- 1NHC Key Laboratory of Carcinogenesis, Hunan Cancer Hospital and the Affiliated Cancer Hospital of Xiangya School of Medicine, Central South University, Changsha, China
- 2Cancer Research Institute, Basic School of Medicine, Central South University, Changsha, China
- 3Department of Neurology, Yiyang Central Hospital, Yiyang City, China
Mitochondria are vital organelles in cells, regulating energy metabolism and apoptosis. Mitochondrial transcellular transfer plays a crucial role during physiological and pathological conditions, such as rescuing recipient cells from bioenergetic deficit and tumorigenesis. Studies have shown several structures that conduct transcellular transfer of mitochondria, including tunneling nanotubes (TNTs), extracellular vesicles (EVs), and Cx43 gap junctions (GJs). The intra- and intercellular transfer of mitochondria is driven by a transport complex. Mitochondrial Rho small GTPase (MIRO) may be the adaptor that connects the transport complex with mitochondria, and myosin XIX is the motor protein of the transport complex, which participates in the transcellular transport of mitochondria through TNTs. In this review, the roles of TNTs, EVs, GJs, and related transport complexes in mitochondrial transcellular transfer are discussed in detail, as well as the formation mechanisms of TNTs and EVs. This review provides the basis for the development of potential clinical therapies targeting the structures of mitochondrial transcellular transfer.
Introduction
Mitochondria are particularly important intracellular organelles, which not only play an important role in oxidative metabolism, but also have key functions in cell signaling, proliferation, metabolism, and death (1). The core functions of mitochondria in cells has led to increased research attention being paid to the transfer of mitochondria between cells, especially from the aspects of the initiation of stem cell differentiation, reprogramming of differentiated cells, and the recovery of lost mitochondrial function by receiving mitochondria from donor cells (2). Functional mitochondrial transfer between cells was first demonstrated a from mesenchymal stem cells (MSCs) to mammalian cells via tunneling nanotubes (TNTs) in 2006 (3). Transcellular transfer of mitochondria facilitates the incorporation of the donated mitochondria into the endogenous network of recipient cells, which results the change in the bioenergetic profile and other functional properties of recipient cell (4). This process plays an important role in diverse pathological conditions, such as repair of tissue injury, inflammatory regulation, oncogenesis and tumor drug-resistance, as well as in physical conditions maintaining tissue homeostasis (5). Studies have shown that transcellular transfer of mitochondria involves multiple mechanisms, including formation of tunneling nanotubes (TNTs), extracellular vesicles (EVs), gap junctions, exocytosis and endocytosis of naked mitochondria, cytoplasmic fusion, and other metastasis modes (6–8). In addition, transfer of mitochondria can also be used as a treatment for mitochondrial dysfunction diseases, including organ degeneration and cancer (9).
Significantly, the most common donor cells in mitochondrial transcellular transfer are stem cells, such as WJMSCs, BMMSCs and iPSC-MSCs (Table 1), which indicates that this process may play a crucial role in stem cell therapy (5). This type of cellular communication requires the transcellular transfer of mitochondria using all the methods mentioned above, among which tunneling nanotubes (TNTs) are the most common type of long-distance cellular connection. Mitochondria transferred by TNTs can change the metabolic and functional characteristics of the recipient cells, which has been reported in both normal cells and cancer cells, and also plays a key role in drug-resistance (41).
Mitochondria are highly dynamic organelles with constant shape and positional change, which allows the quality control of mitochondria and mitochondrial transport to the region where ATP is needed (42). This movement is based on the cytoskeleton. Generally, the microtubule cytoskeleton is the most common way of intracellular movement, while intercellular movement is based on two forms of the microfilament cytoskeleton, extracellular vesicles (EVs) and TNTs (43). Meanwhile, the transport complex, which anchors mitochondria to the cytoskeletons, provides the power that drives mitochondrial movement.
Physiological Function of the Transcellular Transfer of Mitochondria
Intercellular communication is important for the maintenance of physiological functions and the development pathological processes. Intercellular communication can be divided into: Between cells that are far apart, for which signal molecules, such as cytokines or hormones, can be secreted to bind to specific receptors on target cells to transmit regulatory signals and activate specific cellular activities; and gap junctions (GJs) or synapses used between adjacent cells to transfer small molecules, such as inorganic ions, neurotransmitters, reactive oxygen species (ROS) or small molecular proteins, which allows the exchange of electrical or chemical signals between these two cells (44, 45). In recent years, many new communication forms have been discovered, such as TNTs and EVs, which structures are capable of intercellular exchange of ions, small molecules such as ATP, and certain organelles [like lysosomes and autophagosomes (46)].
Mitochondria are vital cellular organelles, regulating energy metabolism and cell apoptosis, which are directly related to cell survival (47). Recent studies have shown that mitochondria can also undergo intercellular transfer. The transcellular transfer of mitochondria plays different roles in different situations:
A. the quality control of mitochondria. When damage or senescence appears in mitochondria, cells can deal with injured mitochondria through mitochondrial fusion, division, mitophagy or transmitophagy; meanwhile, healthy mitochondria can be received from surrounding cells through TNTs or EVs (42), or damaged mitochondria can be transferred into surrounding cells for degradation (27, 48), thus achieving complete quality control of mitochondria, which aims to maintain the population and physiological function of mitochondria (42).
B. the rescue of cells from oxidative stress. When cells suffer oxidative stress (such as cell ischemia/hypoxia, DNA damage, and inflammatory factor stimulation), specific signals of stress, such as ROS, are activated, leading to the formation of TNTs connecting to adjacent cells or the secretion of EVs, resulting in the transfer of healthy mitochondria to stressed cells, which will correct aerobic respiration and improve the level of oxidative phosphorylation (OXPHOS) and ATP of the stressed cells rapidly (Figure 1) (41).
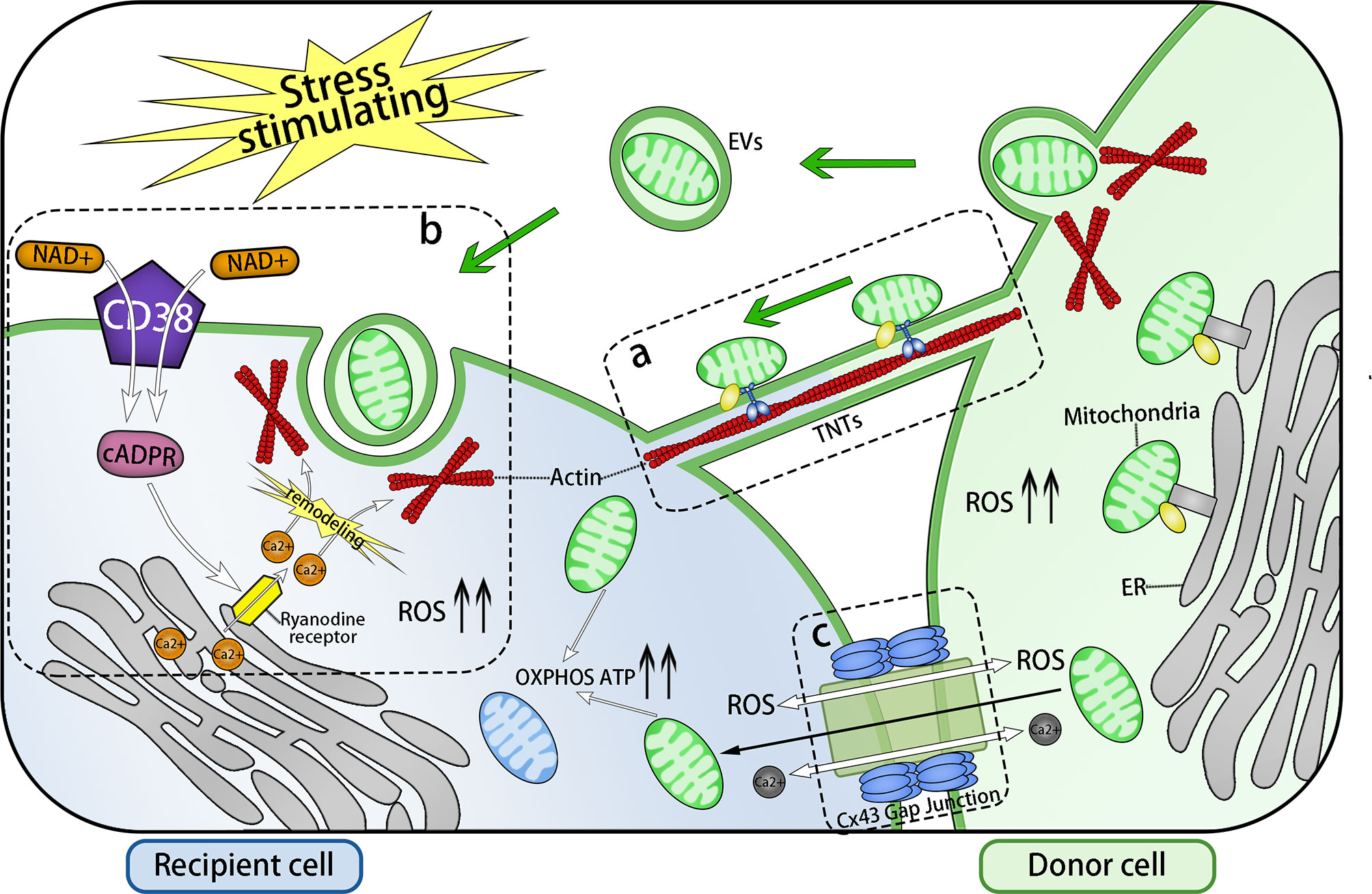
Figure 1 Three Main Forms of Intercellular Communication Related to Transcellular Transfer of Mitochondria. Under the stimulation of energy stress, inflammatory stimulation, or DNA damage, intracellular ROS levels increase and three forms of intercellular communication related to mitochondrial transcellular transfer are formed: TNTs, EVs, and GJs. (A) TNTs connects the cytoplasm of two cells, whose main framework is F-actin. Mitochondria are anchored to the actin skeleton by specific transport complex and driven by them to move from one cell to another via TNTS. (B) EV endocytosis is mediated by the NAD+/CD38/cADPR/Ca2+ pathway: Intracellular NAD+ increases and transfers to the extracellular environment under stress conditions. Then, NAD+ is catalyzed by activated transmembrane protein CD38 to generate cADPR, a second messenger controlling the release of the intracellular Ca2+ pool. cADPR acts on Ryanodine receptors (RyRs) on the endoplasmic reticulum, which leads to an increase in the intracellular Ca2+ concentration, following the remodeling of the actin cytoskeleton and invagination of cell membrane, thereby completing the endocytosis of EVs. The release of EVs and the formation of TNTs might also be mediated by this pathway. Through transcellular transfer of mitochondria, based on the actin cytoskeleton, the OXPHOS, the ATP level, and the viability of the recipient cells are all improved. (C) Cx43 gap junction channel (GJCs) also take part in the transcellular transfer of mitochondria. There are three possible pathways: Ca2+ or ROS exchange via Cx43 GJCs to modulate the formation of channels transferring mitochondria, or the direct transfer of mitochondria. ERMES: a complex that anchors mitochondria to endoplasmic reticulum.
Transcellular transfer of mitochondria is a very common phenomenon in pathological conditions. Bone marrow stromal cells (BMSCs) can transport functional mitochondria to alveolar epithelial cells to counter acute lung injury caused by endotoxins (49). Under the condition of ischemia or hypoxia, astrocytes release mitochondrial granules, which are then are absorbed by neurons via endocytosis to improve the viability of neurons (25, 28). MSCs can infuse mitochondria into a variety of cells, such as vascular smooth muscle cells (20), pulmonary epithelial cells (21, 50), myocardial cells (51), and tumor cells, for example in ovarian cancer and breast cancer (40, 52), which is of great significance for wound healing, immune regulation, maintenance of tissue homeostasis and tumor proliferation (53). Mitochondrial transfer also occurs among monocyte derived macrophages (54). Similarly, tumor cells can induce the transcellular transfer of mitochondria. In multiple myeloma, MSCs deliver mitochondria to myeloma cells through TNTs, promoting their proliferation (55). For pulmonary adenocarcinoma A549 cells, which lose mitochondria during drug treatment, their metabolism can be restored after receiving healthy mitochondria delivered from other cells, making them more invasive (3). In general, transfer of mitochondria can ameliorate aerobic metabolism, restore energy support, and postpone apoptosis of the recipient cells, which acts a protective role in ischemic diseases or other cell dysfunctions; meanwhile, in malignant tumors, it can lead to tumor proliferation and drug resistance. Interestingly, another study seemed to propose the opposite. Normal astrocytes deliver mitochondria to glioma cells, which inhibits their proliferation. In this process, the expression levels of genes related to the tricarboxylic acid cycle were upregulated; aerobic respiration was enhanced, while glycolysis was weakened; and the mitochondrial apoptotic pathway was activated. Meanwhile, the sensitivity of tumors to radiotherapy was increased (28). These changes might be related to the inhibition of the Warburg effect in tumor cells. The following table summarizes some cases of mitochondria transfer between diverse donor and recipient cells (Table 1).
Furthermore, there was also spontaneous transcellular transfer of mitochondria under physical conditions. This phenomenon has been detected under conditions without stimulating factors between mouse cardiomyocytes (CMs) and human multipotent adipose-derived stem cells (hMADs) (16), renal tubular cells (RTCs) and mesenchymal multipotent stromal cells (MMSCs) (56), human vascular smooth muscle cells (hVSMCs) and BMMSCs (20), etc. It plays a potential crucial role in tissue homeostasis and needs further study.
Methods of Transcellular Transfer of Mitochondria
Transfer via TNTs
Tunneling nanotubes (TNTs) are a type of long membrane structure of 100–800 nm in width and 100 μm in length. Based on F-actin as their framework, TNTs are wrapped by a phospholipid bilayer extending from the cell membrane, connecting the cytoplasm of two cells (57). TNTs allow bidirectional and unidirectional substance transport, including a variety of small molecules, proteins, organelles, and even virus particles (58–60). The formation of TNTs has three stages: Formation of a membrane protrusion, elongation of the membrane protrusion, and fusion of the membrane protrusion with the target cell membrane. Transformation of the membrane protrusion is the direct result of actin cytoskeleton remodeling (Figure 2). TNTs exist extensively in a variety of physiological and pathological cells, such as kidney cells (61), PC12 pheochromocytoma cells (62), astrocytes (10), and myocardial cells (17, 63).
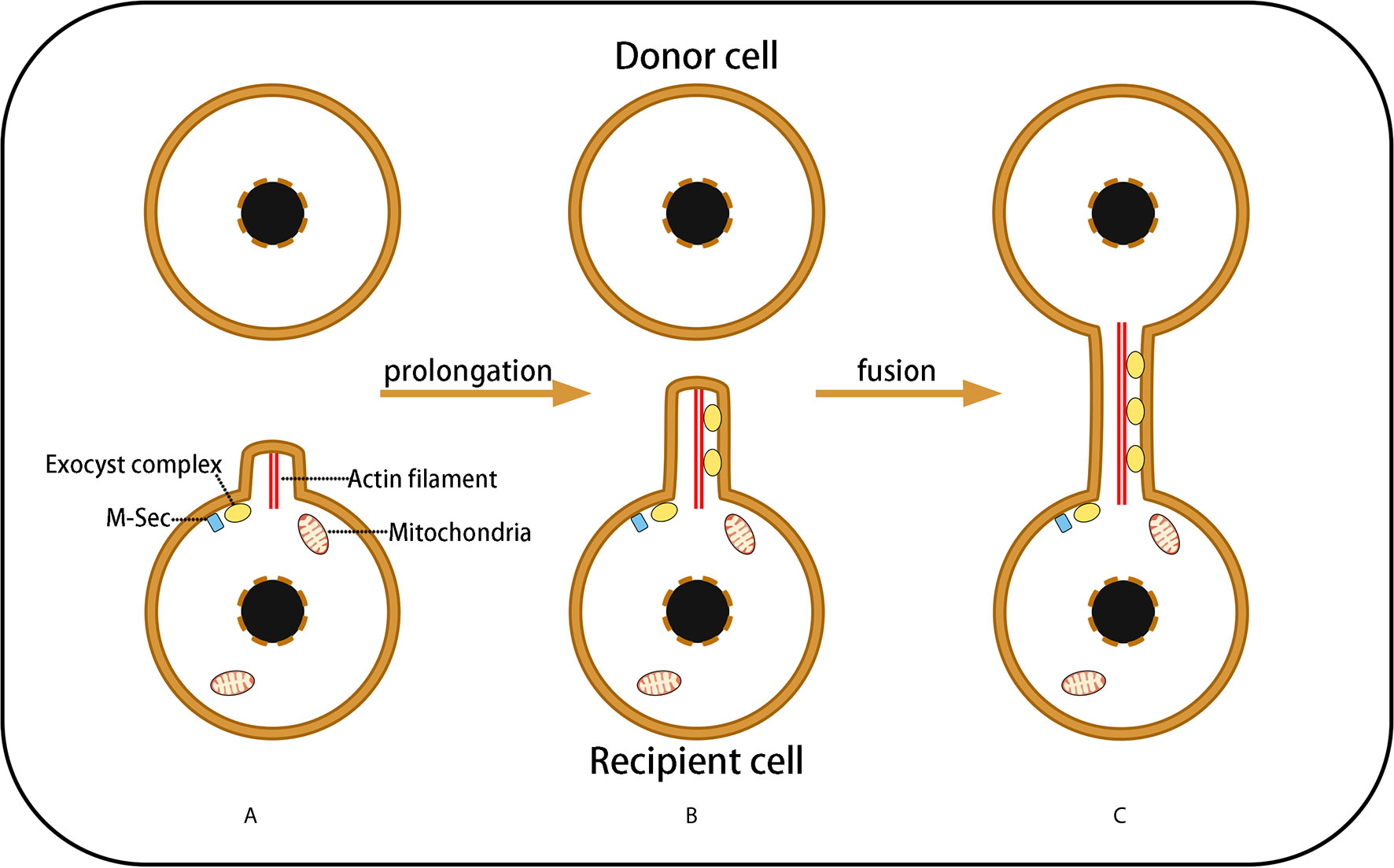
Figure 2 Three Stages of TNT Formation. (A) Under the control of M-sec and exocyst complexes, cells needing healthy mitochondria emit a membrane protrusion containing F-actin; (B) Exocyst complexes induce F-actin remodeling, resulting in membrane protrusion prolongation that forms a filopodium-like membrane structure; (C) The prolongated membrane protrusion contacts the target cell (mitochondrial donor cell) and fuses with the target cell membrane to form a membrane channel connecting the cytoplasm of the two cells, which is termed a TNs. However, the fusion mechanism of the phospholipid bilayers of the donor and recipient cells remains unclear.
Studies have demonstrated that TNTs act as a channel for mitochondrial transfer between various cells (Figure 1A; Table 1). Under condition of oxidative stress, intracellular p53 expression is upregulated and the AKT-PI3K-mTOR signaling pathway is activated, leading to the formation of TNTs from stressed cells to non-stressed cells, and mediating the transcellular transport of four organelles (ER, Golgi, endosome and mitochondria), including mitochondria (10). Interestingly, an experiment conducted two years later contradicted this view, concluding that TNTs did not depend on p53 activation (64). In the bone marrow microenvironment of multiple myeloma, TNT-mediated transcellular transfer of mitochondria was found to rely on the expression of CD38 (55). Although the upstream activation signals may be distinct, there should be a common downstream mechanism guiding the formation of TNTs.
A variety of molecules are involved in the process of TNT formation, including M−Sec (also known as TNF alpha induced protein 2), the exocyst complex, small GTPases, and leukocyte specific transcript 1 (LST1). The exocyst complex, an octameric protein complex, mediates the fusion of secretory vesicles derived from the Golgi body with the plasma membrane during exocytosis of yeast, mammals, and other eukaryotes (65–68). In recent years, it has been found that the exocyst complex also plays a significant role in various processes involved in the morphological transformation of the cell membrane, such as neurites, cilia, filopodia, and EVs (67, 69, 70). The components of the exocyst complex interact with several small GTPases (Rho1, Rho3, Cdc42, and RalA) to promote actin cytoskeletal remodeling, which is closely related to the function of the exocyst complex (69, 71, 72). The exocyst complex also participates in the formation of TNTs, and this process is controlled by M-Sec, a protein that has been proven to control the formation of TNTs (73, 74).
M-Sec can promote the assembly of the exocyst complex and interact with it, as well as with RalA (or Cdc42), leading to actin cytoskeleton remodeling, which is the key step of TNT formation (75). Studies have shown that in the initial stage of TNT formation, the N-terminal polybasic region of M-Sec directly integrates with phosphatidylinositol (4, 5)-diphosphate, so that M-Sec is fixed on the cell membrane. In addition, the interaction between M-Sec and RalA requires a positively charged surface at the C-terminal of M-Sec (76). Filopodia transformation is also a possible mechanism for TNT formation (62), as Cdc42, whose activated protein Ral binding protein 1 (RalBP1) regulates actin remodeling during filopodia formation (77), also participates in TNT formation; however, Cdc42 seems to be only related to the prolongation stage of TNTs (75).
The MHC class III protein, LST1, is a transmembrane protein that can recruit RalA to the submembrane region and accelerates interaction between RalA and the exocyst complex. Meanwhile, LST1 recruits the actin cross-linked protein filamin. LST1, along with M-Sec, RalA, and the exocyst complex, together promote the remodeling and cross-linking of actin filaments, leading to cell membrane protrusion and fusion, ultimately leading to TNT formation (78) (Figure 3). However, the mechanism of the fusion between the membrane protrusion and the target cells remains unclear.
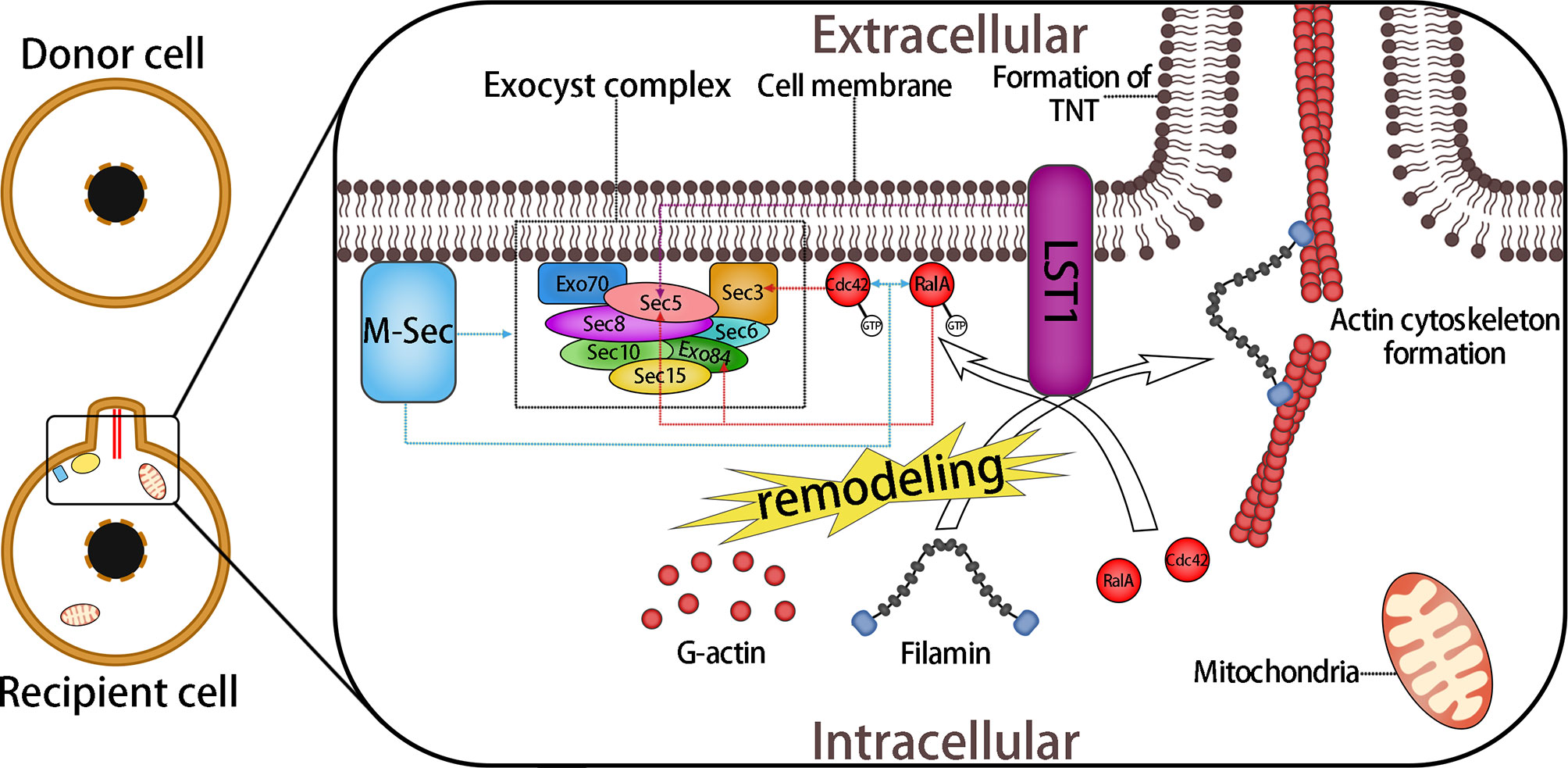
Figure 3 Formation of TNTs Mediated by M-Sec and the Exocyst Complex. The exocyst complex consists of eight proteins: Sec3, Sec35, Sec36, Sec38, Sec310, Sec315, Exo70, and Exo84. During the interactions of M-sec with the exocyst complex, small GTPase RalA and Cdc42 promote the assembly of the exocyst complex and lead to the remodeling of actin. Active RalA (RalA-GTP) interacts with two components of the exocyst complex, Sec5 and Exo84, which bind to RalA competitively. This interaction leads directly to the aggregation of G-actin and the subsequent formation of the membrane protrusion. The combination of Cdc42 and Sec3 plays a role in the prolongation stage of the membrane protrusion. In addition, LST1 recruits RalA to the submembrane region, and promotes its interaction with the exocyst complex and recruits filamin, an actin cross-linked protein. Moreover, LST1 can interact with M-sec, myosin, and myoferlin, which might also participate in the subsequent process of mitochondrial anchoring and transfer. Overall, a variety of molecules are involved in the formation of the membrane protrusion, including some of the molecules mentioned later. Together, they form a multi-molecular complex that regulates the formation of TNTs. Dotted arrows indicate interactions between proteins.
The transport and recycling of vesicles between biomembranes is also thought to be related to TNTs, as both the small GTPase Rab11a, which controls this process, and the downstream Rab8a, are involved in the formation of TNTs (79). In bladder cancer cells, inhibition of RalGPS2, the guanine nucleotide exchange factor (GEF) of RalA, also leads to a large reduction in TNT formation; moreover, RalGPS2 also interacts with LST1 and RalA and participates in the formation of multi-molecular complexes (80). In neurons, the unconventional molecular motor myosin X is also necessary for the formation of TNTs, because TNTs can be developed from filopodia driven by myosin X (81). The concentration gradient of S100 calcium binding protein A (S100A) in nervous tissue determines the extension direction of TNTs. Under oxidative stress, a decrease in S100A levels in neurons leads to a concentration gradient of S100A between astrocytes and neurons. TNTs then extend from neurons with a low concentration S100A and are received by astrocytes with a high concentration S100A (82).
TNT-mediated mitochondrial transfer can act as a survival pathway for cells under stress, for example, saving ischemic damaged cells (83), protecting the alveolar epithelium from injury (49), and repairing tissue (2, 6, 84). TNTs also exist in cancer cells, in which they are related to the survival and drug resistance of cancer cells (41, 85).
Transfer via Extracellular Vesicles
There are two types of EVs: Exosomes (30–150 nm in diameter) and microvesicles (30–1000 nm in diameter) (86). As a form of intercellular communication, EVs can deliver various substances, including small molecules, organelles, and membrane proteins, and thus play a role in mitochondrial transcellular transfer in certain types of cells.
Under conditions of evacuating serum in vitro and stimulating ischemic stroke in vivo, astrocytes were able to release EVs containing mitochondria for phagocytosis and utilization by nearby neurons to reduce nerve damage (25). In a model of allergic airway disease, EVs containing mitochondria derived from airway myeloid-derived regulatory cells (MDRCs) could be ingested by peripheral T cells, resulting in modulation of the bioenergetics of T cells, which is related to immunomodulation and the regulation of inflammatory responses of the disease (33, 87). Retinal ganglion cells can produce cell membrane processes containing axon mitochondria and release vesicles, which are phagocytized and degraded by astrocytes in the optic nerve papilla (27). EVs containing mitochondria were also found in the plasma of patients with melanoma (88).
The NAD+/CD38/cADPR/Ca2+ pathway is the key in EV-mediated mitochondrial transcellular transfer. CD38, a transmembrane protein expressed on various cell membranes, can produce cyclic ADP-ribose (cADPR) using co-enzyme nicotinamide adenine dinucleotide (NAD) as a substrate. cADPR is a second messenger that can open the intracellular Ca2+ pool. cADPR acts on ryanodine receptors (RyRs) (a calcium ion channel) on the endoplasmic reticulum, which promotes the release of Ca2+ stored in the endoplasmic reticulum. Thus, the transient Ca2+ concentration in the cytosol increases, leading to a series of downstream responses (89).
Glioma cells under starvation can accumulate a large amount of NAD+ and release it into the extracellular region. Through the NAD+/CD38/cADPR/Ca2+ pathway, the increasing calcium in the cytosol promotes actin cytoskeleton remodeling, leading to cell membrane invagination, thus completing the endocytosis of EVs (28) (Figure 1B). Inhibition of endocytosis resulted in a reduction of mitochondrial transfer from bone marrow mesenchymal stem cells (BMMSCs) to wounded lung epithelial cells (24). Mitochondrial transcellular transfer by EVs between astrocytes and neurons has also been shown to depend on the NAD+/CD38/cADPR/Ca2+ pathway (25, 26). This pathway controls not only the endocytosis stage of EVs, but also seems to be involved in the exocytosis stages, because the amount of EVs is significantly reduced, not increased, after small interfering RNA (siRNA) inhibition of CD38 expression (25).
The exocyst complex, the critical molecule of actin skeleton remodeling and membrane protrusions formation, as mentioned in section 2.1.2, might also participate in the downstream mechanism of the CD38-mediated EV pathway, because inhibition of the composition of the exocyst complex caused a remarkable reduction in EV formation (70). In addition, the calcium signal that promotes EV formation also participates in TNT formation mediated by the exocyst complex (75). Therefore, we believe that: The exocyst complex can be assembled and activated by the NAD+/CD38/cADPR/Ca2+ pathway and interacts with certain types of small GTPase and unknown regulatory factors, leading to the release or endocytosis, or both, of EVs.
Transfer via Gap Junctions
The GJ protein connexin is a widely distributed in various cells. Six rod-shaped connexins form a connexon or hemichannel across the plasma membrane, with a nanoscale hydrophilic channel in the middle. The hemichannel, as a precursor structure of GJs, can also exist independently as a channel connecting the inside and outside of the cell (90, 91). A head-to-head connection between two hemichannels forms a structure called a gap junction channel (GJC) that connects the cytoplasm of two cells. GJCs allow the exchange of ions (especially calcium ions) or small molecules between the two cells (92), facilitating the exchange of chemical or electrical signal between the two cells. GJCs can provide nutrient substances to surrounding cells, protect cells from injury (93), and are involved in clathrin-dependent EV endocytosis (94, 95).
Cx43 is a type of connexin that has been proven to be involved in ischemia/reperfusion injury of myocardial and cerebral tissue, and can also protect myocardial and cerebral tissue from ischemia/reperfusion injury under certain conditions (96). Cx43 seems to have an important association with mitochondrial function. Cx43 hemichannels on mitochondria participate in maintaining mitochondrial calcium homeostasis and can lead to mitochondria damage and cell apoptosis by passing Ca2+ (97, 98). Cx43 also participates in the transcellular transfer of mitochondria. In a model of LPS-induced acute lung injury, bone marrow stromal cells (BMSCs) delivered mitochondria to the injured alveolar epithelium. This process relies on Ca2+ exchange between the two cells through CX43-GJCs (49).
Cx43 has been proven to be an important regulator involved in the formation and function of TNTs. In a model of airway allergic inflammation, the formation of TNTs connecting induced pluripotent stem cell (IPSC)-MSCs and airway epithelial cells depends on Cx43-GJCs, while downregulating Cx43 expression significantly inhibited the metastatic transfer between the two groups of cells, and simultaneously blocked the protective effect of IPSC-MSCs on lung tissues (12). Cx43 and its related signaling pathways in breast cancer cells, such as Rho associated coiled-coil containing protein kinase (ROCK), protein kinase A (PKA), focal adhesion kinase (FAK) and p38, have novel non-canonical roles of regulating TNT formation (99). Knocking out the CX43 genes of human trabecular meshwork cells also led to a significant reduction in TNTs (100). Cx43-GJCs might play an important role in the formation of TNTs. Cx43-GJCs participate in the coordination mechanism of the two cells. This is likely to be regulated by intercellular ion exchange, especially Ca2+, as calcium signal exchange exists between the two cells connected by TNTs (75). Moreover, functional GJs have been found at the end of the membrane extension of TNTs (101), which also supports this hypothesis.
In addition, Cx43-GJCs participate in the intercellular exchange of ROS (102), and there may even be direct mitochondrial transfer via Cx43 GJCs (9), which might also be a mechanism for TNT formation and the intercellular transport of mitochondria (Figure 1C).
Transfer via Other Routes
TNTs, EVs and Cx43-GJCs are the main routes that mediate transcellular transfer of mitochondria in most studies. However, there are also some other routes, such as mitochondrial extrusion and cytoplasmic fusion.
Naked mitochondria or mitochondrial components can also be extruded and internalized without carrier, which are also called exocytosis and endocytosis (5). Cytoplasmic vacuoles derived from plasma membrane engulf mitochondria and then fuse with cytomembrane to extrude the damaged mitochondria out under the condition of tumor necrosis factor-α (TNFα)-induced apoptosis in a actin and tubulin cytoskeletons-dependent manner (103). Endocytosis of naked mitochondrial was also detected in chloramphenicol (CAP)- and efrapeptin (EF)-sensitive mammalian cells, which internalized free mitochondria isolated from CAP- and EF-resistant fibroblasts in a coincubation medium (104). By endocytosis, vitality and bioenergetics of recipient cells restore (105).
Cytoplasmic fusion is a common phenomenon in which the membrane of two or more cells fuse together and the organelles are shared, in which injury and inflammation may induce this process (106). Cell fusion can regulate the potential of stem cells, which plays an important role in regeneration and oncogenesis (107). Mitochondrial transfer may participate this process (108). However, the extent to which mitochondrial transfer promotes the potential of stem cells is still unknown.
The Transport Complex Drives Intracellular and Intercellular Movement of Mitochondria
Movement of Mitochondria Based on Microtubules and Microfilaments
The mitochondrial transport complex consists of mitochondrial Rho small GTPase (MIRO) and motor proteins based on microtubules or microfilaments. In certain cases, adaptors such as TRAK are also needed (Figure 4). MIRO, a member of the Ras superfamily, is located on the mitochondrial outer membrane, attached by its C-terminal hydrophobic transmembrane domain (109, 110), and binds with motor proteins directly or indirectly (via adaptor proteins) (43). Transport complex allows mitochondria immobilized on cytoskeleton and provides the power driving the movement of mitochondria.
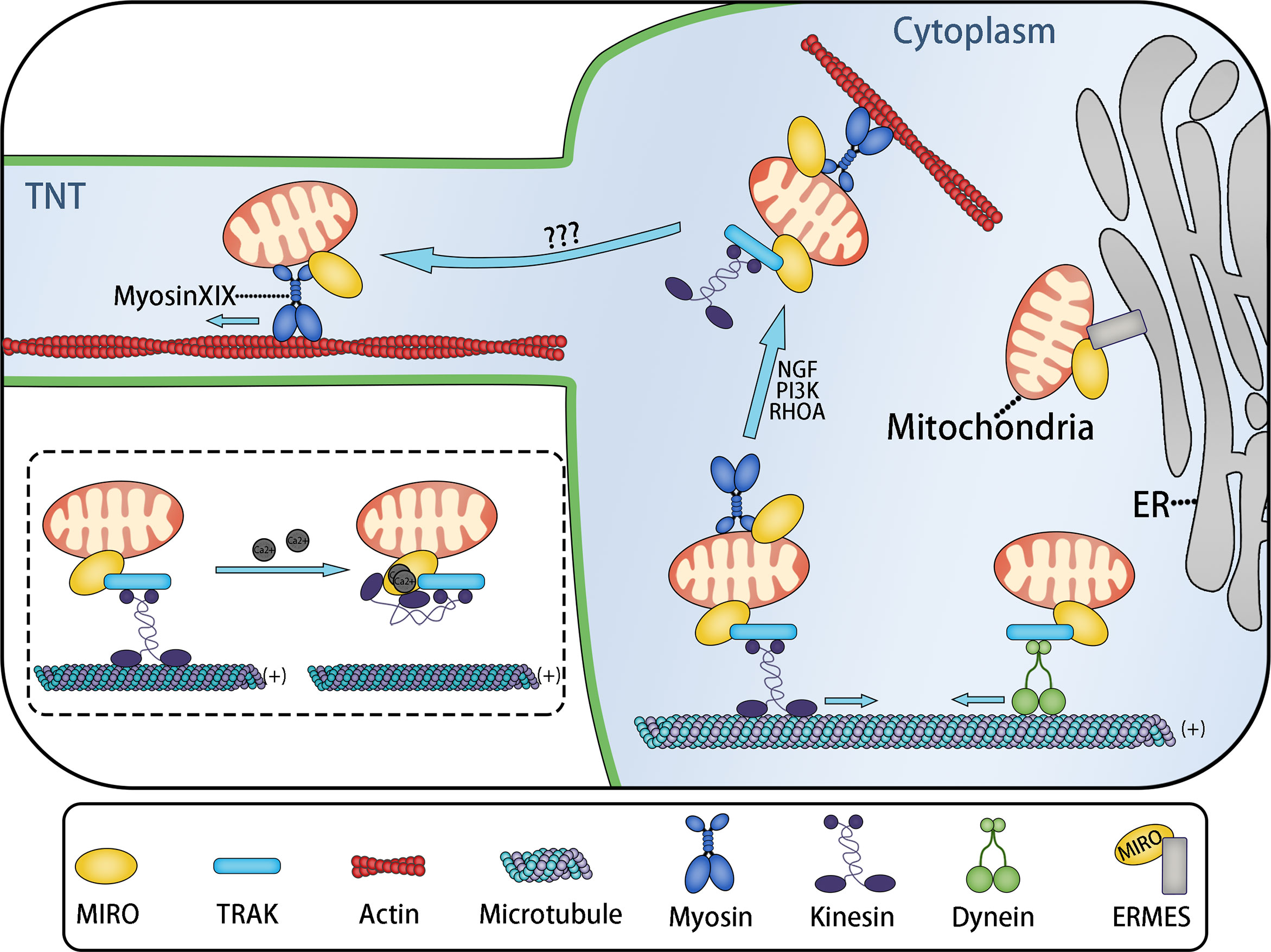
Figure 4 MIRO Mediates the Intracellular and Intercellular Distribution of Mitochondria. Mitochondria move to adapt the ATP demand of different parts of cells. Long-distance transport of mitochondria is usually mediated by microtubules, while short-distance transport of mitochondria is mediated by actin. MIRO might be a key protein involved in mitochondrial transport. MIRO participates in the formation of various mitochondria transport complexes. MIRO/TRAK/kinesin and MIRO/TRAK/dynein transport complexes mediate mitochondrial movement to the plus (+) and minus (−) ends of microtubules, respectively. The ERMES complex, containing MIRO, tethers mitochondria to the endoplasmic reticulum. This might be related to Ca2+ exchange. Under the stimulation of nerve growth factor (NGF), PI3K, and RHOA signals, mitochondrial transport changes from microtubule-based transport to microfilament-based transport, completing the actin−based docking of mitochondria. Actin-based docking of mitochondria might represent the transitional stage of mitochondria entering TNTs, while the mitochondrial movement in TNTs is probably mediated by the MIRO/myosin XIX transport complex. These movements are regulated by the local ATP/ADP ratio. However, the transitional mechanism of mitochondria entering TNTs remains unclear. The dotted box: Two calcium ions bind to the EF-hand Ca2+-binding domains of MIRO, resulting in the change of kinesin configuration: The microtubule binding site of kinesin binds to MIRO, leading to the separation of mitochondria from the microtubule.
MIRO1 and MIRO2 are two subtypes of MIRO in humans. The combination of drosophila MIRO (dMIRO) and Milton mediates the transport of mitochondria based on microtubules in Drosophila (111). Inhibition of dMIRO expression leads to mitochondrial transport disorder in the dendrites and axons of Drosophila neurons (112, 113). As the mitochondrial receptor, MIRO forms the transport complex with Milton and Kinesin to mediate the anterograde transport of mitochondria in axons, in which Milton act as a motor adaptor and Kinesin is a type of motor protein based on microtubules (114). Trafficking Kinesin Protein 1 (TRAK1) and TRAK2, the human Milton orthologs, have also been proven to play an important role in the process of mitochondrial transport by binding with MIRO to form MIRO/TRAK/Kinesin complex (115, 116) (Figure 4). Indeed, not only kinesin, but also other microtubule motor proteins, such as kinesin superfamily KIF5 and dynein can also interact with TRAK and MIRO to form different mitochondrial transport complexes based on microtubules (43, 117, 118). MIRO contains two GTPase domains (located at the N-terminus and C-terminus, respectively) and two EF-hand Ca2+-binding domains (109, 110). Based on the two EF-hand Ca2+-binding domains, Ca2+ can change the configuration of the MIRO/adaptor/motor complex to regulate mitochondrial fixation on microtubules. When the cytosolic Ca2+ concentration increases, the MIRO/TRAK/motor complex is separated from the microtubule, causing the mitochondria fall off the microtubule (43, 119, 120) (Figure 4).
Microtubules are responsible for the long-distance transport of mitochondria, such as the transport in neuron axon, while the short-distance transport mainly depends on the actin microfilament system (43). Mitochondrial transport based on microfilaments is mediated by a transport complex containing actin motor—myosin. Currently known myosin family members that adapt to mitochondria include myosin II, myosin V, myosin VI, and myosin XIX.
Myosin II promotes mitochondrial contraction by inducing deformation of the microfilament skeleton during mitochondrial division (121). Myosin V and VI exert a negative regulatory effect on the microtubule-based axon transport of mitochondria. Downregulating the expression of myosin V and VI can significantly accelerate the speed of mitochondrial transport, which is possibly caused by myosin V and VI mediating the actin-based docking of mitochondria (122). By slowing down the speed of mitochondria and anchoring mitochondria to the cytoskeleton, mitochondrial docking maintains the needed quantity of stationary mitochondria in the regions that need energy and Ca2+-buffering capacity (43). Thus, these regions obtain sufficient ATP. Although the docking receptor has not been identified, it is established that myosin mediates mitochondrial docking (43). This process might also assist the transcellular transport of mitochondria, because the actin-based docking of mitochondria can slow down the speed of mitochondrial movement, which might be a transitional stage in which mitochondria move from the cytoplasm to TNTs or EVs via actin microfilaments (Figure 4).
Myosin XIX Drives the Movement of Mitochondria in TNTs
Myosin XIX is a high-duty ratio actin motor with strong affinity for mitochondria (123, 124), which is fixed to the mitochondrial outer membrane via a 30–45-residue motif (125), while MIRO can recruit and stabilize myosin XIX to the mitochondrial outer membrane as the mitochondrial motor adaptor protein, thus driving the actin-based movement of mitochondria by interacting with myosin XIX (126, 127). This process is regulated by the local ATP/ADP ratio (128). We hereby propose a hypothesis: The movement of mitochondria in TNTs is driven by the MIRO/myosin XIX transport complex, of which myosin XIX is the motor protein (Figure 4).
This hypothesis is based on the following evidence: a. Under the induction of starvation culture or ROS, cells produce abundant filopodia, in which mitochondria exist, and the process of mitochondrial transfer to the top of filopodia has been proved to be mediated by myosin XIX (125, 128, 129); b. As mentioned above, filopodia have many similarities with TNTs: Filopodia and TNTs are both generated by membrane protrusions under oxidative stress and use actin filaments as skeletons. In certain conditions, filopodia can also be converted into TNTs (62); c. Evidence shows that myosin drives TNT-based transcellular material transport (130); and MIRO also mediates TNT-based transcellular mitochondrial transport from mesenchymal stem cells to epithelial cells (21, 131) and nerve cells (132, 133). This process depends on a unique residue (a class specific tryptophan) of the myosin XIX motor domain (129). However, direct evidence of myosin XIX’s presence in TNTs is still needed. Besides, myosin XIX controls actin-based mitochondrial movement during cell mitosis to ensure the symmetrical distribution of mitochondria to daughter cells (134).
Coordination of MIRO in Transcellular Mitochondrial Transport
MIRO, the connecting component of the transport complex, interacts with microtubules and actin motor proteins such as kinesin and myosin, adaptor proteins TRAK1 and TRAK 2, PTEN induced kinase 1 (PINK1) (regulates mitochondrial autophagy) (135), hypoxia up-regulated mitochondrial movement regulator protein (HUMMR) (involved in mitochondrial movement in neurons under hypoxia) (136), and abundant types of cytoskeleton binding proteins. MIRO can also be used as part of the ER-mitochondria encounter structure (ERMES) complex to fix mitochondria on the endoplasmic reticulum (Figure 4) (137, 138). By connecting mitochondria with different transport complexes, MIRO regulates mitochondrial movement based on microtubules and actin, and the transformation between the two forms of mitochondrial movement (126, 127). MIRO not only modulates the intracellular movement of mitochondria, but also mediates the intercellular movement of mitochondria in TNTs.
The evidence above suggests that MIRO is the key protein that modulates intra- and intercellular mitochondria distribution. MIRO might also mediate the transitional stage during which mitochondria move from the cytoplasm to the transcellular transfer structures, such as TNTs or EVs. Mitochondria are separated from the microtubule binding site, and then anchored on an actin filament. After that, mitochondria move to enter TNTs driven by myosin, where mitochondria are anchored on the actin filament of TNTs for subsequent intercellular transport. All these processes are mediated by the transport complexes composed of MIRO and its adaptor proteins and motors (Figure 4). Similarly, MIRO might also play an important role in the process of mitochondrial entry into EVs.
Conclusions
Communication between cells involves the exchange of information (small molecules, ions, complexes, extracellular vesicles, and even various organelles) through various structures such as GJs, TNTs, EVs, endocytosis, and exocytosis. Transcellular transfer of mitochondria is considered a form of intercellular communication that exists commonly in organisms. Cells under stress receive active mitochondria via TNTs, EVs, or GJCs to improve their aerobic respiration and viability, which might represent the self-protection capacity of cells. The use of autologous or infused active mitochondria to rescue dying cells seems to be a prospective research direction for the treatment of ischemic/reperfusion-related deficiency. In animal models of stroke, preliminary experiments associated with mitochondrial transcellular transfer have shown some progress (25, 139, 140), with the potential to develop into clinical therapy. This therapy is called mitochondrial transplantation, which has shown promising effects in several central nervous system (CNS) diseases, such as cerebral ischemia and Parkinson’s disease (4). Moreover, tumor cells can also convert their metabolism to enhance proliferation or apoptosis via the transcellular uptake of mitochondria, which might also be a potential therapeutic target of anti-tumor drugs. Intervention could be possible at multiple nodes in these processes: The formation of TNTs or EVs; the receipt of TNTs and EVs by the target cell; the function of Cx43-GJs; and the transport complex driving mitochondrial movement.
Thee NAD+/CD38/cADPR/Ca2+ pathway and the exocyst complex might participate in regulating the formation of TNTs and EVs; however, currently, the specific regulation mechanism is poorly understood. In particular, the detailed mechanisms of the fusion of TNTs and the target cell membrane, the receipt of signals by surrounding cells signals and the release of EVs, the endocytosis of EVs by target cells, the role of GJs and the cytoskeleton in TNTs and EVs, the accurate regulation of mitochondrial transfer based on microtubules and microfilaments, and the entry of mitochondria remain to be determined in future studies.
Author Contributions
YQ wrote the manuscript and drew the figures. XJ and QY collected the related papers, created the Tables, and helped to revise the manuscript. XJ, QY, and JZ participated in the design of the review. YZ and QZ designed and revised the manuscript. All authors contributed to the article and approved the submitted version.
Funding
This work was supported the Science and Technology Foundation Survey Project of Ministry of Science and Technology of China [grant numbers 2018FY100900 and 2018FY10090004].
Conflict of Interest
The authors declare that the research was conducted in the absence of any commercial or financial relationships that could be construed as a potential conflict of interest.
Abbreviations
TNTs, tunneling nanotubes; EVs, extracellular vesicles; GJs, gap junctions; GJCs, gap junction channels; MSCs, mesenchymal stem cells; ROS, reactive oxygen species; OXPHOS, oxidative phosphorylation; DNA, deoxyribonucleic acid; ATP, adenosine triphosphate; BMSCs, bone marrow stromal cells; CMs, cardiomyocytes; hMADs, human multipotent adipose-derived stem cells; RTCs, renal tubular cells; MMSCs, mesenchymal multipotent stromal cells; hVSMCs, human vascular smooth muscle cells; MELAS, mitochondrial myopathy, encephalomyopathy, lactic acidosis, and stroke-like episodes; LST1, leukocyte specific transcript 1; RalBP1, Ral binding protein 1; GEF, guanine nucleotide exchange factor; MDRCs, myeloid-derived regulatory cells; cADPR, cyclic ADP-ribose; NAD, nicotinamide adenine dinucleotide; RyRs, Ryanodine receptors; ROCK, Rho associated coiled-coil containing protein kinase; FAK, focal adhesion kinase; TNFα, tumor necrosis factor-α; MIRO, mitochondrial Rho small GTPase; TRAK, Trafficking kinesin protein; KIF5, Kinesin family member 5; ERMES, ER-mitochondria encounter structure; HUMMR, hypoxia up-regulated mitochondrial movement regulator; NGF, nerve growth factor.
References
1. Singh B, Modica-Napolitano JS, Singh KK. Defining the Momiome: Promiscuous Information Transfer by Mobile Mitochondria and the Mitochondrial Genome. Semin Cancer Biol (2017) 47:1–17. doi: 10.1016/j.semcancer.2017.05.004
2. Plotnikov EY, Babenko VA, Silachev DN, Zorova LD, Khryapenkova TG, Savchenko ES, et al. Intercellular Transfer of Mitochondria. Biochem Biokhimiia (2015) 80(5):542–8. doi: 10.1134/S0006297915050041
3. Spees JL, Olson SD, Whitney MJ, Prockop DJ. Mitochondrial Transfer Between Cells Can Rescue Aerobic Respiration. Proc Natl Acad Sci USA (2006) 103(5):1283–8. doi: 10.1073/pnas.0510511103
4. Shanmughapriya S, Langford D, Natarajaseenivasan K. Inter and Intracellular Mitochondrial Trafficking in Health and Disease. Ageing Res Rev (2020) 62:101128. doi: 10.1016/j.arr.2020.101128
5. Liu D, Gao Y, Liu J, Huang Y, Yin J, Feng Y, et al. Intercellular Mitochondrial Transfer as a Means of Tissue Revitalization. Signal Transduct Target Ther (2021) 6(1):65. doi: 10.1038/s41392-020-00440-z
6. Paliwal S, Chaudhuri R, Agrawal A, Mohanty S. Regenerative Abilities of Mesenchymal Stem Cells Through Mitochondrial Transfer. J Biomed Sci (2018) 25(1):31. doi: 10.1186/s12929-018-0429-1
7. Chang CY, Liang MZ, Chen L. Current Progress of Mitochondrial Transplantation That Promotes Neuronal Regeneration. Trans Neurodegeneration (2019) 8:17. doi: 10.1186/s40035-019-0158-8
8. Nakamura Y, Park JH, Hayakawa K. Therapeutic Use of Extracellular Mitochondria in CNS Injury and Disease. Exp Neurology (2020) 324:113114. doi: 10.1016/j.expneurol.2019.113114
9. Griessinger E, Moschoi R, Biondani G, Peyron JF. Mitochondrial Transfer in the Leukemia Microenvironment. Trends cancer. (2017) 3(12):828–39. doi: 10.1016/j.trecan.2017.10.003
10. Wang Y, Cui J, Sun X, Zhang Y. Tunneling-Nanotube Development in Astrocytes Depends on P53 Activation. Cell Death Differentiation (2011) 18(4):732–42. doi: 10.1038/cdd.2010.147
11. Lin TK, Chen SD, Chuang YC, Lan MY, Chuang JH, Wang PW, et al. Mitochondrial Transfer of Wharton’s Jelly Mesenchymal Stem Cells Eliminates Mutation Burden and Rescues Mitochondrial Bioenergetics in Rotenone-Stressed MELAS Fibroblasts. Oxid Med Cell Longevity (2019) 2019:9537504. doi: 10.1155/2019/9537504
12. Yao Y, Fan XL, Jiang D, Zhang Y, Li X, Xu ZB, et al. Connexin 43-Mediated Mitochondrial Transfer of Ipsc-Mscs Alleviates Asthma Inflammation. Stem Cell Rep (2018) 11(5):1120–35. doi: 10.1016/j.stemcr.2018.09.012
13. Li CJ, Chen PK, Sun LY, Pang CY. Enhancement of Mitochondrial Transfer by Antioxidants in Human Mesenchymal Stem Cells. Oxid Med Cell Longevity (2017) 2017:8510805. doi: 10.1155/2017/8510805
14. Liu K, Ji K, Guo L, Wu W, Lu H, Shan P, et al. Mesenchymal Stem Cells Rescue Injured Endothelial Cells in an in Vitro Ischemia-Reperfusion Model Via Tunneling Nanotube Like Structure-Mediated Mitochondrial Transfer. Microvascular Res (2014) 92:10–8. doi: 10.1016/j.mvr.2014.01.008
15. Carneiro BA, Kaplan JB, Altman JK, Giles FJ, Platanias LC. Targeting Mtor Signaling Pathways and Related Negative Feedback Loops for the Treatment of Acute Myeloid Leukemia. Cancer Biol Ther (2015) 16(5):648–56. doi: 10.1080/15384047.2015.1026510
16. Acquistapace A, Bru T, Lesault PF, Figeac F, Coudert AE, le Coz O, et al. Human Mesenchymal Stem Cells Reprogram Adult Cardiomyocytes Toward a Progenitor-Like State Through Partial Cell Fusion and Mitochondria Transfer. Stem Cells (Dayton Ohio) (2011) 29(5):812–24. doi: 10.1002/stem.632
17. Koyanagi M, Brandes RP, Haendeler J, Zeiher AM, Dimmeler S. Cell-to-Cell Connection of Endothelial Progenitor Cells With Cardiac Myocytes by Nanotubes: A Novel Mechanism for Cell Fate Changes? Circ Res (2005) 96(10):1039–41. doi: 10.1161/01.RES.0000168650.23479.0c
18. Zhang Y, Yu Z, Jiang D, Liang X, Liao S, Zhang Z, et al. Ipsc-Mscs With High Intrinsic MIRO1 and Sensitivity to TNF-Alpha Yield Efficacious Mitochondrial Transfer to Rescue Anthracycline-Induced Cardiomyopathy. Stem Cell Rep (2016) 7(4):749–63. doi: 10.1016/j.stemcr.2016.08.009
19. Jiang D, Gao F, Zhang Y, Wong DS, Li Q, Tse HF, et al. Mitochondrial Transfer of Mesenchymal Stem Cells Effectively Protects Corneal Epithelial Cells From Mitochondrial Damage. Cell Death Dis (2016) 7(11):e2467. doi: 10.1038/cddis.2016.358
20. Vallabhaneni KC, Haller H, Dumler I. Vascular Smooth Muscle Cells Initiate Proliferation of Mesenchymal Stem Cells by Mitochondrial Transfer Via Tunneling Nanotubes. Stem Cells Dev (2012) 21(17):3104–13. doi: 10.1089/scd.2011.0691
21. Ahmad T, Mukherjee S, Pattnaik B, Kumar M, Singh S, Kumar M, et al. Miro1 Regulates Intercellular Mitochondrial Transport & Enhances Mesenchymal Stem Cell Rescue Efficacy. EMBO J (2014) 33(9):994–1010. doi: 10.1002/embj.201386030
22. Lu J, Zheng X, Li F, Yu Y, Chen Z, Liu Z, et al. Tunneling Nanotubes Promote Intercellular Mitochondria Transfer Followed by Increased Invasiveness in Bladder Cancer Cells. Oncotarget (2017) 8(9):15539–52. doi: 10.18632/oncotarget.14695
23. Ippolito L, Morandi A, Taddei ML, Parri M, Comito G, Iscaro A, et al. Cancer-Associated Fibroblasts Promote Prostate Cancer Malignancy Via Metabolic Rewiring and Mitochondrial Transfer. Oncogene (2019) 38(27):5339–55. doi: 10.1038/s41388-019-0805-7
24. Sinclair KA, Yerkovich ST, Hopkins PM, Chambers DC. Characterization of Intercellular Communication and Mitochondrial Donation by Mesenchymal Stromal Cells Derived From the Human Lung. Stem Cell Res Ther (2016) 7(1):91. doi: 10.1186/s13287-016-0354-8
25. Hayakawa K, Esposito E, Wang X, Terasaki Y, Liu Y, Xing C, et al. Transfer of Mitochondria From Astrocytes to Neurons After Stroke. Nature (2016) 535(7613):551–5. doi: 10.1038/nature18928
26. Gao L, Zhang Z, Lu J, Pei G. Mitochondria are Dynamically Transferring Between Human Neural Cells and Alexander Disease-Associated GFAP Mutations Impair the Astrocytic Transfer. Front Cell Neurosci (2019) 13:316. doi: 10.3389/fncel.2019.00316
27. Davis CH, Kim KY, Bushong EA, Mills EA, Boassa D, Shih T, et al. Transcellular Degradation of Axonal Mitochondria. Proc Natl Acad Sci USA (2014) 111(26):9633–8. doi: 10.1073/pnas.1404651111
28. Sun C, Liu X, Wang B, Wang Z, Liu Y, Di C, et al. Endocytosis-Mediated Mitochondrial Transplantation: Transferring Normal Human Astrocytic Mitochondria Into Glioma Cells Rescues Aerobic Respiration and Enhances Radiosensitivity. Theranostics (2019) 9(12):3595–607. doi: 10.7150/thno.33100
29. Hong CS, Muller L, Boyiadzis M, Whiteside TL. Isolation and Characterization of CD34+ Blast-Derived Exosomes in Acute Myeloid Leukemia. PloS One (2014) 9(8):e103310. doi: 10.1371/journal.pone.0103310
30. Morrison TJ, Jackson MV, Cunningham EK, Kissenpfennig A, McAuley DF, O’Kane CM, et al. Mesenchymal Stromal Cells Modulate Macrophages in Clinically Relevant Lung Injury Models by Extracellular Vesicle Mitochondrial Transfer. Am J Respir Crit Care Med (2017) 196(10):1275–86. doi: 10.1164/rccm.201701-0170OC
31. Phinney DG, Di Giuseppe M, Njah J, Sala E, Shiva S, St Croix CM, et al. Mesenchymal Stem Cells Use Extracellular Vesicles to Outsource Mitophagy and Shuttle Micrornas. Nat Commun (2015) 6:8472. doi: 10.1038/ncomms9472
32. Paggetti J, Haderk F, Seiffert M, Janji B, Distler U, Ammerlaan W, et al. Exosomes Released by Chronic Lymphocytic Leukemia Cells Induce the Transition of Stromal Cells Into Cancer-Associated Fibroblasts. Blood (2015) 126(9):1106–17. doi: 10.1182/blood-2014-12-618025
33. Hough KP, Trevor JL, Strenkowski JG, Wang Y, Chacko BK, Tousif S, et al. Exosomal Transfer of Mitochondria From Airway Myeloid-Derived Regulatory Cells to T Cells. Redox Biol (2018) 18:54–64. doi: 10.1016/j.redox.2018.06.009
34. Moschoi R, Imbert V, Nebout M, Chiche J, Mary D, Prebet T, et al. Protective Mitochondrial Transfer From Bone Marrow Stromal Cells to Acute Myeloid Leukemic Cells During Chemotherapy. Blood (2016) 128(2):253–64. doi: 10.1182/blood-2015-07-655860
35. Pilling AD, Horiuchi D, Lively CM, Saxton WM. Kinesin-1 and Dynein are the Primary Motors for Fast Transport of Mitochondria in Drosophila Motor Axons. Mol Biol Cell (2006) 17(4):2057–68. doi: 10.1091/mbc.e05-06-0526
36. Kumar B, Garcia M, Weng L, Jung X, Murakami JL, Hu X, et al. Acute myeloid leukemia transforms the bone marrow niche into a leukemia-permissive microenvironment through exosome secretion. Leukemia (2018) 32(3):575–87. doi: 10.1038/leu.2017.259
37. Lin HY, Liou CW, Chen SD, Hsu TY, Chuang JH, Wang PW, et al. Mitochondrial Transfer From Wharton’s Jelly-Derived Mesenchymal Stem Cells to Mitochondria-Defective Cells Recaptures Impaired Mitochondrial Function. Mitochondrion (2015) 22:31–44. doi: 10.1016/j.mito.2015.02.006
38. Court AC, Le-Gatt A, Luz-Crawford P, Parra E, Aliaga-Tobar V, Bátiz LF, et al. Mitochondrial Transfer From Mscs to T Cells Induces Treg Differentiation and Restricts Inflammatory Response. EMBO Rep (2020) 21(2):e48052. doi: 10.15252/embr.201948052
39. Cabrera F, Ortega M, Velarde F, Parra E, Gallardo S, Barba D, et al. Primary Allogeneic Mitochondrial Mix (PAMM) Transfer/Transplant by Mitoception to Address Damage in Pbmcs Caused by Ultraviolet Radiation. BMC Biotechnol (2019) 19(1):42. doi: 10.1186/s12896-019-0534-6
40. Caicedo A, Fritz V, Brondello JM, Ayala M, Dennemont I, Abdellaoui N, et al. Mitoception as a New Tool to Assess the Effects of Mesenchymal Stem/Stromal Cell Mitochondria on Cancer Cell Metabolism and Function. Sci Rep (2015) 5:9073. doi: 10.1038/srep09073
41. Hekmatshoar Y, Nakhle J, Galloni M, Vignais ML. The Role of Metabolism and Tunneling Nanotube-Mediated Intercellular Mitochondria Exchange in Cancer Drug Resistance. Biochem J (2018) 475(14):2305–28. doi: 10.1042/BCJ20170712
42. Kiriyama Y, Nochi H. Intra- and Intercellular Quality Control Mechanisms of Mitochondria. Cells. (2017) 7(1):1. doi: 10.3390/cells7010001
43. Sheng ZH, Cai Q. Mitochondrial Transport in Neurons: Impact on Synaptic Homeostasis and Neurodegeneration. Nat Rev Neurosci (2012) 13(2):77–93. doi: 10.1038/nrn3156
44. Sahu P, Jena SR, Samanta L. Tunneling Nanotubes: A Versatile Target for Cancer Therapy. Curr Cancer Drug Targets (2018) 18(6):514–21. doi: 10.2174/1568009618666171129222637
45. Mittal R, Karhu E, Wang JS, Delgado S, Zukerman R, Mittal J, et al. Cell Communication by Tunneling Nanotubes: Implications in Disease and Therapeutic Applications. J Cell Physiol (2019) 234(2):1130–46. doi: 10.1002/jcp.27072
46. de Rooij B, Polak R, Stalpers F, Pieters R, den Boer ML. Tunneling Nanotubes Facilitate Autophagosome Transfer in the Leukemic Niche. Leukemia. (2017) 31(7):1651–4. doi: 10.1038/leu.2017.117
47. Marchi S, Patergnani S, Missiroli S, Morciano G, Rimessi A, Wieckowski MR, et al. Mitochondrial and Endoplasmic Reticulum Calcium Homeostasis and Cell Death. Cell Calcium (2018) 69:62–72. doi: 10.1016/j.ceca.2017.05.003
48. Davis CH, Marsh-Armstrong N. Discovery and Implications of Transcellular Mitophagy. Autophagy (2014) 10(12):2383–4. doi: 10.4161/15548627.2014.981920
49. Islam MN, Das SR, Emin MT, Wei M, Sun L, Westphalen K, et al. Mitochondrial Transfer From Bone-Marrow-Derived Stromal Cells to Pulmonary Alveoli Protects Against Acute Lung Injury. Nat Med (2012) 18(5):759–65. doi: 10.1038/nm.2736
50. Li X, Zhang Y, Yeung SC, Liang Y, Liang X, Ding Y, et al. Mitochondrial Transfer of Induced Pluripotent Stem Cell-Derived Mesenchymal Stem Cells to Airway Epithelial Cells Attenuates Cigarette Smoke-Induced Damage. Am J Respir Cell Mol Biol (2014) 51(3):455–65. doi: 10.1165/rcmb.2013-0529OC
51. Han H, Hu J, Yan Q, Zhu J, Zhu Z, Chen Y, et al. Bone Marrow-Derived Mesenchymal Stem Cells Rescue Injured H9c2 Cells Via Transferring Intact Mitochondria Through Tunneling Nanotubes in an in Vitro Simulated Ischemia/Reperfusion Model. Mol Med Rep (2016) 13(2):1517–24. doi: 10.3892/mmr.2015.4726
52. Pasquier J, Guerrouahen BS, Al Thawadi H, Ghiabi P, Maleki M, Abu-Kaoud N, et al. Preferential Transfer of Mitochondria From Endothelial to Cancer Cells Through Tunneling Nanotubes Modulates Chemoresistance. J Trans Med (2013) 11:94. doi: 10.1186/1479-5876-11-94
53. Rodriguez AM, Nakhle J, Griessinger E, Vignais ML. Intercellular Mitochondria Trafficking Highlighting the Dual Role of Mesenchymal Stem Cells as Both Sensors and Rescuers of Tissue Injury. Cell Cycle (Georgetown Tex) (2018) 17(6):712–21. doi: 10.1080/15384101.2018.1445906
54. Onfelt B, Nedvetzki S, Yanagi K, Davis DM. Cutting Edge: Membrane Nanotubes Connect Immune Cells. J Immunol (Baltimore Md 1950) (2004) 173(3):1511–3. doi: 10.4049/jimmunol.173.3.1511
55. Boise LH, Shanmugam M. Stromal Support of Metabolic Function Through Mitochondrial Transfer in Multiple Myeloma. Cancer Res (2019) 79(9):2102–3. doi: 10.1158/0008-5472.CAN-19-0500
56. Plotnikov EY, Khryapenkova TG, Galkina SI, Sukhikh GT, Zorov DB. Cytoplasm and Organelle Transfer Between Mesenchymal Multipotent Stromal Cells and Renal Tubular Cells in Co-Culture. Exp Cell Res (2010) 316(15):2447–55. doi: 10.1016/j.yexcr.2010.06.009
57. Gerdes HH, Bukoreshtliev NV, Barroso JF. Tunneling Nanotubes: A New Route for the Exchange of Components Between Animal Cells. FEBS Letters (2007) 581(11):2194–201. doi: 10.1016/j.febslet.2007.03.071
58. Dupont M, Souriant S, Lugo-Villarino G, Maridonneau-Parini I, Vérollet C. Tunneling Nanotubes: Intimate Communication Between Myeloid Cells. Front Immunol (2018) 9:43. doi: 10.3389/fimmu.2018.00043
59. Li RF, Zhang W, Man QW, Zhao YF, Zhao Y. Tunneling Nanotubes Mediate Intercellular Communication Between Endothelial Progenitor Cells and Osteoclast Precursors. J Mol Histology (2019) 50(5):483–91. doi: 10.1007/s10735-019-09842-y
60. Panasiuk M, Rychłowski M, Derewońko N, Bieńkowska-Szewczyk K. Tunneling Nanotubes as a Novel Route of Cell-to-Cell Spread of Herpesviruses. J Virol (2018) 92(10):e00090–18. doi: 10.1128/JVI.00090-18
61. Gurke S, Barroso JF, Hodneland E, Bukoreshtliev NV, Schlicker O, Gerdes HH. Tunneling Nanotube (TNT)-Like Structures Facilitate a Constitutive, Actomyosin-Dependent Exchange of Endocytic Organelles Between Normal Rat Kidney Cells. Exp Cell Res (2008) 314(20):3669–83. doi: 10.1016/j.yexcr.2008.08.022
62. Bukoreshtliev NV, Wang X, Hodneland E, Gurke S, Barroso JF, Gerdes HH. Selective Block of Tunneling Nanotube (TNT) Formation Inhibits Intercellular Organelle Transfer Between PC12 Cells. FEBS letters. (2009) 583(9):1481–8. doi: 10.1016/j.febslet.2009.03.065
63. Plotnikov EY, Khryapenkova TG, Vasileva AK, Marey MV, Galkina SI, Isaev NK, et al. Cell-to-Cell Cross-Talk Between Mesenchymal Stem Cells and Cardiomyocytes in Co-Culture. J Cell Mol Med (2008) 12(5a):1622–31. doi: 10.1111/j.1582-4934.2007.00205.x
64. Andresen V, Wang X, Ghimire S, Omsland M, Gjertsen BT, Gerdes HH. Tunneling Nanotube (TNT) Formation is Independent of P53 Expression. Cell Death Differentiation (2013) 20(8):1124. doi: 10.1038/cdd.2013.61
65. Jahn R, Südhof TC. Membrane Fusion and Exocytosis. Annu Rev Biochem (1999) 68:863–911. doi: 10.1146/annurev.biochem.68.1.863
66. Kee Y, Yoo JS, Hazuka CD, Peterson KE, Hsu SC, Scheller RH. Subunit Structure of the Mammalian Exocyst Complex. Proc Natl Acad Sci USA (1997) 94(26):14438–43. doi: 10.1073/pnas.94.26.14438
67. Martin-Urdiroz M, Deeks MJ, Horton CG, Dawe HR, Jourdain I. The Exocyst Complex in Health and Disease. Front Cell Dev Biol (2016) 4:24. doi: 10.3389/fcell.2016.00024
68. Heider MR, Munson M. Exorcising the Exocyst Complex. Traffic (Copenhagen Denmark). (2012) 13(7):898–907. doi: 10.1111/j.1600-0854.2012.01353.x
69. Sugihara K, Asano S, Tanaka K, Iwamatsu A, Okawa K, Ohta Y. The Exocyst Complex Binds the Small Gtpase Rala to Mediate Filopodia Formation. Nat Cell Biol (2002) 4(1):73–8. doi: 10.1038/ncb720
70. Zuo X, Kwon SH, Janech MG, Dang Y, Lauzon SD, Fogelgren B, et al. Primary Cilia and the Exocyst are Linked to Urinary Extracellular Vesicle Production and Content. J Biol Chem (2019) 294(50):19099–110. doi: 10.1074/jbc.RA119.009297
71. He B, Guo W. The Exocyst Complex in Polarized Exocytosis. Curr Opin Cell Biol (2009) 21(4):537–42. doi: 10.1016/j.ceb.2009.04.007
72. Moskalenko S, Henry DO, Rosse C, Mirey G, Camonis JH, White MA. The Exocyst is a Ral Effector Complex. Nat Cell Biol (2002) 4(1):66–72. doi: 10.1038/ncb728
73. Lotfi S, Nasser H, Noyori O, Hiyoshi M, Takeuchi H, Koyanagi Y, et al. M-Sec Facilitates Intercellular Transmission of HIV-1 Through Multiple Mechanisms. Retrovirology (2020) 17(1):20. doi: 10.1186/s12977-020-00528-y
74. Hanna SJ, McCoy-Simandle K, Leung E, Genna A, Condeelis J, Cox D. Tunneling Nanotubes, a Novel Mode of Tumor Cell-Macrophage Communication in Tumor Cell Invasion. J Cell Sci (2019) 132(3):jcs223321. doi: 10.1242/jcs.223321
75. Hase K, Kimura S, Takatsu H, Ohmae M, Kawano S, Kitamura H, et al. M-Sec Promotes Membrane Nanotube Formation by Interacting With Ral and the Exocyst Complex. Nat Cell Biol (2009) 11(12):1427–32. doi: 10.1038/ncb1990
76. Kimura S, Yamashita M, Yamakami-Kimura M, Sato Y, Yamagata A, Kobashigawa Y, et al. Distinct Roles for the N- and C-Terminal Regions of M-Sec in Plasma Membrane Deformation During Tunneling Nanotube Formation. Sci Rep (2016) 6:33548. doi: 10.1038/srep33548
77. Mattila PK, Lappalainen P. Filopodia: Molecular Architecture and Cellular Functions. Nat Rev Mol Cell Biol (2008) 9(6):446–54. doi: 10.1038/nrm2406
78. Schiller C, Diakopoulos KN, Rohwedder I, Kremmer E, von Toerne C, Ueffing M, et al. LST1 Promotes the Assembly of a Molecular Machinery Responsible for Tunneling Nanotube Formation. J Cell Sci (2013) 126(Pt 3):767–77. doi: 10.1242/jcs.114033
79. Zhu S, Bhat S, Syan S, Kuchitsu Y, Fukuda M, Zurzolo C. Rab11a-Rab8a Cascade Regulates the Formation of Tunneling Nanotubes Through Vesicle Recycling. J Cell Sci (2018) 131(19):jcs215889. doi: 10.1242/jcs.215889
80. D’Aloia A, Berruti G, Costa B, Schiller C, Ambrosini R, Pastori V, et al. Ralgps2 is Involved in Tunneling Nanotubes Formation in 5637 Bladder Cancer Cells. Exp Cell Res (2018) 362(2):349–61. doi: 10.1016/j.yexcr.2017.11.036
81. Gousset K, Marzo L, Commere PH, Zurzolo C. Myo10 is a Key Regulator of TNT Formation in Neuronal Cells. J Cell Sci (2013) 126(Pt 19):4424–35. doi: 10.1242/jcs.129239
82. Sun X, Wang Y, Zhang J, Tu J, Wang XJ, Su XD, et al. Tunneling-Nanotube Direction Determination in Neurons and Astrocytes. Cell Death Dis (2012) 3(12):e438. doi: 10.1038/cddis.2012.177
83. Ham PB,3, Raju R. Mitochondrial Function in Hypoxic Ischemic Injury and Influence of Aging. Prog Neurobiology (2017) 157:92–116. doi: 10.1016/j.pneurobio.2016.06.006
84. Spees JL, Lee RH, Gregory CA. Mechanisms of Mesenchymal Stem/Stromal Cell Function. Stem Cell Res Ther (2016) 7(1):125. doi: 10.1186/s13287-016-0363-7
85. Li C, Cheung MKH, Han S, Zhang Z, Chen L, Chen J, et al. Mesenchymal Stem Cells and Their Mitochondrial Transfer: A Double-Edged Sword. Bioscience Rep (2019) 39(5):BSR20182417. doi: 10.1042/BSR20182417
86. Welsh JA, Holloway JA, Wilkinson JS, Englyst NA. Extracellular Vesicle Flow Cytometry Analysis and Standardization. Front Cell Dev Biol (2017) 5:78. doi: 10.3389/fcell.2017.00078
87. Hough KP, Deshane JS. Exosomes in Allergic Airway Diseases. Curr Allergy Asthma Rep (2019) 19(5):26. doi: 10.1007/s11882-019-0857-3
88. Jang SC, Crescitelli R, Cvjetkovic A, Belgrano V, Olofsson Bagge R, Sundfeldt K, et al. Mitochondrial Protein Enriched Extracellular Vesicles Discovered in Human Melanoma Tissues Can Be Detected in Patient Plasma. J Extracellular Vesicles (2019) 8(1):1635420. doi: 10.1080/20013078.2019.1635420
89. Guse AH. Second Messenger Function and the Structure-Activity Relationship of Cyclic Adenosine Diphosphoribose (Cadpr). FEBS J (2005) 272(18):4590–7. doi: 10.1111/j.1742-4658.2005.04863.x
90. D’Hondt C, Iyyathurai J, Himpens B, Leybaert L, Bultynck G. Cx43-Hemichannel Function and Regulation in Physiology and Pathophysiology: Insights From the Bovine Corneal Endothelial Cell System and Beyond. Front Physiol (2014) 5:348. doi: 10.3389/fphys.2014.00348
91. Orellana JA, Martinez AD, Retamal MA. Gap Junction Channels and Hemichannels in the CNS: Regulation by Signaling Molecules. Neuropharmacology. (2013) 75:567–82. doi: 10.1016/j.neuropharm.2013.02.020
92. Simon AM, Goodenough DA. Diverse Functions of Vertebrate Gap Junctions. Trends Cell Biol (1998) 8(12):477–83. doi: 10.1016/S0962-8924(98)01372-5
93. De Vuyst E, Wang N, Decrock E, De Bock M, Vinken M, Van Moorhem M, et al. Ca(2+) Regulation of Connexin 43 Hemichannels in C6 Glioma and Glial Cells. Cell Calcium (2009) 46(3):176–87. doi: 10.1016/j.ceca.2009.07.002
94. Piehl M, Lehmann C, Gumpert A, Denizot JP, Segretain D, Falk MM. Internalization of Large Double-Membrane Intercellular Vesicles by a Clathrin-Dependent Endocytic Process. Mol Biol Cell (2007) 18(2):337–47. doi: 10.1091/mbc.e06-06-0487
95. Gumpert AM, Varco JS, Baker SM, Piehl M, Falk MM. Double-Membrane Gap Junction Internalization Requires the Clathrin-Mediated Endocytic Machinery. FEBS Letters (2008) 582(19):2887–92. doi: 10.1016/j.febslet.2008.07.024
96. Schulz R, Görge PM, Görbe A, Ferdinandy P, Lampe PD, Leybaert L. Connexin 43 is an Emerging Therapeutic Target in Ischemia/Reperfusion Injury, Cardioprotection and Neuroprotection. Pharmacol Ther (2015) 153:90–106. doi: 10.1016/j.pharmthera.2015.06.005
97. Boengler K, Schulz R. Connexin 43 and Mitochondria in Cardiovascular Health and Disease. Adv Exp Med Biol (2017) 982:227–46. doi: 10.1007/978-3-319-55330-6_12
98. Gadicherla AK, Wang N, Bulic M, Agullo-Pascual E, Lissoni A, De Smet M, et al. Mitochondrial Cx43 Hemichannels Contribute to Mitochondrial Calcium Entry and Cell Death in the Heart. Basic Res Cardiol (2017) 112(3):27. doi: 10.1007/s00395-017-0618-1
99. Tishchenko A, Azorín DD, Vidal-Brime L, Muñoz MJ, Arenas PJ, Pearce C, et al. Cx43 and Associated Cell Signaling Pathways Regulate Tunneling Nanotubes in Breast Cancer Cells. Cancers (2020) 12(10):2798. doi: 10.3390/cancers12102798
100. Li X. Gap Junction Protein Connexin43 and Tunneling Nanotubes in Human Trabecular Meshwork Cells. Int J Physiology Pathophysiology Pharmacol (2019) 11(5):212–9.
101. Okafo G, Prevedel L, Eugenin E. Tunneling Nanotubes (TNT) Mediate Long-Range Gap Junctional Communication: Implications for HIV Cell to Cell Spread. Sci Rep (2017) 7(1):16660. doi: 10.1038/s41598-017-16600-1
102. Taniguchi Ishikawa E, Gonzalez-Nieto D, Ghiaur G, Dunn SK, Ficker AM, Murali B, et al. Connexin-43 Prevents Hematopoietic Stem Cell Senescence Through Transfer of Reactive Oxygen Species to Bone Marrow Stromal Cells. Proc Natl Acad Sci USA (2012) 109(23):9071–6. doi: 10.1073/pnas.1120358109
103. Nakajima A, Kurihara H, Yagita H, Okumura K, Nakano H. Mitochondrial Extrusion Through the Cytoplasmic Vacuoles During Cell Death. J Biol Chem (2008) 283(35):24128–35. doi: 10.1074/jbc.M802996200
104. Clark MA, Shay JW. Mitochondrial Transformation of Mammalian Cells. Nature (1982) 295(5850):605–7. doi: 10.1038/295605a0
105. Kitani T, Kami D, Matoba S, Gojo S. Internalization of Isolated Functional Mitochondria: Involvement of Macropinocytosis. J Cell Mol Med (2014) 18(8):1694–703. doi: 10.1111/jcmm.12316
106. Nygren JM, Liuba K, Breitbach M, Stott S, Thorén L, Roell W, et al. Myeloid and Lymphoid Contribution to Non-Haematopoietic Lineages Through Irradiation-Induced Heterotypic Cell Fusion. Nat Cell Biol (2008) 10(5):584–92. doi: 10.1038/ncb1721
107. Aguilar PS, Baylies MK, Fleissner A, Helming L, Inoue N, Podbilewicz B, et al. Genetic Basis of Cell-Cell Fusion Mechanisms. Trends Genet TIG (2013) 29(7):427–37. doi: 10.1016/j.tig.2013.01.011
108. Torralba D, Baixauli F, Sánchez-Madrid F. Mitochondria Know No Boundaries: Mechanisms and Functions of Intercellular Mitochondrial Transfer. Front Cell Dev Biol (2016) 4:107. doi: 10.3389/fcell.2016.00107
109. Fransson S, Ruusala A, Aspenström P. The Atypical Rho Gtpases Miro-1 and Miro-2 Have Essential Roles in Mitochondrial Trafficking. Biochem Biophys Res Commun (2006) 344(2):500–10. doi: 10.1016/j.bbrc.2006.03.163
110. Fransson A, Ruusala A, Aspenström P. Atypical Rho Gtpases Have Roles in Mitochondrial Homeostasis and Apoptosis. J Biol Chem (2003) 278(8):6495–502. doi: 10.1074/jbc.M208609200
111. Giot L, Bader JS, Brouwer C, Chaudhuri A, Kuang B, Li Y, et al. A Protein Interaction Map of Drosophila Melanogaster. Sci (New York NY) (2003) 302(5651):1727–36. doi: 10.1126/science.1090289
112. Guo X, Macleod GT, Wellington A, Hu F, Panchumarthi S, Schoenfield M, et al. The Gtpase Dmiro is Required for Axonal Transport of Mitochondria to Drosophila Synapses. Neuron (2005) 47(3):379–93. doi: 10.1016/j.neuron.2005.06.027
113. Russo GJ, Louie K, Wellington A, Macleod GT, Hu F, Panchumarthi S, et al. Drosophila Miro is Required for Both Anterograde and Retrograde Axonal Mitochondrial Transport. J Neurosci Off J Soc Neurosci (2009) 29(17):5443–55. doi: 10.1523/JNEUROSCI.5417-08.2009
114. Glater EE, Megeath LJ, Stowers RS, Schwarz TL. Axonal Transport of Mitochondria Requires Milton to Recruit Kinesin Heavy Chain and is Light Chain Independent. J Cell Biol (2006) 173(4):545–57. doi: 10.1083/jcb.200601067
115. Brickley K, Stephenson FA. Trafficking Kinesin Protein (TRAK)-Mediated Transport of Mitochondria in Axons of Hippocampal Neurons. J Biol Chem (2011) 286(20):18079–92. doi: 10.1074/jbc.M111.236018
116. MacAskill AF, Brickley K, Stephenson FA, Kittler JT. Gtpase Dependent Recruitment of Grif-1 by Miro1 Regulates Mitochondrial Trafficking in Hippocampal Neurons. Mol Cell Neurosciences (2009) 40(3):301–12. doi: 10.1016/j.mcn.2008.10.016
117. van Spronsen M, Mikhaylova M, Lipka J, Schlager MA, van den Heuvel DJ, Kuijpers M, et al. TRAK/Milton Motor-Adaptor Proteins Steer Mitochondrial Trafficking to Axons and Dendrites. Neuron (2013) 77(3):485–502. doi: 10.1016/j.neuron.2012.11.027
118. Birsa N, Norkett R, Higgs N, Lopez-Domenech G, Kittler JT. Mitochondrial Trafficking in Neurons and the Role of the Miro Family of Gtpase Proteins. Biochem Soc Trans (2013) 41(6):1525–31. doi: 10.1042/BST20130234
119. Tang BL. MIRO Gtpases in Mitochondrial Transport, Homeostasis and Pathology. Cells (2015) 5(1):1. doi: 10.3390/cells5010001
120. Wang X, Schwarz TL. The Mechanism of Ca2+ -Dependent Regulation of Kinesin-Mediated Mitochondrial Motility. Cell (2009) 136(1):163–74. doi: 10.1016/j.cell.2008.11.046
121. Yang C, Svitkina TM. Ultrastructure and Dynamics of the Actin-Myosin II Cytoskeleton During Mitochondrial Fission. Nat Cell Biol (2019) 21(5):603–13. doi: 10.1038/s41556-019-0313-6
122. Pathak D, Sepp KJ, Hollenbeck PJ. Evidence That Myosin Activity Opposes Microtubule-Based Axonal Transport of Mitochondria. J Neurosci Off J Soc Neurosci (2010) 30(26):8984–92. doi: 10.1523/JNEUROSCI.1621-10.2010
123. Quintero OA, DiVito MM, Adikes RC, Kortan MB, Case LB, Lier AJ, et al. Human Myo19 is a Novel Myosin That Associates With Mitochondria. Curr Biol CB (2009) 19(23):2008–13. doi: 10.1016/j.cub.2009.10.026
124. Lu Z, Ma XN, Zhang HM, Ji HH, Ding H, Zhang J, et al. Mouse Myosin-19 is a Plus-End-Directed, High-Duty Ratio Molecular Motor. J Biol Chem (2014) 289(26):18535–48. doi: 10.1074/jbc.M114.569087
125. Shneyer BI, Ušaj M, Henn A. Myo19 is an Outer Mitochondrial Membrane Motor and Effector of Starvation-Induced Filopodia. J Cell Sci (2016) 129(3):543–56. doi: 10.1242/jcs.175349
126. Oeding SJ, Majstrowicz K, Hu XP, Schwarz V, Freitag A, Honnert U, et al. Identification of Miro1 and Miro2 as Mitochondrial Receptors for Myosin XIX. J Cell Sci (2018) 131(17):jcs219469. doi: 10.1242/jcs.219469
127. López-Doménech G, Covill-Cooke C, Ivankovic D, Halff EF, Sheehan DF, Norkett R, et al. Miro Proteins Coordinate Microtubule- and Actin-Dependent Mitochondrial Transport and Distribution. EMBO J (2018) 37(3):321–36. doi: 10.15252/embj.201696380
128. Ušaj M, Henn A. Kinetic Adaptation of Human Myo19 for Active Mitochondrial Transport to Growing Filopodia Tips. Sci Rep (2017) 7(1):11596. doi: 10.1038/s41598-017-11984-6
129. Shneyer BI, Ušaj M, Wiesel-Motiuk N, Regev R, Henn A. ROS Induced Distribution of Mitochondria to Filopodia by Myo19 Depends on a Class Specific Tryptophan in the Motor Domain. Sci Rep (2017) 7(1):11577. doi: 10.1038/s41598-017-11002-9
130. Wang ZG, Liu SL, Tian ZQ, Zhang ZL, Tang HW, Pang DW. Myosin-Driven Intercellular Transportation of Wheat Germ Agglutinin Mediated by Membrane Nanotubes Between Human Lung Cancer Cells. ACS Nano (2012) 6(11):10033–41. doi: 10.1021/nn303729r
131. Las G, Shirihai OS. Miro1: New Wheels for Transferring Mitochondria. EMBO J (2014) 33(9):939–41. doi: 10.1002/embj.201488441
132. Babenko VA, Silachev DN, Popkov VA, Zorova LD, Pevzner IB, Plotnikov EY, et al. Miro1 Enhances Mitochondria Transfer From Multipotent Mesenchymal Stem Cells (MMSC) to Neural Cells and Improves the Efficacy of Cell Recovery. Molecules (Basel Switzerland) (2018) 23(3):687. doi: 10.3390/molecules23030687
133. Tseng N, Lambie SC, Huynh CQ, Sanford B, Patel M, Herson PS, et al. Mitochondrial Transfer From Mesenchymal Stem Cells Improves Neuronal Metabolism After Oxidant Injury in Vitro: The Role of Miro1. J Cereb Blood Flow Metab Off J Int Soc Cereb Blood Flow Metab (2021) 41(4):761–70. doi: 10.1177/0271678x20928147
134. Rohn JL, Patel JV, Neumann B, Bulkescher J, McHedlishvili N, McMullan RC, et al. Myo19 Ensures Symmetric Partitioning of Mitochondria and Coupling of Mitochondrial Segregation to Cell Division. Curr Biol CB (2014) 24(21):2598–605. doi: 10.1016/j.cub.2014.09.045
135. Weihofen A, Thomas KJ, Ostaszewski BL, Cookson MR, Selkoe DJ. Pink1 Forms a Multiprotein Complex With Miro and Milton, Linking Pink1 Function to Mitochondrial Trafficking. Biochemistry (2009) 48(9):2045–52. doi: 10.1021/bi8019178
136. Li Y, Lim S, Hoffman D, Aspenstrom P, Federoff HJ, Rempe DA. HUMMR, a Hypoxia- and HIF-1alpha-Inducible Protein, Alters Mitochondrial Distribution and Transport. J Cell Biol (2009) 185(6):1065–81. doi: 10.1083/jcb.200811033
137. Kornmann B, Currie E, Collins SR, Schuldiner M, Nunnari J, Weissman JS, et al. An ER-Mitochondria Tethering Complex Revealed by a Synthetic Biology Screen. Sci (New York NY) (2009) 325(5939):477–81. doi: 10.1126/science.1175088
138. Michel AH, Kornmann B. The ERMES Complex and ER-Mitochondria Connections. Biochem Soc Trans (2012) 40(2):445–50. doi: 10.1042/BST20110758
139. Li B, Zhang Y, Li H, Shen H, Wang Y, Li X, et al. Miro1 Regulates Neuronal Mitochondrial Transport and Distribution to Alleviate Neuronal Damage in Secondary Brain Injury After Intracerebral Hemorrhage in Rats. Cell Mol Neurobiol (2021) 41(4):795–812. doi: 10.1007/s10571-020-00887-2
Keywords: mitochondria, transcellular transport, tunneling nanotubes, extracellular vesicles, Cx43 gap junction, Miro, myosin XIX
Citation: Qin Y, Jiang X, Yang Q, Zhao J, Zhou Q and Zhou Y (2021) The Functions, Methods, and Mobility of Mitochondrial Transfer Between Cells. Front. Oncol. 11:672781. doi: 10.3389/fonc.2021.672781
Received: 26 February 2021; Accepted: 20 April 2021;
Published: 10 May 2021.
Edited by:
Davide Zella, University of Maryland, Baltimore, United StatesReviewed by:
Francesca Benedetti, University of Maryland, United StatesAyse Banu Demir, İzmir University of Economics, Turkey
Copyright © 2021 Qin, Jiang, Yang, Zhao, Zhou and Zhou. This is an open-access article distributed under the terms of the Creative Commons Attribution License (CC BY). The use, distribution or reproduction in other forums is permitted, provided the original author(s) and the copyright owner(s) are credited and that the original publication in this journal is cited, in accordance with accepted academic practice. No use, distribution or reproduction is permitted which does not comply with these terms.
*Correspondence: Yanhong Zhou, emhvdXlhbmhvbmdAY3N1LmVkdS5jbg==; Qiong Zhou, MTM5NzM3ODQzNThAMTYzLmNvbQ==