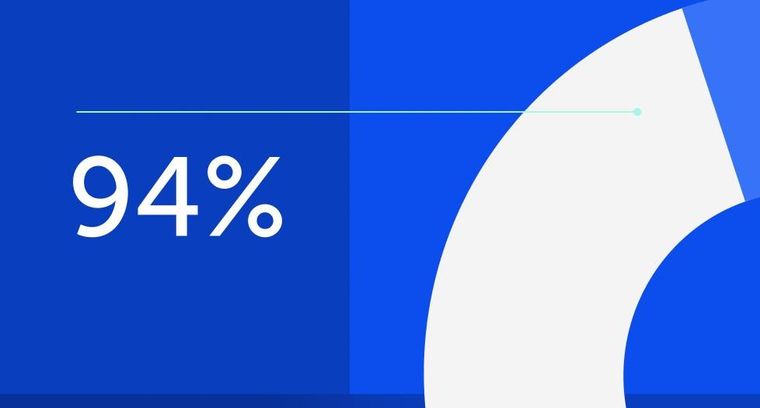
94% of researchers rate our articles as excellent or good
Learn more about the work of our research integrity team to safeguard the quality of each article we publish.
Find out more
REVIEW article
Front. Oncol., 19 October 2021
Sec. Gastrointestinal Cancers: Gastric and Esophageal Cancers
Volume 11 - 2021 | https://doi.org/10.3389/fonc.2021.748331
This article is part of the Research TopicInsights in Gastrointestinal Cancers: 2021View all 16 articles
Radiation therapy (RT) is an integral component of potentially curative management of esophageal cancer (EC). However, RT can cause significant acute and late morbidity due to excess radiation exposure to nearby critical organs, especially the heart and lungs. Sparing these organs from both low and high radiation dose has been demonstrated to achieve clinically meaningful reductions in toxicity and may improve long-term survival. Accruing dosimetry and clinical evidence support the consideration of proton beam therapy (PBT) for the management of EC. There are critical treatment planning and delivery uncertainties that should be considered when treating EC with PBT, especially as there may be substantial motion-related interplay effects. The Particle Therapy Co-operative Group Thoracic and Gastrointestinal Subcommittees jointly developed guidelines regarding patient selection, treatment planning, clinical trials, and future directions of PBT for EC.
Chemoradiotherapy (CRT), delivered either preoperatively or definitively, is critical for the management of locally advanced esophageal cancer (EC) (1, 2). Because of the central anatomic location of the esophagus, organs at risk (OARs) within the chest and upper abdomen receive unintended radiation dose to potentially large volumes when treating with x-ray therapy (XRT), and this may lead to serious acute and/or late toxicities. As such, more conformal XRT techniques like intensity-modulated radiation therapy (IMRT) have been shown to potentially improve clinical outcomes including overall survival (OS) by reducing heart dose and the risk of cardiac death compared to 3D conformal radiation therapy (3DCRT) (3).
For nearly all cases, proton beam therapy (PBT) significantly reduces normal organ dose compared with 3DCRT and IMRT. Proton beams carry charged particles that have relatively low doses in the path proximal to the tumor and deposit most of their energy around the end of its path, called the Bragg peak, the depth of which is determined by the specific energy imparted to the protons, while the OARs beyond the tumor receive essentially no dose. In contrast, the interaction of an x-ray beam within tissue has a relatively superficial dose build-up region and then exponential reduction in dose with increasing depth. Given the excellent conformality of both modern PBT and XRT, the difference in dose to normal tissues is most pronounced at low and moderate levels rather than higher doses at or near prescription dose.
The dosimetric advantages of PBT versus XRT were first demonstrated using the passive scattering (PS) technique, in which apertures and compensators shape the diverging proton beam to achieve appropriate target conformality laterally and distally, respectively. However, a limitation of PS-PBT is reduced conformality proximal to the target. Pencil beam scanning, also commonly referred to as intensity-modulated proton therapy (IMPT), is a modern technique in which “spots” of protons are directed by steering magnets across multiple dose layers, achieving excellent conformality including proximal to the target. Despite these dosimetric advantages, there are a number of PBT planning and delivery uncertainties that should be considered and mitigated using thoughtful treatment planning and delivery techniques.
Prospective and retrospective studies have demonstrated that PBT for EC is well tolerated and clinical benefit may be achieved by significantly reducing normal organ dose (4). With mounting clinical evidence in support of PBT for EC, coupled with an increasing number of PBT centers worldwide, a standardized approach of robust PBT planning and treatment delivery is needed. To meet this growing need, the Particle Therapy Co-Operative Group (PTCOG) Thoracic and Gastrointestinal Subcommittees have jointly generated evidence-based PBT guidelines for EC, highlighting the supporting clinical evidence and recommended treatment planning approaches.
The central anatomic location of the thoracic esophagus makes PBT particularly attractive for reducing normal organ dose (Table 1). In 2008, Zhang et al. were among the first to demonstrate that thoracic OARs could be better spared with PBT as compared to XRT while maintaining excellent target coverage (5). Subsequent comparisons have demonstrated that PBT consistently achieves ≥30%–60% relative reductions in mean heart dose and ≥30%–60% relative reductions in heart V20–V40 compared to IMRT or 3DCRT (6–17). Moreover, PBT achieves ≥40%–60% relative reduction in mean lung dose and ≥30%–50% relative reduction in lung V20. For example, in a study of 55 patients planned to 50.4 Gy in 28 fractions with PS-PBT (typically using a posterior beam and a left lateral beam, weighted 2:1) or IMRT, the PBT plans resulted in significantly lower mean dose to the heart (13.0 vs. 19.9 Gy) and lung (6.3 vs. 9.3 Gy) (8). However, because of the 3D planning approach used for PS-PBT, heart V40 was higher with PS-PBT versus IMRT, owing to the greater conformality index of IMRT.
Table 1 Select dosimetric analyses of proton beam therapy versus intensity modulated radiation therapy for esophageal cancer.
IMPT offers improved conformality over PS-PBT with reduction in higher dose to normal tissues. Shiraishi et al. evaluated dosimetric outcomes in 727 EC patients who received PS-PBT (n = 237), IMPT (n = 13), or IMRT (n = 477) (9). IMPT was associated with significantly lower dose to the heart and various cardiac substructures (left atrium, right atrium left main coronary artery, left circumflex artery) compared to PS-PBT.
In addition to heart and lung sparing, PBT also markedly reduces liver dose compared to XRT. In an evaluation of 10 patients with distal EC who were prescribed 50.4 Gy in 28 fractions, the mean liver dose was 3.6 Gy with PS-PBT compared to 18.1 Gy with IMRT (p = 0.001) and 20.3 Gy with 3DCRT (p = 0.001) (7). Other studies have consistently reported relative mean liver dose reductions of at least 60%–80% and mean liver doses of approximately 5 Gy or less (6, 7, 10).
PBT beam arrangement is an important consideration when evaluating dosimetric differences compared to XRT, especially with respect to the heart and lungs. Welsh et al. evaluated three PBT arrangements (AP/PA, LPO/RPO, and AP/LPO/RPO) and found that the most significant heart sparing was achieved using an LPO/RPO approach (mean: 11.9 vs. 21.2 Gy; V30: 17% vs. 25%), while much smaller reductions were observed when PBT was planned using AP/PA beams (mean: 19.9 vs. 21.2 Gy; V30: 23% vs. 25%) (6). On the other hand, PBT plans using AP/PA beams achieved substantially lower lung dose than LPO/RPO plans (mean: 3.2 vs. 8.3 Gy; V20: 7% vs. 14%). These findings are supported by an analysis from Shiraishi et al. in which certain beam arrangements (especially AP/PA) were associated with high mean heart dose on multivariable linear regression analysis (9). Superior–inferior posterior beams may provide better heart, lung, and liver sparing than LPO/RPO beams (18). Thus, clinical judgment should be used to guide PBT treatment planning with regard to prioritizing OAR sparing and achieving the most clinically appropriate dosimetry for each patient.
The published literature describing clinical outcomes of PBT has expanded over the past decade, including both prospective and retrospective evidence that EC patients receive clinically meaningful benefit (Table 2) (4, 13, 19, 23, 24).
Table 2 Select studies of clinical outcomes comparing proton beam therapy vs. x-ray therapy for esophageal cancer.
In a retrospective study compiling data from three institutions comparing neoadjuvant 3DCRT, IMRT, or PBT with concurrent chemotherapy before esophagectomy, PBT was associated with lower rates of pulmonary and wound healing complications (19). Length of hospitalization was significantly reduced in the PBT group as compared to the XRT cohort, likely a result of reduced postoperative complications. While acute cardiac events were greater in the 3DCRT group, there were no differences between PBT and IMRT. A recent study analyzed cardiovascular events in 479 EC patients treated using IMRT or PBT, in which 18% of patients developed major grade 3+ cardiovascular events with a median follow up of 76 months. Cardiovascular events occurred at a median of 7 months after CRT, the majority of which (81%) occurred within the first 2 years after completing CRT (24). The strongest factors associated with increased risk of grade 3+ events were pre-existing cardiovascular disease and the use of IMRT (vs. PBT). Among patients with pre-existing heart disease, the use of PBT was associated with a significantly lower event rate at 2 years compared to IMRT (11 vs. 30%; p = 0.0018). In addition, a prospective registry study of 125 patients with EC receiving CRT, patients receiving PBS-PT (vs. IMRT) had better preservation of health-related quality of life as assessed by the Functional Assessment of Cancer Therapy-Esophagus (FACT-E) questionnaire during CRT (20).
PBT is expected to deliver radiation dose to a lower volume of the total blood pool compared to XRT, and because of the exquisite radiosensitivity of circulating lymphocytes, this difference may be clinically significant (25, 26). For example, a propensity-matched analysis by Shiraishi and colleagues for 480 EC patients demonstrated a markedly higher incidence of grade 4 lymphopenia among patients receiving IMRT compared to PBT (40.4% vs. 17.6%; p < 0.0001) (27). Investigators from the Mayo Clinic more recently presented similar findings showing a strong association between reduced severe lymphopenia with PBT (28).
Recently, investigators at MD Anderson Cancer Center published results of a phase 2 randomized trial conducted comparing PBT (80% PS-PBT, 20% IMPT) and IMRT (29). The study co-primary endpoints were progression-free survival (PFS) and a novel endpoint called total toxicity burden (TTB), which is a composite index of 11 distinct toxicities of varying grades from the start of CRT up to 1 year. Although the study closed early with 107 patients, it showed a significant improvement in the overall TTB score for PBT by 2.5-fold and a reduction in TTB by 7.6-fold for postoperative complications, without a difference in PFS or OS. This is the first proton versus photon randomized trial across all disease sites with a positive primary endpoint favoring PBT (30). NRG-GI006 (NCT03801876) is a phase 3 randomized controlled trial comparing PBT and IMRT for EC that is currently enrolling patients with the hypothesis that dosimetric advantages of PBT will translate into meaningful clinical benefit. Other prospective clinical trials are ongoing as summarized in Table 3.
For patients with recurrent or de novo EC occurring in the context of prior thoracic RT reirradiation can be considered, although the risks of severe late adverse effects can be significant (31). PBT is expected to achieve a potentially large reduction in cumulative doses to critical OARs, including the spinal cord, heart, lungs, proximal bronchial tree, and liver (32–34). Several cohort studies have demonstrated feasibility and encouraging early clinical outcomes with PBT reirradiation for EC (Table 4) (31, 33, 35, 36). Most recently, DeCesaris et al. reported outcomes in a retrospective cohort of 37 patients treated with PBT reirradiation at four institutions with a median reirradiation dose of 50.4 Gy and median cumulative dose of 104 Gy (37). Most patients (90%) received concurrent chemotherapy. With a median follow-up of 20 months after reirradiation, the 18-month OS was 56% and the 18-month locoregional control rate was 69%. Late grade 3 toxicity was observed in 24% consisting of strictures/stenosis requiring dilation, while some patients experienced late grade 4–5 toxicities (19%).
Patients with cervical esophagus, thoracic esophagus (upper, middle, or lower), and gastroesophageal junction cancers may be considered to receive PBT. PBT is expected to offer patients clinical benefit when used preoperatively, definitively, or postoperatively.
PBT should be most strongly considered in the following situations:
● Treatment is delivered with curative intent, where greater benefit from mitigation of late toxicity is expected compared to patients treated with palliative intent.
● Patients who have severe medical comorbidities, especially cardiac and/or pulmonary, because of superior heart and lung sparing compared to XRT.
● While patients of all ages are likely to benefit from a lower risk of significant late toxicities with PBT versus XRT, elderly patients who are often at higher risk of treatment-related morbidity and postoperative complications may especially benefit from the superior OAR sparing of PBT (38).
● For patients with local and/or regional recurrence of EC, or newly diagnosed EC arising in a previously irradiated region, PBT should be strongly considered over IMRT especially if treatment intent is curative (31). The American Society for Radiation Therapy (ASTRO) Model Policy for PBT considers re-irradiation (where cumulative critical structure dose would exceed tolerance dose) to be a “Group 1 indication” in which PBT is considered “medically necessary” (39). Care should be taken to evaluate composite dose in an attempt to mitigate the risks of severe toxicity including fistula and hemoptysis, as well as grade 5 events.
● PBT should be considered when dose escalation is used because it may mitigate higher risks of toxicities to critical OARs (40).
PBT may be reasonable although it should be used cautiously in the following situations:
● Extensive tumor involvement of the gastric cardia/body (tumor extending ≥5 cm distal to the gastroesophageal junction) may cause potentially sizeable inter- and intra-fractional differences in tumor position resulting in potential geometric miss.
● Variability in stomach filling (air versus fluid) and respiratory motion causing interplay effects should be mitigated, especially when using IMPT (41).
● Use of PBT for patients with pacemakers is considered a relative contraindication, especially for those who are pacemaker dependent, due to concern about neutron dose to the device and risk of subsequent device malfunctioning (42). However, such patients may be treated safely in the context of a well-defined plan for monitoring device function and responding to potential device dysfunction during the course of treatment. Such a plan requires close collaboration with colleagues in cardiology, and preferential use IMPT (vs. PS-PBT) if possible due to lower neutron dose.
Prior to simulation, the physician, physicist, dosimetrist, and simulation therapists should discuss the anticipated treatment volume, immobilization technique, and consideration of internal target motion due to respiration and gastric distention. General guidelines include instructing the patient to have a relatively empty stomach before simulation/treatment (if possible) to limit variability in gastric filling and distention. This can be achieved by patients having nothing by mouth (NPO) 2–3 h prior to simulation and treatment in addition to avoiding foods that may cause excess gas. It is preferred to not use oral contrast to minimize stomach distention, and gastrointestinal luminal structures are often well visualized without contrast agents. If oral contrast is used, then a non-contrast-enhanced CT scan should first be performed for treatment planning as contrast material has a significant impact on calculating proton beam range; a second CT scan would then be obtained after contrast is administered, which would serve as a secondary scan for target delineation purposes.
In most situations, the preferred treatment position is head first, supine, and with the arms up above the head in a custom immobilization device. There are some situations in which arms may be placed at the patient’s side, such as patient intolerance of an “arms up” position or a tumor in the cervical or upper thoracic esophagus in which a thermoplastic head and shoulder immobilization device may be preferred. An “arms down” position is a reasonable alternative for patients treated with PBS, as the typical beam arrangement of posterior/posterior oblique fields avoids the arms. However, if there is gastric extension of the tumor (in which the target volume will extend significantly left of midline), the left arm may be in the path of a left posterior oblique beam. Additional potential technical issues created with the “arms down” position, especially for larger patients, include CT beam hardening artifacts from the arms and difficulty including the entire external body surface within the scan field of view. A full body immobilization device or pad under the patient’s back may be considered to improve setup reproducibility and/or patient comfort, although attention should be paid to potential uncertainty in proton stopping power along the beam path. Immobilization of the hips and lower extremities may help facilitate reproducible alignment of the spine.
A non-contrast, free-breathing four-dimensional CT (4DCT) scan should be acquired for treatment planning. Patients may be treated with a free breathing, internal gross tumor volume (iGTV) approach, assuming appropriate motion-robust planning methods are used, as outlined in the next section. Using a breath hold technique may be appropriate for some patients, and if this is to be used, multiple breath hold scans should be acquired to ensure reproducibility of this technique. Some centers have utilized treatment under mechanical ventilation to control breathing variations and reduce the breathing amplitude. In this case, 4DCT imaging should be performed for the same mechanical ventilation situation as intended for treatment. The scan volume should encompass the entire external body surface and immobilization device in the x- and y-planes and should include the entire lungs and kidneys in the z-plane for dose reporting to these organs. If the upper mediastinum and cervical lymph nodes are to be treated, the scan should also include the full neck to the skull base. The CT scan/reconstruction slice thickness should be ≤3 mm. Intravenous (IV) contrast may be administered to aid in target delineation, although this should be done after acquisition of a non-contrast CT for planning/dose calculation.
Normal tissue and target delineation should be performed on the non-contrast 4DCT data set. Typically, the CT average series will be utilized for segmentation and planning, as this best represents the time-averaged tissue densities and proton stopping power ratio, especially in the region of the diaphragm. Alternatively, the maximum exhalation series (diaphragm at its most cranial position) may be utilized. The planning scan should be registered with the diagnostic positron emission tomography (PET)/CT to aid in target delineation. Additionally, the esophagogastroduodenoscopy (EGD) report(s) should be reviewed for correlation of tumor extent with imaging studies.
Target delineation for the management of EC is similar whether using photons or protons and should be performed according to published guidelines (43). The gross tumor volume (GTV) should include the primary tumor (GTVp) and involved lymph nodes (GTVn), based on the planning CT, PET/CT, and EGD reports. An iGTV should be contoured, if treatment will be with free breathing, including the GTV on all respiratory phases of the 4DCT or using maximal intensity projection (MIP) images and edited through phases. The clinical target volume (CTV) typically includes up to a 3- to 4-cm expansion of the GTVp along the proximal and distal esophagus/stomach to cover potential microscopic mucosal and submucosal spread. This volume is typically further expanded by 1–1.5 cm radially from the esophagus/stomach to cover potential periesophageal and perigastric lymphatic spread, excluding uninvolved OARs like the heart, lungs, and spine. The CTV also includes a 0.5- to 1-cm expansion of the GTVn, excluding uninvolved OARs. Elective lymph node basins (celiac, gastrohepatic, para-aortic for lower esophagus, and GEJ tumors; supraclavicular for upper esophagus tumors) are typically included in the CTV. The CTV may be further modified based on the 4DCT, generating an internal target volume (ITV) to account for respiratory motion.
Typical prescribed doses are 41.4–50.4 Gy (RBE 1.1) in 23–28 fractions of 1.8–2 Gy for preoperative treatment, 50–50.4 Gy (RBE 1.1) in 25–28 fractions of 1.8–2 Gy for definitive treatment, and 50–60 Gy (RBE 1.1) in 25–30 fractions of 1.8–2 Gy for postoperative treatment. The prescription of higher doses is used at some institutions, especially outside of North America, although this is controversial since no randomized data have demonstrated a clinical benefit for dose escalation (44).
Dose painting techniques may be utilized that deliver differential daily dose to separate volumes. For example, some institutions administer 25 fractions, with a dose of 50 Gy (2 Gy/fraction) to the iGTV + 1 cm margin and a dose of 45 Gy (1.8 Gy/fraction) to the typical CTV described above.
Zhang et al. performed a comparative planning study of two-beam PSPT (AP/PA), three-beam PSPT (AP/LPO/RPO), and IMRT for distal esophageal or GEJ cancer (5). While lung sparing was improved using an AP/PA beam arrangement, there was a trade-off with increased heart dose. As such, preferring posterior-oriented beams should be considered to reduce heart dose. Given the increasing clinical evidence that minimizing heart dose should be prioritized, the LPO/RPO beam arrangement has been adopted as the standard clinical class solution for PSPT plan design at many centers, which is believed to strike an appropriate balance between lung and heart sparing compared to AP/PA and 3-beam PSPT approaches.
Major planning parameters for PSPT include aperture margins, distal margins, proximal margins, smearing margins, and border smoothing margins, and should be chosen for each individual beam once the beam angles are decided for a given treatment plan. The beam-specific target can be created using the target (ICTV) expanded with distal and proximal margin in the beam direction determined by range uncertainty and lateral margin due to setup uncertainty (ICTV to PTV expansion margin, typically 5 mm), as opposed to using a PTV for forward planning (45). Zhang et al. discussed the choice of these planning parameters, based on the method suggested by Moyers et al. (5, 46). Zhang et al. used an aperture margin to ensure that all the proton beams had at least 95% of the PTV receiving the prescription dose, whereas the distal and proximal were 3.5% of the distal and proximal range of the spread-out Bragg peak (SOBP) plus an additional 2–3 mm for other uncertainties. The smearing margin accounts for both the setup error and proton scatter to ensure distal coverage if there is setup error. The value of the smearing margin could initially use the methods described by Moyers et al. and then be adjusted to ensure the beam specific target is covered in average, maximum inhale phase (T0) and patient-specific maximum exhale phase (TExp).
After the treatment plan is designed based on the average CT, the treatment plan should be recalculated and evaluated on the T0 and TExp of the CT image data set. Diaphragm density overrides, which have been adopted from XRT, can be considered although may not be necessary in some patients to achieve planning and inter-fractional robustness. Although a PTV is only used for the lateral margins of the treatment plan design, the PTV should be used for the evaluation following the recommendation of ICRU 78 (47). The planning parameters including distal margin, proximal margin, and smearing and aperture margins should be adjusted to ensure proper coverage for GTV, ICTV, and PTV on average, T0, and TExp CTs (48).
Yu et al. reported a water equivalent thickness (WET)-based method to select IMPT beam angles that are robust to respiratory motion for EC treatment, and in specific, diaphragmatic motion (49). Motion robust beam angles were determined by examining the change of WET along the beam path required to cover the target during a full cycle of free-breathing motion at various angles. The beam angles that yielded the smallest value of the maximum temporal change of WET were considered to be the most robust to respiratory motion. The most motion robust beam angles are generally posterior with a median gantry angle of 200°C (range, 180°C–220°C) and couch at 0°C, because these beam angles pass through a relatively less mobile portion of the diaphragm. This choice of beam angles is also optimal from the point of view of avoiding organs with variable filling proximal to the target, such as the stomach. Another consideration could be superior–inferior posterior oblique beams with the couch at 270°C, which may provide better sparing of normal organs lateral to the target at the expense of delivering higher dose to the spinal cord (18). Accordingly, most centers treating distal esophagus/GEJ tumors with IMPT have used two to three posterior oriented beams (18, 20, 33, 50). For treatment of tumors in the cervical and proximal thoracic esophagus, an anterior beam should be considered to reduce lung dose.
Various PBT planning strategies can effectively mitigate the effect of respiratory motion and achieve robust plans. For example, use of larger spot size and rescanning/repainting could reduce the effect of motion (51). For distal/GEJ tumors, the diaphragm is usually not part of the target, although it is likely to traverse in and out of the treatment field and could cause significant interplay effects on dose delivery if not properly taken into account. With motion robust beam angles, a high-quality PBS plan can be generated using a single-field optimization (SFO) technique for most EC patients (49). Similar to the PS-PBT plan design, beam-specific targets could be designed for each beam for SFO treatment planning. The optimization algorithm should ensure that the beam-specific target has the adequate coverage for each beam. Yu et al. suggested that a multi-field optimization (MFO) technique could result in a more conformal plan compared to SFO, but the MFO technique is more sensitive to setup, range, and motion uncertainties. Regardless, robust optimization can achieve robust SFO and MFO plans (10, 52, 53). Planning criteria that include appropriate range uncertainties, along with setup uncertainties compatible to the PTV margins, should be applied to CTV dose coverage objectives. Robust optimization can also be applied on high-risk OARs such as the spinal cord, using the same robustness criteria, if such OARs are expected to receive near their maximum tolerance doses. If the plan involves MFO and/or split target volumes, care must be taken to assure that appropriate dose fall-off gradients are introduced in the field or target sub-volume junction regions to assure junction line dose homogeneity in the presence of setup errors and respiratory motion. In addition, a 4D optimization technique (54), for example, where the IMPT plan is optimized to meet dose constraint on multiple 4DCT phases (55), could be used to create motion robust treatment plans. Regardless of optimization technique that was used for plan design, it is important to perform robustness evaluation with regard to setup, range and motion (52). A commonly used technique for setup and range robustness evaluation is the worst-case evaluation where different scenarios include shifted isocenter of the plan on all cardinal directions, and the beam range modified with assumed max range uncertainty. Dose is then calculated and evaluated on all scenarios to identify the worst case for all plans or all voxels in the patient. Additionally, motion robustness could be evaluated using the recalculated dose on the 4D CT scans at T0 and TExp with the original plan (56). Dose on the robustness evaluation plans should meet the planning criteria for GTV, CTV, and OARs as defined on individual data sets. Yu et al. demonstrated that D95 variation between the nominal dose calculated on the average CT and the dose distribution on T0/TExp verification plans highly correlates with D95 variation between the nominal dose and the full 4D dose calculation (49). These results indicated that dose impact of respiratory motion could be evaluated using verifications on T0 and TExp. Ideally, for a comprehensive robustness analysis, all uncertainty components should be evaluated in combination as suggested by Ribeiro et al. (57).
Figure 1 shows a comparison between IMRT, PS-PBT, and IMPT plans for a distal EC patient. The PS-PBT plan uses two PA/LPO, the IMPT plan uses three posterior oblique beams, whereas IMRT uses two arcs. It can be observed that both PS-PBT and IMPT plans better spare OARs including lung, heart, and liver, while maintaining target coverage. In addition, IMPT achieves better conformity of the target and lower mean dose to the lungs, compared to PS-PBT.
Figure 1 Dosimetric comparison of treatment plans using all three modalities for a patient with distal esophageal cancer. VMAT, Volumetric Arc Therapy; PSPT, Passive Scattering Proton Therapy; IMPT, Intensity Modulated Proton Therapy; MHD, Mean Heart Dose; MLuD, Mean Lung Dose; MLiD, Mean Liver Dose.
The motion management strategy for EC should be similar to those used for treating lung cancers (41). Here, we focus on special considerations of motion management for EC, namely, the impact of respiratory motion.
For treatment planning, the following should be considered:
1. Select robust beam angles that yield the smallest value of the maximum temporal WET change, typically posterior-oriented beams.
2. Adopt a robust optimization strategy. 4D robust optimization is recommended to design PBS treatment plans because it takes density changes along the beam path into consideration.
3. Perform motion evaluation to quantify the geometry motion and voxel WET changes. Diaphragm breathing amplitudes and off-sets can be used as EC motion surrogates to some extent (58). However, to evaluate EC motion in more detail, considering also differential motion, more sophisticated methods (for example, based on deformation vector fields) have to be developed. Further work is required to establish motion mitigation guidelines based on concrete motion limits advising on planning and delivery strategies as, for example, the use of larger spot size (e.g., with range shifters), breath hold, mechanical ventilation, abdominal compression, robust planning, and re-scanning.
For treatment delivery, the following strategies are recommended:
1. For IMPT, use rescanning (either layered or volumetric) to reduce interplay effects.
2. For IMPT, use an optimized delivery sequence, including scanning direction and breath sampling, to minimize interplay effects (52, 59).
3. Use breath hold, mechanical ventilation, abdominal compression, or gating techniques if other strategies are not sufficient to reduce the interplay effect.
4. Use daily image guidance with at least kilovoltage (kV) x-ray imaging. It is strongly recommended that cone beam CT (CBCT), if available, be used at least once weekly to ensure appropriate soft tissue reproducibility.
5. Perform routine quality assurance (QA)/verification CT scans to determine whether adaptive replanning in order to ensure robustness (52). It is recommended that such scans be done at least once during the first 2 weeks of treatment, and then again during the third or fourth week, recognizing that this is subject to patient- and center-specific factors.
Similar to other disease sites, patient-specific QA for EC patients includes various components, plan evaluation, robustness analysis with respect to range and setup uncertainties and motion interplay effect, and measurements in phantoms. Measurements of dose distributions of individual fields for IMPT are challenging because of the complex dose distributions of the treatment fields. Measurements are commonly made with a two-dimensional ion chamber array detector, and 3D detectors are being developed (60). Like other sites in thorax and abdomen, motion interplay effects and the effectiveness of motion mitigation strategies for EC patients should be considered as part of the patient QA process, although they remain in development (21). From treatment planning, one can evaluate the verification plans on the T0 and TExp phases of the 4DCT image data set to estimate systematic errors caused by motion interplay effect. Ideally, one should use a 4D phantom to measure the dose distribution to assess the motion interplay effect. This should be done during commissioning if not every patient. However, the challenge is the lack of any commercially available standardized 4D phantom. Recently, efforts have been made to optimize QA workflows. Efforts such as those described by Meijers et al. basing patient specific quality assurance on independent dose calculation and predicted outcomes should find broader application in the future (61).
To assess the impact of variation in anatomy and tumor size, patients should undergo repeat 4D CT verification scans to determine whether offline adaptive replanning is needed to maintain target coverage (e.g., >95% for the ICTV) and to avoid overdosing critical structures (52). 4D magnetic resonance imaging has become available for motion verification, with advantages over CT being superior soft tissue contrast, no imaging dose, and longer data sampling interval. In-room volumetric imaging techniques such as CBCT and in-room CT could also be used to identify possible anatomy changes. However, dose calculation on CBCT may not be sufficiently accurate. Therefore, verification 4D CT scans should be performed several times during the course of PBT to generate verification plans. If the patient is treated with breath hold technique, the verification CT scans should be performed using similar breath hold technique to verify consistency of diaphragm position and shape and resultant impact on dose distribution. 4D dose reconstruction and accumulation based on available repeated 4D images enables the clinical estimation of actual exhibited interplay and motion effects, facilitating an informed triggering of adaptive replanning (21). If an adaptive plan is deemed necessary, then it should be developed by using techniques as described earlier, and the same patient-specific quality assurance (PSQA) process should be repeated before treatment with the adapted plan. Similarly, if a phase-based gating strategy is utilized for treatment, then the same phases should be used for the verification plan. On the verification scans, careful attention should be paid to potential changes in external anatomy (from setup, immobilization, weight loss or gain), internal anatomy (tumor shrinkage or swelling, change in esophagus/stomach filling, presence/absence of an esophageal stent, presence/absence of pleural effusion, change in diaphragm position/shape), and motion pattern, which could require further systematic monitoring and timely adjustment of treatment plans. Adaptive PBT has the ability to correct for dosimetric effects induced by interfractional anatomic changes and it complements the ability of image guided setup to correct for setup uncertainty.
With increasing awareness that the immune system plays an integral role in cancer-related outcomes, attention should be directed at mitigating radiation-related effects especially on lymphocytes that are radiosensitive even to doses as low as <1 Gy (22). High-grade lymphopenia has been associated with poorer long-term outcomes among EC patients (25) and may be significantly reduced using PBT as compared to photon radiotherapy (26–28). Future consideration should be given to the development of lymphopenia-related organ-at-risk constraints for the heart, lungs, major vascular structures, bone marrow, and spleen that would be routinely incorporated into treatment plan optimization. The importance of mitigating RT-related severe lymphopenia, and thus the benefits or PBT, may become even greater should immune checkpoint inhibitors become standardly delivered for localized EC.
Although photon-based dose escalation studies have been negative for EC (44, 62), this concept could be re-explored in the context of reduced OAR dose afforded by PBT (63). As standard doses utilized for EC management lead to suboptimal rates of locoregional failures, employing proton therapy to better protect adjacent critical structures from unnecessary irradiation and resulting treatment-related morbidity and mortality may more safely allow for dose escalation (62), and a potential improvement in tumor control without added toxicity. This may be particularly important in patients managed without surgery with definitive radiation therapy.
The biologic effects of PBT within tumor and OARs are not well understood and warrant further study. Proton beams have a modestly higher radiobiological effectiveness (RBE) compared to photon beams; therefore, a correction factor of 1.1 is commonly applied to the absorbed dose. However, it is recognized that the RBE is variable over the depth dose curve, namely, it is higher in the region of the Bragg peak, and especially the distal end of the Bragg peak, due to higher LET (64). Because of this, PBT treatment plans have traditionally avoided beams that “range out” into a critical structure for fear of biologic dose enhancement. This may have implications for use of PBT for EC, and especially for PBS techniques, which utilize posterior beams that may “range out” into critical structures including the heart, stomach, and intestine. The clinical effects of this are currently unknown. Sophisticated planning techniques are being developed that allow one to visualize the location of high LET, which could be considered in the planning process and plan optimization that has the potential to further reduce toxicities and also improve tumor control with LET-based planning (Figure 2) (65–67).
Figure 2 Monte Carlo dose calculation for a patient with distal esophageal cancer treated with 5,000 cGy in 25 fractions using pencil beam scanning proton beam therapy with two posterior oblique fields. (A) shows physical dose in cGy, (B) shows linear energy transfer (LET) in keV/μm, and (C) shows modeled biological dose incorporating physical dose and LET. Note that the high LET region is distal to the target in the heart.
Lastly, because interplay effects especially with IMPT can significantly degrade dose distribution in the thorax (67), a PSQA process or similar approaches should routinely consider such effects (10). For IMPT, this can be done by simulating the temporal relationship between the time-dependent spot delivery and respiratory motion. Commerical treatment planning systems are expected to offer interplay effect evaluation in the future.
PBT should be strongly considered for trimodality and non-operative thoracic EC patients based on retrospective and randomized prospective data that demonstrate clinically meaningful reductions in toxicity compared to XRT. Robust PBT plan development and treatment delivery is critical to ensuring appropriate target and surrounding OAR dosimetry. Long-term toxicity and efficacy outcomes of PBT versus XRT are being evaluated in the ongoing NRG-GI006 phase 3 randomized trial (NCT03801876), and we encourage enrollment on that study.
MC, CH, CS, and SL contributed to the conception and design of the manuscript. All authors contributed to the article and approved the submitted version.
The authors declare that the research was conducted in the absence of any commercial or financial relationships that could be construed as a potential conflict of interest.
All claims expressed in this article are solely those of the authors and do not necessarily represent those of their affiliated organizations, or those of the publisher, the editors and the reviewers. Any product that may be evaluated in this article, or claim that may be made by its manufacturer, is not guaranteed or endorsed by the publisher.
We thank all members of the PTCOG Thoracic and Gastrointestinal Subcommittees for their review of this manuscript.
1. van Hagen P, Hulshof MCCM, van Lanschot JJB, Steyerberg EW, van Berge Henegouwen MI, Wijnhoven BPL, et al. Preoperative Chemoradiotherapy for Esophageal or Junctional Cancer. N Engl J Med (2012) 366:2074–84. doi: 10.1056/NEJMoa1112088
2. Tepper J, Krasna MJ, Niedzwiecki D, Hollis D, Reed CE, Goldberg R, et al. Phase Iii Trial of Trimodality Therapy With Cisplatin, Fluorouracil, Radiotherapy, and Surgery Compared With Surgery Alone for Esophageal Cancer: Calgb 9781. J Clin Oncol (2008) 26:1086–92. doi: 10.1200/JCO.2007.12.9593
3. Lin SH, Myles BH, Wang L, Thall PF, Vaporciyan AA, Mehran RJ, et al. Propensity Score–Based Comparison of Outcomes With 3d Conformal Radiotherapy (3d-Crt) Versus Intensity-Modulated Radiation Therapy (Imrt) in Treatment of Esophageal Cancer. J Clin Oncol (2012) 30:66–6. doi: 10.1200/jco.2012.30.4_suppl.66
4. Jethwa KR, Haddock MG, Tryggestad EJ, Hallemeier CL. The Emerging Role of Proton Therapy for Esophagus Cancer. J Gastrointest Oncol (2020) 11:144–56. doi: 10.21037/jgo.2019.11.04
5. Zhang X, Zhao K-L, Guerrero TM, McGuire SE, Yaremko B, Komaki R, et al. Four-Dimensional Computed Tomography-Based Treatment Planning for Intensity-Modulated Radiation Therapy and Proton Therapy for Distal Esophageal Cancer. Int J Radiat Oncol Biol Phys (2008) 72:278–87. doi: 10.1016/j.ijrobp.2008.05.014
6. Welsh J, Gomez D, Palmer MB, Riley BA, Mayankkumar AV, Komaki R, et al. Intensity-Modulated Proton Therapy Further Reduces Normal Tissue Exposure During Definitive Therapy for Locally Advanced Distal Esophageal Tumors: A Dosimetric Study. Int J Radiat Oncol Biol Phys (2011) 81:1336–42. doi: 10.1016/j.ijrobp.2010.07.2001
7. Ling TC, Slater JM, Nookala P, Mifflin R, Grove R, Ly AM, et al. Analysis of Intensity-Modulated Radiation Therapy (Imrt), Proton and 3d Conformal Radiotherapy (3d-Crt) for Reducing Perioperative Cardiopulmonary Complications in Esophageal Cancer Patients. Cancers (2014) 6:2356–68. doi: 10.3390/cancers6042356
8. Wang J, Palmer M, Bilton SD, Vu KN, Greer S, Frame R, et al. Comparing Proton Beam to Intensity Modulated Radiation Therapy Planning in Esophageal Cancer. Int J Particle Ther (2015) 1:866–77. doi: 10.14338/IJPT-14-00018.1
9. Shiraishi Y, Xu C, Yang J, Komaki R, Lin SH. Dosimetric Comparison to the Heart and Cardiac Substructure in a Large Cohort of Esophageal Cancer Patients Treated With Proton Beam Therapy or Intensity-Modulated Radiation Therapy. Radiother Oncol (2017) 125:48–54. doi: 10.1016/j.radonc.2017.07.034
10. Liu C, Bhangoo RS, Sio TT, Yu NY, Shan J, Chiang JS, et al. Dosimetric Comparison of Distal Esophageal Carcinoma Plans for Patients Treated With Small-Spot Intensity-Modulated Proton Versus Volumetric-Modulated Arc Therapies. J Appl Clin Med Phys (2019) 20:15–27. doi: 10.1002/acm2.12623
11. Makishima H, Ishikawa H, Terunuma T, Hashimoto T, Yamanashi K, Sekiguchi T, et al. Comparison of Adverse Effects of Proton and X-Ray Chemoradiotherapy for Esophageal Cancer Using an Adaptive Dose-Volume Histogram Analysis. J Radiat Res (2015) 56:568–76. doi: 10.1093/jrr/rrv001
12. Warren S, Partridge M, Bolsi A, Lomax AJ, Hurt C, Crosby T, et al. An Analysis of Plan Robustness for Esophageal Tumors: Comparing Volumetric Modulated Arc Therapy Plans and Spot Scanning Proton Planning. Int J Radiat Oncol Biol Phys (2016) 95:199–207. doi: 10.1016/j.ijrobp.2016.01.044
13. Xi M, Xu C, Liao Z, Chang JY, Gomez DR, Jeter M, et al. Comparative Outcomes After Definitive Chemoradiotherapy Using Proton Beam Therapy Versus Intensity Modulated Radiation Therapy for Esophageal Cancer: A Retrospective, Single-Institutional Analysis. Int J Radiat OncologyBiologyPhysics (2017) 99:667–76. doi: 10.1016/j.ijrobp.2017.06.2450
14. Hirano Y, Onozawa M, Hojo H, Motegi A, Zenda S, Hotta K, et al. Dosimetric Comparison Between Proton Beam Therapy and Photon Radiation Therapy for Locally Advanced Esophageal Squamous Cell Carcinoma. Radiat Oncol (2018) 13(1):1–9. doi: 10.1186/s13014-018-0966-5
15. Macomber MW, Bowen SR, Gopan O, Yeung R, Apisarnthanarax S, Zeng J, et al. Heart Dose and Outcomes in Radiation Treatment for Esophageal Cancer. Cureus (2018) 10:e2378. doi: 10.7759/cureus.2378
16. Celik E, Baus W, Baues C, Schröder W, Clivio A, Fogliata A, et al. Volumetric Modulated Arc Therapy Versus Intensity-Modulated Proton Therapy in Neoadjuvant Irradiation of Locally Advanced Oesophageal Cancer. Radiat Oncol (2020) 15:120. doi: 10.1186/s13014-020-01570-y
17. Zeng YC, Vyas S, Dang Q, Schultz L, Bowen SR, Shankaran V, et al. Proton Therapy Posterior Beam Approach With Pencil Beam Scanning for Esophageal Cancer : Clinical Outcome, Dosimetry, and Feasibility. Strahlenther Onkol (2016) 192:913–21. doi: 10.1007/s00066-016-1034-4
18. Feng H, Sio TT, Rule WG, Bhangoo RS, Lara P, Patrick CL, et al. Beam Angle Comparison for Distal Esophageal Carcinoma Patients Treated With Intensity-Modulated Proton Therapy. J Appl Clin Med Phys (2020). doi: 10.1002/acm2.13049
19. Lin SH, Merrell KW, Shen J, Verma V, Correa AM, Wang L, et al. Multi-Institutional Analysis of Radiation Modality Use and Postoperative Outcomes of Neoadjuvant Chemoradiation for Esophageal Cancer. Radiother Oncol (2017) 123:376–81. doi: 10.1016/j.radonc.2017.04.013
20. Garant A, Whitaker TJ, Spears GM, Routman DM, Harmsen WS, Wilhite TJ, et al. A Comparison of Patient-Reported Health-Related Quality of Life During Proton Versus Photon Chemoradiation Therapy for Esophageal Cancer. Pract Radiat Oncol (2019) 9:410–7. doi: 10.1016/j.prro.2019.07.003
21. Meijers A, Knopf AC, Crijns APG, Ubbels JF, Niezink AGH, Langendijk JA, et al. Evaluation of Interplay and Organ Motion Effects by Means of 4d Dose Reconstruction and Accumulation. Radiother Oncol (2020) 150:268–74. doi: 10.1016/j.radonc.2020.07.055
22. Trowell OA. The Sensitivity of Lymphocytes to Ionising Radiation. J Pathol Bacteriology (1952) 64:687–704. doi: 10.1002/path.1700640403
23. Lin SH, Hobbs BP, Verma V, Tidwell RS, Smith GL, Lei X, et al. Randomized Phase Iib Trial of Proton Beam Therapy Versus Intensity-Modulated Radiation Therapy for Locally Advanced Esophageal Cancer. J Clin Oncol (2020) 38:1569–79. doi: 10.1200/JCO.19.02503
24. Wang X, Palaskas NL, Yusuf SW, Abe J-I, Lopez-Mattei J, Banchs J, et al. Incidence and Onset of Severe Cardiac Events After Radiotherapy for Esophageal Cancer. J Thorac Oncol (2020) 15:1682–90. doi: 10.1016/j.jtho.2020.06.014
25. Wang X, Wang P, Zhao Z, Mao Q, Yu J, Li M. A Review of Radiation-Induced Lymphopenia in Patients With Esophageal Cancer: An Immunological Perspective for Radiotherapy. Ther Adv Med Oncol (2020) 12:1758835920926822. doi: 10.1177/1758835920926822
26. Davuluri R, Jiang W, Fang P, Xu C, Komaki R, Gomez DR, et al. Lymphocyte Nadir and Esophageal Cancer Survival Outcomes After Chemoradiation Therapy. Int J Radiat Oncol Biol Phys (2017) 99:128–35. doi: 10.1016/j.ijrobp.2017.05.037
27. Shiraishi Y, Fang P, Xu C, Song J, Krishnan S, Koay EJ, et al. Severe Lymphopenia During Neoadjuvant Chemoradiation for Esophageal Cancer: A Propensity Matched Analysis of the Relative Risk of Proton Versus Photon-Based Radiation Therapy. Radiother Oncol (2018) 128:154–60. doi: 10.1016/j.radonc.2017.11.028
28. Routman DM, Garant A, Lester SC, Day CN, Harmsen WS, Sanheuza CT, et al. A Comparison of Grade 4 Lymphopenia With Proton Versus Photon Radiation Therapy for Esophageal Cancer. Adv Radiat Oncol (2019) 4:63–9. doi: 10.1016/j.adro.2018.09.004
29. Kelly RJ, Ajani JA, Kuzdzal J, Zander T, Van Cutsem E, Piessen G, et al. Lba9_pr Adjuvant Nivolumab in Resected Esophageal or Gastroesophageal Junction Cancer (Ec/Gejc) Following Neoadjuvant Chemoradiation Therapy (Crt): First Results of the Checkmate 577 Study. Ann Oncol (2020) 31:S1193–4. doi: 10.1016/j.annonc.2020.08.2299
30. Simone CB 2nd. First Randomized Trial Supporting the Use of Proton Over Photon Chemoradiotherapy in Esophageal Cancer. J Clin Oncol (2020) 38:2952–5. doi: 10.1200/JCO.20.01405
31. Fernandes A, Berman AT, Mick R, Both S, Lelionis K, Lukens JN, et al. A Prospective Study of Proton Beam Reirradiation for Esophageal Cancer. Int J Radiat Oncol Biol Phys (2016) 95:483–7. doi: 10.1016/j.ijrobp.2015.12.005
32. Kim YS, Lee CG, Kim KH, Kim T, Lee J, Cho Y, et al. Re-Irradiation of Recurrent Esophageal Cancer After Primary Definitive Radiotherapy. Radiat Oncol J (2012) 30:182–8. doi: 10.3857/roj.2012.30.4.182
33. DeCesaris CM, McCarroll R, Mishra MV, Glass E, Greenwald BD, Carr S, et al. Assessing Outcomes of Patients Treated With Re-Irradiation Utilizing Proton Pencil-Beam Scanning for Primary or Recurrent Malignancies of the Esophagus and Gastroesophageal Junction. J Thorac Oncol (2020) 15:1054–64. doi: 10.1016/j.jtho.2020.01.024
34. Barsky AR, Reddy VK, Plastaras JP, Ben-Josef E, Metz JM, Wojcieszynski AP. Proton Beam Re-Irradiation for Gastrointestinal Malignancies: A Systematic Review. J Gastrointest Oncol (2020) 11:187–202. doi: 10.21037/jgo.2019.09.03
35. Patel SA, Edgington SK, Adams J, Morse C, Ryan DP, Hong TS. Novel Use of Proton Beam Therapy for Neoadjuvant Treatment of Radiation-Associated Squamous Cell Carcinoma of the Esophagus. J Gastrointest Oncol (2019) 10:155–60. doi: 10.21037/jgo.2018.09.06
36. Katano A, Yamashita H, Nakagawa K. Re-Irradiation of Locoregional Esophageal Cancer Recurrence Following Definitive Chemoradiotherapy: A Report of 6 Cases. Mol Clin Oncol (2017) 7:681–6. doi: 10.3892/mco.2017.1384
37. DeCesaris C, Gutschenritter T, Bhangoo RS, Garda AE, Miller RC, Mehra R, et al. Multi-Institutional Analysis of Patients Undergoing Proton Re-Irradiation for De Novo or Recurrent Malignancies of the Esophagus. Int J Radiat Oncol Biol Phys (2020) 108:e586. doi: 10.1016/j.ijrobp.2020.07.1796
38. Lester SC, Lin SH, Chuong M, Bhooshan N, Liao Z, Arnett AL, et al. A Multi-Institutional Analysis of Trimodality Therapy for Esophageal Cancer in Elderly Patients. Int J Radiat Oncol Biol Phys (2017) 98:820–8. doi: 10.1016/j.ijrobp.2017.02.021
40. Takada A, Nakamura T, Takayama K, Makita C, Suzuki M, Azami Y, et al. Preliminary Treatment Results of Proton Beam Therapy With Chemoradiotherapy for Stage I-Iii Esophageal Cancer. Cancer Med (2016) 5:506–15. doi: 10.1002/cam4.607
41. Chang JY, Zhang X, Knopf A, Li H, Mori S, Dong L, et al. Consensus Guidelines for Implementing Pencil-Beam Scanning Proton Therapy for Thoracic Malignancies on Behalf of the Ptcog Thoracic and Lymphoma Subcommittee. Int J Radiat Oncol Biol Phys (2017) 99:41–50. doi: 10.1016/j.ijrobp.2017.05.014
42. Gomez DR, Poenisch F, Pinnix CC, Sheu T, Chang JY, Memon N, et al. Malfunctions of Implantable Cardiac Devices in Patients Receiving Proton Beam Therapy: Incidence and Predictors. Int J Radiat Oncol Biol Phys (2013) 87:570–5. doi: 10.1016/j.ijrobp.2013.07.010
43. Wu AJ, Bosch WR, Chang DT, Hong TS, Jabbour SK, Kleinberg LR, et al. Expert Consensus Contouring Guidelines for Intensity Modulated Radiation Therapy in Esophageal and Gastroesophageal Junction Cancer. Int J Radiat Oncol Biol Phys (2015) 92:911–20. doi: 10.1016/j.ijrobp.2015.03.030
44. Hulshof MCCM, Geijsen D, Rozema T, Oppedijk V, Buijsen J, Neelis KJ, et al. A Randomized Controlled Phase Iii Multicenter Study on Dose Escalation in Definitive Chemoradiation for Patients With Locally Advanced Esophageal Cancer: Artdeco Study. J Clin Orthod (2020) 38:281–1. doi: 10.1200/JCO.2020.38.4_suppl.281
45. Park PC, Zhu XR, Lee AK, Sahoo N, Melancon AD, Zhang L, et al. A Beam-Specific Planning Target Volume (Ptv) Design for Proton Therapy to Account for Setup and Range Uncertainties. Int J Radiat Oncol Biol Phys (2012) 82:e329–36. doi: 10.1016/j.ijrobp.2011.05.011
46. Moyers MF, Miller DW, Bush DA, Slater JD. Methodologies and Tools for Proton Beam Design for Lung Tumors. Int J Radiat Oncol Biol Phys (2001) 49:1429–38. doi: 10.1016/S0360-3016(00)01555-8
49. Yu J, Zhang X, Liao L, Li H, Zhu R, Park PC, et al. Motion-Robust Intensity-Modulated Proton Therapy for Distal Esophageal Cancer. Med Phys (2016) 43:1111–8. doi: 10.1118/1.4940789
50. Prayongrat A, Xu C, Li H, Lin SH. Clinical Outcomes of Intensity Modulated Proton Therapy and Concurrent Chemotherapy in Esophageal Carcinoma: A Single Institutional Experience. Adv Radiat Oncol (2017) 2:301–7. doi: 10.1016/j.adro.2017.06.002
51. Chang JY, Jabbour SK, De Ruysscher D, Schild SE, Simone CB 2nd, Rengan R, et al. Consensus Statement on Proton Therapy in Early-Stage and Locally Advanced non-Small Cell Lung Cancer. Int J Radiat Oncol Biol Phys (2016) 95:505–16. doi: 10.1016/j.ijrobp.2016.01.036
52. Tryggestad EJ, Liu W, Pepin MD, Hallemeier CL, Sio TT. Managing Treatment-Related Uncertainties in Proton Beam Radiotherapy for Gastrointestinal Cancers. J Gastrointest Oncol (2020) 11:212–24. doi: 10.21037/jgo.2019.11.07
53. Liu W, Zhang X, Li Y, Mohan R. Robust Optimization of Intensity Modulated Proton Therapy. Med Phys (2012) 39:1079–91. doi: 10.1118/1.3679340
54. Liu W, Schild SE, Chang JY, Liao Z, Chang Y-H, Wen Z, et al. Exploratory Study of 4d Versus 3d Robust Optimization in Intensity Modulated Proton Therapy for Lung Cancer. Int J Radiat OncologyBiologyPhysics (2016) 95:523–33. doi: 10.1016/j.ijrobp.2015.11.002
55. Wang X, Li H, Zhu XR, Hou Q, Liao L, Jiang B, et al. Multiple-Ct Optimization of Intensity-Modulated Proton Therapy - Is it Possible to Eliminate Adaptive Planning? Radiother Oncol (2018) 128:167–73. doi: 10.1016/j.radonc.2017.09.032
56. Anakotta RM, van der Laan HP, Visser S, Ribeiro CO, Dieters M, Langendijk JA, et al. Weekly Robustness Evaluation of Intensity-Modulated Proton Therapy for Oesophageal Cancer. Radiother Oncol (2020) 151:66–72. doi: 10.1016/j.radonc.2020.07.015
57. Ribeiro CO, Meijers A, Korevaar EW, Muijs CT, Both S, Langendijk JA, et al. Comprehensive 4d Robustness Evaluation for Pencil Beam Scanned Proton Plans. Radiother Oncol (2019) 136:185–9. doi: 10.1016/j.radonc.2019.03.037
58. Roos CTG, Faiz Z, Visser S, Dieters M, van der Laan HP, den Otter LA, et al. A Comprehensive Motion Analysis - Consequences for High Precision Image-Guided Radiotherapy of Esophageal Cancer Patients. Acta Oncol (2021) 2020:1–8. doi: 10.1080/0284186X.2020.1843707
59. Li H, Zhu XR, Zhang X. Reducing Dose Uncertainty for Spot-Scanning Proton Beam Therapy of Moving Tumors by Optimizing the Spot Delivery Sequence. Int J Radiat Oncol Biol Phys (2015) 93:547–56. doi: 10.1016/j.ijrobp.2015.06.019
60. Hernandez Morales D, Shan J, Liu W, Augustine KE, Bues M, Davis MJ, et al. Automation of Routine Elements for Spot-Scanning Proton Patient-Specific Quality Assurance. Med Phys (2019) 46:5–14. doi: 10.1002/mp.13246
61. Meijers A, Guterres Marmitt G, Ng Wei Siang K, van der Schaaf A, Knopf AC, Langendijk JA, et al. Feasibility of Patient Specific Quality Assurance for Proton Therapy Based on Independent Dose Calculation and Predicted Outcomes. Radiother Oncol (2020) 150:136–41. doi: 10.1016/j.radonc.2020.06.027
62. Minsky BD, Pajak TF, Ginsberg RJ, Pisansky TM, Martenson J, Komaki R, et al. Int 0123 (Radiation Therapy Oncology Group 94-05) Phase Iii Trial of Combined-Modality Therapy for Esophageal Cancer: High-Dose Versus Standard-Dose Radiation Therapy. J Clin Oncol (2002) 20:1167–74. doi: 10.1200/JCO.2002.20.5.1167
63. Mizumoto M, Sugahara S, Okumura T, Hashimoto T, Oshiro Y, Fukumitsu N, et al. Hyperfractionated Concomitant Boost Proton Beam Therapy for Esophageal Carcinoma. Int J Radiat Oncol Biol Phys (2011) 81:e601–6. doi: 10.1016/j.ijrobp.2011.02.041
64. Paganetti H, Blakely E, Carabe-Fernandez A, Carlson DJ, Das IJ, Dong L, et al. Report of the Aapm Tg-256 on the Relative Biological Effectiveness of Proton Beams in Radiation Therapy. Med Phys (2019) 46:e53–78. doi: 10.1002/mp.13390
65. Wan Chan Tseung HS, Ma J, Kreofsky CR, Ma DJ, Beltran C. Clinically Applicable Monte Carlo-Based Biological Dose Optimization for the Treatment of Head and Neck Cancers With Spot-Scanning Proton Therapy. Int J Radiat oncol biol Phys (2016) 95:1535–43. doi: 10.1016/j.ijrobp.2016.03.041
66. Liu C, Patel SH, Shan J, Schild SE, Vargas CE, Wong WW, et al. Robust Optimization for Intensity Modulated Proton Therapy to Redistribute High Linear Energy Transfer From Nearby Critical Organs to Tumors in Head and Neck Cancer. Int J Radiat Oncol Biol Phys (2020) 107:181–93. doi: 10.1016/j.ijrobp.2020.01.013
Keywords: esophageal cancer, proton beam therapy (PBT), chemoradiation, pencil beam scanning, passive scatter proton
Citation: Chuong MD, Hallemeier CL, Li H, Zhu XR, Zhang X, Tryggestad EJ, Yu J, Yang M, Choi JI, Kang M, Liu W, Knopf A, Meijers A, Molitoris JK, Apisarnthanarax S, Giap H, Hoppe BS, Lee P, Chang JY, Simone CB 2nd and Lin SH (2021) Executive Summary of Clinical and Technical Guidelines for Esophageal Cancer Proton Beam Therapy From the Particle Therapy Co-Operative Group Thoracic and Gastrointestinal Subcommittees. Front. Oncol. 11:748331. doi: 10.3389/fonc.2021.748331
Received: 27 July 2021; Accepted: 28 September 2021;
Published: 19 October 2021.
Edited by:
Toshikazu Moriwaki, University of Tsukuba, JapanReviewed by:
Hitoshi Ishikawa, National Institutes for Quantum and Radiological Science and Technology, JapanCopyright © 2021 Chuong, Hallemeier, Li, Zhu, Zhang, Tryggestad, Yu, Yang, Choi, Kang, Liu, Knopf, Meijers, Molitoris, Apisarnthanarax, Giap, Hoppe, Lee, Chang, Simone and Lin. This is an open-access article distributed under the terms of the Creative Commons Attribution License (CC BY). The use, distribution or reproduction in other forums is permitted, provided the original author(s) and the copyright owner(s) are credited and that the original publication in this journal is cited, in accordance with accepted academic practice. No use, distribution or reproduction is permitted which does not comply with these terms.
*Correspondence: Steven H. Lin, c2hsaW5AbWRhbmRlcnNvbi5vcmc=
†These authors share first authorship
Disclaimer: All claims expressed in this article are solely those of the authors and do not necessarily represent those of their affiliated organizations, or those of the publisher, the editors and the reviewers. Any product that may be evaluated in this article or claim that may be made by its manufacturer is not guaranteed or endorsed by the publisher.
Research integrity at Frontiers
Learn more about the work of our research integrity team to safeguard the quality of each article we publish.