. Lancet Oncol (2021) 22(2):198–211. doi: 10.1016/S1470-2045(20)30641-0
109. Carbone D, Ciuleanu T, Cobo M, Kaneko T, Cecchini M, Han VK, et al. OA09.01 first-line nivolumab + ipilimumab + chemo in patients with advanced NSCLC and brain metastases: Results from CheckMate 9LA. J Thorac Oncol (2021) 16(10):S862. doi: 10.1038/s41467-022-33365-y
110. Chu T, Zhong R, Zhong H, Ding S, Yang Y, Liao J, et al. Phase 1b study of sintilimab plus anlotinib as first-line therapy in patients with advanced NSCLC. J Thorac Oncol (2021) 16(4):643–52. doi: 10.1016/j.jtho.2020.11.026
111. Gadgeel S, Rodríguez-Abreu D, Speranza G, Hui R, Csőszi T, Fülöp A, et al. Updated analysis from KEYNOTE-189: Pembrolizumab or placebo plus pemetrexed and platinum for previously untreated metastatic nonsquamous non-Small-Cell lung cancer. J Clin Oncol (2020) 38(14):1505–17. doi: 10.1200/JCO.19.03136
112. Paz-Ares L, Luft A, Vicente D, Stinchcombe TE, Dy GK, Antonia SJ, et al. Pembrolizumab plus chemotherapy for squamous non-Small-Cell lung cancer. N Engl J Med (2018) 379(21):2040–51. doi: 10.1056/NEJMoa1810865
113. Ren S, Chen J, Xu X, Chow LQM, Burgio MA, de Castro Carpeno J, et al. Camrelizumab plus carboplatin and paclitaxel as first-line treatment for advanced squamous NSCLC (CameL-sq): A phase 3 trial. J Thorac Oncol (2022) 17(4):544–57. doi: 10.1016/j.jtho.2021.11.018
114. Zhou C, Wu L, Fan Y, Felip E, Speranza G, Angelis FD, et al. Sintilimab plus platinum and gemcitabine as first-line treatment for advanced or metastatic squamous NSCLC: Results from a randomized, double-blind, phase 3 trial (ORIENT-12). J Thorac Oncol (2021) 16(9):1501–11. doi: 10.1016/j.jtho.2021.04.011
115. Zhang Q, Abdo R, Iosef C, Yu Q, Han B, Cang S, et al. The spatial transcriptomic landscape of non-small cell lung cancer brain metastasis. Nat Commun (2022) 13(1):5983. doi: 10.1038/s41467-022-33365-y
Keywords: NSCLC, brain metastasis, immunotherapy, organ-specific, time
Citation: Xie M and Su C (2023) Microenvironment and the progress of immunotherapy in clinical practice of NSCLC brain metastasis. Front. Oncol. 12:1006284. doi: 10.3389/fonc.2022.1006284
Received: 29 July 2022; Accepted: 28 December 2022;
Published: 24 January 2023.
Edited by:
Lydia Meder, University of Cologne, GermanyReviewed by:
Yang YiJian, Xiangya Hospital, Central South University, ChinaFred Lam, Saint Elizabeth’s Medical Center, United States
Copyright © 2023 Xie and Su. This is an open-access article distributed under the terms of the Creative Commons Attribution License (CC BY). The use, distribution or reproduction in other forums is permitted, provided the original author(s) and the copyright owner(s) are credited and that the original publication in this journal is cited, in accordance with accepted academic practice. No use, distribution or reproduction is permitted which does not comply with these terms.
*Correspondence: Chunxia Su, c3VzdV9tYWlsQDEyNi5jb20=