- 1Molecular Oncology Center, Hospital Sírio-Libanês, São Paulo, Brazil
- 2Ludwig Institute for Cancer Research, University of California San Diego (UCSD), San Diego, CA, United States
There are no effective strategies for the successful treatment of glioblastomas (GBM). Current therapeutic modalities effectively target bulk tumor cells but leave behind marginal GBM cells that escape from the surgical margins and radiotherapy field, exhibiting high migratory phenotype and resistance to all available anti-glioma therapies. Drug resistance is mostly driven by tumor cell plasticity: a concept associated with reactivating transcriptional programs in response to adverse and dynamic conditions from the tumor microenvironment. Autophagy, or “self-eating”, pathway is an emerging target for cancer therapy and has been regarded as one of the key drivers of cell plasticity in response to energy demanding stress conditions. Many studies shed light on the importance of autophagy as an adaptive mechanism, protecting GBM cells from unfavorable conditions, while others recognize that autophagy can kill those cells by triggering a non-apoptotic cell death program, called ‘autophagy cell death’ (ACD). In this review, we carefully analyzed literature data and conclude that there is no clear evidence indicating the presence of ACD under pathophysiological settings in GBM disease. It seems to be exclusively induced by excessive (supra-physiological) stress signals, mostly from in vitro cell culture studies. Instead, pre-clinical and clinical data indicate that autophagy is an emblematic example of the ‘dark-side’ of a rescue pathway that contributes profoundly to a pro-tumoral adaptive response. From a standpoint of treating the real human disease, only combinatorial therapy targeting autophagy with cytotoxic drugs in the adjuvant setting for GBM patients, associated with the development of less toxic and more specific autophagy inhibitors, may inhibit adaptive response and enhance the sensibility of glioma cells to conventional therapies.
Introduction
Glioblastoma (GBM, grade IV astrocytoma) is the most frequent, life-threatening malignant brain tumor and one of the most resilient of all human malignancies. Those tumors are classified and subtyped based on histopathological traits, clinical presentation, and molecular status (1). The current treatment for GBM includes gross neurosurgical resection with the oral use of alkylating agent temozolomide (TMZ), which is given concurrently with radiotherapy (RT) and as an adjuvant monotherapy. Despite aggressive treatments, patients have a low median survival of ~12 months (2–4).
One of the key factors in GBM’s aggressiveness and resilience is their high cell plasticity: a concept associated with phenotype switching, based on the reactivation of transcriptional programs related to the acquisition stem cell properties and the migratory phenotype (5). In the context of anti-glioma therapies, cell plasticity enables tumor cells to change to a cell phenotypic identity, enabling them to survive the dynamic changes of the tumor microenvironment (TME) and to escape surgery and radiotherapy margins by migration. A remarkable example of this plasticity in GBM cells was conceptualized in the “go-or-grow” dichotomous concept in gliomas. It is based on the notion that phenotypically distinct GBM cells (at the “go” or “grow” states) coexist and cooperate to promote tumor growth and clinical relapse: chemoradiation effectively eliminates the bulk population of highly proliferative cells (at the “grow” state), leaving behind a subpopulation of dormant/migratory cells (at the “go” state). “Go” and “grow” states are completely reversible insofar as GBM cells change their phenotypes without genetic mutations. This plasticity is controlled by different signaling pathways that drive adaptive responses and emerge as a non-genetic source of functional intratumoral heterogeneity that, ultimately, mirror tumor resiliency and high patient mortality (2, 6, 7).
Autophagy (greek “self-eating”) is a good example of signaling pathway associated with the phenotype switching and metabolic flexibility of GBM cells. It is primarily a degradative pathway characterized as a fast route by which damaged cytoplasmic materials (collectively named ‘cargo’) are delivered to the lysosomes for recycling. Autophagy can be categorized into 3 subtypes called, micro-autophagy, macro-autophagy, and chaperone-mediated autophagy (for more detailed insights into the different autophagic pathways see (8–10).
Macroautophagy (hereafter referred to as autophagy) must take place on a baseline in each cell to withdraw damaged and functionless organelles, providing metabolites to synthetic pathways and sustaining energetic homeostasis. In the brain, baseline autophagy is important as a clearance mechanism of disease-related proteins in neurons and also in astrocytes, and autophagy dysfunction may contribute to the progression of neurodegenerative diseases (11). However, a selective activation of autophagy can be observed in various pathophysiological and/or stress situations (12–15). For example, in normal brain Beclin-1 (BECN1), a gene with a central role in autophagy induction (16), was not expressed by neurons or glial cells, but showed strong cytoplasmic overexpression in primary GBM cells (17). Moreover, in response to standard of care in patients with GBM (radio- and chemotherapy), the autophagy pathway is upregulated giving tumor cells an advantage for survival. In a series of clinicopathological studies, cancer cells exhibit an increased autophagy activity linked with poor prognosis and aggressive clinical behavior (17–19). Those are emblematic examples of the ‘dark-side’ of autophagy, acting as a therapy-responsive mechanism associated with a pro-tumoral adaptive response (20–25).
On the other hand, there are numerous reports, mostly from in vitro cell-based studies, showing an anti-tumoral function of autophagy. Those reports have clearly shown that excessive activation of the autophagy by prolonged or supraphysiological doses of stress signals, may lead to massive removal of cytosolic material, leading to a specific type of non-apoptotic cell death, named type II programmed cell death, or autophagic cell death (ACD). ACD is characterized by large-scale autophagic vacuolization of the cytoplasm in the absence of chromatin condensation and can be specifically blocked by the inhibition of autophagy-related genes (ATG) (26, 27). Due to this ‘dual’ role in human cancers cells, autophagy is, therefore, often been described metaphorically as a ‘double-edged sword’ in cancers. Importantly, the studies that explore the mechanisms of ACD are mostly from in vitro cell-based approaches, which provide us a precious source of mechanistic insights, but are of limited translational relevance. Of note, there is no doubt that GBM cells activate autophagy shortly before or during their death in according to the external cues or internal stimuli received, but it is still controversial whether this activation contributes to cell death or rather represents a last attempt of survival.
So, to understand the real effect of autophagy in GBM disease is necessary to analyze cancer cells under normal pathophysiological conditions and therapeutic doses. In the next sections, we will focus on the specific extracellular signals that surround tumors and play an important role in controlling autophagy in GBM cells. Important is the notion that our particular emphasis was given to studies that evaluate the relationship between autophagy and GBM from a perspective of understanding and treating human disease. Therefore, studies using in vitro cell-based models, inducing ACD by excessive stress signals, were not fully considered here, except for the mechanistic data.
Autophagy Activation as a Response to Pathophysiological Stress
Necrosis and acidic stress are the most important stress signals in GBM microenvironment related with autophagy activation. Tumor necrosis is a histological hallmark of grade IV astrocytic tumors with prevalence in almost 90% of patients with GBM (1, 28, 29). Necrosis appears as either multifocal areas (micronecrosis) or broad necrotic areas surrounded by hyperproliferative zones of tumor cells, called perinecrotic niches (PNN), which is visible as a soft, gray rim surrounding necrotic areas by magnetic resonance imaging (MRI). During disease progression and treatment response, GBM cells have to change their metabolism to survive in PNN, characterized by intermittent hypoxia (defined by low oxygen levels, pO2 < 3%) and starvation conditions due to poor functional vasculature (30, 31). This configuration is indirectly linked to poor patient outcome and associated with radio and TMZ resistance (32, 33). Hypoxia, per se, is well known to create radiation and chemotherapy resistances (34). As part of the physiological adaptive response, the PNN stimulates the stabilization of hypoxia-induced factors (HIFs), HIF1a and HIF2a, resulting in a driving force for activation of anti-apoptotic and pro-migratory transcriptional programs, supporting angiogenesis (35, 36), and re-expression of markers and properties typical of glioma stem cells (GSCs) (30, 37–42). Interestingly, hypoxia, starvation and conventional anti-glioma therapies stimulate the onset of autophagy above baseline levels in GBM cells.
Hypoxic conditions also shift GBM cells towards aerobic glycolysis, rather than mitochondrial oxidative phosphorylation, promoting an acidic environment, potentially favoring tumor invasion by pH-dependent activation of proteinases (e.g. heparanases and cathepsins) (43). Heparanase (HPSE) is an endo-β-D-glucuronidase that has both enzymatic and non-enzymatic functionalities in a pH-dependent manner. HPSE expression is intrinsically correlated with GBM progression, worse prognosis (44), and cell invasion (45). Intriguingly, autophagy is one of the cellular mechanisms regulated by heparanase activity in various tumors, including brain tumors (46). Notably, autophagy induced by starvation in GBM cells was prevented by the use of a potent heparanase inhibitor. Moreover, in these cells the pro-tumorigenic function of heparanase is mediated by autophagy activation, enhancing chemotherapy resistance in nutrition-stressed environments. The mechanism underlying heparanase-induced autophagy is not fully understood but appears to involve mTOR1 inhibition, which plays a pivotal role in nutrient-sensing and autophagy regulation in vitro (47).
Cathepsins belong to a class of cysteine proteinases that is mainly expressed by GSC subpopulations of IDH wild-type GBM patients (48). Cathepsins can be secreted into the extracellular space and have an optimum activity on acidic environments to further activate MMP proenzymes (49), with have an important role in controlling tumor cell invasion, stem cell phenotypes (50–53) and tumor progression (54). Cathepsin D levels, for example, are strongly and positively correlated with LC3A and LC3B expression in GBM patients (markers for autophagosome levels) (17). Moreover, inhibition of Cathepsin D attenuates autophagy, leading to increased radiosensitivity in GBM cells. In radioresistant cells, Cathepsin D has been positively correlated with LC3-II and negatively correlated with p62 (55), a protein that targets specific cargoes for autophagy (56). The expression levels of another member of family, the Cathepsin L, are higher in GBM compared to low-grade gliomas (57), exerting an important role in migratory phenotype (51, 52, 58, 59) and γ-radiation-induced GBM cell invasion (59). Interestingly, autophagy inhibition by trifluoperazine induces radiosensitivity in GBM cells mediated by Cathepsin L downregulation (60).
Interestingly, at PNN (i.e. under physiological hypoxia), autophagy activation via BNIP3/BNIP3L is a survival mechanism that promotes GBM progression and resistance to anticancer therapies in vivo (61). Recently, a global analysis conducted by Bronisz et al. that included 41 GBM patient’s cohort identified the autophagy pathway as the unique de-regulated pathway in PNNs of primary GBMs (32). These analyses indicate that poorly perfused tumor regions are likely to have increased baseline autophagic levels and, therefore, under hypoxic conditions, the increased autophagic flux may play an adaptive role (62, 63). Under hypoxia, autophagy is activated by BECN1 phosphorylation via the HIF-1a/BECN1 signaling pathway, one of the initial steps in the assembly of autophagosomes from pre-autophagic structures (64–66). Moreover, PNN in GBM disease also show the overexpression of interleukin 6 (IL6), an inflammatory cytokine that is essential for hypoxia-induced autophagy and induction of invasive programs in GBM cells (67–71). At this point, is important to notice that under in vitro prolonged hypoxic stress (48-72h, <1% pO2), the gene BNIP3 (Bcl-2/adenovirus E1B 19kDa-interacting protein 3), a pro-apoptotic Bcl-2 family member, is upregulated, leading to hypoxia-dependent ACD in GBM cells (72). Mechanistically, BNIP3 upregulation releases BECN1 from the complexes with Bcl-2 or Bcl-xL, allowing BECN1 to activate autophagy (73). It becomes especially critical to note that the nature of the autophagic response to hypoxia - a cytoprotective or cytotoxic output - depends on the extent and duration of the microenvironmental stressor, on the experimental design, as well as, on the genetic background of the tumor cells.
Alternative forms to GBM cells to adapt or to avoid poor oxygenation and hostile microenvironment are through the vasculogenic mimicry (VM) phenomenon (74) and the activation of migratory programs by altering the composition of the TME (75–77). VM represents an impressive example of a higher phenotype flexibility of GBM cells. GBM cells capable of VM formation organize themselves into functional vascular-like structures, ensuring tumor blood supply independently of normal blood vessels or angiogenesis. In this scenario, it has been shown that VM formation in glioma patients was associated with the expression of BECN1 (16).
Moreover, as a part of adaptive programs, VM formation is also promoted by Bevacizumab (BVZ)-induced autophagy in GSC, an anti-VEGF antibody that received accelerated approval by the FDA to treat recurrent GBM (78), which is associated with tumor resistance to antiangiogenic therapy (see below) (79). VM was also associated with high expression of HIF-1α (80) and upregulation of the IL-8/CXCR2 pathway (81). It is also conceivable that autophagy may contribute to the increased production of multiple pro-invasive cytokines, including interleukin-6 (IL-6) and -8 (IL-8), which, in turn, may reactivate a pro-invasive and GSC transcriptional programs, leading GBM cells to the “go” state, allowing them to migrate away from cytotoxic niches towards a supportive microenvironment (69, 82).
Decorin (DCN), a member of the small leucine-rich proteoglycans (PGs) family, has a vital role in the hypoxia-dependent activation of autophagy and anti-glioma therapy resistance, mainly due to their binding to VEGFR2 expressed by vECs, particularly in PNNs of glioma samples, or with the binding to c-Met and EGFR receptors expressed by GBM cells (83, 84). High levels of c-Met or DCN correlate with shorter progression-free survival (PFS) and overall survival (OS) in patients with GBM (85–88). The high-affinity DCN/receptor interaction leads to increased expression of paternally expressed gene 3 (Peg3), that physically associates with BECN1, recruiting LC3 into autophagosomes (89, 90). Complementarily, in GSC-enriched environments, GBM cells produce a high amount of PGs, such as DCN and Lumican, promoting chemotherapy resistance and cell survival (91). Curiously, as observed in several types of non-central nervous system tumors (92, 93), soluble DCN potently induces autophagy in GBM cells and contributes to an impairment of GBM cell migration in vitro experiments (94). Other extracellular matrix (ECM) proteins, such as endostatin, perlecan, and endorepellin, can influence tumor progression by regulating autophagy levels in endothelial cells, controlling vessel formation and neo-angiogenesis in response to hypoxia (95, 96).
Autophagy Activation as a Response to Physiological Signals
Once PNN and other stress signals reactivate migration programs to drive plasticity and invasiveness in GBM cells, invasive growth along specific anatomical structures, especially at the vasculature and white matter tracts, is regarded as the main cause of poor therapeutic outcome of patients with GBMs. The migration occurs at perivascular niches (PVN), besides PNN, and considered the preferred and fastest route for GBM cell invasion through brain tissue (97). PVNs are fluid-filled spaces, continuous to the subarachnoid space, surrounding all blood vessels in the brain, including capillaries and arterioles. Based on histological information and in situ experiments, a widely accepted idea is that GBM cells actively seek out PVNs and migrate along with them (98, 99). For example, bradykinin, produced by cerebral vascular endothelial cells (vEC), acts as a strong chemotactic signaling peptide, guiding GBM cells toward PVN. Therefore, when injected into mice brain, the vast majority (over 85%) of human GBM cells move into contact with a blood vessel (100). At PVN, cerebral vECs are in the closest proximity to tumor cells. This heterotypic interaction induces a GSC transdifferentiation, which is critical for the malignant traits of the disease and supports the notion that stemness is a temporary reversible trait of GBM cells. The GSC phenotype is maintained by vECs via mediators, such as nitric oxide (NO), cyclic guanosine monophosphate (cGMP), and Notch1 ligands (97, 101–104). The stemness phenotype has been recently associated to autophagy activation and is one of the most important processes in the PVN responsible for the maintenance of GSC status besides PNN (105, 106). Additionally, the interaction between GBM cells and pericytes at PVN leads to chaperone-mediated autophagy in normal pericytes, building an immunosuppressed microenvironment that induces GSC phenotype and tumor growth (107). Interestingly, activation of protective autophagy in cerebral vECs is one of the essential physiological processes responsible for maintaining vascular homeostasis, and playing an important role in vECs proliferation, migration, and tube formation (108, 109). Other types of vEC-derived molecules also promote autophagy and correlate with stemness in GBM cells. For example, osteopontin (OPN), derived from the vEC, plays an oncogenic role and initiates a stem-promoting cascade and enhances autophagy through an integrin-CD44 dependent activation of HIF genes at PVNs (110, 111). OPN-elicited autophagy could promote cancer cell survival, resistance to chemotherapy drugs, and has been associated with increased glioma grade and migratory potential (112).
The melanoma-differentiation associated protein 9 (MDA-9, also called Syntenin-1) is another ECM protein that sponsors tumor invasion mainly by regulating the cell surface receptor Syndecan (113). In GBM, MDA-9 expression is an important regulator of cell invasion (114), stemness phenotype, and survival of GSCs through STAT3 and Notch1 pathways, respectively (115). Interestingly, the MDA-9 is responsible for activating protective autophagy in GSCs in vitro through the EGFR/FAK and EGFR/PKC axis, inhibiting anoikis (a suspension-induced form of apoptosis) by the hyperphosphorylation of Bcl-2 (116). In this scenario, autophagy often is activated in these cells as a compensatory pro-survival adaptation to detachment stress. In such cases, autophagy precedes (and usually avoids) anoikis by removing pro-apoptotic proteins in the cytosol. For example, depletion of ATG5 or ATG7 inhibits detachment-induced autophagy and enhances anoikis (117, 118). A higher expression of MDA-9 has been linked to higher glioma grade and short-term survival (119).
Autophagy Activation as a Response to Anti-Glioma Therapies
RT plus concomitant and maintenance TMZ is the gold standard treatment and represent a major advance in the field of therapy for high-grade gliomas (7, 120). The addition of BVZ to standard treatment revealed an improvement in progression-free interval but had no effect on OS (121). Intriguingly, virtually all glioma therapies, including RT, TMZ and/or BVZ, are stronger inducers of autophagy pathway: several pre-clinical and clinicopathological studies indicate that increased autophagy activity help to desensitize GBM cells to treatment and it is linked with poor prognosis in different cancers (21). Inversely, others observations shown that excessive intensification of autophagic process lead to cell exhaustion and death (26, 62, 72, 122). So, despite the potential ‘dual’ role of autophagy has been clearly observed in cell-based studies, in ‘real’ disease, the predominant data conduct to the idea that therapy-induced autophagy is acting as an adaptive response and a protective mechanism in GBM cells instead of eliciting cell death.
The study of Natsumeda et al. (2011) is probably the first to show the induction of autophagy by TMZ in glioma cells and in reactive astrocytes of glioma patients by immunohistochemical analysis, indicating some type of stress response in tumor and normal cells (22). The addition of chloroquine (CQ) and its derivative hydroxychloroquine (HCQ) – both inhibitors of autophagy by blocking autophagosome fusion and degradation - to TMZ-treated glioma cells attenuates autophagy flux, induces accumulation of the proautophagy proteins (LC3-II) and promotes endoplasmic reticulum stress and cleavage of PARP (a marker of apoptosis) (123). Many other studies observed that blocking autophagosome formation enhances TMZ cytotoxicity, indicating that the autophagy pathway may protect GBM cells from TMZ-induced cytotoxicity (25, 123). For example, it has been demonstrated that CQ plus TMZ significantly increased the amounts of cleaved PARP (a marker for apoptosis) over those cells treated with TMZ alone. The pharmacological inhibition of autophagy by CQ also negatively dictates the migratory capacity of GBM cells, corroborating the role of autophagy with other aspects of adaptive phenotype and cell plasticity (124). While other authors have suggested that autophagy is the main component of TMZ-induced cytotoxicity and that inhibition of the autophagy significantly influences the antitumor effect of TMZ in vitro (20).
Ionizing radiation is the gold-standard adjuvant treatment for GBM. Radiotherapy also results in enhanced autophagy in GBM cells in vitro (125). When irradiated, many GBM cells undergo cell death by apoptosis, whereas GBM cells that do not undergo apoptosis activate autophagy, suggesting a protective mechanism (24, 125).
It has also been demonstrated that CQ treatments can increase radiosensitivity in GBM cells (25). Moreover, CQ worked synergistically with radiotherapy for induction of apoptosis in GSC; thereby acting as a protective mechanism (126). Another study showed that DNA-protein kinase-deficient GBM cells (DNA-PK), an enzyme that plays a critical role in DNA double-strand breaks repair, underwent massive ACD even after low doses of γ-radiation in cell lines in vitro. Intact DNA-PK pathway prevented ACD, but cells still exhibited a low apoptotic tendency, indicating that genetic background takes a leading role on the sensitivity of treatment and cell fate determination (127).
Another example of therapy-induced autophagy occurs after the use of antiangiogenic therapies in GBM. The addition of BVZ to conventional chemoradiation improved the PFS but did not affect OS (121). At the TME level, BVZ induces a hypoxic niche that results in protective autophagy sponsoring GBM cell resistance and survival. Alternatively, BVZ induced autophagy directly in GBM cells by suppressing the Akt-mTOR signaling pathway (128). Furthermore, BVZ-mediated autophagy is also dependent on interferon regulatory factor 1 (IRF1) expression in gliomas (129). Moreover, GBM cells expressing the stem cell markers CD133 and Sox2, and residing in the PVN, internalize BVZ through micropinocytosis, leading to autophagy activation and cell survival (130). Autophagy inhibition by ATG7 silencing rescued GBM sensitivity to BVZ treatments (131).
Autophagy Activation as a Response to Internal Stimuli
Autophagy in GBM cells is triggered in response to external or internal stimuli. Internal stimuli is manifested directly by alterations in ATG or indirectly by oncogenic proteins commonly found aberrantly expressed in GBM and lower-grade gliomas. The following subsections cover the most important genetic events for gliomagenesis and their specific genetic aberrations associated with autophagy activation.
There are 16 known ATG in humans, four of which (ATG2B, ATG5, ATG9B and ATG12) are frequently mutated in gastric and colorectal cancers, and in hepatocellular carcinoma, and may be causally associated with cancer development by deregulating the autophagy process (132, 133). Large-scale genomic analysis indicates that core autophagy genes are generally not mutated in patients of 11 human cancers, including GBM, suggesting that the autophagy machinery is functional in cancer types investigated (134, 135). At a clinical perspective, several ATG signatures have been emerging as important prognostic factors for GBM patients, and autophagy high scores have been related to worse outcomes (136–138). For example, Wang and colleagues described that a robust 14-mRNA prognostic signature was an independent prognostic factor associated with OS in GBM’s patients (HR=1.9, 95% CI = 1.013-3.644, p value = 0.045) (136). Moreover, several other research groups have correlated the higher expression of ATGs with glioma aggressiveness, including patient’s poor survival and tumor progression (139–143). Despite their prognostic relevance, for future clinical applications, it is also important to integrate with other types of signatures (such as protein signatures).
Large-scale genomic studies showed that primary GBM arises from defects in three main molecular signaling pathways involving p53, Rb, and phosphoinositide 3-kinase (PI3K) (144). The phosphatidylinositol 3-kinase (PI3K)/Akt/mammalian target of rapamycin (mTOR) cascade is recognized as an important sensor of nutrient/growth factor availability and a major pathway regulating autophagy in human cancers. In a permissive microenvironment, active PI3K/Akt/mTOR cascade constitutively suppresses autophagosomes biogenesis by inactivating the ATG1/ULK1 complex or by sequestration and inactivation of BECN1, both considered key initiators of the autophagic pathway (145, 146). Inhibitors of Akt/mTOR activity, such as rapamycin analogs, intensify the autophagic process (147). However, under stressful conditions, PI3K/Akt/mTOR cascade is normally inactivated through extracellular signals, like intermittent hypoxia and depletion of nutrients, leading to the extrinsic activation of protective autophagy. Nevertheless, in GBM samples, activation of PI3K/Akt/mTOR cascade is observed in almost 90% of the cases, and caused by the overexpression of upstream activators, like epidermal growth factor receptor (EGFR) or c-Met, activating mutations of PI3CA (p110) or PIK3R1 (P85) (148–150), and inactivating mutations in the phosphatase and tensin homolog (PTEN), a negative regulator of PI3K activity (loss-of-function mutations in PTEN are present in almost 60-85% of GBMs) (151, 152). Moreover, the use of a potent PI3K inhibitor promotes autophagy activation at the expense of invasion and angiogenesis impairment in GBM cells. Furthermore, PI3K inhibition also restrained tumor growth and significantly prolonged mouse survival (153). In addition, GBM cells harboring mTOR hyper-activation, showed an increment of autophagy after the use of rapamycin (154).
The deregulation of the tumor protein p53 (TP53) pathway accounts for approximately 85% of GBMs, including alterations on CDKN2A, MDM2 and TP53 genes (155). Members of this signaling pathway have been described as modulators of migration, invasion, proliferation, and stemness, leading to poor prognosis in GBM patients (155). Regarding autophagy activation, nuclear p53 induces the expression of the ATGs: DRAM, and Sestrins 1/2. Indeed, DRAM1 is considered the regulator of the autophagy activation mediated by nuclear p53 (156–158), promoting migration and invasion of glioma stem cells (141). Interestingly, cytoplasmic p53 inhibits autophagy, but external stressors, such as nutrition starvation, induces the destruction of cytoplasmic p53, sustaining autophagy activation (159, 160). More recently, it has been shown that combined therapy with TMZ and CQ synergistically reduces cell proliferation and enhances apoptosis in p53-wild type cells. Overexpression of mutant p53 abolishes the autophagic vacuoles (161).
The Retinoblastoma gene (RB1) is a tumor suppressor gene commonly mutated or deleted in GBM and correlated with lower survival rates in astrocytomas patients (162, 163). Functionally, Rb inhibits cell cycle progression and promotes cell survival by controlling the function of the E2F transcription factor (164). Besides cell cycle transition control, Rb also influences tumor cell differentiation, senescence, apoptosis, and autophagy (165). Indeed, Rb downstream effector E2F1 directly mediates the expression of the autophagy-related genes LC3, ATG1, and DRAM (166). In GBM cells, it has been shown that Rb binds to E2F, repressing its activity, and leading to autophagy induction. Indeed, Rb activity or E2F1 silencing induced autophagic flux through increased autophagosome formation (167). Interestingly, while the binding of Rb to E2F promotes the activation of autophagy, Rb phosphorylation represses its binding to E2F and leads to apoptosis activation (168). In this scenario, it has already been shown that the Rb-E2F axis regulates the expression of the BNIP3 gene, an essential gene that mediates hypoxia-induced autophagy, promoting autophagosome formation in nutrient-deficient environments (169). Rb-induced autophagy is considered a resistance mechanism in GBM cells treated with etoposide or cisplatin (170, 171).
The most relevant and frequent oncogenic alterations in GBM patients involve the Epidermal growth factor receptor (EGFR), comprising 57% of patients. These alterations include mutations, rearrangements, amplifications, and splicing variants that lead to enhanced tumor growth, angiogenesis, survival, and stemness (148, 172). Intriguingly, due to the functional impact of EGFR alterations on tumor aggressiveness, lower- grade gliomas harboring EGFR amplification are considered “GBM-like tumors” due their aggressive phenotypic behavior (173). Beyond the known pathological role of EGFR on GBMs, their functions in autophagy regulation are emerging, indicating that it directly acts as a controller of the autophagic flux by mTOR signaling modulation (174, 175). EGFR-mediated autophagy exerts relevant roles in gliomagenesis, tumor progression, and therapy resistance (176). Clinically, GBM patients with low levels of EGFR and high expression of BECN1 have a median overall survival of 30 months, presenting a favorable response to radiotherapy (177). Therapeutically, the combination of tyrosine kinase inhibitors (TKI), such as erlotinib, with CQ increases the antineoplastic effect of the TKI on apoptosis-resistant GBM cells (178). Surprisingly, another EGFR inhibitor, called BIBU, impaired Akt and STAT3 activation, induced apoptosis death, and activated protective autophagy (179). The constitutively active mutant allele of EGFR, known as EGFRvIII is an important mediator of autophagy (180). It occurs in 20–30% of all human GBM, making it the most common EGFR mutant in GBM (181, 182). EGFRvIII- expressing GBMs are intrinsically resistant to apoptosis induced by radio- and chemotherapy (183, 184). Interestingly, these tumors have autophagy over-activation under hypoxic conditions and patients benefit from the use of CQ (180). Intriguingly, GBM cells harboring EGFRvIII alterations are more sensitive to the pharmacological inhibition of mTOR (185).
c-MET (also called HGFR) is a type of Receptor Tyrosine Kinase mutated in 6% and amplified in 4% of patients with GBM, leading to constitutive activity. Patients harboring c-MET gain-of-function alterations present a shorter survival and poor response to treatment (186). The enhancement of c-Met activity induces GBM cell survival, proliferation, invasion, angiogenesis, and stemness (187). The intracellular pathway triggered by c-MET is PI3K/Akt signaling. Additionally, cell invasion mediated by c-MET relies on Focal Adhesion Kinase (FAK) activity (188). Interestingly, c-MET expression was correlated with autophagy activation in GSCs, positively regulating their migratory and invasive capacity (141). c-MET expression abrogation by epigenetic silencing in glioma cells suppresses Akt pathway activation and up-regulates the expression of the autophagy-related protein Atg5, resulting in tumor growth reduction (189).
Isocitrate dehydrogenase 1 and 2 (IDH1/2) mutations are the most important molecular markers in diffuse gliomas due to their high impact on patient survival improvement and tumor development (190, 191). IDH1 mutations (IDHmut) are present in more than 80% of low-grade gliomas (grades II-III) and in secondary GBMs, but are rare in primary GBMs (190, 192). Mutations in IDH2 have been found in fewer than 3% of glial tumors. Patients with lower-grade gliomas (grades II-III) and glioblastoma show significantly longer OS in the presence of IDH1 or IDH2 mutations (192). The prognostic importance of IDH mutation is independent of other known prognostic factors, including age, grade, and MGMT methylation status. IDH mutations promote a metabolic reprogramming mainly due to the accumulation of the oncometabolite 2- hydroxyglutarate (2-HG), which, in turn, induces the epigenetic silencing of several genes from the glycolytic pathway (193, 194). Moreover, IDH1mut is associated with a distinct hypoxia/angiogenesis transcriptome signature and stabilization of HIF-1a levels in glioma cells (195), important autophagy regulators (see above). Recently, four different groups identified distinct autophagy signatures with prognostic value in GBMs. High autophagy risk signatures were correlated with patients’ worse outcomes. Besides the absence of gene intersection between the signatures, all four achieve the same results: patients with IDHmut tumors presented a lower autophagy-related risk signature compared to IDH wild-type (IDHwt) gliomas, denoting an increased autophagy activation in IDHwt GBMs (136, 137, 196, 197). In the same direction, beyond the gene signatures, it has been shown that higher expression levels of the ATG proteins: LC3, Beclin-1, and p62 are more prevalent in IDHwt gliomas in than IDHmut gliomas (139).
Promoter methylation of the O-6-Methylguanine-DNA Methyltransferase (MGMT) gene is a prognostic marker in patients with glioma because MGMT methylation leads to better response to alkylating agents, such as TMZ (198). Indeed, patients harboring MGMT-methylated GBMs had a 10-month and 4-month higher median overall and PFS, respectively, compared with MGMT-non-methylated patients (199). Interestingly, two different groups showed that MGMT-methylated gliomas presented a lower autophagy risk score compared with MGMT-non-methylated patients (136, 137). In agreement with these data, GBM cell lines that naturally do not express MGMT, highly activate autophagy after TMZ treatment. However, when cells were stably transfected with MGMT, the number of autophagic vacuoles was abrogated after TMZ treatment (122).
Finally, beyond the role of oncogenes, tumor suppressor genes and their downstream signaling molecules, the control of autophagy activation in GBM also relies on the signaling pathways involved in stemness (200). These pathways are mainly involved in the acquisition and maintenance of the GSC phenotype, including Notch, Wnt/β-catenin, and Hedgehog pathways. In gliomas, the activation of Notch signaling correlates with more aggressive tumor phenotypes (201). Besides activation of the Notch pathway, its members and ligands are rarely mutated in GBMs (202). The connection between the Notch pathway and autophagy was first described in U87MG and U251 GBM cell lines. When the Notch1 receptor was genetically silenced in these cells, they showed reduced proliferation and viability. GBM growth impairment was correlated with the augmented expression of the autophagy-related proteins Beclin-1 and LC3-II in NOTCH1-silenced cells (203). Complementarily, when autophagy was induced in GSCs by mTOR inhibition, the Notch1 receptor was degraded. Indeed, the impairment of Notch1 signaling induced by autophagy activation led to a decreased tumorigenicity and self-renewal capacity of GSCs (204). Interestingly, the degradation of Notch1 by autophagy is mediated via autophagosome-precursor vesicles positively expressing the autophagy-related protein ATG16L1 (205), and by the direct binding of p62 to Notch1 Intracellular Domain (NICD) (206). In contrast, the pharmacological blockage of Notch1 induces cytoprotective autophagy in GBM cells. However, when these cells were exposed to the combination of Notch1 and autophagy inhibitors, treatment resistance was overcome, thus augmenting apoptotic cell death (207). Interestingly, Notch1 signals can be regulated by autophagy activation via ATG16L1-positive autophagosomes, modulating stem cell development, and neurogenesis (205).
Wnt signaling plays a critical role in GSC phenotype, therapeutic resistance and invasiveness (208, 209). Mutations in the members of Wnt signaling pathway are not common, but epigenetic alterations are frequently observed in GBMs (210). The inhibition of Wnt signaling by the IWR-1 inhibitor leads to an augment of the expression of the autophagy-related proteins, LC3-II, and Beclin-1 (211). Complementarily, another group showed that the silencing of the intracellular players of Wnt signaling, TCF4, and CTNNB1/β-catenin, induced the up-regulation of SQSTM1/p62, increasing the autophagy flux. Interestingly, Wnt pathway inhibition sensitizes GBM cells to autophagy inhibition with CQ (212). Regarding chemotherapy response to TMZ, the blocking of the Wnt/β -catenin pathway by the activity of the DAB2IP protein is responsible for TMZ resistance through the expression of the autophagy-related protein ATG9B. Interestingly, the combination of TMZ with a Wnt signaling inhibitor can overcome this resistance (213). Indeed, autophagy activation mediated by nutrient starvation in GBM cells down-regulates several mediators Wnt signaling, including activated β-catenin (214).
Hedgehog (Hh) signaling enhances the migratory and invasive capacity of cells through the activation of PI3K/Akt pathway in GBM cells (215). Moreover, it stimulates the growth and tumorigenicity of gliomas, mainly by controlling stemness status (216). Besides the lack of mutations on Hh pathway components in GBMs, it has been shown that the glioma-associated oncogene homolog 1 (GLI1) zinc-finger transcription factors, terminal effectors of the Hh pathway, presents two tumor-specific splicing isoforms, which directly influences tumor malignancy (217). Intriguingly, the activation of Hh signaling is correlated with the modulation of autophagy in several cancer types. Indeed, the inhibition of the Hh pathway negatively controls tumor proliferation by activating autophagy (218). In GBM cells, the use of GANT-61, a specific inhibitor of Gli1 and Gli2, activates autophagy, inducing LC3-II expression, and by negatively modulating the expression of stemness markers and tumor proliferation (219). Pharmacologically, the use of GANT-61 enhances the cytotoxic effect of TMZ by the increment of acid vesicles and Beclin-1 expression (220). Furthermore, the regulatory domain of PTCH1, the main receptor of the Hedgehog pathway, interacts physically with the autophagy-related protein ATG101 in a nutrient starvation microenvironment, inhibiting the autophagic process (221). Additionally, GBM cells overexpressing the stem marker SOX3 showed an upregulation in Hh pathway activity and suppression of autophagy, leading to an increment in proliferation and invasion (222).
The Last Frontier: The Therapeutic Potential of Autophagy Inhibitors for the Treatment of GBM
GBM retains a poor prognostic value and remains incurable. Despite our growing understanding of the mechanisms underlying drug resistance, the standard therapy has not changed over the last 16 years (7). Up to date, no new therapies improve OS when added to standard therapy, with an exception for the recent Tumor Treating Fields (TTFields) in GBM (223). As we see above, under pathophysiological circumstances, autophagy is a key driver of GBM resistance, allowing cellular adaptive survival towards extrinsic (e.g. hypoxia, drugs or ionizing radiation) or intrinsic (genetic aberrations) stress stimuli (Figure 1). Accordingly, from a standpoint of treating GBM disease, targeting autophagy emerge as a new potential therapy and it has been considered a potential candidate to improve the treatment of patients with GBM (224, 225). In this scenario, the use of the autophagy inhibitors, such as CQ or HCQ, has been explored in clinical studies. Those trials mainly focused on the therapeutic potential of autophagy inhibition combined to standard therapies for GBM patients.
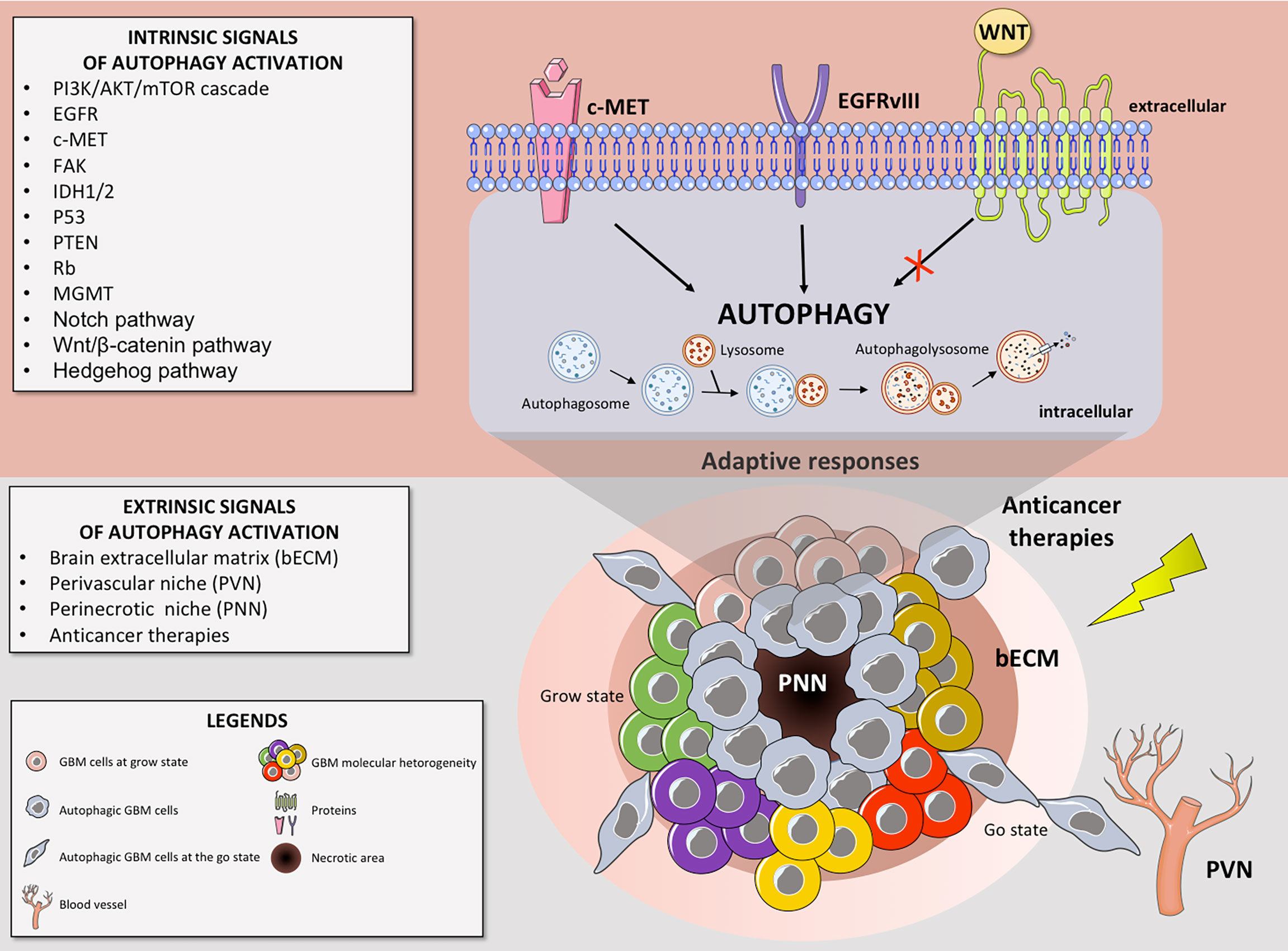
Figure 1 Autophagy can be triggered by intrinsic and/or extrinsic GBM cells signals, contributing to tumor cell proliferation (grow state), invasion (go state) and resistance to therapies. Thus, autophagy may function as a mechanism of tumor cell survival and progression in a hostile microenvironment. The intrinsic signals activating autophagy in GBM consist of specific gene expression levels alterations (like in the cMET gene), mutations (like the mutation in the EGFR gene that gives rise to the active mutant EGFRvIII) and/or specific signaling pathways perturbations (such as in the Wnt pathway). The extrinsic signals associated with autophagy activation in GBM cells are: 1) the perinecrotic niches (PNN), composed by highly proliferative GBM cells and where the autophagy activation may function as a cellular adaptive response to hypoxia; 2) the perivascular niche (PVN), where the vascular endothelial cells can interact with the GBM ones, inducing autophagy and a stemness phenotype of those tumor cells; 3) the brain extracellular matrix (bECM), whose components may regulate autophagy; and 4) the anticancer therapies, which can activate autophagy as a cytoprotective mechanism.
A small phase III trial observed a median overall survival of 24 months for patients treated with CQ plus conventional therapy (i.e. surgery, radiotherapy, and carmustine-based chemotherapy) compared to 11 months for patients treated with conventional therapy (226). In a single institutional study with 123 patients, the same authors showed that the addition of CQ to surgery, radiotherapy, and carmustine-based chemotherapy consistently exerts an adjuvant effect, adding more than 13 months in patients’ median survival in comparison to control patients (227). However, despite the favorable results in GBM patients treated with CQ combined to surgery, radiotherapy, and carmustine, a phase I/II trial combining HCQ with radiotherapy and TMZ-based chemotherapy showed that the maximum tolerated dose of HCQ was unable to consistently inhibit autophagy and showed no improvement in patient OS (228). To transpose those issues, a new phase I/II trial (NCT02432417) was designed to compare patients treated with concurrent ionizing radiation and TMZ- based chemotherapy with patients treated with this combination plus a most appropriate CQ dose. Indeed, recent data published by the group showed that 200 mg of CQ is a feasible dose to use in those patients, since 400 mg of CQ induced several severe adverse events. Moreover, preliminary data analysis showed an improvement of more than 9 months in the OS of GBM patients harboring EGFRvIII alterations compared with patients without this genetic variant (229). The International Cooperative Phase III Trial is an active clinical trial that evaluates the use of CQ or Valproic acid as an adjuvant to conventional therapy in high-grade gliomas (NCT03243461).
Due to these positive results in the early clinical trials, it is essential to invest in studies to evaluate CQ effects on GBM patients’ survival using larger cohorts. Moreover, it is also necessary to determine HCQ efficacy and tolerated doses and invest in discovering new drugs with similar action mechanisms.
Future Directions: Crossing the Valley of Death
The discrepancy between pro- and anti-tumor functions of autophagy, modulating GBM cell plasticity or alternative mechanism of cell death, emphasizes a question that has emerged as critical in translational science: how wide gap exists between basic and clinical biomedical data? The establishment of interdisciplinary research institutes stimulating collaborations between clinicians, physician-scientists, and basic biologists are critical to bring these areas together, but the importance of the critical interpretive reviews of literature data is also fundamental.
By examining carefully the literature we realize that explanations for the controversies of whether the autophagy pathway promotes survival or death are still elusive. Sometimes the balance between autophagic-dependent pro-survival or pro-death signals depends greatly on the quantitative relationship between them: over to moderate level of autophagy activation is cytoprotective, whereas high levels of autophagy are cytotoxic. Sometimes there are even conflicting reports with the same drug treatment in the same experimental model. Pre-clinical and clinical data indicate that autophagy is an emblematic example of a rescue pathway that contributes profoundly to a pro-tumoral adaptive response. On the other hand, high levels of activation lead to cytotoxic autophagy, which seems to be exclusively induced by excessive and homogeneous stress signals from in vitro cell-based studies.
From a standpoint of understanding the real GBM disease, the spatial and temporal heterogeneity of the external and internal stimuli must be considered. Steep gradients in pO2, pH, nutrient availability and drug perfusions ranging from pathological/therapeutic conditions to those found in normal tissues, added to the high levels of genetic intratumoral heterogeneity, are hallmark features of GBM. Thus, it implies that the levels of autophagy activation may also show extensive spatial heterogeneity in subpopulations from the same tumor determining divergent cell fates.
Despite the ability of many compounds, like CQ and HCQ, to inhibit autophagy and demonstrated good efficacy in preclinical studies, clinical trials for GBM continue showing no significant survival or clinical benefit, due to sparse anti-glioma activity or severe side effects. Thus, the last frontier to test the therapeutic potential of autophagy pathway in GBM awaits the development of compounds that can achieve more consistent inhibition.
Finally, only combinatorial therapy targeting autophagy with cytotoxic drugs in the adjuvant setting for GBM patients, associated with the development of less toxic and higher specific autophagy inhibitors, may inhibit adaptive response and enhance the sensibility of glioma cells to conventional therapies. In the context of an incurable human disease, pharmacological inhibition of autophagy would represent a promisor therapeutic target for radio- and chemosensitization of GBM cells.
Author Contributions
EHFJ, MB, and LTI wrote the review and designed the figures. FBF and AAC critically revised the final version and ETC edited and supervised the whole work. All authors contributed to the article and approved the submitted version.
Funding
This work was supported by grants from FAPESP (16/07463-4, 16/06857-9), Ludwig Institute for Cancer Research and Hospital Sírio-Libanês.
Conflict of Interest
The authors declare that the research was conducted in the absence of any commercial or financial relationships that could be construed as a potential conflict of interest.
References
1. Louis DN, Perry A, Reifenberger G, von Deimling A, Figarella-Branger D, Cavenee WK, et al. The 2016 World Health Organization Classification of Tumors of the Central Nervous System: A Summary. Acta Neuropathol (2016) 131(6):803–20. doi: 10.1007/s00401-016-1545-1
2. Furnari FB, Fenton T, Bachoo RM, Mukasa A, Stommel JM, Stegh A, et al. Malignant Astrocytic Glioma: Genetics, Biology, and Paths to Treatment. Genes Dev (2007) 21(21):2683–710. doi: 10.1101/gad.1596707
3. Lowe S, Bhat KP, Olar A. Current Clinical Management of Patients With Glioblastoma. Cancer Rep (Hoboken) (2019) 2(6):e1216. doi: 10.1002/cnr2.1216
4. Tan AC, Ashley DM, López GY, Malinzak M, Friedman HS, Khasraw M. Management of Glioblastoma: State of the Art and Future Directions. CA Cancer J Clin (2020) 70(4):299–312. doi: 10.3322/caac.21613
5. Shen S, Clairambault J. Cell Plasticity in Cancer Cell Populations. F1000Res (2020) 9:F1000. doi: 10.12688/f1000research.24803.1
6. Ostrom QT, Cioffi G, Gittleman H, Patil N, Waite K, Kruchko C, et al. CBTRUS Statistical Report: Primary Brain and Other Central Nervous System Tumors Diagnosed in the United States in 2012-2016. Neuro Oncol (2019) 21(Suppl 5):v1–v100. doi: 10.1093/neuonc/noz150
7. Stupp R, Mason WP, van den Bent MJ, Weller M, Fisher B, Taphoorn MJ, et al. Radiotherapy Plus Concomitant and Adjuvant Temozolomide for Glioblastoma. N Engl J Med (2005) 352(10):987–96. doi: 10.1056/NEJMoa043330
8. Andrade-Tomaz M, de Souza I, Rocha CRR, Gomes LR. The Role of Chaperone-Mediated Autophagy in Cell Cycle Control and Its Implications in Cancer. Cells (2020) 9(9):2140. doi: 10.3390/cells9092140
9. Auzmendi-Iriarte J, Matheu A. Impact of Chaperone-Mediated Autophagy in Brain Aging: Neurodegenerative Diseases and Glioblastoma. Front Aging Neurosci (2020) 12:630743. doi: 10.3389/fnagi.2020.630743
10. Coelho BP, Fernandes CFL, Boccacino JM, Souza MCDS, Melo-Escobar MI, Alves RN, et al. Multifaceted WNT Signaling at the Crossroads Between Epithelial-Mesenchymal Transition and Autophagy in Glioblastoma. Front Oncol (2020) 10:597743. doi: 10.3389/fonc.2020.597743
11. Sung K, Jimenez-Sanchez M. Autophagy in Astrocytes and its Implications in Neurodegeneration. J Mol Biol (2020) 432(8):2605–21. doi: 10.1016/j.jmb.2019.12.041
12. Teckman JH, Perlmutter DH. Retention of Mutant Alpha(1)-Antitrypsin Z in Endoplasmic Reticulum is Associated With an Autophagic Response. Am J Physiol Gastrointest Liver Physiol (2000) 279(5):G961–74. doi: 10.1152/ajpgi.2000.279.5.G961
13. Elmore SP, Qian T, Grissom SF, Lemasters JJ. The Mitochondrial Permeability Transition Initiates Autophagy in Rat Hepatocytes. FASEB J (2001) 15(12):2286–7. doi: 10.1096/fj.01-0206fje
14. Xue L, Fletcher GC, Tolkovsky AM. Mitochondria are Selectively Eliminated From Eukaryotic Cells After Blockade of Caspases During Apoptosis. Curr Biol (2001) 11(5):361–5. doi: 10.1016/s0960-9822(01)00100-2
15. Kondo Y, Kanzawa T, Sawaya R, Kondo S. The Role of Autophagy in Cancer Development and Response to Therapy. Nat Rev Cancer (2005) 5(9):726–34. doi: 10.1038/nrc1692
16. Duan S. Silencing the Autophagy-Specific Gene Beclin-1 Contributes to Attenuated Hypoxia-Induced Vasculogenic Mimicry Formation in Glioma. Cancer Biomark (2018) 21(3):565–74. doi: 10.3233/cbm-170444
17. Giatromanolaki A, Sivridis E, Mitrakas A, Kalamida D, Zois CE, Haider S, et al. Autophagy and Lysosomal Related Protein Expression Patterns in Human Glioblastoma. Cancer Biol Ther (2014) 15(11):1468–78. doi: 10.4161/15384047.2014.955719
18. Sivridis E, Koukourakis MI, Zois CE, Ledaki I, Ferguson DJ, Harris AL, et al. LC3A-Positive Light Microscopy Detected Patterns of Autophagy and Prognosis in Operable Breast Carcinomas. Am J Pathol (2010) 176(5):2477–89. doi: 10.2353/ajpath.2010.090049
19. Koukourakis MI, Kalamida D, Mitrakas A, Pouliliou S, Kalamida S, Sivridis E, et al. Intensified Autophagy Compromises the Efficacy of Radiotherapy Against Prostate Cancer. Biochem Biophys Res Commun (2015) 461(2):268–74. doi: 10.1016/j.bbrc.2015.04.014
20. Kanzawa T, Germano IM, Komata T, Ito H, Kondo Y, Kondo S. Role of Autophagy in Temozolomide-Induced Cytotoxicity for Malignant Glioma Cells. Cell Death Differ (2004) 11(4):448–57. doi: 10.1038/sj.cdd.4401359
21. Sui X, Chen R, Wang Z, Huang Z, Kong N, Zhang M, et al. Autophagy and Chemotherapy Resistance: A Promising Therapeutic Target for Cancer Treatment. Cell Death Dis (2013) 4:e838. doi: 10.1038/cddis.2013.350
22. Natsumeda M, Aoki H, Miyahara H, Yajima N, Uzuka T, Toyoshima Y, et al. Induction of Autophagy in Temozolomide Treated Malignant Gliomas. Neuropathology (2011) 31(5):486–93. doi: 10.1111/j.1440-1789.2010.01197.x
23. Min Z, Ting Y, Mingtao G, Xiaofei T, Dong Y, Chenguang Z, et al. Monitoring Autophagic Flux Using p62/SQSTM1 Based Luciferase Reporters in Glioma Cells. Exp Cell Res (2018) 363(1):84–94. doi: 10.1016/j.yexcr.2017.12.027
24. Ito H, Daido S, Kanzawa T, Kondo S, Kondo Y. Radiation-Induced Autophagy is Associated With LC3 and its Inhibition Sensitizes Malignant Glioma Cells. Int J Oncol (2005) 26(5):1401–10. doi: 10.3892/ijo.26.5.1401
25. Yuan X, Du J, Hua S, Zhang H, Gu C, Wang J, et al. Suppression of Autophagy Augments the Radiosensitizing Effects of STAT3 Inhibition on Human Glioma Cells. Exp Cell Res (2015) 330(2):267–76. doi: 10.1016/j.yexcr.2014.09.006
26. Kroemer G, Levine B. Autophagic Cell Death: The Story of a Misnomer. Nat Rev Mol Cell Biol (2008) 9(12):1004–10. doi: 10.1038/nrm2529
27. Galluzzi L, Vitale I, Aaronson SA, Abrams JM, Adam D, Agostinis P, et al. Molecular Mechanisms of Cell Death: Recommendations of the Nomenclature Committee on Cell Death 2018. Cell Death Differ (2018) 25(3):486–541. doi: 10.1038/s41418-017-0012-4
28. Noch E, Khalili K. Molecular Mechanisms of Necrosis in Glioblastoma: The Role of Glutamate Excitotoxicity. Cancer Biol Ther (2009) 8(19):1791–7. doi: 10.4161/cbt.8.19.9762
29. Barker FG, Davis RL, Chang SM, Prados MD. Necrosis as a Prognostic Factor in Glioblastoma Multiforme. Cancer (1996) 77(6):1161–6. doi: 10.1002/(sici)1097-0142(19960315)77:6<1161::aid-cncr24>3.0.co;2-z
30. Rong Y, Durden DL, Van Meir EG, Brat DJ. ‘Pseudopalisading’ Necrosis in Glioblastoma: A Familiar Morphologic Feature That Links Vascular Pathology, Hypoxia, and Angiogenesis. J Neuropathol Exp Neurol (2006) 65(6):529–39. doi: 10.1097/00005072-200606000-00001
31. Monteiro AR, Hill R, Pilkington GJ, Madureira PA. The Role of Hypoxia in Glioblastoma Invasion. Cells (2017) 6(4):45. doi: 10.3390/cells6040045
32. Bronisz A, Salińska E, Chiocca EA, Godlewski J. Hypoxic Roadmap of Glioblastoma-Learning About Directions and Distances in the Brain Tumor Environment. Cancers (Basel) (2020) 12(5):1213. doi: 10.3390/cancers12051213
33. Jawhari S, Ratinaud MH, Verdier M. Glioblastoma, Hypoxia and Autophagy: A Survival-Prone ‘Ménage-À-Trois’. Cell Death Dis (2016) 7(10):e2434. doi: 10.1038/cddis.2016.318
34. Semenza GL. Targeting HIF-1 for Cancer Therapy. Nat Rev Cancer (2003) 3(10):721–32. doi: 10.1038/nrc1187
35. Li Z, Bao S, Wu Q, Wang H, Eyler C, Sathornsumetee S, et al. Hypoxia-Inducible Factors Regulate Tumorigenic Capacity of Glioma Stem Cells. Cancer Cell (2009) 15(6):501–13. doi: 10.1016/j.ccr.2009.03.018
36. Wolf A, Agnihotri S, Guha A. Targeting Metabolic Remodeling in Glioblastoma Multiforme. Oncotarget (2010) 1(7):552–62. doi: 10.18632/oncotarget.190
37. Jensen RL, Mumert ML, Gillespie DL, Kinney AY, Schabel MC, Salzman KL. Preoperative Dynamic Contrast-Enhanced MRI Correlates With Molecular Markers of Hypoxia and Vascularity in Specific Areas of Intratumoral Microenvironment and is Predictive of Patient Outcome. Neuro Oncol (2014) 16(2):280–91. doi: 10.1093/neuonc/not148
38. Teicher BA. Hypoxia and Drug Resistance. Cancer Metastasis Rev (1994) 13(2):139–68. doi: 10.1007/bf00689633
39. Harris AL. Hypoxia–a Key Regulatory Factor in Tumour Growth. Nat Rev Cancer (2002) 2(1):38–47. doi: 10.1038/nrc704
40. Persano L, Pistollato F, Rampazzo E, Della Puppa A, Abbadi S, Frasson C, et al. BMP2 Sensitizes Glioblastoma Stem-Like Cells to Temozolomide by Affecting HIF-1α Stability and MGMT Expression. Cell Death Dis (2012) 3:e412. doi: 10.1038/cddis.2012.153
41. Ge X, Pan MH, Wang L, Li W, Jiang C, He J, et al. Hypoxia-Mediated Mitochondria Apoptosis Inhibition Induces Temozolomide Treatment Resistance Through miR-26a/Bad/Bax Axis. Cell Death Dis (2018) 9(11):1128. doi: 10.1038/s41419-018-1176-7
42. Pope WB, Xia Q, Paton VE, Das A, Hambleton J, Kim HJ, et al. Patterns of Progression in Patients With Recurrent Glioblastoma Treated With Bevacizumab. Neurology (2011) 76(5):432–7. doi: 10.1212/WNL.0b013e31820a0a8a
43. Swietach P, Vaughan-Jones RD, Harris AL. Regulation of Tumor Ph and the Role of Carbonic Anhydrase 9. Cancer Metastasis Rev (2007) 26(2):299–310. doi: 10.1007/s10555-007-9064-0
44. Kundu S, Xiong A, Spyrou A, Wicher G, Marinescu VD, Edqvist PD, et al. Heparanase Promotes Glioma Progression and Is Inversely Correlated With Patient Survival. Mol Cancer Res (2016) 14(12):1243–53. doi: 10.1158/1541-7786.Mcr-16-0223
45. Tran VM, Wade A, McKinney A, Chen K, Lindberg OR, Engler JR, et al. Heparan Sulfate Glycosaminoglycans in Glioblastoma Promote Tumor Invasion. Mol Cancer Res (2017) 15(11):1623–33. doi: 10.1158/1541-7786.Mcr-17-0352
46. Sanderson RD, Elkin M, Rapraeger AC, Ilan N, Vlodavsky I. Heparanase Regulation of Cancer, Autophagy and Inflammation: New Mechanisms and Targets for Therapy. FEBS J (2017) 284(1):42–55. doi: 10.1111/febs.13932
47. Shteingauz A, Boyango I, Naroditsky I, Hammond E, Gruber M, Doweck I, et al. Heparanase Enhances Tumor Growth and Chemoresistance by Promoting Autophagy. Cancer Res (2015) 75(18):3946–57. doi: 10.1158/0008-5472.Can-15-0037
48. Koh SP, Wickremesekera AC, Brasch HD, Marsh R, Tan ST, Itinteang T. Expression of Cathepsins B, D, and G in Isocitrate Dehydrogenase-Wildtype Glioblastoma. Front Surg (2017) 4:28. doi: 10.3389/fsurg.2017.00028
49. Yadati T, Houben T, Bitorina A, Shiri-Sverdlov R. Physiological Function and Role in Disease Management. Cells (2020) 9(7):1679. doi: 10.3390/cells9071679
50. Bischof J, Westhoff MA, Wagner JE, Halatsch ME, Trentmann S, Knippschild U, et al. Cancer Stem Cells: The Potential Role of Autophagy, Proteolysis, and Cathepsins in Glioblastoma Stem Cells. Tumour Biol (2017) 39(3):1010428317692227. doi: 10.1177/1010428317692227
51. Formolo CA, Williams R, Gordish-Dressman H, MacDonald TJ, Lee NH, Hathout Y. Secretome Signature of Invasive Glioblastoma Multiforme. J Proteome Res (2011) 10(7):3149–59. doi: 10.1021/pr200210w
52. Gole B, Huszthy PC, Popović M, Jeruc J, Ardebili YS, Bjerkvig R, et al. The Regulation of Cysteine Cathepsins and Cystatins in Human Gliomas. Int J Cancer (2012) 131(8):1779–89. doi: 10.1002/ijc.27453
53. Pišlar A, Jewett A, Kos J. Cysteine Cathepsins: Their Biological and Molecular Significance in Cancer Stem Cells. Semin Cancer Biol (2018) 53:168–77. doi: 10.1016/j.semcancer.2018.07.010
54. Sivaparvathi M, Sawaya R, Chintala SK, Go Y, Gokaslan ZL, Rao JS. Expression of Cathepsin D During the Progression of Human Gliomas. Neurosci Lett (1996) 208(3):171–4. doi: 10.1016/0304-3940(96)12584-2
55. Zheng W, Chen Q, Wang C, Yao D, Zhu L, Pan Y, et al. Inhibition of Cathepsin D (CTSD) Enhances Radiosensitivity of Glioblastoma Cells by Attenuating Autophagy. Mol Carcinog (2020) 59(6):651–60. doi: 10.1002/mc.23194
56. Lamark T, Kirkin V, Dikic I, Johansen T. NBR1 and p62 as Cargo Receptors for Selective Autophagy of Ubiquitinated Targets. Cell Cycle (2009) 8(13):1986–90. doi: 10.4161/cc.8.13.8892
57. Sivaparvathi M, Yamamoto M, Nicolson GL, Gokaslan ZL, Fuller GN, Liotta LA, et al. Expression and Immunohistochemical Localization of Cathepsin L During the Progression of Human Gliomas. Clin Exp Metastasis (1996) 14(1):27–34. doi: 10.1007/bf00157683
58. Levicar N, Dewey RA, Daley E, Bates TE, Davies D, Kos J, et al. Selective Suppression of Cathepsin L by Antisense cDNA Impairs Human Brain Tumor Cell Invasion In Vitro and Promotes Apoptosis. Cancer Gene Ther (2003) 10(2):141–51. doi: 10.1038/sj.cgt.7700546
59. Xiong Y, Ji W, Fei Y, Zhao Y, Wang L, Wang W, et al. Cathepsin L is Involved in X-ray-induced Invasion and Migration of Human Glioma U251 Cells. Cell Signal (2017) 29:181–91. doi: 10.1016/j.cellsig.2016.10.012
60. Zhang X, Xu R, Zhang C, Xu Y, Han M, Huang B, et al. Trifluoperazine, a Novel Autophagy Inhibitor, Increases Radiosensitivity in Glioblastoma by Impairing Homologous Recombination. J Exp Clin Cancer Res (2017) 36(1):118. doi: 10.1186/s13046-017-0588-z
61. Wu J, Lei Z, Yu J. Hypoxia Induces Autophagy in Human Vascular Endothelial Cells in a Hypoxia-Inducible Factor 1−Dependent Manner. Mol Med Rep (2015) 11(4):2677–82. doi: 10.3892/mmr.2014.3093
62. Denton D, Nicolson S, Kumar S. Cell Death by Autophagy: Facts and Apparent Artefacts. Cell Death Differ (2012) 19(1):87–95. doi: 10.1038/cdd.2011.146
63. Park SY, Sun EG, Lee Y, Kim MS, Kim JH, Kim WJ, et al. Autophagy Induction Plays a Protective Role Against Hypoxic Stress in Human Dental Pulp Cells. J Cell Biochem (2018) 119(2):1992–2002. doi: 10.1002/jcb.26360
64. Lu N, Li X, Tan R, An J, Cai Z, Hu X, et al. HIF-1α/Beclin1-Mediated Autophagy is Involved in Neuroprotection Induced by Hypoxic Preconditioning. J Mol Neurosci (2018) 66(2):238–50. doi: 10.1007/s12031-018-1162-7
65. Menon MB, Dhamija S. Beclin 1 Phosphorylation - at the Center of Autophagy Regulation. Front Cell Dev Biol (2018) 6:137. doi: 10.3389/fcell.2018.00137
66. Qian X, Li X, Cai Q, Zhang C, Yu Q, Jiang Y, et al. Phosphoglycerate Kinase 1 Phosphorylates Beclin1 to Induce Autophagy. Mol Cell (2017) 65(5):917–31. doi: 10.1016/j.molcel.2017.01.027
67. Rolhion C, Penault-Llorca F, Kémény JL, Lemaire JJ, Jullien C, Labit-Bouvier C, et al. Interleukin-6 Overexpression as a Marker of Malignancy in Human Gliomas. J Neurosurg (2001) 94(1):97–101. doi: 10.3171/jns.2001.94.1.0097
68. Joseph JV, Conroy S, Pavlov K, Sontakke P, Tomar T, Eggens-Meijer E, et al. Hypoxia Enhances Migration and Invasion in Glioblastoma by Promoting a Mesenchymal Shift Mediated by the HIF1α-ZEB1 Axis. Cancer Lett (2015) 359(1):107–16. doi: 10.1016/j.canlet.2015.01.010
69. Xue H, Yuan G, Guo X, Liu Q, Zhang J, Gao X, et al. A Novel Tumor-Promoting Mechanism of IL6 and the Therapeutic Efficacy of Tocilizumab: Hypoxia-induced IL6 is a Potent Autophagy Initiator in Glioblastoma Via the p-STAT3-MIR155-3p-CREBRF Pathway. Autophagy (2016) 12(7):1129–52. doi: 10.1080/15548627.2016.1178446
70. Xue H, Zhang J, Guo X, Wang J, Li J, Gao X, et al. CREBRF is a Potent Tumor Suppressor of Glioblastoma by Blocking Hypoxia-Induced Autophagy Via the CREB3/ATG5 Pathway. Int J Oncol (2016) 49(2):519–28. doi: 10.3892/ijo.2016.3576
71. Liu Q, Li G, Li R, Shen J, He Q, Deng L, et al. IL-6 Promotion of Glioblastoma Cell Invasion and Angiogenesis in U251 and T98G Cell Lines. J Neurooncol (2010) 100(2):165–76. doi: 10.1007/s11060-010-0158-0
72. Azad MB, Chen Y, Henson ES, Cizeau J, McMillan-Ward E, Israels SJ, et al. Hypoxia Induces Autophagic Cell Death in Apoptosis-Competent Cells Through a Mechanism Involving BNIP3. Autophagy (2008) 4(2):195–204. doi: 10.4161/auto.5278
73. Bellot G, Garcia-Medina R, Gounon P, Chiche J, Roux D, Pouysségur J, et al. Hypoxia-Induced Autophagy is Mediated Through Hypoxia-Inducible Factor Induction of BNIP3 and BNIP3L Via Their BH3 Domains. Mol Cell Biol (2009) 29(10):2570–81. doi: 10.1128/mcb.00166-09
74. López-Camarillo C, Ruiz-García E, Starling N, Marchat LA. Editorial: Neovascularization, Angiogenesis and Vasculogenic Mimicry in Cancer. Front Oncol (2020) 10:1140. doi: 10.3389/fonc.2020.01140
75. Ulrich TA, de Juan Pardo EM, Kumar S. The Mechanical Rigidity of the Extracellular Matrix Regulates the Structure, Motility, and Proliferation of Glioma Cells. Cancer Res (2009) 69(10):4167–74. doi: 10.1158/0008-5472.CAN-08-4859
76. Giese A, Kluwe L, Laube B, Meissner H, Berens ME, Westphal M. Migration of Human Glioma Cells on Myelin. Neurosurgery (1996) 38(4):755–64. doi: 10.1227/00006123-199604000-00026
77. Bellail AC, Hunter SB, Brat DJ, Tan C, Van Meir EG. Microregional Extracellular Matrix Heterogeneity in Brain Modulates Glioma Cell Invasion. Int J Biochem Cell Biol (2004) 36(6):1046–69. doi: 10.1016/j.biocel.2004.01.013
78. Kreisl TN, Kim L, Moore K, Duic P, Royce C, Stroud I, et al. Phase II Trial of Single-Agent Bevacizumab Followed by Bevacizumab Plus Irinotecan at Tumor Progression in Recurrent Glioblastoma. J Clin Oncol (2009) 27(5):740–5. doi: 10.1200/jco.2008.16.3055
79. Wu HB, Yang S, Weng HY, Chen Q, Zhao XL, Fu WJ, et al. Autophagy-Induced KDR/VEGFR-2 Activation Promotes the Formation of Vasculogenic Mimicry by Glioma Stem Cells. Autophagy (2017) 13(9):1528–42. doi: 10.1080/15548627.2017.1336277
80. Angara K, Rashid MH, Shankar A, Ara R, Iskander A, Borin TF, et al. Vascular Mimicry in Glioblastoma Following Anti-Angiogenic and Anti-20-HETE Therapies. Histol Histopathol (2017) 32(9):917–28. doi: 10.14670/HH-11-856
81. Angara K, Borin TF, Rashid MH, Lebedyeva I, Ara R, Lin PC, et al. CXCR2-Expressing Tumor Cells Drive Vascular Mimicry in Antiangiogenic Therapy-Resistant Glioblastoma. Neoplasia (2018) 20(10):1070–82. doi: 10.1016/j.neo.2018.08.011
82. West AJ, Tsui V, Stylli SS, Nguyen HPT, Morokoff AP, Kaye AH, et al. The Role of Interleukin-6-STAT3 Signalling in Glioblastoma. Oncol Lett (2018) 16(4):4095–104. doi: 10.3892/ol.2018.9227
83. Gubbiotti MA, Iozzo RV. Proteoglycans Regulate Autophagy Via Outside-in Signaling: An Emerging New Concept. Matrix Biol (2015) 48:6–13. doi: 10.1016/j.matbio.2015.10.002
84. Neill T, Buraschi S, Kapoor A, Iozzo RV. Proteoglycan-Driven Autophagy: A Nutrient-Independent Mechanism to Control Intracellular Catabolism. J Histochem Cytochem (2020) 68(11):733–46. doi: 10.1369/0022155420937370
85. Petterson SA, Dahlrot RH, Hermansen SK, K A Munthe S, Gundesen MT, Wohlleben H, et al. High Levels of c-Met is Associated With Poor Prognosis in Glioblastoma. J Neurooncol (2015) 122(3):517–27. doi: 10.1007/s11060-015-1723-3
86. Olmez OF, Cubukcu E, Evrensel T, Kurt M, Avci N, Tolunay S, et al. The Immunohistochemical Expression of c-Met is an Independent Predictor of Survival in Patients With Glioblastoma Multiforme. Clin Transl Oncol (2014) 16(2):173–7. doi: 10.1007/s12094-013-1059-4
87. Liu W, Fu Y, Xu S, Ding F, Zhao G, Zhang K, et al. c-Met Expression is Associated With Time to Recurrence in Patients With Glioblastoma Multiforme. J Clin Neurosci (2011) 18(1):119–21. doi: 10.1016/j.jocn.2010.05.010
88. Tsidulko AY, Kazanskaya GM, Volkov AM, Suhovskih AV, Kiselev RS, Kobozev VV, et al. Chondroitin Sulfate Content and Decorin Expression in Glioblastoma are Associated With Proliferative Activity of Glioma Cells and Disease Prognosis. Cell Tissue Res (2020) 379(1):147–55. doi: 10.1007/s00441-019-03127-2
89. Buraschi S, Neill T, Goyal A, Poluzzi C, Smythies J, Owens RT, et al. Decorin Causes Autophagy in Endothelial Cells Via Peg3. Proc Natl Acad Sci USA (2013) 110(28):E2582–91. doi: 10.1073/pnas.1305732110
90. Buraschi S, Neill T, Iozzo RV. Decorin is a Devouring Proteoglycan: Remodeling of Intracellular Catabolism Via Autophagy and Mitophagy. Matrix Biol (2019) 75-76:260–70. doi: 10.1016/j.matbio.2017.10.005
91. Farace C, Oliver JA, Melguizo C, Alvarez P, Bandiera P, Rama AR, et al. Microenvironmental Modulation of Decorin and Lumican in Temozolomide-Resistant Glioblastoma and Neuroblastoma Cancer Stem-Like Cells. PloS One (2015) 10(7):e0134111. doi: 10.1371/journal.pone.0134111
92. Bi X, Pohl NM, Qian Z, Yang GR, Gou Y, Guzman G, et al. Decorin-Mediated Inhibition of Colorectal Cancer Growth and Migration is Associated With E-Cadherin In Vitro and in Mice. Carcinogenesis (2012) 33(2):326–30. doi: 10.1093/carcin/bgr293
93. Stock C, Jungmann O, Seidler DG. Decorin and Chondroitin-6 Sulfate Inhibit B16V Melanoma Cell Migration and Invasion by Cellular Acidification. J Cell Physiol (2011) 226(10):2641–50. doi: 10.1002/jcp.22612
94. Yao T, Zhang CG, Gong MT, Zhang M, Wang L, Ding W. Decorin-Mediated Inhibition of the Migration of U87MG Glioma Cells Involves Activation of Autophagy and Suppression of TGF-β Signaling. FEBS Open Bio (2016) 6(7):707–19. doi: 10.1002/2211-5463.12076
95. Douglass S, Goyal A, Iozzo RV. The Role of Perlecan and Endorepellin in the Control of Tumor Angiogenesis and Endothelial Cell Autophagy. Connect Tissue Res (2015) 56(5):381–91. doi: 10.3109/03008207.2015.1045297
96. Neill T, Andreuzzi E, Wang ZX, Peiper SC, Mongiat M, Iozzo RV. Endorepellin Remodels the Endothelial Transcriptome Toward a Pro-Autophagic and Pro-Mitophagic Gene Signature. J Biol Chem (2018) 293(31):12137–48. doi: 10.1074/jbc.RA118.002934
97. Calabrese C, Poppleton H, Kocak M, Hogg TL, Fuller C, Hamner B, et al. A Perivascular Niche for Brain Tumor Stem Cells. Cancer Cell (2007) 11(1):69–82. doi: 10.1016/j.ccr.2006.11.020
98. Seano G. Targeting the Perivascular Niche in Brain Tumors. Curr Opin Oncol (2018) 30(1):54–60. doi: 10.1097/cco.0000000000000417
99. Cuddapah VA, Robel S, Watkins S, Sontheimer H. A Neurocentric Perspective on Glioma Invasion. Nat Rev Neurosci (2014) 15(7):455–65. doi: 10.1038/nrn3765
100. Montana V, Sontheimer H. Bradykinin Promotes the Chemotactic Invasion of Primary Brain Tumors. J Neurosci (2011) 31(13):4858–67. doi: 10.1523/jneurosci.3825-10.2011
101. Hossain A, Gumin J, Gao F, Figueroa J, Shinojima N, Takezaki T, et al. Mesenchymal Stem Cells Isolated From Human Gliomas Increase Proliferation and Maintain Stemness of Glioma Stem Cells Through the IL-6/gp130/STAT3 Pathway. Stem Cells (2015) 33(8):2400–15. doi: 10.1002/stem.2053
102. Charles N, Holland EC. The Perivascular Niche Microenvironment in Brain Tumor Progression. Cell Cycle (2010) 9(15):3012–21. doi: 10.4161/cc.9.15.12710
103. Diksin M, Smith SJ, Rahman R. The Molecular and Phenotypic Basis of the Glioma Invasive Perivascular Niche. Int J Mol Sci (2017) 18(11):2342. doi: 10.3390/ijms18112342
104. Schiffer D, Annovazzi L, Casalone C, Corona C, Mellai M. Glioblastoma: Microenvironment and Niche Concept. Cancers (Basel) (2018) 11(1):5. doi: 10.3390/cancers11010005
105. Molina ML, García-Bernal D, Martinez S, Valdor R. Autophagy in the Immunosuppressive Perivascular Microenvironment of Glioblastoma. Cancers (Basel) (2019) 12(1):102. doi: 10.3390/cancers12010102
106. Clarke AJ, Simon AK. Autophagy in the Renewal, Differentiation and Homeostasis of Immune Cells. Nat Rev Immunol (2019) 19(3):170–83. doi: 10.1038/s41577-018-0095-2
107. Valdor R, García-Bernal D, Riquelme D, Martinez CM, Moraleda JM, Cuervo AM, et al. Glioblastoma Ablates Pericytes Antitumor Immune Function Through Aberrant Up-Regulation of Chaperone-Mediated Autophagy. Proc Natl Acad Sci USA (2019) 116(41):20655–65. doi: 10.1073/pnas.1903542116
108. Nussenzweig SC, Verma S, Finkel T. The Role of Autophagy in Vascular Biology. Circ Res (2015) 116(3):480–8. doi: 10.1161/circresaha.116.303805
109. Ma Y, Wang P, Xue Y, Qu C, Zheng J, Liu X, et al. PVT1 Affects Growth of Glioma Microvascular Endothelial Cells by Negatively Regulating Mir-186. Tumour Biol (2017) 39(3):1010428317694326. doi: 10.1177/1010428317694326
110. Pietras A, Katz AM, Ekström EJ, Wee B, Halliday JJ, Pitter KL, et al. Osteopontin-CD44 Signaling in the Glioma Perivascular Niche Enhances Cancer Stem Cell Phenotypes and Promotes Aggressive Tumor Growth. Cell Stem Cell (2014) 14(3):357–69. doi: 10.1016/j.stem.2014.01.005
111. Moorman HR, Poschel D, Klement JD, Lu C, Redd PS, Liu K. Osteopontin: A Key Regulator of Tumor Progression and Immunomodulation. Cancers (Basel) (2020) 12(11):3379. doi: 10.3390/cancers12113379
112. Jan HJ, Lee CC, Shih YL, Hueng DY, Ma HI, Lai JH, et al. Osteopontin Regulates Human Glioma Cell Invasiveness and Tumor Growth in Mice. Neuro Oncol (2010) 12(1):58–70. doi: 10.1093/neuonc/nop013
113. Pradhan AK, Maji S, Das SK, Emdad L, Sarkar D, Fisher PB. Mda-9/Syntenin/SDCBP: New Insights Into a Unique Multifunctional Scaffold Protein. Cancer Metastasis Rev (2020) 39(3):769–81. doi: 10.1007/s10555-020-09886-7
114. Kegelman TP, Das SK, Hu B, Bacolod MD, Fuller CE, Menezes ME, et al. MDA-9/syntenin is a Key Regulator of Glioma Pathogenesis. Neuro Oncol (2014) 16(1):50–61. doi: 10.1093/neuonc/not157
115. Talukdar S, Das SK, Pradhan AK, Emdad L, Shen XN, Windle JJ, et al. Novel Function of MDA-9/Syntenin (SDCBP) as a Regulator of Survival and Stemness in Glioma Stem Cells. Oncotarget (2016) 7(34):54102–19. doi: 10.18632/oncotarget.10851
116. Talukdar S, Pradhan AK, Bhoopathi P, Shen XN, August LA, Windle JJ, et al. MDA-9/Syntenin Regulates Protective Autophagy in Anoikis-Resistant Glioma Stem Cells. Proc Natl Acad Sci USA (2018) 115(22):5768–73. doi: 10.1073/pnas.1721650115
117. Fung C, Lock R, Gao S, Salas E, Debnath J. Induction of Autophagy During Extracellular Matrix Detachment Promotes Cell Survival. Mol Biol Cell (2008) 19(3):797–806. doi: 10.1091/mbc.e07-10-1092
118. Chen C, Kapoor A, Iozzo RV. Methods for Monitoring Matrix-Induced Autophagy. Methods Mol Biol (2019) 1952:157–91. doi: 10.1007/978-1-4939-9133-4_14
119. Bacolod MD, Das SK, Sokhi UK, Bradley S, Fenstermacher DA, Pellecchia M, et al. Examination of Epigenetic and Other Molecular Factors Associated With Mda-9/Syntenin Dysregulation in Cancer Through Integrated Analyses of Public Genomic Datasets. Adv Cancer Res (2015) 127:49–121. doi: 10.1016/bs.acr.2015.04.006
120. Stupp R, Hegi ME, Mason WP, van den Bent MJ, Taphoorn MJ, Janzer RC, et al. Effects of Radiotherapy With Concomitant and Adjuvant Temozolomide Versus Radiotherapy Alone on Survival in Glioblastoma in a Randomised Phase III Study: 5-Year Analysis of the EORTC-NCIC Trial. Lancet Oncol (2009) 10(5):459–66. doi: 10.1016/S1470-2045(09)70025-7
121. Gilbert MR, Dignam JJ, Armstrong TS, Wefel JS, Blumenthal DT, Vogelbaum MA, et al. A Randomized Trial of Bevacizumab for Newly Diagnosed Glioblastoma. N Engl J Med (2014) 370(8):699–708. doi: 10.1056/NEJMoa1308573
122. Knizhnik AV, Roos WP, Nikolova T, Quiros S, Tomaszowski KH, Christmann M, et al. Survival and Death Strategies in Glioma Cells: Autophagy, Senescence and Apoptosis Triggered by a Single Type of Temozolomide-Induced DNA Damage. PloS One (2013) 8(1):e55665. doi: 10.1371/journal.pone.0055665
123. Golden EB, Cho HY, Jahanian A, Hofman FM, Louie SG, Schönthal AH, et al. Chloroquine Enhances Temozolomide Cytotoxicity in Malignant Gliomas by Blocking Autophagy. Neurosurg Focus (2014) 37(6):E12. doi: 10.3171/2014.9.Focus14504
124. Liu X, Sun K, Wang H, Dai Y. Inhibition of Autophagy by Chloroquine Enhances the Antitumor Efficacy of Sorafenib in Glioblastoma. Cell Mol Neurobiol (2016) 36(7):1197–208. doi: 10.1007/s10571-015-0318-z
125. Yao KC, Komata T, Kondo Y, Kanzawa T, Kondo S, Germano IM. Molecular Response of Human Glioblastoma Multiforme Cells to Ionizing Radiation: Cell Cycle Arrest, Modulation of the Expression of Cyclin-Dependent Kinase Inhibitors, and Autophagy. J Neurosurg (2003) 98(2):378–84. doi: 10.3171/jns.2003.98.2.0378
126. Ye H, Chen M, Cao F, Huang H, Zhan R, Zheng X. Chloroquine, an Autophagy Inhibitor, Potentiates the Radiosensitivity of Glioma Initiating Cells by Inhibiting Autophagy and Activating Apoptosis. BMC Neurol (2016) 16(1):178. doi: 10.1186/s12883-016-0700-6
127. Daido S, Yamamoto A, Fujiwara K, Sawaya R, Kondo S, Kondo Y. Inhibition of the DNA-dependent Protein Kinase Catalytic Subunit Radiosensitizes Malignant Glioma Cells by Inducing Autophagy. Cancer Res (2005) 65(10):4368–75. doi: 10.1158/0008-5472.Can-04-4202
128. Huang H, Song J, Liu Z, Pan L, Xu G. Autophagy Activation Promotes Bevacizumab Resistance in Glioblastoma by Suppressing Akt/mTOR Signaling Pathway. Oncol Lett (2018) 15(2):1487–94. doi: 10.3892/ol.2017.7446
129. Liang J, Piao Y, Henry V, Tiao N, de Groot JF. Interferon-Regulatory Factor-1 (IRF1) Regulates Bevacizumab Induced Autophagy. Oncotarget (2015) 6(31):31479–92. doi: 10.18632/oncotarget.5491
130. Müller-Greven G, Carlin CR, Burgett ME, Ahluwalia MS, Lauko A, Nowacki AS, et al. Macropinocytosis of Bevacizumab by Glioblastoma Cells in the Perivascular Niche Affects Their Survival. Clin Cancer Res (2017) 23(22):7059–71. doi: 10.1158/1078-0432.Ccr-17-0249
131. Hu YL, DeLay M, Jahangiri A, Molinaro AM, Rose SD, Carbonell WS, et al. Hypoxia-Induced Autophagy Promotes Tumor Cell Survival and Adaptation to Antiangiogenic Treatment in Glioblastoma. Cancer Res (2012) 72(7):1773–83. doi: 10.1158/0008-5472.Can-11-3831
132. Kang MR, Kim MS, Oh JE, Kim YR, Song SY, Kim SS, et al. Frameshift Mutations of Autophagy-Related Genes ATG2B, Atg5, ATG9B and ATG12 in Gastric and Colorectal Cancers With Microsatellite Instability. J Pathol (2009) 217(5):702–6. doi: 10.1002/path.2509
133. An CH, Kim MS, Yoo NJ, Park SW, Lee SH. Mutational and Expressional Analyses of ATG5, an Autophagy-Related Gene, in Gastrointestinal Cancers. Pathol Res Pract (2011) 207(7):433–7. doi: 10.1016/j.prp.2011.05.002
134. Lebovitz CB, Robertson AG, Goya R, Jones SJ, Morin RD, Marra MA, et al. Cross-Cancer Profiling of Molecular Alterations Within the Human Autophagy Interaction Network. Autophagy (2015) 11(9):1668–87. doi: 10.1080/15548627.2015.1067362
135. Amaravadi R, Kimmelman AC, White E. Recent Insights Into the Function of Autophagy in Cancer. Genes Dev (2016) 30(17):1913–30. doi: 10.1101/gad.287524.116
136. Wang QW, Liu HJ, Zhao Z, Zhang Y, Wang Z, Jiang T, et al. Prognostic Correlation of Autophagy-Related Gene Expression-Based Risk Signature in Patients With Glioblastoma. Onco Targets Ther (2020) 13:95–107. doi: 10.2147/ott.S238332
137. Wang Y, Zhao W, Xiao Z, Guan G, Liu X, Zhuang M. A Risk Signature With Four Autophagy-Related Genes for Predicting Survival of Glioblastoma Multiforme. J Cell Mol Med (2020) 24(7):3807–21. doi: 10.1111/jcmm.14938
138. Wang Z, Gao L, Guo X, Feng C, Lian W, Deng K, et al. Development and Validation of a Nomogram With an Autophagy-Related Gene Signature for Predicting Survival in Patients With Glioblastoma. Aging (Albany NY) (2019) 11(24):12246–69. doi: 10.18632/aging.102566
139. Tamrakar S, Yashiro M, Kawashima T, Uda T, Terakawa Y, Kuwae Y, et al. Clinicopathological Significance of Autophagy-related Proteins and its Association With Genetic Alterations in Gliomas. Anticancer Res (2019) 39(3):1233–42. doi: 10.21873/anticanres.13233
140. Zhao M, Xu H, Zhang B, Hong B, Yan W, Zhang J. Impact of Nuclear Factor Erythroid-Derived 2-Like 2 and p62/sequestosome Expression on Prognosis of Patients With Gliomas. Hum Pathol (2015) 46(6):843–9. doi: 10.1016/j.humpath.2015.02.009
141. Galavotti S, Bartesaghi S, Faccenda D, Shaked-Rabi M, Sanzone S, McEvoy A, et al. The Autophagy-Associated Factors DRAM1 and p62 Regulate Cell Migration and Invasion in Glioblastoma Stem Cells. Oncogene (2013) 32(6):699–712. doi: 10.1038/onc.2012.111
142. Li H, Li J, Zhang G, Da Q, Chen L, Yu S, et al. HMGB1-Induced P62 Overexpression Promotes Snail-Mediated Epithelial-Mesenchymal Transition in Glioblastoma Cells Via the Degradation of GSK-3β. Theranostics (2019) 9(7):1909–22. doi: 10.7150/thno.30578
143. Wen ZP, Zeng WJ, Chen YH, Li H, Wang JY, Cheng Q, et al. Knockdown ATG4C Inhibits Gliomas Progression and Promotes Temozolomide Chemosensitivity by Suppressing Autophagic Flux. J Exp Clin Cancer Res (2019) 38(1):298. doi: 10.1186/s13046-019-1287-8
144. Brennan CW, Verhaak RG, McKenna A, Campos B, Noushmehr H, Salama SR, et al. The Somatic Genomic Landscape of Glioblastoma. Cell (2013) 155(2):462–77. doi: 10.1016/j.cell.2013.09.034
145. Kim J, Kundu M, Viollet B, Guan KL. AMPK and mTOR Regulate Autophagy Through Direct Phosphorylation of Ulk1. Nat Cell Biol (2011) 13(2):132–41. doi: 10.1038/ncb2152
146. Wang RC, Wei Y, An Z, Zou Z, Xiao G, Bhagat G, et al. Akt-Mediated Regulation of Autophagy and Tumorigenesis Through Beclin 1 Phosphorylation. Science (2012) 338(6109):956–9. doi: 10.1126/science.1225967
147. Martelli AM, Evangelisti C, Follo MY, Ramazzotti G, Fini M, Giardino R, et al. Targeting the Phosphatidylinositol 3-Kinase/Akt/Mammalian Target of Rapamycin Signaling Network in Cancer Stem Cells. Curr Med Chem (2011) 18(18):2715–26. doi: 10.2174/092986711796011201
148. Eskilsson E, Røsland GV, Solecki G, Wang Q, Harter PN, Graziani G, et al. EGFR Heterogeneity and Implications for Therapeutic Intervention in Glioblastoma. Neuro Oncol (2018) 20(6):743–52. doi: 10.1093/neuonc/nox191
149. Li X, Wu C, Chen N, Gu H, Yen A, Cao L, et al. Pi3k/Akt/mTOR Signaling Pathway and Targeted Therapy for Glioblastoma. Oncotarget (2016) 7(22):33440–50. doi: 10.18632/oncotarget.7961
150. Parsons DW, Jones S, Zhang X, Lin JC, Leary RJ, Angenendt P, et al. An Integrated Genomic Analysis of Human Glioblastoma Multiforme. Science (2008) 321(5897):1807–12. doi: 10.1126/science.1164382
151. Koul D. PTEN Signaling Pathways in Glioblastoma. Cancer Biol Ther (2008) 7(9):1321–5. doi: 10.4161/cbt.7.9.6954
152. Álvarez-Garcia V, Tawil Y, Wise HM, Leslie NR. Mechanisms of PTEN Loss in Cancer: It’s All About Diversity. Semin Cancer Biol (2019) 59:66–79. doi: 10.1016/j.semcancer.2019.02.001
153. Koul D, Shen R, Kim YW, Kondo Y, Lu Y, Bankson J, et al. Cellular and In Vivo Activity of a Novel PI3K Inhibitor, PX-866, Against Human Glioblastoma. Neuro Oncol (2010) 12(6):559–69. doi: 10.1093/neuonc/nop058
154. Ferese R, Lenzi P, Fulceri F, Biagioni F, Fabrizi C, Gambardella S, et al. Quantitative Ultrastructural Morphometry and Gene Expression of Mtor-Related Mitochondriogenesis Within Glioblastoma Cells. Int J Mol Sci (2020) 21(13):4570. doi: 10.3390/ijms21134570
155. Zhang Y, Dube C, Gibert M, Cruickshanks N, Wang B, Coughlan M, et al. The P53 Pathway in Glioblastoma. Cancers (Basel) (2018) 10(9):297. doi: 10.3390/cancers10090297
156. Crighton D, Wilkinson S, O’Prey J, Syed N, Smith P, Harrison PR, et al. DRAM, a p53-induced Modulator of Autophagy, is Critical for Apoptosis. Cell (2006) 126(1):121–34. doi: 10.1016/j.cell.2006.05.034
157. Crighton D, Wilkinson S, Ryan KM. DRAM Links Autophagy to p53 and Programmed Cell Death. Autophagy (2007) 3(1):72–4. doi: 10.4161/auto.3438
158. Takahashi M, Kakudo Y, Takahashi S, Sakamoto Y, Kato S, Ishioka C. Overexpression of DRAM Enhances p53-Dependent Apoptosis. Cancer Med (2013) 2(1):1–10. doi: 10.1002/cam4.39
159. Green DR, Kroemer G. Cytoplasmic Functions of the Tumour Suppressor P53. Nature (2009) 458(7242):1127–30. doi: 10.1038/nature07986
160. Tasdemir E, Maiuri MC, Galluzzi L, Vitale I, Djavaheri-Mergny M, D’Amelio M, et al. Regulation of Autophagy by Cytoplasmic P53. Nat Cell Biol (2008) 10(6):676–87. doi: 10.1038/ncb1730
161. Lee SW, Kim HK, Lee NH, Yi HY, Kim HS, Hong SH, et al. The Synergistic Effect of Combination Temozolomide and Chloroquine Treatment is Dependent on Autophagy Formation and p53 Status in Glioma Cells. Cancer Lett (2015) 360(2):195–204. doi: 10.1016/j.canlet.2015.02.012
162. Aoki K, Nakamura H, Suzuki H, Matsuo K, Kataoka K, Shimamura T, et al. Prognostic Relevance of Genetic Alterations in Diffuse Lower-Grade Gliomas. Neuro Oncol (2018) 20(1):66–77. doi: 10.1093/neuonc/nox132
163. Bäcklund LM, Nilsson BR, Liu L, Ichimura K, Collins VP. Mutations in Rb1 Pathway-Related Genes are Associated With Poor Prognosis in Anaplastic Astrocytomas. Br J Cancer (2005) 93(1):124–30. doi: 10.1038/sj.bjc.6602661
164. Mao H, Lebrun DG, Yang J, Zhu VF, Li M. Deregulated Signaling Pathways in Glioblastoma Multiforme: Molecular Mechanisms and Therapeutic Targets. Cancer Invest (2012) 30(1):48–56. doi: 10.3109/07357907.2011.630050
165. Delou JM, Biasoli D, Borges HL. The Complex Link Between Apoptosis and Autophagy: A Promising New Role for RB. Acad Bras Cienc (2016) 88(4):2257–75. doi: 10.1590/0001-3765201620160127
166. Polager S, Ofir M, Ginsberg D. E2F1 Regulates Autophagy and the Transcription of Autophagy Genes. Oncogene (2008) 27(35):4860–4. doi: 10.1038/onc.2008.117
167. Jiang H, Martin V, Gomez-Manzano C, Johnson DG, Alonso M, White E, et al. The RB-E2F1 Pathway Regulates Autophagy. Cancer Res (2010) 70(20):7882–93. doi: 10.1158/0008-5472.Can-10-1604
168. Jiang H, Martin V, Alonso M, Gomez-Manzano C, Fueyo J. Rb-E2F1: Molecular Rheostat for Autophagy and Apoptosis. Autophagy (2010) 6(8):1216–7. doi: 10.4161/auto.6.8.13695
169. Tracy K, Dibling BC, Spike BT, Knabb JR, Schumacker P, Macleod KF. BNIP3 is an RB/E2F Target Gene Required for Hypoxia-Induced Autophagy. Mol Cell Biol (2007) 27(17):6229–42. doi: 10.1128/mcb.02246-06
170. Biasoli D, Kahn SA, Cornélio TA, Furtado M, Campanati L, Chneiweiss H, et al. Retinoblastoma Protein Regulates the Crosstalk Between Autophagy and Apoptosis, and Favors Glioblastoma Resistance to Etoposide. Cell Death Dis (2013) 4:e767. doi: 10.1038/cddis.2013.283
171. Liu X, Sun K, Wang H, Dai Y. Knockdown of Retinoblastoma Protein may Sensitize Glioma Cells to Cisplatin Through Inhibition of Autophagy. Neurosci Lett (2016) 620:137–42. doi: 10.1016/j.neulet.2016.04.001
172. An Z, Aksoy O, Zheng T, Fan QW, Weiss WA. Epidermal Growth Factor Receptor and EGFRvIII in Glioblastoma: Signaling Pathways and Targeted Therapies. Oncogene (2018) 37(12):1561–75. doi: 10.1038/s41388-017-0045-7
173. Bale TA, Jordan JT, Rapalino O, Ramamurthy N, Jessop N, DeWitt JC, et al. Financially Effective Test Algorithm to Identify an Aggressive, EGFR-amplified Variant of IDH-wildtype, Lower-Grade Diffuse Glioma. Neuro Oncol (2019) 21(5):596–605. doi: 10.1093/neuonc/noy201
174. Fraser J, Cabodevilla AG, Simpson J, Gammoh N. Interplay of Autophagy, Receptor Tyrosine Kinase Signalling and Endocytic Trafficking. Essays Biochem (2017) 61(6):597–607. doi: 10.1042/ebc20170091
175. Sigismund S, Avanzato D, Lanzetti L. Emerging Functions of the EGFR in Cancer. Mol Oncol (2018) 12(1):3–20. doi: 10.1002/1878-0261.12155
176. Wu M, Zhang P. EGFR-Mediated Autophagy in Tumourigenesis and Therapeutic Resistance. Cancer Lett (2020) 469:207–16. doi: 10.1016/j.canlet.2019.10.030
177. Tini P, Belmonte G, Toscano M, Miracco C, Palumbo S, Pastina P, et al. Combined Epidermal Growth Factor Receptor and Beclin1 Autophagic Protein Expression Analysis Identifies Different Clinical Presentations, Responses to Chemo- and Radiotherapy, and Prognosis in Glioblastoma. BioMed Res Int (2015) 2015:208076. doi: 10.1155/2015/208076
178. Eimer S, Belaud-Rotureau MA, Airiau K, Jeanneteau M, Laharanne E, Véron N, et al. Autophagy Inhibition Cooperates With Erlotinib to Induce Glioblastoma Cell Death. Cancer Biol Ther (2011) 11(12):1017–27. doi: 10.4161/cbt.11.12.15693
179. Ghildiyal R, Dixit D, Sen E. EGFR Inhibitor BIBU Induces Apoptosis and Defective Autophagy in Glioma Cells. Mol Carcinog (2013) 52(12):970–82. doi: 10.1002/mc.21938
180. Jutten B, Keulers TG, Peeters HJM, Schaaf MBE, Savelkouls KGM, Compter I, et al. EgfrvIII Expression Triggers a Metabolic Dependency and Therapeutic Vulnerability Sensitive to Autophagy Inhibition. Autophagy (2018) 14(2):283–95. doi: 10.1080/15548627.2017.1409926
181. Sugawa N, Ekstrand AJ, James CD, Collins VP. Identical Splicing of Aberrant Epidermal Growth Factor Receptor Transcripts From Amplified Rearranged Genes in Human Glioblastomas. Proc Natl Acad Sci USA (1990) 87(21):8602–6. doi: 10.1073/pnas.87.21.8602
182. Frederick L, Wang XY, Eley G, James CD. Diversity and Frequency of Epidermal Growth Factor Receptor Mutations in Human Glioblastomas. Cancer Res (2000) 60(5):1383–7.
183. Nagane M, Levitzki A, Gazit A, Cavenee WK, Huang HJ. Drug Resistance of Human Glioblastoma Cells Conferred by a Tumor-Specific Mutant Epidermal Growth Factor Receptor Through Modulation of Bcl-XL and caspase-3-like Proteases. Proc Natl Acad Sci USA (1998) 95(10):5724–9. doi: 10.1073/pnas.95.10.5724
184. Chakravarti A, Chakladar A, Delaney MA, Latham DE, Loeffler JS. The Epidermal Growth Factor Receptor Pathway Mediates Resistance to Sequential Administration of Radiation and Chemotherapy in Primary Human Glioblastoma Cells in a RAS-dependent Manner. Cancer Res (2002) 62(15):4307–15.
185. Gini B, Zanca C, Guo D, Matsutani T, Masui K, Ikegami S, et al. The mTOR Kinase Inhibitors, CC214-1 and CC214-2, Preferentially Block the Growth of EGFRvIII-activated Glioblastomas. Clin Cancer Res (2013) 19(20):5722–32. doi: 10.1158/1078-0432.Ccr-13-0527
186. Lee JK, Joo KM, Lee J, Yoon Y, Nam DH. Targeting the Epithelial to Mesenchymal Transition in Glioblastoma: The Emerging Role of MET Signaling. Onco Targets Ther (2014) 7:1933–44. doi: 10.2147/ott.S36582
187. Cheng F, Guo D. MET in Glioma: Signaling Pathways and Targeted Therapies. J Exp Clin Cancer Res (2019) 38(1):270. doi: 10.1186/s13046-019-1269-x
188. Cruickshanks N, Zhang Y, Yuan F, Pahuski M, Gibert M, Abounader R. Role and Therapeutic Targeting of the HGF/MET Pathway in Glioblastoma. Cancers (Basel) (2017) 9(7):87. doi: 10.3390/cancers9070087
189. Lee JS, Oh E, Yoo JY, Choi KS, Yoon MJ, Yun CO. Adenovirus Expressing Dual c-Met-specific shRNA Exhibits Potent Antitumor Effect Through Autophagic Cell Death Accompanied by Senescence-Like Phenotypes in Glioblastoma Cells. Oncotarget (2015) 6(6):4051–65. doi: 10.18632/oncotarget.3018
190. Cohen AL, Holmen SL, Colman H. IDH1 and IDH2 Mutations in Gliomas. Curr Neurol Neurosci Rep (2013) 13(5):345. doi: 10.1007/s11910-013-0345-4
191. Barthel FP, Johnson KC, Varn FS, Moskalik AD, Tanner G, Kocakavuk E, et al. Longitudinal Molecular Trajectories of Diffuse Glioma in Adults. Nature (2019) 576(7785):112–20. doi: 10.1038/s41586-019-1775-1
192. Yan H, Parsons DW, Jin G, McLendon R, Rasheed BA, Yuan W, et al. IDH1 and IDH2 Mutations in Gliomas. N Engl J Med (2009) 360(8):765–73. doi: 10.1056/NEJMoa0808710
193. Han S, Liu Y, Cai SJ, Qian M, Ding J, Larion M, et al. IDH Mutation in Glioma: Molecular Mechanisms and Potential Therapeutic Targets. Br J Cancer (2020) 122(11):1580–9. doi: 10.1038/s41416-020-0814-x
194. Braun Y, Filipski K, Bernatz S, Baumgarten P, Roller B, Zinke J, et al. Linking Epigenetic Signature and Metabolic Phenotype in IDH Mutant and IDH Wildtype Diffuse Glioma. Neuropathol Appl Neurobiol (2020) 47(3):379–93 doi: 10.1111/nan.12669
195. Kickingereder P, Sahm F, Radbruch A, Wick W, Heiland S, Deimling A, et al. IDH Mutation Status is Associated With a Distinct Hypoxia/Angiogenesis Transcriptome Signature Which is non-Invasively Predictable With rCBV Imaging in Human Glioma. Sci Rep (2015) 5:16238. doi: 10.1038/srep16238
196. Fan Y, Peng X, Li B, Zhao G. Development of Autophagy Signature-Based Prognostic Nomogram for Refined Glioma Survival Prognostication. BioMed Res Int (2020) 2020:1872962. doi: 10.1155/2020/1872962
197. Xu Y, Li R, Li X, Dong N, Wu D, Hou L, et al. An Autophagy-Related Gene Signature Associated With Clinical Prognosis and Immune Microenvironment in Gliomas. Front Oncol (2020) 10:571189. doi: 10.3389/fonc.2020.571189
198. Binabaj MM, Bahrami A, ShahidSales S, Joodi M, Joudi Mashhad M, Hassanian SM, et al. The Prognostic Value of MGMT Promoter Methylation in Glioblastoma: A Meta-Analysis of Clinical Trials. J Cell Physiol (2018) 233(1):378–86. doi: 10.1002/jcp.25896
199. Alnahhas I, Alsawas M, Rayi A, Palmer JD, Raval R, Ong S, et al. Characterizing Benefit From Temozolomide in MGMT Promoter Unmethylated and Methylated Glioblastoma: A Systematic Review and Meta-Analysis. Neurooncol Adv (2020) 2(1):vdaa082. doi: 10.1093/noajnl/vdaa082
200. Ryskalin L, Gaglione A, Limanaqi F, Biagioni F, Familiari P, Frati A, et al. The Autophagy Status of Cancer Stem Cells in Gliobastoma Multiforme: From Cancer Promotion to Therapeutic Strategies. Int J Mol Sci (2019) 20(15):3824. doi: 10.3390/ijms20153824
201. Bazzoni R, Bentivegna A. Role of Notch Signaling Pathway in Glioblastoma Pathogenesis. Cancers (Basel) (2019) 11(3):292. doi: 10.3390/cancers11030292
202. Takebe N, Nguyen D, Yang SX. Targeting Notch Signaling Pathway in Cancer: Clinical Development Advances and Challenges. Pharmacol Ther (2014) 141(2):140–9. doi: 10.1016/j.pharmthera.2013.09.005
203. Yao J, Zheng K, Li C, Liu H, Shan X. Interference of Notch1 Inhibits the Growth of Glioma Cancer Cells by Inducing Cell Autophagy and Down-Regulation of Notch1-Hes-1 Signaling Pathway. Med Oncol (2015) 32(6):610. doi: 10.1007/s12032-015-0610-2
204. Tao Z, Li T, Ma H, Yang Y, Zhang C, Hai L, et al. Autophagy Suppresses Self-Renewal Ability and Tumorigenicity of Glioma-Initiating Cells and Promotes Notch1 Degradation. Cell Death Dis (2018) 9(11):1063. doi: 10.1038/s41419-018-0957-3
205. Wu X, Fleming A, Ricketts T, Pavel M, Virgin H, Menzies FM, et al. Autophagy Regulates Notch Degradation and Modulates Stem Cell Development and Neurogenesis. Nat Commun (2016) 7:10533. doi: 10.1038/ncomms10533
206. Zhang T, Guo L, Wang Y, Yang Y. Macroautophagy Regulates Nuclear NOTCH1 Activity Through Multiple P62 Binding Sites. IUBMB Life (2018) 70(10):985–94. doi: 10.1002/iub.1891
207. Natsumeda M, Maitani K, Liu Y, Miyahara H, Kaur H, Chu Q, et al. Targeting Notch Signaling and Autophagy Increases Cytotoxicity in Glioblastoma Neurospheres. Brain Pathol (2016) 26(6):713–23. doi: 10.1111/bpa.12343
208. Lee Y, Lee JK, Ahn SH, Lee J, Nam DH. WNT Signaling in Glioblastoma and Therapeutic Opportunities. Lab Invest (2016) 96(2):137–50. doi: 10.1038/labinvest.2015.140
209. Tompa M, Kalovits F, Nagy A, Kalman B. Contribution of the Wnt Pathway to Defining Biology of Glioblastoma. Neuromolecular Med (2018) 20(4):437–51. doi: 10.1007/s12017-018-8514-x
210. Zuccarini M, Giuliani P, Ziberi S, Carluccio M, Iorio PD, Caciagli F, et al. The Role of Wnt Signal in Glioblastoma Development and Progression: A Possible New Pharmacological Target for the Therapy of This Tumor. Genes (Basel) (2018) 9(2):105. doi: 10.3390/genes9020105
211. Wang T, Chen Z, Zhang W. Regulation of Autophagy Inhibition and Inflammatory Response in Glioma by Wnt Signaling Pathway. Oncol Lett (2017) 14(6):7197–200. doi: 10.3892/ol.2017.7103
212. Nàger M, Sallán MC, Visa A, Pushparaj C, Santacana M, Macià A, et al. Inhibition of WNT-CTNNB1 Signaling Upregulates SQSTM1 and Sensitizes Glioblastoma Cells to Autophagy Blockers. Autophagy (2018) 14(4):619–36. doi: 10.1080/15548627.2017.1423439
213. Yun EJ, Kim S, Hsieh JT, Baek ST. Wnt/β-Catenin Signaling Pathway Induces Autophagy-Mediated Temozolomide-Resistance in Human Glioblastoma. Cell Death Dis (2020) 11(9):771. doi: 10.1038/s41419-020-02988-8
214. Colella B, Faienza F, Carinci M, D’Alessandro G, Catalano M, Santoro A, et al. Autophagy Induction Impairs Wnt/β-Catenin Signalling Through β-Catenin Relocalisation in Glioblastoma Cells. Cell Signal (2019) 53:357–64. doi: 10.1016/j.cellsig.2018.10.017
215. Chang L, Zhao D, Liu HB, Wang QS, Zhang P, Li CL, et al. Activation of Sonic Hedgehog Signaling Enhances Cell Migration and Invasion by Induction of Matrix Metalloproteinase-2 and -9 Via the Phosphoinositide-3 Kinase/AKT Signaling Pathway in Glioblastoma. Mol Med Rep (2015) 12(5):6702–10. doi: 10.3892/mmr.2015.4229
216. Clement V, Sanchez P, de Tribolet N, Radovanovic I, Ruiz i Altaba A. Hedgehog-GLI1 Signaling Regulates Human Glioma Growth, Cancer Stem Cell Self-Renewal, and Tumorigenicity. Curr Biol (2007) 17(2):165–72. doi: 10.1016/j.cub.2006.11.033
217. Carpenter RL, Lo HW. Hedgehog Pathway and GLI1 Isoforms in Human Cancer. Discovery Med (2012) 13(69):105–13.
218. Tang X, Deng L, Chen Q, Wang Y, Xu R, Shi C, et al. Inhibition of Hedgehog Signaling Pathway Impedes Cancer Cell Proliferation by Promotion of Autophagy. Eur J Cell Biol (2015) 94(5):223–33. doi: 10.1016/j.ejcb.2015.03.003
219. Carballo GB, Ribeiro JH, Lopes GPF, Ferrer VP, Dezonne RS, Pereira CM, et al. GANT-61 Induces Autophagy and Apoptosis in Glioblastoma Cells Despite Their Heterogeneity. Cell Mol Neurobiol (2020). doi: 10.1007/s10571-020-00891-6
220. Honorato JR, Hauser-Davis RA, Saggioro EM, Correia FV, Sales-Junior SF, Soares LOS, et al. Role of Sonic Hedgehog Signaling in Cell Cycle, Oxidative Stress, and Autophagy of Temozolomide Resistant Glioblastoma. J Cell Physiol (2020) 235(4):3798–814. doi: 10.1002/jcp.29274
221. Chen X, Morales-Alcala CC, Riobo-Del Galdo NA. Autophagic Flux is Regulated by Interaction Between the C-terminal Domain of PATCHED1 and ATG101. Mol Cancer Res (2018) 16(5):909–19. doi: 10.1158/1541-7786.Mcr-17-0597
222. Marjanovic Vicentic J, Drakulic D, Garcia I, Vukovic V, Aldaz P, Puskas N, et al. SOX3 can Promote the Malignant Behavior of Glioblastoma Cells. Cell Oncol (Dordr) (2019) 42(1):41–54. doi: 10.1007/s13402-018-0405-5
223. Stupp R, Taillibert S, Kanner AA, Kesari S, Steinberg DM, Toms SA, et al. Maintenance Therapy With Tumor-Treating Fields Plus Temozolomide vs Temozolomide Alone for Glioblastoma: A Randomized Clinical Trial. JAMA (2015) 314(23):2535–43. doi: 10.1001/jama.2015.16669
224. Escamilla-Ramírez A, Castillo-Rodríguez RA, Zavala-Vega S, Jimenez-Farfan D, Anaya-Rubio I, Briseño E, et al. Autophagy as a Potential Therapy for Malignant Glioma. Pharmaceuticals (Basel) (2020) 13(7):156. doi: 10.3390/ph13070156
225. Zhang X, Deibert CP, Kim WJ, Jaman E, Rao AV, Lotze MT, et al. Autophagy Inhibition is the Next Step in the Treatment of Glioblastoma Patients Following the Stupp Era. Cancer Gene Ther (2020). doi: 10.1038/s41417-020-0205-8
226. Sotelo J, Briceño E, López-González MA. Adding Chloroquine to Conventional Treatment for Glioblastoma Multiforme: A Randomized, Double-Blind, Placebo-Controlled Trial. Ann Intern Med (2006) 144(5):337–43. doi: 10.7326/0003-4819-144-5-200603070-00008
227. Briceño E, Calderon A, Sotelo J. Institutional Experience With Chloroquine as an Adjuvant to the Therapy for Glioblastoma Multiforme. Surg Neurol (2007) 67(4):388–91. doi: 10.1016/j.surneu.2006.08.080
228. Rosenfeld MR, Ye X, Supko JG, Desideri S, Grossman SA, Brem S, et al. A Phase I/II Trial of Hydroxychloroquine in Conjunction With Radiation Therapy and Concurrent and Adjuvant Temozolomide in Patients With Newly Diagnosed Glioblastoma Multiforme. Autophagy (2014) 10(8):1359–68. doi: 10.4161/auto.28984
Keywords: autophagy, glioblastoma, intratumoral heterogeneity (ITH), drug resistance, cell invasion, pro-tumoral
Citation: Jandrey EHF, Bezerra M, Inoue LT, Furnari FB, Camargo AA and Costa ET (2021) A Key Pathway to Cancer Resilience: The Role of Autophagy in Glioblastomas. Front. Oncol. 11:652133. doi: 10.3389/fonc.2021.652133
Received: 11 January 2021; Accepted: 17 May 2021;
Published: 10 June 2021.
Edited by:
Eugenia Morselli, Pontificia Universidad Católica de chile, ChileReviewed by:
Ilya Ulasov, I.M. Sechenov First Moscow State Medical University, RussiaGabriele Multhoff, Technical University of Munich, Germany
Copyright © 2021 Jandrey, Bezerra, Inoue, Furnari, Camargo and Costa. This is an open-access article distributed under the terms of the Creative Commons Attribution License (CC BY). The use, distribution or reproduction in other forums is permitted, provided the original author(s) and the copyright owner(s) are credited and that the original publication in this journal is cited, in accordance with accepted academic practice. No use, distribution or reproduction is permitted which does not comply with these terms.
*Correspondence: Érico Tosoni Costa, ZWNvc3RhQG1vY2hzbC5vcmcuYnI=
†These authors share first authorship