- 1Chifeng Municipal Hospital, Chifeng, China
- 2Qingdao Geneis Institute of Big Data Mining and Precision Medicine, Qingdao, China
- 3Geneis Beijing Co., Ltd., Beijing, China
- 4China National Intellectual Property Administration, Beijing, China
- 5School of Life Sciences, Jiangsu University, Zhenjiang, China
- 6Genetron Health (Beijing) Co. Ltd., Beijing, China
Background: Non-small cell lung cancer (NSCLC) is one of the most prevalent causes of cancer-related death worldwide. Recently, there are many important medical advancements on NSCLC, such as therapies based on tyrosine kinase inhibitors and immune checkpoint inhibitors. Most of these therapies require tumor molecular testing for selecting patients who would benefit most from them. As invasive biopsy is highly risky, NSCLC molecular testing based on liquid biopsy has received more and more attention recently.
Objective: We aimed to introduce liquid biopsy and its potential clinical applications in NSCLC patients, including cancer diagnosis, treatment plan prioritization, minimal residual disease detection, and dynamic monitoring on the response to cancer treatment.
Method: We reviewed recent studies on circulating tumor DNA (ctDNA) testing, which is a minimally invasive approach to identify the presence of tumor-related mutations. In addition, we evaluated potential clinical applications of ctDNA as blood biomarkers for advanced NSCLC patients.
Results: Most studies have indicated that ctDNA testing is critical in diagnosing NSCLC, predicting clinical outcomes, monitoring response to targeted therapies and immunotherapies, and detecting cancer recurrence. Moreover, the changes of ctDNA levels are associated with tumor mutation burden and cancer progression.
Conclusion: The ctDNA testing is promising in guiding the therapies on NSCLC patients.
Introduction
Liquid biopsy refers most often to the analysis of tumor-derived materials, including circulating tumor cell (CTC), circulating tumor DNA (ctDNA), circulating tumor RNA (ctRNA), exosome, and tumor-educated platelet (TEP) from blood plasma (Figure 1). CTCs are released from tumor tissue; ctDNA is secreted from apoptotic or necrotic tumor cells; and exosomes are membrane-bound vesicles released from tumor cells. Until now, ctDNA is the only circulating biomarker approved for selecting patients with targeted therapy, whereas other liquid biopsy sources, such as CTCs, RNA, exosomes, and TEP, are still at clinical research phase. Therefore, this review will focus on the interpretation of ctDNA testing and its potential clinical applications.
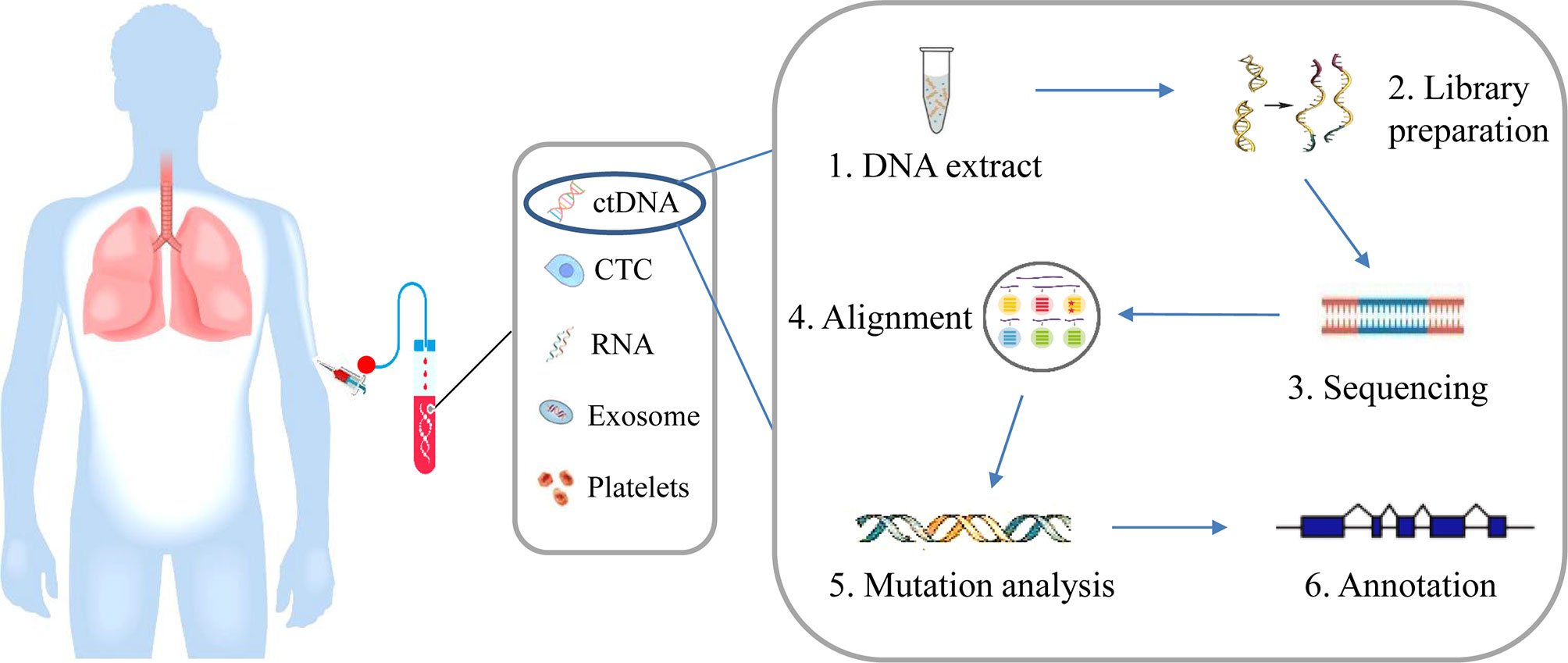
Figure 1 Overview of liquid biopsy. Blood is sampled from patients, which contains ctDNA, CTC, RNA, exosome, and tumor-educated platelets. CtDNA is extracted from blood plasma and gene variation can be analyzed by next generation sequencing involving a few steps, including DNA extraction, DNA library preparation, sequencing, sequence alignment, mutation annotation, and so on.
As reported by Mandel and Metais for the first time in 1948, circulating cell-free DNA (cfDNA) refers to double-stranded DNA fragments in liquid biopsy with lengths close to or lower than 200 base pair (bp) (1). CfDNA is present at low levels in the plasma of healthy persons, but at high levels in those of cancer patients (2, 3). CfDNA of tumor origin is referred to as ctDNA. Recent studies have confirmed that the fraction of ctDNA in total cfDNA greatly varied in cancer patients. Patients with early stage tumors present lower fractions of ctDNA than those in the advanced stage (4–6). Although the concentration of ctDNA has been suggested to predict the outcomes of patients with non-small cell lung cancer (NSCLC), the criterion for appropriate cutoffs is unavailable in clinical utility (7). However, even in the same cancer type, substantial variability has been observed indifferent patients (4, 8, 9). Mutational analysis of ctDNA has already been proven to be promising in early cancer detection and cancer recurrence evaluation (10–15).
In the last decade, important advancements have been achieved in NSCLC (16, 17). For example, small molecule tyrosine kinase inhibitors (TKIs) were proven to be effective for patients with advanced lung adenocarcinoma who harbor somatic mutation of epidermal growth factor receptor (EGFR), as well as the rearrangement of echinoderm microtubule-associated protein-like 4 (EML4) with anaplastic lymphoma (ALK) (18–22). More recently, immune checkpoint inhibitors (ICI) therapies have shown significant benefit in the treatment of patients with NSCLC (16, 23). Besides programmed cell death 1 (PD-1) and programmed cell death ligand 1 (PD-L1), tumor mutation burden (TMB) is a promising biomarker in predicting the outcomes of NSCLC patients with immunotherapy (24–27). Therefore, the accurate information of a cancer patient’s genetic status is critical in guiding personalized medication. Although tissue biopsy remains the gold standard for molecular testing of cancers, it presents some disadvantages in clinical situations. First, tumor biopsy is highly risky because of its invasive nature and is dangerous for extremely serious patients. Second, tumor biopsy is inappropriate in a few occasions like cancer early detection and recurrence surveillance, where multiple testing at different time points is necessary. Finally, tumor biopsy can only examine one tissue at a time and usually cannot reflect the mutational landscape of the whole body (28–31).
CtDNA testing provides a powerful and effective alternative method to tissue biopsy for cancer diagnosis, treatment, and prognosis. In the past 5 years, a high concordance has been confirmed between plasma and tissue samples, further encouraging the exploration of ctDNA in clinical applications (30, 32–34). Some studies have suggested that ctDNA levels can be used to monitor the response of patients to local and systemic therapies (35–37). Additionally, ctDNA testing can effectively predict responses to targeted therapies in multiple tumor types (4, 38–41). Despite the important achievements in ctDNA, there is no systematic review comprehensively introducing recent development in clinical applications of ctNDA as a biomarker in NSCLC, to our best knowledge.
In this review, we aim to describe the potential clinical application of ctDNA testing, from aiding cancer diagnosis to guiding patients’ treatment and detection of minimal residual disease, and to monitoring the response to cancer treatment in a dynamic way (Figure 2). Finally, a few promising approaches are highlighted, which may become available in a wide range of clinical applications in the future.
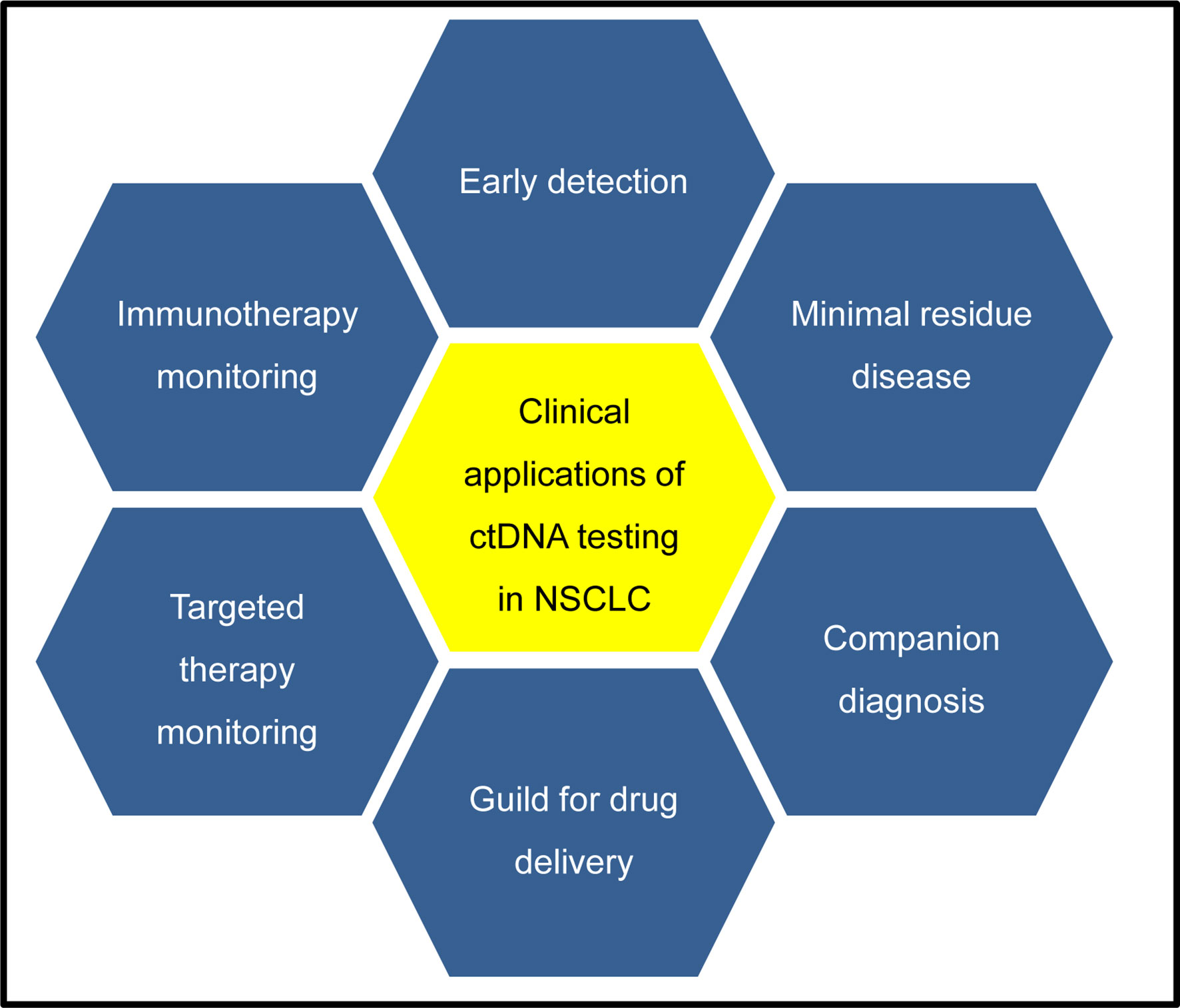
Figure 2 Potential clinical applications of ctDNA testing in NSCLC patients. CtDNA testing in early tumors detection are under development, which may identify patients with cancers at early stage when they are more likely to be curable. CtDNA testing in minimal residual disease detection after surgery can provide the evidence of tumor relapse, which may offer an opportunity for early intervention for patients according to risk of recurrence. For companion diagnosis, ctDNA testing is available to identify many somatic alterations, which can provide a guide for treatment decisions for patients with targeted therapies. Analysis of cfDNA has been used to monitor the response to targeted or immunotherapy, which can provide a molecular basis to guide the subsequent therapy choice.
Approaches of CtDNA Testing
Since cfDNA is derived from various cells and rapidly cleared in the circulation system, it usually presents in body fluid in a short time and with a limited level. Hence, it is critical to optimize experimental techniques for cfDNA (ctDNA) isolation and analysis. For cfDNA isolation, the anticoagulant EDTA can stabilize cfDNA and prevent the contamination with germline DNA released from normal blood cells. However, blood samples have to be processed by methods like leukocyte fixation within 6 h after collection. Leukocyte fixation allows for easy shipping and centralized processing, which can stabilize cfDNA and normal blood cells for two days (42). A recent study reported that specialized cfDNA collection tube with the stabilization reagent provides even higher flexibility for sample processing, i.e., up to 14 days without affecting cfDNA detection (43).
The fraction of ctDNA in total cfDNA can vary greatly form less than 0.1% to more than 90% (4). Several high-sensitivity approaches are available to analyze ctDNA even at low levels (Table 1), including peptide nucleic acids (PNA)-based methods (44), quantitative polymerase chain reaction (qPCR) and droplet digital PCR (ddPCR), beads emulsion amplification and magnetics (BEAMing) (45). However, those methods are not adequate to analyze multiple genes through a high-throughput screening. Several targeted next-generation sequencings (NGS) have been developed for ctDNA testing, including tagged amplicon-based sequencing (TAm-Seq) (46), cancer personalized profiling by deep sequencing (CAPP-Seq) (36), the targeted error correction sequencing (TEC-Seq) (47). TAm-Seq includes two steps of amplification. The first step of amplification is performed to capture the starting molecules present in the template by all primers, then, the second step of amplification is followed with a limited couple of primers in the access array. This approach is only detected in single-nucleotide variants (SNV), insertion, or deletions (indels) (46). CAPP-Seq is a capture-based NGS ctDNA detection method, the defined mutated regions are hybridized by the biotinylated probes. Several types of somatic mutations could be detected by CAPP-Seq, including SNV, indels, copy number alterations (CNAs), and rearrangements (36). TEC-Seq is based on targeted capture of multiple regions of the genome and deep sequencing of DNA fragments, which allow sensitive and specific detection of low abundance sequence alterations (47). Whole-exome sequencing or whole-genome sequencing with deep depth can provide a more comprehensive profiling of ctDNA (12, 48). However, because of the high costs and large volume of blood per patient, the application of these approaches to patients with advanced lung cancer is limited.
CtDNA Testing for Companion Diagnosis
Tumor molecular testing has become a fundamental practice to guide the selection of therapy in cancer medicine. Tissue biopsy remains the gold standard for patients with lung cancer diagnosis. However, invasive biopsy can be high risk, and patients would prefer liquid biopsy. This makes ctDNA testing an attractive tool, which could replace tissue biopsy in some specific situations. Although previous studies revealed a low concordance between the somatic variations detected from plasma and those detected from tissue samples (49, 50). For example, Torga and Pienta observed big differences in somatic mutations called from two different commercial ctDNA testing assays and those from tissue samples in 40 patients with metastatic prostate cancer (51). However, these studies may have bias. For example, tumor samples were collected at baseline time, whereas plasma samples were collected during the therapy. In addition, the enrolled patients had received therapy, which might lead to the alteration of mutation profiles. As a result, the discordance might be caused at least in partial by inappropriate experimental designs.
In the past years, a few larger and more carefully enrolled studies have indicated a relative high concordance between plasma and tissue samples obtained simultaneously, encouraging the clinical usage of ctDNA testing (30, 32–34). Recently, Roche’s Cobas plasma EGFR mutation test v2 was approved as the first ctDNA-testing tool by the Food and Drug Administration (FDA), which paved the way of liquid biopsy in clinical applications. Cobas plasma EGFR mutation test v2 can identify multiple mutations in exons 18, 19, 20, and 21 of EGFR in NSCLC patients, including exon 19 deletion, and L858R, G719X, S768I, L861Q, and T790M substitution. Among those, exon 19 deletion and L858R mutation are related to the increase of sensitivity to tyrosine kinase inhibitors (52). However, T790M mutation is often resistant to tyrosine kinase inhibitors, such as erlotinib, gefitinib, and afatinib, whereas it responds to osimertinib (29, 53). Hence, the test can guide the usage of tyrosine kinase inhibitors in treating NSCLC patients (54).
In addition to checking EGFR mutational status, ctDNA testing can also be used to detect mutations in other genes, such as BRAF mutations and ALK rearrangements. It has been indicated that NSCLC patients harboring BRAF mutations have clinical benefits from targeted therapies (55). ctDNA testing has been indicated to be effective in identifying both ALK point mutations and fusions in lung cancer patients, who will most likely to respond to crizotinib and other ALK tyrosine kinase inhibitors (11, 56). To display comprehensive mutation profiles in patients with NSCLC, large gene panels were developed to identify oncogenes and tumor suppressor genes via ctDNA testing (10, 36). A recent prospective study demonstrated genetic variations in eight genes, including BRAF, EGFR, and ERBB2 mutations, ALK, RET, and ROS1 rearrangements, MET amplifications and exon 14 skipping, were recommended as biomarkers via the Guardant360 test based on eight 70-gene NGS panels in metastatic NSCLC (10). These genetic variations were detected with a high concordance rate between ctDNA testing and tissue genotyping, which even reached more than 98% when only the FDA-approved markers (i.e., ALK, BRAF, EGFR, and ROS1) were considered (10). Hence, these approaches can provide a guide for treatment decisions of patients with NSCLC with lots of targeted therapies available or in progress.
CtDNA Testing for Detecting Minimal Residue Disease
Currently, there is a clinical challenge to determine which patients have a minimal residue disease after surgical resection, which may result in recurrence. However, adjuvant chemo-radiation therapy is not routinely used for cancer patients because of its toxic nature. Nowadays, serial computed tomography (CT) and positron emission tomography combined CT (PET/CT) imaging are used for surveilling advanced NSCLC patients after surgery or chemo-radiation therapy. However, identification of disease recurrence is always delayed by CT imaging because of the uncertain recurrent location and small size of tumor. Hence, liquid biopsy might provide an aid to predict the risk of disease recurrence.
The sensitivity of ctDNA testing relies upon the level of tumor DNA released into blood and collecting samples at optimal time. Several liquid biopsy approaches have been developed to identify low level of ctDNA even less than 0.01% of total cfDNA (37, 57). Indeed, the recent studies have demonstrated that ctDNA testing has an ability to detect minimal residue disease and tumor relapse after surgery therapy in several cancer types, including lung cancer (35, 58, 59). Interestingly, several key studies have shown that ctDNA testing can detect resistance mutations or disease progression prior to CT imaging (35, 60, 61).
The recent prospective study of the West Japan Oncology Group 8114LTR (WJOG8114LTR) evaluated the clinical significance of monitoring ctDNA in 57 patients with advanced lung adenocarcinoma harboring EGFR mutations during afatinib treatment. They indicated that a remarkable long progress-free survival (PFS) was observed in patients with undetectable EGFR mutations, whereas a short PFS was observed in patients with positive EGFR mutations (62). Similarly, another study has provided an evidence of ctDNA testing monitoring disease progression of patients with advanced NSCLC during treatment with erlotinib, and EGFR T790M mutation was detected by ctDNA testing earlier than clinically evident disease (60). Hence, ctDNA testing can monitor recurrence and save time for patients to receive available intervention and may prevent tumor cells spread and proliferation.
CtDNA Testing for Therapeutic Response
For monitoring therapeutic response, a repeat or serial molecular testing is required after one or more lines of therapy. Patients would prefer minimally invasive ctDNA testing if it can provide an effective power to predict the treatment, other than repeat invasive tumor biopsies because of their high risks. CtDNA was released into blood can be triggered by tumor cell death. Hence, dynamic changes of ctDNA concentrations may predict the response to the treatment. Indeed, previous studies have indicated that ctDNA levels are associated with disease situation in patients with NSCLC patients during therapy. The decreasing ctDNA levels are related with response to therapy, whereas the increasing ctDNA levels are related with progressive disease (7, 35–37, 60). A complete response could be expected from undetectable levels of ctDNA after serial testing. Therefore, ctDNA testing may provide an aid to guide chemotherapy decision or dose-based radiation therapy.
Circulating tumor DNA testing has also been confirmed to be able to detect targetable mutations that are involved in driving acquired resistance to therapy (12, 38, 39). One of the earliest studies has illustrated the development of resistance to cancer therapy using a serial ctDNA testing. In particular, the authors demonstrated that the emergence of EGFR T790M gatekeeper mutation was detected by ctDNA testing in patients with NSCLC after gefitinib treatment. This finding supported the hypothesis of selective pressure resulted from therapy. Several studies have made efforts to identify the presence of EGFR T790M by ctDNA testing in NSCLC patients might benefit from osimertinib, a third-generation EGFR inhibitor (29, 53). Interestingly, acquired EGFR C797S mutation was identified by ctDNA testing from 15 patients with NSCLC harboring EGFR T790M mutation undergoing osimertinib treatment, illustrating a novel acquired resistance mechanism to this EGFR inhibitor (63). Remarkably, EGFR T790M and other EGFR mutations can be identified by Roche’s Cobas plasma EGFR mutation test v2 which has been approved by FDA as an aid to guide decisions of specific EGFR inhibitors (54). It paves the way to utilize the approach of liquid biopsy to monitor targeted therapy, whereas it is a limitation to track other genetic alterations besides of EGFR mutation. Therefore, a comprehensive ctDNA analysis is required for the identification of genetic alterations to guide in the response to targeted therapies.
Indeed, several studies revealed the EGFR-independent mechanism of primary or acquired resistance to treatments of EGFR inhibitors, a bypass signaling pathway activated by the occurrence of gene variations mutation, such as mutations in BRAF, KRAS, PIK3CA, and amplification of MET (64–70). In particularly, the last update of the phase III AURA3 trial (no. NCT02151981) showed that emergence of MET amplification is detected by ctDNA testing in advanced NSCLC patients with EGFR T790M mutation during osimertinib treatment, which indicated that MET amplification is one of resistance mechanisms to this treatment. To overcome resistance, several case reports observed the therapeutic efficacy of MET inhibitor combined with EGFR inhibitor in patients with lung cancer appearing MET amplification detected by ctDNA testing after resistance to EGFR inhibitor (71–73). Moreover, a key clinical trial investigated the combination of capmatinib with gefitinib applied in patients with NSCLC, acquiring MET amplification after failure of EGFR inhibitor therapy (74).
In addition to EGFR mutations, ctDNA testing was also used for monitoring response or resistance to ALK inhibitors therapy in patients with lung cancer via identification of appearance of ALK variations, including ALK point mutations and rearrangements (11, 56). For example, the recent study identified novel ALK point mutations by ctDNA testing at the progression line after advanced ALK-positive NSCLC patients resistant to crizotinib treatment, revealed the resistance mechanisms on disease progression (56).
CtDNA Testing for Immunotherapy
Besides small molecule tyrosine kinase inhibitors therapy, immune checkpoint inhibitor (ICI) therapies have shown significant benefit in the treatment of different tumor types including NSCLC (16). However, only a subtype of NSCLC patients could benefit from ICI immunotherapies. In addition to PD-1 and PD-L1 biomarkers, tumor mutational burden (TMB) is a promising biomarker to predict clinical outcomes of NSCLC patients to ICI immunotherapies (24–26), and was approved by the FDA in 2020. Tissue biopsy remains the gold standard for molecular testing, whereas it is a clinical challenge to obtain adequate tumor tissue from advanced NSCLC patients by invasion biopsy. Thus, it needs to explore minimally invasive approach to aid in distinguishing patients who can benefit from ICI immunotherapies.
Nowadays, the concordance of blood TMB (bTMB) with tissue TMB (tTMB) has been confirmed by the whole-exon sequencing (WES) (75, 76). However, it is a challenge to apply WES approach in clinical routine, mainly because of the high gDNA inputs and high costs. Therefore, the feasibility of blood TMB analyzed by targeted NGS panels needs to be assessed. In a preliminary study, the authors used targeted NGS panels to assess the concordance of blood TMB (bTMB) with tissue TMB (tTMB) in the enrolled 97 patients, whereas the obtained correlation was unsatisfying due to the low concordance between bTMB and tTMB (77). A possible reason is the lack of standard method for TMB assessment in this study. For example, the Foundation-One panel was used for tissue TMB analysis, while the Guardant360 was used for blood TMB analysis. The different sequencing panels resulted in different coverage of genomic regions. Moreover, the mutation types used for TMB calculation were different in the studies. Furthermore, the cutoff of TMB values were varied to classify these patients. Therefore, the standard sequencing panels used for comparing bTMB and tTMB should be considered carefully in future studies.
Remarkably, the very recent studies evaluated the concordance of bTMB with tTMB in advanced NSCLC patients and revealed that bTMB estimated by ctDNA testing is feasible to predict the clinical outcomes of ICI immunotherapy (78, 79). For example, Wang et al. optimized gene panel size and established 150 genes panel to estimate the bTMB value with response to ICI immunotherapies. The authors showed a high bTMB value of ≥ 6, which is related to long progression-free survival, suggesting that bTMB may be a promising biomarker to predict clinical benefit for advanced NSCLC patients to anti-PD-1 and anti-PD-L1 therapies (78). These studies provided the strong evidences to support bTMB determined by the targeted NGS panels. However, it still improves the sensitivity of ctDNA testing and develops a robust targeted NGS panels, and more clinical trials are required to confirm the abilities of bTMBas, a biomarker in liquid biopsy in the prediction of the clinical benefit of NSCLC patients from ICI immunotherapies.
Typically, a very recent study showed a set of neoantigens genes detected by personalized ctDNA testing, which can monitor the clinical response to ICI immunotherapies for advanced NSCLC patients (80). It is known that mutated neoantigens are the key targets of tumor-specific T-cells undergoing ICI immunotherapies. Therefore, the study focused on neoantigens-coding mutations detected by ctDNA testing during ICI immunotherapies. The authors showed the neoantigen-related mutations detected in nine of ten patients after ICI immunotherapies, but undetectable only in one patient, and suggested that activation of tumor-specific T cells might contribute to the response to ICI immunotherapies (80). However, it needs to validate the feasibility of personalized ctDNA testing with a large cohort in future studies to maximize the clinical outcomes of NSCLC patients in ICI immunotherapies.
Conclusions
In conclusion, ctDNA testing is going to become a powerful approach applied in the clinical management of NSCLC patients at diagnosis, dynamic monitoring drug treatment or disease progression. However, it is still a great challenge because of the very low level of ctDNA released in the blood. In this regard, several ultra-sensitive and specific approaches were developed to detect somatic alterations by NGS-based ctDNA testing (36, 47, 81). In addition, several studies have shown tumor-derived DNA detected in other body fluids (82–84). Indeed, tumor-derived DNA from pleural effusion in patients with lung cancer was detected with high levels, suggesting that pleural effusion testing is an alternative and feasible method for mutations identification (82). However, more clinical trials are required for verifying these findings and for providing the standard operation procedure. Moreover, ctDNA testing has attracted great attention in early tumor detection of several common cancer types (57, 85–87), although it is still a long way to apply ctDNA testing in the clinical routine.
Author Contributions
JY, BG, TM, and LL conceived the concept of the work. JY, YH, MZ, BJ, GT, and YG performed the experiments. YZ, JY, YH, and MZ wrote the paper. All authors contributed to the article and approved the submitted version.
Funding
This research was funded by the Scientific Research Projects of the Inner Mongolian (no. 201702135), the Natural Science Foundation of Hunan province (no. 2018JJ3570), and the Project to Introduce Intelligence from Oversea Experts to Changsha City (no. 2089901).
Conflict of Interest
JY, BJ, and GT were employed by Geneis Beijing Co., Ltd. BG and TM were employed by Genetron Health (Beijing) Co. Ltd.
The remaining authors declare that the research was conducted in the absence of any commercial or financial relationships that could be construed as a potential conflict of interest.
Publisher’s Note
All claims expressed in this article are solely those of the authors and do not necessarily represent those of their affiliated organizations, or those of the publisher, the editors and the reviewers. Any product that may be evaluated in this article, or claim that may be made by its manufacturer, is not guaranteed or endorsed by the publisher.
References
1. Mandel P, Metais P, Métais P. Les Acides Nucléiques Du Plasma Sanguin Chez L’homme. Cr Acad Sci Paris (1948) 142(3–4):241–3.
2. Leon SA, Shapiro B, Sklaroff DM, Yaros MJ. Free DNA in the Serum of Cancer Patients and the Effect of Therapy. Cancer Res (1977) 37(3):646–50.
3. Giacona MB, Ruben GC, Iczkowski KA, Roos TB, Porter DM, Sorenson GD. Cell-Free DNA in Human Blood Plasma: Length Measurements in Patients With Pancreatic Cancer and Healthy Controls. Pancreas (1998) 17(1):89–97. doi: 10.1097/00006676-199807000-00012
4. Bettegowda C, Sausen M, Leary RJ, Kinde I, Wang Y, Agrawal N, et al. Detection of Circulating Tumor DNA in Early- and Late-Stage Human Malignancies. Sci Transl Med (2014) 6(224):224ra24. doi: 10.1158/1538-7445.AM2014-5606
5. Diehl F, Schmidt K, Choti MA, Romans K, Goodman S, Li M, et al. Circulating Mutant DNA to Assess Tumor Dynamics. Nat Med (2008) 14(9):985–90. doi: 10.1038/nm.1789
6. Shao X, He Y, Ji M, Chen X, Qi J, Shi W, et al. Quantitative Analysis of Cell-Free DNA in Ovarian Cancer. Oncol Lett (2015) 10(6):3478–82. doi: 10.3892/ol.2015.3771
7. Corcoran RB, Chabner BA. Application of Cell-Free DNA Analysis to Cancer Treatment. N Engl J Med (2018) 379(18):1754–65. doi: 10.1056/NEJMra1706174
8. He B, Lang J, Wang B, Liu X, Lu Q, He J, et al. TOOme: A Novel Computational Framework to Infer Cancer Tissue-of-Origin by Integrating Both Gene Mutation and Expression. Front Bioeng Biotechnol (2020) 8:394. doi: 10.3389/fbioe.2020.00394
9. Liang Y, Wang H, Yang J, Li X, Dai C, Shao P, et al. A Deep Learning Framework to Predict Tumor Tissue-of-Origin Based on Copy Number Alteration. Front Bioeng Biotechnol (2020) 8:701. doi: 10.3389/fbioe.2020.00701
10. Leighl NB, Page RD, Raymond VM, Daniel DB, Divers SG, Reckamp KL, et al. Clinical Utility of Comprehensive Cell-Free DNA Analysis to Identify Genomic Biomarkers in Patients With Newly Diagnosed Metastatic Non-Small Cell Lung Cancer. Clin Cancer Res (2019) 25(15):4691–700. doi: 10.1158/1078-0432.CCR-19-0624
11. McCoach CE, Blakely CM, Banks KC, Levy B, Chue BM, Raymond VM, et al. Clinical Utility of Cell-Free DNA for the Detection of ALK Fusions and Genomic Mechanisms of ALK Inhibitor Resistance in Non-Small Cell Lung Cancer. Clin Cancer Res (2018) 24(12):2758–70. doi: 10.1158/1078-0432.CCR-17-2588
12. Murtaza M, Dawson SJ, Tsui DW, Gale D, Forshew T, Piskorz AM, et al. Non-Invasive Analysis of Acquired Resistance to Cancer Therapy by Sequencing of Plasma DNA. Nature (2013) 497(7447):108–12. doi: 10.1038/nature12065
13. Song Z, Chen X, Shi Y, Huang R, Wang W, Zhu K, et al. Evaluating the Potential of T Cell Receptor Repertoires in Predicting the Prognosis of Resectable Non-Small Cell Lung Cancers. Mol Ther Methods Clin Dev (2020) 18:73–83. doi: 10.1016/j.omtm.2020.05.020
14. He B, Zhang Y, Zhou Z, Wang B, Liang Y, Lang J, et al. A Neural Network Framework for Predicting the Tissue-of-Origin of 15 Common Cancer Types Based on RNA-Seq Data. Front Bioeng Biotechnol (2020) 8:737. doi: 10.3389/fbioe.2020.00737
15. Liu F, Peng L, Tian G, Yang J, Chen H, Hu Q, et al. Identifying Small Molecule-miRNA Associations Based on Credible Negative Sample Selection and Random Walk. Front Bioeng Biotechnol (2020) 8:131. doi: 10.3389/fbioe.2020.00131
16. Herbst RS, Morgensztern D, Boshoff C. The Biology and Management of Non-Small Cell Lung Cancer. Nature (2018) 553(7689):446–54. doi: 10.1038/nature25183
17. Testa U, Castelli G, Pelosi E. Lung Cancers: Molecular Characterization, Clonal Heterogeneity and Evolution, and Cancer Stem Cells. Cancers (Basel) (2018) 10(8):248. doi: 10.3390/cancers10080248
18. Paez JG, Janne PA, Lee JC, Tracy S, Greulich H, Gabriel S, et al. EGFR Mutations in Lung Cancer: Correlation With Clinical Response to Gefitinib Therapy. Science (2004) 304(5676):1497–500. doi: 10.1126/science.1099314
19. Soda M, Choi YL, Enomoto M, Takada S, Yamashita Y, Ishikawa S, et al. Identification of the Transforming EML4-ALK Fusion Gene in Non-Small-Cell Lung Cancer. Nature (2007) 448(7153):561–6. doi: 10.1038/nature05945
20. Robichaux JP, Elamin YY, Tan Z, Carter BW, Zhang S, Liu S, et al. Mechanisms and Clinical Activity of an EGFR and HER2 Exon 20-Selective Kinase Inhibitor in Non-Small Cell Lung Cancer. Nat Med (2018) 24(5):638–46. doi: 10.1038/s41591-018-0007-9
21. Ramalingam SS, Vansteenkiste J, Planchard D, Cho BC, Gray JE, Ohe Y, et al. Overall Survival With Osimertinib in Untreated, EGFR-Mutated Advanced NSCLC. N Engl J Med (2020) 382(1):41–50. doi: 10.1056/NEJMoa1913662
22. Liu C, Wei D, Xiang J, Ren F, Huang L, Lang J, et al. An Improved Anticancer Drug-Response Prediction Based on an Ensemble Method Integrating Matrix Completion and Ridge Regression. Mol Ther Nucleic Acids (2020) 21:676–86. doi: 10.1016/j.omtn.2020.07.003
23. Cai Y, Huang T, Yang J. Applications of Bioinformatics and Systems Biology in Precision Medicine and Immunooncology. BioMed Res Int (2018) 2018:1427978. doi: 10.1155/2018/1427978
24. Samstein RM, Lee CH, Shoushtari AN, Hellmann MD, Shen R, Janjigian YY, et al. Tumor Mutational Load Predicts Survival After Immunotherapy Across Multiple Cancer Types. Nat Genet (2019) 51(2):202–6. doi: 10.1038/s41588-018-0312-8
25. Carbone DP, Reck M, Paz-Ares L, Creelan B, Horn L, Steins M, et al. First-Line Nivolumab in Stage IV or Recurrent Non-Small-Cell Lung Cancer. N Engl J Med (2017) 376(25):2415–26. doi: 10.1056/NEJMoa1613493
26. Hellmann MD, Ciuleanu TE, Pluzanski A, Lee JS, Otterson GA, Audigier-Valette C, et al. Nivolumab Plus Ipilimumab in Lung Cancer With a High Tumor Mutational Burden. N Engl J Med (2018) 378(22):2093–104. doi: 10.1056/NEJMoa1801946
27. Zhou X, Dong Q, Kan X, Peng L, Xu X, Fang Y, et al. Immunomodulatory Activity of a Novel Polysaccharide From Lonicera Japonica in Immunosuppressed Mice Induced by Cyclophosphamide. PloS One (2018) 13(10):e0204152. doi: 10.1371/journal.pone.0204152
28. Hata A, Katakami N, Yoshioka H, Kaji R, Masago K, Fujita S, et al. Spatiotemporal T790M Heterogeneity in Individual Patients With EGFR-Mutant Non-Small-Cell Lung Cancer After Acquired Resistance to EGFR-TKI. J Thorac Oncol (2015) 10(11):1553–9. doi: 10.1097/JTO.0000000000000647
29. Oxnard GR, Thress KS, Alden RS, Lawrance R, Paweletz CP, Cantarini M, et al. Association Between Plasma Genotyping and Outcomes of Treatment With Osimertinib (AZD9291) in Advanced Non-Small-Cell Lung Cancer. J Clin Oncol (2016) 34(28):3375–82. doi: 10.1200/JCO.2016.66.7162
30. Zill OA, Greene C, Sebisanovic D, Siew LM, Leng J, Vu M, et al. Cell-Free DNA Next-Generation Sequencing in Pancreatobiliary Carcinomas. Cancer Discov (2015) 5(10):1040–8. doi: 10.1158/2159-8290.CD-15-0274
31. Liu H, Qiu C, Wang B, Bing P, Tian G, Zhang X, et al. Evaluating DNA Methylation, Gene Expression, Somatic Mutation, and Their Combinations in Inferring Tumor Tissue-Of-Origin. Front Cell Dev Biol (2021) 9:619330. doi: 10.3389/fcell.2021.619330
32. Adalsteinsson VA, Ha G, Freeman SS, Choudhury AD, Stover DG, Parsons HA, et al. Scalable Whole-Exome Sequencing of Cell-Free DNA Reveals High Concordance With Metastatic Tumors. Nat Commun (2017) 8(1):1324. doi: 10.1038/s41467-017-00965-y
33. Schrock AB, Pavlick D, Klempner SJ, Chung JH, Forcier B, Welsh A, et al. Hybrid Capture-Based Genomic Profiling of Circulating Tumor DNA From Patients With Advanced Cancers of the Gastrointestinal Tract or Anus. Clin Cancer Res (2018) 24(8):1881–90. doi: 10.1158/1078-0432.CCR-17-3103
34. Strickler JH, Loree JM, Ahronian LG, Parikh AR, Niedzwiecki D, Pereira AAL, et al. Genomic Landscape of Cell-Free DNA in Patients With Colorectal Cancer. Cancer Discov (2018) 8(2):164–73. doi: 10.1158/2159-8290.CD-17-1009
35. Chaudhuri AA, Chabon JJ, Lovejoy AF, Newman AM, Stehr H, Azad TD, et al. Early Detection of Molecular Residual Disease in Localized Lung Cancer by Circulating Tumor DNA Profiling. Cancer Discov (2017) 7(12):1394–403. doi: 10.1158/2159-8290.CD-17-0716
36. Newman AM, Bratman SV, To J, Wynne JF, Eclov NC, Modlin LA, et al. An Ultrasensitive Method for Quantitating Circulating Tumor DNA With Broad Patient Coverage. Nat Med (2014) 20(5):548–54. doi: 10.1038/nm.3519
37. Chaudhuri AA, Binkley MS, Osmundson EC, Alizadeh AA, Diehn M. Predicting Radiotherapy Responses and Treatment Outcomes Through Analysis of Circulating Tumor DNA. Semin Radiat Oncol (2015) 25(4):305–12. doi: 10.1016/j.semradonc.2015.05.001
38. Corcoran RB, Andre T, Atreya CE, Schellens JHM, Yoshino T, Bendell JC, et al. Combined BRAF, EGFR, and MEK Inhibition in Patients With BRAF(V600E)-Mutant Colorectal Cancer. Cancer Discov (2018) 8(4):428–43. doi: 10.1158/2159-8290.CD-17-1226
39. Dawson SJ, Tsui DW, Murtaza M, Biggs H, Rueda OM, Chin SF, et al. Analysis of Circulating Tumor DNA to Monitor Metastatic Breast Cancer. N Engl J Med (2013) 368(13):1199–209. doi: 10.1056/NEJMoa1213261
40. Douillard JY, Ostoros G, Cobo M, Ciuleanu T, Cole R, McWalter G, et al. Gefitinib Treatment in EGFR Mutated Caucasian NSCLC: Circulating-Free Tumor DNA as a Surrogate for Determination of EGFR Status. J Thorac Oncol (2014) 9(9):1345–53. doi: 10.1097/JTO.0000000000000263
41. Gray ES, Rizos H, Reid AL, Boyd SC, Pereira MR, Lo J, et al. Circulating Tumor DNA to Monitor Treatment Response and Detect Acquired Resistance in Patients With Metastatic Melanoma. Oncotarget (2015) 6(39):42008–18. doi: 10.18632/oncotarget.5788
42. Kang Q, Henry NL, Paoletti C, Jiang H, Vats P, Chinnaiyan AM, et al. Comparative Analysis of Circulating Tumor DNA Stability In K3EDTA, Streck, and CellSave Blood Collection Tubes. Clin Biochem (2016) 49(18):1354–60. doi: 10.1016/j.clinbiochem.2016.03.012
43. Alidousty C, Brandes D, Heydt C, Wagener S, Wittersheim M, Schafer SC, et al. Comparison of Blood Collection Tubes From Three Different Manufacturers for the Collection of Cell-Free DNA for Liquid Biopsy Mutation Testing. J Mol Diagn (2017) 19(5):801–4. doi: 10.1016/j.jmoldx.2017.06.004
44. Newton CR, Graham A, Heptinstall LE, Powell SJ, Summers C, Kalsheker N, et al. Analysis of Any Point Mutation in DNA. The Amplification Refractory Mutation System (ARMS). Nucleic Acids Res (1989) 17(7):2503–16. doi: 10.1093/nar/17.7.2503
45. Wan JCM, Massie C, Garcia-Corbacho J, Mouliere F, Brenton JD, Caldas C, et al. Liquid Biopsies Come of Age: Towards Implementation of Circulating Tumour DNA. Nat Rev Cancer (2017) 17(4):223–38. doi: 10.1038/nrc.2017.7
46. Gale D, Lawson ARJ, Howarth K, Madi M, Durham B, Smalley S, et al. Development of a Highly Sensitive Liquid Biopsy Platform to Detect Clinically-Relevant Cancer Mutations at Low Allele Fractions in Cell-Free DNA. PloS One (2018) 13(3):e0194630. doi: 10.1371/journal.pone.0194630
47. Phallen J, Sausen M, Adleff V, Leal A, Hruban C, White J, et al. Direct Detection of Early-Stage Cancers Using Circulating Tumor DNA. Sci Transl Med (2017) 9(403):eaan2415. doi: 10.1126/scitranslmed.aan2415
48. Leary RJ, Sausen M, Kinde I, Papadopoulos N, Carpten JD, Craig D, et al. Detection of Chromosomal Alterations in the Circulation of Cancer Patients With Whole-Genome Sequencing. Sci Transl Med (2012) 4(162):162ra154. doi: 10.1126/scitranslmed.3004742
49. Kuderer NM, Burton KA, Blau S, Rose AL, Parker S, Lyman GH, et al. Comparison of 2 Commercially Available Next-Generation Sequencing Platforms in Oncology. JAMA Oncol (2017) 3(7):996–8. doi: 10.1001/jamaoncol.2016.4983
50. Chae YK, Davis AA, Carneiro BA, Chandra S, Mohindra N, Kalyan A, et al. Concordance Between Genomic Alterations Assessed by Next-Generation Sequencing in Tumor Tissue or Circulating Cell-Free DNA. Oncotarget (2016) 7(40):65364–73. doi: 10.18632/oncotarget.11692
51. Torga G, Pienta KJ. Patient-Paired Sample Congruence Between 2 Commercial Liquid Biopsy Tests. JAMA Oncol (2018) 4(6):868–70. doi: 10.1001/jamaoncol.2017.4027
52. Karachaliou N, Mayo-de las Casas C, Queralt C, de Aguirre I, Melloni B, Cardenal F, et al. Association of EGFR L858R Mutation in Circulating Free DNA With Survival in the EURTAC Trial. JAMA Oncol (2015) 1(2):149–57. doi: 10.1001/jamaoncol.2014.257
53. Chabon JJ, Simmons AD, Lovejoy AF, Esfahani MS, Newman AM, Haringsma HJ, et al. Circulating Tumour DNA Profiling Reveals Heterogeneity of EGFR Inhibitor Resistance Mechanisms in Lung Cancer Patients. Nat Commun (2016) 7:11815. doi: 10.1038/ncomms11815
54. Malapelle U, Sirera R, Jantus-Lewintre E, Reclusa P, Calabuig-Farinas S, Blasco A, et al. Profile of the Roche Cobas(R) EGFR Mutation Test V2 for non-Small Cell Lung Cancer. Expert Rev Mol Diagn (2017) 17(3):209–15. doi: 10.1080/14737159.2017.1288568
55. Guibert N, Pradines A, Casanova A, Farella M, Keller L, Soria JC, et al. Detection and Monitoring of the BRAF Mutation in Circulating Tumor Cells and Circulating Tumor DNA in BRAF-Mutated Lung Adenocarcinoma. J Thorac Oncol (2016) 11(9):e109–12. doi: 10.1016/j.jtho.2016.05.001
56. Bordi P, Tiseo M, Rofi E, Petrini I, Restante G, Danesi R, et al. Detection of ALK and KRAS Mutations in Circulating Tumor DNA of Patients With Advanced ALK-Positive NSCLC With Disease Progression During Crizotinib Treatment. Clin Lung Cancer (2017) 18(6):692–7. doi: 10.1016/j.cllc.2017.04.013
57. Cohen JD, Li L, Wang Y, Thoburn C, Afsari B, Danilova L, et al. Detection and Localization of Surgically Resectable Cancers With a Multi-Analyte Blood Test. Science (2018) 359(6378):926–30. doi: 10.1126/science.aar3247
58. Garcia-Murillas I, Schiavon G, Weigelt B, Ng C, Hrebien S, Cutts RJ, et al. Mutation Tracking in Circulating Tumor DNA Predicts Relapse in Early Breast Cancer. Sci Transl Med (2015) 7(302):302ra133. doi: 10.1126/scitranslmed.aab0021
59. Sausen M, Phallen J, Adleff V, Jones S, Leary RJ, Barrett MT, et al. Clinical Implications of Genomic Alterations in the Tumour and Circulation of Pancreatic Cancer Patients. Nat Commun (2015) 6:7686. doi: 10.1158/1538-7445.AM2015-619
60. Sorensen BS, Wu L, Wei W, Tsai J, Weber B, Nexo E, et al. Monitoring of Epidermal Growth Factor Receptor Tyrosine Kinase Inhibitor-Sensitizing and Resistance Mutations in the Plasma DNA of Patients With Advanced Non-Small Cell Lung Cancer During Treatment With Erlotinib. Cancer (2014) 120(24):3896–901. doi: 10.1002/cncr.28964
61. Abbosh C, Birkbak NJ, Wilson GA, Jamal-Hanjani M, Constantin T, Salari R, et al. Phylogenetic ctDNA Analysis Depicts Early-Stage Lung Cancer Evolution. Nature (2017) 545(7655):446–51. doi: 10.1038/nature22364
62. Akamatsu H, Koh Y, Okamoto I, Fujimoto D, Bessho A, Azuma K, et al. Clinical Significance of Monitoring EGFR Mutation in Plasma Using Multiplexed Digital PCR in EGFR Mutated Patients Treated With Afatinib (West Japan Oncology Group 8114LTR Study). Lung Cancer (2019) 131:128–33. doi: 10.1016/j.lungcan.2019.03.021
63. Thress KS, Paweletz CP, Felip E, Cho BC, Stetson D, Dougherty B, et al. Acquired EGFR C797S Mutation Mediates Resistance to AZD9291 in Non-Small Cell Lung Cancer Harboring EGFR T790M. Nat Med (2015) 21(6):560–2. doi: 10.1038/nm.3854
64. Hong S, Gao F, Fu S, Wang Y, Fang W, Huang Y, et al. Concomitant Genetic Alterations With Response to Treatment and Epidermal Growth Factor Receptor Tyrosine Kinase Inhibitors in Patients With EGFR-Mutant Advanced Non-Small Cell Lung Cancer. JAMA Oncol (2018) 4(5):739–42. doi: 10.1001/jamaoncol.2018.0049
65. Jakobsen JN, Santoni-Rugiu E, Grauslund M, Melchior L, Sorensen JB. Concomitant Driver Mutations in Advanced EGFR-Mutated non-Small-Cell Lung Cancer and Their Impact on Erlotinib Treatment. Oncotarget (2018) 9(40):26195–208. doi: 10.18632/oncotarget.25490
66. Rachiglio AM, Fenizia F, Piccirillo MC, Galetta D, Crino L, Vincenzi B, et al. The Presence of Concomitant Mutations Affects the Activity of EGFR Tyrosine Kinase Inhibitors in EGFR-Mutant Non-Small Cell Lung Cancer (NSCLC) Patients. Cancers (Basel) (2019) 11(3):341. doi: 10.3390/cancers11030341
67. Del Re M, Bordi P, Rofi E, Restante G, Valleggi S, Minari R, et al. The Amount of Activating EGFR Mutations in Circulating Cell-Free DNA Is a Marker to Monitor Osimertinib Response. Br J Cancer (2018) 119(10):1252–8. doi: 10.1038/s41416-018-0238-z
68. Minari R, Bordi P, La Monica S, Squadrilli A, Leonetti A, Bottarelli L, et al. Concurrent Acquired BRAF V600E Mutation and MET Amplification as Resistance Mechanism of First-Line Osimertinib Treatment in a Patient With EGFR-Mutated NSCLC. J Thorac Oncol (2018) 13(6):e89–91. doi: 10.1016/j.jtho.2018.03.013
69. Liu X, Yang J, Zhang Y, Fang Y, Wang F, Wang J, et al. A Systematic Study on Drug-Response Associated Genes Using Baseline Gene Expressions of the Cancer Cell Line Encyclopedia. Sci Rep (2016) 6:22811. doi: 10.1038/srep22811
70. Xu X, Long H, Xi B, Ji B, Li Z, Dang Y, et al. Molecular Network-Based Drug Prediction in Thyroid Cancer. Int J Mol Sci (2019) 20(2):263. doi: 10.3390/ijms20020263
71. Zhu VW, Schrock AB, Ali SM, Ou SI. Differential Response to a Combination of Full-Dose Osimertinib and Crizotinib in a Patient With EGFR-Mutant non-Small Cell Lung Cancer and Emergent MET Amplification. Lung Cancer (Auckl) (2019) 10:21–6. doi: 10.2147/LCTT.S190403
72. Deng L, Kiedrowski LA, Ravera E, Cheng H, Halmos B. Response to Dual Crizotinib and Osimertinib Treatment in a Lung Cancer Patient With MET Amplification Detected by Liquid Biopsy Who Acquired Secondary Resistance to EGFR Tyrosine Kinase Inhibition. J Thorac Oncol (2018) 13(9):e169–72. doi: 10.1016/j.jtho.2018.04.007
73. Yang J, Peng S, Zhang B, Houten S, Schadt E, Zhu J, et al. Human Geroprotector Discovery by Targeting the Converging Subnetworks of Aging and Age-Related Diseases. Geroscience (2020) 42(1):353–72. doi: 10.1007/s11357-019-00106-x
74. Wu YL, Zhang L, Kim DW, Liu X, Lee DH, Yang JC, et al. Phase Ib/II Study of Capmatinib (INC280) Plus Gefitinib After Failure of Epidermal Growth Factor Receptor (EGFR) Inhibitor Therapy in Patients With EGFR-Mutated, MET Factor-Dysregulated Non-Small-Cell Lung Cancer. J Clin Oncol (2018) 36(31):3101–9. doi: 10.1200/JCO.2018.77.7326
75. Khagi Y, Goodman AM, Daniels GA, Patel SP, Sacco AG, Randall JM, et al. Hypermutated Circulating Tumor DNA: Correlation With Response to Checkpoint Inhibitor-Based Immunotherapy. Clin Cancer Res (2017) 23(19):5729–36. doi: 10.1158/1078-0432.CCR-17-1439
76. Koeppel F, Blanchard S, Jovelet C, Genin B, Marcaillou C, Martin E, et al. Whole Exome Sequencing for Determination of Tumor Mutation Load in Liquid Biopsy From Advanced Cancer Patients. PloS One (2017) 12(11):e0188174. doi: 10.1371/journal.pone.0188174
77. Fenizia F, Pasquale R, Roma C, Bergantino F, Iannaccone A, Normanno N. Measuring Tumor Mutation Burden in Non-Small Cell Lung Cancer: Tissue Versus Liquid Biopsy. Transl Lung Cancer Res (2018) 7(6):668–77. doi: 10.21037/tlcr.2018.09.23
78. Wang Z, Duan J, Cai S, Han M, Dong H, Zhao J, et al. Assessment of Blood Tumor Mutational Burden as a Potential Biomarker for Immunotherapy in Patients With Non-Small Cell Lung Cancer With Use of a Next-Generation Sequencing Cancer Gene Panel. JAMA Oncol (2019) 5(5):696–702. doi: 10.1001/jamaoncol.2018.7098
79. Gandara DR, Paul SM, Kowanetz M, Schleifman E, Zou W, Li Y, et al. Blood-Based Tumor Mutational Burden as a Predictor of Clinical Benefit in Non-Small-Cell Lung Cancer Patients Treated With Atezolizumab. Nat Med (2018) 24(9):1441–8. doi: 10.1038/s41591-018-0134-3
80. Jia Q, Chiu L, Wu S, Bai J, Peng L, Zheng L, et al. Tracking Neoantigens by Personalized Circulating Tumor DNA Sequencing During Checkpoint Blockade Immunotherapy in Non-Small Cell Lung Cancer. Adv Sci (Weinh) (2020) 7(9):1903410. doi: 10.1002/advs.201903410
81. Pecuchet N, Rozenholc Y, Zonta E, Pietrasz D, Didelot A, Combe P, et al. Analysis of Base-Position Error Rate of Next-Generation Sequencing to Detect Tumor Mutations in Circulating DNA. Clin Chem (2016) 62(11):1492–503. doi: 10.1373/clinchem.2016.258236
82. Villatoro S, Mayo-de-Las-Casas C, Jordana-Ariza N, Viteri-Ramirez S, Garzon-Ibanez M, Moya-Horno I, et al. Prospective Detection of Mutations in Cerebrospinal Fluid, Pleural Effusion, and Ascites of Advanced Cancer Patients to Guide Treatment Decisions. Mol Oncol (2019) 13(12):2633–45. doi: 10.1002/1878-0261.12574
83. Wang Y, Springer S, Zhang M, McMahon KW, Kinde I, Dobbyn L, et al. Detection of Tumor-Derived DNA in Cerebrospinal Fluid of Patients With Primary Tumors of the Brain and Spinal Cord. Proc Natl Acad Sci USA (2015) 112(31):9704–9. doi: 10.1073/pnas.1511694112
84. Hummelink K, Muller M, Linders TC, van der Noort V, Nederlof PM, Baas P, et al. Cell-Free DNA in the Supernatant of Pleural Effusion can be Used to Detect Driver and Resistance Mutations, and can Guide Tyrosine Kinase Inhibitor Treatment Decisions. ERJ Open Res (2019) 5(1):00016–2019. doi: 10.1183/23120541.00016-2019
85. Cristiano S, Leal A, Phallen J, Fiksel J, Adleff V, Bruhm DC, et al. Genome-Wide Cell-Free DNA Fragmentation in Patients With Cancer. Nature (2019) 570(7761):385–9. doi: 10.1038/s41586-019-1272-6
86. Shen SY, Singhania R, Fehringer G, Chakravarthy A, Roehrl MHA, Chadwick D, et al. Sensitive Tumour Detection and Classification Using Plasma Cell-Free DNA Methylomes. Nature (2018) 563(7732):579–83. doi: 10.1038/s41586-018-0703-0
Keywords: non-small cell lung cancer, circulating tumor DNA, molecular testing, liquid biopsy, immunotherapies, therapeutic response
Citation: Yang J, Hui Y, Zhang Y, Zhang M, Ji B, Tian G, Guo Y, Tang M, Li L, Guo B and Ma T (2021) Application of Circulating Tumor DNA as a Biomarker for Non-Small Cell Lung Cancer. Front. Oncol. 11:725938. doi: 10.3389/fonc.2021.725938
Received: 16 June 2021; Accepted: 19 July 2021;
Published: 05 August 2021.
Edited by:
Jing Sun, George Washington University, United StatesReviewed by:
Min Chen, Hunan Institute of Technology, ChinaPengshuo Yang, Huazhong University of Science and Technology, China
Copyright © 2021 Yang, Hui, Zhang, Zhang, Ji, Tian, Guo, Tang, Li, Guo and Ma. This is an open-access article distributed under the terms of the Creative Commons Attribution License (CC BY). The use, distribution or reproduction in other forums is permitted, provided the original author(s) and the copyright owner(s) are credited and that the original publication in this journal is cited, in accordance with accepted academic practice. No use, distribution or reproduction is permitted which does not comply with these terms.
*Correspondence: Bella Guo, YmVsbGFndW8yMDA4QG91dGxvb2suY29t; Tonghui Ma, dG9uZ2h1aW1hQHllYWgubmV0; Lianxing Li, NTQ3NjM1MTc5QHFxLmNvbQ==
†These authors have contributed equally to this work