- 1Graduate School of Biomedical Sciences, Texas Tech University Health Sciences Center El Paso, El Paso, TX, United States
- 2Earle A. Chiles Research Institute, Providence Cancer Institute, Portland, OR, United States
- 3Center of Emphasis in Cancer, Department of Molecular and Translational Medicine, Paul L. Foster School of Medicine, Texas Tech University Health Sciences Center El Paso, El Paso, TX, United States
Background: Increased expression of the progesterone receptor membrane component 1, a heme and progesterone binding protein, is frequently found in triple negative breast cancer tissue. The basis for the expression of PGRMC1 and its regulation on cellular signaling mechanisms remain largely unknown. Therefore, we aim to study microRNAs that target selective genes and mechanisms that are regulated by PGRMC1 in TNBCs.
Methods: To identify altered miRNAs, whole human miRNome profiling was performed following AG-205 treatment and PGRMC1 silencing. Network analysis identified miRNA target genes while KEGG, REACTOME and Gene ontology were used to explore altered signaling pathways, biological processes, and molecular functions.
Results: KEGG term pathway analysis revealed that upregulated miRNAs target specific genes that are involved in signaling pathways that play a major role in carcinogenesis. While multiple downregulated miRNAs are known oncogenes and have been previously demonstrated to be overexpressed in a variety of cancers. Overlapping miRNA target genes associated with KEGG term pathways were identified and overexpression/amplification of these genes was observed in invasive breast carcinoma tissue from TCGA. Further, the top two genes (CCND1 and YWHAZ) which are highly genetically altered are also associated with poorer overall survival.
Conclusions: Thus, our data demonstrates that therapeutic targeting of PGRMC1 in aggressive breast cancers leads to the activation of miRNAs that target overexpressed genes and deactivation of miRNAs that have oncogenic potential.
Introduction
Breast cancer is the most commonly diagnosed cancer in women in the U.S (1). Treatment for breast cancers are guided by the identification of hormone receptors, Estrogen receptor (ER), Progesterone receptor (PR), and Human Epidermal Growth Factor Receptor 2 (HER2) (2, 3). Based on receptor status, breast cancers are categorized into four major molecular subtypes: Luminal A, Luminal B, HER2-enriched, and triple negative/basal-like (3). Among these triple negative breast cancers (TNBCs) are the most aggressive breast cancers with an overall poorer prognosis compared to other subtypes (4, 5). Because TNBC lack ER, PR and HER2, endocrine and antibody-based therapy are ineffective (6–8). Therefore, it is important to identify novel molecular drivers that enable TNBC growth and metastasis and target or reprogram these markers to better treat patients with aggressive metastatic cancers.
Recent evidence in multiple cancers (9–13) including breast cancer (14–16) identify microRNAs (miRNAs) as novel gene expression regulators and potential biomarkers (17–19). miRNAs are small non-coding RNAs approximately 19 to 25 nucleotides in length; they control gene expression by targeting selective-sequences of mRNAs, inducing translational repression or complete mRNA degradation (20). miRNA expression profiles have the ability to identify molecular breast cancer subtypes (21, 22) and can differentiate between basal and luminal subtypes (23). Their effect on hormone receptor expression, regulation, and activity remains in its infant stage. Ongoing studies however, have a major focus for miRNAs that target genes that are altered in aggressive breast cancers while dysregulation of miRNAs has been directly linked to aggressive basal-like breast cancers (24–28). Although one miRNA can target hundreds of genes, treatments that can switch-on specific miRNAs could lead to direct targeted gene suppression of multiple genes that are overexpressed or have oncogenic potential.
PGRMC1 a member of the membrane-associated progesterone receptor (MAPR) family with the ability to initiate non-classical signaling has been described in breast cancers (29–33). PGRMC1 overexpression is observed in more aggressive phenotypes and is associated with poor prognosis in patients diagnosed with ER-negative breast cancers (34). In addition, in vitro and in vivo studies demonstrate that PGRMC1 possess the ability to promote the growth and survival of human breast cancer cells and xenografted breast tumors (35, 36). Although PGRMC1 expression has been observed in multiple cancers (36–40), it’s signaling mechanism remains unknown.
Sequencing and microarray technology has opened new insights into the genetic and genomic landscape of all breast cancers including TNBC (41, 42). For example, amplification of MYC and loss-of-function mutation of BRCA1 are often described in TNBCs (43, 44). Further, the most frequently mutated or amplified genes in TNBCs include PI3KCA (55%), AKT1 (13%) and CDH1 (13%) (45). These genes can activate downstream cell-cycle regulators that can either activate (cyclin D1) or repress (p53), leading to sustained proliferation and inhibition of apoptosis of breast cancers (46). Our recent work demonstrated that PGRMC1 activates EGFR and PI3K/AKT signaling pathways, leading to increased cell proliferation of TNBC cells (33). While, other studies have demonstrated cell-specific effects between PGRMC1 and AKT signaling (47–49). Historically, the PI3K/AKT pathway is one of the most altered signaling mechanisms in human cancers (50–53). It plays a key role in controlling cellular processes such as cell proliferation and tumor growth (54, 55). Although directly targeting amplified genes such as PI3KCA and AKT1 has proven to be difficult but promising (56, 57), novel genes that behave in a similar fashion should be identified.
To uncover genes and pathways associated with PGRMC1 in TNBCs we performed human miRNome profiling. We impaired PGRMC1 signaling using a chemical inhibitor and RNA interference. Whole human miRNome profiling identified miRNAs that were both up and down regulated following PGRMC1 impairment. Using an array of online databases and datasets we identified direct miRNA target genes. We proceeded to study these genes by identifying their involvement in the different signaling pathways that were altered following PGRMC1 suppression. More importantly, these genes were differentially expressed in human metastatic tumor samples. From all of the miRNA target genes observed, CyclinD1 (CCND1) and 14-3-3 protein zeta/delta (YWHAZ) had the highest gene expression in human tumors and were involved in various signaling pathways. Patient samples with high expression of either gene were associated with overall poorer survival probability. Increased relative gene expression and copy number variation of CCND1 and YWHAZ was observed in MDA-MB-468 breast cancer cells and silencing PGRMC1 reduced the expression of these genes. Interestingly, multiple miRNAs (miR-224, miR-550a, miR-181a, miR-664a, miR-30b, miR-345, miR-93) that were downregulated upon PGRMC1 impairment are known to be overexpressed in multiple cancers and are described as possible oncogenes. Our results demonstrate that targeting PGRMC1 regulates miRNAs that directly target amplified genes and downregulates oncogenic miRNAs in TNBCs.
Materials and Methods
Cell Culture
MDA-MB-468 cells were obtained from the American Type Culture Collection (Manassas, VA, USA). Cells were cultured in RPMI-1640 media supplemented with 100 units/mL of penicillin, 100 μg/mL of streptomycin (Life Technologies, Grand Island, NY, USA), and 10% fetal bovine serum (FBS). Cells were incubated at 37°C in 5% CO2 and maintained at an atmosphere of 95% air.
Treatment With Small Molecule Inhibitor and Gene Silencing
MDA-MB-468 cells were plated in six-well plates at a density of 5x105 cells/well and allowed to attach overnight. Cells were then either treated with 50 μM AG-205 for 24 h or transfected with PGRMC1 siRNA for 48 h. Using MIrus bio TransIT siQUEST transfection reagent (Mirus Bio) with either a control scrambled-sequence or siRNAs targeting PGRMC1-sequence (Origene). Three different siRNA sequences (A, B and C) and multiple concentrations ranging from 20 to 60 nM were used to effectively silence PGRMC1. To minimize toxicity, the ratio of siRNA to transfection reagent was maintained at 1:1, in accordance with the manufacture’s protocol. siRNA sequences used were as follows:
SR323253A-rGrArUrCrArArCrUrUrUrUrArGrUrCrArUrGrArUrGrUrUCT
SR323253B-rCrArArUrUrGrArCrUrUrArArCrUrGrCrArUrGrArUrUrUCT
SR323253C-rUrCrArArCrUrUrUrUrArGrUrCrArUrGrArUrGrUrUrCrUGT
Quantitative RT-PCR
Total RNA was isolated from MDA-MB-468 breast cancer cells using the TRIzol reagent (Invitrogen, Carlsbad, CA, USA). RNA was then reverse transcribed using the RT2 first strand kit (Qiagen; Cat. No. 330401). qRT-PCR was performed using the StepOnePlus real time PCR system (Applied Biosystems, Foster City, CA, USA). The comparative Ct (2-ΔΔCT) method was used to analyze the results. The primers used for PGRMC1, CCND1, YWHAZ and 18S are as follows:
PGRMC1
Forward: 5′-CGACGGCGTCCAGGACCC-3′
Reverse: 5′-TCTTCCTCATCTGAGTACACAG-3′
CCND1
Forward: 5′-ATGGAACATCAGCTGCTGT-3′
Reverse: 5′-TCAGATGTCCACATCCCGC-3′
YWHAZ
Forward: 5′-ATGCAACCAACACATCCTATC-3′
Reverse: 5′- GCATTATTAGCGTGCTGTCTT-3′
18S
Forward: 5′-CCTCGATGCTCTTAGCTGAGT-3′
Reverse: 5′-TCCTAGCTGCGGTATCCAG-3′
miRNome Profiling
Global microRNA profiling was generated using the SABiosciences PCR miScript PCR Array Human miRNome (Cat No. MIHS-216Z). Briefly, total RNA was extracted using TRIzol reagent (Life Technologies) from MDA-MB-468 cells treated with 50 μM AG-205 for 24 h or 48 h post siRNA transfection. Human miRNome array was performed following the synthesis of cDNA using miScript II RT kit (SABiosciences). miScript miRNA PCR array was performed using miScript SYBR Green PCR Kit (SABiosciences). All of the differentially expressed miRNAs were well-characterized in the human genome as annotated by miRNet (http://www.mirnet.ca/).
Identifying Pathways Altered by PGRMC1 Using KEGG, Gene Ontology and Reactome
Using KEGG and gene ontology terms we analyzed the signaling pathways that were significantly altered following PGRMC1 disruption. The Reactome Analysis Tool (http://reactome.org) (58, 59) was used to visualize the genome-wide hierarchy of enriched pathways in response to PGRMC1. The most significantly enriched pathways are represented as yellow and are maintained in the middle of the circular representation and the less or non-significantly enriched pathways are labeled in grey. A list of all the miRNA target genes was uploaded into the Reactome database and significantly enriched pathway analysis was defined by FDR < 0.05.
Determining PGRMC1-Induced Genetic Alterations Using In Silico Analysis
To study possible genetic alterations such as inframe, missense, truncating mutations as well as gene amplification and deep deletion of the miRNA target genes observed following PGRMC1 disruption. We uploaded the DEG dataset onto the cbioportal (http://www.cbioportal.org/) database and analyzed it in reference to the cancer genome atlas (TCGA). Oncoprint diagrams were used to visualize genetic alterations from invasive breast carcinoma samples (60). Because we impaired PGRMC1 in TNBC cells, using the xena platform (https://xenabrowser.net) database, we studied the altered gene expression in response to PGRMC1 disruption. More specifically we obtained data from the breast cancer cell line Heiser 2012 (54 breast and breast cancer cell lines), breast cancer cell line encyclopedia (68 breast and breast cancer cell lines) as well as TCGA Breast Cancer (BRCA) dataset (n = 1,247 samples).
Assessing PGRMC1 Signaling and Overall Survival in Breast Cancer Patients Using KM Plotter and Interaction of miRNA Target Genes Using Genemania
The cBioportal (http://www.cbioportal.org/) database was used to study overall cumulative survival of patients with high and low expression of the miRNA target genes observed following PGRMC1 impairment. Kaplan-Meier plots were generated from TCGA breast invasive carcinoma samples (n=817). To study the impact of individual genes on overall survival probability, we used the KM plotter (http://kmplot.com/) database and generated Kaplan-Meier plots from ER-negative/HER2-negative breast cancer samples (n=869). Finally, using genemania 3 (http://genemania.org) we explored the interconnection between miRNA target genes involved in the pathways that were significantly altered following PGRMC1 impairment.
Statistical Analysis
All data are expressed as the mean ± SD. The differences between control and experimental groups were compared using Student’s t-test. P < 0.05 was considered to be statistically significant. Statistical analysis was conducted using GraphPad Prism 7 software, version 7.0 (GraphPad Prism Software, San Diego, CA, USA).
Results
Disrupting PGRMC1 Signaling the Human miRNome
To identify miRNAs regulated by PGRMC1, whole human miRNome profiling was performed using a miScript miRNA PCR array (miRNome V16) where a total of 1,084 mature miRNAs including their respective controls were measured. MDA-MB-468 breast cancer cells were treated with 50 µM AG-205. AG-205 is known to disrupt the downstream signaling of PGRMC1 possibly causing it to accumulate in the membrane. Therefore, it was not surprising to observe an increase in PGRMC1 mRNA expression (Figure 1A) as earlier studies have shown increased protein expression of PGRMC1 following AG-205 treatment (33, 38). Human miRNome profiling following AG-205 treatment identified alterations in the expression of various miRNAs (Figure 1B). The 20 most upregulated and downregulated miRNAs were observed (Figures 1C, D). Because AG-205 increased PGRMC1 mRNA expression, we proceeded to silence PGRMC1 to further study its impact on miRNA expression (Figure 1E). Following successful PGRMC1 silencing, human miRNome profiling identified alterations to 776 miRNAs (Figure 1F). Here again, the 20 most upregulated and downregulated miRNAs, were identified (Figures 1G, H). We then identified the target genes for the 20 most altered miRNAs using the miRNet database. Following AG-205 treatment the 20 most upregulated miRNAs targeted 2,898 genes while the 20 most downregulated miRNAs targeted 2,501 genes (Figure 1I and Supplementary Tables 1, 2). Similarly, the top 20 most upregulated miRNAs accounted for 1,788 target genes. While, the 20 most downregulated miRNAs targeted 3,029 genes after PGRMC1 was silenced (Figure 1J and Supplementary Tables 3, 4).
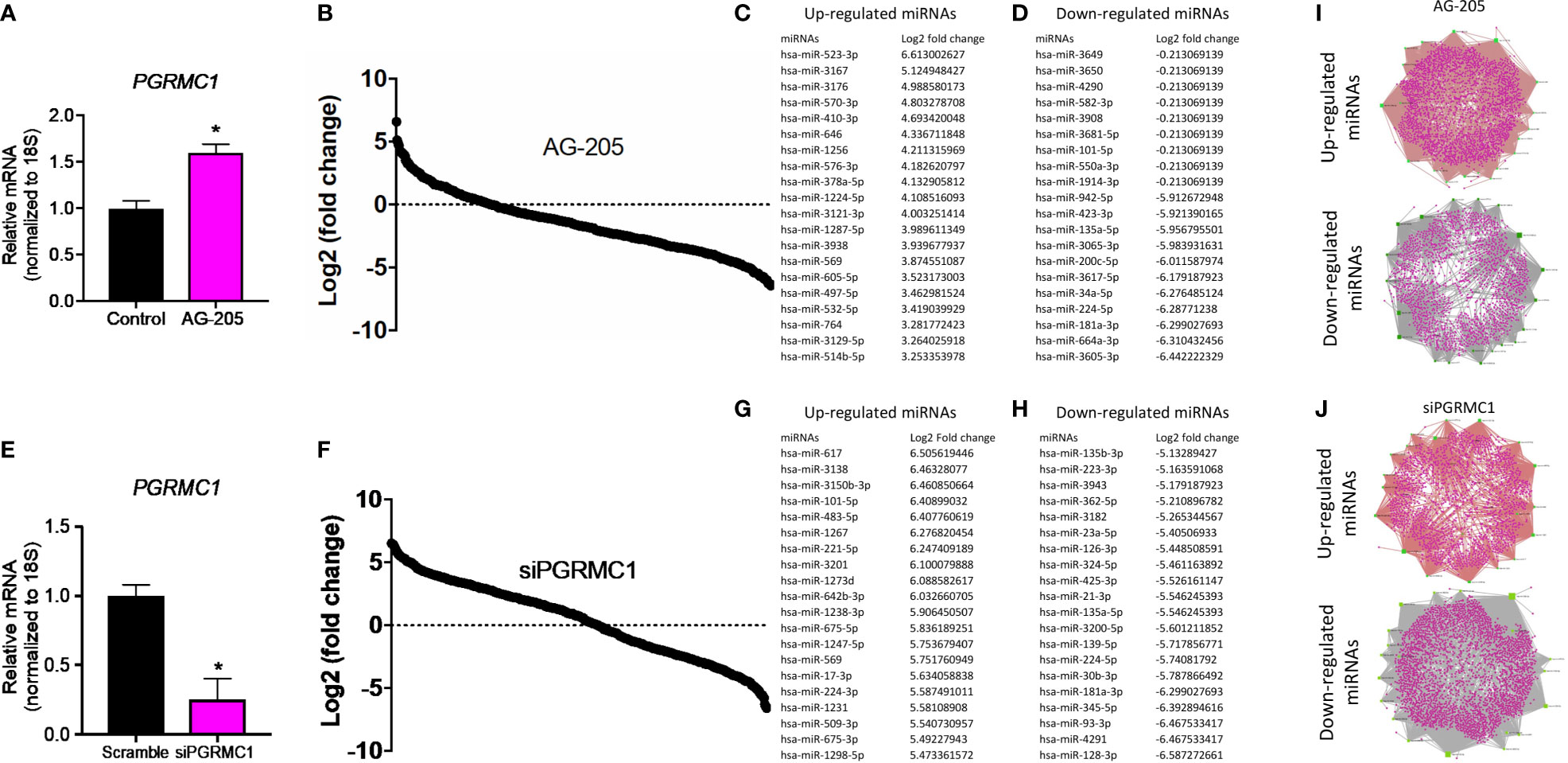
Figure 1 Human miRNome profiling identified differentially regulated miRNAs following PGRMC1 signal disruption and silencing. (A) Relative mRNA expression of PGRMC1 in MDA-MB-468 breast cancer cells following 50 µM AG-205 after 24 h. (B) Whole human miRNome profiling identified differentially expressed miRNAs following signaling disruption by AG-205 treatment. (C) The top 20 most upregulated miRNAs were identified all which had a log2 (fold change) greater than 3. (D) The 20 most downregulated miRNAs, all which had a log2 (fold change) less than 1. (E) Relative mRNA expression of PGRMC1 in MDA-MB-468 cells following PGRMC1 silencing after 48 h. (F) miRNome profiling identified differentially expressed miRNAs following PGRMC1 silencing. (G) The 20 most upregulated miRNAs with a log2 (fold change) greater than 5. (H) The 20 most downregulated miRNAs were identified all which had a log2 (fold change) less than -5. (I) Interaction network hubs of the top 20 up and downregulated miRNAs and their mRNA target genes following AG-205 treatment. (J) Interaction network hubs of the top 20 up and downregulated miRNAs and their mRNA target genes following PGRMC1 silencing. Four individual networks are demonstrated with miRNAs illustrated in green, miRNA-mRNA interacting nodes in brown and target genes represented in pink. *P < 0.05.
PGRMC1 Signal Disruption Alters miRNAs Involved in Pathways Associated With Cancers
Since our earlier analysis with the top 20 miRNAs altered by PGRMC1 resulted in a large number of target genes, we proceeded to study the network analysis of the top 10 most upregulated and downregulated miRNAs following AG-205 treatment. Network analysis of the top 10 most upregulated miRNAs (hsa-miR-523-3p, hsa-miR-3167, hsa-miR-3176, hsa-miR-570-3p, hsa-miR-410-3p, hsa-miR-646, hsa-miR-1256, hsa-miR-576-3p, hsa-miR-378a-5p and hsa-miR-1224-5p) identified 1,479 target genes (Figure 2A and Supplementary Table 5) while the top 10 most downregulated miRNAs (hsa-miR-3681-5p, hsa-miR-3617-5p, hsa-miR-34a-5p, hsa-miR-101-5p, hsa-miR-224-5p, hsa-miR-550a-3p, hsa-miR-181a-3p, hsa-miR-1914-3p, hsa-miR-664a-3p and hsa-miR-3605-3p) targeted 1,402 genes (Figure 2B and Supplementary Table 6). Studying the top miRNAs made our study more focused on miRNAs that may be more effectively regulated by PGRMC1. To identify miRNA target genes that could have a significant impact, we narrowed down our search by performing KEGG and gene ontology analysis. KEGG terms of the computed 1,479 target genes allowed us to pin-point and identify target genes of PGRMC1 altered miRNAs that are uniquely involved within the top signaling pathways, which interestingly included, p53 signaling pathway, cell cycle and pathways in cancers (Figure 2C; Supplementary Figure 1 and Supplementary Table 7). Interestingly, the downregulated miRNAs also significantly altered pathways in cancer, cell cycle and p53 signaling pathways (Figure 2D; Supplementary Figure 2 and Supplementary Table 8). Further, gene functions including kinase binding, single-stranded DNA binding, gene silencing, intrinsic apoptotic signaling pathway, regulated program cell death, enzyme binding, and nucleotide binding were classified using gene ontology based molecular functions and biological processes of both up and downregulated miRNAs (Figures 2E, F). The candidate 10 most up and downregulated miRNAs following AG-205 treatment and their respective target genes were listed (Tables 1, 2).
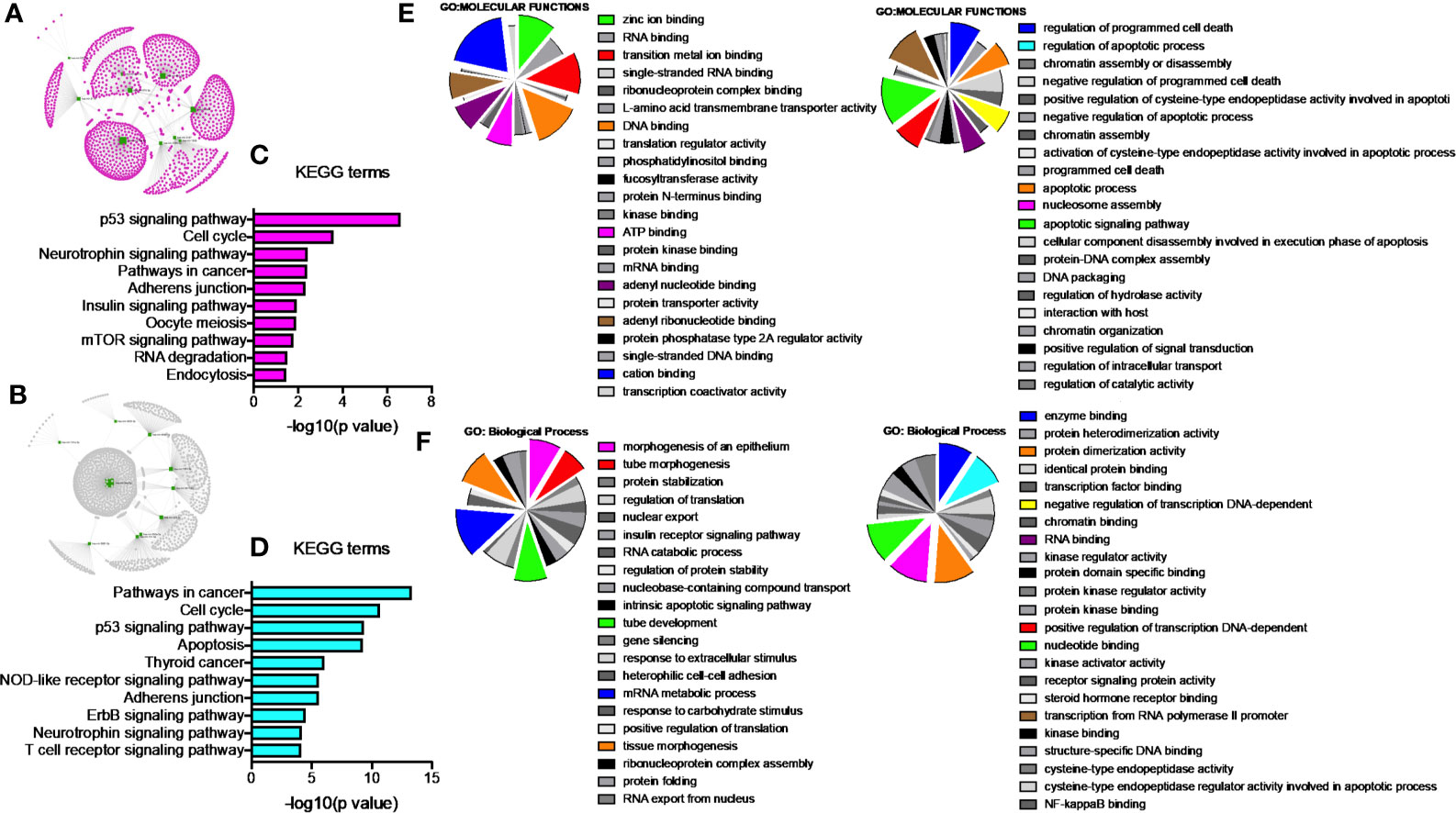
Figure 2 Network analysis identifies mRNA target genes involved in altered pathways following AG-205 treatment. (A) The top ten upregulated miRNAs depicted in green, identify target genes highlighted in pink. (B) The top ten downregulated miRNAs are also depicted in green with their respective target genes highlighted in grey. (C) and (D) KEGG pathway analysis identified the top 10 significantly enriched pathways (non-disease related) involved within the miRNA network hub, adjusted p < 0.05. (E, F). GO: term Molecular functions and Biological process involved within the observed miRNAs.
miRNAs Regulated Signaling Pathways Identified Following PGRMC1 Silencing
Network analysis following PGRMC1 silencing identified 1,015 genes as targets of the 10 most upregulated miRNAs (hsa-miR-617, hsa-miR-3138, hsa-miR-3150b-3p, hsa-miR-101-5p, hsa-miR-483-5p, hsa-miR-1267, hsa-miR-221-5p, hsa-miR-3201, hsa-miR-1273d and hsa-miR-642b-3p) (Figure 3A and Supplementary Table 9). While, 2,010 genes were identified to be direct targets of the top 10 most downregulated miRNAs (hsa-miR-135a-5p, hsa-miR-3200-5p, hsa-miR-139-5p, hsa-miR-224-5p, hsa-miR-30b-3p, hsa-miR-181a-3p, hsa-miR-345-5p, hsa-miR-93-3p, hsa-miR-4291 and hsa-miR-128-3p) (Figure 3B and Supplementary Table 10). KEGG analysis of the upregulated (Figure 3C; Supplementary Figure 4 and Supplementary Table 11) and downregulated (Figure 3D; Supplementary Figure 5 and Supplementary Table 12) miRNAs following PGRMC1 silencing identified enrichment to similar KEGG terms observed in the AG-205 treatment group, such as p53 signaling pathway, cell cycle and pathways in cancers. Gene ontology terms, identified important molecular functions and biological processes including protein kinase binding, transcription factor binding, MAPK kinase activity, inactivation of MAPK activity, intrinsic apoptotic signaling pathway, purine nucleotide binding, adenyl nucleotide binding, protein phosphorylation, and regulation of phosphorylation (Figures 3E, F). The candidate 10 most up and downregulated miRNAs following PGRMC1 silencing and their respective target genes were listed (Tables 3, 4).
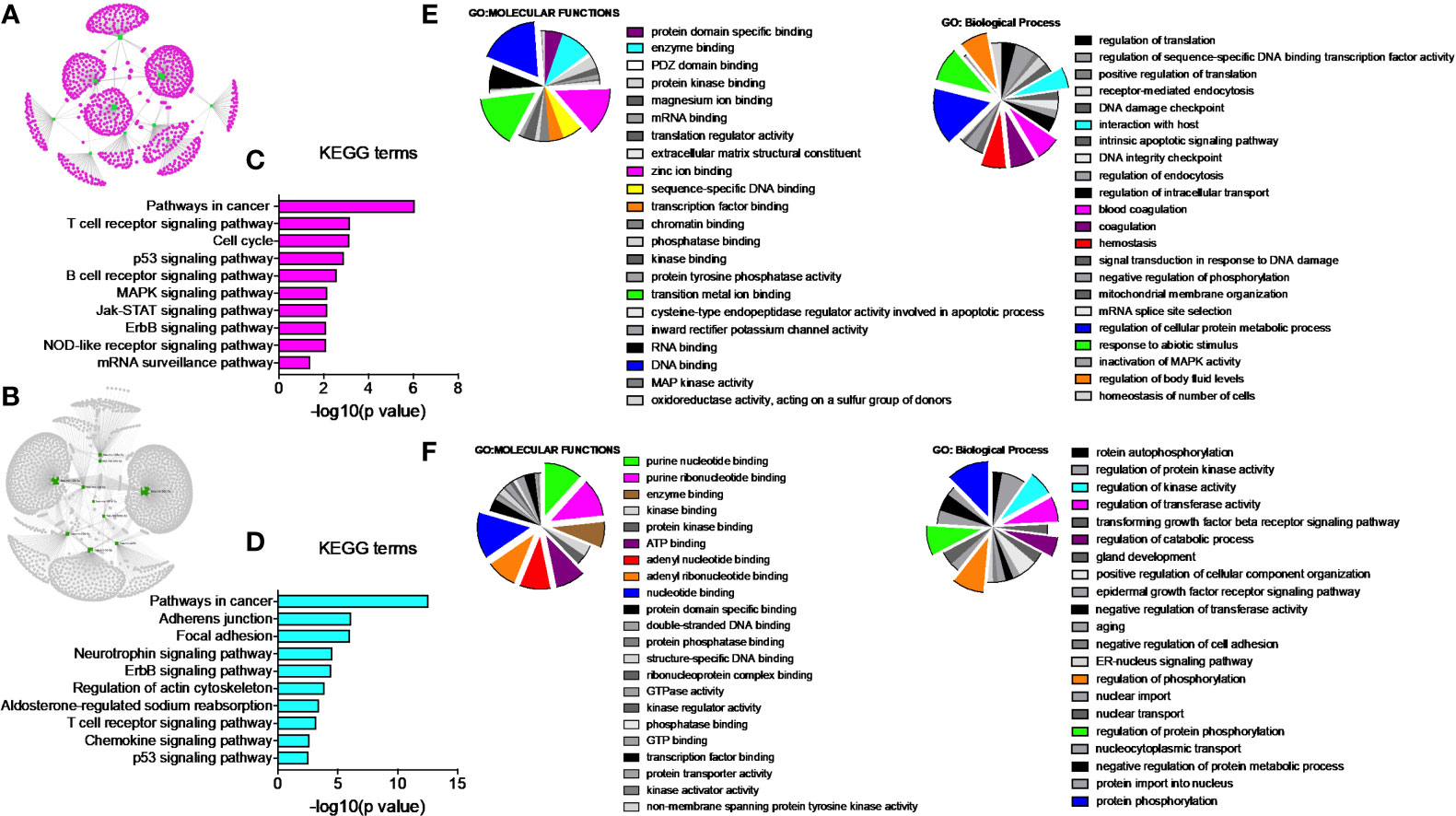
Figure 3 PGRMC1 silencing alters pathways that are have miRNA target genes involved. Silencing PGRMC1 upregulates different miRNAs (from AG-205 treatment) that target similar miRNA target genes which are also upregulated in metastatic breast cancer samples. (A) Target genes highlighted in pink of the top ten most upregulated miRNAs highlighter in green. (B) The top ten most downregulated miRNAs highlighted in green and their direct targets highlighted in grey. (C) and (D) The top 10 most significantly enriched pathways (non-disease related) were identified by KEGG analysis, adjusted p < 0.05. (E, F) miRNA target genes show involvement in GO: terms Molecular functions and Biological process.
PGRMC1 Signal Disruption and Silencing Alters miRNAs That Target Genes Involved in Breast Cancers
Once we identified the altered pathways following PGRMC1 signal disruption by AG-205 treatment we wanted to identify if the genes that are directly involved within these pathways are observed in breast cancer patient samples. Therefore, the identified genes were taken and computed into the xenabrowser database. TCGA data from primary and metastatic tumor samples was downloaded and plotted. Genes from p53 signaling pathway, cell cycle neutrophin signaling pathways, pathways in cancer, adherens junction, insulin signaling pathway, oocyte meiosis, mTOR signaling pathway, RNA degradation, and endocytosis were differentially expressed in both metastatic and primary tumor tissue samples (Figure 4). Target genes of downregulated miRNAs were also differentially expressed in similar pathways including pathways in cancer, cell cycle, and p53 signaling pathway (Supplementary Figure 5). Identified genes involved within each pathway following PGRMC1 silencing were similarly computed into the xenabrowser database. TCGA data analyzed from metastatic tumor samples identified upregulated miRNA target genes to be involved in pathways in cancer, T cell receptor signaling pathway, cell cycle, p53 signaling pathway, B cell receptor signaling pathway, MAPK signaling pathway, JAK-STAT signaling pathway, ErbB signaling pathway, NOD-like receptor signaling pathway, and mRNA surveillance pathway (Figure 5). Intriguingly, downregulated miRNAs had similarly altered miRNA target genes in pathways in cancer, p53 signaling pathway, T cell receptor signaling pathway and ErbB signaling pathway (Supplementary Figure 6). However, some miRNA target genes were also observed in adherens junctions, focal adhesion, neurotrophin signaling pathway, regulation of actin cytoskeleton, aldosterone-regulated sodium reabsorption and chemokine signaling pathway (Supplementary Figure 6).
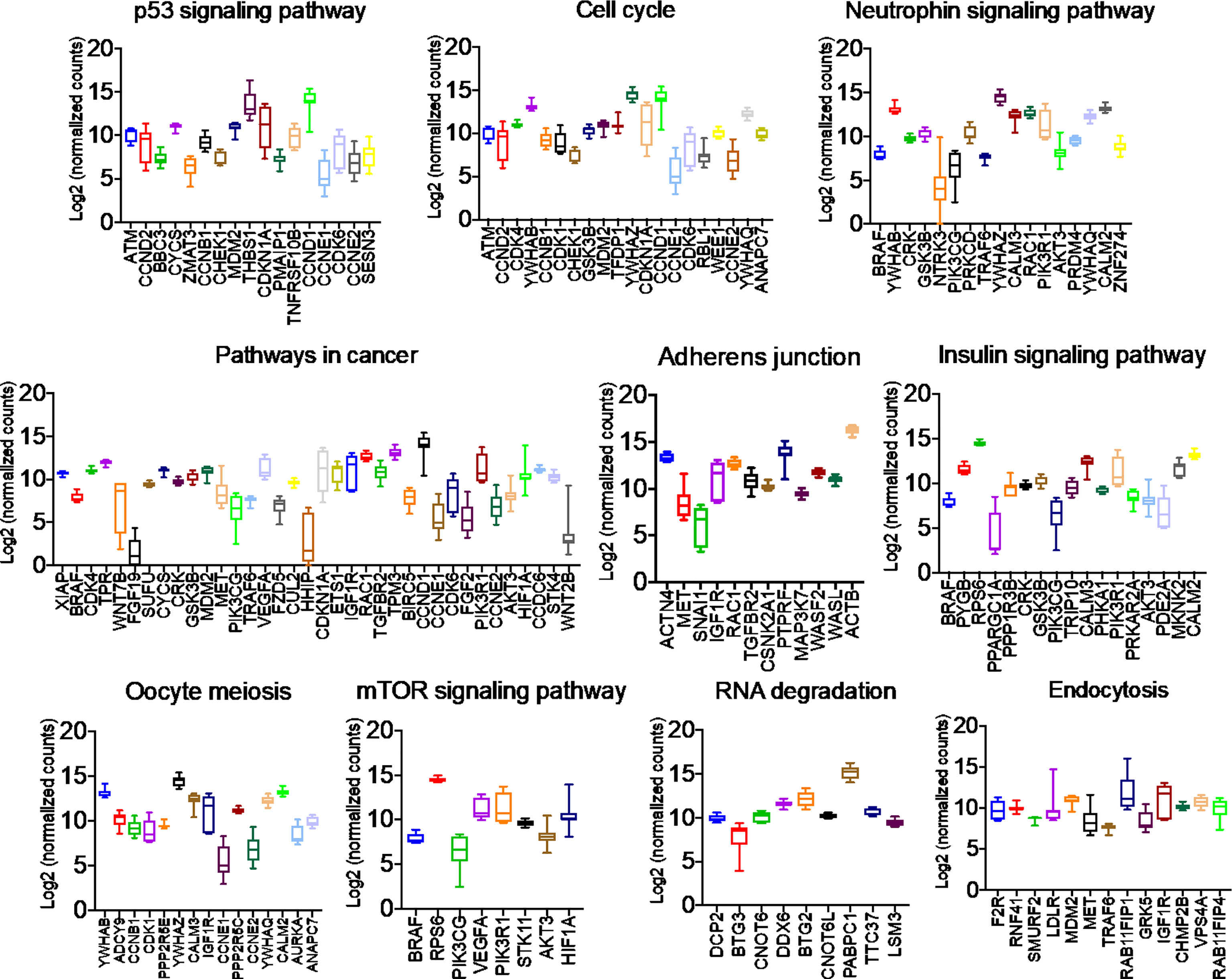
Figure 4 Network analysis identified miRNA target genes to be upregulated in breast cancers following AG-205 treatment. miRNAs target differentially expressed genes miRNA target genes that are upregulated in metastatic breast tumor samples. A Log2 (normalized_counts) expression of upregulated miRNA target genes in metastatic breast tumor samples downloaded from TCGA database. miRNA target genes are involved in term pathways identified by KEGG analysis and are direct targets of the top miRNAs.
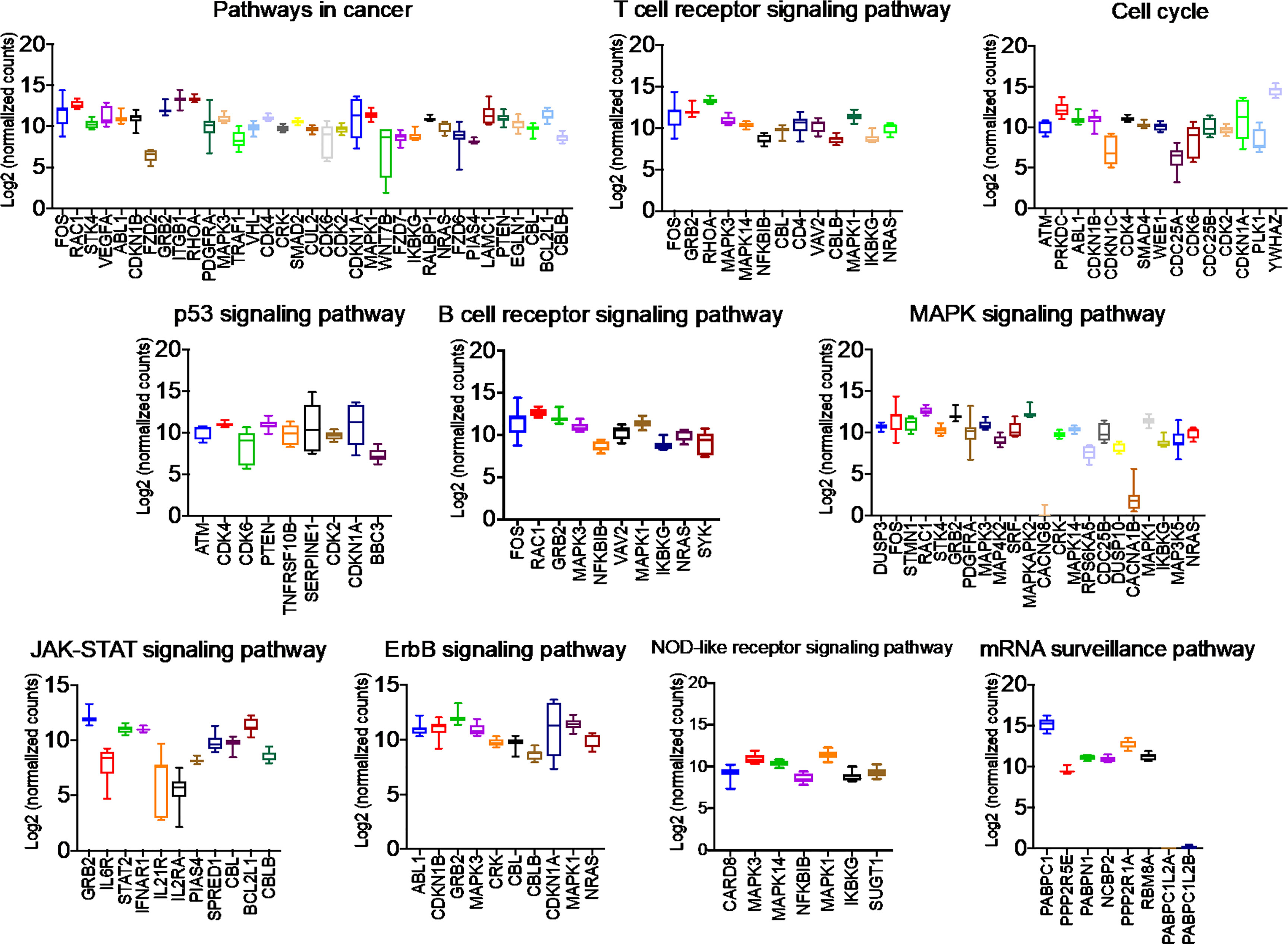
Figure 5 Network analysis identified miRNA target genes to be upregulated in breast cancers following AG-205 treatment. The top upregulated miRNA target genes involved in KEGG pathway analysis have upregulated Log2 (normalized_counts) expression in metastatic breast tumor samples obtained from TCGA database.
PGRMC1 Regulates miRNAs Involved in Cell Cycle, Disease Signal and Transduction Processes
Gene network analysis allowed us to identify novel target genes and we were able to classify them using KEGG term enrichment following AG-205 treatment of PGRMC1 silencing. We employed the Reactome database to study pathway-topology analysis using the miRNA target genes from KEGG and GO analysis. Using the Reactome pathway identifier we were able to observe genes that are mapped to pathways and over-represented within those pathways (58, 61). Following AG-205 treatment, we identified over-representation of miRNA target genes in pathways involved in cell cycle, gene expression (Transcription), disease, and signal transduction (Figure 6A). Similarly, following PGRMC1 silencing we observed over-representation of miRNA target genes in pathways involved in immune system, signal transduction, gene expression (transcription), and cell cycle (Figure 6B).
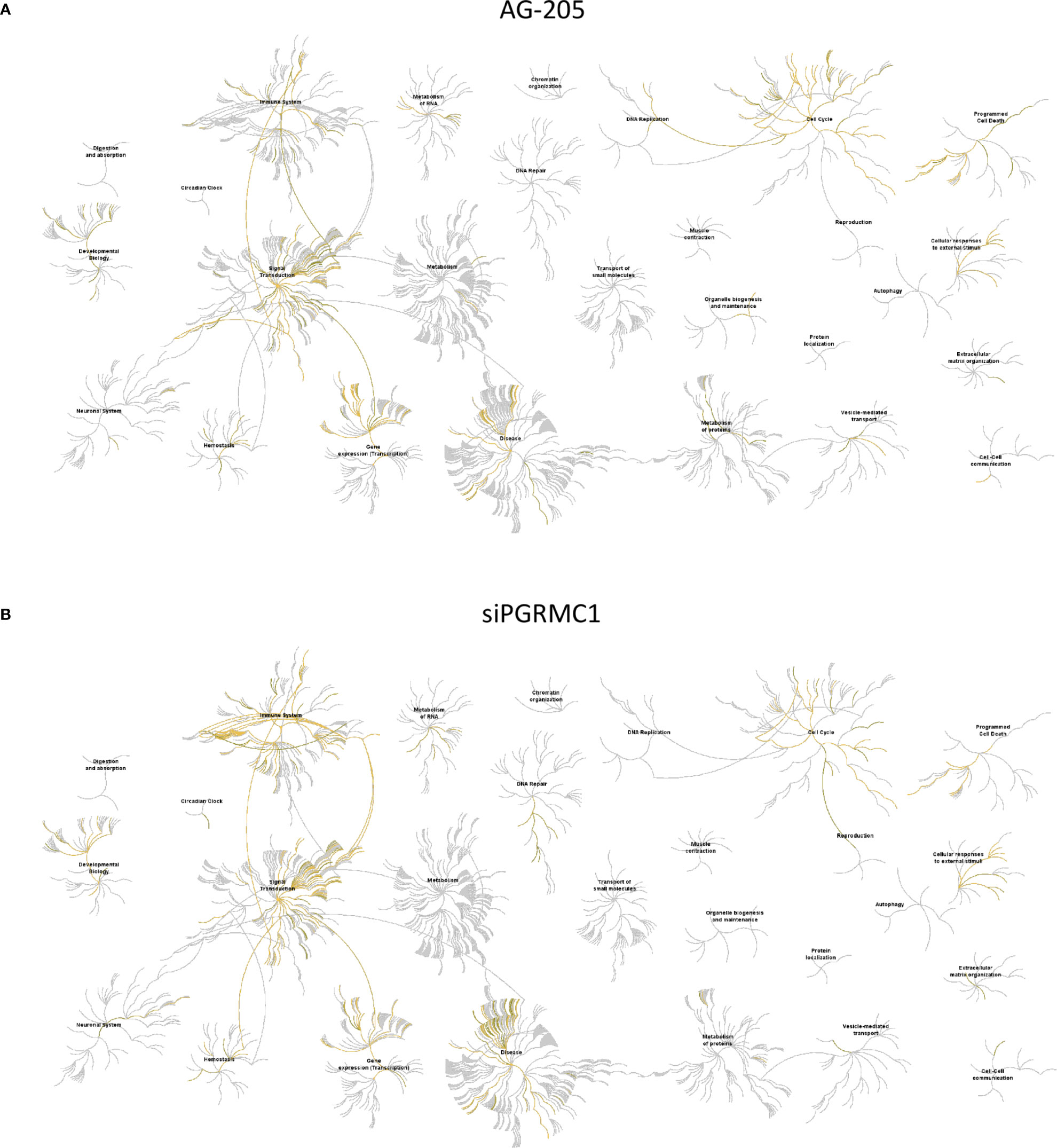
Figure 6 Reactome pathway analysis of the genes identified by KEGG term analysis. (A) Reactome pathways analysis of the miRNA target genes (n = 112) identified following AG-205 treatment illustrates increased pathway involvement. (B) Top pathways involved within the miRNA target genes (n = 84) observed following PGRMC1 silencing were also mapped. Over-represented pathways are highlighted in yellow. All overexpressed pathways are from gene lists of formerly annotated and published signatures.
Functional Annotation Analysis of PGRMC1 Altered miRNA Target Genes in Invasive Breast Carcinomas Samples Using TCGA Dataset
TCGA data was used to study possible genetic alterations of the miRNA target genes due to miRNA alterations in response to PGRMC1 disruption. From the miRNA target genes observed, the top 22 that displayed increased mRNA expression within the spectrum of signaling pathways identified by KEGG were further analyzed. Using the cBioportal database we were able to observe and differentiate between the miRNA target genes based on genetic alteration. Using oncoprint we visualized the genetic alterations in the 22 miRNA target genes (CCND1, YWHAZ, TPM3, BTG2, PABPC1, IGF1R, RAB11FIP1, PRKDC, MAPKAPK2, MAPK3, THBS1, CALM2, PIK3R1, RPS6, ACTB, PTPRF, ITGB1, RHOA, MAPK1, BCL2L1, RAC1 and PPP2R1A) (Figure 7A and Supplementary Figure 7). However, the percentage of genetic alteration varied within each gene and most miRNA target genes that displayed an alteration in > 5 percent were mainly amplified (Figure 7A). Patients that displayed high expression of these genes had a cumulative lower survival rate (Figure 7B). Network analysis by the Genemania database demonstrated that these amplified genes have tight interactions within signaling pathways. The light-red lines connect genes that are known to directly interact with one another within signaling pathways that are well studied (Figure 7C). Although, cumulatively these genes displayed a lower survival rate, only high expression of CCDN1 and YWHAZ in ER-negative breast cancer patients displayed significant overall lower survival probability (Figure 7D and Supplementary Figure 8). Finally, gene expression data analysis from the breast cancer cell line dataset and copy number variation from the cancer cell line encyclopedia dataset similarly demonstrated increased expression/CN variation of CCND1 and YWHAZ in TNBC cell lines (Figure 7E). Further, we also confirmed the decreased expression of CCND1 and YWHAZ in PGRMC1 silenced MDA-MB-468 cells (Figure 7F). Overall, our in vitro and in silico analysis demonstrates that PGRMC1 plays a major role in influencing the miRNome in such a way that these alterations favor breast tumor growth and progression.
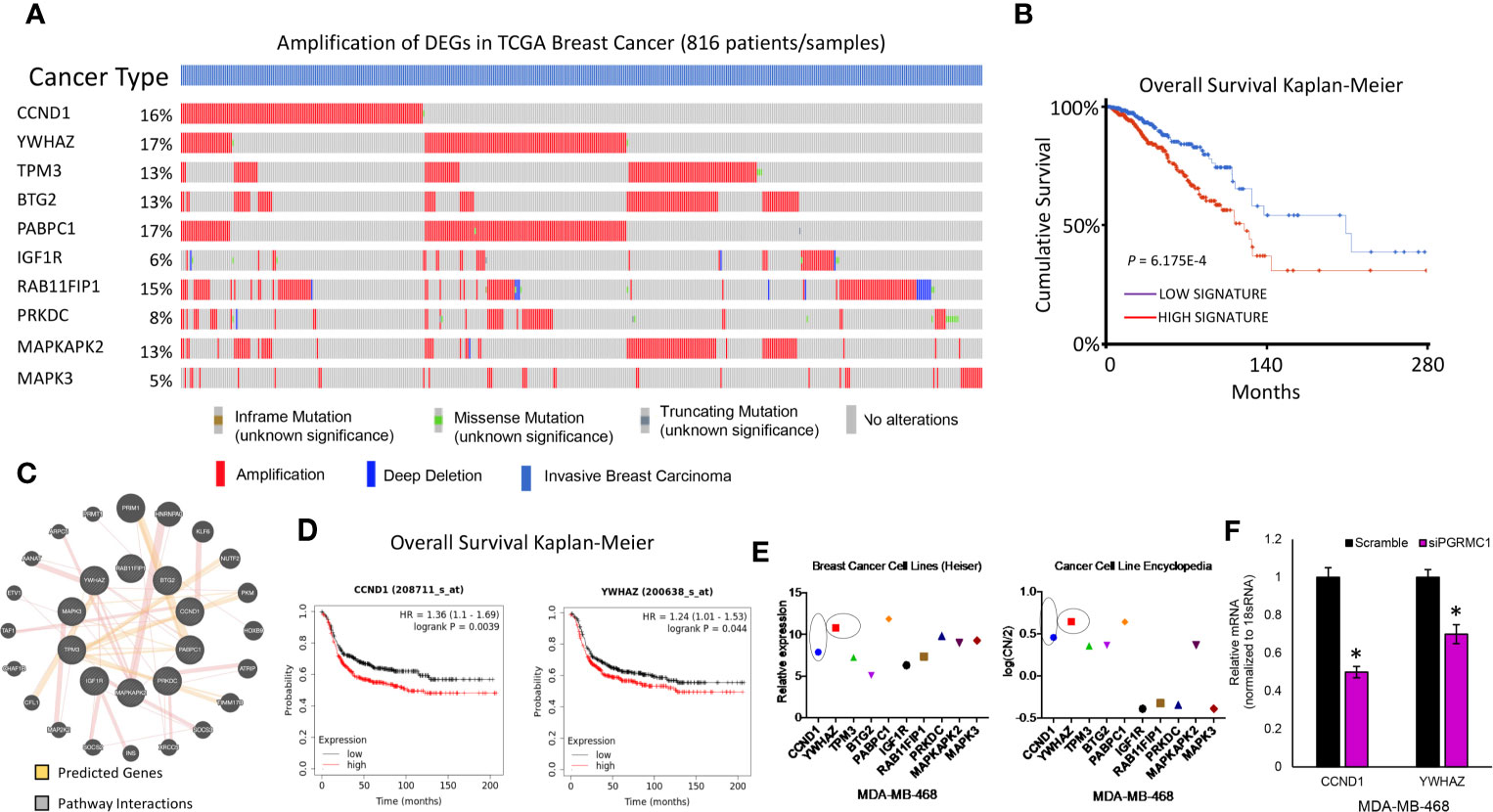
Figure 7 PGRMC1 impairment identified miRNA target genes to be amplified in invasive breast carcinoma patients. (A) Oncoprint illustrates genetic alterations such as inframe mutations, missense mutation, truncating mutation, amplification and deep deletion of breast cancer tumor samples (n=816). miRNA target genes that had a greater than 5% genetic alteration were considered for further analysis. (B) Cumulatively patient samples that have high signature/expression of miRNA target genes exhibiting > 5% genetic alterations are associated with poorer overall survival. (C) Network analysis links the top ten miRNA target genes with associated pathway interactions and predicts interactions within known pathways. (D) The top two miRNA target genes, CCND1 and YWHAZ are associated with significantly poorer overall survival in ER-negative breast tumor samples (P < 0.05 was considered significant). (E) Increased relative gene expression and copy number variation of CCND1 and YWHAZ, are observed in MDA-MB-468 breast cancer cell lines. (F) Relative mRNA expression of CCND1 and YWHAZ in PGRMC1 silenced MDA-MB-468 cells. *P < 0.05.
Discussion
TNBCs account for approximately 12-14% of breast cancers diagnosed in the United States, with most exhibiting BRCA1/2 and p53 germline mutations (62, 63). TNBCs are the most aggressive type of breast cancer and most patients do not respond well to conventional chemotherapy (64, 65). The concept of gene therapy has been brought up as an alternative to chemotherapy to treat these aggressive cancers (66, 67) in this case RNAi could be used to target mutated proteins which are a product of missense mutations, leading to high constitutive expression of mutated proteins such as TP53 (68). However, suppressing genes with RNAi requires effective delivery methods, which have proven to be effective in some cases but difficult in both in vivo and in vitro systems (69–71). Therefore, other means of gene targeting therapies could be valued options.
miRNAs have emerged as important biological regulators of normal development (72) and evidence suggest that they play a major role in human cancers (73). miRNAs are abundantly found in multiple human cells and have the ability to regulate gene expression of approximately 60% of all mammalian genes (74, 75) hence they promote themselves as an attractive therapeutic option. Several miRNAs have been shown to be altered in TNBCs (24–28). Two examples of this are through the activation of STAT3, a transcription factor that is well documented in cancers (76). Activation of STAT3 is observed in TNBC tumors where epigenetic suppression of miR-146b leads to constitutive STAT3 activation and tumor growth (77, 78). Secondly, through the activation of the miRNA-200 family, these miRNAs are known to negatively regulate the epithelial to mesenchymal transition (EMT) and can specifically target ZEB1/2 (79, 80). Thereby, leading to the question, if miRNAs such as miR-14b or the miR-200 family of miRNAs were to be up-regulated could they then target genes that are overexpressed or active like STAT3 and EMT inducers to inhibit tumor growth?
PGRMC1 has been deemed a novel tumor biomarker due to its elevated levels in human cancers (49, 81–84). Because PGRMC1 plays a role in chemoresistance, tumor progression and growth it has become an attractive therapeutic target (36). Intriguingly, PGRMC1 is commonly observed in aggressive TNBC tissue (35). This is particularly interesting because TNBCs lack the classical signaling hormone receptors, ER and PR yet TNBCs that overexpress PGRMC1 could respond to steroid hormones via PGRMC1. Our previous studies showed that PGRMC1 is clearly overexpressed in the TNBC cell line MDA-MB-468 and using a known inhibitor (AG-205) and PGRMC1 silencing we demonstrated that it promotes TNBC cell proliferation through the EGFR/PI3K/AKT pathway (33). However, our study also focused on signaling pathways associated with ER-positive breast cancers (33). Here, we mainly focused on TNBCs as alternative mechanisms regulated by PGRMC1 in TNBCs should be further explored. To study and uncover novel mechanisms behind PGRMC1 we performed miRNome profiling following AG-205 treatment and PGRMC1 silencing. Studying the human miRNome enabled us to identify miRNAs that were significantly altered following PGRMC1 signal disruption and silencing. This presents itself as an important way to identify signaling pathways and genes involved within these pathways that could be associated with PGRMC1.
Human miRNome profiling identified alteration of 1,008 miRNAs following AG-205 treatment and 776 miRNAs after PGRMC1 siRNA transfection. Using a variety of gene mining platforms (miRNet, xenabrowser, cbioportal, Reactome, Kaplan-Meier plotter and GeneMANIA) we identified miRNA-mRNA network hubs that are altered when PGRMC1 is impaired. Network analysis by miRNet, an all in one, high-performance, analytics tool was used to predict PGRMC1 altered miRNAs targets (85). miRNet, incorporates data from TarBase, miRTarBase, starBase, EpimiR, PharmacomiR, SM2miR, PhenomiR, HMDD, miR2Disease, miRanda and miRecords making it a reliable data mining source (86). The top 10 most upregulated and downregulated miRNAs following AG-205 treatment and PGRMC1 silencing were identified. KEGG pathway analysis identified matching enriched pathways between the two treatment groups which included, pathways in cancer, cell cycle and p53 signaling pathway. In addition, TCGA derived gene expression data analysis taken from metastatic tissue identified the 22 most overexpressed genes in response to PGRMC1 signaling inhibition and silencing. Based on the above data, miRNAs that were upregulated following PGRMC1 impairment directly target and have the capability to suppress genes that are overexpressed in TNBC patient samples. However, because of their function we proceeded to study the downregulated miRNAs but considered them to be possible biomarkers. Interestingly, miR-30b, miR-664a-3p and miR-93-3p, miR-224-5p all which were downregulated following PGRMC1 impairment are commonly observed in multiple cancers including ovarian (87), prostate (88), gastric (89) and metastatic breast cancer (90–92). Furthermore, miR-181a-3p, miR-224-5p, miR-345-5p and miR-93-3p act like oncogenes and all have been associated with chemoresistance, migration, metastasis and stemness (87, 88, 91, 93). Based on the available literature disrupting PGRMC1 downregulates miRNAs that display oncogenic potential.
To get a better understanding of the signaling mechanism involved within the upregulated miRNA target genes we employed the Reactome pathway analyzer. This enabled us to study different signaling pathways that are not associated with the KEGG analysis from the miRNet database. We observed the upregulated genes to be involved in cell cycle and signal transduction mechanisms. This agrees with our previous findings of cell cycle involvement; interestingly upregulated genes involved in signal transduction mechanisms could be directly regulated by PGRMC1, as signal transduction mechanisms are known to be directly involved in cellular membranes where PGRMC1 is primarily located (94). To further study the clinical impact of these genes, we studied genetic alterations using OncoPrint. It was particularly interesting to see that only 10 genes displayed significant genetic alteration among the 22 genes that were overexpressed. However, of the ten genes the top two most genetically altered, CCND1 and YWHAZ seemed to be overexpressed due to amplification and had overall lower survival probability. CCND1 has long been considered an oncogene and has been demonstrated to be amplified in 10-20% in one study while in another study CCND1 amplification was seen in 78.6% of breast cancer cases (95–97). CCND1 is thought to play a major role in ER-positive but not in ER-negative breast cancers (98). One of the reasons could be because it is a known downstream target of PR that can promote breast cancer cell proliferation (99, 100). One interesting thought could be that in TNBCs that overexpress PGRMC1, it could be enhancing the transcription of CCND1 even in tumors that lack ER and PR making it a potential target in TNBCs. The YWHAZ gene has been described in multiple cancers including non-small lung cancer (101), hepatocellular carcinoma (102), gastric cancer (103), bladder cancer (104), and in breast cancers (105). Overexpression of YWHAZ in breast cancers has been associated with chemoresistance to anthracyclines particularly associated with metastatic recurrence (105). This is also extremely interesting as PGRMC1 has been linked to chemoresistance (106) and it would be strongly warranted to further explore the possibility of a PGRMC1/YWHAZ axis in metastatic breast cancers that do not respond to chemotherapy.
Conclusion
In summary, our study identified that impairing PGRMC1 can alter miRNAs, specifically hsa-mir-646 that directly targets CCND1 (107) as well as hsa-mir-410-3p and hsa-mir-3150b-3p which target YWHAZ (108–113). Interestingly, both genes were amplified in patients with aggressive TNBCs and patients that express high levels of either gene have lower overall survival probability. Lastly, PGRMC1 impairment downregulates oncogenic miRNAs (miR-30b, miR-664a-3p and miR-93-3p, miR-224-5p, miR-181a-3p and miR-345-5p) in TNBC cells. Therefore, targeting PGRMC1 with AG-205 or a novel compound that can downregulate PGRMC1 expression could be potential therapeutic options for TNBC patients that overexpress PGRMC1.
Data Availability Statement
The original contributions presented in the study are included in the article/Supplementary Material. Further inquiries can be directed to the corresponding author.
Author Contributions
Conception and design: RL and DP. Methodology was developed by DP and VR. Data acquisition: DP, MR, and VR. Data was interpreted by RL, DP, MR, VR, RS, and AE. The manuscript was written and/or revised by DP, MR, RS, VM, TG, and RL. This study was supervised by RL. All authors contributed to the article and approved the submitted version.
Funding
Breast Cancer Discretionary Fund from Texas Tech University Health Sciences Center El Paso.
Conflict of Interest
The authors declare that the research was conducted in the absence of any commercial or financial relationships that could be construed as a potential conflict of interest.
Acknowledgments
We would like to thank Texas Tech University Health Sciences Center El Paso for supporting this research.
Supplementary Material
The Supplementary Material for this article can be found online at: https://www.frontiersin.org/articles/10.3389/fonc.2021.710337/full#supplementary-material
References
1. Siegel RL, Miller KD, Jemal A. Cancer Statistics, 2020. CA Cancer J Clin (2020) 70(1):7–30. doi: 10.3322/caac.21590
2. Prat A, Perou CM. Deconstructing the Molecular Portraits of Breast Cancer. Mol Oncol (2011) 5(1):5–23. doi: 10.1016/j.molonc.2010.11.003
3. Clark SE, Warwick J, Carpenter R, Bowen RL, Duffy SW, Jones JL. Molecular Subtyping of DCIS: Heterogeneity of Breast Cancer Reflected in Pre-Invasive Disease. Br J Cancer (2011) 104(1):120–7. doi: 10.1038/sj.bjc.6606021
4. McGuire A, Lowery AJ, Kell MR, Kerin MJ, Sweeney KJ. Locoregional Recurrence Following Breast Cancer Surgery in the Trastuzumab Era: A Systematic Review by Subtype. Ann Surg Oncol (2017) 24(11):3124–32. doi: 10.1245/s10434-017-6021-1
5. Cheang MC, Martin M, Nielsen TO, Prat A, Voduc D, Rodriguez-Lescure A, et al. Defining Breast Cancer Intrinsic Subtypes by Quantitative Receptor Expression. Oncologist (2015) 20(5):474–82. doi: 10.1634/theoncologist.2014-0372
6. Jhan JR, Andrechek ER. Triple-Negative Breast Cancer and the Potential for Targeted Therapy. Pharmacogenomics (2017) 18(17):1595–609. doi: 10.2217/pgs-2017-0117
7. Gradishar WJ, Anderson BO, Abraham J, Aft R, Agnese D, Allison KH, et al. Breast Cancer, Version 3.2020, NCCN Clinical Practice Guidelines in Oncology. J Natl Compr Canc Netw (2020) 18(4):452–78. doi: 10.6004/jnccn.2020.0016
8. Li X, Yang J, Peng L, Sahin AA, Huo L, Ward KC, et al. Triple-Negative Breast Cancer has Worse Overall Survival and Cause-Specific Survival Than non-Triple-Negative Breast Cancer. Breast Cancer Res Treat (2017) 161(2):279–87. doi: 10.1007/s10549-016-4059-6
9. Hasegawa S, Eguchi H, Nagano H, Konno M, Tomimaru Y, Wada H, et al. MicroRNA-1246 Expression Associated With CCNG2-mediated Chemoresistance and Stemness in Pancreatic Cancer. Br J Cancer (2014) 111(8):1572–80. doi: 10.1038/bjc.2014.454
10. Schreiber R, Mezencev R, Matyunina LV, McDonald JF. Evidence for the Role of microRNA 374b in Acquired Cisplatin Resistance in Pancreatic Cancer Cells. Cancer Gene Ther (2016) 23:241–5. doi: 10.1038/cgt.2016.23
11. Asakura K, Kadota T, Matsuzaki J, Yoshida Y, Yamamoto Y, Nakagawa K, et al. A miRNA-based Diagnostic Model Predicts Resectable Lung Cancer in Humans With High Accuracy. Commun Biol (2020) 3:134. doi: 10.1038/s42003-020-0863-y
12. Yokoi A, Matsuzaki J, Yamamoto Y, Yoneoka Y, Takahashi K, Shimizu H, et al. Integrated Extracellular microRNA Profiling for Ovarian Cancer Screening. Nat Commun (2018) 9:4319. doi: 10.1038/s41467-018-06434-4
13. Le Rhun E, Seoane J, Salzet M, Soffietti R, Weller M. Liquid Biopsies for Diagnosing and Monitoring Primary Tumors of the Central Nervous System. Cancer Lett (2020) 480:24–8. doi: 10.1016/j.canlet.2020.03.021
14. Nandy SB, Arumugam A, Subramani R, Pedroza D, Hernandez K, Saltzstein E, et al. MicroRNA-125a Influences Breast Cancer Stem Cells by Targeting Leukemia Inhibitory Factor Receptor Which Regulates the Hippo Signaling Pathway. Oncotarget (2015) 6:17366–78. doi: 10.18632/oncotarget.3953
15. Nandy SB, Orozco A, Lopez-Valdez R, Roberts R, Subramani R, Arumugam A, et al. Glucose Insult Elicits Hyperactivation of Cancer Stem Cells Through miR-424-cdc42-prdm14 Signalling Axis. Br J Cancer (2017) 117(11):1665–75. doi: 10.1038/bjc.2017.335
16. Sherafatian M. Tree-Based Machine Learning Algorithms Identified Minimal Set of miRNA Biomarkers for Breast Cancer Diagnosis and Molecular Subtyping. Gene (2018) 677:111–8. doi: 10.1016/j.gene.2018.07.057
17. Subramani R, Gangwani L, Nandy SB, Arumugam A, Chattopadhyay M, Lakshmanaswamy R. Emerging Roles of microRNAs in Pancreatic Cancer Diagnosis, Therapy and Prognosis (Review). Int J Oncol (2015) 47(4):1203–10. doi: 10.3892/ijo.2015.3129
18. Ivanov Y, Pleshakova T, Malsagova K, Kurbatov L, Popov V, Glukhov A, et al. Detection of Marker Mirnas, Associated With Prostate Cancer, in Plasma Using SOI-NW Biosensor in Direct and Inversion Modes. Sensors (Basel) (2019) 19(23). doi: 10.3390/s19235248
19. Blenkiron C, Miska EA. miRNAs in Cancer: Approaches, Aetiology, Diagnostics and Therapy. Hum Mol Genet (2007) 16 Spec No 1:R106–13. doi: 10.1093/hmg/ddm056
20. Piva R, Spandidos DA, Gambari R. From microRNA Functions to microRNA Therapeutics: Novel Targets and Novel Drugs in Breast Cancer Research and Treatment (Review). Int J Oncol (2013) 43:985–94. doi: 10.3892/ijo.2013.2059
21. van Schooneveld E, Wouters MC, Van der Auwera I, Peeters DJ, Wildiers H, Van Dam PA, et al. Expression Profiling of Cancerous and Normal Breast Tissues Identifies microRNAs That are Differentially Expressed in Serum From Patients With (Metastatic) Breast Cancer and Healthy Volunteers. Breast Cancer Res (2012) 14(1):R34. doi: 10.1186/bcr3127
22. Van der Auwera I, Yu W, Suo L, Van Neste L, van Dam P, Van Marck EA, et al. Array-Based DNA Methylation Profiling for Breast Cancer Subtype Discrimination. PLoS One (2010) 5(9):e12616. doi: 10.1371/journal.pone.0012616
23. van Schooneveld E, Wildiers H, Vergote I, Vermeulen PB, Dirix LY, Van Laere SJ. Dysregulation of microRNAs in Breast Cancer and Their Potential Role as Prognostic and Predictive Biomarkers in Patient Management. Breast Cancer Res (2015) 17:21. doi: 10.1186/s13058-015-0526-y
24. Piasecka D, Braun M, Kordek R, Sadej R, Romanska H. MicroRNAs in Regulation of Triple-Negative Breast Cancer Progression. J Cancer Res Clin Oncol (2018) 144(8):1401–11. doi: 10.1007/s00432-018-2689-2
25. Chang YY, Kuo WH, Hung JH, Lee CY, Lee YH, Chang YC, et al. Deregulated microRNAs in Triple-Negative Breast Cancer Revealed by Deep Sequencing. Mol Cancer (2015) 14:36. doi: 10.1186/s12943-015-0301-9
26. Lü L, Mao X, Shi P, He B, Xu K, Zhang S, et al. MicroRNAs in the Prognosis of Triple-Negative Breast Cancer: A Systematic Review and Meta-Analysis. Med (Baltimore) (2017) 96:e7085. doi: 10.1097/MD.0000000000007085
27. Zhu H, Dai M, Chen X, Qin S, Dai S. Integrated Analysis of the Potential Roles of miRNA−mRNA Networks in Triple Negative Breast Cancer. Mol Med Rep (2017) 16:1139–46. doi: 10.3892/mmr.2017.6750
28. Paszek S, Gabło N, Barnaś E, Szybka M, Morawiec J, Kołacińska A, et al. Dysregulation of microRNAs in Triple-Negative Breast Cancer. Ginekol Pol (2017) 88:530–6. doi: 10.5603/GP.a2017.0097
29. Mueck AO, Ruan X, Seeger H, Fehm T, Neubauer H. Genomic and non-Genomic Actions of Progestogens in the Breast. J Steroid Biochem Mol Biol (2014) 142:62–7. doi: 10.1016/j.jsbmb.2013.08.011
30. Wang C, Liu Y, Cao JM. G Protein-Coupled Receptors: Extranuclear Mediators for the non-Genomic Actions of Steroids. Int J Mol Sci (2014) 15:15412–25. doi: 10.3390/ijms150915412
31. Zhang Y, Ruan X, Mi X, Mueck AO. Expression of PGRMC1 in Paraffin-Embedded Tissues of Breast Cancer. Int J Clin Exp Pathol (2017) 10:9639–43.
32. Pedroza DA, Subramani R, Lakshmanaswamy R. Classical and Non-Classical Progesterone Signaling in Breast Cancers. Cancers (Basel) (2020) 12(9). doi: 10.3390/cancers12092440
33. Pedroza DA, Rajamanickam V, Subramani R, Bencomo A, Galvez A, Lakshmanaswamy R. Progesterone Receptor Membrane Component 1 Promotes the Growth of Breast Cancers by Altering the Phosphoproteome and Augmenting EGFR/PI3K/AKT Signalling. Br J Cancer (2020). doi: 10.1038/s41416-020-0992-6
34. Ruan X, Zhang Y, Mueck AO, Willibald M, Seeger H, Fehm T, et al. Increased Expression of Progesterone Receptor Membrane Component 1 is Associated With Aggressive Phenotype and Poor Prognosis in ER-positive and Negative Breast Cancer. Menopause (2017) 24:203–9. doi: 10.1097/GME.0000000000000739
35. Clark NC, Friel AM, Pru CA, Zhang L, Shioda T, Rueda BR, et al. Progesterone Receptor Membrane Component 1 Promotes Survival of Human Breast Cancer Cells and the Growth of Xenograft Tumors. Cancer Biol Ther (2016) 17:262–71. doi: 10.1080/15384047.2016.1139240
36. Rohe HJ, Ahmed IS, Twist KE, Craven RJ. PGRMC1 (Progesterone Receptor Membrane Component 1): A Targetable Protein With Multiple Functions in Steroid Signaling, P450 Activation and Drug Binding. Pharmacol Ther (2009) 121:14–9. doi: 10.1016/j.pharmthera.2008.09.006
37. Cahill MA, Jazayeri JA, Catalano SM, Toyokuni S, Kovacevic Z, Richardson DR. The Emerging Role of Progesterone Receptor Membrane Component 1 (PGRMC1) in Cancer Biology. Biochim Biophys Acta (2016) 1866:339–49. doi: 10.1016/j.bbcan.2016.07.004
38. Ahmed IS, Rohe HJ, Twist KE, Mattingly MN, Craven RJ. Progesterone Receptor Membrane Component 1 (Pgrmc1): A Heme-1 Domain Protein That Promotes Tumorigenesis and is Inhibited by a Small Molecule. J Pharmacol Exp Ther (2010) 333:564–73. doi: 10.1124/jpet.109.164210
39. Willibald M, Wurster I, Meisner C, Vogel U, Seeger H, Mueck AO, et al. High Level of Progesteron Receptor Membrane Component 1 (PGRMC 1) in Tissue of Breast Cancer Patients is Associated With Worse Response to Anthracycline-Based Neoadjuvant Therapy. Horm Metab Res (2017) 49:595–603. doi: 10.1055/s-0043-113635
40. Shih CC, Chou HC, Chen YJ, Kuo WH, Chan CH, Lin YC, et al. Role of PGRMC1 in Cell Physiology of Cervical Cancer. Life Sci (2019) 231:116541. doi: 10.1016/j.lfs.2019.06.016
41. Network, C. G. A. Comprehensive Molecular Portraits of Human Breast Tumours. Nature (2012) 490:61–70. doi: 10.1038/nature11412
42. Stephens PJ, Tarpey PS, Davies H, Van Loo P, Greenman C, Wedge DC, et al. The Landscape of Cancer Genes and Mutational Processes in Breast Cancer. Nature (2012) 486:400–4. doi: 10.1038/nature11017
43. Kawazu M, Kojima S, Ueno T, Totoki Y, Nakamura H, Kunita A, et al. Integrative Analysis of Genomic Alterations in Triple-Negative Breast Cancer in Association With Homologous Recombination Deficiency. PLoS Genet (2017) 13:e1006853. doi: 10.1371/journal.pgen.1006853
44. Rice JC, Ozcelik H, Maxeiner P, Andrulis I, Futscher BW. Methylation of the BRCA1 Promoter is Associated With Decreased BRCA1 mRNA Levels in Clinical Breast Cancer Specimens. Carcinogenesis (2000) 21:1761–5. doi: 10.1093/carcin/21.9.1761
45. Bareche Y, Venet D, Ignatiadis M, Aftimos P, Piccart M, Rothe F, et al. Unravelling Triple-Negative Breast Cancer Molecular Heterogeneity Using an Integrative Multiomic Analysis. Ann Oncol (2018) 29:895–902. doi: 10.1093/annonc/mdy024
46. Harbeck N, Penault-Llorca F, Cortes J, Gnant M, Houssami N, Poortmans P, et al. Breast Cancer. Nat Rev Dis Primers (2019) 5:66. doi: 10.1038/s41572-019-0111-2
47. Zhu X, Han Y, Fang Z, Wu W, Ji M, Teng F, et al. Progesterone Protects Ovarian Cancer Cells From Cisplatin-Induced Inhibitory Effects Through Progesterone Receptor Membrane Component 1/2 as Well as AKT Signaling. Oncol Rep (2013) 30:2488–94. doi: 10.3892/or.2013.2680
48. Liu L, Wang J, Zhao L, Nilsen J, McClure K, Wong K, et al. Progesterone Increases Rat Neural Progenitor Cell Cycle Gene Expression and Proliferation Via Extracellularly Regulated Kinase and Progesterone Receptor Membrane Components 1 and 2. Endocrinology (2009) 150:3186–96. doi: 10.1210/en.2008-1447
49. Neubauer H, Clare SE, Wozny W, Schwall GP, Poznanovic S, Stegmann W, et al. Breast Cancer Proteomics Reveals Correlation Between Estrogen Receptor Status and Differential Phosphorylation of PGRMC1. Breast Cancer Res (2008) 10:R85. doi: 10.1186/bcr2155
50. Polivka J, Janku F. Molecular Targets for Cancer Therapy in the PI3K/AKT/mTOR Pathway. Pharmacol Ther (2014) 142:164–75. doi: 10.1016/j.pharmthera.2013.12.004
51. Engelman JA. Targeting PI3K Signalling in Cancer: Opportunities, Challenges and Limitations. Nat Rev Cancer (2009) 9:550–62. doi: 10.1038/nrc2664
52. Liu P, Cheng H, Roberts TM, Zhao JJ. Targeting the Phosphoinositide 3-Kinase Pathway in Cancer. Nat Rev Drug Discov (2009) 8:627–44. doi: 10.1038/nrd2926
53. Fruman DA, Rommel C. PI3K and Cancer: Lessons, Challenges and Opportunities. Nat Rev Drug Discov (2014) 13:140–56. doi: 10.1038/nrd4204
54. Thorpe LM, Yuzugullu H, Zhao JJ. PI3K in Cancer: Divergent Roles of Isoforms, Modes of Activation and Therapeutic Targeting. Nat Rev Cancer (2015) 15:7–24. doi: 10.1038/nrc3860
55. Janku F, Yap TA, Meric-Bernstam F. Targeting the PI3K Pathway in Cancer: Are We Making Headway? Nat Rev Clin Oncol (2018) 15:273–91. doi: 10.1038/nrclinonc.2018.28
56. Shapiro GI, LoRusso P, Kwak E, Pandya S, Rudin CM, Kurkjian C, et al. Phase Ib Study of the MEK Inhibitor Cobimetinib (GDC-0973) in Combination With the PI3K Inhibitor Pictilisib (GDC-0941) in Patients With Advanced Solid Tumors. Invest New Drugs (2020) 38:419–32. doi: 10.1007/s10637-019-00776-6
57. Basho RK, Gilcrease M, Murthy RK, Helgason T, Karp DD, Meric-Bernstam F, et al. Targeting the PI3K/AKT/mTOR Pathway for the Treatment of Mesenchymal Triple-Negative Breast Cancer: Evidence From a Phase 1 Trial of Mtor Inhibition in Combination With Liposomal Doxorubicin and Bevacizumab. JAMA Oncol (2017) 3:509–15. doi: 10.1001/jamaoncol.2016.5281
58. Jassal B, Matthews L, Viteri G, Gong C, Lorente P, Fabregat A, et al. The Reactome Pathway Knowledgebase. Nucleic Acids Res (2020) 48:D498–503. doi: 10.1093/nar/gkz1031
59. Milacic M, Haw R, Rothfels K, Wu G, Croft D, Hermjakob H, et al. Annotating Cancer Variants and Anti-Cancer Therapeutics in Reactome. Cancers (Basel) (2012) 4:1180–211. doi: 10.3390/cancers4041180
60. Ciriello G, Gatza ML, Beck AH, Wilkerson MD, Rhie SK, Pastore A, et al. Comprehensive Molecular Portraits of Invasive Lobular Breast Cancer. Cell (2015) 163:506–19. doi: 10.1016/j.cell.2015.09.033
61. Fabregat A, Sidiropoulos K, Viteri G, Forner O, Marin–Garcia P, Arnau V, et al. Reactome Pathway Analysis: A High-Performance in-Memory Approach. BMC Bioinf (2017) 18:142. doi: 10.1186/s12859-017-1559-2
62. Hwang SY, Park S, Kwon Y. Recent Therapeutic Trends and Promising Targets in Triple Negative Breast Cancer. Pharmacol Ther (2019) 199:30–57. doi: 10.1016/j.pharmthera.2019.02.006
63. Shah SP, Roth A, Goya R, Oloumi A, Ha G, Zhao Y, et al. The Clonal and Mutational Evolution Spectrum of Primary Triple-Negative Breast Cancers. Nature (2012) 486:395–9. doi: 10.1038/nature10933
64. Dent R, Trudeau M, Pritchard KI, Hanna WM, Kahn HK, Sawka CA, et al. Triple-Negative Breast Cancer: Clinical Features and Patterns of Recurrence. Clin Cancer Res (2007) 13:4429–34. doi: 10.1158/1078-0432.CCR-06-3045
65. de Ruijter TC, Veeck J, de Hoon JP, van Engeland M, Tjan-Heijnen VC. Characteristics of Triple-Negative Breast Cancer. J Cancer Res Clin Oncol (2011) 137:183–92. doi: 10.1007/s00432-010-0957-x
66. O’Toole SA, Beith JM, Millar EK, West R, McLean A, Cazet A, et al. Therapeutic Targets in Triple Negative Breast Cancer. J Clin Pathol (2013) 66:530–42. doi: 10.1136/jclinpath-2012-201361
67. Turner N, Moretti E, Siclari O, Migliaccio I, Santarpia L, D'Incalci M, et al. Targeting Triple Negative Breast Cancer: Is p53 the Answer? Cancer Treat Rev (2013) 39:541–50. doi: 10.1016/j.ctrv.2012.12.001
68. Coradini D, Biganzoli E, Ardoino I, Ambrogi F, Boracchi P, Demicheli R, et al. p53 Status Identifies Triple-Negative Breast Cancer Patients Who do Not Respond to Adjuvant Chemotherapy. Breast (2015) 24:294–7. doi: 10.1016/j.breast.2015.01.007
69. Davis ME, Zuckerman JE, Choi CH, Seligson D, Tolcher A, Alabi CA, et al. Evidence of RNAi in Humans From Systemically Administered siRNA Via Targeted Nanoparticles. Nature (2010) 464:1067–70. doi: 10.1038/nature08956
70. Lares MR, Rossi JJ, Ouellet DL. Rnai and Small Interfering RNAs in Human Disease Therapeutic Applications. Trends Biotechnol (2010) 28:570–9. doi: 10.1016/j.tibtech.2010.07.009
71. Hu B, Zhong L, Weng Y, Peng L, Huang Y, Zhao Y, et al. Therapeutic siRNA: State of the Art. Signal Transduct Target Ther (2020) 5:101. doi: 10.1038/s41392-020-0207-x
72. Ivey KN, Srivastava D. microRNAs as Developmental Regulators. Cold Spring Harb Perspect Biol (2015) 7:a008144. doi: 10.1101/cshperspect.a008144
73. Peng Y, Croce CM. The Role of MicroRNAs in Human Cancer. Signal Transduct Target Ther (2016) 1:15004. doi: 10.1038/sigtrans.2015.4
74. Friedman RC, Farh KK, Burge CB, Bartel DP. Most Mammalian mRNAs are Conserved Targets of Micrornas. Genome Res (2009) 19:92–105. doi: 10.1101/gr.082701.108
75. Lim LP, Lau NC, Weinstein EG, Abdelhakim A, Yekta S, Rhoades MW, et al. The microRNAs of Caenorhabditis Elegans. Genes Dev (2003) 17:991–1008. doi: 10.1101/gad.1074403
76. Yu H, Lee H, Herrmann A, Buettner R, Jove R. Revisiting STAT3 Signalling in Cancer: New and Unexpected Biological Functions. Nat Rev Cancer (2014) 14:736–46. doi: 10.1038/nrc3818
77. Xiang M, Birkbak NJ, Vafaizadeh V , Walker SR, Yeh JE, Liu S, et al. STAT3 Induction of miR-146b Forms a Feedback Loop to Inhibit the NF-κb to IL-6 Signaling Axis and STAT3-driven Cancer Phenotypes. Sci Signal (2014) 7:ra11. doi: 10.1126/scisignal.2004497
78. Paliouras AR, Monteverde T, Garofalo M. Oncogene-Induced Regulation of microRNA Expression: Implications for Cancer Initiation, Progression and Therapy. Cancer Lett (2018) 421:152–60. doi: 10.1016/j.canlet.2018.02.029
79. Korpal M, Lee ES, Hu G, Kang Y. The miR-200 Family Inhibits Epithelial-Mesenchymal Transition and Cancer Cell Migration by Direct Targeting of E-cadherin Transcriptional Repressors ZEB1 and ZEB2. J Biol Chem (2008) 283:14910–4. doi: 10.1074/jbc.C800074200
80. Wang J, Tsouko E, Jonsson P, Bergh J, Hartman J, Aydogdu E, et al. miR-206 Inhibits Cell Migration Through Direct Targeting of the Actin-Binding Protein Coronin 1C in Triple-Negative Breast Cancer. Mol Oncol (2014) 8:1690–702. doi: 10.1016/j.molonc.2014.07.006
81. Zhang D, Xia X, Wang X, Zhang P, Lu W, Yu Y, et al. PGRMC1 Is a Novel Potential Tumor Biomarker of Human Renal Cell Carcinoma Based on Quantitative Proteomic and Integrative Biological Assessments. PLoS One (2017) 12:e0170453. doi: 10.1371/journal.pone.0170453
82. Craven RJ. PGRMC1: A New Biomarker for the Estrogen Receptor in Breast Cancer. Breast Cancer Res (2008) 10(6):113. doi: 10.1186/bcr2191
83. Peluso JJ, Liu X, Saunders MM, Claffey KP, Phoenix K. Regulation of Ovarian Cancer Cell Viability and Sensitivity to Cisplatin by Progesterone Receptor Membrane Component-1. J Clin Endocrinol Metab (2008) 93(5):1592–9. doi: 10.1210/jc.2007-2771
84. Mir SU, Ahmed IS, Arnold S, Craven RJ. Elevated Progesterone Receptor Membrane Component 1/Sigma-2 Receptor Levels in Lung Tumors and Plasma From Lung Cancer Patients. Int J Cancer (2012) 131(2):E1–9. doi: 10.1002/ijc.26432
85. Fan Y, Xia J. Mirnet-Functional Analysis and Visual Exploration of Mirna-Target Interactions in a Network Context. Methods Mol Biol (2018) 1819:215–33. doi: 10.1007/978-1-4939-8618-7_10
86. Fan Y, Siklenka K, Arora SK, Ribeiro P, Kimmins S, Xia J. miRNet - Dissecting miRNA-target Interactions and Functional Associations Through Network-Based Visual Analysis. Nucleic Acids Res (2016) 44(W1):W135–41. doi: 10.1093/nar/gkw288
87. Zhao H, Bi T, Qu Z, Jiang J, Cui S, Wang Y. Expression of miR-224-5p is Associated With the Original Cisplatin Resistance of Ovarian Papillary Serous Carcinoma. Oncol Rep (2014) 32(3):1003–12. doi: 10.3892/or.2014.3311
88. Tinay I, Tan M, Gui B, Werner L, Kibel AS, Jia L. Functional Roles and Potential Clinical Application of miRNA-345-5p in Prostate Cancer. Prostate (2018) 78(12):927–37. doi: 10.1002/pros.23650
89. Wang L, Li B, Zhang L, Li Q, He Z, Zhang X, et al. miR-664a-3p Functions as an Oncogene by Targeting Hippo Pathway in the Development of Gastric Cancer. Cell Prolif (2019) 52(3):e12567. doi: 10.1111/cpr.12567
90. Wu L, Li Y, Li J, Ma D. Microrna-664 Targets Insulin Receptor Substrate 1 to Suppress Cell Proliferation and Invasion in Breast Cancer. Oncol Res (2019) 27(4):459–67. doi: 10.3727/096504018X15193500663936
91. Li HY, Liang JL, Kuo YL, Lee HH, Calkins MJ, Chang HT, et al. miR-105/93-3p Promotes Chemoresistance and Circulating miR-105/93-3p Acts as a Diagnostic Biomarker for Triple Negative Breast Cancer. Breast Cancer Res (2017) 19(1):133. doi: 10.1186/s13058-017-0918-2
92. Estevão-Pereira H, Lobo J, Salta S, Amorim M, Lopes P, Cantante M, et al. Overexpression of Circulating MiR-30b-5p Identifies Advanced Breast Cancer. J Transl Med (2019) 17(1):435. doi: 10.1186/s12967-019-02193-y
93. Lu Q, Chen Y, Sun D, Wang S, Ding K, Liu M, et al. Microrna-181a Functions as an Oncogene in Gastric Cancer by Targeting Caprin-1. Front Pharmacol (2018) 9:1565. doi: 10.3389/fphar.2018.01565
94. Groves JT, Kuriyan J. Molecular Mechanisms in Signal Transduction at the Membrane. Nat Struct Mol Biol (2010) 17(6):659–65. doi: 10.1038/nsmb.1844
95. Gillett C, Fantl V, Smith R, Fisher C, Bartek J, Dickson C, et al. Amplification and Overexpression of Cyclin D1 in Breast Cancer Detected by Immunohistochemical Staining. Cancer Res (1994) 54(7):1812–7.
96. Zhang SY, Caamano J, Cooper F, Guo X, Klein-Szanto AJ. Immunohistochemistry of Cyclin D1 in Human Breast Cancer. Am J Clin Pathol (1994) 102(5):695–8. doi: 10.1093/ajcp/102.5.695
97. Mohammadizadeh F, Hani M, Ranaee M, Bagheri M. Role of Cyclin D1 in Breast Carcinoma. J Res Med Sci (2013) 18(12):1021–5.
98. Ahlin C, Lundgren C, Embretén-Varro E, Jirström K, Blomqvist C, Fjällskog M. High Expression of Cyclin D1 is Associated to High Proliferation Rate and Increased Risk of Mortality in Women With ER-positive But Not in ER-negative Breast Cancers. Breast Cancer Res Treat (2017) 164(3):667–78. doi: 10.1007/s10549-017-4294-5
99. Giulianelli S, Vaqué JP, Soldati R, Wargon V, Vanzulli SI, Martins R, et al. Estrogen Receptor Alpha Mediates Progestin-Induced Mammary Tumor Growth by Interacting With Progesterone Receptors at the Cyclin D1/MYC Promoters. Cancer Res (2012) 72(9):2416–27. doi: 10.1158/0008-5472.CAN-11-3290
100. Diep CH, Ahrendt H, Lange CA. Progesterone Induces Progesterone Receptor Gene (PGR) Expression Via Rapid Activation of Protein Kinase Pathways Required for Cooperative Estrogen Receptor Alpha (ER) and Progesterone Receptor (PR) Genomic Action at ER/PR Target Genes. Steroids (2016) 114:48–58. doi: 10.1016/j.steroids.2016.09.004
101. Deng Y, Zheng J, Ma J. The Clinical and Prognostic Significance of YWHAZ in non-Small-Cell Lung Cancer Patients: Immunohistochemical Analysis. J Cell Biochem (2019) 120(4):6290–8. doi: 10.1002/jcb.27915
102. Zhao JF, Zhao Q, Hu H, Liao JZ, Lin JS, Xia C, et al. The ASH1-miR-375-YWHAZ Signaling Axis Regulates Tumor Properties in Hepatocellular Carcinoma. Mol Ther Nucleic Acids (2018) 11:538–53. doi: 10.1016/j.omtn.2018.04.007
103. Guo F, Jiao D, Sui GQ, Sun LN, Gao YJ, Fu QF, et al. Anticancer Effect of YWHAZ Silencing Via Inducing Apoptosis and Autophagy in Gastric Cancer Cells. Neoplasma (2018) 65(5):693–700. doi: 10.4149/neo_2018_170922N603
104. Liu S, Jiang H, Wen H, Ding Q, Feng C. Knockdown of Tyrosine 3-Monooxygenase/Tryptophan 5-Monooxygenase Activation Protein Zeta (YWHAZ) Enhances Tumorigenesis Both In Vivo and In Vitro in Bladder Cancer. Oncol Rep (2018) 39(5):2127–35. doi: 10.3892/or.2018.6294
105. Li Y, Zou L, Li Q, Haibe-Kains B, Tian R, Desmedt C, et al. Amplification of LAPTM4B and YWHAZ Contributes to Chemotherapy Resistance and Recurrence of Breast Cancer. Nat Med (2010) 16(2):214–8. doi: 10.1038/nm.2090
106. Kabe Y, Nakane T, Koike I, Yamamoto T, Sugiura Y, Harada E, et al. Haem-Dependent Dimerization of PGRMC1/Sigma-2 Receptor Facilitates Cancer Proliferation and Chemoresistance. Nat Commun (2016) 7:11030. doi: 10.1038/ncomms11030
107. Farazi TA, Ten Hoeve JJ, Brown M, Mihailovic A, Horlings HM, van de Vijver MJ, et al. Identification of Distinct miRNA Target Regulation Between Breast Cancer Molecular Subtypes Using AGO2-PAR-CLIP and Patient Datasets. Genome Biol (2014) 15(1):R9. doi: 10.1186/gb-2014-15-1-r9
108. Memczak S, Jens M, Elefsinioti A, Torti F, Krueger J, Rybak A, et al. Circular RNAs are a Large Class of Animal RNAs With Regulatory Potency. Nature (2013) 495:333–8. doi: 10.1038/nature11928
109. Kishore S, Jaskiewicz L, Burger L, Hausser J, Khorshid M, Zavolan M. A Quantitative Analysis of CLIP Methods for Identifying Binding Sites of RNA-binding Proteins. Nat Methods (2011) 8(7):559–64. doi: 10.1038/nmeth.1608
110. Hafner M, Landthaler M, Burger L, Khorshid M, Hausser J, Berninger P, et al. Transcriptome-Wide Identification of RNA-binding Protein and microRNA Target Sites by PAR-CLIP. Cell (2010) 141(1):129–41. doi: 10.1016/j.cell.2010.03.009
111. Karginov FV, Hannon GJ. Remodeling of Ago2-mRNA Interactions Upon Cellular Stress Reflects miRNA Complementarity and Correlates With Altered Translation Rates. Genes Dev (2013) 27(14):1624–32. doi: 10.1101/gad.215939.113
112. Xue Y, Ouyang K, Huang J, Zhou Y, Ouyang H, Li H, et al. Direct Conversion of Fibroblasts to Neurons by Reprogramming PTB-regulated microRNA Circuits. Cell (2013) 152(1–2):82–96. doi: 10.1016/j.cell.2012.11.045
Keywords: PGRMC1, miRNA, miRNome, TNBC, KEGG, REACTOME, Gene Ontology
Citation: Pedroza DA, Ramirez M, Rajamanickam V, Subramani R, Margolis V, Gurbuz T, Estrada A and Lakshmanaswamy R (2021) miRNome and Functional Network Analysis of PGRMC1 Regulated miRNA Target Genes Identify Pathways and Biological Functions Associated With Triple Negative Breast Cancer. Front. Oncol. 11:710337. doi: 10.3389/fonc.2021.710337
Received: 15 May 2021; Accepted: 24 June 2021;
Published: 19 July 2021.
Edited by:
Wenwen Zhang, Nanjing Medical University, ChinaReviewed by:
Weifeng Ding, Nantong University, ChinaAmmad Ahmad Farooqi, Institute of Biomedical and Genetic Engineering (IBGE), Pakistan
Copyright © 2021 Pedroza, Ramirez, Rajamanickam, Subramani, Margolis, Gurbuz, Estrada and Lakshmanaswamy. This is an open-access article distributed under the terms of the Creative Commons Attribution License (CC BY). The use, distribution or reproduction in other forums is permitted, provided the original author(s) and the copyright owner(s) are credited and that the original publication in this journal is cited, in accordance with accepted academic practice. No use, distribution or reproduction is permitted which does not comply with these terms.
*Correspondence: Rajkumar Lakshmanaswamy, cmFqa3VtYXIubGFrc2htYW5hc3dhbXlAdHR1aHNjLmVkdQ==