- 1Division of Radiotherapy and Imaging, Institute of Cancer Research, London, United Kingdom
- 2Head and Neck Unit, Royal Marsden National Health Service (NHS) Hospital Trust, London, United Kingdom
- 3Department of Radiotherapy, Royal Marsden National Health Service (NHS) Hospital Trust, London, United Kingdom
Cancer-associated fibroblasts (CAFs) play a fundamental role in the development of cancers and their response to therapy. In recent years, CAFs have returned to the spotlight as researchers work to unpick the mechanisms by which they impact tumour evolution and therapy responses. However, study of CAFs has largely been restricted to a select number of common cancers, whereas research into CAF biology in bladder cancer has been relatively neglected. In this review, we explore the basics of CAF biology including the numerous potential cellular origins of CAFs, alongside mechanisms of CAF activation and their diverse functionality. We find CAFs play an important role in the progression of bladder cancer with significant implications on tumour cell signaling, epithelial to mesenchymal transition and the capacity to modify components of the immune system. In addition, we highlight some of the landmark papers describing CAF heterogeneity and find trends in the literature to suggest that the iCAF and myCAF subtypes defined in bladder cancer share common characteristics with CAF subtypes described in other settings such as breast and pancreatic cancer. Moreover, based on findings in other common cancers we identify key therapeutic challenges associated with CAFs, such as the lack of specific CAF markers, the paucity of research into bladder-specific CAFs and their relationship with therapies such as radiotherapy. Of relevance, we describe a variety of strategies used to target CAFs in several common cancers, paying particular attention to TGFβ signaling as a prominent regulator of CAF activation. In doing so, we find parallels with bladder cancer that suggest CAF targeting may advance therapeutic options in this setting and improve the current poor survival outcomes in bladder cancer which sadly remain largely unchanged over recent decades.
1 Introduction
1.1 Brief overview of bladder cancer
Approximately 20,000 people are diagnosed with bladder cancer each year in the UK (1). Typically, patients are aged 75 years or greater at diagnosis and are predominantly male. However, bladder cancer affects all genders and also occurs in younger patients, and the most clearly established risk factor for bladder cancer is smoking (2). In the past 30 to 40 years, there have been minimal advances in the survival outcomes of patients diagnosed with bladder cancer. Indeed, the 5-year survival following radical cystectomy or chemo-radiation remains at approximately 50% (3). Therefore, there is an urgent need to better understand bladder cancer biology to help find more effective curative treatments.
The most common form of bladder cancer arises from cells in the bladder lining, or urothelium, and is consequently known as urothelial bladder carcinoma. The anatomy of the bladder and stages of bladder cancer, defined according to how far the cancer cells have invaded into the bladder wall, are shown in Figure 1. Early stage, non-muscle-invasive bladder cancers (NMIBC) reside within the bladder lining and are risk-stratified depending on the tumour grade, a measure of tumour cell growth rate. In contrast, muscle-invasive bladder cancer (MIBC) describes tumours that have grown into the muscle wall of the bladder, referred to as the muscularis propria.
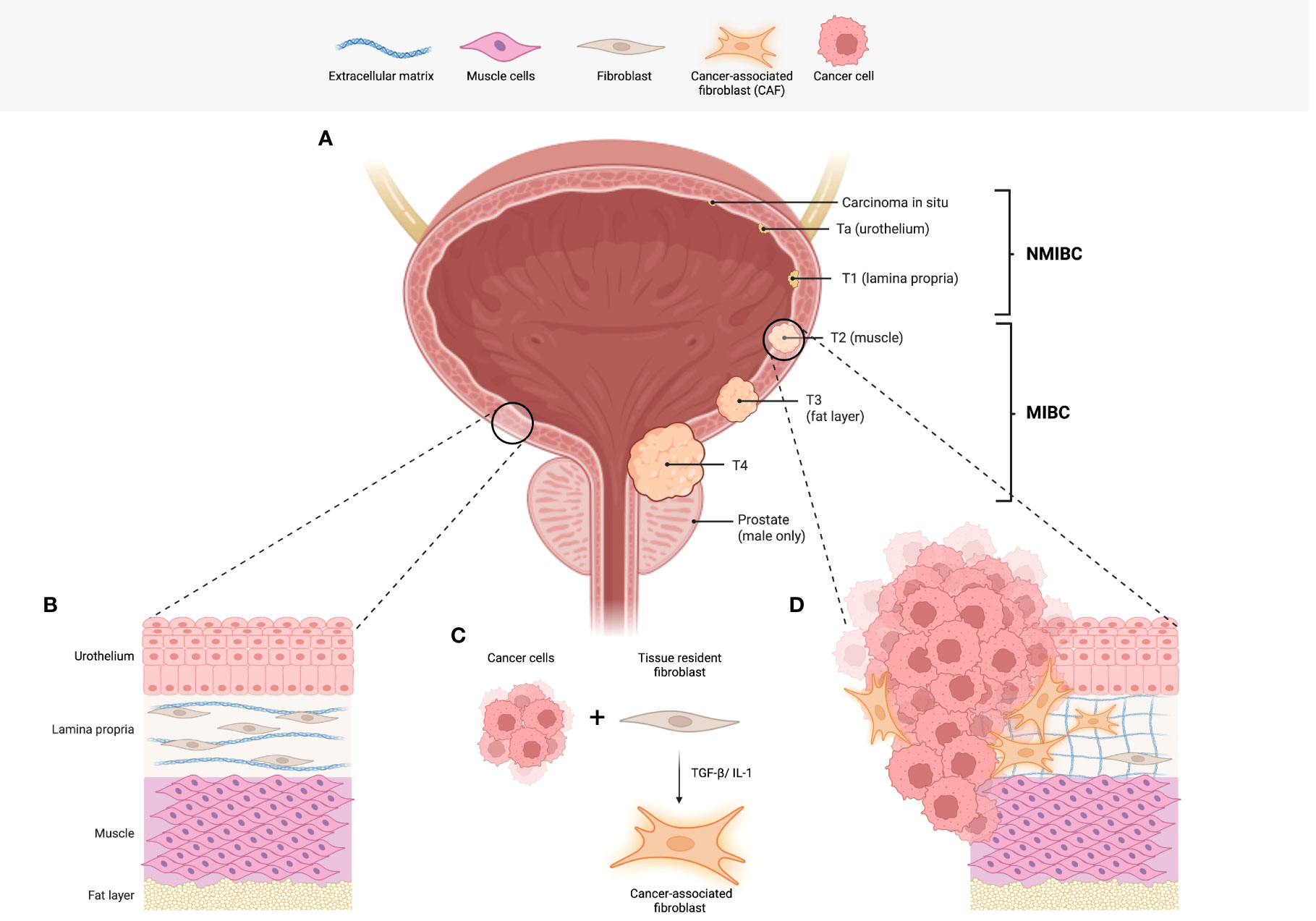
Figure 1 Bladder cancer staging and the role of fibroblasts. (A) An illustration of the human bladder, including the tumour (T stages) of bladder cancer. Commencing in the urothelium, NMIBCs describe carcinoma in situ (in the inner most layer of the urothelium) Ta (urothelium only) and T1 tumours. T1 tumours have infiltrated the first sub-urothelial layer known as the lamina propria. To progress to stages T2-4, invasion into the muscle layer is required and is the determining factor in the diagnosis of MIBC. (B) A representation of the physiological histology observed in the human bladder. Of note, fibroblasts found in the lamina propria function to produce and maintain collagen and other fibres that make up the loose connective tissue. (C) Interactions between tumour cells and tissue resident fibroblasts are one of the likely origins of cancer-associated fibroblasts (CAFs). (D) The illustration depicts the histological changes observed in bladder cancer including the presence of CAFs. In addition, there is an increase in extracellular matrix deposition and remodelling. Abbreviations: non-muscle invasive bladder cancer (NMIBC), muscle invasive bladder cancer (MIBC), cancer-associated fibroblasts (CAFs). Figure created with Biorender.com.
Comprehensive analysis of gene expression data resulted in the molecular classification of MIBC and the identification of molecular subtypes by several research groups (4–9). In a recent consensus classification, six subtypes have been defined: luminal papillary, luminal non-specified, luminal unstable, stroma-rich, basal/squamous, and neuroendocrine-like (10). The molecular classification of MIBC has helped to increase knowledge of bladder cancer and find potential associations between subtypes, clinical characteristics, response to therapies, and survival. While the neuroendocrine-like subtype is associated with the worst prognosis, it is interesting to note that the stroma-rich subtype appears to have a particularly poor response to neo-adjuvant chemotherapy (10). Moreover, the stroma-rich subtype has an over-expression of gene signatures associated with smooth muscle, endothelial cells, fibroblasts and myofibroblasts (10).
1.2 Treatment of NMIBC vs MIBC
Currently, all patients with bladder cancer undergo a biopsy-like procedure known as a transurethral resection of bladder tumour (TURBT) which acts as both a diagnostic tool to clarify the presence or absence of tumour cell invasion into muscle and a debulking procedure to remove as much of the visible tumour mass as possible. In patients with high grade NMIBC, immunotherapeutic agents such as Bacillus Calmette–Guérin (BCG) are commonly used after TURBT; BCG works to stimulate an anti-tumour immune response to tackle residual cancer cells. Intriguingly, BCG remains one of the longest-established immunotherapies ever used in cancer treatment.
If muscle invasion is identified during the pathological assessment of the TURBT tissue, MIBC patients are treated surgically with a cystectomy or bladder-sparing treatments (BST) such as radiotherapy. Following the improved locoregional control seen with the addition of concomitant 5-fluorouracil and mitomycin C to radiotherapy in the phase III UK BC2001 trial, led by James et al., provision of BST typically includes radiotherapy in combination with chemotherapy (11). This combinatorial approach can offer quality of life benefits in selected patients and can also be a useful option for patients who are unable to undergo cystectomy (11, 12). Of relevance, following BST, patients will require lifelong cystoscopic surveillance due to the risk of recurrence (13), alongside the cross-sectional imaging and clinical surveillance carried out following treatment in all patients.
1.3 Research challenges and scope of this review
At present, we lack predictive biomarkers to identify which patients may be more likely to relapse following BST and could therefore be better surgical candidates or require treatment intensification. A strong predictive biomarker is therefore a key unmet clinical need to aid radical treatment decisions.
Recently, stromal cell populations in the tumour microenvironment of solid tumours, known as cancer-associated fibroblasts (CAFs), have gained prominence as an important cell type influencing bladder cancer survival outcomes.
In metastatic bladder cancer, poor responses to immune checkpoint inhibitors have been associated with TGFβ signaling in fibroblasts (14) and high EMT/stromal related gene expression (15). Moreover, in cases with poor responses, CD8 T-cells are frequently found trapped in the peri-tumoural regions amongst fibroblasts and collagen-rich matrix (14). High EMT/stromal gene expression and low tumour-infiltrating T cell abundance have both also been associated with inferior outcomes following cystectomy (15). Unfortunately, research into the effect of CAFs on radiotherapy responses, and, similarly, the impact of radiotherapy on CAF biology, is in its infancy and warrants further investigation in the setting of bladder cancer.
In this review we will outline the latest consensus views on CAF biology with a specific focus on bladder cancer. We will introduce some of the current controversies regarding CAF heterogeneity and disease-specific CAF subtypes. This review will discuss the gaps in our current understanding of the biological relevance of CAFs in bladder cancer – here, we will draw from studies of CAFs in other cancers to explore longitudinal changes in CAF biology during therapy. Finally, we will discuss whether the addition of therapeutic approaches to specifically target CAFs may improve survival in bladder cancer.
2 The tumour microenvironment and the role of CAFs
The tumour microenvironment (TME) describes the ecosystem formed by many different cell populations coexisting within the tumour. Within the TME, CAFs help to create the structural framework, including the extracellular matrix (ECM), within which all other cells in the TME reside. Such cells include, but are not limited to, cancer cells, cytotoxic and regulatory immune cells, antigen-presenting cells and cells of the vasculature including endothelial cells.
2.1 Origins and activation of CAFs
2.1.1 Origins
CAFs are often classified as non-neoplastic, not epithelial, endothelial, or immune cells. As opposed to normal fibroblasts that can be temporarily activated, CAFs are persistently activated, usually via epigenetic reprogramming (16).
CAFs are derived from several potential sources: the most commonly cited and likely origin is from normal fibroblasts resident in the tissue of tumour origin that have undergone activation via processes outlined below (17) (see Figure 1); secondly, CAFs may be mesenchymal stem cells derived from the bone marrow (18). Some anecdotal studies suggest that CAFs can arise from cells of the vasculature such as endothelial cells, as well as pericytes and adipocytes (19). Furthermore, the specific cell of origin may be indicative of the CAFs eventual function and role in the TME (20). A fascinating recent study utilised single cell RNA sequencing (scRNAseq) data atlases for healthy and disease state tissues of human and mouse derivation to trace the lineage of fibroblasts (21). In doing so, they suggest that all fibroblasts, whether healthy, tissue-specific, or activated in disease, may all share a common ancestor. From such an ancestor, tissue-specific functions are subsequently acquired in situ, typically via changes to their epigenetic and transcriptional profile (21).
2.1.2 Activation
In addition to their cellular origin, the mechanism by which CAFs are activated seemingly imparts an additional layer of information that can provide functional and spatial stratification of CAFs. Activation of CAFs by cancer cells has been explored in several studies. For example, using in vitro and in vivo experiments, Strell et al. showed direct cell to cell contact between ductal breast carcinoma in situ (DCIS) cancer cells and fibroblasts induces changes in the expression profile of fibroblasts, eventually resulting in a platelet-derived growth factor (PDGF) receptor (PDGFR)α low, PDGFRβ high subset of CAFs (22). Interestingly, assessment of PDGFRα and PDGFRβ expression in tissue specimens from 458 primary DCIS patients showed an increased proportion of the PDGFRα low PDGFRβ high CAFs was associated with a higher risk of recurrence (22).
In addition to the direct cell contact driving activation of CAFs described above, paracrine crosstalk between cancer cells and fibroblasts can also lead to acquisition of the CAF phenotype. In vitro studies of bladder cancer cell lines revealed a high content of interleukin (IL)-1α in tumour conditioned media (CM) (23). When fibroblasts were cultured in IL-1α rich CM, this led to the release of cytokines and pro-tumour factors such as IL-8, granulocyte-macrophage colony-stimulating factor (GM-CSF), monocyte chemoattractant protein-1 (MCP-1) and hepatocyte growth factor (HGF), i.e. acquisition of an “activated CAF phenotype” which subsequently promoted cancer cell migration in Boyden chamber assays (23).
In a further study, Öhlund et al. demonstrated that both cell to cell contact and paracrine crosstalk between cancer cells and CAFs occur in distinct regions of pancreatic tumours with differing results (24). Contact between fibroblast activation protein (FAP)+ pancreatic stellate cells (PSC) and pancreatic cancer cells resulted in activation and conversion of PSC into CAFs, represented by an increase in expression of alpha smooth muscle actin (αSMA). These CAFs were subsequently classified as myofibroblastic CAFs (myCAFs) with the ability to deposit components of the ECM. In contrast, PSCs with less proximity to cancer cells were activated in a paracrine manner resulting in a reduced expression of αSMA and an increase in the secretion of IL-6; these CAFs were designated as inflammatory CAFs (iCAFs). Under differing conditions, CAFs appear to have the capacity to be directed into either myCAFs or iCAFs by modifying their expression profiles, suggesting that different, spatially distinct CAF subtypes exist, with functional characteristics dependent on activation (24). Of further interest, using scRNAseq, the same group identified an additional CD74+ MHC class II+ CAF subtype, denoted as antigen-presenting CAFs (apCAF) (17).
Studying single cell sequencing outputs of fibroblasts extracted from normal and pancreatic ductal adenocarcinoma (PDAC) mouse models, Dominguez et al. developed an evolutionary model to characterise CAF heterogeneity (25). Using dimensionality reduction and pseudotime analysis, they demonstrated that the previously described iCAF and myCAF populations were derived from a common early CAF subtype (25). This supported earlier work that showed mechanistically how signalling via IL-1 and transforming growth factor beta (TGFβ) drives the differentiation of iCAFs and myCAFs, respectively, from a common “early CAF” ancestor (26). The diversity of CAF subtypes is of considerable biological importance, and we will return to this concept in greater detail below.
2.2 Functions of CAFs
Pathologically, CAFs exhibit a wide range of functions that can modify the TME via diverse non-immune and immune mechanisms. This section will explore the functions of CAFs with reference to both bladder cancer and wider tumour biology. To help understand pathological CAF function, we will first describe the role of physiologically normal fibroblasts in the bladder: Such fibroblasts are situated in the sub-urothelial lamina propria layer of the bladder where their function includes the production of extracellular matrix components such as collagen and fibronectin (27). Uniquely, in the bladder, myofibroblasts help to transmit signals from the urothelium in response to bladder filling or pathological stressors (28). As discussed earlier, these normal fibroblasts are likely to be an important source of persistently activated CAFs with functions as outlined below:
2.2.1 Production and remodeling of the ECM
One of the key functions of CAFs is to deposit and modify the filamentous ECM which facilitates tumour cell invasion (29). This has several biomechanical consequences including the generation of greater contractile strength and tension in the tissue and increased tumour stiffness. Such increased stiffness can result in further activation of CAFs, thus creating a self-perpetuating positive feedback loop. This loop is thought to arise following the activation of transcription factor Yes-associated protein (YAP) which subsequently regulates the downstream expression of genes associated with cytoskeletal and matrix remodeling (29). The increased stiffness can also impact oncogenic signalling within the tumour cell, including via the mitogen-activated protein kinase (MAPK) pathway (30).
2.2.2 Impact on tumour cell signalling
CAFs have been shown to directly impact the proliferation and invasiveness of bladder cancer cells via several mechanisms including the exosomal transfer of non-coding RNA fragments such as LINC00355 (31). Components of the CAF secretome, such as microfibrillar-associated protein 5 (MFAP5), have a similar pro-tumour effect (32). Additionally, conducting co-culture studies of CAFs with bladder cancer cell lines showed that cancer cell proliferation and invasion was enhanced in conditions where CAFs were induced to undergo autophagy (33).
Further in vitro studies support the proposed pro-tumour impact of CAFs on bladder cancer cells. One such study showed that CAFs, but not normal fibroblasts, secrete pro-tumour cytokines such as IL-1β which activates the Wnt signalling pathway in bladder cancer cell lines. Indeed, inhibition of Wnt signalling abolished this pro-tumour effect (34). Similar effects were seen following the exosomal transfer of miR-148b-3p which induced increased invasive behaviour of bladder cancer cells via Wnt signalling, an effect which could be attenuated via the upregulation of phosphatase and tensin homolog (PTEN) (35). Overall, this suggests CAFs may impart a pro-tumour effect in bladder cancer via Wnt signalling pathway activation.
In addition to the pro-tumour signaling described above, CAFs have also been shown to contribute to metabolic reprogramming of tumours (36). As with the Warburg effect witnessed in cancer cells, CAFs are capable of upregulating components of the glycolytic pathway leading to an upregulation in lactate production (37). Moreover, exposure to CAF conditioned media has been shown to enhance the glycolytic activity of pancreatic cancer cells (38) helping to support the metabolic needs of surrounding cancer cells.
2.2.3 Induction of epithelial to mesenchymal transition
CAFs can also have a pro-tumour effect via the induction of epithelial to mesenchymal transition (EMT). Indeed, secretion of IL-6 by tumour-activated CAFs was shown to induce EMT in bladder cancer cell lines (39). Here, observations included the upregulation of proteins associated with EMT such as N-cadherin and vimentin and an increase in EMT-inducing transcription factors SNAIL, TWIST and ZEB1 in bladder cancer cell lines (39). These findings are further supported by immunohistochemistry analysis of urinary bladder carcinoma which revealed the upregulation of several CAF markers was associated with an increase in markers of EMT on multiple cell types within the TME (40).
2.2.4 Immunomodulatory functions of CAFs
CAFs have multiple immunomodulatory functions, which are generally, but not exclusively, considered to be immunosuppressive in nature. Such functions can be driven by the physical properties of CAFs for example the sequestration or trapping of immune cells in the matrix or by secretion of immunomodulatory chemokines for example TGFβ (41, 42). Obviously, both physical and secretory immunomodulatory functions may co-exist within a single tumour.
In a landmark paper published in 2018, Mariathasan et al. were one of the first authors to describe the role of CAFs in the creation of an immune excluded phenotype, and the associated lack of response to anti-programmed death-ligand 1 (PD-L1) checkpoint inhibitor, atezolizumab (14). Of note, this finding was demonstrated in the setting of metastatic urothelial bladder carcinoma and therefore highlights the fundamental role CAFs play in this disease (14). We will explore additional findings from this paper in subsequent sections. Below we have detailed additional studies which have explored the interplay between CAFs and cells of the immune system.
In bladder cancer, FAP+ CAFs have been associated with immune cold TMEs with poor infiltration of CD8+ T-cells and with considerable loss of human leukocyte antigen (HLA-I) expression on tumour cells (43). Poor CD8+ T cell infiltration is also likely to be due to the deposition of a heavily crosslinked ECM which acts as a physical barrier to exclude lymphocytes in peritumour regions, preventing them from reaching the tumours cells and unleashing their full cytotoxic potential (44).
To note, CAFs impact many immune populations other than lymphocytes. For example, in lung squamous cell carcinoma, CAFs have been associated with a higher infiltrate of immunosuppressive cells such as tumour-associated macrophages (45).
Although the immunomodulatory effects of CAFs are predominantly immunosuppressive and tumour-promoting, in melanoma a podoplanin (PDPN)+, FAP- CAF subtype has been shown to act in an immunostimulatory manner via development of tumour-associated tertiary lymphoid structures (TA-TLS). In this setting, an increase of TA-TLS was positively correlated with improved patient survival and response to immune checkpoint therapies (46). Tertiary lymphoid structures (TLS) are equally relevant in bladder cancer. In a study of immune checkpoint inhibitors targeting PD-L1 and CTLA-4, Gao et al. report the enhanced treatment response associated with a high density of TLS in the pre-treatment biopsies of patients with high grade urothelial carcinoma (47). It would be very interesting to determine the phenotype of CAFs in these patients, including the CAFs specifically present in the TLS.
Considering the diversity in the functions of CAFs described above, it is of great importance that we correctly characterise CAF subtypes and consider any implications that may follow depletion of some or all CAF populations.
2.3 CAF heterogeneity
Some markers such as FAP and αSMA are used frequently in CAF research and are often considered to be “canonical”. Using individual canonical CAF markers, many studies have attempted to identify the total CAF population leading to conflicting results - this is likely to be due to the heterogeneous nature of CAFs. Indeed, at present there is no known single marker that is capable of identifying all CAF populations or of segregating them from other stromal populations.
To explore CAF heterogeneity and characterise distinct CAF subtypes a variety of methods have been used across multiple cancer types, including scRNASeq, spatial transcriptomics, flow cytometry and immunohistochemistry. Each method offers a unique opportunity to study the underlying biology but can come with disadvantages. Briefly, bulk RNA sequencing facilitates a broad overview of gene signatures and signalling pathways that may be up or down regulated in the transcriptome but lacks the single cell resolution that is provided by scRNAseq. Protein expression can be studied at single cell resolution using flow cytometry, however spatial information is lost in all three of these methods. Techniques such as multiplex immunofluorescence and imaging mass cytometry help to overcome these challenges and provide insights into the interactions between different cell types. However, these techniques come with time and cost limitations that can restrict high throughput analysis.
Across many studies, the concept of CAF heterogeneity, i.e. different CAF subtypes in a single tumour, generally holds true and typically shows the distinction between iCAFs and myCAFs described above. However, beyond this, subtype classification, descriptions of functionality and biomarker selection are inconsistent and vary greatly depending on the method of CAF profiling, tumour sample quality and disease type. The type of biopsy used to collect tissue and thus stratify CAFs can also have implications on the interpretations of results. In bladder cancer, a TURBT may only provide access to superficial regions of tumour and thus not reveal the full extent of the CAF infiltrated tumour layers, resulting in a loss of heterogeneity. In contrast, a full cystectomy will typically provide access to the whole tumour and surrounding healthy tissue. These limitations surrounding inter and intra tumoural heterogeneity are not restricted to bladder cancer; the renal and lung TRACERx studies (48–50) have demonstrated the importance of evaluation of biopsies from multiple different sites within a tumour to comprehensively profile cancer biology, rather than a single snapshot.
To study inter-tissue CAF heterogeneity, Galbo et al. utilised scRNAseq data from melanoma, head and neck, and lung cancer to create a series of gene signatures describing pan-cancer CAF subtypes (pan-CAF) (51). In total they described 5 pan-CAFs, each with distinct gene expression patterns; myofibroblast (myCAF), desmoplastic (dCAF), inflammatory like (iCAF and iCAF-2) and proliferating (pCAF) - each named to represent their predicted function. Applying the newly defined pan-CAF gene sets to bulk RNA sequencing data across a variety of cancer types not only confirmed the presence of multiple CAF subtypes across different tumours, but survival analysis also revealed that in different settings, different pan-CAFs subtypes were predictive of poor outcomes. In bladder cancer, a high infiltrate of the myCAF signature correlated with poor prognosis in The Cancer Genome Atlas (TCGA) dataset, which consists primarily of cystectomy specimens (51).
Studying CAF heterogeneity in breast cancer, Cremasco et al. identified two distinct populations in mouse and human tumours; FAP+ PDPN+ CAFs and FAP+ PDPN- cancer-associated pericytes (CAPs) (52). They showed that FAP+ PDPN+ CAFs, but not CAPs, were capable of supressing T cell proliferation and, via deposition of ECM, trapping infiltrating immune cells in the peritumoral regions - a process which appears to be driven by TGFβ (52).
A further key study exploring CAFs subtypes in breast cancer by Costa et al. used flow cytometry with several common CAF markers to reveal the presence of four distinct CAFs (53). These subtypes were differentially expressed in luminal A, triple negative (TNBC) and human epidermal growth factor receptor 2 (HER2)+ breast cancer and showed distinct protein expression signatures and spatial patterns. Two of the identified CAFs were αSMA+ and were classified as CAF-S1 and CAF-S4; each had distinct transcriptomic profiles and pro-tumour functions. Of note, the CAF-S1 (CD29Med, FAPHi, fibroblast-specific protein 1 (FSP1)Low-Hi, αSMAHi, PDGFRβMed-Hi, caveolin 1 (CAV1)Low) subset was associated with an immunosuppressive phenotype via the recruitment of Foxp3+ CD4+ CD25+ regulatory T-cells (Tregs) and inhibition of cytotoxic CD8+ T-cells. In contrast CAF-S4 did not play a role in the immune response and instead was found to be associated with muscle contraction, regulation of the actin cytoskeleton and oxidative metabolism (53).
CAF-S1 and CAF-S4 were also identified in high grade serous ovarian cancer where the immunosuppressive function of CAF-S1 was ascribed to the expression of CXCL12β which recruits Tregs via CXCR4, this cell-cell contact subsequently enhances survival and differentiation of Tregs (54). CAF-S4 cells on the other hand, did not express CXCL12β, likely due to silencing by micro RNAs miR-141 and miR-200a (54), reinforcing the functional difference between CAF-S1 and CAF-S4.
Obradovic et al. recapitulated Costa’s findings in head and neck squamous cell carcinoma identifying all CAF-S1 to CAF-S4 subsets (55). In addition, they showed that clustering CAFs by flow cytometry lacks resolution and instead inferred protein activity from gene expression data and the enrichment of transcriptional targets using the Virtual Inference of Protein-activity by Enriched Regulon (VIPER) algorithm. In doing so, this added depth to CAF subtype classification and showed that the CAF-S1 subtypes could be further divided into 3 groups with differing effects on response to programmed cell death protein 1 (PD-1) checkpoint inhibition (55). This work highlights potential tissue-specific differences in CAF biology, but, crucially, helps to establish consistency in the results produced by independent groups in different tissue types (Figure 2).
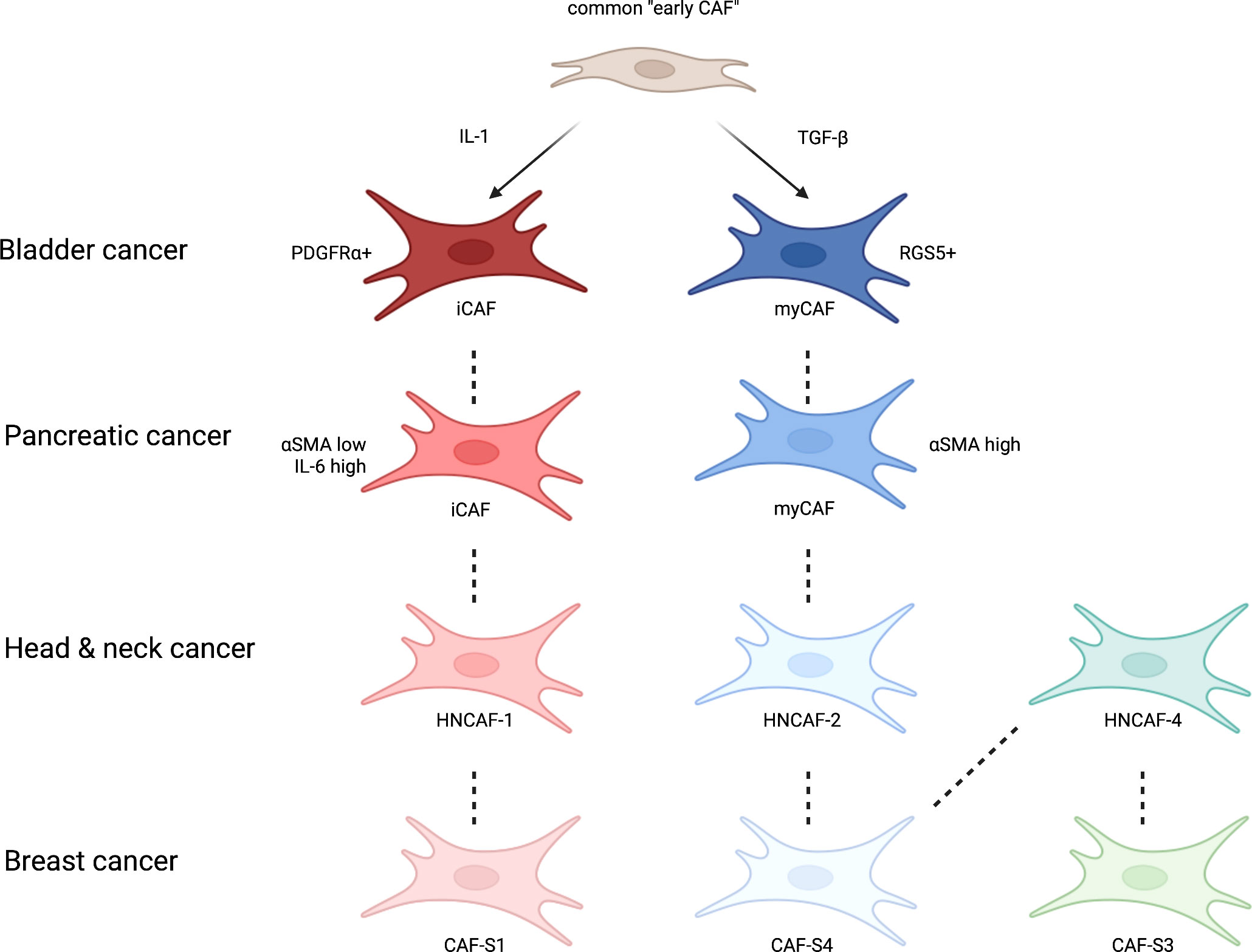
Figure 2 A simplified schematic of CAF subtypes centred around bladder cancer and their similarity with CAFs reported in other common cancers. Derived from a common CAF progenitor (25) and activated by IL-1 and TGFβ (26), in bladder cancer inflammatory CAFs (iCAFs) and myofibroblast CAFs (myCAFs) are delineated by PDGFRα and RGS5 (56). These CAF subtypes and others have been described in other common cancers, such as pancreatic (24), head and neck (55) and breast (53) with their own respective protein markers or transcriptional profiles. Figure adapted from Obradovic et al., 2022. Figure created with Biorender.com.
In addition to diversity driven by the cell of origin, plasticity in CAF profiles has been shown to change temporally throughout tumour development (57). Not only does the amount of stroma increase with stage, but Elwakeel et al. showed a dramatic increase in the proportion of regulator of G protein signalling 5 (RGS5)+ CAFs, denoted as vasculature CAFs (vCAFs), in the TME of late-stage mouse mammary tumours (57). In contrast, matrix CAFs (mCAFs) were more prevalent in untransformed mammary glands and indeed declined with tumorigenesis. The contribution of a third subset of CAFs was unchanged (57). A temporal shift in CAF biology may be indicative of environmental cues that change within the TME as the tumour progresses. It is therefore of great interest to explore how different therapeutic options can alter signals in the TME and potentially exploit CAF plasticity.
3 CAFs in bladder cancer
3.1 Bladder cancer-specific CAF heterogeneity – current knowledge
In recent years, with the aid of single cell sequencing technologies, our understanding of the cell populations in the TME of bladder cancer and their respective contributions to disease progression has substantially developed. The application of this technology in bladder cancer is limited to a handful of studies with conservative patient numbers, but, interestingly, findings to date suggest a prominent role for CAFs in bladder cancer biology.
Chen et al. performed scRNAseq on 8 bladder cancer patients and found that the fibroblast population could be stratified into iCAFs or myCAFs by the expression of PDGFRα and RGS5 respectively (56). Comparing differentially expressed genes revealed distinct functions of the two CAF subgroups and suggested that iCAFs are likely to be more pro-tumorigenic via functions associated with migration, proliferation, and angiogenesis. Moreover, when evaluated in the TCGA bladder dataset, abundance of iCAFs was associated with poor survival outcomes (56). Wang et al. were also able to segregate fibroblasts in this manner and found RGS5+ myCAFs and PDGFRα+ iCAFs in tissue from healthy, NMIBC, MIBC and metastatic bladder cancer tissue from 13 patients (58) thus corroborating the work by Chen et al.
3.2 A potential prognostic/predictive biomarker?
Acknowledging the existence of distinct CAF subtypes, Mesheyeuski et al. studied the expression of several canonical CAF markers (FAP, αSMA, CD90, PDGFRα or PDGFRβ) alone, and in combination, using immunohistochemistry, in a mixed cohort of NMIBC and MIBC, to explore associations with survival (59). Unlike other groups (Table 1) that have studied survival in the context of a single marker, none of the CAF markers studied by Mezheyeuski et al. were significantly associated with survival when studied alone. Instead, by combining the CAF markers, they showed statistically significant associations with survival and specifically demonstrated that patients with a FAP dominant stromal score had the shortest 5-year survival. Further emphasising the need to explore multiple components of the TME in combination, Mezheyeuski et al. also found that patients with a CD90 dominant stroma and a high CD8+ T-cell infiltrate had a longer 5-year survival rate (59).
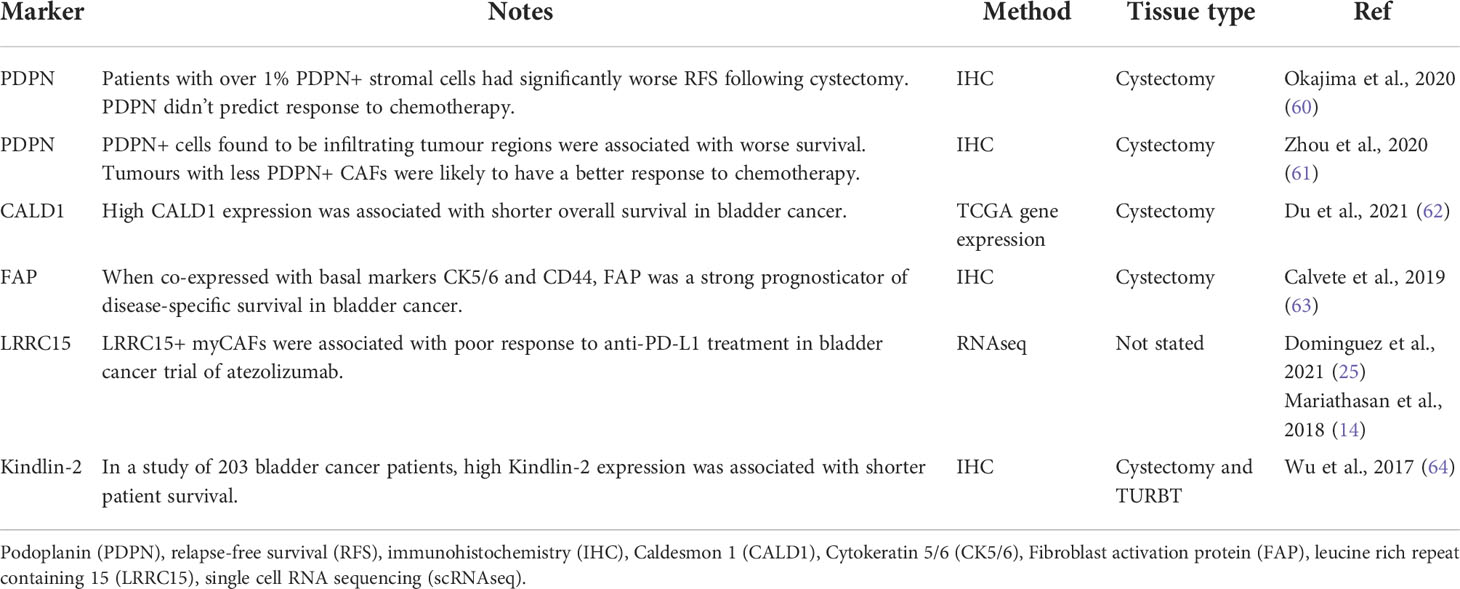
Table 1 An overview of research studies that have evaluated individual markers of CAFs in bladder cancer.
3.3 The impact of CAFs on response to treatment
3.3.1 CAFs and the treatment of bladder cancer
Disease progression following bladder-sparing treatments is a real barrier to success in the treatment of bladder cancer. As outlined in the introduction, the survival rates for bladder cancer have remained unchanged for the last five decades. In contrast other common cancers such as prostate and breast cancer have seen large improvements in survival (65).
In metastatic bladder cancer, anti-PD-1/PD-L1 immune checkpoint inhibitors such as nivolumab, pembrolizumab and atezolizumab are routinely used following key phase II and III trials that showed improved overall survival with these agents (66, 67). One of such studies was the CheckMate 275 phase II trial of the anti-PD-1 antibody nivolumab in which biological profiling of tumours was carried out by Wang et al. (15). By exploring stromal parameters in combination with CD8+ T cell infiltration, the study showed that tumours with a high CD8 infiltrate but low stromal scores had the best response to nivolumab. In contrast, a combination of high CD8 infiltrate and high stromal score was detrimental to outcome, and was attributed to an immune excluded phenotype generated by the CAFs and ECM, as discussed above (15).
Having demonstrated the benefits of checkpoint inhibition in the metastatic setting, a separate phase III trial, IMvigor010, considered the use of the anti-PD-L1 monoclonal antibody, atezolizumab, as an adjuvant therapy for patients with high-risk muscle-invasive urothelial carcinoma (68). Despite being generally well tolerated, the trial didn’t reach the intended efficacy endpoints and did not support the use of atezolizumab in this setting (68). However, further investigation of samples produced in the IMvigor010 trial showed that patients with blood samples that were positive for circulating tumour DNA (ctDNA), were better responders to atezolizumab (69). With further validation, ctDNA could help to predict which candidates are likely to respond to adjuvant immune checkpoint inhibition (69). As researchers continue to explore the ways in which immune checkpoint inhibition may improve treatment options for patients with MIBC, perhaps more attention to dual targeting of both CAFs and immune cells would be beneficial.
One of the very few studies to specifically investigate the impact of CAFs on the treatment of bladder cancer found that, of the 28 MIBC patients treated with neo-adjuvant chemotherapy, those with less CAFs in their pre-treatment biopsy were more likely to have a pathological complete response (70). In addition, the tumour cell expression of oestrogen receptor β (ERβ) was lower in tumours with a complete response compared to those with a partial or non-response. Furthermore, the patients with both a higher infiltrate of CAFs and increased expression of ERβ in their post-treatment tumours had a particularly poor response. The same group demonstrated a chemoprotective effect and enhanced proliferation in bladder cancer cell lines when co-cultured with CAFs, versus monoculture, and attributed this effect to the secretion of insulin-like growth factor (IGF) 1 (70).
3.3.2 CAFs in the treatment of other cancers
From the few studies in bladder cancer, it appears that CAFs play a broad role in reducing the efficacy of cancer therapies. However, there is a lack of bladder cancer-specific data on the impact of CAFs on some important therapies including radiotherapy. To expand our understanding of CAFs and their impact on treatments such as radiotherapy, we must look to studies in other cancer settings.
A protective effect of CAFs has been identified in response to radiotherapy for pancreatic cancer. In vitro and in vivo studies of pancreatic cancer cell lines showed that in monoculture, the growth rate of various pancreatic cancer cells markedly slows following treatment with single dose or fractionated radiotherapy. However, when co-cultured with PSC, the growth rate of pancreatic cancer cells is less affected by irradiation (71). Moreover, co-culturing pancreatic cancer cells with PSC resulted in an increase in the expression of markers associated with EMT and cancer cell stemness induced by TGFβ (72). In a separate in vivo study of radiotherapy in PDAC, treatment with radiotherapy resulted in elevated levels of inducible nitric oxide synthase (iNOS) and nitric oxide secreted by CAFs. A combination of radiotherapy with iNOS inhibition delayed PDAC tumour growth in PDAC mouse models (73), which is thought to have occurred because iNOS inhibition reduced CAF-induced pro-tumour effects.
In colorectal cancer, radiotherapy has also been shown to induce the secretion of paracrine factors from CAFs which subsequently enhanced metabolic changes and activation of IGF receptor (IGFR) in neighbouring tumour cells (74). Signalling via the IGF1R–Akt–mTOR pathway provides a survival advantage to colorectal tumour cells targeted with radiotherapy. Neutralising IGFR is therefore a potential therapeutic strategy to enhance sensitivity to radiotherapy in patients with CAF-enriched tumours (74).
In rectal cancer, patients with inferior responses to chemoradiotherapy had higher numbers of CAFs in their tumours, and low levels of IL-1 receptor α (IL-1RA) in their serum (75). In searching for the mechanistic basis of these clinical observations, Nicolas et al. showed that reciprocal signalling between CAFs and tumour cells enhanced radiotherapy resistance (76). In an array of studies, they show that IL-1α present in the ex vivo culture media of murine tumour cells was responsible for the pre-conditioning of CAFs, resulting in polarisation to an inflammatory iCAF phenotype. Accordingly, they found that increasing levels of nitrite production and signs of oxidative DNA damage made the iCAFs more vulnerable to conversion into a senescent state when treated with irradiation. In this state, iCAFs maintained their capacity to deposit ECM and so continued to create an immune cold and hostile TME that is markedly resistant to therapy. Importantly, reversal of iCAF polarisation via IL-1α inhibition seemingly sensitised tumours to radiotherapy and therefore may be a useful combination therapy (76).
Lastly, exploring differences in responder and non-responder breast cancer patients treated with neo-adjuvant chemotherapy, Su et al. found that responders had less CAFs (77). In contrast, the non-responders had a markedly higher CAF population that could be uniquely identified by the surface expression of CD10 and G protein-coupled receptor 77(GPR77). Not only did they find this CAF subtype to be chemoresistant in itself, but the subtype also reduced tumour cell sensitivity to chemotherapy. Furthermore, targeting this CAF subtype with antibodies against GPR77 reversed the chemoresistant effects (77).
Collectively, the evidence reported here suggests that CAFs play a role in the resistance to radiotherapy, chemotherapy, and combinatorial approaches. Likewise, it is apparent that in some cases radiotherapy may exacerbate the pro-tumour CAF phenotype.
4 Targeting CAFs – the future of bladder cancer treatment?
The findings discussed earlier in this review indicate that a number of factors are associated with the activation and functionality of distinct CAFs in the TME. This pleiotropic biology, and the lack of a single unifying CAF marker, poses a challenge for therapeutic targeting. However, recent reports have focused on IL-1 and TGFβ.
In this section we will explore the role of TGFβ, a pathway of particular relevance in bladder cancer. We have also indicated a number of studies that have attempted to target CAFs using several strategies in a broad spectrum of different cancers. Although we acknowledge the existence of tumour site specific biology that may affect CAF functionality and expression of phenotypic markers, we also recognise consistent trends throughout the literature that suggest that targeting CAFs in bladder cancer may result in improvements to outcomes, particularly when we consider the impact of CAFs on therapy responses.
4.1 The role of TGFβ in bladder cancer
TGFβ has a complex role in the progression of cancer which has been eloquently summarised by Barcellos-Hoff (78). TGFβ has a number of extracellular and intracellular sources, including the ECM which harbours large deposits of latent TGFβ in the form of latency-associated peptide. Under a variety of stimuli, including irradiation of tissue (79), TGFβ is released from the latency complex, resulting in activation of downstream signalling (80). Other TGFβ sources include cancer cells exosomes which can be an important mechanism to activate CAFs (81).
4.1.1 Epithelial mesenchymal transition
In bladder cancer, TGFβ signalling has been shown to be an important stimulus for the induction of EMT (82). Several studies have attempted to define precisely how TGFβ contributes to this outcome and point towards a two-part mechanism whereby TGFβ acts both up and downstream of CAFs.
Comparing the effect of multiple growth factors; TGFβ, acidic fibroblast growth factor (aFGF) and PDGF, Schulte et al. showed that only TGFβ was responsible for the upregulation of markers associated with stromal activation such as αSMA, FSP1 and FAP (40). Furthermore, fibroblasts activated by TGFβ alone, or TGFβ in combination with aFGF, were the most effective at inducing the invasion of RT112 bladder cancer cell lines. Likewise, the combination of TGFβ and aFGF led to a marked increase in the expression of markers associated with EMT (40). To uncover the mechanisms by which CAFs induce EMT, Zhuang et al. showed TGFβ was a vital component of the CAF conditioned media responsible for the induction of EMT in bladder cancer cells (82). They also identified that expression of a long non-coding RNA, ZEB2NAT, in bladder cancer cells, was essential for TGFβ-induced EMT to occur (82).
4.1.2 Immune exclusion
As discussed earlier, TGFβ is a key factor in the stromal exclusion of infiltrating immune cells. Intriguingly, in metastatic bladder cancer, TGFβ signalling status can be used in combination with PD-L1 expression and a score of tumour mutational burden to predict patient responses to the anti-PD-L1 antibody atezolizumab, illustrating how important TGFβ biology is in determining the response to immunotherapy (14). Furthermore, preliminary mouse models, representative of the immune excluded TMEs typically found in metastatic bladder cancer, have been used to demonstrate the effectiveness of TGFβ inhibition in combination with anti-PD-L1. With this combination therapy, a reduction in tumour burden was accompanied by an increase in the number of tumour-infiltrating CD8+ T lymphocytes and crucially such lymphocytes were released from stromal traps to unleash anti-tumour immunity in the appropriate tumour regions (14).
4.1.3 Reprogramming of CAFs
As described earlier, RGS5 has recently emerged as an important marker of the myCAF population in bladder cancer (56). Interestingly, in the pancreas, a high expression of RGS5 on pericytes is typically associated with apoptosis of these cells (83). In contrast, Dasgupta et al. found RGS5+ CAFs in the TME of pancreatic cancers do not undergo apoptosis as expected and instead survive and expand (83). In further exploring this observation, they found TGFβ plays a pivotal role in the reprogramming of CAFs via conversion of signalling pathways to allow RGS5 to interact with pSmad2/3 - this resulted in the transcription of genes associated with pro-tumour and proliferative functions (83). If TGFβ is capable of inducing profound reprogramming of RGS5+ CAFs towards a pro-tumour phenotype, it would be interesting to explore how manipulation of TGFβ signalling may affect the RGS5+ myCAF population identified in bladder cancer. We will continue to explore this concept below.
4.2 Improvements to therapy responses via targeting of CAFs
4.2.1 Manipulation of TGFβ signalling
As we have indicated throughout this review, TGFβ has a fundamental role in the activation and differentiation of CAFs where it typically exerts profound pro-tumour effects. In some cancers, there is evidence that “natural” TGFβ downregulation corresponds with favourable outcomes. This was evident in patients with HPV+ Head and neck squamous cell carcinoma who had less CAFs in their TME and an improved prognosis (84). Wang et al. suggest that miRNA exosomes derived from the tumour cells of these patients were capable of infiltrating CAFs and altering active signalling pathways by reducing the expression of NADPH oxidase (NOX)4 and the presence of reactive oxygen species (ROS). As a result, TGFβ signalling is inhibited (84).
Although the pleiotropic effects of TGFβ make it a challenging target, the above observations encourage further research into novel strategies for manipulation of TGFβ signalling to reduce the pro-tumour impact of CAFs (Table 2). It should be noted that TGFβ signaling is important for the regulation of many non-stromal cell types, indeed TGFβ can contribute to both tumour suppression and promotion (91). Therefore targeting TGFβ may result in undesirable consequences in some situations and should be approached with caution. As more of the specific biology associated with TGFβ signalling in CAFs is uncovered, there is hope that a more precise therapeutic approach may become available (25).
One novel method to manipulate TGFβ uses a dual TGFβ and PD-L1 targeting approach with a novel fusion protein called bintrafusp alpha. In combination with radiotherapy, bintrafusp alpha proved effective at reducing the fibrotic networks induced by TGFβ-activated CAFs, and increased the infiltration of cytotoxic CD8+ T-cells in multiple immunocompetent mouse modes of PDAC, glioblastoma, neuroendocrine colonic carcinoma and mouse mammary carcinoma (85).
Similar findings have been observed in the phase Ib/IIa MP-VAC-204 trial which involved a combination of the TGFβ receptor type 1 inhibitor Vactosertib with the PD-1 inhibitor pembrolizumab to treat patients with microsatellite stable metastatic colorectal carcinoma (mCRC) (86). The initial results of MP-VAC-204 are promising and show a decrease in biomarkers of TGFβ signalling and crucially an increase in CD8+ T-cell infiltration. Where previously pembrolizumab was effective for a small percentage of patients with microsatellite instability mCRC, the addition of TGFβ inhibition appears to increase the number of patients who may benefit from checkpoint inhibition (86).
Taking a different approach, Yegodayev et al. found a stromal increase in TGFβ signalling to be associated with a poor response to the epidermal growth factor receptor (EGFR) antibody cetuximab in patient-derived xenograft models of head and neck cancers (87). Moreover, cetuximab efficacy could be greatly improved with the combined use of a SMAD3 inhibitor to block the downstream effects of TGFβ (87).
Promising strategies to target the NOX4/TGFβ axis have also been explored. They include the NOX1/NOX4 inhibitor GKT137831, which reduced the production of ROS in TGFβ-activated CAFs in prostate cancer and further dissipated the pro-tumour functions of CAFs (92). In a number of mouse models, GKT137831 “normalised” CAFs and, as a result, stranded CD8+ T-cells were able to infiltrate tumours and were more responsive to immunotherapeutic agents such as anti-PD-1 (88). Similarly, in vitro studies of bladder cancer have demonstrated the efficacy of the NOX4 inhibitor diphenylene iodonium, which slowed cancer cell growth via a reduction in the expression of ROS (89). Lastly, magnesium isoglycyrrhizinate has also been tested in vitro and in vivo in the bladder cancer setting and showed promise as a potential NOX4 inhibitor that prevents tumour growth (90).
4.2.2 Alternative strategies to target CAFs
Strategies to combine CAF targeting agents other than TGFβ inhibitors with immune checkpoint blockade have also shown pre-clinical promise. Nintedanib is a tyrosine kinase inhibitor which has anti-fibrotic effects in the TME and contributes to an anti-tumour response via the release of previously excluded immune cells (93). The addition of anti-PD-1 treatment enhanced responses to treatment with nintedanib and slowed the growth of B16-F10 melanoma tumours in vivo (93). Intriguingly, nintedanib has recently shown promise when combined with neo-adjuvant chemotherapy in localised MIBC. In the NEOBLADE trial, addition of nintedanib to standard radical treatment significantly improved overall survival, suggesting this drug warrants further consideration in bladder cancer (94).
Alternative attempts to target CAFs, and therefore slow the progression of cancer, have included the use of FAP-specific chimeric antigen receptor (CAR) T-cells. In vitro and in vivo studies have demonstrated the feasibility of targeting CAFs using this approach, and show early signs of promise that FAP-specific CAR T-cells can reduce tumour burden (95, 96). However, by targeting CAFs we have continued to learn about crucial elements of their biology. Several attempts to deplete the fibroblast component of the TME and the associated ECM have unfortunately resulted in enhanced tumour progression. This finding not only supports the notion that CAFs are a heterogenous population, but that CAFs can also function in a manner that restrains tumour growth in specific contexts. Although we do not wish to over-emphasize this point, it is important we include this for completion.
One such example was demonstrated in a genetically modified mouse model of PDAC in which αSMA+ myofibroblasts could be depleted at a given time point in a drug dependent manner. Depletion at both early and late stages of PDAC development resulted in reorganisation of the associated ECM, a reduction in infiltrating effector T-cells, and an increase in regulatory T-cells, all of which combined to create significantly worse survival outcomes compared to control mice (97).
Similarly, progression of PDAC tumours was observed when the stromal driving factor sonic hedgehog (Shh) was deleted from tumour cells. Under these conditions, the PDAC TME contained less stromal cells, but was more vascular and proliferative (98). Deletion of stromal components such as type one collagen, a crucial part of the ECM, had a similar effect (99).
Although there have been very few studies targeting CAFs in bladder cancer, we can apply our knowledge of bladder cancer biology to infer similarities with tumour types such as PDAC (97). For example, Shin et al. showed that in mouse and human tumours loss of Shh was associated with progression from carcinoma in situ to MIBC (100). As such tumour cells were no longer able to induce stromal cell differentiation via Shh, this resulted in a proliferative tumour lacking restraint from neighbouring stromal cells (100).
Rather than deplete specific CAF populations, a new trial reported by Mizutani et al. considered the plasticity of CAFs and proposed a new treatment regimen for patients with advanced pancreatic cancer that could convert tumour-promoting CAFs (pCAFs) into tumour-restraining CAFs (rCAFs) via the addition of AM80 (101). In combination with chemotherapy, AM80, a synthetic retinoid, converted pCAFs into rCAFs marked by the upregulation of Meflin and downregulation of the canonical CAF marker αSMA. Although this phase I trial is in the initial stages, it offers an insight into the potential direction of research into CAFs and the future strategies that may be undertaken to target them (101).
In a similar manner, manipulation and reversal of CAF activation has been attempted using Vitamin D (102, 103). Indeed, in colorectal carcinoma (CRC) an increase in the expression of Vitamin D receptors (VDR) on fibroblasts in the TME was associated with improved survival outcomes (102). Ferrer-Mayorga et al. subsequently found that an increase in the active form of Vitamin D (1,25(OH)2D3) prevented activation of normal fibroblasts into CAFs and induced a gene signature that was associated with improved survival outcomes in CRC patients (102). Experiments using the VDR ligand calcipotriol had a similar effect in PDAC (103).
Understanding CAF biology not only helps to develop complementary treatment options, but it can also help to optimise current ones. One CAF targeting strategy takes an alternative approach and uses a 68Ga-radiolabeled inhibitor of FAP (FAPI) to visualise CAFs on PET/CT scans for patients with head and neck squamous cell carcinoma (104). In this novel study, visualisation of FAP+ CAFs acts as both a diagnostic tool and a therapeutic guide helping to risk stratify patients and improve the delivery of radiotherapy (104).
5 Conclusion
This review has discussed how further research into the role of CAFs in bladder cancer is necessary to fill important gaps in our knowledge of this disease and expand therapeutic options for patients. Additionally, it is particularly important to understand how CAFs impact the response to therapies currently available to patients. Based on the evidence presented in this review, we hypothesise that CAF enrichment of bladder tumours is associated with inferior responses to radical radiotherapy for MIBC. Poor responses to radiotherapy are seen in other tumour types with a high stromal score and an increased number of CAFs, such as rectal cancer (75). If, as we hypothesize, MIBC enriched for CAFs show similar behavior, this highlights a cohort of patients that we propose would benefit from a combinatorial approach that includes targeting of CAFs. In addition, CAF targeting approaches may have a role in metastatic disease. In the United Kingdom, the RE-ARM trial is exploring the combination of immune checkpoint blockade with “experimental” palliative radiotherapy in patients with metastatic urothelial cancer (105). The clinical outcomes of this trial, including the comprehensive translational profiling that is embedded in RE-ARM, may help to identify groups of patients that could benefit from CAF targeting approaches. In doing so, this could expand the number of patients that ultimately benefit from immune checkpoint inhibition.
In conclusion, we propose that the various strategies to target CAFs discussed in this review have considerable therapeutic potential, both as single agents, and in combination with existing therapies, to improve survival outcomes for patients with bladder cancer. In order to inform treatment advances, we consider that it is a research priority to better understand bladder cancer-specific CAF subtypes and their spatial relationship with other cells of the TME at baseline, as well as longitudinal changes in CAF biology following treatments such as radiotherapy.
Author contributions
AB wrote and edited the manuscript and created the figures. AW proposed the conception, edited and reviewed the manuscript. AR edited and reviewed the manuscript. All authors listed in the paper have made a substantial, direct, and intellectual contribution to the work and approved it for publication. All authors contributed to the article and approved the submitted version.
Funding
The PhD studentship awarded to AB is supported by the MRC iCASE Doctoral Training Partnership in collaboration with AstraZeneca. Award number: MR/R01583X/1. We acknowledge NHS funding to the NIHR Biomedical Research Centre at The Royal Marsden and the ICR. AW acknowledges funding from the ICR/RMH CRUK RadNet centre.
Acknowledgments
This study represents independent research supported by the National Institute for Health and Care Research (NIHR) Biomedical Research Centre at The Royal Marsden NHS Foundation Trust and the Institute of Cancer Research, London. The views expressed are those of the author(s) and not necessarily those of the NIHR or the Department of Health and Social Care. AW acknowledges funding from the RMH/ICR Cancer Research UK RadNet Centre.
Conflict of interest
AB declares funding from AstraZeneca to support the work of their PhD project. AW declares potential funding from imCORE for translational profiling in the RE-ARM study which is sponsored by Roche.
The remaining authors declare that the research was conducted in the absence of any commercial or financial relationships that could be construed as a potential conflict of interest.
Publisher’s note
All claims expressed in this article are solely those of the authors and do not necessarily represent those of their affiliated organizations, or those of the publisher, the editors and the reviewers. Any product that may be evaluated in this article, or claim that may be made by its manufacturer, is not guaranteed or endorsed by the publisher.
References
1. Detailed statistics from the 'Get data out' programme > bladder, urethra, renal pelvis and ureter. Available at: https://www.cancerdata.nhs.uk/getdataout/bladder.
2. Cancer Research UK. What is bladder cancer (2018). Available at: https://about-cancer.cancerresearchuk.org/about-cancer/bladder-cancer/about.
3. Cancer Research UK. Bladder cancer survival statistics (2020). Available at: https://www.cancerresearchuk.org/health-professional/cancer-statistics/statistics-by-cancer-type/bladder-cancer/survival#heading-Zero.
4. Mo Q, Nikolos F, Chen F, Tramel Z, Lee YC, Hayashi K, et al. Prognostic power of a tumor differentiation gene signature for bladder urothelial carcinomas. J Natl Cancer Inst (2018) 110(5):448–59. doi: 10.1093/jnci/djx243
5. Damrauer JS, Hoadley KA, Chism DD, Fan C, Tiganelli CJ, Wobker SE, et al. Intrinsic subtypes of high-grade bladder cancer reflect the hallmarks of breast cancer biology. Proc Natl Acad Sci USA (2014) 111(8):3110–5. doi: 10.1073/pnas.1318376111
6. Robertson AG, Kim J, Al-Ahmadie H, Bellmunt J, Guo G, Cherniack AD, et al. Comprehensive molecular characterization of muscle-invasive bladder cancer. Cell (2017) 171(3):540–556.e25. doi: 10.1016/j.cell.2017.09.007
7. Choi W, Porten S, Kim S, Willis D, Plimack ER, Hoffman-Censits J, et al. Identification of distinct basal and luminal subtypes of muscle-invasive bladder cancer with different sensitivities to frontline chemotherapy. Cancer Cell (2014) 25(2):152–65. doi: 10.1016/j.ccr.2014.01.009
8. Rebouissou S, Bernard-Pierrot I, de Reyniès A, Lepage ML, Krucker C, Chapeaublanc E, et al. EGFR as a potential therapeutic target for a subset of muscle-invasive bladder cancers presenting a basal-like phenotype. Sci Transl Med (2014) 6(244):244ra91. doi: 10.1126/scitranslmed.3008970
9. Marzouka NAD, Eriksson P, Rovira C, Liedberg F, Sjödahl G, Höglund M. A validation and extended description of the Lund taxonomy for urothelial carcinoma using the TCGA cohort. Sci Rep (2018) 8(1):3737. doi: 10.1038/s41598-018-22126-x
10. Kamoun A, de Reyniès A, Allory Y, Sjödahl G, Robertson AG, Seiler R, et al. A consensus molecular classification of muscle-invasive bladder cancer. Eur Urol (2020) 77(4):420–33. doi: 10.1016/j.eururo.2019.09.006
11. James ND, Hussain SA, Hall E, Jenkins P, Tremlett J, Rawlings C, et al. Radiotherapy with or without chemotherapy in muscle-invasive bladder cancer. N Engl J Med (2012) 366(16):1477–88. doi: 10.1056/NEJMoa1106106
12. Hall E, Hussain SA, Porta N, Lewis R, Crundwell M, Jenkins P, et al. Chemoradiotherapy in muscle-invasive bladder cancer: 10-yr follow-up of the phase 3 randomised controlled BC2001 trial. Eur Urol (2022) 82(3):273–9. doi: 10.1016/j.eururo.2022.04.017
13. El-Taji OM, Alam S, Hussain SA. Bladder sparing approaches for muscle-invasive bladder cancers. Curr Treat Options Oncol (2016) 17(3):15. doi: 10.1007/s11864-016-0390-8
14. Mariathasan S, Turley SJ, Nickles D, Castiglioni A, Yuen K, Wang Y, et al. TGFβ attenuates tumour response to PD-L1 blockade by contributing to exclusion of T cells. Nature (2018) 554(7693):544–8. doi: 10.1038/nature25501
15. Wang L, Saci A, Szabo PM, Chasalow SD, Castillo-Martin M, Domingo-Domenech J, et al. EMT and stroma-related gene expression and resistance to pd-1 blockade in urothelial cancer. Nat Comms (2018) 9(3503):3503. doi: 10.1038/s41467-018-05992-x
16. Kalluri R. The biology and function of fibroblasts in cancer. Nat Rev Cancer (2016) 16(9):582–98. doi: 10.1038/nrc.2016.73
17. Elyada E, Bolisetty M, Laise P, Flynn WF, Courtois ET, Burkhart RA, et al. Cross-species single-cell analysis of pancreatic ductal adenocarcinoma reveals antigen-presenting cancer-associated fibroblasts. Cancer Discov (2019) 9(8):1102–23. doi: 10.1158/2159-8290.CD-19-0094
18. Raz Y, Cohen N, Shani O, Bell RE, Novitskiy SV, Abramovitz L, et al. Bone marrow-derived fibroblasts are a functionally distinct stromal cell population in breast cancer. J Exp Med (2018) 215(12):3075–93. doi: 10.1084/jem.20180818
19. Park D, Sahai E, Rullan A. SnapShot: Cancer-associated fibroblasts. Cell (2020) 181(2):486.e1. doi: 10.1016/j.cell.2020.03.013
20. Bartoschek M, Oskolkov N, Bocci M, Lövrot J, Larsson C, Sommarin M, et al. Spatially and functionally distinct subclasses of breast cancer-associated fibroblasts revealed by single cell RNA sequencing. Nat Commun (2018) 9(1):5150. doi: 10.1038/s41467-018-07582-3
21. Buechler MB, Pradhan RN, Krishnamurty AT, Cox C, Calviello AK, Wang AW, et al. Cross-tissue organization of the fibroblast lineage. Nature (2021) 593(7860):575–9. doi: 10.1038/s41586-021-03549-5
22. Strell C, Paulsson J, Jin S-B, Tobin NP, Mezheyeuski A, Roswall P, et al. Impact of epithelial-stromal interactions on peritumoral fibroblasts in ductal carcinoma in situ. J Natl Cancer Institute (2019) 111(9):983–95. doi: 10.1093/jnci/djy234
23. Grimm S, Jennek S, Singh R, Enkelmann A, Junker K, Rippaus N. Malignancy of bladder cancer cells is enhanced by tumor-associated fibroblasts through a multifaceted cytokine-chemokine loop. Exp Cell Res (2015) 335(1):1–11. doi: 10.1016/j.yexcr.2015.04.001
24. Öhlund D, Handly-Santana A, Biffi G, Elyada E, Almeida AS, Ponz-Sarvise M, et al. Distinct populations of inflammatory fibroblasts and myofibroblasts in pancreatic cancer. J Exp Med (2017) 214(3):579–96. doi: 10.1084/jem.20162024
25. Dominguez CX, Müller S, Keerthivasan S, Koeppen H, Hung J, Gierke S, et al. Single-cell RNA sequencing reveals stromal evolution into LRRC15+ myofibroblasts as a determinant of patient response to cancer immunotherapy. Cancer Discov (2020) 10(2):232–53. doi: 10.1158/2159-8290.CD-19-0644
26. Biffi G, Oni TE, Spielman B, Hao Y, Elyada E, Park Y, et al. Il1-induced Jak/STAT signaling is antagonized by TGFβ to shape CAF heterogeneity in pancreatic ductal adenocarcinoma. Cancer Discovery (2019) 9(2):282–301. doi: 10.1158/2159-8290.CD-18-0710
27. Coplen DE, Howard PS, Duckett JW, Snyder HM, Macarak EJ. Characterization of a fibroblast cell from the urinary bladder wall. Vitro Cell Dev Biol Anim (1994) 30A(9):604–8. doi: 10.1007/BF02631259
28. Fry CH, Sui GP, Kanai AJ, Wu C. The function of suburothelial myofibroblasts in the bladder. Neurourol Urodyn (2007) 26(6 Suppl):914–9. doi: 10.1002/nau.20483
29. Calvo F, Ege N, Grande-Garcia A, Hooper S, Jenkins RP, Chaudhry SI, et al. Mechanotransduction and YAP-dependent matrix remodelling is required for the generation and maintenance of cancer-associated fibroblasts. Nat Cell Biol (2013) 15(6):637–46. doi: 10.1038/ncb2756
30. Belhabib I, Zaghdoudi S, Lac C, Bousquet C, Jean C. Extracellular matrices and cancer-associated fibroblasts: Targets for cancer diagnosis and therapy? Cancers (Basel) (2021) 13(14):3466. doi: 10.3390/cancers13143466
31. Yan L, Wang P, Fang W, Liang C. Cancer-associated fibroblasts–derived exosomes-mediated transfer of LINC00355 regulates bladder cancer cell proliferation and invasion. Cell Biochem Funct (2020) 38(3):257–65. doi: 10.1002/cbf.3462
32. Zhou Z, Cui D, Sun MH, Huang JL, Deng Z, Han BM. CAFs-derived MFAP5 promotes bladder cancer malignant behavior through NOTCH2/HEY1 signaling. FASEB J (2020) 34(6):7970–88. doi: 10.1096/fj.201902659R
33. Dong D, Yao Y, Song J, Sun L, Zhang G. Cancer-associated fibroblasts regulate bladder cancer invasion and metabolic phenotypes through autophagy. Dis Markers (2021) 2021:6645220. doi: 10.1155/2021/6645220
34. Yang F, Guo Z, He C, Qing L, Wang H, Wu J, et al. Cancer-associated fibroblasts promote cell proliferation and invasion via paracrine Wnt/IL1β signaling pathway in human bladder cancer. Neoplasma (2021) 68(1):79–86. doi: 10.4149/neo_2020_200202N101
35. Shan G, Zhou X, Gu J, Zhou D, Cheng W, Wu H, et al. Downregulated exosomal microRNA-148b-3p in cancer associated fibroblasts enhance chemosensitivity of bladder cancer cells by downregulating the wnt/β-catenin pathway and upregulating PTEN. Cell Oncol (2021) 44(1):45–59. doi: 10.1007/s13402-020-00500-0
36. Sazeides C, Le A. Metabolic relationship between cancer-associated fibroblasts and cancer cells. Heterogeneity Cancer Metab (2021) 1311:189–204. doi: 10.1007/978-3-030-65768-0_14
37. Pavlides S, Whitaker-Menezes D, Castello-Cros R, Flomenberg N, Witkiewicz AK, Frank PG, et al. The reverse warburg effect: aerobic glycolysis in cancer associated fibroblasts and the tumor stroma. Cell Cycle (2009) 8(23):3984–4001. doi: 10.4161/cc.8.23.10238
38. Shan T, Chen S, Chen X, Lin WR, Li W, Ma J, et al. Cancer-associated fibroblasts enhance pancreatic cancer cell invasion by remodeling the metabolic conversion mechanism. Oncol Rep (2017) 37(4):1971–9. doi: 10.3892/or.2017.5479
39. Goulet CR, Champagne A, Bernard G, Vandal D, Chabaud S, Pouliot F, et al. Cancer-associated fibroblasts induce epithelial-mesenchymal transition of bladder cancer cells through paracrine IL-6 signalling. BMC Cancer (2019) 19(1):137. doi: 10.1186/s12885-019-5353-6
40. Schulte J, Weidig M, Balzer P, Richter P, Franz M, Junker K., et al. Expression of the e-cadherin repressors snail, slug and Zeβ1 in urothelial carcinoma of the urinary bladder: Relation to stromal fibroblast activation and invasive behaviour of carcinoma cells. Histochem Cell Biol (2012) 138(6):847–60. doi: 10.1007/s00418-012-0998-0
41. Sahai E, Astsaturov I, Cukierman E, DeNardo DG, Egeblad M, Evans RM, et al. A framework for advancing our understanding of cancer-associated fibroblasts. Nat Rev Cancer (2020) 20(3):174–86. doi: 10.1038/s41568-019-0238-1
42. Chakravarthy A, Khan L, Bensler NP, Bose P, De Carvalho DD. TGF-beta-associated extracellular matrix genes link cancer-associated fibroblasts to immune evasion and immunotherapy failure. Nat Commun (2018) 9(1):4692. doi: 10.1038/s41467-018-06654-8
43. Gil-Julio H, Perea F, Rodriguez-Nicolas A, Cozar JM, González-Ramirez AR, Concha A, et al. Tumor escape phenotype in bladder cancer is associated with loss of HLA class I expression, T-cell exclusion and stromal changes. Int J Mol Sci (2021) 22(14):7248. doi: 10.3390/ijms22147248
44. Salmon H, Franciszkiewicz K, Damotte D, Dieu-Nosjean MC, Validire P, Trautmann A, et al. Matrix architecture defines the preferential localization and migration of T cells into the stroma of human lung tumors. J Clin Invest (2012) 122(3):899–910. doi: 10.1172/JCI45817
45. Suzuki J, Aokage K, Neri S, Sakai T, Hashimoto H, Su Y, et al. Relationship between podoplanin-expressing cancer-associated fibroblasts and the immune microenvironment of early lung squamous cell carcinoma. Lung Cancer (2021) 153:1–10. doi: 10.1016/j.lungcan.2020.12.020
46. Rodriguez AB, Peske JD, Woods AN, Leick KM, Mauldin IS, Meneveau MO, et al. Immune mechanisms orchestrate tertiary lymphoid structures in tumors via cancer-associated fibroblasts. Cell Rep (2021) 36(3). doi: 10.1016/j.celrep.2021.109422
47. Gao J, Navai N, Alhalabi O, Siefker-Radtke A, Campbell MT, Tidwell RS, et al. Neoadjuvant PD-L1 plus CTLA-4 blockade in patients with cisplatin-ineligible operable high-risk urothelial carcinoma. Nat Med (2020) 26(12):1845–51. doi: 10.1038/s41591-020-1086-y
48. Jamal-Hanjani M, Wilson GA, McGranahan N, Birkbak NJ, Watkins TBK, Veeriah S, et al. Tracking the evolution of non-Small-Cell lung cancer. N Engl J Med (2017) 376(22):2109–21. doi: 10.1056/NEJMoa1616288
49. Turajlic S, Xu H, Litchfield K, Rowan A, Chambers T, Lopez JI, et al. Tracking cancer evolution reveals constrained routes to metastases: TRACERx renal. Cell (2018) 173(3):581–594.e12. doi: 10.1016/j.cell.2018.03.057
50. AbdulJabbar K, Raza SEA, Rosenthal R, Jamal-Hanjani M, Veeriah S, Akarca A, et al. Geospatial immune variability illuminates differential evolution of lung adenocarcinoma. Nat Med (2020) 26(7):1054–62. doi: 10.1038/s41591-020-0900-x
51. Galbo PM, Zang X, Zheng D. Molecular features of cancer-associated fibroblast subtypes and their implication on cancer pathogenesis, prognosis, and immunotherapy resistance. Clin Cancer Res (2021) 27(9):2636–47. doi: 10.1158/1078-0432.CCR-20-4226
52. Cremasco V, Astarita JL, Grauel AL, Keerthivasan S, MacIsaac K, Woodruff MC, et al. FAP delineates heterogeneous and functionally divergent stromal cells in immune-excluded breast tumors. Cancer Immunol Res (2018) 6(12):1472–85. doi: 10.1158/2326-6066.CIR-18-0098
53. Costa A, Kieffer Y, Scholer-Dahirel A, Pelon F, Bourachot B, Cardon M, et al. Fibroblast heterogeneity and immunosuppressive environment in human breast cancer. Cancer Cell (2018) 33(3):463–79.e10. doi: 10.1016/j.ccell.2018.01.011
54. Givel AM, Kieffer Y, Scholer-Dahirel A, Sirven P, Cardon M, Pelon F, et al. MiR200-regulated CXCL12β promotes fibroblast heterogeneity and immunosuppression in ovarian cancers. Nat Commun (2018) 9(1):1056. doi: 10.1038/s41467-018-03348-z
55. Obradovic A, Graves D, Korrer M, Wang Y, Roy S, Naveed A, et al. Immunostimulatory cancer-associated fibroblast subpopulations can predict immunotherapy response in head and neck cancer. Clin Cancer Res (2022) 28(10):2094–109. doi: 10.1158/1078-0432.CCR-21-3570
56. Chen Z, Zhou L, Liu L, Hou Y, Xiong M, Yang Y, et al. Single-cell RNA sequencing highlights the role of inflammatory cancer-associated fibroblasts in bladder urothelial carcinoma. Nat Commun (2020) 11(1):5077. doi: 10.1038/s41467-020-18916-5
57. Elwakeel E, Brüggemann M, Fink AF, Schulz MH, Schmid T, Savai R, et al. Phenotypic plasticity of fibroblasts during mammary carcinoma development. Int J Mol Sci (2019) 20(18):4438. doi: 10.3390/ijms20184438
58. Wang H, Mei Y, Luo C, Huang Q, Wang Z, Lu GM, et al. Single-cell analyses reveal mechanisms of cancer stem cell maintenance and epithelial-mesenchymal transition in recurrent bladder cancer. Clin Cancer Res (2021) 27(22):6265–78. doi: 10.1158/1078-0432.CCR-20-4796
59. Mezheyeuski A, Segersten U, Leiss LW, Malmström PU, Hatina J, Östman A, et al. Fibroblasts in urothelial bladder cancer define stroma phenotypes that are associated with clinical outcome. Sci Rep (2020) 10(1):281. doi: 10.1038/s41598-019-55013-0
60. Okajima E, Tomizawa M, Shimada K, Negishi T, Nishiyama N, Kitamura H. D2-40/podoplanin expression in cancer stroma by immunohistochemical staining is associated with poor prognosis in bladder cancer patients after radical cystectomy. Urologic Oncology: Semin Original Investigations (2020) 38(10):797.e7–797.e13. doi: 10.1016/j.urolonc.2020.05.020
61. Zhou Q, Wang Z, Zeng H, Zhang H, Liu Z, Huang Q, et al. Identification and validation of poor prognosis immunoevasive subtype of muscle-invasive bladder cancer with tumor-infiltrating podoplanin+ cell abundance. OncoImmunology (2020) 9(1):1747333. doi: 10.1080/2162402X.2020.1747333
62. Du YH, Jiang X, Wang B, Cao J, Wang Y, Yu J, et al. The cancer-associated fibroblasts related gene CALD1 is a prognostic biomarker and correlated with immune infiltration in bladder cancer. Cancer Cell Int (2021) 21(1):283. doi: 10.1186/s12935-021-01896-x
63. Calvete J, Larrinaga G, Errarte P, Martín AM, Dotor A, Esquinas C, et al. The coexpression of fibroblast activation protein (FAP) and basal-type markers (CK 5/6 and CD44) predicts prognosis in high-grade invasive urothelial carcinoma of the bladder. Hum Pathol (2019) 91:61–8. doi: 10.1016/j.humpath.2019.07.002
64. Wu J, Yu C, Cai L, Lu Y, Jiang L, Liu C, et al. Effects of increased kindlin-2 expression in bladder cancer stromal fibroblasts. Oncotarget (2017) 8(31):50692–703. doi: 10.18632/oncotarget.17021
65. Cancer Research UK. Cancer survival for common cancers. Available at: https://www.cancerresearchuk.org/health-professional/cancer-statistics/survival/common-cancers-compared#heading-Three.
66. Balar AV, Galsky MD, Rosenberg JE, Powles T, Petrylak DP, Bellmunt J, et al. Atezolizumab as first-line treatment in cisplatin-ineligible patients with locally advanced and metastatic urothelial carcinoma: a single-arm, multicentre, phase 2 trial. Lancet (2017) 389(10064):67–76. doi: 10.1016/S0140-6736(16)32455-2
67. Bellmunt J, Bajorin DF. Pembrolizumab for advanced urothelial carcinoma. N Engl J Med (2017) 376(23):2304. doi: 10.1056/NEJMc1704612
68. Bellmunt J, Hussain M, Gschwend JE, Albers P, Oudard S, Castellano D, et al. Adjuvant atezolizumab versus observation in muscle-invasive urothelial carcinoma (IMvigor010): a multicentre, open-label, randomised, phase 3 trial. Lancet Oncol (2021) 22(4):525–37. doi: 10.1016/S1470-2045(21)00004-8
69. Powles T, Assaf ZJ, Davarpanah N, Banchereau R, Szabados BE, Yuen KC, et al. ctDNA guiding adjuvant immunotherapy in urothelial carcinoma. Nature (2021) 595(7867):432–7. doi: 10.1038/s41586-021-03642-9
70. Long X, Xiong W, Zeng X, Qi L, Cai Y, Mo M. Cancer-associated fibroblasts promote cisplatin resistance in bladder cancer cells by increasing IGF-1/ERβ/Bcl-2 signalling. Cell Death Dis (2019) 10(5):375. doi: 10.1038/s41419-019-1581-6
71. Mantoni TS, Lunardi S, Al-Assar O, Masamune A, Brunner TB. Pancreatic stellate cells radioprotect pancreatic cancer cells through β1-integrin signaling. Cancer Res (2011) 71(10):3453–8. doi: 10.1158/0008-5472.CAN-10-1633
72. Al-Assar O, Demiciorglu F, Lunardi S, Gaspar-Carvalho MM, McKenna WG, Muschel RM. Contextual regulation of pancreatic cancer stem cell phenotype and radioresistance by pancreatic stellate cells. Radiotherapy Oncol (2014) 111(2):243–51. doi: 10.1016/j.radonc.2014.03.014
73. Pereira PMR, Edwards KJ, Mandleywala K, Carter LM, Escorcia FE, Campesato LF, et al. iNOS regulates the therapeutic response of pancreatic cancer cells to radiotherapy. Cancer Res (2020) 80(8):1681–92. doi: 10.1158/0008-5472.CAN-19-2991
74. Tommelein J, De Vlieghere E, Verset L, Melsens E, Leenders J, Descamps B, et al. Radiotherapy-activated cancer-associated fibroblasts promote tumor progression through paracrine IGF1R activation. Cancer Res (2018) 78(3):659–70. doi: 10.1158/0008-5472.CAN-17-0524
75. Wilkins A, Fontana E, Nyamundanda G, Ragulan C, Patil Y, Mansfield D, et al. Differential and longitudinal immune gene patterns associated with reprogrammed microenvironment and viral mimicry in response to neoadjuvant radiotherapy in rectal cancer. J ImmunoTherapy Cancer (2021) 9(3). doi: 10.1136/jitc-2020-001717corr1
76. Nicolas AM, Pesic M, Engel E, Ziegler PK, Diefenhardt M, Kennel KB, et al. Inflammatory fibroblasts mediate resistance to neoadjuvant therapy in rectal cancer. Cancer Cell (2022) 40(2):168–84.e13. doi: 10.1016/j.ccell.2022.01.004
77. Su S, Chen J, Yao H, Liu J, Yu S, Lao L, et al. CD10+GPR77+ cancer-associated fibroblasts promote cancer formation and chemoresistance by sustaining cancer stemness. Cell (2018) 172(4):841–56.e16. doi: 10.1016/j.cell.2018.01.009
78. Barcellos-Hoff MH. The radiobiology of TGFbeta. Semin Cancer Biol (2022) S1044-579X(22)00022-0. doi: 10.1016/j.semcancer.2022.02.001
79. Ehrhart EJ, Segarini P, Tsang ML, Carroll AG, Barcellos-Hoff MH. Latent transforming growth factor beta1 activation in situ: quantitative and functional evidence after low-dose gamma-irradiation. FASEB J (1997) 11(12):991–1002. doi: 10.1096/fasebj.11.12.9337152
80. Sturm G, Finotello F, Petitprez F, Zhang JD, Baumbach J, Fridman WH, et al. Comprehensive evaluation of transcriptome-based cell-type quantification methods for immuno-oncology. Bioinformatics (2019) 35(14):i436–45. doi: 10.1093/bioinformatics/btz363
81. Goulet CR, Bernard G, Tremblay S, Chabaud S, Bolduc S, Pouliot F, et al. Exosomes induce fibroblast differentiation into cancer-associated fibroblasts through TGFb signaling. Mol Cancer Res (2018) 16(7):1196–204. doi: 10.1158/1541-7786.MCR-17-0784
82. Zhuang J, Lu Q, Shen B, Huang X, Shen L, Zheng X, et al. TGFβ1 secreted by cancer-associated fibroblasts induces epithelial-mesenchymal transition of bladder cancer cells through lncRNA-ZEB2NAT. Sci Rep (2015) 5:11924. doi: 10.1038/srep11924
83. Dasgupta S, Ghosh T, Dhar J, Bhuniya A, Nandi P, Das A, et al. RGS5-TGFbeta-Smad2/3 axis switches pro- to anti-apoptotic signaling in tumor-residing pericytes, assisting tumor growth. Cell Death Differ (2021) 28(11):3052–76. doi: 10.1038/s41418-021-00801-3
84. Wang B, Zhang S, Tong F, Wang Y, Wei L. HPV(+) HNSCC-derived exosomal miR-9-5p inhibits TGF-beta signaling-mediated fibroblast phenotypic transformation through NOX4. Cancer Sci (2022) 113(4):1475–87. doi: 10.1111/cas.15281
85. Lan Y, Moustafa M, Knoll M, Xu C, Furkel J, Lazorchak A, et al. Simultaneous targeting of TGF-β/PD-L1 synergizes with radiotherapy by reprogramming the tumor microenvironment to overcome immune evasion. Cancer Cell (2021) 39(10):1388–403. doi: 10.1016/j.ccell.2021.08.008
86. Kim TW, Malek E, Choi SH, Ignatz-Hoover JJ, Driscoll JJ. Efficacy and safety of vactosertib and pembrolizumab combination in patients with previously treated microsatellite stable metastatic colorectal cancer. J Clin Oncol (2021) 39(15). doi: 10.1200/JCO.2021.39.15_suppl.3573
87. Yegodayev KM, Novoplansky O, Golden A, Prasad M, Levin L, Jagadeeshan S, et al. TGF-beta-activated cancer-associated fibroblasts limit cetuximab efficacy in preclinical models of head and neck cancer. Cancers (2020) 12(2):339. doi: 10.3390/cancers12020339
88. Ford K, Hanley CJ, Mellone M, Szyndralewiez C, Heitz F, Wiesel P, et al. NOX4 inhibition potentiates immunotherapy by overcoming cancer-associated fibroblast-mediated CD8 T-cell exclusion from tumors. Cancer Res (2020) 80(9):1846–60. doi: 10.1158/0008-5472.CAN-19-3158
89. Shimada K, Fujii T, Anai S, Fujimoto K, Konishi N. ROS generation via NOX4 and its utility in the cytological diagnosis of urothelial carcinoma of the urinary bladder. BMC Urol (2011) 11:22. doi: 10.1186/1471-2490-11-22
90. Yuan Z, Guo G, Sun G, Li Q, Wang L, Qiao B. Magnesium isoglycyrrhizinate suppresses bladder cancer progression by modulating the miR-26b/Nox4 axis. Bioengineered (2022) 13(4):7986–99. doi: 10.1080/21655979.2022.2031677
91. Batlle E, Massague J. Transforming growth factor-beta signaling in immunity and cancer. Immunity (2019) 50(4):924–40. doi: 10.1016/j.immuni.2019.03.024
92. Sampson N, Brunner E, Weber A, Puhr M, Schäfer G, Szyndralewiez C, et al. Inhibition of Nox4-dependent ROS signaling attenuates prostate fibroblast activation and abrogates stromal-mediated protumorigenic interactions. Int J Cancer (2018) 143(2):383–95. doi: 10.1002/ijc.31316
93. Kato R, Haratani K, Hayashi H, Sakai K, Sakai H, Kawakami H, et al. Nintedanib promotes antitumour immunity and shows antitumour activity in combination with PD-1 blockade in mice: potential role of cancer-associated fibroblasts. Br J Cancer (2021) 124(5):914–24. doi: 10.1038/s41416-020-01201-z
94. Hussain SA, Lester JF, Jackson R, Gornall M, Qureshi M, Elliott A, et al. Addition of nintedanib or placebo to neoadjuvant gemcitabine and cisplatin in locally advanced muscle-invasive bladder cancer (NEOBLADE): a double-blind, randomised, phase 2 trial. Lancet Oncol (2022) 23(5):650–8. doi: 10.1016/S1470-2045(22)00158-9
95. Wang LCS, Lo A, Scholler J, Sun J, Majumdar RS, Kapoor V, et al. Targeting fibroblast activation protein in tumor stroma with chimeric antigen receptor T cells can inhibit tumor growth and augment host immunity without severe toxicity. Cancer Immunol Res (2014) 2(2):154–66. doi: 10.1158/2326-6066.CIR-13-0027
96. Lo A, Wang LS, Scholler J, Monslow J, Avery D, Newick K, et al. Tumor-promoting desmoplasia is disrupted by depleting FAP-expressing stromal cells. Cancer Res (2015) 75(14):2800–10. doi: 10.1158/0008-5472.CAN-14-3041
97. Özdemir BC, Pentcheva-Hoang T, Carstens JL, Zheng X, Wu CC, Simpson TR, et al. Depletion of carcinoma-associated fibroblasts and fibrosis induces immunosuppression and accelerates pancreas cancer with reduced survival. Cancer Cell (2014) 25(6):719–34. doi: 10.1016/j.ccr.2014.04.005
98. Rhim AD, Oberstein PE, Thomas DH, Mirek ET, Palermo CF, Sastra SA, et al. Stromal elements act to restrain, rather than support, pancreatic ductal adenocarcinoma. Cancer Cell (2014) 25(6):735–47. doi: 10.1016/j.ccr.2014.04.021
99. Chen Y, Kim J, Yang S, Wang H, Wu CJ, Sugimoto H, et al. Type I collagen deletion in αSMA+ myofibroblasts augments immune suppression and accelerates progression of pancreatic cancer. Cancer Cell (2021) 39(4):548–65. doi: 10.1016/j.ccell.2021.02.007
100. Shin K, Lim A, Zhao C, Sahoo D, Pan Y, Spiekerkoetter Ec, et al. Hedgehog signaling restrains bladder cancer progression by eliciting stromal production of urothelial differentiation factors. Cancer Cell (2014) 26(4):521–33. doi: 10.1016/j.ccell.2014.09.001
101. Mizutani Y, Iida T, Ohno E, Ishikawa T, Kinoshita F, Kuwatsuka Y, et al. Safety and efficacy of MIKE-1 in patients with advanced pancreatic cancer: a study protocol for an open-label phase I/II investigator-initiated clinical trial based on a drug repositioning approach that reprograms the tumour stroma. BMC Cancer (2022) 22(1):205. doi: 10.1186/s12885-022-09272-2
102. Ferrer-Mayorga G, Gómez-López G, Barbáchano A, Fernández-Barral A, Peña C, Pisano DG, et al. Vitamin d receptor expression and associated gene signature in tumour stromal fibroblasts predict clinical outcome in colorectal cancer. Gut (2017) 66(8):1449–62. doi: 10.1136/gutjnl-2015-310977
103. Sherman MH, Yu RT, Engle DD, Ding N, Atkins AR, Tiriac H, et al. Vitamin d receptor-mediated stromal reprogramming suppresses pancreatitis and enhances pancreatic cancer therapy. Cell (2014) 159(1):80–93. doi: 10.1016/j.cell.2014.08.007
104. Syed M, Flechsig P, Liermann J, Windisch P, Staudinger F, Akbaba S, et al. Fibroblast activation protein inhibitor (FAPI) PET for diagnostics and advanced targeted radiotherapy in head and neck cancers. Eur J Nucl Med Mol Imaging (2020) 47(12):2836–45. doi: 10.1007/s00259-020-04859-y
Keywords: cancer-associated fibroblast, muscle-invasive bladder cancer, bladder cancer, caf, immunotherapy, radiotherapy
Citation: Burley A, Rullan A and Wilkins A (2022) A review of the biology and therapeutic implications of cancer-associated fibroblasts (CAFs) in muscle-invasive bladder cancer. Front. Oncol. 12:1000888. doi: 10.3389/fonc.2022.1000888
Received: 22 July 2022; Accepted: 22 September 2022;
Published: 13 October 2022.
Edited by:
Rong Na, The University of Hong Kong, Hong Kong, SAR ChinaReviewed by:
Fernando Calvo, Spanish National Research Council (CSIC), SpainStéphane Chabaud, Centre de Recherche du CHU de Québec, Canada
Doug Ward, University of Birmingham, United Kingdom
Copyright © 2022 Burley, Rullan and Wilkins. This is an open-access article distributed under the terms of the Creative Commons Attribution License (CC BY). The use, distribution or reproduction in other forums is permitted, provided the original author(s) and the copyright owner(s) are credited and that the original publication in this journal is cited, in accordance with accepted academic practice. No use, distribution or reproduction is permitted which does not comply with these terms.
*Correspondence: Anna Wilkins, YW5uYS53aWxraW5zQGljci5hYy51aw==
†These authors have contributed equally to this work and share senior authorship