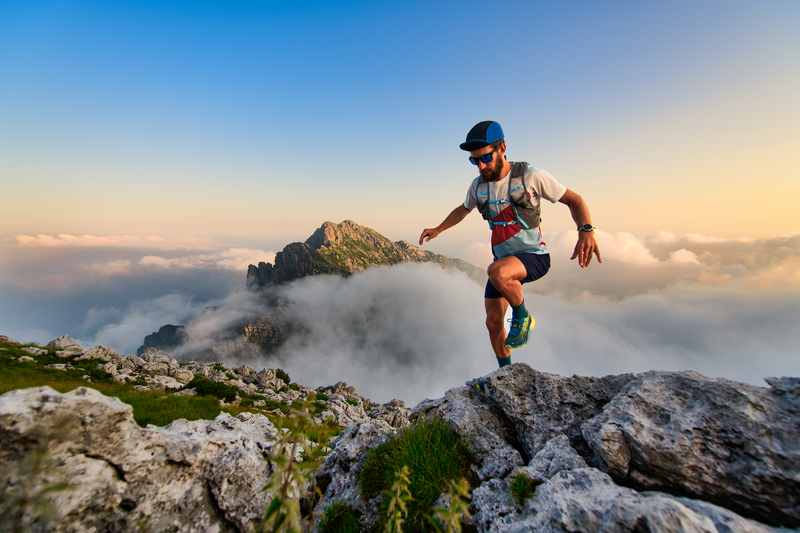
95% of researchers rate our articles as excellent or good
Learn more about the work of our research integrity team to safeguard the quality of each article we publish.
Find out more
REVIEW article
Front. Oncol. , 28 October 2021
Sec. Breast Cancer
Volume 11 - 2021 | https://doi.org/10.3389/fonc.2021.731535
This article is part of the Research Topic Triple-Negative Breast Cancer: Heterogeneity, Tumor Microenvironment and Targeted Therapy View all 12 articles
Triple-negative breast cancer (TNBC) is the most aggressive subtype of breast cancer, which is characterized by the absence of estrogen receptor (ER) and progesterone receptor (PR) expression and the absence of human epidermal growth factor receptor 2 (HER2) expression/amplification. Conventional chemotherapy is the mainstay of systemic treatment for TNBC. However, lack of molecular targeted therapies and poor prognosis of TNBC patients have prompted a great effort to discover effective targets for improving the clinical outcomes. For now, poly (ADP-ribose) polymerase (PARP) inhibitors (PARPi’s) and immune checkpoint inhibitors have been approved for the treatment of TNBC. Moreover, agents that target signal transduction, angiogenesis, epigenetic modifications, and cell cycle are under active preclinical or clinical investigations. In this review, we highlight the current major developments in targeted therapies of TNBC, with some descriptions about their (dis)advantages and future perspectives.
Breast cancer is the type of cancer with the best-characterized molecular classification or subtyping. Clinical therapeutic efficacies vary enormously among the different subtypes, with luminal A/B subtypes and triple-negative breast cancer (TNBC) showing the best and worst outcomes, respectively (1). For TNBC, although initially responsive to chemotherapy, which is the mainstay of systemic treatment in TNBC, resistance occurs eventually in a significant portion of patients, leading to relapse of these patients. Due to the aggressive nature and lack of defined molecular targets, the poor overall survival (OS) of metastatic TNBC has remained essentially unchanged over the past two or three decades. Generally speaking, metastatic TNBC has a median OS of approximately 13 months (2), rendering improvement of the clinical outcomes an urgent task in the management of TNBC. Fortunately, we are now seeing encouraging clinical results from molecularly targeted approaches in TNBC, which include poly (ADP-ribose) polymerase inhibition and, most recently, immune checkpoint inhibition. Other potential promising targeted therapeutic strategies that are being actively investigated for TNBC include inhibition of signaling kinases (serine/threonine- or tyrosine-type), angiogenesis, epigenetic modifications, and cell cycle. The targeted therapeutic strategies of TNBC examined in clinical and preclinical studies are summarized in Table 1.
Poly (ADP-ribose) polymerases (PARPs) are a family of proteins involved in DNA damage repair and multiple other cellular processes. So far, 17 PARP members have been identified in human (49). Among them, PARP1 is the best-characterized family member and is responsible for 85-90% of the total PARP activity. It is activated by single-strand breaks (SSBs), thus catalyzing the synthesis of poly (ADP-ribose) chains that serve as a signal and platform to recruit other DNA repair proteins. Failure to repair SSBs because of the PARP deficiency or inhibition leads to the formation of double-strand breaks (DSBs).
In the cells that are functional for breast cancer susceptibility gene products (BRCA1 and BRCA2), DSBs can be repaired by a process called homologous recombination (HR). Therefore, BRCA-mutated tumors are more sensitive to inhibition of PARPs due to combined loss of PARP and HR repair, an effect called “synthetic lethality” (50, 51). In the presence of PARP inhibitors (PARPi’s), the cells with BRCA defects cannot repair the DNA damage and die, whereas the cells with functional BRCAs could perform effective DNA damage repair and survive (Figure 1). Up to 80% of ER/PR-negative breast cancers have reduced or undetectable BRCA1 expression (52). Although germline mutations in BRCA1/2 are generally low, these mutations can confer a lifetime risk of up to 85% of developing breast cancer, with the majority (around 90%) of these tumors being triple-negative (53). Therefore, TNBC can theoretically be treated by a strategy of synthetic lethality that is based on PARP inhibition in BRCA-mutated tumors.
Figure 1 Synthetic lethality induced by PARPi’s and BRCA deficiency. Exposure of cells to PARPi’s (olaparib, rucaparib, niraparib, and talazoparib) leads to the formation of double strand breaks (DSBs) from single strand breaks (SSBs). Cells with intact BRCA function could survive since these breaks can be repaired by homologous recombination, while those with defective BRCA die because DSBs cannot be repaired. This phenomenon is known as “synthetic lethality”.
Various PARPi’s, which hamper DNA repair by blocking PARP-mediated PARylation, have been developed to induce synthetic lethality. Up to now, four PARPi’s, i.e., olaparib, rucaparib, niraparib, and talazoparib, have been approved by the US Food and Drug Administration (FDA) for cancer treatment. Two of them, olaparib and talazoparib, have been approved for BRCA-mutated metastatic breast cancer (54). While PARPi’s have been evaluated in clinical trials for TNBC as monotherapies, combination of PARPi’s with DNA-damaging chemotherapy appears to be a more promising approach due to increased efficacy of PARPi’s in BRCA-mutated breast cancer patients.
Olaparib is effective for patients with germline/somatic BRCA mutation or other HR-related gene mutations. The phase III OlympiA trial (NCT02032823) accessed olaparib treatment in HER2-negative breast cancer patients with germline BRCA1/2 mutations who had received neoadjuvant or adjuvant chemotherapy. The 3-year invasive disease-free survival (IDFS), 3-year distant disease-free survival (DDFS), and OS were significantly higher in the olaparib group than in the placebo group (3).
The phase II TBCRC 048 trial (NCT03344965) assessed olaparib response in metastatic breast cancer patients with germline mutations of non-BRCA1/2 HR-related genes (cohort 1) and somatic mutations of BRCA1/2 or other HR-related genes (cohort 2). The objective response rate was 33% in cohort 1 and 31% in cohort 2. Confirmed responses were only seen in patients with gPALB2 or sBRCA1/2 mutations. The median progression-free survival (PFS) for gPALB2 and sBRCA1/2 mutation carriers were 13.3 and 6.3 months, respectively. Among the gPALB2 and sBRCA1/2 mutation carriers, responses occurred in 67% of TNBC patients. No responses were observed with ATM or CHK2 mutations alone (4). This study revealed that patients with mutations of other HR-related genes might benefit from PARP inhibition.
With no BRCA mutation, patients would benefit more from combination treatment with chemotherapy and olaparib. A phase I study (NCT01445418) investigated olaparib combined with carboplatin in metastatic or recurrent TNBC patients with no germline BRCA mutation or with BRCAPro scores < 10% and negative family history. The objective response rate was 22%, with 1 patient having complete response. Hematologic toxicity was the most common adverse event (AE), with 36% of patients having Grade 3 and 4 neutropenia (5).
Veliparib, a novel PARPi that has favorable toxicity profile but is not FDA-approved yet, has been extensively studied in combination with various chemotherapeutic drugs. In a phase I clinical trial, the combination of veliparib with cisplatin and vinorelbine (a microtubule-destabilizing agent) gave rise to an overall response rate (ORR) of 73% in TNBC patients with mutated BRCA1/2 (55). In a phase III trial (NCT02032277), veliparib has been combined with paclitaxel plus carboplatin for the treatment of TNBC in standard neoadjuvant chemotherapy (56).
BRCA1/2 and other HR-related gene mutations could cause a defect in DSB repair called homologous recombination deficiency (HRD), leading to genomic instability and thus enhanced sensitivity to PARPi’s. Therefore, HRD status (including but not limited to BRCA1/2 mutations) could be evaluated to predict the response of PARPi’s (5, 57, 58).
I-SPY 2 trial (NCT01042379) showed that the PARPi veliparib combined with carboplatin had higher rate of pathological complete response (pCR) than standard therapy alone, specifically in TNBC (6). Further study revealed that BRCA1/2 mutation carriers were more likely to achieve a pCR compared to wild-type patients in the veliparib/carboplatin arm (7). In the PrECOG 0105 (NCT00813956) trial, patients with TNBC were treated with iniparib and chemotherapy, and the mean homologous recombination deficiency loss of heterozygosity (HRD-LOH) scores were higher in responders compared with non-responders (8). Jiang et al. reported that TNBC patients with higher HRD scores might have better prognosis and benefit from DNA repair inhibitors (9).
The above studies suggest that PARPi’s have shown great promise in TNBC patients and may be used as an effective therapeutic strategy for the treatment of BRCA-mutated or even BRCA-intact TNBC. Further more excited clinical findings are expected with the optimization of the therapeutic regimen.
Recently, there is enormous interest in cancer immunotherapy, particularly immune checkpoint-based immunotherapy. This is demonstrated by the awarding of the Nobel Prize in Physiology or Medicine in 2018 to James P. Allison at the University of Texas MD Anderson Cancer Center and Tasuku Honjo at Kyoto University, for their seminal work in identification of immune checkpoint molecules, i.e., programmed cell death-1 (PD1), programmed death-ligand 1 (PDL1), and cytotoxic T lymphocyte-associated protein 4 (CTLA4).
The discovery of these molecules led to the development of several FDA-approved humanized antibodies, so called immune checkpoint inhibitors, such as nivolumab, atezolizumab, and ipilimumab. These antibodies have demonstrated very well documented benefit for a variety of cancers (59) (Figure 2). Breast cancer, in general, is not an immunologically highly active cancer. However, the TNBC subtype shows higher presence of tumor-infiltrating lymphocytes (TILs) and is likely to respond to immunotherapy (60).
Figure 2 Immune checkpoint blockade in TNBC. Major histocompatibility complexes (MHCs) are antigens on the surface of the cancer cell for recognition by the cytotoxic T lymphocyte (CTL) via the TCR. The binding of PD1 on the surface of the CTL with its ligand PDL1 functions to suppress the activation of the CTL, leading to its cell death. CTLA4 is another inhibitory immune checkpoint molecule expressed on CTL. Antibodies (anti-CTLA4/ipilimumab, anti-PD1/pembrolizumab and nivolumab, anti-PDL1/atezolizumab and durvalumab) inhibit these immune checkpoint proteins to restore the activity of CTLs and kill cancer cells.
KEYNOTE-522 trial (NCT03036488) evaluated the safety and efficacy of pembrolizumab plus chemotherapy as neoadjuvant therapy, followed by definitive surgery and pembrolizumab as adjuvant therapy in patients who had early TNBC. Most treatment-related AEs occurred during the neoadjuvant phase, with higher percentage of patients having grade 3 or more serious AEs in the pembrolizumab plus chemotherapy group than in the placebo plus chemotherapy group. Consistent results were observed in the adjuvant phase. At the first and second interim analysis, patients in the pembrolizumab plus chemotherapy group had a higher pCR rate, which also occurred in PDL1-positive and PDL1-negative population, indicating that PDL1 expression was not a suitable predictor of response in early TNBC (10).
IMpassion050 trial (NCT03726879) evaluated the efficacy and safety of atezolizumab compared with placebo when it was combined with chemotherapy in high risk, HER2-positive early breast cancer. Results showed that this combination didn’t increase pCR either in the intention-to-treat population or in the PDL1-positive population. In the neoadjuvant phase, patients with Grade 3/4 or more serious AEs were increased in the atezolizumab group. There were 4 patients with Grade 5 AEs, including alveolitis, septic shock, sepsis, and COVID-19, in the neoadjuvant phase and 1 patient in the adjuvant phase (11).
Clinical trials have shown a correlation between high expression of PDL1 and efficacy of immune checkpoint inhibitors in metastatic TNBC. Thus, PDL1 could be a potential predictive biomarker of response to immunotherapy. Two antibody-based companion diagnostics for PDL1 expression are available. The PDL1 IHC 22C3 pharmDx (Agilent Technologies) is approved for selecting patients for treatment with pembrolizumab, using a cutoff of combined positive score (CPS) of 10. The Ventana PDL1 (SP142) assay (Roche Diagnostics) is approved for treatment with atezolizumab in metastatic TNBC, using a cutoff of immune cell (IC) score of 1% (61, 62).
In the phase III KEYNOTE-355 trial (NCT02819518), metastatic TNBC patients were randomly assigned 2:1 to receive pembrolizumab plus chemotherapy or placebo plus chemotherapy. PDL1 expression of formalin-fixed tumor samples was assessed by the PDL1 IHC 22C3 pharmDx assay and characterised by CPS. Among patients with CPS of 10 or more, median PFS was significantly prolonged in the pembrolizumab plus chemotherapy group (12).
In the IMpassion130 trial (NCT02425891), patients with untreated metastatic TNBC were randomly assigned in a 1:1 ratio to receive PDL1 antibody atezolizumab plus nab-paclitaxel or placebo plus nab-paclitaxel. The PDL1 expression on tumor-infiltrating immune cells was evaluated by PDL1 (SP142) immunohistochemical assay (IC score ≥ 1%, PDL1-positive). Kaplan-Meier analysis showed that atezolizumab plus nab-paclitaxel prolonged PFS in both intention-to-treat population and PDL1-positive population (13).
Besides PDL1 expression, tumor mutation burden (TMB) and immune infiltration could also be predictors for immune checkpoint inhibitor response. In the phase II GeparNuevo study (NCT02685059), patients with early TNBC were randomly assigned to receive durvalumab or placebo in addition to chemotherapy. Increased pCR rate was observed in both durvalumab and placebo group with higher stromal TILs or positive PDL1 expression (14). Whole exome sequencing and RNA sequencing of these samples showed that median TMB was significantly higher in patients with a pCR. The pCR rate of patients with high TMB and high immune gene expression profile (GEP) or TILs was notably higher compared with patients with low TMB and low immune GEP or TILs, which indicated both TMB and immune GEP or TILs were pCR predictors (15).
These findings are expected to lead to new effective treatment options for patients with TNBC. The immune checkpoint-based strategy for the therapy of TNBC is the topic of our recently published review. For sake of saving time and space, immune checkpoint inhibition in TNBC will not be described redundantly here. Interested readers please refer to our review (63) and another review published last year by Keenan et al. (64).
It should be noted that the benefit of immune checkpoint inhibition in TNBC is dependent on the protein level of the immune checkpoint molecules. For example, patients with PDL1-positive immune cells had prolonged PFS treated with atezolizumab (13). Furthermore, the status of post-translational modifications such as glycosylation of the PDL1 protein also significantly impacts the therapeutic efficacy of immune checkpoint inhibition in TNBC (65). For patients with unresectable locally advanced or metastatic TNBC whose tumors have PDL1 expression ≥ 1%, atezolizumab plus nab-paclitaxel is an effective therapeutic option (66). Therefore, it will be pivotal to screen predictors of response to immune checkpoint inhibitors for better option. In addition, combination with chemotherapy would benefit more than immune checkpoint inhibition alone.
Antibody-drug conjugates (ADCs) are complex engineered therapeutics composed of monoclonal antibodies that specifically recognize tumor-associated antigens and cytotoxic agents that bind to the antibody via a linker. ADCs could precisely target the cells and are internalized through endocytosis. Then they are decomposed to release cytotoxic agents, which induce cell death eventually. This targeted therapeutic delivery approach could reduce off-target toxicity by limiting normal tissues exposed to the cytotoxic agents (67).
Sacituzumab govitecan comprises an antibody targeting trophoblast cell-surface antigen 2 (Trop2), which couples to SN-38, a topoisomerase I inhibitor, through cleavable CL2A linker. A phase III ASCENT trial (NCT02574455) evaluated the efficacy of sacituzumab govitecan comparing with single-agent chemotherapy in patients with relapsed or refractory metastatic TNBC. In the sacituzumab govitecan group, PFS and OS were significantly prolonged and pCR rate was increased (16). According to the therapeutic effect, sacituzumab govitecan is recently approved for metastatic TNBC patients who have received two prior lines of therapy.
Ladiratuzumab vedotin (or SGN-LIV1A) is an investigational anti-LIV1 antibody-drug conjugate. The antibody binds to monomethyl auristatin E via a protease-cleavable linker. A phase Ib/II trial (NCT03310957) studied the combination of ladiratuzumab vedotin with pembrolizumab in patients with metastatic TNBC. Preliminary results showed ladiratuzumab vedotin was well tolerated and the combination with pembrolizumab produced a synergistic effect through immunogenic cell death that might enhance anti-PD1 activity (17, 18).
In cancer cells, some signaling pathways are highly activated, such as EGFR and its downstream PI3K/Akt/mTOR pathway (Figure 3), which could accelerate tumor initiation and progression. Thus, inhibiting these signaling pathways might be a potential therapeutic strategy for TNBC patients.
Figure 3 EGFR and its downstream signaling pathways inhibition in TNBC. Epidermal growth factor receptor (EGFR) could be activated by its ligand EGF or transforming growth factor α (TGFα). After its activation, it can dimerize with all members of HER family and create homo- or hetero-dimers, triggering a myriad of downstream signaling pathways, such as PI3K/Akt/mTOR, Ras/Raf/MEK/ERK and PLCγ/PKC. EGFR inhibitors (cetuximab, TKIs, panitumumab, and erlotinib), PI3K inhibitor (BKM120), mTORC1 inhibitor (rapamycin) and Akt inhibitor (ipatasertib) could hamper tumorigenesis and tumor progression by suppressing the process of signal transduction.
Epidermal growth factor receptor (EGFR) is a glycoprotein located on the surface of the cell membrane, which belongs to the HER family of transmembrane receptors. EGFR is activated by binding to its ligand including epidermal growth factor (EGF) and transforming growth factor α (TGFα). Following ligand binding, it can dimerize with all members of the HER family and generate homo- or hetero-dimers which could be autophosphorylated (68). The autophosphorylation triggers a myriad of downstream signaling pathways, such as PI3K/Akt, Ras/Raf/MEK/ERK and PLCγ/PKC, that play an important role in cell survival, proliferation, differentiation, motility, apoptosis, migration, adhesion, and angiogenesis (69). In TNBC, EGFR was overexpressed and was closely related with carcinogenesis and tumor progression (70). The expression of EGFR was negatively correlated with prognosis of TNBC patients (71).
EGFR could be targeted by monoclonal antibodies (cetuximab, panitumumab) and tyrosine kinase inhibitors (TKIs). Monoclonal antibodies and TKIs are approved for the treatment of advanced cancers, such as colorectal cancers and non-small cell lung cancers (72). However, two randomized phase II trials targeting EGFR in TNBC have not demonstrated significant beneficial effects. In the TBCRC 001 study, metastatic TNBC patients were treated with cetuximab alone and then plus carboplatin in progression compared to the combination therapy from the beginning. In another phase II study (NCT00463788), patients with metastatic TNBC received cisplatin plus cetuximab or cisplatin alone. The ORR was 20% with cisplatin plus cetuximab and 10% with cisplatin alone. Patients treated with cisplatin plus cetuximab had longer PFS than those treated with cisplatin alone (19).
Despite the unsatisfactory clinical data, the results should not be ignored when considering the potential of anti-EGFR agents in TNBC. A preclinical study from MD Anderson Cancer Center demonstrated that the EGFR tyrosine kinase inhibitor erlotinib inhibited tumor growth and metastasis and reversed a change from mesenchymal to epithelial phenotype by increasing the expression of E-cadherin and decreasing the expression of vimentin in TNBC cells (20). Another preclinical research showed that erlotinib inhibited tumor growth and metastasis in a SUM149 xenograft mouse model, which might be non-specific effect of EGFR inhibition since erlotinib could inhibit other kinases (21). The above results suggest that EMT modulation by targeting EGFR may reduce metastasis of TNBC, and inhibiting EGFR may be a potential therapeutic approach to patients with TNBC.
Phosphoinositide 3-kinase (PI3K) is a lipid kinase which is activated by receptor tyrosine kinases (RTKs) and catalyzes phosphatidylinositol 4,5-bisphosphate (PIP2) to inositol 1,4,5-trisphosphate (IP3) subsequently. Phosphoinositide-dependent kinase 1 (PDK1) and Akt are both recruited by IP3 and located near the plasma membrane. Then, Akt is phosphorylated at Thr308 by PDK1, leading to its partial activation. Full activation of Akt occurs upon the phosphorylation at Ser473 by mTORC2 (73). The PI3K/Akt/mTOR signaling pathway plays a vital role in cell growth, proliferation, angiogenesis, and metabolism (74), which is negatively regulated by PTEN and INPP4B (75).
The PI3K/Akt/mTOR pathway is an important oncogenic driver in TNBC. The activation mutations of PIK3CA, the gene encoding the catalytic subunit of PI3K (76), are 23.7% in TNBC. The inhibition of the PI3K/Akt/mTOR signaling pathway has exhibited a promising prospect in treating TNBC. In patient-derived xenograft (PDX) models originating from TNBC, the PI3K inhibitor BKM120 was used to evaluate their response by measuring tumor growth. It has been shown that BKM120 therapy led to significant tumor growth inhibition in all models, with the percentage of tumor growth inhibition (%TGI) ranging from 35% in the least sensitive model WHIM12 (PTEN-deficient) and 84% in the most sensitive model WHIM4 (PTEN-normal) (22). Lin et al. proposed another strategy for using an mTORC1 inhibitor, rapamycin, to combat metastatic TNBC with upregulated Gαh, also known as tissue transglutaminase (tTG) or transglutaminase 2 (TG2) (23). Patients from a randomized, double-blind, phase II trial (NCT02162719) received intravenous paclitaxel with or without Akt inhibitor ipatasertib until disease progression or unacceptable toxicity. Results showed that median PFS in the ipatasertib group was 6.2 months, compared with 4.9 months in the placebo group. These are the first results supporting Akt-targeted therapy for TNBC (24). The development of drugs targeting the PI3K/Akt/mTOR pathway for the treatment of TNBC is an emerging field, and we look forward to more promising clinical trials.
Solid tumors couldn’t grow beyond a certain size or metastasize to another organ without blood vessels (77). Thus, blocking tumor angiogenesis could cut off intertumoral oxygen and nutritional supply and arrest tumor growth (Figure 4). Vascular endothelial growth factor (VEGF) and its receptor VEGFR have been demonstrated to be major contributors to angiogenesis (78). The VEGF signaling stimulates cellular pathways that promote the formation of intertumoral blood vessels, leading to rapid tumor growth and metastatic potential (79).
Figure 4 Angiogenesis inhibition in TNBC. Tumor cells produce VEGF which interacts with VEGFR contributing to angiogenesis. VEGF inhibitor bevacizumab and VEGFR inhibitor apatinib could prevent VEGF interacting with VEGFR, thus blocking tumor angiogenesis.
VEGF is highly expressed in TNBC and a higher VEGF content is significantly correlated with shorter relapse-free survival (RFS) as well as OS (80). Bevacizumab is a humanized antibody binding to VEGF-A, the prototype VEGF family member, which prevents VEGF from interacting with its receptor, VEGFR. A randomized phase III RIBBON-2 trial revealed that second-line bevacizumab-containing therapy for TNBC patients improved median PFS from 2.7 months to 6.0 months, median OS from 12.6 months to 17.9 months, and ORR from 18% to 41%, respectively (25). A first-line bevacizumab-containing therapy showed a 49% response rate, median time to progression (TTP) of 7.2 months, and median OS of 18.3 months, respectively, for metastatic TNBC (26). In the GeparQuinto trial indicated the addition of bevacizumab to neoadjuvant anthracycline-taxane-containing chemotherapy significantly increased the pCR rate from 27.9% to 39.3% in TNBC patients (27). Results from a phase II neoadjuvant trial showed bevacizumab combined with docetaxel and carboplatin as neoadjuvant chemotherapy resulted in an encouraging pCR rate (42%) in TNBC (28). However, no differences in 3-year invasive disease-free survival (IDFS) and OS were noted in a phase III BEATRICE study (NCT00528567), in which TNBC patients received chemotherapy with or without bevacizumab (29). Moreover, in CALGB 40603 trail (NCT00861705), the efficacy of carboplatin or bevacizumab combined neoadjuvant chemotherapy were evaluated in stage II to III TNBC. Patients treated with carboplatin had higher pCR breast and pCR breast/axilla rates, while patients received bevacizumab only had higher pCR breast rate. Those received both agents had the highest pCR rate, with no significant interaction between their effects (30). A multicenter phase II study (NCT01176669) of VEGFR inhibitor apatinib treating metastatic TNBC patients revealed that the ORR and clinical benefit rate were 10.7% and 25.0%, respectively. Median PFS and OS were 3.3 months and 10.6 months, respectively (31). These angiogenesis inhibitors have shown objective efficacy in clinical trials of TNBC and had controllable toxicity, but testing in breast cancer that is highly angiogenesis-dependent might provide more convincing evidence for novel strategy of TNBC treatments.
Epigenetic modifications often specify stably heritable changes in phenotype resulting from changes in a chromosome without alterations in the DNA sequence (81). With decades of research, epigenetic modifications have emerged as fundamental players in cancer development and progression, which mainly include DNA modifications (such as DNA methylation) and histone modifications (such as histone deacetylation) (Figure 5) (82). DNA methylation recruits proteins involved in gene repression or inhibits the binding of transcription factors to DNA to regulate gene expression (83). Histone modifications could influence chromatin compaction and accessibility through many ways, including acetylation, phosphorylation, ubiquitinylation, and sumoylation (84). Additionally, epigenetic modifications are being developed as clinical biomarkers for diagnostic, prognostic, and therapeutic applications in tumors (85, 86). Therefore, inhibiting DNA methylation and histone deacetylation may be a probable targeted therapeutic strategy.
Figure 5 DNA methylation and histone deacetylation inhibition in TNBC. DNA is methylated by DNMT and histone is deacetylated by HDAC, which could be inhibited by DNMTi’s (entinostat/ENT, suberoylanilide hydroxamic acid/SAHA) and HDACi’s (5-azacytidine/AZA, decitabine/DAC), respectively. This would induce tumor cell apoptosis and inhibit angiogenesis, cell migration and invasion.
DNA methylation refers to the process that a methyl group is added to the 5′ position of the cytosine ring in CpG dinucleotides. Tumor suppressor genes, such as BRCA1, could be inhibited in tumors by promoter hypermethylation, which may be an important mechanism of primary breast cancer progression (87, 88). A research based on the analysis of a large number of breast cancer cases confirmed that BRCA1 is abnormally methylated in sporadic tumors and methylation of BRCA1 played a key role in breast tumorigenesis. Moreover, methylation of BRCA1 is negatively correlated with ER and PR expression (89).
DNA methylation is initiated by DNA methyltransferases (DNMTs). The DNMT family enzymes consist of DNMT1, DNMT2, DNMT3A, and DNMT3B, among which DNMT1 is the crucial maintenance methyltransferase in humans (90). DNMT1 was highly expressed in TNBC compared to other subtypes. The expression of DNMT1 was negatively associated with OS in breast cancer (91). A preclinical study showed that PARPi’s plus DNMT inhibitors (DNMTi’s, 5-azacytidine/AZA, decitabine/DAC) increased PARPi efficacy and resulted in additional tumor inhibition in TNBC cells harboring wild-type BRCA1 compared with each drug alone (32). Although it was only a preclinical study in TNBC, DNMTi’s had been approved by the US FDA for treating other cancers, such as myeloid malignancies and could be promising agents for TNBC treatment (33).
Histone deacetylase (HDAC) is an enzyme that deacetylates histone proteins. The deacetylation of histones leads to chromatin condensation, which ultimately represses the transcription of gene expression. The negative regulation of tumor suppressor gene is associated with tumor cell invasion, migration, proliferation, and angiogenesis. In contrast, HDAC inhibitors (HDACi’s) could reverse the gene expression suppression through histone hyperacetylation and chromatin relaxation. More specifically, HDACi’s could induce tumor cell apoptosis and inhibit angiogenesis, cell migration, and invasion (92, 93).
In a preclinical study, researchers found the HDACi entinostat (ENT) increased the expression of estrogen receptor-α (ERα) and aromatase in breast cancer cells and restored the sensitization of breast cancer cells to the aromatase inhibitor letrozole both in vitro and in vivo. These results suggested that combination of histone deacetylase and aromatase inhibitors could be used to treat ER-negative and endocrine therapy-resistant breast cancer (34). Sulaiman et al. have revealed that the expression of mTORC1 and HDAC were higher in TNBC than in luminal breast cancer. Co-inhibition of mTORC1 and HDAC with rapamycin plus valproic acid reproducibly promoted estrogen receptor 1 (ESR1) gene expression in TNBC cells (35). HDACi’s increase PDL1 and HLA-DR expression in TNBC and reduce the proportion of CD4Foxp3+ T cells. PD1 and CTLA4 blockade promoted TIL infiltration, cell apoptosis, and tumor regression. Thus, HDAC inhibition by HDACi’s could potentiate the tumor-suppressive effects of immunotherapy in TNBC (36). Another study has demonstrated that the HDACi suberoylanilide hydroxamic acid (SAHA) could enhance the anti-tumor effects of the PARPi olaparib in TNBC cells by regulating the expression of homologous recombination repair (HRR)-related genes and hampering DNA repair (37).
The cell cycle involves four ordered phases denoted G1 (resting stage), S (DNA synthesis), G2 (protein synthesis), and M (mitosis) (Figure 6). To ensure the fidelity of the cell cycle, several checkpoints arrest cell cycle to allow cells to properly repair defects during DNA synthesis and chromosome segregation (94). Cyclin-dependent kinases (CDKs) are activated and promote cell cycle progression with binding to cyclins that are synthesized and cleared during the cell cycle (95). Tumors with dysregulated CDKs often induce unscheduled proliferation (94).
Figure 6 Cell cycle inhibition in TNBC. Cell cycle involves G1, S, G2, and M phases. CDK4/6 inhibitor (palbociclib, ribociclib, and abemaciclib) blocks the cell cycle at G1 to S transition by triggering dephosphorylation of retinoblastoma tumor suppressor protein (Rb). TTK inhibitor (BOS172722) binds to TTK that controls the spindle assembly checkpoint. PLK4 inhibitor (CFI-400945) reduces centriole duplication to prevent tumor growth.
It is well-known that the CDK4/6 inhibitors, blocking the cell cycle at the G1 to S transition by triggering the dephosphorylation of retinoblastoma tumor suppressor protein (Rb) (96), play a vital role in preventing the proliferation of cancer cells. For now, three CDK4/6 inhibitors (palbociclib, ribociclib, and abemaciclib) received FDA approval for the treatment of HR-positive or HER2-negative breast cancer (97–100). However, the therapeutic effect of CDK4/6 inhibitors in TNBC is poor since loss of Rb often occurs. Extensive studies have revealed that combination with other molecules inhibition or therapy, such as PI3K inhibition, AR inhibition, immune checkpoint blockage, and chemotherapy, might help to overcome drug resistance in TNBC (38). In a preclinical study, dual blockade of PI3K and CDK4/6 had synergistic effect and could generate immunogenic cell death in TNBC cells (39). Pretreatment with palbociclib could improve the sensitivity of Rb-positive TNBC cells to paclitaxel (40). Phase I/II clinical trials of the safety and efficacy of CDK4/6 inhibition with or without other agents (anti-androgen medication, anti-PDL1 antibody, and chemotherapeutic drugs) in TNBC are ongoing (38).
Another class of agents targeting the cell cycle is TTK protein kinase inhibitors. TTK, namely monopolar spindle 1 (MPS1), controls the spindle assembly checkpoint (SAC) that ensures the integrity and stability of the genome in mitosis (101). TNBC has high expression levels of mitotic checkpoint molecules, and consequently, TTK inhibitors might prevent TNBC growth and proliferation (41). A preclinical trial demonstrated MPS1/TTK inhibitors have anti-proliferative effects in basal BC cell lines, with the half-maximal inhibitory concentration (IC50) values ranging from 0.05 to 1.0 μM (42). Anderhub et al. showed that in multiple xenograft models of human TNBC, the combination of MPS1 inhibitor BOS172722 and paclitaxel results in significant in vivo efficacy, showing significant tumor regression compared with either drug alone (43).
Polo-like kinase 4 (PLK4), a regulator of the centriole duplication, is crucial to the maintenance of centriole and centrosome numerical integrity. PLK4 inhibitors would potentiate aneuploidy and genomic instability and lead to cancer cell death (102). An in vitro experimental study showed that a novel inhibitor of PLK4, CFI-400945, in combination with radiation, exhibited a synergistic anti-cancer effect in TNBC cell lines and patient-derived organoids and led to a significant increase in survival to tumor endpoint in xenograft models in vivo, compared to control or single-agent treatment (44). However, overactivation of PLK4 is always correlated with centrosome amplification (CA) promoting a high risk of breast cancer (103). Further preclinical studies are warranted to characterize molecular mechanisms of action of this combination and its potential clinical applications, and lay a theoretical foundation for PLK4 to be used as a promising target in TNBC.
Beyond this, ATR, CHK1, WEE1, and TRAIL might also be targets in TNBC. Preclinical studies showed that ATR or CHK1 inhibitor could delay the radiation-induced DNA repair and inhibit cell survival in TNBC cells (45, 46), while WEE1 inhibition could overcome cisplatin resistance in TNBC cells (47), and TRAIL receptor agonist could induce apoptosis in TNBC cells that expressed vimentin and Axl (48).
TNBC is a complex disease with poor prognosis and rare effective targeted therapy. It is urgent to explore novel targeted therapeutic strategies. For now, PARP inhibition has shown great promise in BRCA1/2-mutated TNBC patients. It is of great hope to combine PARPi’s with DNA-damaging chemotherapy for TNBC patients harboring wild-type BRCA1/2. Meanwhile, results of clinical and preclinical studies have revealed that immunotherapy with checkpoint blockage gives rise to a good outcome in PD1/PDL1-positive TNBC patients. Targeting VEGF/VEGFR alone provides potential efficacy by inhibiting angiogenesis. However, many patients develop drug resistance while interconnected or compensatory pathways could overcome VEGF/VEGFR-targeted inhibition (78). As the “genomic medicines”, epigenetic drugs (DNMTi’s, HDACi’s, etc) have shown great application prospects in treating TNBC patients. Targeting epigenetic modifications have exhibited great efficacy when used jointly with other therapies such as chemotherapy or immunotherapy (104). CDK4/6 is the main target of cell cycle in breast cancer. When combined with other targeted therapeutic agents, CDK4/6 inhibitors could benefit more TNBC patients.
In summary, each targeted therapy in TNBC has its advantages and disadvantages when applied alone. Thus, combination of various targeted therapies would be a better strategy to enhance the therapeutic effectiveness and benefit more TNBC patients. Additionally, it is also warranted to conduct more and in-depth studies to identify novel effective therapeutic targets in TNBC. Hopefully, TNBC patients will have more individualized treatment options and better outcomes in the near future.
YL, ZZ, and XY: Reviewing the literature and writing the original manuscript. SF: Writing, revising, and editing the manuscript. XD: Revising and reviewing the manuscript. All authors contributed to the article and approved the submitted version.
This work was supported by National Natural Science Foundation of China (82103342), Natural Science Foundation of Hunan Province (2021JJ40366), Key Grant of Research and Development in Hunan Province (2020DK2002), Changsha Municipal Natural Science Foundation (kq2014080), National Students’ Platform for Innovation and Entrepreneurship Training Program (S202010542026), and Hunan Normal University School of Medicine Open Project Fund (KF2021019).
The authors declare that the research was conducted in the absence of any commercial or financial relationships that could be construed as a potential conflict of interest.
All claims expressed in this article are solely those of the authors and do not necessarily represent those of their affiliated organizations, or those of the publisher, the editors and the reviewers. Any product that may be evaluated in this article, or claim that may be made by its manufacturer, is not guaranteed or endorsed by the publisher.
1. Zheng C, He G, Deng X. Chapter ONE: Overview of Triple-Negative Breast Cancer. In Triple-Negative Breast Cancer. Singapore: World Scientific Publishing. (2020) pp. 1–20. doi: 10.1142/9789813277762_0001
2. Andre F, Zielinski CC. Optimal Strategies for the Treatment of Metastatic Triple-Negative Breast Cancer With Currently Approved Agents. Ann Oncol (2012) 23 Suppl 6:vi46–51. doi: 10.1093/annonc/mds195
3. Tutt ANJ, Garber JE, Kaufman B, Viale G, Fumagalli D, Rastogi P, et al. Adjuvant Olaparib for Patients With BRCA1- or BRCA2-Mutated Breast Cancer. N Engl J Med (2021) 384(25):2394–405. doi: 10.1056/NEJMoa2105215
4. Tung NM, Robson ME, Ventz S, Santa-Maria CA, Nanda R, Marcom PK, et al. TBCRC 048: Phase II Study of Olaparib for Metastatic Breast Cancer and Mutations in Homologous Recombination-Related Genes. J Clin Oncol (2020) 38(36):4274–82. doi: 10.1200/JCO.20.02151
5. Lee JM, Hays JL, Chiou VL, Annunziata CM, Swisher EM, Harrell MI, et al. Phase I/Ib Study of Olaparib and Carboplatin in Women With Triple Negative Breast Cancer. Oncotarget (2017) 8(45):79175–87. doi: 10.18632/oncotarget.16577
6. Rugo HS, Olopade OI, DeMichele A, Yau C, van ‘t Veer LJ, Buxton MB, et al. Adaptive Randomization of Veliparib-Carboplatin Treatment in Breast Cancer. N Engl J Med (2016) 375(1):23–34. doi: 10.1056/NEJMoa1513749
7. Wolf DM, Yau C, Sanil A, Glas A, Petricoin E, Wulfkuhle J, et al. DNA Repair Deficiency Biomarkers and the 70-Gene Ultra-High Risk Signature as Predictors of Veliparib/Carboplatin Response in the I-SPY 2 Breast Cancer Trial. NPJ Breast Cancer (2017) 3:31. doi: 10.1038/s41523-017-0025-7
8. Telli ML, Jensen KC, Vinayak S, Kurian AW, Lipson JA, Flaherty PJ, et al. Phase II Study of Gemcitabine, Carboplatin, and Iniparib As Neoadjuvant Therapy for Triple-Negative and BRCA1/2 Mutation-Associated Breast Cancer With Assessment of a Tumor-Based Measure of Genomic Instability: PrECOG 0105. J Clin Oncol (2015) 33(17):1895–901. doi: 10.1200/JCO.2014.57.0085
9. Jiang YZ, Ma D, Suo C, Shi J, Xue M, Hu X, et al. Genomic and Transcriptomic Landscape of Triple-Negative Breast Cancers: Subtypes and Treatment Strategies. Cancer Cell (2019) 35(3):428–40 e5. doi: 10.1016/j.ccell.2019.02.001
10. Schmid P, Cortes J, Pusztai L, McArthur H, Kummel S, Bergh J, et al. Pembrolizumab for Early Triple-Negative Breast Cancer. N Engl J Med (2020) 382(9):810–21. doi: 10.1056/NEJMoa1910549
11. Huober J, Barrios CH, Niikura N, Jarzab M, Chang YC, Huggins-Puhalla SL, et al. VP6-2021: IMpassion050: A Phase III Study of Neoadjuvant Atezolizumab D Pertuzumab D Trastuzumab D Chemotherapy (Neoadj A D PH D CT) in High-Risk, HER2-Positive Early Breast Cancer (EBC). Ann Oncol (2021) 32(8):1061–62. doi: 10.1016/j.annonc.2021.05.800
12. Cortes J, Cescon DW, Rugo HS, Nowecki Z, Im SA, Yusof MM, et al. Pembrolizumab Plus Chemotherapy Versus Placebo Plus Chemotherapy for Previously Untreated Locally Recurrent Inoperable or Metastatic Triple-Negative Breast Cancer (KEYNOTE-355): A Randomised, Placebo-Controlled, Double-Blind, Phase 3 Clinical Trial. Lancet (2020) 396(10265):1817–28. doi: 10.1016/S0140-6736(20)32531-9
13. Schmid P, Adams S, Rugo HS, Schneeweiss A, Barrios CH, Iwata H, et al. Atezolizumab and Nab-Paclitaxel in Advanced Triple-Negative Breast Cancer. N Engl J Med (2018) 379(22):2108–21. doi: 10.1056/NEJMoa1809615
14. Loibl S, Untch M, Burchardi N, Huober J, Sinn BV, Blohmer JU, et al. A Randomised Phase II Study Investigating Durvalumab in Addition to an Anthracycline Taxane-Based Neoadjuvant Therapy in Early Triple-Negative Breast Cancer: Clinical Results and Biomarker Analysis of GeparNuevo Study. Ann Oncol (2019) 30(8):1279–88. doi: 10.1093/annonc/mdz158
15. Karn T, Denkert C, Weber KE, Holtrich U, Hanusch C, Sinn BV, et al. Tumor Mutational Burden and Immune Infiltration as Independent Predictors of Response to Neoadjuvant Immune Checkpoint Inhibition in Early TNBC in GeparNuevo. Ann Oncol (2020) 31(9):1216–22. doi: 10.1016/j.annonc.2020.05.015
16. Bardia A, Hurvitz SA, Tolaney SM, Loirat D, Punie K, Oliveira M, et al. Sacituzumab Govitecan in Metastatic Triple-Negative Breast Cancer. N Engl J Med (2021) 384(16):1529–41. doi: 10.1056/NEJMoa2028485
17. Han HS, Alemany CA, Brown-Glaberman UA, Pluard TJ, Sinha R, Sterrenberg D, et al. SGNLVA-002: Single-Arm, Open Label Phase Ib/II Study of Ladiratuzumab Vedotin (LV) in Combination With Pembrolizumab for First-Line Treatment of Patients With Unresectable Locally Advanced or Metastatic Triple-Negative Breast Cancer. J Clin Oncol (2019) 37(15_suppl):TPS1110. doi: 10.1200/JCO
18. Hyo (Heather) H, Sami D, Carlos A, Reva B, Ursa BG, Jane M, et al. Open Label Phase 1b/2 Study of Ladiratuzumab Vedotin in Combination With Pembrolizumab for First-Line Treatment of Patients With Unresectable Locally-Advanced or Metastatic Triple-Negative Breast Cancer [Abstract]. San Antonio Breast Cancer Symposium Cancer Res (2020) 80(4 Suppl):Abstract nr PD1–06. doi: 10.1158/1538-7445
19. Baselga J, Gomez P, Greil R, Braga S, Climent MA, Wardley AM, et al. Randomized Phase II Study of the Anti-Epidermal Growth Factor Receptor Monoclonal Antibody Cetuximab With Cisplatin Versus Cisplatin Alone in Patients With Metastatic Triple-Negative Breast Cancer. J Clin Oncol (2013) 31(20):2586–92. doi: 10.1200/JCO.2012.46.2408
20. Zhang D, LaFortune TA, Krishnamurthy S, Esteva FJ, Cristofanilli M, Liu P, et al. Epidermal Growth Factor Receptor Tyrosine Kinase Inhibitor Reverses Mesenchymal to Epithelial Phenotype and Inhibits Metastasis in Inflammatory Breast Cancer. Clin Cancer Res (2009) 15(21):6639–48. doi: 10.1158/1078-0432.CCR-09-0951
21. Ueno NT, Zhang D. Targeting EGFR in Triple Negative Breast Cancer. J Cancer (2011) 2:324–8. doi: 10.7150/jca.2.324
22. Guo Z, Primeau T, Luo J, Zhang C, Sun H, Hoog J, et al. Proteomic Resistance Biomarkers for PI3K Inhibitor in Triple Negative Breast Cancer Patient-Derived Xenograft Models. Cancers (Basel) (2020) 12(12):3857. doi: 10.3390/cancers12123857
23. Lin HY, Kuei CH, Lee HH, Lin CH, Zheng JQ, Chiu HW, et al. The Galphah/phospholipase C-Delta1 Interaction Promotes Autophagosome Degradation by Activating the Akt/mTORC1 Pathway in Metastatic Triple-Negative Breast Cancer. Aging (Albany NY) (2020) 12(13):13023–37. doi: 10.18632/aging.103390
24. Kim SB, Dent R, Im SA, Espié M, Blau S, Tan AR, et al. Ipatasertib Plus Paclitaxel Versus Placebo Plus Paclitaxel as First-Line Therapy for Metastatic Triple-Negative Breast Cancer (LOTUS): A Multicentre, Randomised, Double-Blind, Placebo-Controlled, Phase 2 Trial. Lancet Oncol (2017) 18(10):1360–72. doi: 10.1016/s1470-2045(17)30450-3
25. Brufsky A, Valero V, Tiangco B, Dakhil S, Brize A, Rugo HS, et al. Second-Line Bevacizumab-Containing Therapy in Patients With Triple-Negative Breast Cancer: Subgroup Analysis of the RIBBON-2 Trial. Breast Cancer Res Treat (2012) 133(3):1067–75. doi: 10.1007/s10549-012-2008-6
26. Thomssen C, Pierga JY, Pritchard KI, Biganzoli L, Cortes-Funes H, Petráková K, et al. First-Line Bevacizumab-Containing Therapy for Triple-Negative Breast Cancer: Analysis of 585 Patients Treated in the ATHENA Study. Oncology (2012) 82(4):218–27. doi: 10.1159/000336892
27. Gerber B, Loibl S, Eidtmann H, Rezai M, Fasching PA, Tesch H, et al. Neoadjuvant Bevacizumab and Anthracycline-Taxane-Based Chemotherapy in 678 Triple-Negative Primary Breast Cancers; Results From the Geparquinto Study (GBG 44). Ann Oncol (2013) 24(12):2978–84. doi: 10.1093/annonc/mdt361
28. Kim HR, Jung KH, Im SA, Im YH, Kang SY, Park KH, et al. Multicentre Phase II Trial of Bevacizumab Combined With Docetaxel-Carboplatin for the Neoadjuvant Treatment of Triple-Negative Breast Cancer (KCSG BR-0905). Ann Oncol (2013) 24(6):1485–90. doi: 10.1093/annonc/mds658
29. Cameron D, Brown J, Dent R, Jackisch C, Mackey J, Pivot X, et al. Adjuvant Bevacizumab-Containing Therapy in Triple-Negative Breast Cancer (BEATRICE): Primary Results of a Randomised, Phase 3 Trial. Lancet Oncol (2013) 14(10):933–42. doi: 10.1016/s1470-2045(13)70335-8
30. Sikov WM, Berry DA, Perou CM, Singh B, Cirrincione CT, Tolaney SM, et al. Impact of the Addition of Carboplatin and/or Bevacizumab to Neoadjuvant Once-Per-Week Paclitaxel Followed by Dose-Dense Doxorubicin and Cyclophosphamide on Pathologic Complete Response Rates in Stage II to III Triple-Negative Breast Cancer: CALGB 40603 (Alliance). J Clin Oncol (2015) 33(1):13–21. doi: 10.1200/JCO.2014.57.0572
31. Hu X, Zhang J, Xu B, Jiang Z, Ragaz J, Tong Z, et al. Multicenter Phase II Study of Apatinib, a Novel VEGFR Inhibitor in Heavily Pretreated Patients With Metastatic Triple-Negative Breast Cancer. Int J Cancer (2014) 135(8):1961–9. doi: 10.1002/ijc.28829
32. Muvarak NE, Chowdhury K, Xia L, Robert C, Choi EY, Cai Y, et al. Enhancing the Cytotoxic Effects of PARP Inhibitors With DNA Demethylating Agents - A Potential Therapy for Cancer. Cancer Cell (2016) 30(4):637–50. doi: 10.1016/j.ccell.2016.09.002
33. Jhan JR, Andrechek ER. Triple-Negative Breast Cancer and the Potential for Targeted Therapy. Pharmacogenomics (2017) 18(17):1595–609. doi: 10.2217/pgs-2017-0117
34. Sabnis GJ, Goloubeva O, Chumsri S, Nguyen N, Sukumar S, Brodie AM. Functional Activation of the Estrogen Receptor-Alpha and Aromatase by the HDAC Inhibitor Entinostat Sensitizes ER-Negative Tumors to Letrozole. Cancer Res (2011) 71(5):1893–903. doi: 10.1158/0008-5472.CAN-10-2458
35. Sulaiman A, McGarry S, Lam KM, El-Sahli S, Chambers J, Kaczmarek S, et al. Co-Inhibition of Mtorc1, HDAC and ESR1alpha Retards the Growth of Triple-Negative Breast Cancer and Suppresses Cancer Stem Cells. Cell Death Dis (2018) 9(8):815. doi: 10.1038/s41419-018-0811-7
36. Terranova-Barberio M, Thomas S, Ali N, Pawlowska N, Park J, Krings G, et al. HDAC Inhibition Potentiates Immunotherapy in Triple Negative Breast Cancer. Oncotarget (2017) 8(69):114156–72. doi: 10.18632/oncotarget.23169
37. Min A, Im SA, Kim DK, Song SH, Kim HJ, Lee KH, et al. Histone Deacetylase Inhibitor, Suberoylanilide Hydroxamic Acid (SAHA), Enhances Anti-Tumor Effects of the Poly (ADP-Ribose) Polymerase (PARP) Inhibitor Olaparib in Triple-Negative Breast Cancer Cells. Breast Cancer Res (2015) 17:33. doi: 10.1186/s13058-015-0534-y
38. Hu Y, Gao J, Wang M, Li M. Potential Prospect of CDK4/6 Inhibitors in Triple-Negative Breast Cancer. Cancer Manage Res (2021) 13:5223–37. doi: 10.2147/CMAR.S310649
39. Teo ZL, Versaci S, Dushyanthen S, Caramia F, Savas P, Mintoff CP, et al. Combined CDK4/6 and PI3Kalpha Inhibition Is Synergistic and Immunogenic in Triple-Negative Breast Cancer. Cancer Res (2017) 77(22):6340–52. doi: 10.1158/0008-5472.CAN-17-2210
40. Cretella D, Fumarola C, Bonelli M, Alfieri R, La Monica S, Digiacomo G, et al. Pre-Treatment With the CDK4/6 Inhibitor Palbociclib Improves the Efficacy of Paclitaxel in TNBC Cells. Sci Rep (2019) 9(1):13014. doi: 10.1038/s41598-019-49484-4
41. Daniel J, Coulter J, Woo JH, Wilsbach K, Gabrielson E. High Levels of the Mps1 Checkpoint Protein are Protective of Aneuploidy in Breast Cancer Cells. Proc Natl Acad Sci USA (2011) 108(13):5384–9. doi: 10.1073/pnas.1007645108
42. Sugimoto Y, Sawant DB, Fisk HA, Mao L, Li C, Chettiar S, et al. Novel Pyrrolopyrimidines as Mps1/TTK Kinase Inhibitors for Breast Cancer. Bioorg Med Chem (2017) 25(7):2156–66. doi: 10.1016/j.bmc.2017.02.030
43. Anderhub SJ, Mak GW, Gurden MD, Faisal A, Drosopoulos K, Walsh K, et al. High Proliferation Rate and a Compromised Spindle Assembly Checkpoint Confers Sensitivity to the MPS1 Inhibitor BOS172722 in Triple-Negative Breast Cancers. Mol Cancer Ther (2019) 18(10):1696–707. doi: 10.1158/1535-7163.MCT-18-1203
44. Parsyan A, Cruickshank J, Hodgson K, Wakeham D, Pellizzari S, Bhat V, et al. Anticancer Effects of Radiation Therapy Combined With Polo-Like Kinase 4 (PLK4) Inhibitor CFI-400945 in Triple Negative Breast Cancer. Breast (2021) 58:6–9. doi: 10.1016/j.breast.2021.03.011
45. Tu X, Kahila MM, Zhou Q, Yu J, Kalari KR, Wang L, et al. ATR Inhibition Is a Promising Radiosensitizing Strategy for Triple-Negative Breast Cancer. Mol Cancer Ther (2018) 17(11):2462–72. doi: 10.1158/1535-7163.MCT-18-0470
46. Zhou ZR, Yang ZZ, Wang SJ, Zhang L, Luo JR, Feng Y, et al. The Chk1 Inhibitor MK-8776 Increases the Radiosensitivity of Human Triple-Negative Breast Cancer by Inhibiting Autophagy. Acta Pharmacol Sin (2017) 38(4):513–23. doi: 10.1038/aps.2016.136
47. Zheng H, Shao F, Martin S, Xu X, Deng CX. WEE1 Inhibition Targets Cell Cycle Checkpoints for Triple Negative Breast Cancers to Overcome Cisplatin Resistance. Sci Rep (2017) 7:43517. doi: 10.1038/srep43517
48. Dine JL, O’Sullivan CC, Voeller D, Greer YE, Chavez KJ, Conway CM, et al. The TRAIL Receptor Agonist Drozitumab Targets Basal B Triple-Negative Breast Cancer Cells That Express Vimentin and Axl. Breast Cancer Res Treat (2016) 155(2):235–51. doi: 10.1007/s10549-015-3673-z
49. Slade D. Mitotic Functions of Poly(ADP-Ribose) Polymerases. Biochem Pharmacol (2019) 167:33–43. doi: 10.1016/j.bcp.2019.03.028
50. Ashworth A. A Synthetic Lethal Therapeutic Approach: Poly(ADP) Ribose Polymerase Inhibitors for the Treatment of Cancers Deficient in DNA Double-Strand Break Repair. J Clin Oncol: Off J Am Soc Clin Oncol (2008) 26(22):3785–90. doi: 10.1200/jco.2008.16.0812
51. Telli ML, Ford JM. Novel Treatment Approaches for Triple-Negative Breast Cancer. Clin Breast Cancer (2010) 10(Suppl 1):E16–22. doi: 10.3816/CBC.2010.s.003
52. Wilson CA, Ramos L, Villaseñor MR, Anders KH, Press MF, Clarke K, et al. Localization of Human BRCA1 and its Loss in High-Grade, non-Inherited Breast Carcinomas. Nat Genet (1999) 21(2):236–40. doi: 10.1038/6029
53. Thompson D, Easton DF. Cancer Incidence in BRCA1 Mutation Carriers. J Natl Cancer Inst (2002) 94(18):1358–65. doi: 10.1093/jnci/94.18.1358
54. Zimmer AS, Gillard M, Lipkowitz S, Lee JM. Update on PARP Inhibitors in Breast Cancer. Curr Treat Pptions Oncol (2018) 19(5):21. doi: 10.1007/s11864-018-0540-2
55. Lee A, Djamgoz MBA. Triple Negative Breast Cancer: Emerging Therapeutic Modalities and Novel Combination Therapies. Cancer Treat Rev (2018) 62:110–22. doi: 10.1016/j.ctrv.2017.11.003
56. Loibl S, O’Shaughnessy J, Untch M, Sikov WM, Rugo HS, McKee MD, et al. Addition of the PARP Inhibitor Veliparib Plus Carboplatin or Carboplatin Alone to Standard Neoadjuvant Chemotherapy in Triple-Negative Breast Cancer (BrighTNess): A Randomised, Phase 3 Trial. Lancet Oncol (2018) 19(4):497–509. doi: 10.1016/s1470-2045(18)30111-6
57. Timms KM, Abkevich V, Hughes E, Neff C, Reid J, Morris B, et al. Association of BRCA1/2 Defects With Genomic Scores Predictive of DNA Damage Repair Deficiency Among Breast Cancer Subtypes. Breast Cancer Res (2014) 16(6):475. doi: 10.1186/s13058-014-0475-x
58. Garufi G OA, Palazzo A, Tortora G, Bria E, Carbognin L. Homologous Repair Deficiency Status and Response to Neoadjuvant Chemotherapy for Triple-Negative Breast Cancer: The Best Current Biomarker to Select the Most Appropriate Treatment? J Cancer Sci Clin Ther (2021) 5(1):124–33. doi: 10.26502/jcsct.5079106
59. Tsai HF, Hsu PN. Cancer Immunotherapy by Targeting Immune Checkpoints: Mechanism of T Cell Dysfunction in Cancer Immunity and New Therapeutic Targets. J Biomed Sci (2017) 24(1):35. doi: 10.1186/s12929-017-0341-0
60. Vikas P, Borcherding N, Zhang W. The Clinical Promise of Immunotherapy in Triple-Negative Breast Cancer. Cancer Manage Res (2018) 10:6823–33. doi: 10.2147/cmar.s185176
61. Adel NG. Current Treatment Landscape and Emerging Therapies for Metastatic Triple-Negative Breast Cancer. Am J Manag Care (2021) 27(5 Suppl):S87–96. doi: 10.37765/ajmc.2021.88626
62. Arora S, Velichinskii R, Lesh RW, Ali U, Kubiak M, Bansal P, et al. Existing and Emerging Biomarkers for Immune Checkpoint Immunotherapy in Solid Tumors. Adv Ther (2019) 36(10):2638–78. doi: 10.1007/s12325-019-01051-z
63. Yi H, Li Y, Tan Y, Fu S, Tang F, Deng X. Immune Checkpoint Inhibition for Triple-Negative Breast Cancer: Current Landscape and Future Perspectives. Front Oncol (2021) 11:648139(1501). doi: 10.3389/fonc.2021.648139
64. Keenan TE, Tolaney SM. Role of Immunotherapy in Triple-Negative Breast Cancer. J Natl Compr Cancer Network: JNCCN (2020) 18(4):479–89. doi: 10.6004/jnccn.2020.7554
65. Li CW, Lim SO, Chung EM, Kim YS, Park AH, Yao J, et al. Eradication of Triple-Negative Breast Cancer Cells by Targeting Glycosylated PD-L1. Cancer Cell (2018) 33(2):187–201.e10. doi: 10.1016/j.ccell.2018.01.009
66. Kang C, Syed YY. Atezolizumab (in Combination With Nab-Paclitaxel): A Review in Advanced Triple-Negative Breast Cancer. Drugs (2020) 80(6):601–7. doi: 10.1007/s40265-020-01295-y
67. Birrer MJ, Moore KN, Betella I, Bates RC. Antibody-Drug Conjugate-Based Therapeutics: State of the Science. J Natl Cancer Inst (2019) 111(6):538–49. doi: 10.1093/jnci/djz035
68. Hsu JL, Hung MC. The Role of HER2, EGFR, and Other Receptor Tyrosine Kinases in Breast Cancer. Cancer Metastasis Rev (2016) 35(4):575–88. doi: 10.1007/s10555-016-9649-6
69. Yarden Y, Sliwkowski MX. Untangling the ErbB Signalling Network. Nat Rev Mol Cell Biol (2001) 2(2):127–37. doi: 10.1038/35052073
70. Gluz O, Liedtke C, Gottschalk N, Pusztai L, Nitz U, Harbeck N. Triple-Negative Breast Cancer–Current Status and Future Directions. Ann Oncol (2009) 20(12):1913–27. doi: 10.1093/annonc/mdp492
71. Viale G, Rotmensz N, Maisonneuve P, Bottiglieri L, Montagna E, Luini A, et al. Invasive Ductal Carcinoma of the Breast With the “Triple-Negative” Phenotype: Prognostic Implications of EGFR Immunoreactivity. Breast Cancer Res Treat (2009) 116(2):317–28. doi: 10.1007/s10549-008-0206-z
72. Duffy MJ, O’Donovan N, Crown J. Use of Molecular Markers for Predicting Therapy Response in Cancer Patients. Cancer Treat Rev (2011) 37(2):151–9. doi: 10.1016/j.ctrv.2010.07.004
73. Yang SX, Polley E, Lipkowitz S. New Insights on PI3K/AKT Pathway Alterations and Clinical Outcomes in Breast Cancer. Cancer Treat Rev (2016) 45:87–96. doi: 10.1016/j.ctrv.2016.03.004
74. Ediriweera MK, Tennekoon KH, Samarakoon SR. Role of the PI3K/AKT/mTOR Signaling Pathway in Ovarian Cancer: Biological and Therapeutic Significance. Semin Cancer Biol (2019) 59:147–60. doi: 10.1016/j.semcancer.2019.05.012
75. Guerrero-Zotano A, Mayer IA, Arteaga CL. PI3K/AKT/mTOR: Role in Breast Cancer Progression, Drug Resistance, and Treatment. Cancer Metastasis Rev (2016) 35(4):515–24. doi: 10.1007/s10555-016-9637-x
76. Arafeh R, Samuels Y. PIK3CA in Cancer: The Past 30 Years. Semin Cancer Biol (2019) 59:36–49. doi: 10.1016/j.semcancer.2019.02.002
77. Carmeliet P, Jain RK. Angiogenesis in Cancer and Other Diseases. Nature (2000) 407(6801):249–57. doi: 10.1038/35025220
78. Zhao Y, Adjei AA. Targeting Angiogenesis in Cancer Therapy: Moving Beyond Vascular Endothelial Growth Factor. Oncol (2015) 20(6):660–73. doi: 10.1634/theoncologist.2014-0465
79. Hicklin DJ, Ellis LM. Role of the Vascular Endothelial Growth Factor Pathway in Tumor Growth and Angiogenesis. J Clin Oncol (2005) 23(5):1011–27. doi: 10.1200/jco.2005.06.081
80. Linderholm BK, Hellborg H, Johansson U, Elmberger G, Skoog L, Lehtiö J, et al. Significantly Higher Levels of Vascular Endothelial Growth Factor (VEGF) and Shorter Survival Times for Patients With Primary Operable Triple-Negative Breast Cancer. Ann Oncol (2009) 20(10):1639–46. doi: 10.1093/annonc/mdp062
81. Nebbioso A, Tambaro FP, Dell’Aversana C, Altucci L. Cancer Epigenetics: Moving Forward. PloS Genet (2018) 14(6):e1007362. doi: 10.1371/journal.pgen.1007362
82. Dawson MA, Kouzarides T. Cancer Epigenetics: From Mechanism to Therapy. Cell (2012) 150(1):12–27. doi: 10.1016/j.cell.2012.06.013
83. Moore LD, Le T, Fan G. DNA Methylation and its Basic Function. Neuropsychopharmacology (2013) 38(1):23–38. doi: 10.1038/npp.2012.112
84. Lawrence M, Daujat S, Schneider R. Lateral Thinking: How Histone Modifications Regulate Gene Expression. Trends Genet (2016) 32(1):42–56. doi: 10.1016/j.tig.2015.10.007
85. Miranda Furtado CL, Dos Santos Luciano MC, Silva Santos RD, Furtado GP, Moraes MO, Pessoa C. Epidrugs: Targeting Epigenetic Marks in Cancer Treatment. Epigenetics (2019) 14(12):1164–76. doi: 10.1080/15592294.2019.1640546
86. Costa-Pinheiro P, Montezuma D, Henrique R, Jeronimo C. Diagnostic and Prognostic Epigenetic Biomarkers in Cancer. Epigenomics (2015) 7(6):1003–15. doi: 10.2217/epi.15.56
87. Esteller M, Silva JM, Dominguez G, Bonilla F, Matias-Guiu X, Lerma E, et al. Promoter Hypermethylation and BRCA1 Inactivation in Sporadic Breast and Ovarian Tumors. J Natl Cancer Inst (2000) 92(7):564–9. doi: 10.1093/jnci/92.7.564
88. Jones PA. DNA Methylation and Cancer. Oncogene (2002) 21(35):5358–60. doi: 10.1038/sj.onc.1205597
89. Catteau A, Harris WH, Xu CF, Solomon E. Methylation of the BRCA1 Promoter Region in Sporadic Breast and Ovarian Cancer: Correlation With Disease Characteristics. Oncogene (1999) 18(11):1957–65. doi: 10.1038/sj.onc.1202509
90. Siedlecki P, Zielenkiewicz P. Mammalian DNA Methyltransferases. Acta Biochim Pol (2006) 53(2):245–56. doi: 10.18388/abp.2006_3337
91. Shin E, Lee Y, Koo JS. Differential Expression of the Epigenetic Methylation-Related Protein DNMT1 by Breast Cancer Molecular Subtype and Stromal Histology. J Transl Med (2016) 14:87. doi: 10.1186/s12967-016-0840-x
92. Yang X, Ferguson AT, Nass SJ, Phillips DL, Butash KA, Wang SM, et al. Transcriptional Activation of Estrogen Receptor Alpha in Human Breast Cancer Cells by Histone Deacetylase Inhibition. Cancer Res (2000) 60(24):6890–4.
93. Kim IA, Kim JH, Shin JH, Kim IH, Kim JS, Wu HG, et al. A Histone Deacetylase InhibitorEnhances Radiosensitivity by Abrogating G2/M Arrest in Human Carcinoma Cells. Cancer Res Treat (2005) 37(2):122–8. doi: 10.4143/crt.2005.37.2.122
94. Malumbres M, Barbacid M. Cell Cycle, CDKs and Cancer: A Changing Paradigm. Nat Rev Cancer (2009) 9(3):153–66. doi: 10.1038/nrc2602
95. Malumbres M, Barbacid M. Mammalian Cyclin-Dependent Kinases. Trends Biochem Sci (2005) 30(11):630–41. doi: 10.1016/j.tibs.2005.09.005
96. Russo AA, Tong L, Lee JO, Jeffrey PD, Pavletich NP. Structural Basis for Inhibition of the Cyclin-Dependent Kinase Cdk6 by the Tumour Suppressor P16ink4a. Nature (1998) 395(6699):237–43. doi: 10.1038/26155
97. Finn RS, Crown JP, Lang I, Boer K, Bondarenko IM, Kulyk SO, et al. The Cyclin-Dependent Kinase 4/6 Inhibitor Palbociclib in Combination With Letrozole Versus Letrozole Alone as First-Line Treatment of Oestrogen Receptor-Positive, HER2-Negative, Advanced Breast Cancer (PALOMA-1/TRIO-18): A Randomised Phase 2 Study. Lancet Oncol (2015) 16(1):25–35. doi: 10.1016/S1470-2045(14)71159-3
98. Hortobagyi GN, Stemmer SM, Burris HA, Yap YS, Sonke GS, Paluch-Shimon S, et al. Ribociclib as First-Line Therapy for HR-Positive, Advanced Breast Cancer. N Engl J Med (2016) 375(18):1738–48. doi: 10.1056/NEJMoa1609709
99. Dickler MN, Tolaney SM, Rugo HS, Cortes J, Dieras V, Patt D, et al. MONARCH 1, A Phase II Study of Abemaciclib, a CDK4 and CDK6 Inhibitor, as a Single Agent, in Patients With Refractory HR(+)/HER2(-) Metastatic Breast Cancer. Clin Cancer Res (2017) 23(17):5218–24. doi: 10.1158/1078-0432.CCR-17-0754
100. Sledge GW Jr., Toi M, Neven P, Sohn J, Inoue K, Pivot X, et al. MONARCH 2: Abemaciclib in Combination With Fulvestrant in Women With HR+/HER2- Advanced Breast Cancer Who Had Progressed While Receiving Endocrine Therapy. J Clin Oncol (2017) 35(25):2875–84. doi: 10.1200/JCO.2017.73.7585
101. Rudner AD, Murray AW. The Spindle Assembly Checkpoint. Curr Opin Cell Biol (1996) 8(6):773–80. doi: 10.1016/s0955-0674(96)80077-9
102. Dominguez-Brauer C, Thu KL, Mason JM, Blaser H, Bray MR, Mak TW. Targeting Mitosis in Cancer: Emerging Strategies. Mol Cell (2015) 60(4):524–36. doi: 10.1016/j.molcel.2015.11.006
103. Denu RA, Zasadil LM, Kanugh C, Laffin J, Weaver BA, Burkard ME. Centrosome Amplification Induces High Grade Features and is Prognostic of Worse Outcomes in Breast Cancer. BMC Cancer (2016) 16:47. doi: 10.1186/s12885-016-2083-x
Keywords: targeted therapy, triple-negative breast cancer, poly (ADP-ribose) polymerase, immune checkpoint, epigenetic modification
Citation: Li Y, Zhan Z, Yin X, Fu S and Deng X (2021) Targeted Therapeutic Strategies for Triple-Negative Breast Cancer. Front. Oncol. 11:731535. doi: 10.3389/fonc.2021.731535
Received: 27 June 2021; Accepted: 12 October 2021;
Published: 28 October 2021.
Edited by:
Noam Falbel Pondé, A.C.Camargo Cancer Center, BrazilReviewed by:
Luisa Carbognin, Agostino Gemelli University Polyclinic, ItalyCopyright © 2021 Li, Zhan, Yin, Fu and Deng. This is an open-access article distributed under the terms of the Creative Commons Attribution License (CC BY). The use, distribution or reproduction in other forums is permitted, provided the original author(s) and the copyright owner(s) are credited and that the original publication in this journal is cited, in accordance with accepted academic practice. No use, distribution or reproduction is permitted which does not comply with these terms.
*Correspondence: Shujun Fu, c2h1anVuZnUyMDIwQGh1bm51LmVkdS5jbg==; Xiyun Deng, ZGVuZ3hpeXVubWVkQGh1bm51LmVkdS5jbg==
†These authors have contributed equally to this work and share first authorship
Disclaimer: All claims expressed in this article are solely those of the authors and do not necessarily represent those of their affiliated organizations, or those of the publisher, the editors and the reviewers. Any product that may be evaluated in this article or claim that may be made by its manufacturer is not guaranteed or endorsed by the publisher.
Research integrity at Frontiers
Learn more about the work of our research integrity team to safeguard the quality of each article we publish.