- 1Department of Biochemistry and Molecular Biology, School of Pre-Clinical Science, Guangxi Medical University, Nanning, China
- 2National Center for International Research of Bio-targeting Theranostics, Guangxi Key Laboratory of Bio-targeting Theranostics, Collaborative Innovation Center for Targeting Tumor Diagnosis and Therapy, Guangxi Medical University, Nanning, China
Memory T cells include T memory stem cells (TSCM) and central memory T cells (TCM). Compared with effector memory T cells (TEM) and effector T cells (TEFF), they have better durability and anti-tumor immunity. Recent studies have shown that although TSCM has excellent self-renewal ability and versatility, if it is often exposed to antigens and inflammatory signals, TSCM will behave as a variety of inhibitory receptors such as PD-1, TIM-3 and LAG-3 expression, and metabolic changes from oxidative phosphorylation to glycolysis. These changes can lead to the exhaustion of T cells. Cumulative evidence in animal experiments shows that it is the least differentiated cell in the memory T lymphocyte system and is a central participant in many physiological and pathological processes in humans. It has a good clinical application prospect, so it is more and more important to study the factors affecting the formation of TSCM. This article summarizes and prospects the phenotypic and functional characteristics of TSCM, the regulation mechanism of formation, and its application in treatment of clinical diseases.
Introduction
Immunotherapy has become one of the most promising strategies in cancer treatment, and has shown good efficacy in clinical trials (1). In particular, chimeric antigen receptor-engineered T cells (CAR-T) can specifically and effectively kill tumor cells, bringing new hopes for the treatment of patients with malignant tumors (2–7). However, whether it is traditional immune cell therapy or new CAR-T cells and T-cell receptor T cells (TCR-T) therapy, all are based on terminally differentiated effector T (TTE) cells, making it difficult to exert long-lasting anti-tumor effects in the body (8). Adoptive T cell therapy (ACT) is the in vitro expansion and reinfusion of tumor-reactive T cells, and is a potential treatment method for the treatment of advanced cancer (9–14). In infections and cancers, T lymphocytes expand and differentiate into effector cells and memory cells that clear pathogens. These cells can survive for a long time and ensure that they have a protective effect against pathogens when they are re-attacked by antigens (15). Human T lymphocytes are generally divided into naive T cell (TN), central memory T cell (TCM), effector memory T cell (TEM) and effector T cell (TEFF). In 2005, in the study of graft versus host disease (GVHD) in mice, a group of special memory T cell subsets with super proliferation and differentiation ability was observed for the first time. It produces persistent graft-versus-host disease, which the researchers named “stem like memory T cells” (TSCM) (16). Studies have shown that adoptively infused young T cells can self-renew and differentiate in mice, having the ability to survive for a long time, and exhibit significantly better anti-tumor capabilities than TTE cells. The progressive differentiation of T lymphocytes leads to a gradual loss of function and therapeutic potential. These studies suggest that poorly differentiated immune cells may have more application potential in clinical treatment (17–21).
TSCM cells have great potential in overcoming the limitations of current T cell-based immunotherapy (22–24). In mouse tumor models and human hematopoietic stem cell transplantation (HSCT) patients, TSCM cells have higher antitumor activity and survival rate. However, the proportion of TSCM cells in peripheral blood is low, which limits its application in immunotherapy. In this review, we summarize the latest findings, and discuss in depth the phenotype, function, differentiation mechanism and clinical application of memory T cells. It is hoped that using the therapeutic potential of TSCM cells for adoptive immunotherapy provides new ideas. The conceptual work and key discoveries that formed this field of investigation are shown in Figure 1 (25–38), which mainly summarizes the main discoveries in the process of TSCM cell research in recent years and the new research on the occurrence and development of diseases, some of which are introduced in articles.
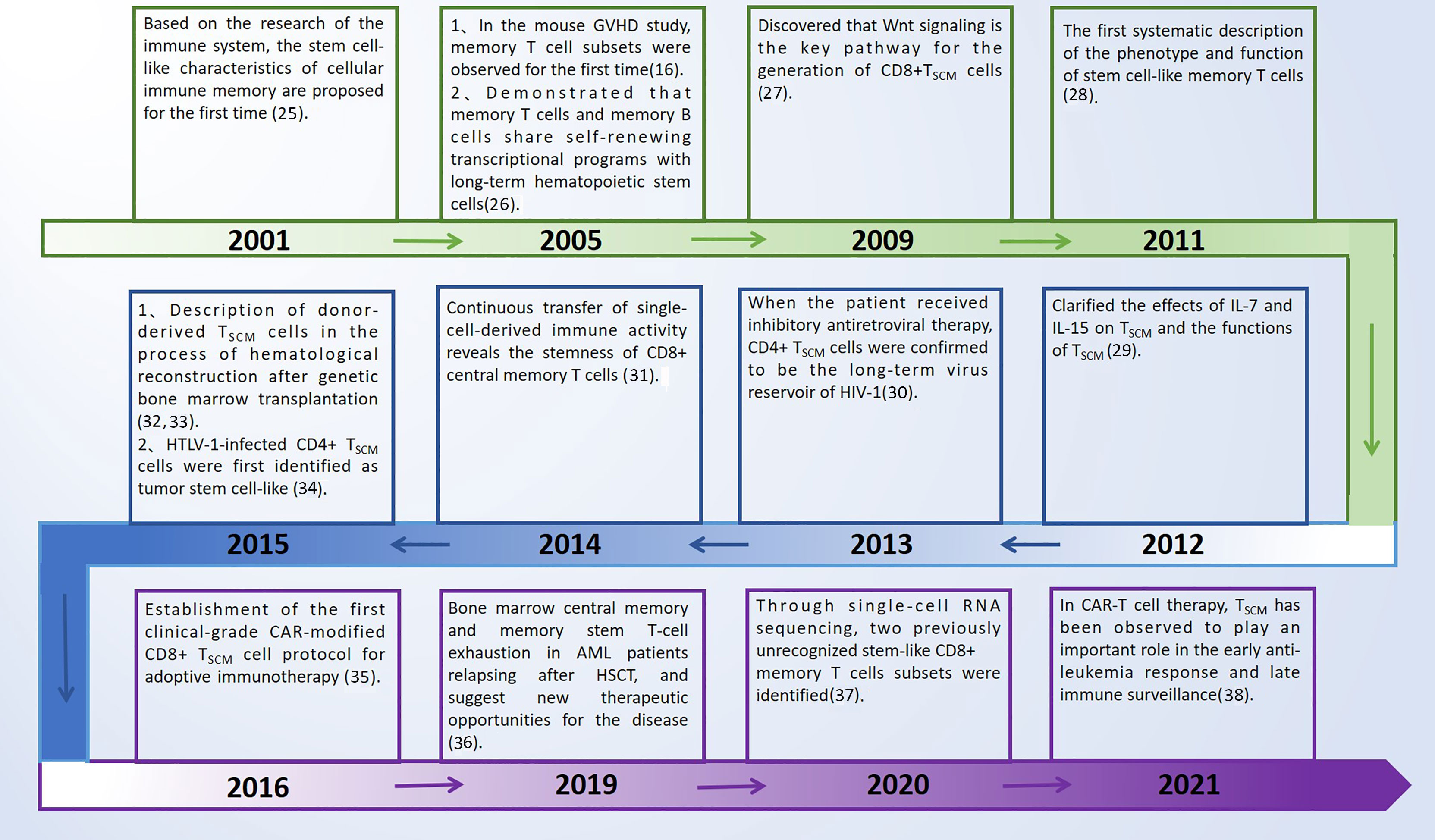
Figure 1 Key discoveries on TSCM cells. GVHD, graft versus host disease; TSCM cells, T memory stem cells; HTLV-1, human T cell lymphotropic virus type 1; HIV-1, human immunodeficiency virus type 1; CAR-T, chimeric antigen receptor-engineered T cells; AML, acute myeloid leukemia; HSCT, hematopoietic stem cell transplantation.
Phenotypic and Functional Characteristics of Memory T Cells
TSCM is a T cell subset with self-renewal ability and pluripotency potential. This group of memory T cells can play the role of acquired immune function in the process of the body’s fight against viruses or tumors (36, 39). T cell populations are classified by some surface markers, and distinguished according to their functions and sources, and the production of their effector cytokines. Memory T cells can be divided into TCM and TEM. TEM cells and TCM cells circulate in the blood and target the secondary lymphoid tissues. The degree of differentiation of TCM cells is lower than that of TEM and effector cells, and its telomeres are found to be longer, and the expression of perforin, granzyme and other effector molecules is lower (40). In addition, the TSCM pool should be limited to lymph nodes and secondary lymphoid organs, which are T cells that have antigen experience. The current research results also show that TCM has the function of T memory stem cells. TCM has stronger immune replacement ability and stronger survival ability in vivo than TEM cells. TSCM is developed from naive T cells in a resting state. It is a group of cells between TN and TCM. It also has the characteristics of TN cells and memory T cells (TM), and then differentiates into TCM and TEM. Good et al. (41) used single-cell mass cytometry to track the proliferation history of T cells. By analyzing the changes in phenotype and protein expression of T cells at different times and in different division states, it assisted in confirming the T cell differentiation theory: TN → TSCM → TCM → TEM. It is worth noting that only naive T cells and TSCM cells can reconstruct the heterogeneity of the entire memory T cell subset. At present, malignant tumors are one of the important diseases threatening human health, and there is no effective method to treat them. Due to their own characteristics, TSCM cells have shown their strong potential for tumor therapy.
According to the different expressions of cell surface chemokine receptor (CCR7) and lymph node homing molecules (CD62L), memory T cells are divided into TCM and TEM. TCM highly expresses CCR7 and CD62L, homing to secondary lymphoid organs, but low expression in TEM, which preferentially transports to peripheral tissues and mediates rapid effector functions. TSCM cells express naive cell phenotypes (CD45RA, CD62L, CCR7, CD95, CD27, CD122), and are characterized by rapid response to antigens, expression of a variety of effector molecules, and generation of memory effector cells. CD45RA+ is closely related to its memory ability. Naive cells express two molecules CD27 and CD45RA at the same time. Memory and effector cells only express CD27 or CD45RA respectively. TSCM cells highly express IL-2, IFN-γ, TNF-α, Bcl- 2. IL-7 and other molecules related to early differentiation of T cells, low expression of CD57 and other molecules related to T cell senescence, showing stronger degranulation ability and the ability to produce inflammatory cytokines. Recent studies have found that by detecting the expression of CD122 or CXCR3 in healthy people by flow cytometry, the TSCM CD122hi-expressing subset demonstrate greater proliferation, greater multipotency and enhanced polyfunctionality with higher frequencies of triple positive (TNF-α, IL-2, IFN-γ) cytokine-producing cells upon exposure to recall antigen. The cell proliferation and multifunctional cytokine production of the TSCM CXCR3lo population are also significantly increased (42). Loss of CXCR3 promotes stem-like memory precursor differentiation (43). According to these surface markers, TSCM cells can be accurately distinguished. TSCM cells represent a subset of minimally differentiated T cells, which are characterized by phenotypic and functional characteristics that connect naive and conventional memory cells.
The above mainly describes the surface markers of human T cell subsets. In addition to the specific T cell receptor (TCR), both human and murine TSCM express common markers of memory T cells (mouse CD62L, human CCR7, human CD45RO), and anti-apoptotic marker molecules (Bcl- 2), the cytokine receptor markers related to survival and proliferation CD122 (co-receptor of IL-2, IL-7 and IL-15) and CD127 (IL-7 receptor), stem cell marker (Sca-1). Human and murine T cell subsets are defined by different phenotypes (16, 27) (Table 1).
Development of TSCM Cells
Manipulation to Produce TSCM Cells in (Ex) Vivo
The relative scarcity of circulating TSCM cells limits their use in tumor therapy, which has led to manufacturing protocols that expand this cell type in vitro. As an important participant in the function of T cells, cytokines play an important role in the maintenance and expansion of TSCM subset. Recently reported related cytokines that can promote TSCM expansion are shown in Figure 2. A large number of studies have shown that adding different cytokines to the immune cell culture system can make it differentiate into memory or effector T cells (44–47). γc-cytokine IL-2, as a T cell growth factor, is still the most common cytokine used to expand therapeutic T cell products for patients (29, 48–50). γc-cytokine IL-2, as a T cell growth factor, is still the most common cytokine used to expand therapeutic T cell products for patients. However, high IL-2 levels reduced the overall production of early memory T cells by reducing central memory T cells and augmenting effectors. The number of early memory T cells in the T cell subset could be increased by simply reducing the amount of IL-2 (51). In the in vitro expansion process, repeated use of IL-2 to stimulate T cells would also cause T cell depletion and reduced T cell persistence (52). IL-7 could also promote the proliferation of TSCM cells by down-regulating the expression of programmed cell death protein 1 (PD-1) and Foxp3, and promoted the ability of CD4+ T cells to produce IFN-γ, IL-2, TNF-α and granzyme B. The involvement of STAT5 in IL-7-induced polyfunctionality, this the polyfunctional phenotype driven by IL-7 is associated with increased histone acetylation effector gene promoters and reveals previously unknown characteristics of IL-7 (53–56). The current study, CAR-T cells expanded in IL-15 (CAR-T/IL-15) preserved a less-differentiated TSCM phenotype, defined by expression of CD62L+CD45RA+CCR7+, as compared to cells cultured in IL-2 (CAR-T/IL-2). What’s more, CAR-T/IL-15 cells exhibited reduced expression of exhaustion markers, higher anti-apoptotic properties, and increased proliferative capacity when it was attacked by antigens (57). The combined use of IL-7 and IL-15 can preserve the TSCM phenotype and enhance the effectiveness of CAR-T cells (11, 29, 58–61). IL-21 was critical for the long-term maintenance and functionality of CD8+T cells and the control of chronic lymphocytic choriomeningitis virus (LCWV) infection in mice. In the process of chronic infection, cell-autonomous IL-21 receptor (IL-21R)–dependent signaling by CD8+ T cells was required for sustained cell proliferation and cytokine production (62, 63). IL-21 also can promote the generation of TSCM cells. It activates the Janus kinase signal transducer and activator of transcription 3 pathway by upregulating signal transducer and activator of transcription 3 phosphorylation and thereby promoting the expression of T-bet and suppressor of cytokine signaling 1, while decreasing the expression of eomesodermin (Eomes) and GATA binding protein 3 (64). In the absence of IL-10, IL-21 or STAT3, virus-specific CD8+ T cells (VSTs) maintain the terminal effect (TE) differentiation state and couldn’t mature into self-renewing TCM cells. The maturation of protective memory T cells and memory CD8+ T cell precursors was an active process that depended on the IL-10-IL-21-STAT3 signal (64, 65). Whether the formation of TSCM depends on this pathway still needs further research, but it provides new ideas for subsequent research. Lactate dehydrogenase (LDH) inhibition combined with IL-21 could increase the formation of TSCM cells, thereby producing more profound antitumor responses and prolonging the survival time of the host (66). In addition, a new study found that by fusion of IL-21 to anti-PD-1 antibody, IL-21 can target tumor-reactive T cells to promote TSCM production. PD-1Ab21 therapy has shown greater antitumor effects in established tumor-bearing mice (67). At present, a large number of experiments have confirmed that these cytokines can promote the production of TSCM and have potential antitumor effects. However, the mechanism of using cytokines, drugs and checkpoint blockade to promote the differentiation of memory T cells remains to be studied.
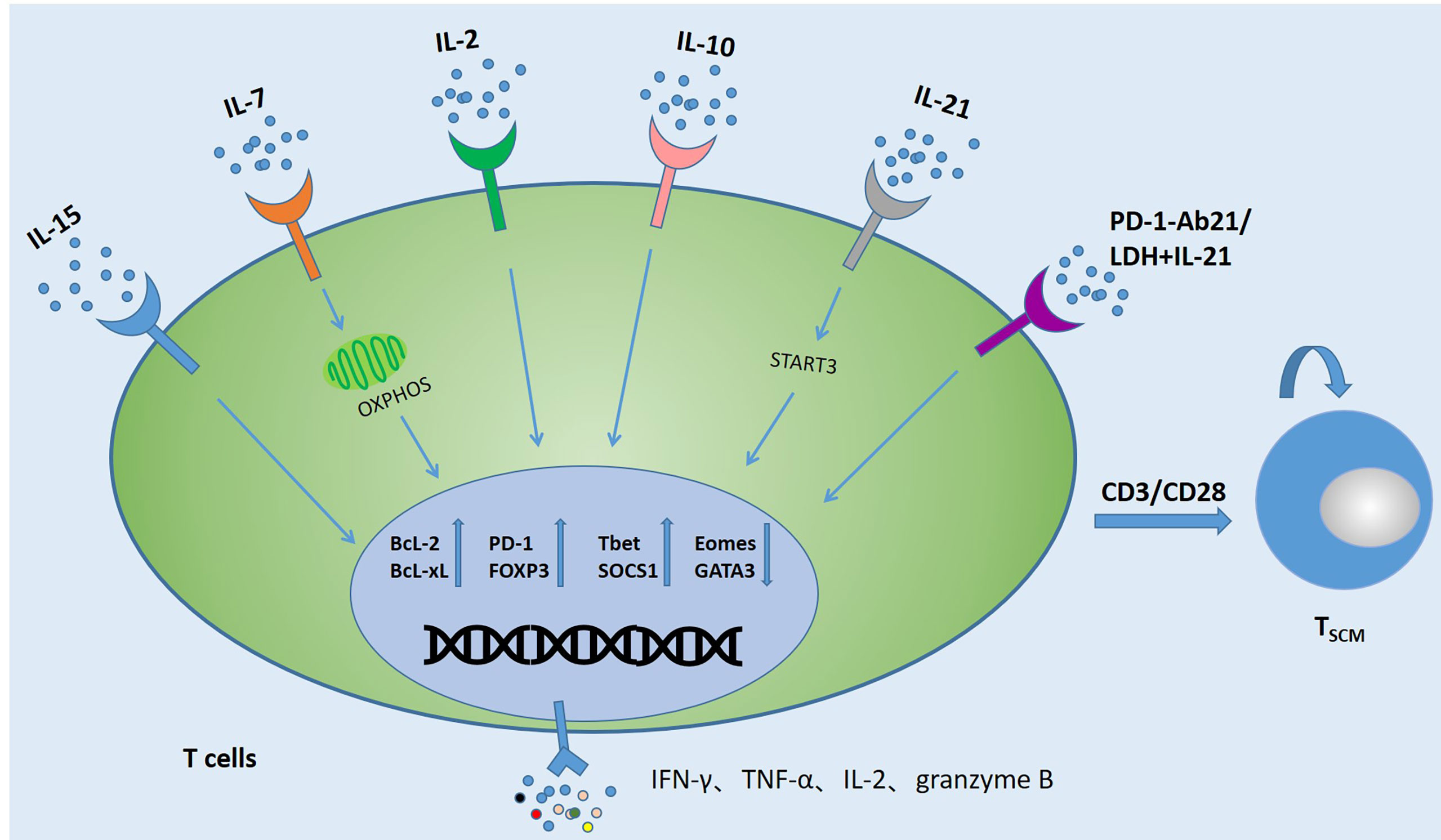
Figure 2 Several strategies to induce the generation of TSCM. Activating T cells (CAR-T cells, TCR-T cells, TILs, VSTs) with anti-CD3/CD28 antibodies and co-cultivating them with cytokines or combined with PD-1 and LDH can promote the production of TSCM cells and change the expression levels of related anti-apoptotic proteins and metabolic molecules. In addition, the expression of TNF-α, IFN-γ, IL-2 and Granzyme B also increased significantly.
Oxidative Metabolic Pathway of TSCM Cells
The naive T cells in the circulation are quiescent and have low metabolic requirements. They mainly use oxidative phosphorylation (OXPHOS) to produce ATP (53, 68). Generally speaking, differentiated T cells use glycolysis to proliferate, while memory T cells tend to use fatty acid oxidation (FAO)-dependent oxidative phosphorylation (OXPHOS) to produce ATP, which helps to perform long-lasting antitumor response in the tumor microenvironment (69–74). In the tumor microenvironment, tumor cells inhibit the metabolic reprogramming of T cells by competitively using glycolysis, so that the formation of memory T cells is inhibited (75, 76). It is reported that important transcription factors and cytokines, as well as MEKi and other inhibitors in the process of T cell differentiation, induce the generation of TSCM by regulating T cell-related metabolic enzymes (77–79) (Figure 3).
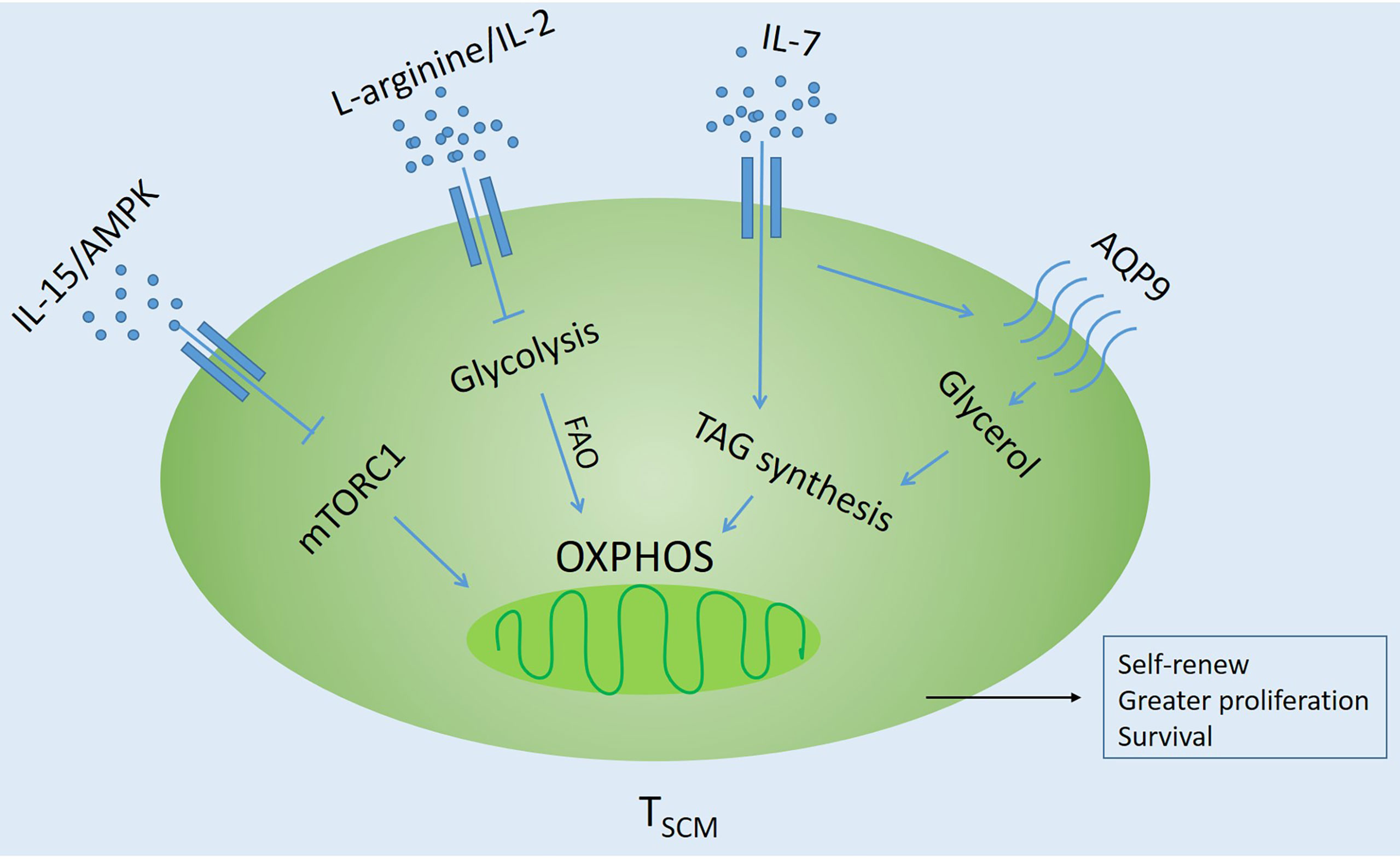
Figure 3 Influencing factors regulating oxidative metabolism of TSCM Cells. Inhibit glycolysis through different pathways and promote fatty acid oxidation (FAO)-dependent oxidative phosphorylation (OXPHOS). AQP9, Glycerol channel aquaporin 9; AMPK, AMP-activated protein kinase; TAG, triglyceride; mTORC1, Rapamycin Complex 1.
Signals from TCR, costimulatory molecules, and growth factors lead to the activation of signaling pathways that promote transcriptional programs that are critical to effector function (80–82). In memory T cells, cellular stress, such as growth factor deprivation or a low ratio of ATP/AMP, will activate AMP-activated protein kinase (AMPK) and inhibit mTOR signaling (83). IL-15 also showed a similar function (57, 83).
Good et al. (41, 84) have proved through a large number of experiments that blocking the mTOR pathway by adding inhibitors can allow T cells to differentiate towards TSCM-like cells, such as ITK (IL-2-inducible T-cell kinase), TWS119 and BTK (Bruton’s tyrosine kinase) inhibitors. In addition, the glycolytic function of TSCM cells is reduced, and different inhibitors promote the in vitro generation of TSCM-like cells with unique metabolic characteristics and retained polyfunctionality. It is worth noting that the drug-induced TSCM cells have superior functional characteristics and self-renewing capacity after adoptive transfer. The research compound Akt inhibitor VIII inhibits AKT in vitro, which can preserve the differentiation and function of minor histocompatibility antigen (MIHA)-specific CD8+ T cells. Moreover, transcriptome profiling revealed that AKT-inhibited CD8+T cells clustered closely to naturally occurring stem cell-memory CD8+ T cells. Moreover, AKT-inhibited MiHA-specific CD8+ T cells showed increased polyfunctionality with co-secretion of IFN-γ and IL-2 upon antigen recall (79). Glycerol channel aquaporin 9 (AQP9) deficiency could impair the entry of glycerol into memory CD8+ T cells for fatty acid esterification and triglyceride (TAG) synthesis and storage. While IL-7 could induce expression of the AQP9 in virus-specific memory CD8+ T cells, but not naive cells. AQP9 is essential for their long-term survival. TAG synthase could restore the survival of lipid storage and memory T cells through ectopic expression, and it was found that TAG synthase is the central component of IL-7-mediated survival of human and mouse memory CD8+ T cells (75). Three transcription factors, BAZ1B, PSIP1 and TSN, could regulate the level of L-arginine and promoted the survival of T cells. Activated T cells transform from glycolysis to oxidative phosphorylation, which promotes the production of TSCM with higher survival ability and has antitumor activity in mouse models (85). Recent new studies have found that TSCM induced by Meki/2 inhibition (Meki) has a natural phenotype, self-renewal ability, and enhanced pluripotency and proliferation. It is also achieved by regulating metabolism without affecting T cell receptor-mediated activation. DNA methylation analysis showed that Meki-induced TSCM cells exhibited plasticity and loci-specific profiles, similar to those of TSCM truly isolated from healthy donors, and had similar characteristics to naive and TCM cells. Meki treatment of tumor-bearing mice also showed strong immune-mediated antitumor effects (86). These studies indicate that the regulation of glycolysis and metabolism is the key factor in inducing the formation of TSCM. Therefore, targeted metabolic checkpoints can make T cells differentiate into memory and provide more young T cells for immunotherapy (74, 81, 82, 86).
The Molecules of Exhausted T Cells
T cell exhaustion is a phenomenon widely observed in humans. TSCM or CAR-modified TSCM expresses high levels of PD-1, TIM-3 or CTLA-4 after infiltrating the tumor, indicating that they have become exhausted T cells. Mostly due to T cell exhaustion and dysfunction by continuous TCR and cytokine stimulation. In addition, the effect of immune checkpoint inhibitors is very dependent on endogenous T cell function. However, they cannot reverse the exhaustion of T cells in cells that have undergone epigenetic changes. Therefore, this limits the long-term efficacy and wide application of cancer immunotherapy. Therefore, an in-depth understanding of the mechanism of T cell exhaustion is necessary for the study of TSCM and its better clinical application. The term “ exhausted T cells” was originally derived from a mouse model of LCMV. It is now widely used to define the dysfunction state of T cells under chronic infection or tumor-induced long-term high antigen load stimulation (87). Enhanced and sustained T cell receptor stimulation is a key driver of T cell exhaustion. In recent years, the definition and identification of exhausted T cells have been divided from phenotype to transcriptional and epigenetic levels (88–90). Exhausted T cells are characterized by increased expression levels of inhibitory receptors such as PD-1, LAG3, 2B4, TIM-3 and CD28, and the gradual loss of effector functions, including impaired ability to secrete IFN-γ and tumor necrosis factor (91–95). PD-1 is mainly expressed on the surface of activated T cells and can inhibit T cell activation and proliferation. It is an important immunosuppressive molecule that plays an important role in suppressing immune responses and promoting self-tolerance (96–98). Programmed cell death ligand 1 (PD-L1) is a transmembrane protein, which is mainly expressed on the surface of antigen-presenting cells (APCs) such as dendritic cells (DCs), and can also be expressed on the surface of cancer cells and tumor infiltrating lymphocytes (TIL) (99–102). TOX is a nuclear DNA binding protein. TOX plays an important role in the development of thymus CD4+ T cells, NK cells and intrinsic lymphocytes, and is critical in the differentiation of tumor-specific T cells. Recent studies have described the important role of TOX in the differentiation of exhaustive CD8+ T cells and its molecular mechanism. It is unanimously found that the high expression of TOX is related to the high expression of a variety of inhibitory receptors (PD-1, TIM-3, TIGIT, CTLA-4, etc.) and the low expression of TCF1 (103). So inhibiting TOX expression may hinder the exhaustion of T cells (104–109). Many laboratories have identified a kind of exhausted T cell precursors (TPEX), which highly express molecules related to memory T cells, such as TCF1. TCF1 is a transcription factor and histone deacetylase (HDAC), which is related to the formation of T cell memory. Through single-cell RNA sequencing (scRNA-seq) and lineage tracing, the TCF1+Ly108+PD-1+CD8+ T cell population was identified. It was found that PD-1 stabilized the TCF1+TeX precursor cell pool and confirmed that PD-1 was this early stage protector of the TCF1 population (91, 110). Exhaustion first appeared in TCF1+ precursor T cells and then spread to the antigen-specific T cell pool. These findings will be important in the future to further investigate the developmental relationships in the later stages of exhaustion (111, 112).
At present, the specific mechanism of T cell exhaustion has not been fully elucidated. T cell exhaustion may be a parallel process with T cell differentiation. T cells at any stage of differentiation can be induced into exhausted T cells, which involves changes in different phenotypes and molecules. Excessive stimulation of precursor cells may be the origin of T cell failure. Under chronic infection or long-term tumor antigen stimulation, memory T cells and exhausted T cell precursors show different differentiation characteristics. Whether there is a link between the differentiation between these two subgroups should be a priority research area in the future. The possible potential developmental trajectories of exhausted T cells are shown in Figure 4.
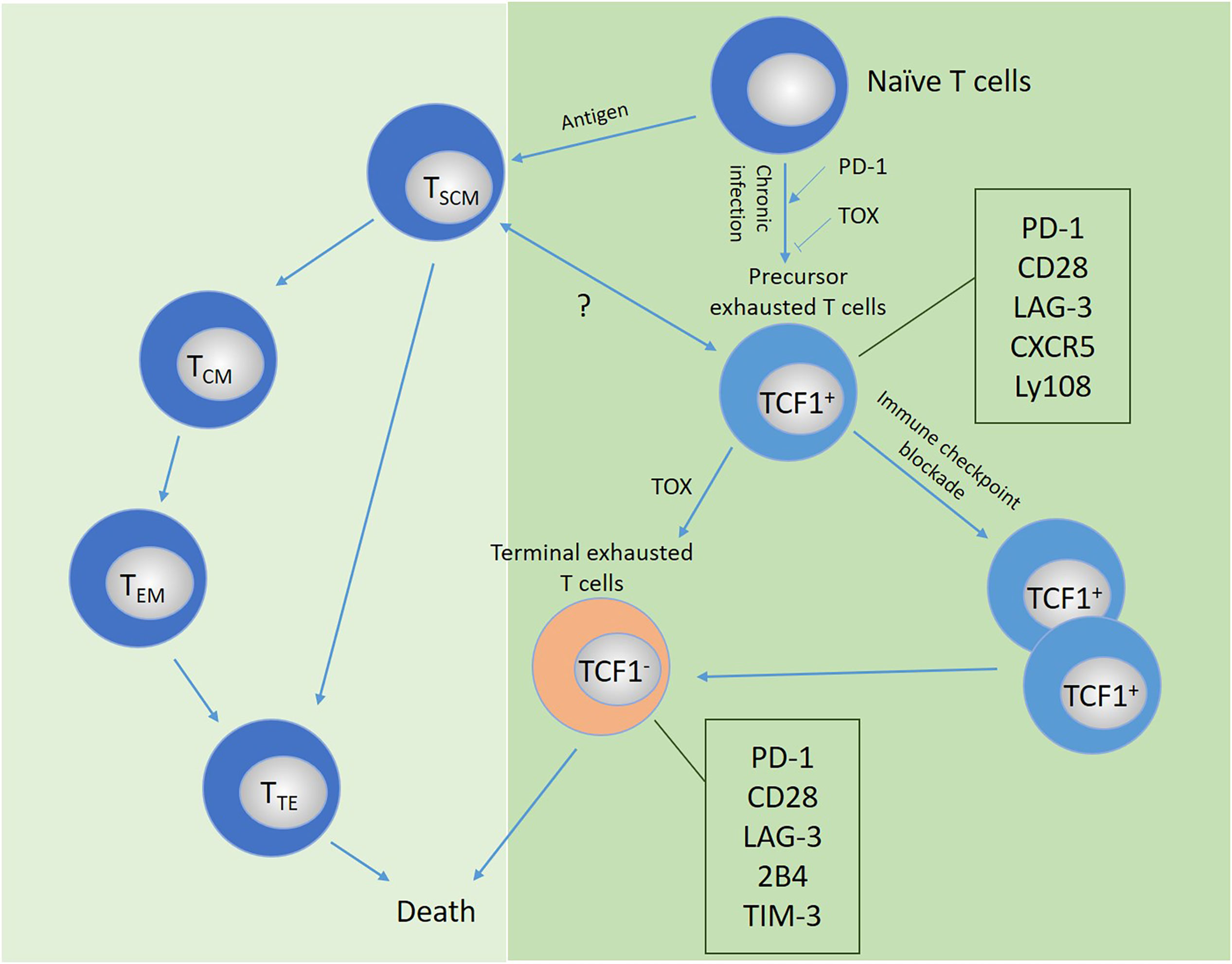
Figure 4 Possible developmental trajectory of exhausted T cells and the comparison and relationship with memory or effector T cells. Under continuous antigen stimulation, T cells transform from precursor exhausted cells into terminally exhausted T cell populations, which mainly depends on the expression of the transcription factor TCF-1, accompanied by the high expression of a variety of inhibitory receptors. The relationship between the differentiation of T cell subsets and exhausted T cells remains to be explored. PD-1, PD ligand 1; TCF1, T cell factor-1; TIM-3, T-cell immunoglobulin domain and mucin domain protein 3; LAG-3, lymphocyte activation gene 3; TOX, thymocyte selection-associated high-motility group (HMG) box protein.
At present, drugs for T cell exhaustion are still in the laboratory research or clinical trial stage. By reducing T cell exhaustion to promote the self-renewal ability and polyfunctionality of TSCM cells (Table 2). Therefore, we do not know how to regulate the exhaustion process of T cells and reverse the exhausted state. Is it feasible to reach a certain effector state, and will there be side effects? Whether TSCM can be designed to be exhaustion resistant? In general, the molecular mechanism of TSCM cell formation is very complicated, and we describe them as clearly as possible in the review. More and more evidence supports the therapeutic potential of targeting exhausted T cells (115–118). We have already begun to understand the molecular mechanism of T cell exhaustion and early memory formation. Transforming exhausted T cells into rejuvenated TSCM cells is the goal of our research.
Clinical Application
The Antitumor Effect of TSCM
TSCM cells are the least differentiated cells located at the top of the memory T lymphocyte hierarchy system. Compared with other T cells, they have stronger self-renewal ability and anti-tumor ability (84, 119, 120). As early as in previous studies, it has been found that TSCM is considered a key determinant of immune memory and is involved in diversification of immune memory after allogeneic HSCT (32, 33). Play an important role in adult T-cell leukemia (34). With the FDA approval of CAR-T cell therapy for hematological malignancies, ACT has become a hot spot of continuous attention (63, 121–128). The clinical application of TSCM cells is hindered because they are relatively rare in the circulation. According to reports, the CAR-T cell-modified TSCM was cocultured with IL-2, IL-7 or IL-15 and then injected intravenously into tumor-bearing mice. It was found that the CAR-T/IL-15 group have the best anti-tumor effect (57). Guan et al. (129) prepared allogeneic antigen-specific CD8+ TSCM. It showed a proliferation history and rapidly differentiated into effector cells upon the E007 [the EB virus (EBV) transformed B lymphoblastoid cell lines (LCLs)] re-stimulation. Importantly, the prepared TSCM cells could survive for a long time and reconstituted other T cell subsets in vivo, and could effectively eliminate E007 cells after being transferred to LCL burden mice. KUN et al. (120) presented a novel tumor therapeutic modality of the cryo-thermal therapy. After 90 days of cryo-thermal therapy, it can enhance the cytolytic function of CD8+ T cells, induce CD8+ T cells to differentiate into TSCM, and CD4+ T cells to differentiate into dominant CD4- CTL, Th1 and TFH subets. Cryo-thermal therapy not only inhibits lung metastasis, but also promotes the regression of implanted melanoma and prolongs survival time (35, 130, 131). It was found that after antigen chimeric modification of TSCM, CD19-specific CAR T cell adoptive transfer has a significant antitumor effect on leukemia and lymphoma, and the therapeutic potential seems to be related to persistence in vivo (128, 129, 132, 133).
In SIP (an ex-vivo culture system modeled after the temporal changes of essential cytokines in an acute infection), TIL in the bone marrow of patients diagnosed with acute myeloid leukemia (AML) was treated with similar SIP, and it was found that these lymphocytes can be re-transformed into mutant CD45RA+ central memory T lymphocytes (TCMRA) with similar characteristics of TSCM. The expression of pro-inflammatory cytokines, TNF-α, IFN-γ and IL-2 increased, and TCMRA also exhibited cytotoxicity against autologous AML blast cells (134). In addition, similar effects have been shown in the treatment of Hodgkin’s lymphoma. It showed a survival advantage, had higher tumor invasion and enhanced antitumor effect (133). Tumor immunotherapy is a promising treatment method. Transfect antigen-specific TCR gene or CAR vector to TSCM to obtain CAR-T cells with poor differentiation and greater proliferation ability (135–139). A clinical trial study found that the genetically modified TSCM can survive in the body for up to 12 years and has good safety and function (140). Recent studies have found that through integration site analysis, it is possible to study the fate of different types of CAR-T cells in patients, and it has been observed that TSCM plays a central role in the early anti-leukemia response and late immune surveillance (38). This shows that this small portion of T cells is critical to the long-term success of CAR-T cell therapy. This new insight may help us improve CAR-T cell therapy and find out which patients are at higher risk of recurrence, and may benefit from stem cell transplantation after CAR-T cell therapy.
To date, CAR-T cells have achieved remarkable results in the treatment of hematological malignancies. However, despite extensive research, CAR-T cells have not been so successful in the treatment of solid tumors (141). Therefore, how to increase the trafficking and extravasation of T cells to the tumor sites and encourage the proliferation of T cells in the tumor is a problem that needs to be solved urgently. TSCM have been shown to eradicate large tumors even when limited numbers of cells were transferred (28). Studies have found that chimeric T cells with multiple antigens may be a new direction for the treatment of solid tumors (71, 141, 142). At present, there are relatively few reports on the treatment of solid tumors with CAR-T-modified TSCM, so it is more challenging for CAR-T-modified TSCM to target solid tumors. The future should be a priority research area. In summary, memory T cell subsets have good clinical application prospects in clinical antitumor immunotherapy, and can provide personalized treatment plans for improving the prognosis of patients (134, 143). In short, these studies provide a strong scientific basis and practical methods for the rapid advancement of TSCM cells in clinical trials of human adoptive immunotherapy.
The Importance of TSCM in HIV-1 Immunotherapy and Vaccine Research
TSCM cells play a key role in the pathogenesis of human immunodeficiency virus (HIV) infection (30, 144–146). The exhaustion of these cells will lead to the deterioration of the immune system and the development of AIDS. HIV-1 is an important part of the virus reservoirs. During HIV-1 infection, CD4+ TSCM cells are confirmed to be the longest-lived HIV-1. Virus storage is one of the factors that cause persistent HIV-1 infection (147, 148). Therefore, CD4+ TSCM cells can be used as a new target to clear the HIV-1 virus reservoir. The virus-latent cells are mainly concentrated in CD4+ TSCM. CD4+ TSCM expresses lower levels of CCR5, but can still support the production and latent infection of R5-tropic HIV-1 (149, 150). In addition, CD4+ TSCM is highly permissible for VSV-G-HIV-1 virus infection in vitro, and expresses relatively low levels of intracellular viral restriction factors, such as SAMHD1, Trim5alpha, and APOBEC3G. Moreover, these restriction factors can prevent HIV-1 from replicating in myeloid and dendritic cells (151–153). It was found that the CD4+ TSCM of untreated HIV-1 infected persons contained high levels of HIV-1 RNA, which all indicated the sensitivity of CD4+ TSCM cells to HIV-1. The study also found that in patients undergoing antiretroviral therapy (ART), CD4+ TSCM cells also have viral DNA that can be activated. Moreover, among the subsets of CD4+ T memory cells, the number of HIV-1 DNA in TSCM cells is the highest. During HIV infection, T cells play an important role in controlling virus replication. In patients receiving inhibitory antiretroviral therapy, CD8+ TSCM with stem cell characteristics was found to be more abundant than untreated patients (154). In addition, prolonging the treatment time can increase the ratio of CD8+ TSCM, and preferentially secrete IL-2 under viral stimulation, indicating that CD8+ TSCM is an important part of the cellular immune response to HIV-1. Able to maintain long-term, non-antigen-dependent cellular immune memory for HIV-1, which plays a key role in HIV control, but it seems unable to survive and proliferate during untreated infections (149). It is worth noting that HIV-1 specific CD8+ TSCM cells may not directly participate in the antiviral process, but play a role by secreting IL-2 to maintain their own proliferation and differentiation (155–157). Recent studies have found that vaccination of the subtype C prophylactic HIV-1 vaccine candidate can induce more TSCM and antiviral. Compared with MVA alone and placebo, it induces more peripheral CD8+ TSCM cells and a higher level of CD8+ T cell-mediated inhibition of the replication of different HIV-1 branches can respond to acute HIV infection or effectively control the chronic replication of HIV (152). Recently, a cross-sectional study of 20 cases of HIV-infected patients on treatment alone and 20 cases of ART has revealed a new subset of CD4+ T cells: follicular regulatory T cells (TFR). The TFR of HIV+ patients had anti-apoptotic properties, high proliferation rate and TSCM-like properties, which leaded to the expansion of TFR, which in turn leaded to the dysfunction of TFH. Therefore, TFR cells may also become a new and potential therapeutic target for the treatment of HIV infection (158). How to target TSCM therapy to provide new ideas for the development of new strategies for HIV-1 vaccines and immunotherapy still needs to continue to be explored and studied.
TSCM and Autoimmune Diseases
TSCM cells provide long-term protective immunity for anti-tumor immunity, which is probably based on reactivity to self-antigens. Therefore, as a by-product of antitumor, TSCM-mediated autoimmunity is inevitable (18, 159). Recent related studies have reported that TSCM cells are associated with a variety of autoimmune diseases. Systemic lupus erythematosus (SLE) is a chronic connective tissue disease involving multiple organs that occurs in young women. Compared with healthy controls, the percentage of CD4+ and CD8+ TSCM cells in SLE patients increased significantly. Differentiated TFH cells increase the antibodies produced by their own B cells. TSCM cells play a role in the pathogenesis of SLE by maintaining TFH cells (132). Moreover, compared with healthy controls, the CD4+ TSCM of rheumatoid arthritis (RA) patients increased significantly (160). In the presence of IL-6, TCRs are easily activated to produce inflammatory cytokines. TSCM cells may be a continuous source of the pathogenicity of RA (161). In patients with immune thrombocytopenia (ITP), the ratio of CD4+ and CD8+ T cells in the peripheral blood is unbalanced. The percentage of CD8+ TSCM in peripheral blood of ITP patients was significantly reduced after glucocorticoid treatment, indicating that the imbalance of the ratio of CD8+ TSCM may be involved in the occurrence and development of ITP (162). In addition, the frequency of acquired aplastic anemia (AA) CD8+ TSCM after immunosuppressive treatment was significantly higher than that of healthy controls. The frequency of CD8+ TSCM is also elevated in patients with autoimmune uveitis or sickle cell disease (130). B-cell-specific CD8+ TSCM cells with high expression of glucose transporter 1 (GLUT1) can be detected in T1D patients. WZB117, a selective inhibitor of Gult-1, effectively inhibits TSCM cells in type 1 diabetes (T1D) patients by inhibiting glucose metabolism (53). Long-term autoreactive or abnormally activated TSCM cells may induce self-renewing inflammatory cell responses. Studies have found that rapamycin (mTORC1 inhibitor) is outstanding in the treatment of autoimmune diseases (163). The above studies indicate that TSCM may be a potential therapeutic target for these autoimmune diseases. The possible role of TSCM cells in other diseases with severe cellular immune response, such as autoimmune hepatitis, thyroiditis, and certain types of glomerulonephritis, is currently unclear, but represents a priority research area in the future.
Conclusion
TSCM is a long-lived memory cell with self-renewal ability and multi-differentiation potential. Different subsets of memory T cells can be identified based on their surface markers, gene expression profiles, and metabolic methods. At the same time, clinical-grade memory T cells can be obtained through in vitro induction and culture for cell transfer. The formation of memory T cells in the body has been confirmed in pre-clinical trials. The genetically modified TSCM can survive in the body for up to 12 years and has good safety and function (140, 164). Convincing evidence in mice and humans shows that TSCM cells are an important tool for adoptive immunity in tumor immunotherapy (143, 162). On the contrary, it is precisely because of their powerful immune reconstruction ability that they play a double-edged role in human diseases, and they are also potential therapeutic targets for autoimmune diseases and HIV (Figure 5). However, there are still many problems that need to be solved, elucidating the molecular mechanism of maintaining the phenotype of TSCM cells and the influence of epigenetic modification, how to obtain a sufficient number of clinical grade TSCM for induction culture. The infused TSCM cells are easily affected by the immune microenvironment and are difficult to exert antitumor effects, and how the TSCM cells target the tumor site to kill tumor cells is a problem worthy of attention at present. CAR-modified TSCM cells, although there is good preclinical evidence that they have anti-tumor activity, when they are intravenously infused into solid tumor patients, they still lack persistence and efficacy (71, 133, 142). At the same time, it is worth noting that a single treatment method cannot effectively eliminate tumor cells. Immune cell therapy should be combined with PD-1 monoclonal antibody, CTLA-4 monoclonal antibody or radiotherapy, chemotherapy and other treatment methods, so that patients can get better efficacy (165). TSCM has long existed in the HIV-1 virus reservoir, so future research is necessary to determine whether the low virus accumulation in TSCM cells represents a significant feature of HIV-1 infection. More effort is needed to clarify the changes between the different states of TSCM cells in health and disease. Although significant progress has been made in tumor therapy, there is still a gap in our understanding of the role of TSCM cells in autoimmunity and viral infections.
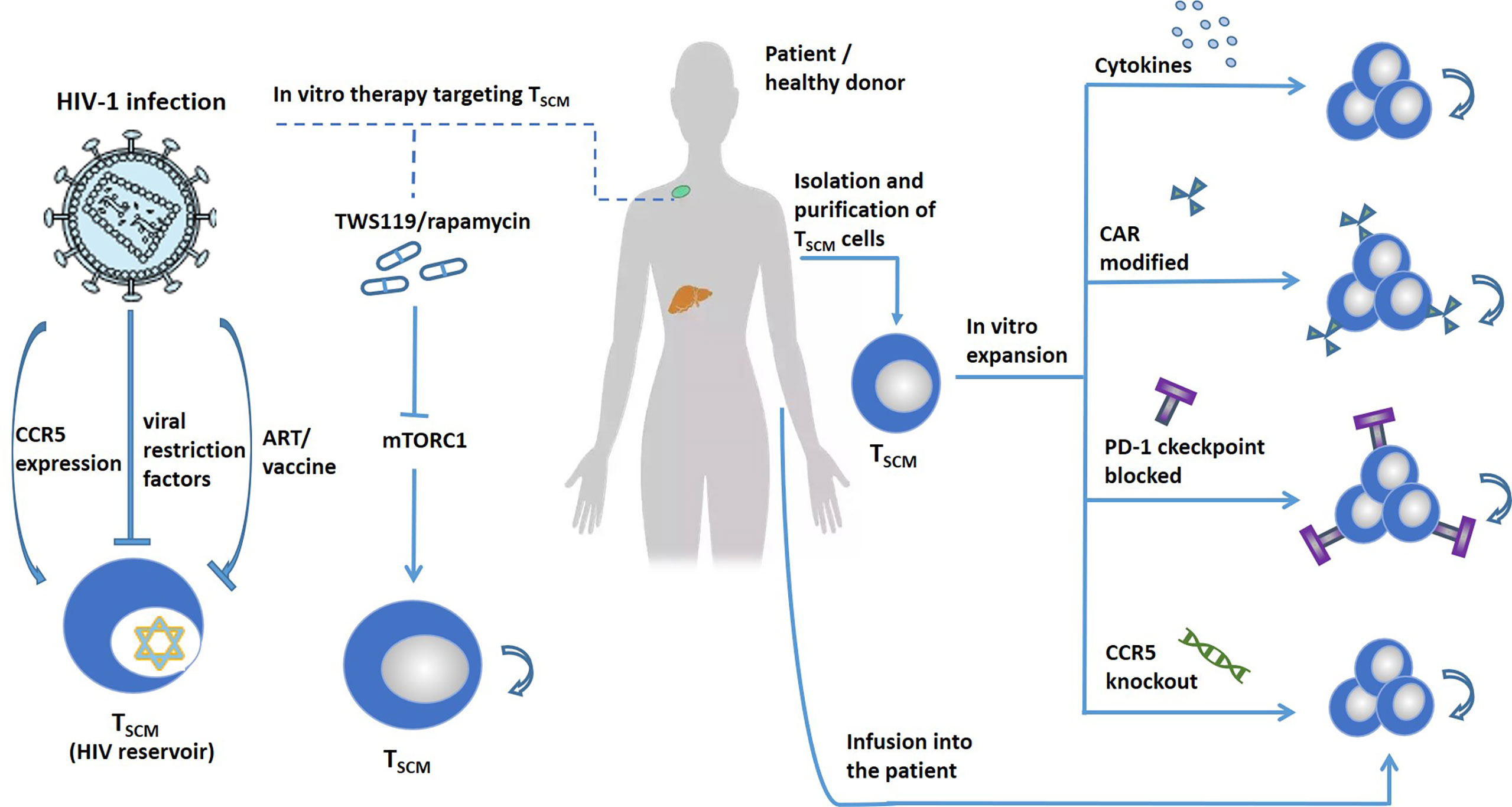
Figure 5 Target TSCM cells to treat human diseases. TSCM cells can exacerbate human disease. Left, treat TSCM-driven diseases, such as autoimmune diseases, HIV, etc., by blocking the production of TSCM. The expression of CCR5 promotes the infection of TSCM cells with HIV. Viral restriction factors and vaccines can target TSCM cells to treat HIV. Blocking the mTORC1 pathway promotes the self-renewal and differentiation of TSCM. Right, TSCM cells are expanded in vitro by adding cytokines, CAR modification, immune checkpoint blocking, and gene editing. Stars represent cells latently infected with HIV.
Author Contributions
YL designed the study and wrote the manuscript. DW and XY collected the literature. All authors contributed to the article and approved the submitted version.
Funding
This work was funded by the Guangxi Science and Technology Research Base and Talent-specific Project (AD18126021); National Science and Technology Major Project for New Drug Innovation (2018ZX09733001-004-002); National Natural Science Foundation of China (NSFC) project (No. 81872491); Key Laboratory of the Ministry of Education Project for Early Prevention and Treatment of Regional High-risk Tumors (GKE2018-03, GKE2019-09, GKE-ZZ202007).
Conflict of Interest
The authors declare that the research was conducted in the absence of any commercial or financial relationships that could be construed as a potential conflict of interest.
Publisher’s Note
All claims expressed in this article are solely those of the authors and do not necessarily represent those of their affiliated organizations, or those of the publisher, the editors and the reviewers. Any product that may be evaluated in this article, or claim that may be made by its manufacturer, is not guaranteed or endorsed by the publisher.
References
1. Miller JD, van der Most RG, Akondy RS, Glidewell JT, Albott S, Masopust D, et al. Human Effector and Memory CD8+ T Cell Responses to Smallpox and Yellow Fever Vaccines. Immunity (2008) 28(5):710–22. doi: 10.1016/j.immuni.2008.02.020
2. Figueroa JA, Reidy A, Mirandola L, Trotter K, Suvorava N, Figueroa A, et al. Chimeric Antigen Receptor Engineering: A Right Step in the Evolution of Adoptive Cellular Immunotherapy. Int Rev Immunol (2015) 34(2):154–87. doi: 10.3109/08830185.2015.1018419
3. Khouri R, Silva-Santos G, Dierckx T, Menezes SM, Decanine D, Theys K, et al. A Genetic IFN/STAT1/FAS Axis Determines CD4 T Stem Cell Memory Levels and Apoptosis in Healthy Controls and Adult T-Cell Leukemia Patients. Oncoimmunology (2018) 7(5):e1426423. doi: 10.1080/2162402X.2018.1426423
4. Li X, Chen W. Mechanisms of Failure of Chimeric Antigen Receptor T-Cell Therapy. Curr Opin Hematol (2019) 26(6):427–33. doi: 10.1097/MOH.0000000000000548
5. Martinez M, Moon EK. CAR T Cells for Solid Tumors: New Strategies for Finding, Infiltrating, and Surviving in the Tumor Microenvironment. Front Immunol (2019) 10:128. doi: 10.3389/fimmu.2019.00128
6. Zhao J, Song Y, Liu D. Clinical Trials of Dual-Target CAR T Cells, Donor-Derived CAR T Cells, and Universal CAR T Cells for Acute Lymphoid Leukemia. J Hematol Oncol (2019) 12(1):17. doi: 10.1186/s13045-019-0705-x
7. Xu L, Yao D, Tan J, He Z, Yu Z, Chen J, et al. Memory T Cells Skew Toward Terminal Differentiation in the CD8+ T Cell Population in Patients With Acute Myeloid Leukemia. J Hematol Oncol (2018) 11(1):93. doi: 10.1186/s13045-018-0636-y
8. Thommen DS, Schumacher TN. T Cell Dysfunction in Cancer. Cancer Cell (2018) 33(4):547–62. doi: 10.1016/j.ccell.2018.03.012
9. Sheng SY, Gu Y, Chuan Gang L, Tang YY, Yong Zou J, Qing Zhang Y, et al. The Characteristics of Naive-Like T Cells in Tumor-Infiltrating Lymphocytes From Human Lung Cancer. J Immunother (2017) 40(1):1–10. doi: 10.1097/CJI.0000000000000147
10. Zheng C, Zheng L, Yoo JK, Guo H, Zhang Y, Guo X, et al. Landscape of Infiltrating T Cells in Liver Cancer Revealed by Single-Cell Sequencing. Cell (2017) 169(7):1342–56.e1316. doi: 10.1016/j.cell.2017.05.035
11. Arcangeli S, Falcone L, Camisa B, De Girardi F, Biondi M, Giglio F, et al. Next-Generation Manufacturing Protocols Enriching TSCM CAR T Cells Can Overcome Disease-Specific T Cell Defects in Cancer Patients. Front Immunol (2020) 11:1217. doi: 10.3389/fimmu.2020.01217
12. Krishna S, Lowery FJ, Copeland AR, Bahadiroglu E, Mukherjee R, Jia L, et al. Stem-Like CD8 T Cells Mediate Response of Adoptive Cell Immunotherapy Against Human Cancer. Science (2020) 370(6522):1328–34. doi: 10.1126/science.abb9847
13. Vahidi Y, Bagheri M, Ghaderi A, Faghih Z. CD8-Positive Memory T Cells in Tumor-Draining Lymph Nodes of Patients With Breast Cancer. BMC Cancer (2020) 20(1):257. doi: 10.1186/s12885-020-6714-x
14. Wu TD, Madireddi S, de Almeida PE, Banchereau R, Chen YJ, Chitre AS, et al. Peripheral T Cell Expansion Predicts Tumour Infiltration and Clinical Response. Nature (2020) 579(7798):274–8. doi: 10.1038/s41586-020-2056-8
15. Liu Q, Sun Z, Chen L. Memory T Cells: Strategies for Optimizing Tumor Immunotherapy. Protein Cell (2020) 11(8):549–64. doi: 10.1007/s13238-020-00707-9
16. Zhang Y, Joe G, Hexner E, Zhu J, Emerson SG. Host-Reactive CD8+ Memory Stem Cells in Graft-Versus-Host Disease. Nat Med (2005) 11(12):1299–305. doi: 10.1038/nm1326
17. Qian C, W Y, Cai H, Laroye C, De Carvalho Bittencourt M, Clement L, et al. Adenovirus-Specific T-Cell Subsets in Human Peripheral Blood and After IFN-γ Immunomagnetic Selection. J Immunother (Qian 2016) 39(1):27–35. doi: 10.1097/CJI.0000000000000105
18. Gao S, Liang X, Wang H, Bao B, Zhang K, Zhu Y, et al. Stem Cell-Like Memory T Cells: A Perspective From the Dark Side. Cell Immunol (2021) 361:104273. doi: 10.1016/j.cellimm.2020.104273
19. Xu L, Zhang Y, Luo G, Li Y. The Roles of Stem Cell Memory T Cells in Hematological Malignancies. J Hematol Oncol (2015) 8:113. doi: 10.1186/s13045-015-0214-5
20. Lugli E, Gattinoni L, Roberto A, Mavilio D, Price DA, Restifo NP, et al. Identification, Isolation and In Vitro Expansion of Human and Nonhuman Primate T Stem Cell Memory Cells. Nat Protoc (2013) 8(1):33–42. doi: 10.1038/nprot.2012.143
21. Ando M, Ito M, Srirat T, Kondo T, Yoshimura A. Memory T Cell, Exhaustion, and Tumor Immunity. Immunol Med (2020) 43(1):1–9. doi: 10.1080/25785826.2019.1698261
22. Morrot A. Human Stem Memory T Cells (TSCM) as Critical Players in the Long-Term Persistence of Immune Responses. Ann Transl Med (2017) 5(5):120. doi: 10.21037/atm.2017.02.28
23. Guo X, Zhang Y, Zheng L, Zheng C, Song J, Zhang Q, et al. Global Characterization of T Cells in Non-Small-Cell Lung Cancer by Single-Cell Sequencing. Nat Med (2018) 24(7):978–85. doi: 10.1038/s41591-018-0045-3
24. Chebloune Y, Moussa M, Arrode-Brusés G, Ronfort C, Bose D, Gagnon J, et al. A Single Lentivector DNA Based Immunization Contains a Late Heterologous SIVmac251 Mucosal Challenge Infection. Vaccine (2020) 38(21):3729–39. doi: 10.1016/j.vaccine.2020.03.053
25. Fearon DT, Manders P, Wagner SD. Arrested Differentiation, The Self-Renewing Memory Lymphocyte, and Vaccination. Science (2001) 293(5528):248–50. doi: 10.1126/science.1062589
26. Luckey CJ, B D, Goldrath AW, Weissman IL, Benoist C, Mathis D. Memory T and Memory B Cells Share a Transcriptional Program of Self-Renewal With Long-Term Hematopoietic Stem Cells. Proc Natl Acad Sci USA (2006) 103(9):3304–9. doi: 10.1073/pnas.0511137103
27. Gattinoni L, Zhong XS, Palmer DC, Ji Y, Hinrichs CS, Yu Z, et al. Wnt Signaling Arrests Effector T Cell Differentiation and Generates CD8+ Memory Stem Cells. Nat Med (2009) 15(7):808–13. doi: 10.1038/nm.1982
28. Gattinoni L, Lugli E, Ji Y, Pos Z, Paulos CM, Quigley MF, et al. A Human Memory T Cell Subset With Stem Cell-Like Properties. Nat Med (2011) 17(10):1290–7. doi: 10.1038/nm.2446
29. Cieri N, Camisa B, Cocchiarella F, Forcato M, Oliveira G, Provasi E, et al. IL-7 and IL-15 Instruct the Generation of Human Memory Stem T Cells From Naive Precursors. Blood (2013) 121(4):573–84. doi: 10.1182/blood-2012-05-431718
30. Buzon MJ, Sun H, Li C, Shaw A, Seiss K, Ouyang Z, et al. HIV-1 Persistence in CD4+ T Cells With Stem Cell-Like Properties. Nat Med (2014) 20(2):139–42. doi: 10.1038/nm.3445
31. Graef P, Buchholz VR, Stemberger C, Flossdorf M, Henkel L, Schiemann M, et al. Serial Transfer of Single-Cell-Derived Immunocompetence Reveals Stemness of CD8(+) Central Memory T Cells. Immunity (2014) 41(1):116–26. doi: 10.1016/j.immuni.2014.05.018
32. Roberto A, Castagna L, Zanon V, Bramanti S, Crocchiolo R, McLaren JE, et al. Role of Naive-Derived T Memory Stem Cells in T-Cell Reconstitution Following Allogeneic Transplantation. Blood (2015) 125(18):2855–64. doi: 10.1182/blood-2014-11-608406
33. Cieri N, Oliveira G, Greco R, Forcato M, Taccioli C, Cianciotti B, et al. Generation of Human Memory Stem T Cells After Haploidentical T-Replete Hematopoietic Stem Cell Transplantation. Blood (2015) 125(18):2865–74. doi: 10.1182/blood-2014-11-608539
34. Nagai Y, Kawahara M, Hishizawa M, Shimazu Y, Sugino N, Fujii S, et al. T Memory Stem Cells are the Hierarchical Apex of Adult T-Cell Leukemia. Blood (2015) 125(23):3527–35. doi: 10.1182/blood-2014-10-607465
35. Sabatino M, Hu J, Sommariva M, Gautam S, Fellowes V, Hocker JD, et al. Generation of Clinical-Grade CD19-Specific CAR-Modified CD8+ Memory Stem Cells for the Treatment of Human B-Cell Malignancies. Blood (2016) 128(4):519–28. doi: 10.1182/blood-2015-11-683847
36. Noviello M, Manfredi F, Ruggiero E, Perini T, Oliveira G, Cortesi F, et al. Bone Marrow Central Memory and Memory Stem T-Cell Exhaustion in AML Patients Relapsing After HSCT. Nat Commun (2019) 10(1):1065. doi: 10.1038/s41467-019-08871-1
37. Galletti G, De Simone G, Mazza EMC, Puccio S, Mezzanotte C, Bi TM, et al. Two Subsets of Stem-Like CD8(+) Memory T Cell Progenitors With Distinct Fate Commitments in Humans. Nat Immunol (2020) 21(12):1552–62. doi: 10.1038/s41590-020-0791-5
38. Biasco L, Izotova N, Rivat C, Ghorashian S, Richardson R, Guvenel A, et al. Clonal Expansion of T Memory Stem Cells Determines Early Anti-Leukemic Responses and Long-Term CAR T Cell Persistence in Patients. Nat Cancer (2021) 2(6):629–42. doi: 10.1038/s43018-021-00207-7
39. Yan C, Chang J, Song X, Yan F, Yu W, An Y, et al. Memory Stem T Cells Generated by Wnt Signaling From Blood of Human Renal Clear Cell Carcinoma Patients. Cancer Biol Med (2019) 16(1):109–24. doi: 10.20892/j.issn.2095-3941.2018.0118
40. Costa Del Amo P, Lahoz-Beneytez J, Boelen L, Ahmed R, Miners KL, Zhang Y, et al. Human TSCM Cell Dynamics In Vivo Are Compatible With Long-Lived Immunological Memory and Stemness. PloS Biol (2018) 16(6):e2005523. doi: 10.1371/journal.pbio.2005523
41. Good Z, Borges L, Vivanco Gonzalez N, Sahaf B, Samusik N, Tibshirani R, et al. Proliferation Tracing With Single-Cell Mass Cytometry Optimizes Generation of Stem Cell Memory-Like T Cells. Nat Biotechnol (2019) 37(3):259–66. doi: 10.1038/s41587-019-0033-2
42. Zhao Y, Cai C, Samir J, Palgen JL, Keoshkerian E, Li H, et al. Human CD8 T-Stem Cell Memory Subsets Phenotypic and Functional Characterization are Defined by Expression of CD122 or CXCR3. Eur J Immunol (2021) 51(7):1732–47. doi: 10.1002/eji.202049057
43. Duckworth BC, Lafouresse F, Wimmer VC, Broomfield BJ, Dalit L, Alexandre YO, et al. Effector and Stem-Like Memory Cell Fates are Imprinted in Distinct Lymph Node Niches Directed by CXCR3 Ligands. Nat Immunol (2021) 22(4):434–48. doi: 10.1038/s41590-021-00878-5
44. Santegoets SJAM, Turksma AW, Suhoski MM, Stam AGM, Albelda SM, Hooijberg E, et al. IL-21 Promotes the Expansion of CD27+ CD28+ Tumor Infiltrating Lymphocytes With High Cytotoxic Potential and Low Collateral Expansion of Regulatory T Cells. Transl Med (2013) 11(1):37. doi: 10.1186/1479-5876-11-37
45. Becker TC, Wherry EJ, Boone D, Murali-Krishna K, Antia R, Ma A, et al. Interleukin 15 Is Required for Proliferative Renewal of Virus-Specific Memory CD8 T Cells. J Exp Med (2002) 195(12):1541–8. doi: 10.1084/jem.20020369
46. Yi JS, Du M, Zajac AJ. A Vital Role for Interleukin-21 in the Control of a Chronic Viral Infection. Science (2009) 324(5934):1572–6. doi: 10.1126/science.1175194
47. Hashimoto M, Im SJ, Araki K, Ahmed R. Cytokine-Mediated Regulation of CD8 T-Cell Responses During Acute and Chronic Viral Infection. Cold Spring Harb Perspect Biol (2019) 11(1):a028464. doi: 10.1101/cshperspect.a028464
48. Hurton LV, Singh H, Najjar AM, Switzer KC, Mi T, Maiti S, et al. Tethered IL-15 Augments Antitumor Activity and Promotes a Stem-Cell Memory Subset in Tumor-Specific T Cells. Proc Natl Acad Sci USA (2016) 113(48):E7788–97. doi: 10.1073/pnas.1610544113
49. Martkamchan S, Onlamoon N, Wang S, Pattanapanyasat K, Ammaranond P. The Effects of Anti-CD3/CD28 Coated Beads and IL-2 on Expanded T Cell for Immunotherapy. Adv Clin Exp Med (2016) 25(5):821–8. doi: 10.17219/acem/35771
50. Perdomo-Celis F, Medina-Moreno S, Davis H, Bryant J, Taborda NA, Rugeles MT, et al. Characterization of CXCR5(+) CD8(+) T-Cells in Humanized NSG Mice. Immunobiology (2020) 225(2):151885. doi: 10.1016/j.imbio.2019.11.020
51. Kaartinen T, Luostarinen A, Maliniemi P, Keto J, Arvas M, Belt H, et al. Low Interleukin-2 Concentration Favors Generation of Early Memory T Cells Over Effector Phenotypes During Chimeric Antigen Receptor T-Cell Expansion. Cytotherapy (2017) 19(6):689–702. doi: 10.1016/j.jcyt.2017.03.067
52. Gattinoni L, Klebanoff CA, Palmer DC, Wrzesinski C, Kerstann K, Yu Z, et al. Acquisition of Full Effector Function In Vitro Paradoxically Impairs the In Vivo Antitumor Efficacy of Adoptively Transferred CD8+ T Cells. J Clin Invest (2005) 115(6):1616–26. doi: 10.1172/JCI24480
53. Vignali D, Cantarelli E, Bordignon C, Canu A, Citro A, Annoni A, et al. Detection and Characterization of CD8 + Autoreactive Memory Stem T Cells in Patients With Type 1 Diabetes. Diabetes (2018) 67(5):936–45. doi: 10.2337/db17-1390
54. Abdelsamed HA, Moustaki A, Fan Y, Dogra P, Ghoneim HE, Zebley CC, et al. Human Memory CD8 T Cell Effector Potential Is Epigenetically Preserved During In Vivo Homeostasis. J Exp Med (2017) 214(6):1593–606. doi: 10.1084/jem.20161760
55. Adachi K, Kano Y, Nagai T, Okuyama N, Sakoda Y, Tamada K. IL-7 and CCL19 Expression in CAR-T Cells Improves Immune Cell Infiltration and CAR-T Cell Survival in the Tumor. Nat Biotechnol (2018) 36(4):346–51. doi: 10.1038/nbt.4086
56. Zanon V, Lugli E. Differentiation of Diverse Progenies of Memory T Cells From Naive CD8(+) T Cell Precursors. Methods Mol Biol (2017) 1514:103–10. doi: 10.1007/978-1-4939-6548-9_8
57. Alizadeh D, Wong RA, Yang X, Wang D, Pecoraro JR, Kuo CF, et al. IL15 Enhances CAR-T Cell Antitumor Activity by Reducing Mtorc1 Activity and Preserving Their Stem Cell Memory Phenotype. Cancer Immunol Res (2019) 7(5):759–72. doi: 10.1158/2326-6066.CIR-18-0466
58. Kondo T, Imura Y, Chikuma S, Hibino S, Omata-Mise S, Ando M, et al. Generation and Application of Human Induced-Stem Cell Memory T Cells for Adoptive Immunotherapy. Cancer Sci (2018) 109(7):2130–40. doi: 10.1111/cas.13648
59. Herda S, Heimann A, Obermayer B, Ciraolo E, Althoff S, Russ J, et al. Long-Term In Vitro Expansion Ensures Increased Yield of Central Memory T Cells as Perspective for Manufacturing Challenges. Int J Cancer (2021) 148(12):3097–110. doi: 10.1002/ijc.33523
60. Manfredi F, Abbati D, Cianciotti BC, Stasi L, Potenza A, Ruggiero E, et al. Flow Cytometry Data Mining by Cytochain Identifies Determinants of Exhaustion and Stemness in TCR-Engineered T Cells. Eur J Immunol (2021) 51(8):1992–2005. doi: 10.1002/eji.202049103
61. Castella M, Caballero-Banos M, Ortiz-Maldonado V, Gonzalez-Navarro EA, Sune G, Antonana-Vidosola A, et al. Point-Of-Care CAR T-Cell Production (ARI-0001) Using a Closed Semi-Automatic Bioreactor: Experience From an Academic Phase I Clinical Trial. Front Immunol (2020) 11:482. doi: 10.3389/fimmu.2020.00482
62. Fröhlich A, Kisielow J, Schmitz I, Freigang S, Shamshiev AT, Weber J, et al. IL-21R on T Cells Is Critical for Sustained Functionality and Control of Chronic Viral Infection. Science (2009) 324(5934):1576–80. doi: 10.1126/science.1172815
63. Wu S, Zhu W, Peng Y, Wang L, Hong Y, Huang L, et al. The Antitumor Effects of Vaccine-Activated CD8(+) T Cells Associate With Weak TCR Signaling and Induction of Stem-Like Memory T Cells. Cancer Immunol Res (2017) 5(10):908–19. doi: 10.1158/2326-6066.CIR-17-0016
64. Chen Y, Y F, Jiang Y, Chen J, Wu K, Chen X, et al. Adoptive Transfer of Interleukin-21-Stimulated Human CD8+ T Memory Stem Cells Efficiently Inhibits Tumor Growth. Immunother (2018) 41(6):274–83. doi: 10.1097/CJI.0000000000000229
65. Cui W, Liu Y, Weinstein JS, Craft J, Kaech SM. An Interleukin-21-Interleukin-10-STAT3 Pathway Is Critical for Functional Maturation of Memory CD8+ T Cells. Immunity (2011) 35(5):792–805. doi: 10.1016/j.immuni.2011.09.017
66. Hermans D, Gautam S, Garcia-Canaveras JC, Gromer D, Mitra S, Spolski R, et al. Lactate Dehydrogenase Inhibition Synergizes With IL-21 to Promote CD8(+) T Cell Stemness and Antitumor Immunity. Proc Natl Acad Sci USA (2020) 117(11):6047–55. doi: 10.1073/pnas.1920413117
67. Li Y, Cong Y, Jia M, He Q, Zhong H, Zhao Y, et al. Targeting IL-21 to Tumor-Reactive T Cells Enhances Memory T Cell Responses and Anti-PD-1 Antibody Therapy. Nat Commun (2021) 12(1):951. doi: 10.1038/s41467-021-21241-0
68. Pearce EL, Walsh MC, Cejas PJ, Harms GM, Shen H, Wang LS, et al. Enhancing CD8 T-Cell Memory by Modulating Fatty Acid Metabolism. Nature (2009) 460(7251):103–7. doi: 10.1038/nature08097
69. Johnson MO, Wolf MM, Madden MZ, Andrejeva G, Sugiura A, Contreras DC, et al. Distinct Regulation of Th17 and Th1 Cell Differentiation by Glutaminase-Dependent Metabolism. Cell (2018) 175(7):1780–95.e1719. doi: 10.1016/j.cell.2018.10.001
70. Zhang JY, Zhao YL, Lv YP, Cheng P, Chen W, Duan M, et al. Modulation of CD8(+) Memory Stem T Cell Activity and Glycogen Synthase Kinase 3beta Inhibition Enhances Anti-Tumoral Immunity in Gastric Cancer. Oncoimmunology (2018) 7(4):e1412900. doi: 10.1080/2162402X.2017.1412900
71. Klampatsa A, Dimou V, Albelda SM. Mesothelin-Targeted CAR-T Cell Therapy for Solid Tumors. Expert Opin Biol Ther (2021) 21(4):473–86. doi: 10.1080/14712598.2021.1843628
72. Sinclair LV, Rolf J, Emslie E, Shi Y-B, Taylor PM, Cantrell DA. Control of Amino-Acid Transport by Antigen Receptors Coordinates the Metabolic Reprogramming Essential for T Cell Differentiation. Nat Immunol (2013) 14(5):500–8. doi: 10.1038/ni.2556
73. O'Sullivan D, van der Windt GJ, Huang SC, Curtis JD, Chang CH, Buck MD, et al. Memory CD8(+) T Cells Use Cell-Intrinsic Lipolysis to Support the Metabolic Programming Necessary for Development. Immunity (2014) 41(1):75–88. doi: 10.1016/j.immuni.2014.06.005
74. Kondo T, Ando M, Nagai N, Tomisato W, Srirat T, Liu B, et al. The NOTCH-FOXM1 Axis Plays a Key Role in Mitochondrial Biogenesis in the Induction of Human Stem Cell Memory-Like CAR-T Cells. Cancer Res (2020) 80(3):471–83. doi: 10.1158/0008-5472.CAN-19-1196
75. Cui G, Staron MM, Gray SM, Ho PC, Amezquita RA, Wu J, et al. IL-7-Induced Glycerol Transport and TAG Synthesis Promotes Memory CD8+ T Cell Longevity. Cell (2015) 161(4):750–61. doi: 10.1016/j.cell.2015.03.021
76. Chang CH, Pearce EL. Emerging Concepts of T Cell Metabolism as a Target of Immunotherapy. Nat Immunol (2016) 17(4):364–8. doi: 10.1038/ni.3415
77. Hu Z, Zou Q, Su B. Regulation of T Cell Immunity by Cellular Metabolism. Front Med (2018) 12(4):463–72. doi: 10.1007/s11684-018-0668-2
78. Ma R, Ji T, Zhang H, Dong W, Chen X, Xu P, et al. A Pck1-Directed Glycogen Metabolic Program Regulates Formation and Maintenance of Memory CD8(+) T Cells. Nat Cell Biol (2018) 20(1):21–7. doi: 10.1038/s41556-017-0002-2
79. Mousset CM, Hobo W, Ji Y, Fredrix H, De Giorgi V, Allison RD, et al. Ex Vivo AKT-Inhibition Facilitates Generation of Polyfunctional Stem Cell Memory-Like CD8(+) T Cells for Adoptive Immunotherapy. Oncoimmunology (2018) 7(10):e1488565. doi: 10.1080/2162402X.2018.1488565
80. Giuliani C. The Flavonoid Quercetin Induces AP-1 Activation in FRTL-5 Thyroid Cells. Antioxidants (Basel) (2019) 8(5):112. doi: 10.3390/antiox8050112
81. Kared H, Tan SW, Lau MC, Chevrier M, Tan C, How W, et al. Immunological History Governs Human Stem Cell Memory CD4 Heterogeneity via the Wnt Signaling Pathway. Nat Commun (2020) 11(1):821. doi: 10.1038/s41467-020-14442-6
82. Kolan SS, Li G, Wik JA, Malachin G, Guo S, Kolan P, et al. Cellular Metabolism Dictates T Cell Effector Function in Health and Disease. Scand J Immunol (2020) 92(5):e12956. doi: 10.1111/sji.12956
83. Ma EH, Poffenberger MC, Wong AH, Jones RG. The Role of AMPK in T Cell Metabolism and Function. Curr Opin Immunol (2017) 46:45–52. doi: 10.1016/j.coi.2017.04.004
84. Scholz G, Jandus C, Zhang L, Grandclement C, Lopez-Mejia IC, Soneson C, et al. Modulation of mTOR Signalling Triggers the Formation of Stem Cell-Like Memory T Cells. EBioMedicine (2016) 4:50–61. doi: 10.1016/j.ebiom.2016.01.019
85. Geiger R, Rieckmann JC, Wolf T, Basso C, Feng Y, Fuhrer T, et al. L-Arginine Modulates T Cell Metabolism and Enhances Survival and Anti-Tumor Activity. Cell (2016) 167(3):829–42.e813. doi: 10.1016/j.cell.2016.09.031
86. Verma V, Jafarzadeh N, Boi S, Kundu S, Jiang Z, Fan Y, et al. MEK Inhibition Reprograms CD8(+) T Lymphocytes Into Memory Stem Cells With Potent Antitumor Effects. Nat Immunol (2021) 22(1):53–66. doi: 10.1038/s41590-020-00818-9
87. Utzschneider DT, Charmoy M, Chennupati V, Pousse L, Ferreira DP, Calderon-Copete S, et al. T Cell Factor 1-Expressing Memory-Like CD8(+) T Cells Sustain the Immune Response to Chronic Viral Infections. Immunity (2016) 45(2):415–27. doi: 10.1016/j.immuni.2016.07.021
88. Wu T, Ji Y, Moseman EA, Xu HC, Manglani M, Kirby M, et al. The TCF1-Bcl6 Axis Counteracts Type I Interferon to Repress Exhaustion and Maintain T Cell Stemness. Sci Immunol (2016) 1(6):eaai8593. doi: 10.1126/sciimmunol.aai8593
89. Kondo T, Morita R, Okuzono Y, Nakatsukasa H, Sekiya T, Chikuma S, et al. Notch-Mediated Conversion of Activated T Cells Into Stem Cell Memory-Like T Cells for Adoptive Immunotherapy. Nat Commun (2017) 8:15338. doi: 10.1038/ncomms15338
90. Huang X, Yang Y. Driving an Improved CAR for Cancer Immunotherapy. J Clin Invest (2016) 126(8):2795–8. doi: 10.1172/JCI88959
91. Chen Z, Ji Z, Ngiow SF, Manne S, Cai Z, Huang AC, et al. TCF-1-Centered Transcriptional Network Drives an Effector Versus Exhausted CD8 T Cell-Fate Decision. Immunity (2019) 51(5):840–55.e845. doi: 10.1016/j.immuni.2019.09.013
92. Raghu D, Xue HH, Mielke LA. Control of Lymphocyte Fate, Infection, and Tumor Immunity by TCF-1. Trends Immunol (2019) 40(12):1149–62. doi: 10.1016/j.it.2019.10.006
93. He QF, Xu Y, Li J, Huang ZM, Li XH, Wang X. CD8+ T-Cell Exhaustion in Cancer: Mechanisms and New Area for Cancer Immunotherapy. Brief Funct Genomics (2019) 18(2):99–106. doi: 10.1093/bfgp/ely006
94. Kurachi M. CD8(+) T Cell Exhaustion. Semin Immunopathol (2019) 41(3):327–37. doi: 10.1007/s00281-019-00744-5
95. Sawant DV, Yano H, Chikina M, Zhang Q, Liao M, Liu C, et al. Adaptive Plasticity of IL-10(+) and IL-35(+) Treg Cells Cooperatively Promotes Tumor T Cell Exhaustion. Nat Immunol (2019) 20(6):724–35. doi: 10.1038/s41590-019-0346-9
96. Siddiqui I, Schaeuble K, Chennupati V, Fuertes Marraco SA, Calderon-Copete S, Pais Ferreira D, et al. Intratumoral Tcf1(+)PD-1(+)CD8(+) T Cells With Stem-Like Properties Promote Tumor Control in Response to Vaccination and Checkpoint Blockade Immunotherapy. Immunity (2019) 50(1):195–211.e110. doi: 10.1016/j.immuni.2018.12.021
97. Dosset M, Joseph EL, Rivera Vargas T, Apetoh L. Modulation of Determinant Factors to Improve Therapeutic Combinations With Immune Checkpoint Inhibitors. Cells (2020) 9(7):1727. doi: 10.3390/cells9071727
98. Utzschneider DT, Gabriel SS, Chisanga D, Gloury R, Gubser PM, Vasanthakumar A, et al. Early Precursor T Cells Establish and Propagate T Cell Exhaustion in Chronic Infection. Nat Immunol (2020) 21(10):1256–66. doi: 10.1038/s41590-020-0760-z
99. Saeidi A, Zandi K, Cheok YY, Saeidi H, Wong WF, Lee CYQ, et al. T-Cell Exhaustion in Chronic Infections: Reversing the State of Exhaustion and Reinvigorating Optimal Protective Immune Responses. Front Immunol (2018) 9:2569. doi: 10.3389/fimmu.2018.02569
100. Woroniecka K, Chongsathidkiet P, Rhodin K, Kemeny H, Dechant C, Farber SH, et al. T-Cell Exhaustion Signatures Vary With Tumor Type and Are Severe in Glioblastoma. Clin Cancer Res (2018) 24(17):4175–86. doi: 10.1158/1078-0432.CCR-17-1846
101. Blank CU, Haining WN, Held W, Hogan PG, Kallies A, Lugli E, et al. Defining 'T Cell Exhaustion'. Nat Rev Immunol (2019) 19(11):665–74. doi: 10.1038/s41577-019-0221-9
102. Fribourg M, Anderson L, Fischman C, Cantarelli C, Perin L, La Manna G, et al. T-Cell Exhaustion Correlates With Improved Outcomes in Kidney Transplant Recipients. Kidney Int (2019) 96(2):436–49. doi: 10.1016/j.kint.2019.01.040
103. Kim K, Park S, Park SY, Kim G, Park SM, Cho JW, et al. Single-Cell Transcriptome Analysis Reveals TOX as a Promoting Factor for T Cell Exhaustion and a Predictor for Anti-PD-1 Responses in Human Cancer. Genome Med (2020) 12(1):22. doi: 10.1186/s13073-020-00722-9
104. Heim K, Binder B, Sagar, Wieland D, Hensel N, Llewellyn-Lacey S, et al. TOX Defines the Degree of CD8+ T Cell Dysfunction in Distinct Phases of Chronic HBV Infection. Gut (2020) 70(8):1550–60. doi: 10.1136/gutjnl-2020-322404
105. Seo H, Chen J, Gonzalez-Avalos E, Samaniego-Castruita D, Das A, Wang YH, et al. TOX and TOX2 Transcription Factors Cooperate With NR4A Transcription Factors to Impose CD8(+) T Cell Exhaustion. Proc Natl Acad Sci USA (2019) 116(25):12410–5. doi: 10.1073/pnas.1905675116
106. Wang X, He Q, Shen H, Xia A, Tian W, Yu W, et al. TOX Promotes the Exhaustion of Antitumor CD8(+) T Cells by Preventing PD1 Degradation in Hepatocellular Carcinoma. J Hepatol (2019) 71(4):731–41. doi: 10.1016/j.jhep.2019.05.015
107. Yao C, Sun HW, Lacey NE, Ji Y, Moseman EA, Shih HY, et al. Single-Cell RNA-Seq Reveals TOX as a Key Regulator of CD8(+) T Cell Persistence in Chronic Infection. Nat Immunol (2019) 20(7):890–901. doi: 10.1038/s41590-019-0403-4
108. Khan O, Giles JR, McDonald S, Manne S, Ngiow SF, Patel KP, et al. TOX Transcriptionally and Epigenetically Programs CD8(+) T Cell Exhaustion. Nature (2019) 571(7764):211–8. doi: 10.1038/s41586-019-1325-x
109. Xu-Monette ZY, Zhou J, Young KH. PD-1 Expression and Clinical PD-1 Blockade in B-Cell Lymphomas. Blood (2018) 131(1):68–83. doi: 10.1182/blood-2017-07-740993
110. Zeng Z, Wei F, Ren X. Exhausted T Cells and Epigenetic Status. Cancer Biol Med (2020) 17(4):923–36. doi: 10.20892/j.issn.2095-3941.2020.0338
111. Beltra JC, Manne S, Abdel-Hakeem MS, Kurachi M, Giles JR, Chen Z, et al. Developmental Relationships of Four Exhausted CD8(+) T Cell Subsets Reveals Underlying Transcriptional and Epigenetic Landscape Control Mechanisms. Immunity (2020) 52(5):825–41.e828. doi: 10.1016/j.immuni.2020.04.014
112. Cai MC, Zhao X, Cao M, Ma P, Chen M, Wu J, et al. T-Cell Exhaustion Interrelates With Immune Cytolytic Activity to Shape the Inflamed Tumor Microenvironment. J Pathol (2020) 251(2):147–59. doi: 10.1002/path.5435
113. Alvarez-Fernandez C, Escriba-Garcia L, Vidal S, Sierra J, Briones J. A Short CD3/CD28 Costimulation Combined With IL-21 Enhance the Generation of Human Memory Stem T Cells for Adoptive Immunotherapy. J Transl Med (2016) 14(1):214. doi: 10.1186/s12967-016-0973-y
114. Pilipow K, Scamardella E, Lugli E. Generating Stem-Like Memory T Cells With Antioxidants for Adoptive Cell Transfer Immunotherapy of Cancer. Methods Enzymol (2020) 631:137–58. doi: 10.1016/bs.mie.2019.08.016
115. Tuluc F, Spitsin S, Tustin NB, Murray JB, Tustin R 3rd, Schankel LA, et al. Decreased PD-1 Expression on CD8 Lymphocyte Subsets and Increase in CD8 Tscm Cells in Children With HIV Receiving Raltegravir. AIDS Res Hum Retroviruses (2017) 33(2):133–42. doi: 10.1089/AID.2016.0108
116. Kallies A, Zehn D, Utzschneider DT. Precursor Exhausted T Cells: Key to Successful Immunotherapy? Nat Rev Immunol (2020) 20(2):128–36. doi: 10.1038/s41577-019-0223-7
117. Terranova-Barberio M, Pawlowska N, Dhawan M, Moasser M, Chien AJ, Melisko ME, et al. Exhausted T Cell Signature Predicts Immunotherapy Response in ER-Positive Breast Cancer. Nat Commun (2020) 11(1):3584. doi: 10.1038/s41467-020-17414-y
118. Verdon DJ, Mulazzani M, Jenkins MR. Cellular and Molecular Mechanisms of CD8(+) T Cell Differentiation, Dysfunction and Exhaustion. Int J Mol Sci (2020) 21(19):7357. doi: 10.3390/ijms21197357
119. Kagoya Y, Nakatsugawa M, Yamashita Y, Ochi T, Guo T, Anczurowski M, et al. BET Bromodomain Inhibition Enhances T Cell Persistence and Function in Adoptive Immunotherapy Models. J Clin Invest (2016) 126(9):3479–94. doi: 10.1172/JCI86437
120. He K, Liu P, Xu LX. The Cryo-Thermal Therapy Eradicated Melanoma in Mice by Eliciting CD4(+) T-Cell-Mediated Antitumor Memory Immune Response. Cell Death Dis (2017) 8(3):e2703. doi: 10.1038/cddis.2017.125
121. Anderson KG, Stromnes IM, Greenberg PD. Obstacles Posed by the Tumor Microenvironment to T Cell Activity: A Case for Synergistic Therapies. Cancer Cell (2017) 31(3):311–25. doi: 10.1016/j.ccell.2017.02.008
122. He W, Zhang H, Han F, Chen X, Lin R, Wang W, et al. CD155T/TIGIT Signaling Regulates CD8(+) T-Cell Metabolism and Promotes Tumor Progression in Human Gastric Cancer. Cancer Res (2017) 77(22):6375–88. doi: 10.1158/0008-5472.CAN-17-0381
123. Hashimoto M, Kamphorst AO, Im SJ, Kissick HT, Pillai RN, Ramalingam SS, et al. CD8 T Cell Exhaustion in Chronic Infection and Cancer: Opportunities for Interventions. Annu Rev Med (2018) 69:301–18. doi: 10.1146/annurev-med-012017-043208
124. Choi BD, Maus MV, June CH, Sampson JH. Immunotherapy for Glioblastoma: Adoptive T-Cell Strategies. Clin Cancer Res (2019) 25(7):2042–8. doi: 10.1158/1078-0432.CCR-18-1625
125. Atrash S, Bano K, Harrison B, Abdallah AO. CAR-T Treatment for Hematological Malignancies. J Investig Med (2020) 68(5):956–64. doi: 10.1136/jim-2020-001290
126. Abramson JS. Anti-CD19 CAR T-Cell Therapy for B-Cell Non-Hodgkin Lymphoma. Transfus Med Rev (2020) 34(1):29–33. doi: 10.1016/j.tmrv.2019.08.003
127. Kasakovski D, Xu L, Li Y. T Cell Senescence and CAR-T Cell Exhaustion in Hematological Malignancies. J Hematol Oncol (2018) 11(1):91. doi: 10.1186/s13045-018-0629-x
128. Blaeschke F, Stenger D, Kaeuferle T, Willier S, Lotfi R, Kaiser AD, et al. Induction of a Central Memory and Stem Cell Memory Phenotype in Functionally Active CD4(+) and CD8(+) CAR T Cells Produced in an Automated Good Manufacturing Practice System for the Treatment of CD19(+) Acute Lymphoblastic Leukemia. Cancer Immunol Immunother (2018) 67(7):1053–66. doi: 10.1007/s00262-018-2155-7
129. Guan L, Li X, Wei J, Liang Z, Yang J, Weng X, et al. Antigen-Specific CD8+ Memory Stem T Cells Generated From Human Peripheral Blood Effectively Eradicate Allogeneic Targets in Mice. Stem Cell Res Ther (2018) 9(1):337. doi: 10.1186/s13287-018-1080-1
130. Hosokawa K, Muranski P, Feng X, Townsley DM, Liu B, Knickelbein J, et al. Memory Stem T Cells in Autoimmune Disease: High Frequency of Circulating CD8+ Memory Stem Cells in Acquired Aplastic Anemia. J Immunol (2016) 196(4):1568–78. doi: 10.4049/jimmunol.1501739
131. Wang Y, Whittall T, Neil S, Britton G, Mistry M, Rerks-Ngarm S, et al. A Novel Mechanism Linking Memory Stem Cells With Innate Immunity in Protection Against HIV-1 Infection. Sci Rep (2017) 7(1):1057. doi: 10.1038/s41598-017-01188-3
132. Lee YJ, Park JA, Kwon H, Choi YS, Jung KC, Park SH, et al. Role of Stem Cell-Like Memory T Cells in Systemic Lupus Erythematosus. Arthritis Rheumatol (2018) 70(9):1459–69. doi: 10.1002/art.40524
133. Alvarez-Fernandez C, Escriba-Garcia L, Caballero AC, Escudero-Lopez E, Ujaldon-Miro C, Montserrat-Torres R, et al. Memory Stem T Cells Modified With a Redesigned CD30-Chimeric Antigen Receptor Show an Enhanced Antitumor Effect in Hodgkin Lymphoma. Clin Transl Immunol (2021) 10(4):e1268. doi: 10.1002/cti2.1268
134. Teo YWB, Linn YC, Goh YT, Li S, Ho LP. Tumor Infiltrating Lymphocytes From Acute Myeloid Leukemia Marrow can be Reverted to CD45RA+ Central Memory State by Reactivation in SIP (Simulated Infective Protocol). Immunobiology (2019) 224(4):526–31. doi: 10.1016/j.imbio.2019.05.001
135. Hoffmann JM, Schubert ML, Wang L, Huckelhoven A, Sellner L, Stock S, et al. Differences in Expansion Potential of Naive Chimeric Antigen Receptor T Cells From Healthy Donors and Untreated Chronic Lymphocytic Leukemia Patients. Front Immunol (2017) 8:1956. doi: 10.3389/fimmu.2017.01956
136. Liu J, Zhong JF, Zhang X, Zhang C. Allogeneic CD19-CAR-T Cell Infusion After Allogeneic Hematopoietic Stem Cell Transplantation in B Cell Malignancies. J Hematol Oncol (2017) 10(1):35. doi: 10.1186/s13045-017-0405-3
137. Mollanoori H, Shahraki H, Rahmati Y, Teimourian S. CRISPR/Cas9 and CAR-T Cell, Collaboration of Two Revolutionary Technologies in Cancer Immunotherapy, an Instruction for Successful Cancer Treatment. Hum Immunol (2018) 79(12):876–82. doi: 10.1016/j.humimm.2018.09.007
138. Agarwal S, Weidner T, Thalheimer FB, Buchholz CJ. In Vivo Generated Human CAR T Cells Eradicate Tumor Cells. Oncoimmunology (2019) 8(12):e1671761. doi: 10.1080/2162402X.2019.1671761
139. Brudno JN, Kochenderfer JN. Recent Advances in CAR T-Cell Toxicity: Mechanisms, Manifestations and Management. Blood Rev (2019) 34:45–55. doi: 10.1016/j.blre.2018.11.002
140. Biasco L, Scala S, Ricci LB, Dionisio F, Baricordi C, Calabria A, et al. In Vivo Tracking of T Cells in Humans Unveils Decade-Long Survival and Activity of Genetically Modified T Memory Stem Cells. Transl Med (2015) 7(273):273ra213. doi: 10.1126/sciimmunol.aay0555
141. Jin L, Tao H, Karachi A, Long Y, Hou AY, Na M, et al. CXCR1- or CXCR2-Modified CAR T Cells Co-Opt IL-8 for Maximal Antitumor Efficacy in Solid Tumors. Nat Commun (2019) 10(1):4016. doi: 10.1038/s41467-019-11869-4
142. Choe JH, W PB, Simic MS, Gilbert RD, Li AW, Krasnow NA, et al. SynNotch-CAR T Cells Overcome Challenges of Specificity, Heterogeneity, and Persistence in Treating Glioblastoma. Sci Transl Med (2021) 13(591):eabe7378. doi: 10.1126/scitranslmed.abe7378
143. Padgett LE, Dinh HQ, Wu R, Gaddis DE, Araujo DJ, Winkels H, et al. Naive CD8(+) T Cells Expressing CD95 Increase Human Cardiovascular Disease Severity. Arterioscler Thromb Vasc Biol (2020) 40(12):2845–59. doi: 10.1161/ATVBAHA.120.315106
144. Flynn JK, Gorry PR. Stem Memory T Cells (TSCM)-Their Role in Cancer and HIV Immunotherapies. Clin Transl Immunol (2014) 3(7):e20. doi: 10.1038/cti.2014.16
145. Flynn JK, Paukovics G, Cashin K, Borm K, Ellett A, Roche M, et al. Quantifying Susceptibility of CD4+ Stem Memory T-Cells to Infection by Laboratory Adapted and Clinical HIV-1 Strains. Viruses (2014) 6(2):709–26. doi: 10.3390/v6020709
146. Chahroudi A, Silvestri G, Lichterfeld M. T Memory Stem Cells and HIV: A Long-Term Relationship. Curr HIV/AIDS Rep (2015) 12(1):33–40. doi: 10.1007/s11904-014-0246-4
147. Calascibetta F, Micci L, Carnathan D, Lawson B, Vanderford TH, Bosinger SE, et al. Antiretroviral Therapy in Simian Immunodeficiency Virus-Infected Sooty Mangabeys: Implications for AIDS Pathogenesis. J Virol (2016) 90(16):7541–51. doi: 10.1128/JVI.00598-16
148. Cartwright EK, Palesch D, Mavigner M, Paiardini M, Chahroudi A, Silvestri G. Initiation of Antiretroviral Therapy Restores CD4+ T Memory Stem Cell Homeostasis in Simian Immunodeficiency Virus-Infected Macaques. J Virol (2016) 90(15):6699–708. doi: 10.1128/JVI.00492-16
149. Vigano S, Negron J, Ouyang Z, Rosenberg ES, Walker BD, Lichterfeld M, et al. Prolonged Antiretroviral Therapy Preserves HIV-1-Specific CD8 T Cells With Stem Cell-Like Properties. J Virol (2015) 89(15):7829–40. doi: 10.1128/JVI.00789-15
150. Lu X, Song B, Weng W, Xia H, Su B, Wu H, et al. CD4(+) T Memory Stem Cells Correlate With Disease Progression in Chronically HIV-1-Infected Patients. Viral Immunol (2017) 30(9):642–8. doi: 10.1089/vim.2017.0017
151. Guardo AC, Zarama A, Gonzalez T, Bargallo ME, Rojas J, Martinez E, et al. Effects on Immune System and Viral Reservoir of a Short-Cycle Antiretroviral Therapy in Virologically Suppressed HIV-Positive Patients. AIDS (2019) 33(6):965–72. doi: 10.1097/QAD.0000000000002169
152. Munusamy Ponnan S, Hayes P, Fernandez N, Thiruvengadam K, Pattabiram S, Nesakumar M, et al. Evaluation of Antiviral T Cell Responses and TSCM Cells in Volunteers Enrolled in a Phase I HIV-1 Subtype C Prophylactic Vaccine Trial in India. PloS One (2020) 15(2):e0229461. doi: 10.1371/journal.pone.0229461
153. Manganaro L, Hong P, Hernandez MM, Argyle D, Mulder LCF, Potla U, et al. IL-15 Regulates Susceptibility of CD4(+) T Cells to HIV Infection. Proc Natl Acad Sci USA (2018) 115(41):E9659–67. doi: 10.1073/pnas.1806695115
154. Utay NS, Vigil KJ, Somasunderam A, Aulicino PC, Smulevitz B, Chiadika S, et al. Timing of Antiretroviral Therapy Initiation Determines Rectal Natural Killer Cell Populations. AIDS Res Hum Retroviruses (2020) 36(4):314–23. doi: 10.1089/AID.2019.0225
155. Finley J. Elimination of Cancer Stem Cells and Reactivation of Latent HIV-1 via AMPK Activation: Common Mechanism of Action Linking Inhibition of Tumorigenesis and the Potential Eradication of HIV-1. Med Hypotheses (2017) 104:133–46. doi: 10.1016/j.mehy.2017.05.032
156. Fenwick C, Joo V, Jacquier P, Noto A, Banga R, Perreau M, et al. T-Cell Exhaustion in HIV Infection. Immunol Rev (2019) 292(1):149–63. doi: 10.1111/imr.12823
157. Sharov KS. HIV/SARS-CoV-2 Co-Infection: T Cell Profile, Cytokine Dynamics and Role of Exhausted Lymphocytes. Int J Infect Dis (2021) 102:163–9. doi: 10.1016/j.ijid.2020.10.049
158. Zhao S, Xu W, Tu B, Hong WG, Zhang Z, Chen WW, et al. Alterations of the Frequency and Functions of Follicular Regulatory T Cells and Related Mechanisms in HIV Infection. J Infect (2020) 81(5):776–84. doi: 10.1016/j.jinf.2020.09.014
159. Roederer M, Quaye L, Mangino M, Beddall MH, Mahnke Y, Chattopadhyay P, et al. The Genetic Architecture of the Human Immune System: A Bioresource for Autoimmunity and Disease Pathogenesis. Cell (2015) 161(2):387–403. doi: 10.1016/j.cell.2015.02.046
160. Cianciotti BC, Ruggiero E, Campochiaro C, Oliveira G, Magnani ZI, Baldini M, et al. CD4+ Memory Stem T Cells Recognizing Citrullinated Epitopes Are Expanded in Patients With Rheumatoid Arthritis and Sensitive to Tumor Necrosis Factor Blockade. Arthritis Rheumatol (2020) 72(4):565–75. doi: 10.1002/art.41157
161. Lee YJ, Park EH, Park JW, Jung KC, Lee EB. Proinflammatory Features of Stem Cell-Like Memory T Cells From Human Patients With Rheumatoid Arthritis. J Immunol (2021) 207(2):381–8. doi: 10.4049/jimmunol.2000814
162. Cao J, Zhang C, Han X, Cheng H, Chen W, Qi K, et al. Emerging Role of Stem Cell Memory-Like T Cell in Immune Thrombocytopenia. Scand J Immunol (2019) 89(3):e12739. doi: 10.1111/sji.12739
163. Bride KL, Vincent T, Smith-Whitley K, Lambert MP, Bleesing JJ, Seif AE, et al. Sirolimus Is Effective in Relapsed/Refractory Autoimmune Cytopenias: Results of a Prospective Multi-Institutional Trial. Blood (2016) 127(1):17–28. doi: 10.1182/blood-2015-07-657981
164. Flynn JK, Gorry PR. T Cell Therapies-are T Memory Stem Cells the Answer? Ann Transl Med (2015) 3(17):251. doi: 10.3978/j.issn.2305-5839.2015.08.13
Keywords: T memory stem cells, stemness, tumor immunotherapy, HIV, autoimmune diseases
Citation: Li Y, Wu D, Yang X and Zhou S (2021) Immunotherapeutic Potential of T Memory Stem Cells. Front. Oncol. 11:723888. doi: 10.3389/fonc.2021.723888
Received: 11 June 2021; Accepted: 23 August 2021;
Published: 17 September 2021.
Edited by:
Ira Ida Skvortsova, Innsbruck Medical University, AustriaReviewed by:
Xia Liu, Baylor College of Medicine, United StatesSukhbir Kaur, National Institutes of Health (NIH), United States
Copyright © 2021 Li, Wu, Yang and Zhou. This is an open-access article distributed under the terms of the Creative Commons Attribution License (CC BY). The use, distribution or reproduction in other forums is permitted, provided the original author(s) and the copyright owner(s) are credited and that the original publication in this journal is cited, in accordance with accepted academic practice. No use, distribution or reproduction is permitted which does not comply with these terms.
*Correspondence: Sufang Zhou, enNmMjAwMDAwQDE2My5jb20=