- 1Department of Pharmacy, Women’s Hospital, School of Medicine, Zhejiang University, Hangzhou, China
- 2Key Lab Women’s Reproductive Health, Women’s Hospital, School of Medicine, Zhejiang University, Hangzhou, China
Increasing evidence shows that the extracellular matrix (ECM) is an important regulator of breast cancer (BC). The ECM comprises of highly variable and dynamic components. Compared with normal breast tissue under homeostasis, the ECM undergoes many changes in composition and organization during BC progression. Induced ECM proteins, including fibrinogen, fibronectin, hyaluronic acid, and matricellular proteins, have been identified as important components of BC metastatic cells in recent years. These proteins play major roles in BC progression, invasion, and metastasis. Importantly, several specific ECM molecules, receptors, and remodeling enzymes are involved in promoting resistance to therapeutic intervention. Additional analysis of these ECM proteins and their downstream signaling pathways may reveal promising therapeutic targets against BC. These potential drug targets may be combined with new nanoparticle technologies. This review summarizes recent advances in functional nanoparticles that target the ECM to treat BC. Accurate nanomaterials may offer a new approach to BC treatment.
Introduction
According to global cancer data, the incidence and mortality rates of breast cancer (BC) in women in 2019 were 24.20% and 15.00%, respectively, and both ranked first among female malignant tumors. Despite significant advances in the diagnosis and early treatment of BC, this malignant tumor still accounts for more than 600,000 deaths annually. Most cancer-related deaths are associated with cancer cells metastasizing to other sites of the body and causing new tumors in secondary organs (1). Although early BC has good prognosis after treatment, controlling cancer metastasis in clinical settings remains challenging (2, 3). There is an urgent need to better understand the mechanisms of metastasis and exploit novel strategies to prevent and treat advanced BC.
The extracellular matrix (ECM) is an important component of cellular biology (4). Proteins in the ECM include collagen, laminin (LN), fibronectin (FN), hyaluronan, and matricellular proteins that serve as structural scaffolds to maintain tissue integrity and sustainability (5). The ECM is crucial for embryonic development, production of new tissue structures, and maintenance of human tissues and homeostasis (6, 7). However, the ECM is not just a simple framework; it is a highly dynamic and complex network of molecules surrounding cells within the tissue. ECM components provide biochemical and biomechanical contexts for cells and have received increasing attention for their important biological roles in BC progression and metastasis (8). Evidence suggests that ECM components are dynamic during cancer progression and may promote metastatic spread (9). Regulation of key ECM components via targeting of matrix-mediated pre-tumorigenic signals or via promotion of tumor suppressive signals may be a promising strategy to address BC invasion and metastasis (10).
Nanoparticles (NPs) are widely used in biomedical research and are increasingly applied in oncologic research because of their excellent physicochemical properties (11). They generally consist of an outer shell and an inner core of buried drugs or proteins (12). Myriad NPs are used in antitumor therapy; liposomes, micelles, polymers, metal NPs, viral NPs, antibodies, and dendrimers have been widely applied in biological applications, including as drug carriers, tumor monitors, and cell markers (11, 12). Abnormalities of some receptors, enzymes and other components in the ECM allow breast tumors to be distinguished from the normal mammary gland. Specific metabolic pathways and rapid tumor proliferation lead to pathological blood vessel formation, low pH, low oxygen tension, and high interstitial pressure—aspects that are fundamental to the design of NP drug delivery systems (13). ECM serves as the soil for tumor cells to grow in, but it also contains complex factors to interfere with tumor progression and prognosis. By re-educating the ECM, the tumor constructs a micro-ecosystem to develop itself, escape immune attack, and even resist exogenous injury. NPs based on ECM regulation have been extensively studied because of their ability to accurately target tumor ECM components and reverse tumor progression (14–17).
In this review, we discuss the roles for ECM in the development and metastasis of BC, including ECM composition, molecular mechanisms related to ECM dynamics and remodeling in cancer, therapy resistance, and potential therapeutic targets. We also highlight ECM-regulated nanotherapeutic strategies, including degradation of the tumor ECM, mimic of the ECM to inhibit tumor progression, and alteration of the ECM fabrication as approaches to efficient BC suppression. Finally, we consider the future expectations for and challenges of ECM-targeted nanotherapeutics for clinical cancer therapy.
ECM Composition in BC Progression
Increasing evidence indicates that the ECM composition continues to change during BC progression and may promote metastatic spread. The morphology, physical strength, biochemical characteristics, and other parameters of the ECM in BC differ from the ECM of normal breast tissue (18–20). ECM components can be divided into three groups: (1) structural proteins, such as collagen and elastin, that give the ECM strength and toughness; (2) glycosaminoglycans (GAGs) and proteoglycans that can form water-based colloids and that contain many other matrix components; and (3) adhesive proteins, such as FN and LN, that are used to bind to the stroma (21).
Collagens
Excessive production of collagens is a common feature of breast fibrosis and malignant BC. Collagens are the most abundant proteins in animals, accounting for more than 30% of the total protein content in the human body. Collagen is found in various organs and tissues and serves as the ECM framework. It can be synthesized and secreted into the ECM by fibroblasts, chondrocytes, osteoblasts, and some epithelial cells. At least 28 different types of collagen have been found; they are encoded by different structural genes and have various chemical structures and immunological properties (22, 23). The composition of collagen changes significantly in BC, with increased accumulation of type I, III, and V fibrillar collagens and decreased amounts of type IV collagen. The marked reduction of type IV collagen in BC is mainly due to basement membrane degradation (24). Studies have shown that certain collagen genes expressed in patients with primary BC are associated with an increased risk of metastasis. Increased expression of fibrillar collagen (e.g., type I and type III collagen) in BC may be associated with tumor invasion and aggressive tumor behavior. The relationship between changes in collagen production and BC progression may be functionally important (25–27).
The structural and physical properties of the ECM are continually changing in BC (28). Collagen type I is usually highly linearized, unlike the nondirectional fibrils. Linearized collagen type I is aligned along the epithelium or vertically into the tissue. Collagen can be used as a scaffold to facilitate the migration of cancer cells or stromal cells. Consistent with these observations, malignancy-associated fibrotic reactions, called fibrous hyperplasia, have been associated with poor prognoses in patients with BC. Collagen in the ECM of BC is heavily deposited in early cancer progression and plays a major role in maintaining tissue hardness (the stroma of BC is 10 times harder than normal), thereby triggering signal transduction in noncancer and tumor epithelial cells (29).
Certain collagens processed in the ECM are catalyzed by specific proteases, and the expression of enzymes associated with ECM remodeling is often upregulated in human cancers (30, 31). In many cancers, heparanases, cysteine cathepsin, 6-o-sulfonase, urokinase, and matrix metalloproteinases (MMPs) have been overexpressed. Lysyl oxidase (LOX) and lysine hydroxylase catalyze cross-linking between collagen and elastin molecules, further altering cellular behavior by regulating the ECM elasticity and strength (32). Any type of modification or addition of a cross-linked matrix makes BC tissue stiffer and enhances cell growth, survival, and migration-related signaling pathways (33).
Elastin
Elastin gives tissues the flexibility they need to stretch and return to their original state. This property is helpful to lungs, skin, blood vessels, and neck ligaments. Smooth muscle and fibroblasts contain much elastin. Tropoelastins are the precursors of elastin; they are bound to a chaperone when they are secreted; they are additionally modified when they come into contact with mature elastin fibers, when then chemically transform into elastin chains. Lack of elastin in the ECM can lead to cutis laxa and Williams syndrome (34–36).
Elastosis is generally believed to be caused by the abnormal increase in the expression of elastic fiber components such as elastin and the degradation of normal elastic fiber. Elastosis is a common feature in BC (37). Ductal elastosis is closely related to cancer, especially invasive cancer. Elastosis reportedly increases with increasing severity of breast disease (37). BC elastosis is a complex phenomenon resulting in both deposition of elastotic masses and local production of elastin fragments. These two manifestations must be distinguished within the matrix. Signals from the fragments of the degraded matrix differ substantially from those provided by their parent proteins. For example, stromal cells adhere to elastin but cause cell migration and/or proliferation when they are incubated with elastin fragments (38).
Studies have shown that elastin-derived peptides have numerous biological activities in cancer cells and their surrounding stroma (36). They enhance tumor cell migration and stromal invasion (39, 40). Elastin-derived peptides also stimulate the migration and proliferation of skin fibroblasts and monocytes. They upregulate the expression of MMPs through a fibroblast-induced remodeling program, thus facilitating the invasion of melanoma cells (41, 42). In addition, they promote angiogenesis, chemotaxis for inflammatory cells, and the release of elastase. Finally, elastin-derived peptides provide a powerful survival signal, because they promote resistance to apoptosis (43, 44).
Glycosaminoglycans and Proteoglycans
GAGs are unbranched long-chain polysaccharides composed of repeating disaccharides. The disaccharide unit usually consists of amino hexose (glucosamine or glucosamine galactose) and uronic acid; however, in keratinic sulfate, the uronic acid is replaced with galactose (45). Hyaluronic acid (HA) is the only aminoglycan that does not undergo sulfation, and its sugar chain is extremely long. Aminoglycans generally consist of fewer than 300 monosaccharides, whereas HA may contain 100,000 glycosyl groups. HA molecules in solution are in an irregular, curled state; if forced to stretch, their length can reach 20 m. The entire molecule consists of the repeated arrangement of glucuronic acid and acetylglucosamine disaccharides. HA can bind numerous water molecules because of the large number of negatively charged hydrophilic groups on its surface. Even at a very low concentration, it can form a viscous gel that occupies a large space and produces turgor. HA forms a significant part of the ECM and plays many biological roles in physiological and pathophysiological processes, depending on the size of its polymer and its interaction with other secreted proteins and cellular receptors (46, 47).
HA is involved in cancer progression and is remarkably increased in BC versus in normal breast tissue (48). Clinically, HA may be associated with highly invasive BC. Serum HA levels in patients with metastatic BC were significantly higher than those in patients without metastatic BC (49). HA regulates a variety of cellular behaviors, such as adhesion, growth, motility, and differentiation, and acts through surface receptors, such as the HA-mediated motor receptor (50) and CD44 (51). Hyaluronan synthase (HAS) is the key enzyme in HA biosynthesis (52). In animal models of BC, HAS2 expression promoted breast tumor progression and metastasis (53). Furthermore, in BC models, inhibition of HAS2 significantly reduced cancer progression, suggesting that it plays an important role in this process (54). Additionally, HA induces the invasive behavior of cancer cells themselves and causes increased hydration and interstitial pressure via CD44, which promotes fibroblast penetration as well as the migration and invasion activity of cancer cells (55).
Laminin
LN is a large glycoprotein that, with collagen type IV, forms the ECM base. LN is involved in the embryonic development of ECM components at the earliest phase. LN molecules have at least eight binding sites. For example, the IKVAV pentapeptide sequence on the chain binds to neuronal cells and promotes nerve growth. The RGD sequence on the first chain of rat LN can bind to αvβ3 integrins (5, 56). LN has high sugar content (accounting for 15%–28%), and it has approximately 50 sugar chains connected with N-terminal. It is the most complex glycoprotein with a sugar chain structure identified so far. Moreover, the multiple receptors of LN recognize and bind its sugar chain structure. The basement membrane is the soft, specialized ECM under epithelial cells that also surrounds muscle, fat, and Schwann cells. It protects and filters cells, and it determines their polarity, which affects cell metabolism, survival, migration, proliferation, and differentiation (57).
Several LN subtypes play important roles in the development of BC. These subtypes include LM-111, LM-332, and LM-511 (58–60). LM-111, an important component of the basement membrane, is secreted by normal breast myofibroblasts and maintains epithelial cell polarity. LM-111 promotes prolactin-induced mammary epithelial cell maturation. In breast tumors, LM-111 expression is often lost, which results in changes in cell polarity. Studies have shown that LM-111 can induce cell–cell adhesion, suggesting that LM-111 may inhibit the spread of BC (61). There is growing evidence that other laminins containing α4 subunits, such as LM-332 and LM-511, may promote tumor progression. Expression of LM-332 is associated with aggressive BC, and cancer-derived LM-332 has promoted anchor-independent survival by interacting with α6β4 integrin receptors (62). In addition, LM-332 has induced migration and invasion of BC cells through α3 integrin. LM-511 has mediated adhesion, migration, and invasion in vitro and metastasis in vivo through integrin interactions in experimental models of BC. LM-511 interacted with the integrin α6β1 receptor in a subpopulation of BC cells capable of self-renewal and tumor initiation (63).
Fibronectin
FN is a large glycoprotein found in all vertebrates; it has a molecular sugar content of 4.5%–9.5%. The sugar chain structure varies according to the origin and differentiation state of the tissue’s cells (64). FN connects cells to the ECM. Some of the short peptide sequences in FN are the smallest structural units for recognizing and binding to FN receptors on the cell surface. For example, the RGD (ARG-gly-ASP) sequence exists in the cell-binding module at the center of the peptide chain, where cell surface integrin receptors recognize and bind to them. FN molecules on the cell surface and in the ECM are cross-linked by disulfide bonds and assembled into fibers (65). Unlike collagen, FN does not self-assemble into fibers. Instead, it is directed by cell surface receptors that exist only on the surface of certain cells (e.g., fibroblasts). The decrease or loss of FN fibers on the surface of transformed cells and tumor cells is due to the abnormality of FN receptors on the cell surface (66, 67).
In cancer, FN is expressed by cancer-associated fibroblasts (CAFs) and by the cancer cells themselves (68). The upregulation of FN in cancer cells may occur through different mechanisms. Mechanical compression can lead an over expression of FN in cancer cells and increased invasion and migration behavior in tumors (69, 70). In general, FN expression in BC is associated with adverse clinical outcomes. Some studies have shown that cancer-derived FN is particularly associated with poor outcomes in BC, including increased metastases and reduced overall survival (70). In addition, FN expression has been detected in circulating tumor cells of patients with BC. Evidence from samples from patients with BC suggests that circulating tumor cells may have the property of epithelial-mesenchymal transformation; as a result, loss of cell polarity and cell–cell adhesion are observed in the epithelium, and a mesenchymal phenotype with high motility is acquired. FN is an established mesenchymal marker, and it has promoted transforming growth factor β–induced epithelial-mesenchymal transformation (25).
ECM Remodeling Enzymes
During tissue regeneration and cancer, changes in the ECM also affect remodeling enzymes (18). Multiple ECM remodeling enzymes that promote stem/progenitor cell signaling pathways and metastasis are induced in BC (71). The process of ECM reconstruction in BC involves different signaling pathways of ECM regulation, including Wnt, PI3K/Akt, extracellular signal-regulated kinase, jun N-terminal kinase, Src-focal adhesion kinase (FAK), and others (20). The main induced proteins in the ECM are fibrinogen, proteoglycans, and matricellular cell proteins—all of which may be potential drug targets (29, 72). ECM remodeling enzymes such as MMPs, heparanase, urokinase plasminogen activator, cross-linking enzymes of the LOX family, and cathepsin, are often upregulated in breast tumors and have great significance to BC progression and metastasis (73–76). These enzymes modify the ECM in different ways to enable pathways of minimal resistance that promote cancer cell invasion and migration (18, 19). They can directly affect the biological properties and functions of ECM components by exposing cryptic sites or releasing ECM-bound growth factors or soluble domains of ECM proteins. Moreover, remodeling enzymes can change the physical properties of ECM structures by means of cross-linking and other modifications. These functions promoted by ECM remodeling enzymes are critical for BC progression and metastasis.
Treatment Resistance Induced by ECM
The ECM in breast tumors is integral to the invasion and metastasis of BC. In recent years, increasing evidence has indicated that the ECM may also play an important role in mediating resistance to existing treatments (77–79). It provides an adhesion matrix and specific matrix components that promote survival mechanisms to support and induce stem cell pathways and enhance cell metastasis and invasion (80). Some researchers have found that BC matrix components are resistant to the chemotherapy drugs 5-fluorouracil (81), epirubicin (82), and cyclophosphamide (83). The ECM plays an important role in promoting resistance of cancer cells to treatment. Matrix proteins that induce chemotherapeutic drug resistance include the matricellular cell proteins, namely secreted phosphoprotein 1, tenascin C (84), B-lymphoma Mo-MLV insertion region 1, and phosphatase and tensin homolog (85). Hypoxia-induced ECM remodeling has been reported in BC (86). LOX is a key inducer of chemotherapeutic resistance. Inhibition of LOX can reduce collagen cross-linking and fibrinogen assembly, increase drug penetration, and downregulate ITGA5/FN1 expression, thereby inhibiting the Src/FAK signaling pathway and inducing apoptosis and chemotherapeutic resensitization. In addition, the ECM is associated with resistance to endocrine-targeted therapy and radiotherapy. Helleman et al. (2008) reported that ECM gene clusters were associated with resistance to first-line tamoxifen treatment in patients with metastatic BC. They indicated that expression levels of FN1, LOX, SPARC, and tissue inhibitor of metalloproteinase 3 were associated with prognosis in patients with BC, whereas tenascin C was associated with tamoxifen resistance (87).
Furthermore, the constitutive activation of PI3K as a result of both PIK3CA mutation and PTEN deletion are associated with resistance to human epidermal growth factor receptor 2–targeted therapy. This mechanism may explain the poor prognoses of some patients after trastuzumab treatment (88).
Finally, the ECM also promotes resistance to radiation therapy in BC. Resistance to single or combined drugs and radiation exposure, as mediated by cell adhesion, is driven by specific ECM proteins. Specifically, FN and LN enhance the resistance of human tumor cells and normal cells to ionizing radiation and cytotoxic drugs in vitro (89).
Potential Therapeutic Targets in the ECM for BC
An increasing number of experimental studies have explored the biological roles of the ECM in BC and indicate that ECM components and ECM-mediated functions might be promising therapeutic targets (90–92). Many approaches are available to interfere with ECM function in BC. Chemical and biological agents that modulate the ECM in cancer are being investigated as potential treatments for BC (Table 1).
Inhibition of ECM Components
Inhibiting ECM components that promote tumor progression and metastasis might be an attractive treatment strategy for BC (20). One possible approach is to suppress the synthesis of ECM components with pro-tumor functions. Preclinical studies in animal models have shown that this approach is feasible and can be translated into clinical practice (101–103). A recent study found that 4-methylumbelliferone can significantly reduce the migration, adhesion, and invasion of estrogen receptor cells in BC and reduce the expressions and activities of several pro-tumor matrix-degrading enzymes and pro-inflammatory molecules. The results suggest that 4-methylumbelliferone could be a new treatment for specific BC subtypes according to estrogen receptor status by inhibiting the synthesis of HA and regulating HAS2, CD44, matrix-degrading enzymes, and inflammatory mediators (93). Direct neutralization of tumor-causing ECM components is an attractive approach. Recent preclinical models have yielded encouraging results, showing that ECM components can be directly blocked by targeted peptides, neutralizing antibodies, or DNA aptamers (19). For example, Li et al. (91) found that R5 (a neutralizing antibody to Robo1) significantly inhibited BC growth and metastasis in an MMTV-PyMT transgenic mouse model and in a xenografted BC model. Lida et al. sed the SELEX (systematic evolution of ligand by exponential enrichment) method to develop DNA aptamers that specifically recognize the CD44 exon V10. These special aptamers significantly inhibited BC cell migration. The pull-down analysis indicated that the exon interacted with EphA2, which plays a key role in promoting tumor invasion and metastasis; this interaction was inhibited by the aptamers. The results of this study suggest that a novel molecular complex composed of CD44 and EphA2 can promote the progression of triple-negative BC (92).
Targeting the ECM Remodeling Enzymes
Collagen cross-linking and ECM remodeling enzymes play vital roles in the development of rigid fibrotic tissue. They promote BC progression and metastatic spread. Antifibrotic therapies that target ECM remodeling enzymes may represent a good cancer treatment strategy (19).
MMPs are considered promising targets for cancer treatment. Numerous evidence indicates that MMPs play important roles in tumor invasion, metastasis, and angiogenesis; these findings have led to the development and clinical application of MMP inhibitors (MPIs). The new generation of MPIs is more selective for pre-metastatic MMPs and is currently being developed and tested in preclinical cancer models (104–107). In different models, tumor necrosis and apoptosis were induced by drug administration alone or in combination with chemotherapy. The clinical applications of many MPIs are not ideal because of their high musculoskeletal toxicity, poor bioavailability, low selectivity, or lack of efficiency (108). The conclusion from early trials was that inhibitor specificity was the key problem. Devel et al. optimized S1′ pocket interactions and recruited phosphinate or P2′ glutamate as alternatives to more traditional zinc-chelating groups, resulting in very selective MMP-12 inhibitors (109–111). A unique approach to limiting MPI toxicity was recently reported in a study aimed at achieving selectivity not only to the target enzyme but also through targeted drug delivery. Although MMP-2 is not generally considered a drug target in cancer because of its ubiquitous presence and involvement in many physiological processes, the importance of this enzyme in the progression of bone-metastatic BC suggests that it may be a useful target for this particular environment (94). Tauro et al. investigated phosphonic acid–based inhibitors selective for MMP-2 instead of the similar MMP-9. Because of the affinity of bisphosphonate for hydroxyapatite, these MPIs efficiently localized in the bone microenvironment. In a mouse model, the MPIs specifically inhibited MMP-2 and prevented breast tumor growth and its associated bone destruction (94).
Experimental evidence suggests that inhibiting heparanase may inhibit BC growth and metastasis. Therefore, drugs that have this effect may be feasible treatments for breast tumors. Several carbohydrate-based heparanase inhibitors have entered clinical trials. These compounds are highly similar to enzymes and work in combination with the heparin/Heparan sulfate (HS) -substrate binding domain of the enzyme, thus blocking their accessibility to the natural HS substrates (112). Weissmann et al. designed two monoclonal antibodies (9E8 and H1023) that neutralized the enzymatic activity of heparanase and significantly inhibited cell invasion and tumor metastasis without significant toxicity to BC cells themselves. This result suggests that monoclonal antibodies affect the tumor microenvironment rather than the BC cells, thus offering a newer and safer mode to obstruct both tumor growth and metastasis (95).
Antibodies Against Remodeled ECM
Certain ECM components are highly expressed in areas of active tumor invasion and thus can be used as biomarkers. Anti-remodeling ECM antibodies may introduce bioactive-inhibiting cues or radioactive substances into tumor sites. This approach can enhance the effectiveness of radiation, chemotherapy, or targeted therapy by concentrating radioisotopes, drugs, or antitumor biologics on the active tumor sites while minimizing their distribution in healthy tissue (113–116). Huang et al. enhanced the potency of a whole-cell BC vaccine in mice with an antibody–interleukin (IL)-2 immunocytokine that targets exposed phosphatidylserine. Immune cytokines (2AG4-IL2) were prepared by linking IL-2 to the 2aG4 gene, a targeted antibody that blocks the immunosuppressive effects of phosphatidylserine. The 2AG4-IL2/4T1 vaccine was prepared after phosphatidylserine-exposed, irradiated 4T1 cells were coated with 2AG4-IL2. The incidence and number of spontaneous lung metastases in the 2AG4-IL2/4T1 group were significantly lower than those in other groups. Spleen cells of mice immunized with 2AG4-IL2/4T1 showed significantly higher 4T1-specific cytotoxicity and demonstrated a greater ability to secret interferon-γ than spleen cells of other groups. These results suggest that 2AG4-IL2 encapsulation of radioactive tumor cells can produce an effective whole-cell vaccine (117). Sussman et al. investigated a novel antibody-drug conjugate (ADC; SGN–LIV-1A) that targeted the zinc transporter LIV-1 (SLC39A6) for the treatment of metastatic BC. LIV-1 is expressed by estrogen receptor–positive BC. The data on the latest ADCs and their recent successes support the use of SGN–LIV-1A as a new treatment for refractory metastatic BC and other LIV-1–positive indications (96).
Cell Surface Receptor Blockers
Cell surface receptor blockers that interact with ECM components may be a way to treat metastatic BC. Interactions between cells and ECM proteins are often mediated by integrins. Integrins belong to the transmembrane protein family and act as cell surface receptors that mediate cell-to-cell and cell-to-matrix adhesion by identifying components of ECM, such as FN, laminin, collagen, and transmit information from ECM to cells (118), leading to the recruitment and activation of intracellular signaling proteins, which in turn initiates a signaling cascade that promotes breast cancer cell migration, proliferation, and survival (119, 120). The increase of integrins will facilitate the tumor cells adhesion to the basement membrane and promote the invasion and metastasis of tumor cells. Therefore, integrins represent an interesting target for metastatic BC therapies. In addition, recent researches in the xenograft mouse model showed that surface integrin α2β1 contacts and activates Wnt-β-catenin. The integrin α2β1 plays a pro-metastatic role in BC progression, and integrin α2β1–silencing has a potential effect in inhibiting breast cancer metastasis (121, 122). A study conducted by Archis Bagati et al. indicated that SOX4 expression is regulated by the integrin αvβ6 receptor on the tumor cell surface, which activates TGFβ from a potential precursor. They identified that SOX4 transcription factor acts as an important T cell-mediated cytotoxic resistance mechanism of TNBC. An integrin αvβ6/8-blocking monoclonal antibody (mAb) inhibits SOX4 expression and sensitizes TNBC cells to cytotoxic T cells. In a highly metastatic mouse model of TNBC, this integrin mAb induced a substantial survival benefit (123).
Targeting Cancer Associated Fibroblasts
CAFs are the major players in dysregulation of collagen transformation during tumor progression, leading to tumor fibrosis characterized by excessive depositions of collagen around the tumor (124). Unlike normal fibroblasts, CAFs overexpress a number of biomarker proteins. Generally speaking, the interaction between CAFs and tumor cells promotes tumor progression mainly through the release of various secreted proteins (e.g., transforming growth factor-β, insulin-like growth factor, and IL-6), direct interaction with tumor cells, immune response regulation, and ECM remodeling. These multiple actions suggest that several stromal therapeutic targets exist (25, 125, 126). Suvarna et al. demonstrated that NIH3T3 fibroblast migration was enhanced after co-cultured with MCF7 BC cells. They found that a small-molecule ligand (NPD8733) of valosin-containing protein/p97 inhibited cancer cell–accelerated fibroblast migration. This mechanism offers potential for BC treatment (97). Many activated CAFs are present in the BC stroma, and they have the ability to promote tumor formation and development. Studies have shown that CAFs are the main factor involved in ECM remodeling (127, 128). Activated CAFs can specifically change some ECM components, transforming the ECM from loose and irregular to linear, and driving changes in biomechanical conduction (69). Cell polarity changes when cancer cells perceive changes in extracellular stress; invasion and metastasis. The mechanism by which CAFs remodel the ECM and thus affect the function of cancer cells has been discovered gradually. CAFs come from numerous sources, especially from normal fibroblasts (70). The transformation of normal fibroblasts to CAFs is a process of cell differentiation, and miRNA plays an important role in embryonic development (129–132). A Study has shown that miR-200 mediates the differentiation of normal fibroblasts into CAFs by regulating the expression of cell differentiation–related transcription factors, TCL12 and FLI-1, and miR-200 maintains the activation state of CAFs. The continuously activated CAFs directly or interactively regulate the expression of ECM-related genes, participate in the remodeling of ECM, and further promote the occurrence and development of breast tumors (71).
Targeting Endostatin
Some ECM cues suppress tumor and metastasis development, so enhancing these biological effects may offer a feasible approach to BC treatment (19). Angiogenesis plays a critical role in the pathogenesis, growth, invasion, and metastasis of solid tumors. Endostatin, which was first isolated in 1997, is one of the most effective anti-angiogenic factors and has significantly reduced blood vessel formation in tumors (133). Guo et al. demonstrated that endostatin induced RAW264.7 phenotype polarization to M1 in vitro. They proposed that endostatin may inhibit mouse BC growth in vivo by regulating the polarization of tumor-associated macrophages via two possible mechanisms: by shifting the polarity of the tumor-associated macrophage from an M2-like to an M1-like functional phenotype or by increasing the proportion of M1-like tumor-associated macrophage via specific inhibition of M2 polarity (99). Chen et al. conducted a phase III, multicenter, prospective, randomized, controlled clinical trial to explore the efficacy and safety of rh-endostatin. Patients received neoadjuvant therapy with docetaxel and epirubicin or that combination plus rh-endostatin. After three cycles of neoadjuvant therapy, the objective effective rates of the docetaxel and epirubicin group and of rh-endostatin group were 77.9% and 91.0%, respectively (p < 0.001). Chemotherapy combined with rh-endostatin is more effective than chemotherapy alone and is considered a promising treatment strategy for BC (100).
Novel Nanoparticle-Based Approaches to Modulate ECM Components in BC
The strategies for ECM-based therapy have been attractive in recent years, so many drugs are emerging to target the ECM. Currently, targeted drug delivery systems can effectively solve the problems conventional chemotherapy caused. They can preferentially deliver drugs to tumor tissue, thus preventing the dose-limiting adverse effects that occur in normal tissues (11–13, 134). Several nano-based therapies targeting the ECM have been used for tumor theranostics. Some of these NPs are single drug carriers, but many more are drugs, genes, antibodies, or aptamers combined; some have multiple functions, such as diagnostics, therapeutics, and monitoring. These emerging nanotherapeutics that can regulate the ECM have roughly three approaches: (1) degrading the tumor ECM; (2) mimicing tumor ECM to inhibit tumor progression; and (3) intervening the native ECM fabrication. The first is aimed at breaking barriers to improve tumor penetration of the nanomedicine delivery system; the second is aimed at enhancing measures to block tumor metastasis in the early stages of tumor progression; and the last is aimed at specific stages in the body when the tumor’s ECM is made. This review presents the recent developments of NPs in ECM-targeted therapy (Table 2).
Nanoparticle Based ECM Degradation
The cleavage of ECM components depends on the balance of proteases and protease inhibitors, which promotes the release of bioactive molecules. In particular, MMPs and tissue inhibitors of metalloproteinases play important roles in controlling the ECM (13). The strategy to degrade ECM components and increase NP affinity is widely used. Because NPs cannot reach deep-seated tumors via conventional methods, many contractible NPs have been designed to penetrate tumor vasculature and tissue. Through the interaction between the coated gelatin layer and the MMP-2 in the ECM, large-sized gold NPs become smaller, which enables deeper tumor infiltration (135). Kessenbrock et al. designed a liposome modified with a GPLPLR peptide sequence to target MT1-MMP, which was more effective than uncoated liposomes in binding and treating tumors (137). They also designed gold NPs combined with MMP-sensitive peptides for drug delivery and tumor imaging. These gold NPs have efficient drug delivery and imaging capabilities at tumor sites. Suresh et al. (136) found that MMP-2–sensitive gold NPs improved targeted delivery to human BC cells and increased cellular uptake. The proteases collagenase and hyaluronidase are also commonly used to control ECM degradation. For example, Liu et al. synthesized collagenase-encapsulated nanoscale coordination polymers based on Mn2+ and an acid-sensitive benzoic-imine organic linker that was then modified by polyethylene glycol (PEG). The results indicated that released collagenase could specifically degrade collagens, leading to a loosened ECM structure, enhanced tumor perfusion, and decreased hypoxia (Figure 1) (138).
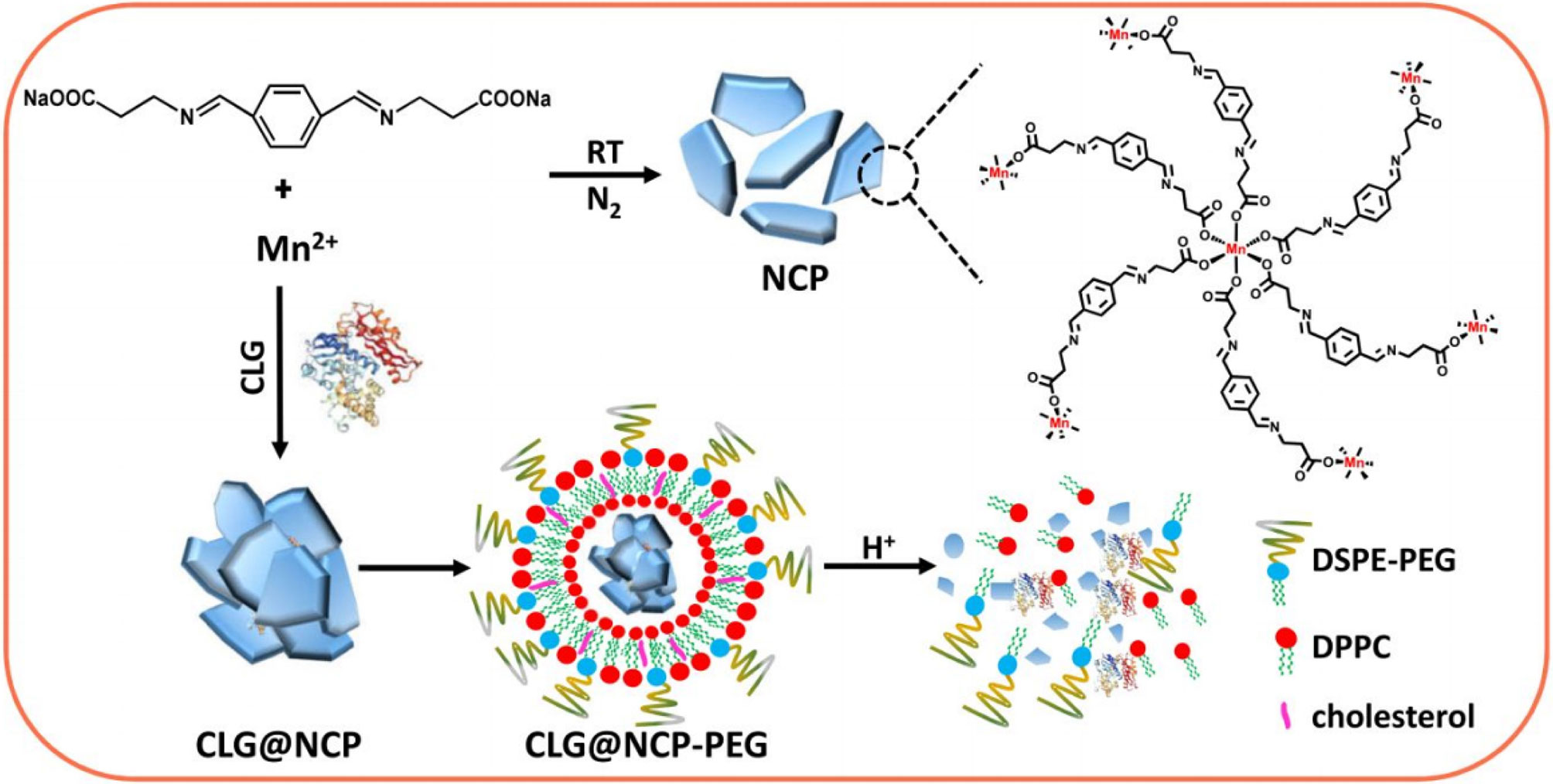
Figure 1 Synthesis and characterization of CLG@NCP-PEG NPs. Scheme for the preparation of NCP NPs and CLG-encapsulated CLG@NCP nanoparticles, surface modification, and pH-sensitive degradation. Reproduced with permission from (138).
Mimicing the Tumor ECM to Inhibit Tumor Progression
Tumor metastasis refers to the formation of a new nidus at a distant site from the original tumor; it is the main reason for the high cancer-associated mortality rate. As mentioned above, the tumor ECM is critical to progression, and it provides an important protective layer to prevent tumor metastasis. Tumor cells overexpress some enzymes, such as MMP, to overcome ECM barriers and prepare for tumor metastasis. Many scientists have proposed mimicing the tumor ECM to inhibit tumor metastasis, and some promising results have been achieved (144–146). For example, Suo et al. (140) designed a dual-degradable and injectable HA hydrogel to mimic the ECM for a 3D culture of MCF-7 BC cells.
Inspired by the natural ECM formation, our laboratory constructed a “fibrin integrin receptor” structural peptide (AANL-KLVFFK-GGDGR-YIGSR) to initiate and participate in the physiological reconstruction of the ECM in BC. LN is one of the most important ECM components (147); the YIGSR sequence in the LNβ1 chain and the RGD sequence in the LNα chain are considered essential to integrin identification. They also combine specific loci, including metal ions (Ca2+, Mg2+) adhesive dots, and produce the mechanical force behind cell actin filaments during ECM self-assembly. YIGSR targets integrin’s RGD receptor. In normal cells and on a mature endothelial cell surface, integrin αvβ3 expression is low, but it is highly expressed during tumor angiogenesis (148). The drug delivery system can specifically bind tumor cells that express integrins, effectively mediate the drug delivery system to enable tumor cell entry, reduce distribution in normal tissue, and prevent fibrosis of the normal tissue cell matrix. In addition, we are currently synthesizing a peptide-PEG-PLGA three-block conjugate to obtain biodegradable polymer micelles through self-assembly. These novel NPs comprise three units: (1) YIGSR and RGD targets to induce nanomicelle transfer to the tumor site; (2) enzyme-sensitive groups of peptide-PEG-PLGA, under the action of a legumain, to easily dissociate in a tumor pH (pH 4–6.5) (148); and (3) KLVFF peptides that participate in ECM remodeling, curing the tumor tissue protein fiber layer. Then, after PLGA-PEG/PTX enters the cell, glutathione dissolves the disulfide bond between PLGA and PEG to release PTX. This release inhibits tumor cell tubulin depolymerization and promotes cell apoptosis in BC (Figure 2; data to be published).
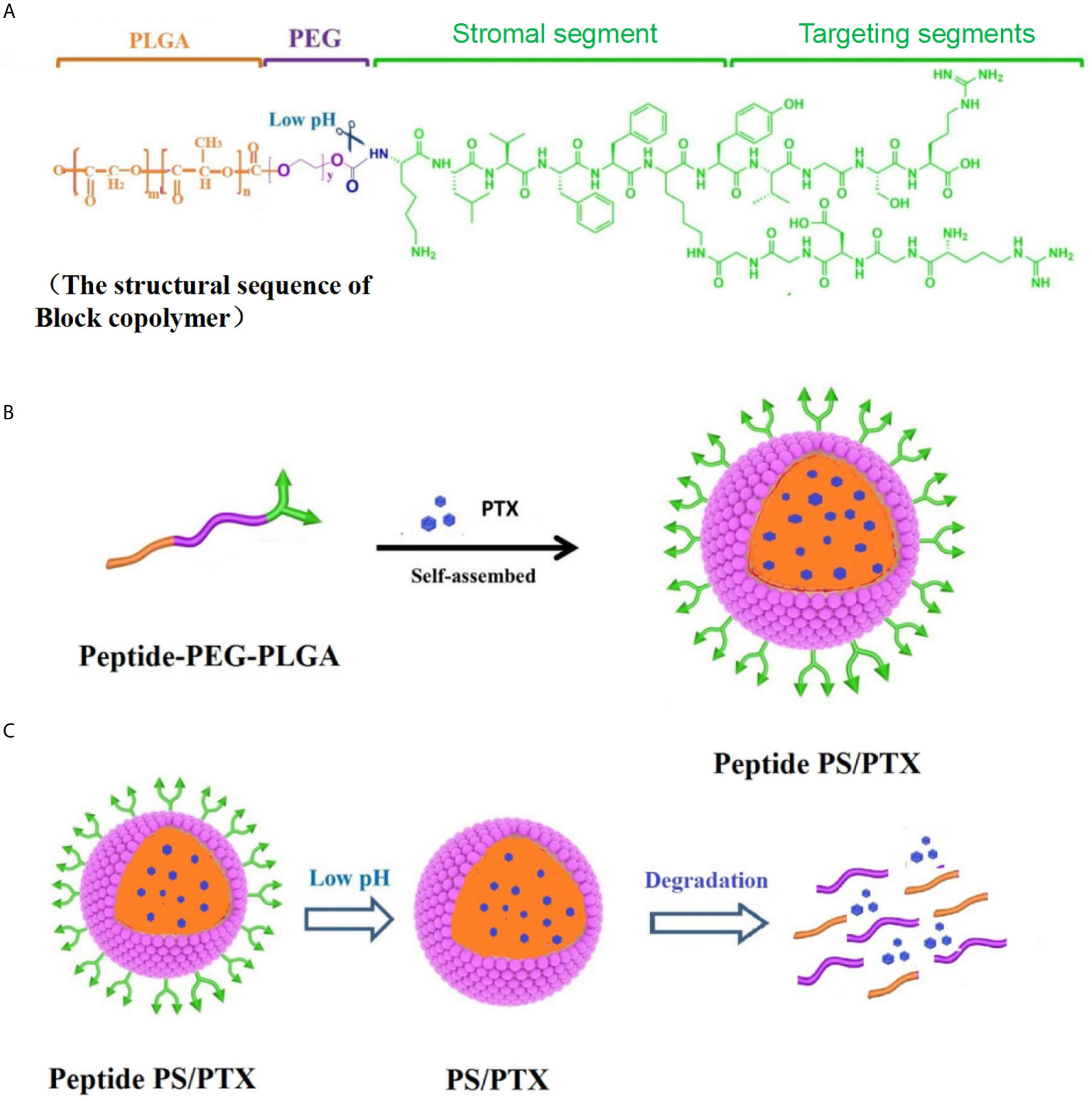
Figure 2 Synthesis and characterization of Peptide PS/PTX micelles. (A) Scheme of the lock copolymer structural sequence. (B) Peptide-PEG-PLGA conjugate is mixed with PTX and self-assembled into Peptide PS/PTX micelles. (C) Surface-modified peptide PS/PTX micelles and is pH-sensitive and glutathione-sensitive degradation.
Intervening in Native ECM Fabrication
With a better understanding of the natural assembly process of the native tumor ECM, it is now possible to interfere with the specific stages of ECM fabrication in a tumor. An anti–LOX-like 2 antibody can alter the natural assembly of endogenous fibrous collagen. LOX-like 2 interference with collagen can significantly affect the adhesion and invasion of human BC cells, and tumor growth can be effectively inhibited by LOX-like 2 antibody treatment in mice bearing mammary tumors (149–151). PLGA-based LOX-traceable NPs consisting of LOX antibodies and paclitaxel were successfully synthesized and can be used as a tumor-targeting drug carrier for chemotherapy and targeted radiotherapy. LOX antibodies have high specificity to target tumors in xenograft models (141). These NPs can affect ECM fabrication in tumors by altering collagen assembly; in addition, they can increase drug concentrations in tumor radiotherapy sites and enhance the chemotherapeutic effect of paclitaxel, which achieves the same result as the method described in 6.2.
Discussion
BC metastasis is a complex process with different regulatory mechanisms that involve many processes, including changes in the ECM (74). The ECM is a proven barrier to cells. During the process of tumor invasion and metastasis, a series of dynamic changes occurs in tumor cells and the surrounding ECM (19). These events involve MMPs (e.g., MMP-2 and MMP-9), cathepsin, fiber protease release, type IV collagen, LN, gelatin, fiber connection proteins, other components outside the matrix involved in synthesis and degradation, collagen enzyme inhibitors (e.g., tissue inhibitors of metalloproteinase 1 and 2), adhesion molecules, and tumor cells that release fibroblast growth factor, vascular endothelial growth factor, and angiogenesis inhibitors (20). Greater insight into the occurrence of metastasis and its mechanisms in BC, as well as a better understanding of ECM structure and regulation, will help spur the development of new therapeutic targets for antineoplastic drugs (12, 13, 146).
NPs offer an innovative technology with great potential for biomedical applications. A growing number of studies has demonstrated that ECM-targeted NPs hold great promise for early BC diagnosis and treatment. Nanomaterials are useful vehicles for improving anticancer drug efficacy. New advances have been made in the study of multifunctional NPs that provide simultaneous targeting of both tumor cells and the ECM (152, 153). However, many challenges remain in designing effective nanodrugs for clinical cancer treatment. For example, the interaction between the ECM and tumor cells is still largely unknown. Appropriate experimental and preclinical models must be designed to better describe the ECM and accelerate the development of existing NP systems. In addition, many problems in tumor metastasis and drug resistance urgently need solved. An immunosuppressive ECM impedes the implementation of effective immunotherapies (154). These cancer therapy obstacles require an advanced NP system that can precisely target the molecular determinants of the ECM. Future research should focus on developing NP systems that regulate ECM at the metabolic and immune levels; such a system would likely yield great clinical achievements.
Conclusions
This review focused on the role of the ECM in the development and metastasis of BC and on the application of NPs that target this structure. The ECM composition dynamically changes in BC and is very different from that of a normal mammary gland. Various ECM-modifying enzymes play an important role in BC progression and metastasis. Moreover, the ECM may be involved in regulating resistance to treatments, including chemotherapy, endocrine targeted therapy, and radiotherapy. The ECM provides many potential therapeutic targets, and NPs are promising carriers for improving drug activity and efficacy. Additional research is needed to promote the development of ECM-targeted nanotherapeutics that effectively control BC tumors.
Author Contributions
YCZ and XZ drafted the manuscript. CZ conceptualized the study. YQZ assisted in reviewing literature. The manuscript was modified by YC and WF. FW helped review the first draft of the manuscript. The final version was reviewed and edited by YCZ, XZ, and CZ. All authors contributed to the article and approved the submitted version.
Funding
This work was financially supported by the National Natural Science Foundation of China (nos. 81802630, 81802587, 81873838) and Public Welfare Technology Research Project of Zhejiang Province (LGF21H160023).
Conflict of Interest
The authors declare that the research was conducted in the absence of any commercial or financial relationships that could be construed as a potential conflict of interest.
Abbreviations
ADC, antibody-drug conjugate; BC, breast cancer; CAF, cancer-associated fibroblast; ECM, extracellular matrix; FAK, Src-focal adhesion kinase; FN, fibronectin; GAG, glycosaminoglycan; HA, hyaluronic acid; HAS2, hyaluronan synthase; IL, interleukin; LN, laminin; LOX, lysyl oxidase; MMP, matrix metalloproteinase; MPI, MMP inhibitor; NP, nanoparticle; PEG, polyethylene glycol.
References
1. Zeeshan R, Mutahir Z. Cancer metastasis - tricks of the trade. Bosn J Basic Med Sci (2017) 17(3):172–82. doi: 10.17305/bjbms.2017.1908
2. Kozłowski J, Kozłowska A, Kocki J. Breast cancer metastasis - insight into selected molecular mechanisms of the phenomenon. Postepy Hig Med Dosw (Online) (2015) 69:447–51. doi: 10.5604/17322693.1148710
3. Tian Q, Wang Y, Guo H, Xie G, Li J, Zhang M, et al. Recent perspectives of management of breast cancer metastasis - an update. J BUON (2017) 22(2):295–300.
4. Humphrey JD, Dufresne ER, Schwartz MA. Mechanotransduction and extracellular matrix homeostasis. Nat Rev Mol Cell Biol (2014) 15(12):802–12. doi: 10.1038/nrm3896
5. Halper J, Kjaer M. Basic components of connective tissues and extracellular matrix: elastin, fibrillin, fibulins, fibrinogen, fibronectin, laminin, tenascins and thrombospondins. Adv Exp Med Biol (2014) 802:31–47. doi: 10.1007/978-94-007-7893-1_3
6. Høye AM, Erler JT. Structural ECM components in the premetastatic and metastatic niche. Am J Physiol Cell Physiol (2016) 310(11):C955–67. doi: 10.1152/ajpcell.00326.2015
7. Levi N, Papismadov N, Solomonov I, Sagi I, Krizhanovsky V. The ECM path of senescence in aging: components and modifiers. FEBS J (2020) 287(13):2636–46. doi: 10.1111/febs.15282
8. Acerbi I, Cassereau L, Dean I, Shi Q, Au A, Park C, et al. Human breast cancer invasion and aggression correlates with ECM stiffening and immune cell infiltration. Integr Biol (Camb) (2015) 7(10):1120–34. doi: 10.1039/c5ib00040h
9. Lu P, Weaver VM, Werb Z. The extracellular matrix: a dynamic niche in cancer progression. J Cell Biol (2012) 196(4):395–406. doi: 10.1083/jcb.201102147
10. Fleming JM, Yeyeodu ST, McLaughlin A, Schuman D, Taylor DK. In Situ Drug Delivery to Breast Cancer-Associated Extracellular Matrix. ACS Chem Biol (2018) 13(10):2825–40. doi: 10.1021/acschembio.8b00396
11. Kumari P, Ghosh B, Biswas S. Nanocarriers for cancer-targeted drug delivery. J Drug Target (2016) 24(3):179–91. doi: 10.3109/1061186X.2015.1051049
12. Baetke SC, Lammers T, Kiessling F. Applications of nanoparticles for diagnosis and therapy of cancer. Br J Radiol (2015) 88(1054):20150207. doi: 10.1259/bjr.20150207
13. Li M, Zhang F, Su Y, Zhou J, Wang W. Nanoparticles designed to regulate tumor microenvironment for cancer therapy. Life Sci (2018) 201:37–44. doi: 10.1016/j.lfs.2018.03.044
14. Arosio D, Casagrande C. Advancement in integrin facilitated drug delivery. Adv Drug Deliv Rev (2016) 97:111–43. doi: 10.1016/j.addr.2015.12.001
15. López-Ruiz E, Jiménez G, Álvarez de Cienfuegos L, Antic C, Sabata R, Marchal JA, et al. Advances of hyaluronic acid in stem cell therapy and tissue engineering, including current clinical trials. Eur Cell Mater (2019) 37:186–213. doi: 10.22203/eCM.v037a12
16. Tang M, Gandhi NS, Burrage K, Gu Y. Interaction of gold nanosurfaces/nanoparticles with collagen-like peptides. Phys Chem Chem Phys (2019) 21(7):3701–11. doi: 10.1039/c8cp05191g
17. Zinger A, Koren L, Adir O, Poley M, Alyan M, Yaari Z, et al. Collagenase Nanoparticles Enhance the Penetration of Drugs into Pancreatic Tumors. ACS Nano (2019) 13(10):11008–21. doi: 10.1021/acsnano.9b02395
18. Lu P, Takai K, Weaver VM, Werb Z. Extracellular matrix degradation and remodeling in development and disease. Cold Spring Harb Perspect Biol (2011) 3(12):a005058. doi: 10.1101/cshperspect.a005058
19. Insua-Rodríguez J, Oskarsson T. The extracellular matrix in breast cancer. Adv Drug Deliv Rev (2016) 97:41–55. doi: 10.1016/j.addr.2015.12.017
20. Jena MK, Janjanam J. Role of extracellular matrix in breast cancer development: a brief update. F1000Res (2018) 7:274. doi: 10.12688/f1000research.14133.2
21. Manou D, Caon I, Bouris P, Triantaphyllidou IE, Giaroni C, Passi A, et al. The Complex Interplay Between Extracellular Matrix and Cells in Tissues. Methods Mol Biol (2019) 1952:1–20. doi: 10.1007/978-1-4939-9133-4_1
22. Gatseva A, Sin YY, Brezzo G, Van Agtmael T. Basement membrane collagens and disease mechanisms. Essays Biochem (2019) 63(3):297–312. doi: 10.1042/EBC20180071
23. Nissen NI, Karsdal M, Willumsen N. Collagens and Cancer associated fibroblasts in the reactive stroma and its relation to Cancer biology. J Exp Clin Cancer Res (2019) 38(1):115. doi: 10.1186/s13046-019-1110-6
24. Egeblad M, Rasch MG, Weaver VM. Dynamic interplay between the collagen scaffold and tumor evolution. Curr Opin Cell Biol (2010) 22(5):697–706. doi: 10.1016/j.ceb.2010.08.015
25. Ren J, Smid M, Iaria J, Salvatori D, van Dam H, Zhu HJ, et al. Cancer-associated fibroblast-derived Gremlin 1 promotes breast cancer progression. Breast Cancer Res (2019) 21(1):109. doi: 10.1186/s13058-019-1194-0
26. Berger AJ, Renner CM, Hale I, Yang X, Ponik SM, Weisman PS, et al. Scaffold stiffness influences breast cancer cell invasion via EGFR-linked Mena upregulation and matrix remodeling. Matrix Biol (2020) 85-86:80–93. doi: 10.1016/j.matbio.2019.07.006
27. Nolan J, Mahdi AF, Dunne CP, Kiely PA. Collagen and fibronectin promote an aggressive cancer phenotype in breast cancer cells but drive autonomous gene expression patterns. Gene (2020) 761:145024. doi: 10.1016/j.gene.2020.145024
28. Wolf K, Alexander S, Schacht V, Coussens LM, von Andrian UH, van Rheenen J, et al. Collagen-based cell migration models in vitro and in vivo. Semin Cell Dev Biol (2009) 20(8):931–41. doi: 10.1016/j.semcdb.2009.08.005
29. Wang K, Wu F, Seo BR, Fischbach C, Chen W, Hsu L, et al. Breast cancer cells alter the dynamics of stromal fibronectin-collagen interactions. Matrix Biol (2017) 60-61:86–95. doi: 10.1016/j.matbio.2016.08.001
30. Afratis NA, Klepfish M, Karamanos NK, Sagi I. The apparent competitive action of ECM proteases and cross-linking enzymes during fibrosis: Applications to drug discovery. Adv Drug Deliv Rev (2018) 129:4–15. doi: 10.1016/j.addr.2018.03.004
31. Shekhter AB, Balakireva AV, Kuznetsova NV, Vukolova MN, Litvitsky PF, Zamyatnin AA Jr. Collagenolytic Enzymes and their Applications in Biomedicine. Curr Med Chem (2019) 26(3):487–505. doi: 10.2174/0929867324666171006124236
32. Tenti P, Vannucci L. Lysyl oxidases: linking structures and immunity in the tumor microenvironment. Cancer Immunol Immunother (2020) 69(2):223–35. doi: 10.1007/s00262-019-02404-x
33. Cao H, Lee M, Yang H, Sze SK, Tan NS, Tay CY. Mechanoregulation of Cancer-Associated Fibroblast Phenotype in Three-Dimensional Interpenetrating Hydrogel Networks. Langmuir (2019) 35(23):7487–95. doi: 10.1021/acs.langmuir.8b02649
34. Mongiat M, Marastoni S, Ligresti G, Lorenzon E, Schiappacassi M, Perris R, et al. The extracellular matrix glycoprotein elastin microfibril interface located protein 2: a dual role in the tumor microenvironment. Neoplasia (2010) 12(4):294–304. doi: 10.1593/neo.91930
35. Bülow RD, Boor P. Extracellular Matrix in Kidney Fibrosis: More Than Just a Scaffold. J Histochem Cytochem (2019) 67(9):643–61. doi: 10.1369/0022155419849388
36. Wang Y, Song EC, Resnick MB. Elastin in the Tumor Microenvironment. Adv Exp Med Biol (2020) 1272:1–16. doi: 10.1007/978-3-030-48457-6_1
37. Salesse S, Odoul L, Chazée L, Garbar C, Duca L, Martiny L, et al. Elastin molecular aging promotes MDA-MB-231 breast cancer cell invasiveness. FEBS Open Bio (2018) 8(9):1395–404. doi: 10.1002/2211-5463.12455
38. Zakout YM, Abdullah SM, Ali MA. Assessment of elastosis in invasive ductal carcinoma of the breast compared to fibroadenoma among Sudanese patients using conventional histochemical methods. Biotech Histochem (2012) 87(2):122–5. doi: 10.3109/10520295.2011.565805
39. Devy J, Duca L, Cantarelli B, Joseph-Pietras D, Scandolera A, Rusciani A, et al. Elastin-derived peptides enhance melanoma growth in vivo by upregulating the activation of Mcol-A (MMP-1) collagenase. Br J Cancer (2010) 103(10):1562–70. doi: 10.1038/sj.bjc.6605926
40. Desforges M, Harris LK, Aplin JD. Elastin-derived peptides stimulate trophoblast migration and invasion: a positive feedback loop to enhance spiral artery remodelling. Mol Hum Reprod (2015) 21(1):95–104. doi: 10.1093/molehr/gau089
41. Ntayi C, Labrousse AL, Debret R, Birembaut P, Bellon G, Antonicelli F, et al. Elastin-derived peptides upregulate matrix metalloproteinase-2-mediated melanoma cell invasion through elastin-binding protein. J Invest Dermatol (2004) 122(2):256–65. doi: 10.1046/j.0022-202X.2004.22228.x
42. Toupance S, Brassart B, Rabenoelina F, Ghoneim C, Vallar L, Polette M, et al. Elastin-derived peptides increase invasive capacities of lung cancer cells by post-transcriptional regulation of MMP-2 and uPA. Clin Exp Metastasis (2012) 29(5):511–22. doi: 10.1007/s10585-012-9467-3
43. Dale MA, Xiong W, Carson JS, Suh MK, Karpisek AD, Meisinger TM, et al. Elastin-Derived Peptides Promote Abdominal Aortic Aneurysm Formation by Modulating M1/M2 Macrophage Polarization. J Immunol (2016) 196(11):4536–43. doi: 10.4049/jimmunol.1502454
44. Lefebvre T, Rybarczyk P, Bretaudeau C, Vanlaeys A, Cousin R, Brassart-Pasco S, et al. TRPM7/RPSA Complex Regulates Pancreatic Cancer Cell Migration. Front Cell Dev Biol (2020) 8:549. doi: 10.3389/fcell.2020.00549
45. Caon I, Bartolini B, Parnigoni A, Caravà E, Moretto P, Viola M, et al. Revisiting the hallmarks of cancer: The role of hyaluronan. Semin Cancer Biol (2020) 62:9–19. doi: 10.1016/j.semcancer.2019.07.007
46. Shendi D, Marzi J, Linthicum W, Rickards AJ, Dolivo DM, Keller S, et al. Hyaluronic acid as a macromolecular crowding agent for production of cell-derived matrices. Acta Biomater (2019) 100:292–305. doi: 10.1016/j.actbio.2019.09.042
47. Vasvani S, Kulkarni P, Rawtani D. Hyaluronic acid: A review on its biology, aspects of drug delivery, route of administrations and a special emphasis on its approved marketed products and recent clinical studies. Int J Biol Macromol (2020) 151:1012–29. doi: 10.1016/j.ijbiomac.2019.11.066
48. Murugaiah V, Agostinis C, Varghese PM, Belmonte B, Vieni S, Alaql FA, et al. Hyaluronic Acid Present in the Tumor Microenvironment Can Negate the Pro-apototic Effect of a Recombinant Fragment of Human Surfactant Protein D on Breast Cancer Cells. Front Immunol (2020) 11:1171. doi: 10.3389/fimmu.2020.01171
49. Jariyal H, Gupta C, Srivastava A. Hyaluronic acid induction on breast cancer stem cells unfolds subtype specific variations in stemness and epithelial-to-mesenchymal transition. Int J Biol Macromol (2020) 160:1078–89. doi: 10.1016/j.ijbiomac.2020.05.236
50. Ye S, Liu Y, Fuller AM, Katti R, Ciotti GE, Chor S, et al. TGFβ and Hippo Pathways Cooperate to Enhance Sarcomagenesis and Metastasis through the Hyaluronan-Mediated Motility Receptor (HMMR). Mol Cancer Res (2020) 18(4):560–73. doi: 10.1158/1541-7786.MCR-19-0877
51. Gaio E, Conte C, Esposito D, Reddi E, Quaglia F, Moret F. CD44 Targeting Mediated by Polymeric Nanoparticles and Combination of Chlorine TPCS2a-PDT and Docetaxel-Chemotherapy for Efficient Killing of Breast Differentiated and Stem Cancer Cells In Vitro. Cancers (Basel) (2020) 12(2):278. doi: 10.3390/cancers12020278
52. Narvaez CJ, LaPorta E, Robilotto S, Liang J, Welsh J. Inhibition of HAS2 and hyaluronic acid production by 1,25-Dihydroxyvitamin D3 in breast cancer. Oncotarget (2020) 11(30):2889–905. doi: 10.18632/oncotarget.27587
53. Heldin P, Basu K, Olofsson B, Porsch H, Kozlova I, Kahata K. Deregulation of hyaluronan synthesis, degradation and binding promotes breast cancer. J Biochem (2013) 154(5):395–408. doi: 10.1093/jb/mvt085
54. Masarwah A, Tammi M, Sudah M, Sutela A, Oikari S, Kosma VM, et al. The reciprocal association between mammographic breast density, hyaluronan synthesis and patient outcome. Breast Cancer Res Treat (2015) 153(3):625–34. doi: 10.1007/s10549-015-3567-0
55. Li J, Zha XM, Wang R, Li XD, Xu B, Xu YJ, et al. Regulation of CD44 expression by tumor necrosis factor-α and its potential role in breast cancer cell migration. BioMed Pharmacother (2012) 66(2):144–50. doi: 10.1016/j.biopha.2011.11.021
56. Ahn S, Lee KY, Parker KK, Shin K. Formation of Multi-Component Extracellular Matrix Protein Fibers. Sci Rep (2018) 8(1):1913. doi: 10.1038/s41598-018-20371-8
57. Cloutier G, Sallenbach-Morrissette A, Beaulieu JF. Non-integrin laminin receptors in epithelia. Tissue Cell (2019) 56:71–8. doi: 10.1016/j.tice.2018.12.005
58. Chang C, Goel HL, Gao H, Pursell B, Shultz LD, Greiner DL, et al. A laminin 511 matrix is regulated by TAZ and functions as the ligand for the α6Bβ1 integrin to sustain breast cancer stem cells. Genes Dev (2015) 29(1):1–6. doi: 10.1101/gad.253682.114
59. Simonova OA, Kuznetsova EB, Poddubskaya EV, Kekeeva TV, Kerimov RA, Trotsenko ID, et al. [DNA methylation in the promoter regions of the laminin family genes in normal and breast carcinoma tissues]. Mol Biol (Mosk) (2015) 49(4):667–77. doi: 10.7868/S0026898415040163
60. Carpenter PM, Ziogas A, Markham EM, Cantillep AS, Yan R, Anton-Culver H. Laminin 332 expression and prognosis in breast cancer. Hum Pathol (2018) 82:289–96. doi: 10.1016/j.humpath.2018.08.003
61. Fiore A, Spencer VA, Mori H, Carvalho HF, Bissell MJ, Bruni-Cardoso A. Laminin-111 and the Level of Nuclear Actin Regulate Epithelial Quiescence via Exportin-6. Cell Rep (2017) 19(10):2102–15. doi: 10.1016/j.celrep.2017.05.050
62. Kim BG, Gao MQ, Choi YP, Kang S, Park HR, Kang KS, et al. Invasive breast cancer induces laminin-332 upregulation and integrin β4 neoexpression in myofibroblasts to confer an anoikis-resistant phenotype during tissue remodeling. Breast Cancer Res (2012) 14(3):R88. doi: 10.1186/bcr3203
63. Kusuma N, Denoyer D, Eble JA, Redvers RP, Parker BS, Pelzer R, et al. Integrin-dependent response to laminin-511 regulates breast tumor cell invasion and metastasis. Int J Cancer (2012) 130(3):555–66. doi: 10.1002/ijc.26018
64. Singh P, Carraher C, Schwarzbauer JE. Assembly of fibronectin extracellular matrix. Annu Rev Cell Dev Biol (2010) 26:397–419. doi: 10.1146/annurev-cellbio-100109-104020
65. Fei D, Meng X, Yu W, Yang S, Song N, Cao Y, et al. Fibronectin (FN) cooperated with TLR2/TLR4 receptor to promote innate immune responses of macrophages via binding to integrin β1. Virulence (2018) 9(1):1588–600. doi: 10.1080/21505594.2018.1528841
66. Davidson LA, Keller R, DeSimone DW. Assembly and remodeling of the fibrillar fibronectin extracellular matrix during gastrulation and neurulation in Xenopus laevis. Dev Dyn (2004) 231(4):888–95. doi: 10.1002/dvdy.20217
67. Sevilla CA, Dalecki D, Hocking DC. Extracellular matrix fibronectin stimulates the self-assembly of microtissues on native collagen gels. Tissue Eng Part A (2010) 16(12):3805–19. doi: 10.1089/ten.TEA.2010.0316
68. Li K, Kang H, Wang Y, Hai T, Rong G, Sun H. Letrozole-induced functional changes in carcinoma-associated fibroblasts and their influence on breast cancer cell biology. Med Oncol (2016) 33(7):64. doi: 10.1007/s12032-016-0779-z
69. Luo H, Tu G, Liu Z, Liu M. Cancer-associated fibroblasts: a multifaceted driver of breast cancer progression. Cancer Lett (2015) 361(2):155–63. doi: 10.1016/j.canlet.2015.02.018
70. Erdogan B, Ao M, White LM, Means AL, Brewer BM, Yang L, et al. Cancer-associated fibroblasts promote directional cancer cell migration by aligning fibronectin. J Cell Biol (2017) 216(11):3799–816. doi: 10.1083/jcb.201704053
71. Tang X, Hou Y, Yang G, Wang X, Tang S, Du YE, et al. Stromal miR-200s contribute to breast cancer cell invasion through CAF activation and ECM remodeling. Cell Death Differ (2016) 23(1):132–45. doi: 10.1038/cdd.2015.78
72. Gilkes DM, Bajpai S, Chaturvedi P, Wirtz D, Semenza GL. Hypoxia-inducible factor 1 (HIF-1) promotes extracellular matrix remodeling under hypoxic conditions by inducing P4HA1, P4HA2, and PLOD2 expression in fibroblasts. J Biol Chem (2013) 288(15):10819–29. doi: 10.1074/jbc.M112.442939
73. Ulisse S, Baldini E, Sorrenti S, D’Armiento M. The urokinase plasminogen activator system: a target for anti-cancer therapy. Curr Cancer Drug Targets (2009) 9(1):32–71. doi: 10.2174/156800909787314002
74. Chen LC, Tu SH, Huang CS, Chen CS, Ho CT, Lin HW, et al. Human breast cancer cell metastasis is attenuated by lysyl oxidase inhibitors through down-regulation of focal adhesion kinase and the paxillin-signaling pathway. Breast Cancer Res Treat (2012) 134(3):989–1004. doi: 10.1007/s10549-012-1986-8
75. Zhang X, Huang S, Guo J, Zhou L, You L, Zhang T, et al. Insights into the distinct roles of MMP-11 in tumor biology and future therapeutics (Review). Int J Oncol (2016) 48(5):1783–93. doi: 10.3892/ijo.2016.3400
76. Jin H, Zhou S. The Functions of Heparanase in Human Diseases. Mini Rev Med Chem (2017) 17(6):541–8. doi: 10.2174/1389557516666161101143643
77. Eke I, Cordes N. Focal adhesion signaling and therapy resistance in cancer. Semin Cancer Biol (2015) 31:65–75. doi: 10.1016/j.semcancer.2014.07.009
78. Kesh K, Gupta VK, Durden B, Garrido V, Mateo-Victoriano B, Lavania SP, et al. Therapy Resistance, Cancer Stem Cells and ECM in Cancer: The Matrix Reloaded. Cancers (Basel) (2020) 12(10):3067. doi: 10.3390/cancers12103067
79. Leask A. A centralized communication network: Recent insights into the role of the cancer associated fibroblast in the development of drug resistance in tumors. Semin Cell Dev Biol (2020) 101:111–4. doi: 10.1016/j.semcdb.2019.10.016
80. Li M, Chen X, Yan J, Zhou L, Wang Y, He F, et al. Inhibition of osteoclastogenesis by stem cell-derived extracellular matrix through modulation of intracellular reactive oxygen species. Acta Biomater (2018) 71:118–31. doi: 10.1016/j.actbio.2018.03.003
81. Hoshiba T, Tanaka M. Decellularized matrices as in vitro models of extracellular matrix in tumor tissues at different malignant levels: Mechanism of 5-fluorouracil resistance in colorectal tumor cells. Biochim Biophys Acta (2016) 1863(11):2749–57. doi: 10.1016/j.bbamcr.2016.08.009
82. Brodaczewska K, Bielecka Z, Maliszewska-Olejniczak K, Szczylik C, Porta C, Bartnik E, et al. Metastatic renal cell carcinoma cells growing in 3D on poly−D−lysineor laminin present a stem−like phenotype and drug resistance. Oncol Rep (2019) 42(5):1878–92. doi: 10.3892/or.2019.7321.
83. Sethi T, Rintoul RC, Moore SM, MacKinnon AC, Salter D, Choo C, et al. Extracellular matrix proteins protect small cell lung cancer cells against apoptosis: a mechanism for small cell lung cancer growth and drug resistance in vivo. Nat Med (1999) 5(6):662–8. doi: 10.1038/9511
84. Insua-Rodríguez J, Pein M, Hongu T, Meier J, Descot A, Lowy CM, et al. Stress signaling in breast cancer cells induces matrix components that promote chemoresistant metastasis. EMBO Mol Med (2018) 10(10):e9003. doi: 10.15252/emmm.201809003
85. Janaki Ramaiah M, Vaishnave S. BMI1 and PTEN are key determinants of breast cancer therapy: A plausible therapeutic target in breast cancer. Gene (2018) 678:302–11. doi: 10.1016/j.gene.2018.08.022
86. Saatci O, Kaymak A, Raza U, Ersan PG, Akbulut O, Banister CE, et al. Targeting lysyl oxidase (LOX) overcomes chemotherapy resistance in triple negative breast cancer. Nat Commun (2020) 11(1):2416. doi: 10.1038/s41467-020-16199-4
87. Helleman J, Jansen MP, Ruigrok-Ritstier K, van Staveren IL, Look MP, Meijer-van Gelder ME, et al. Association of an extracellular matrix gene cluster with breast cancer prognosis and endocrine therapy response. Clin Cancer Res (2008) 14(17):5555–64. doi: 10.1158/1078-0432.CCR-08-0555
88. de Melo Gagliato D, Jardim DL, Marchesi MS, Hortobagyi GN. Mechanisms of resistance and sensitivity to anti-HER2 therapies in HER2+ breast cancer. Oncotarget (2016) 7(39):64431–46. doi: 10.18632/oncotarget.7043
89. Cordes N, Blaese MA, Plasswilm L, Rodemann HP, Van Beuningen D. Fibronectin and laminin increase resistance to ionizing radiation and the cytotoxic drug Ukrain in human tumour and normal cells in vitro. Int J Radiat Biol (2003) 79(9):709–20. doi: 10.1080/09553000310001610240
90. Afratis NA, Sagi I. Novel Approaches for Extracellular Matrix Targeting in Disease Treatment. Methods Mol Biol (2019) 1952:261–75. doi: 10.1007/978-1-4939-9133-4_21
91. Li Q, Cao J, He Y, Liu X, Mao G, Wei B, et al. R5, a neutralizing antibody to Robo1, suppresses breast cancer growth and metastasis by inhibiting angiogenesis via down-regulating filamin A. Exp Cell Res (2020) 387(1):111756. doi: 10.1016/j.yexcr.2019.111756
92. Iida J, Clancy R, Dorchak J, Somiari RI, Somiari S, Cutler ML, et al. DNA aptamers against exon v10 of CD44 inhibit breast cancer cell migration. PloS One (2014) 9(2):e88712. doi: 10.1371/journal.pone.0088712
93. Karalis TT, Heldin P, Vynios DH, Neill T, Buraschi S, Iozzo RV, et al. Tumor-suppressive functions of 4-MU on breast cancer cells of different ER status: Regulation of hyaluronan/HAS2/CD44 and specific matrix effectors. Matrix Biol (2019) 78-79:118–38. doi: 10.1016/j.matbio.2018.04.007
94. Tauro M, Shay G, Sansil SS, Laghezza A, Tortorella P, Neuger AM, et al. Bone-Seeking Matrix Metalloproteinase-2 Inhibitors Prevent Bone Metastatic Breast Cancer Growth. Mol Cancer Ther (2017) 16(3):494–505. doi: 10.1158/1535-7163.MCT-16-0315-T
95. Weissmann M, Arvatz G, Horowitz N, Feld S, Naroditsky I, Zhang Y, et al. Heparanase-neutralizing antibodies attenuate lymphoma tumor growth and metastasis. Proc Natl Acad Sci USA (2016) 113(3):704–9. doi: 10.1073/pnas.1519453113
96. Sussman D, Smith LM, Anderson ME, Duniho S, Hunter JH, Kostner H, et al. SGN-LIV1A: a novel antibody-drug conjugate targeting LIV-1 for the treatment of metastatic breast cancer. Mol Cancer Ther (2014) 13(12):2991–3000. doi: 10.1158/1535-7163.MCT-13-0896
97. Suvarna K, Honda K, Muroi M, Kondoh Y, Osada H, Watanabe N. A small-molecule ligand of valosin-containing protein/p97 inhibits cancer cell-accelerated fibroblast migration. J Biol Chem (2019) 294(9):2988–96. doi: 10.1074/jbc.RA118.004741
98. Flamini MI, Uzair ID, Pennacchio GE, Neira FJ, Mondaca JM, Cuello-Carrión FD, et al. Thyroid Hormone Controls Breast Cancer Cell Movement via Integrin αV/β3/SRC/FAK/PI3-Kinases. Horm Cancer (2017) 8(1):16–27. doi: 10.1007/s12672-016-0280-3
99. Guo H, Liu Y, Gu J, Wang Y, Liu L, Zhang P, et al. Endostatin inhibits the growth and migration of 4T1 mouse breast cancer cells by skewing macrophage polarity toward the M1 phenotype. Cancer Immunol Immunother (2016) 65(6):677–88. doi: 10.1007/s00262-016-1824-7
100. Chen J, Yao Q, Huang M, Wang B, Zhang J, Wang T, et al. A randomized Phase III trial of neoadjuvant recombinant human endostatin, docetaxel and epirubicin as first-line therapy for patients with breast cancer (CBCRT01). Int J Cancer (2018) 142(10):2130–8. doi: 10.1002/ijc.31217
101. Saito T, Tamura D, Nakamura T, Makita Y, Ariyama H, Komiyama K, et al. 4-methylumbelliferone leads to growth arrest and apoptosis in canine mammary tumor cells. Oncol Rep (2013) 29(1):335–42. doi: 10.3892/or.2012.2100
102. Gioiella F, Urciuolo F, Imparato G, Brancato V, Netti PA. An Engineered Breast Cancer Model on a Chip to Replicate ECM-Activation In Vitro during Tumor Progression. Adv Healthc Mater (2016) 5(23):3074–84. doi: 10.1002/adhm.201600772
103. Tan JX, Wang XY, Su XL, Li HY, Shi Y, Wang L, et al. Upregulation of HYAL1 expression in breast cancer promoted tumor cell proliferation, migration, invasion and angiogenesis. PloS One (2011) 6(7):e22836. doi: 10.1371/journal.pone.0022836
104. Stradecki HM, Jaworski DM. Hyperphagia and leptin resistance in tissue inhibitor of metalloproteinase-2 deficient mice. J Neuroendocrinol (2011) 23(3):269–81. doi: 10.1111/j.1365-2826.2010.02105.x
105. Shukla V, Kumar Shakya A, Dhole TN, Misra UK. Upregulated expression of matrix metalloproteinases and tissue inhibitors of matrix metalloproteinases in BALB/c mouse brain challenged with Japanese encephalitis virus. Neuroimmunomodulation (2012) 19(4):241–54. doi: 10.1159/000335182
106. Arpino V, Brock M, Gill SE. The role of TIMPs in regulation of extracellular matrix proteolysis. Matrix Biol (2015) 44-46:247–54. doi: 10.1016/j.matbio.2015.03.005
107. Kubota Y, Nishiwaki K, Ito M, Sugimoto A. The Role of Tissue Inhibitors of Metalloproteinases in Organ Development and Regulation of ADAMTS Family Metalloproteinases in Caenorhabditis elegans. Genetics (2019) 212(2):523–35. doi: 10.1534/genetics.119.301795
108. Radisky ES, Raeeszadeh-Sarmazdeh M, Radisky DC. Therapeutic Potential of Matrix Metalloproteinase Inhibition in Breast Cancer. J Cell Biochem (2017) 118(11):3531–48. doi: 10.1002/jcb.26185
109. Devel L, Rogakos V, David A, Makaritis A, Beau F, Cuniasse P, et al. Development of selective inhibitors and substrate of matrix metalloproteinase-12. J Biol Chem (2006) 281(16):11152–60. doi: 10.1074/jbc.M600222200
110. Devy L, Huang L, Naa L, Yanamandra N, Pieters H, Frans N, et al. Selective inhibition of matrix metalloproteinase-14 blocks tumor growth, invasion, and angiogenesis. Cancer Res (2009) 69(4):1517–26. doi: 10.1158/0008-5472.CAN-08-3255
111. Devel L, Garcia S, Czarny B, Beau F, LaJeunesse E, Vera L, et al. Insights from selective non-phosphinic inhibitors of MMP-12 tailored to fit with an S1’ loop canonical conformation. J Biol Chem (2010) 285(46):35900–9. doi: 10.1074/jbc.M110.139634
112. Arvatz G, Weissmann M, Ilan N, Vlodavsky I. Heparanase and cancer progression: New directions, new promises. Hum Vaccin Immunother (2016) 12(9):2253–6. doi: 10.1080/21645515.2016.1171442
113. Stagg J, Divisekera U, McLaughlin N, Sharkey J, Pommey S, Denoyer D, et al. Anti-CD73 antibody therapy inhibits breast tumor growth and metastasis. Proc Natl Acad Sci USA (2010) 107(4):1547–52. doi: 10.1073/pnas.0908801107
114. Doddamane I, Butler R, Jhaveri A, Chung GG, Cheng D. Where does radioimmunotherapy fit in the management of breast cancer? Immunotherapy (2013) 5(8):895–904. doi: 10.2217/imt.13.78
115. Trail PA, Dubowchik GM, Lowinger TB. Antibody drug conjugates for treatment of breast cancer: Novel targets and diverse approaches in ADC design. Pharmacol Ther (2018) 181:126–42. doi: 10.1016/j.pharmthera.2017.07.013
116. Pondé N, Aftimos P, Piccart M. Antibody-Drug Conjugates in Breast Cancer: a Comprehensive Review. Curr Treat Options Oncol (2019) 20(5):37. doi: 10.1007/s11864-019-0633-6
117. Huang X, Ye D, Thorpe PE. Enhancing the potency of a whole-cell breast cancer vaccine in mice with an antibody-IL-2 immunocytokine that targets exposed phosphatidylserine. Vaccine (2011) 29(29-30):4785–93. doi: 10.1016/j.vaccine.2011.04.082
118. Zeltz C, Primac I, Erusappan P, Alam J, Noel A, Gullberg D. Cancer-associated fibroblasts in desmoplastic tumors: emerging role of integrins. Semin Cancer Biol (2020) 62:166–81. doi: 10.1016/j.semcancer.2019.08.004
119. Hoshino A, Costa-Silva B, Shen TL, Rodrigues G, Hashimoto A, Tesic Mark M, et al. Tumour exosome integrins determine organotropic metastasis. Nature (2015) 527(7578):329–35. doi: 10.1038/nature15756
120. Primac I, Maquoi E, Blacher S, Heljasvaara R, Van Deun J, Smeland HY, et al. Stromal integrin α11 regulates PDGFR-β signaling and promotes breast cancer progression. J Clin Invest (2019) 129(11):4609–28. doi: 10.1172/JCI125890
121. Zuo XX, Yang Y, Zhang Y, Zhang ZG, Wang XF, Shi YG. Correction to: Platelets promote breast cancer cell MCF-7 metastasis by direct interaction: surface integrin α2β1-contacting-mediated activation of Wnt-β-catenin pathway. Cell Commun Signal (2020) 18(1):125. doi: 10.1186/s12964-020-00641-7
122. Zuo XX, Yang Y, Zhang Y, Zhang ZG, Wang XF, Shi YG. Platelets promote breast cancer cell MCF-7 metastasis by direct interaction: surface integrin α2β1-contacting-mediated activation of Wnt-β-catenin pathway. Cell Commun Signal (2019) 17(1):142. doi: 10.1186/s12964-019-0464-x
123. Bagati A, Kumar S, Jiang P, Pyrdol J, Zou AE, Godicelj A, et al. Integrin αvβ6-TGFβ-SOX4 Pathway Drives Immune Evasion in Triple-Negative Breast Cancer. Cancer Cell (2021) 39(1):54–67.e9. doi: 10.1016/j.ccell.2020.12.001
124. Pankova D, Chen Y, Terajima M, Schliekelman MJ, Baird BN, Fahrenholtz M, et al. Cancer-Associated Fibroblasts Induce a Collagen Cross-link Switch in Tumor Stroma. Mol Cancer Res (2016) 14(3):287–95. doi: 10.1158/1541-7786.MCR-15-0307
125. Ershaid N, Sharon Y, Doron H, Raz Y, Shani O, Cohen N, et al. NLRP3 inflammasome in fibroblasts links tissue damage with inflammation in breast cancer progression and metastasis. Nat Commun (2019) 10(1):4375. doi: 10.1038/s41467-019-12370-8
126. Wen S, Hou Y, Fu L, Xi L, Yang D, Zhao M, et al. Cancer-associated fibroblast (CAF)-derived IL32 promotes breast cancer cell invasion and metastasis via integrin β3-p38 MAPK signalling. Cancer Lett (2019) 442:320–32. doi: 10.1016/j.canlet.2018.10.015
127. Houthuijzen JM, Jonkers J. Cancer-associated fibroblasts as key regulators of the breast cancer tumor microenvironment. Cancer Metastasis Rev (2018) 37(4):577–97. doi: 10.1007/s10555-018-9768-3
128. Ferrari N, Ranftl R, Chicherova I, Slaven ND, Moeendarbary E, Farrugia AJ, et al. Dickkopf-3 links HSF1 and YAP/TAZ signalling to control aggressive behaviours in cancer-associated fibroblasts. Nat Commun (2019) 10(1):130. doi: 10.1038/s41467-018-07987-0
129. Zhao L, Sun Y, Hou Y, Peng Q, Wang L, Luo H, et al. MiRNA expression analysis of cancer-associated fibroblasts and normal fibroblasts in breast cancer. Int J Biochem Cell Biol (2012) 44(11):2051–9. doi: 10.1016/j.biocel.2012.08.005
130. Verghese ET, Drury R, Green CA, Holliday DL, Lu X, Nash C, et al. MiR-26b is down-regulated in carcinoma-associated fibroblasts from ER-positive breast cancers leading to enhanced cell migration and invasion. J Pathol (2013) 231(3):388–99. doi: 10.1002/path.4248
131. Donnarumma E, Fiore D, Nappa M, Roscigno G, Adamo A, Iaboni M, et al. Cancer-associated fibroblasts release exosomal microRNAs that dictate an aggressive phenotype in breast cancer. Oncotarget (2017) 8(12):19592–608. doi: 10.18632/oncotarget.14752
132. Santolla MF, Lappano R, Cirillo F, Rigiracciolo DC, Sebastiani A, Abonante S, et al. miR-221 stimulates breast cancer cells and cancer-associated fibroblasts (CAFs) through selective interference with the A20/c-Rel/CTGF signaling. J Exp Clin Cancer Res (2018) 37(1):94. doi: 10.1186/s13046-018-0767-6
133. Li K, Shi M, Qin S. Current Status and Study Progress of Recombinant Human Endostatin in Cancer Treatment. Oncol Ther (2018) 6(1):21–43. doi: 10.1007/s40487-017-0055-1
134. Xia F, Niu J, Hong Y, Li C, Cao W, Wang L, et al. Matrix metallopeptidase 2 targeted delivery of gold nanostars decorated with IR-780 iodide for dual-modal imaging and enhanced photothermal/photodynamic therapy. Acta Biomater (2019) 89:289–99. doi: 10.1016/j.actbio.2019.03.008
135. Guo W, Gao X, Zhan R, Zhao Z, Xu K, Tang B. Tricolor imaging of MMPs to investigate the promoting roles of inflammation on invasion and migration of tumor cells. Talanta (2021) 222:121525. doi: 10.1016/j.talanta.2020.121525
136. Suresh AK, Weng Y, Li Z, Zerda R, Van Haute D, Williams JC, et al. Matrix metalloproteinase-triggered denuding of engineered gold nanoparticles for selective cell uptake. J Mater Chem B (2013) 1(18):2341–9. doi: 10.1039/c3tb00435j
137. Kessenbrock K, Plaks V, Werb Z. Matrix metalloproteinases: regulators of the tumor microenvironment. Cell (2010) 141(1):52–67. doi: 10.1016/j.cell.2010.03.015
138. Liu J, Tian L, Zhang R, Dong Z, Wang H, Liu Z. Collagenase-Encapsulated pH-Responsive Nanoscale Coordination Polymers for Tumor Microenvironment Modulation and Enhanced Photodynamic Nanomedicine. ACS Appl Mater Interfaces (2018) 10(50):43493–502. doi: 10.1021/acsami.8b17684
139. Hu XX, He PP, Qi GB, Gao YJ, Lin YX, Yang C, et al. Transformable Nanomaterials as an Artificial Extracellular Matrix for Inhibiting Tumor Invasion and Metastasis. ACS Nano (2017) 11(4):4086–96. doi: 10.1021/acsnano.7b00781
140. Suo A, Xu W, Wang Y, Sun T, Ji L, Qian J. Dual-degradable and injectable hyaluronic acid hydrogel mimicking extracellular matrix for 3D culture of breast cancer MCF-7 cells. Carbohydr Polym (2019) 211:336–48. doi: 10.1016/j.carbpol.2019.01.115
141. Cho W, Kim MS, Lee KH, Park SJ, Shin HJ, Lee YJ, et al. Ionizing radiation attracts tumor targeting and apoptosis by radiotropic lysyl oxidase traceable nanoparticles. Nanomedicine (2020) 24:102141. doi: 10.1016/j.nano.2019.102141
142. Zhang L, Wang Y, Yang Y, Liu Y, Ruan S, Zhang Q, et al. High Tumor Penetration of Paclitaxel Loaded pH Sensitive Cleavable Liposomes by Depletion of Tumor Collagen I in Breast Cancer. ACS Appl Mater Interfaces (2015) 7(18):9691–701. doi: 10.1021/acsami.5b01473
143. Cun X, Ruan S, Chen J, Zhang L, Li J, He Q, et al. A dual strategy to improve the penetration and treatment of breast cancer by combining shrinking nanoparticles with collagen depletion by losartan. Acta Biomater (2016) 31:186–96. doi: 10.1016/j.actbio.2015.12.002
144. Guo Z, Hu K, Sun J, Zhang T, Zhang Q, Song L, et al. Fabrication of hydrogel with cell adhesive micropatterns for mimicking the oriented tumor-associated extracellular matrix. ACS Appl Mater Interfaces (2014) 6(14):10963–8. doi: 10.1021/am5023946
145. Baker BM, Trappmann B, Wang WY, Sakar MS, Kim IL, Shenoy VB, et al. Cell-mediated fibre recruitment drives extracellular matrix mechanosensing in engineered fibrillar microenvironments. Nat Mater (2015) 14(12):1262–8. doi: 10.1038/nmat4444
146. Chen Q, Liu G, Liu S, Su H, Wang Y, Li J, et al. Remodeling the Tumor Microenvironment with Emerging Nanotherapeutics. Trends Pharmacol Sci (2018) 39(1):59–74. doi: 10.1016/j.tips.2017.10.009
147. Yu YP, Wang Q, Liu YC, Xie Y. Molecular basis for the targeted binding of RGD-containing peptide to integrin αVβ3. Biomaterials (2014) 35(5):1667–75. doi: 10.1016/j.biomaterials.2013.10.072
148. Fittkau MH, Zilla P, Bezuidenhout D, Lutolf MP, Human P, Hubbell JA, et al. The selective modulation of endothelial cell mobility on RGD peptide containing surfaces by YIGSR peptides. Biomaterials (2005) 26(2):167–74. doi: 10.1016/j.biomaterials.2004.02.012
149. Barker HE, Chang J, Cox TR, Lang G, Bird D, Nicolau M, et al. LOXL2-mediated matrix remodeling in metastasis and mammary gland involution. Cancer Res (2011) 71(5):1561–72. doi: 10.1158/0008-5472.CAN-10-2868
150. Grossman M, Ben-Chetrit N, Zhuravlev A, Afik R, Bassat E, Solomonov I, et al. Tumor Cell Invasion Can Be Blocked by Modulators of Collagen Fibril Alignment That Control Assembly of the Extracellular Matrix. Cancer Res (2016) 76(14):4249–58. doi: 10.1158/0008-5472.CAN-15-2813
151. Barker HE, Chang J, Cox TR, Lang G, Bird D, Nicolau M, et al. Correction: LOXL2-Mediated Matrix Remodeling in Metastasis and Mammary Gland Involution. Cancer Res (2019) 79(19):5123. doi: 10.1158/0008-5472.CAN-19-2420
152. Tang S, Yin Q, Su J, Sun H, Meng Q, Chen Y, et al. Inhibition of metastasis and growth of breast cancer by pH-sensitive poly (β-amino ester) nanoparticles co-delivering two siRNA and paclitaxel. Biomaterials (2015) 48:1–15. doi: 10.1016/j.biomaterials.2015.01.049
153. Mauro N, Scialabba C, Puleio R, Varvarà P, Licciardi M, Cavallaro G, et al. SPIONs embedded in polyamino acid nanogels to synergistically treat tumor microenvironment and breast cancer cells. Int J Pharm (2019) 555:207–19. doi: 10.1016/j.ijpharm.2018.11.046
Keywords: extracellular matrix, breast cancer, therapeutic targets, nanoparticles, remodeling enzymes
Citation: Zhao Y, Zheng X, Zheng Y, Chen Y, Fei W, Wang F and Zheng C (2021) Extracellular Matrix: Emerging Roles and Potential Therapeutic Targets for Breast Cancer. Front. Oncol. 11:650453. doi: 10.3389/fonc.2021.650453
Received: 08 January 2021; Accepted: 25 March 2021;
Published: 22 April 2021.
Edited by:
Qingxin Mu, University of Washington, United StatesReviewed by:
Alexander Deneka, Fox Chase Cancer Center, United StatesLuis E. Arias-Romero, National Autonomous University of Mexico, Mexico
Copyright © 2021 Zhao, Zheng, Zheng, Chen, Fei, Wang and Zheng. This is an open-access article distributed under the terms of the Creative Commons Attribution License (CC BY). The use, distribution or reproduction in other forums is permitted, provided the original author(s) and the copyright owner(s) are credited and that the original publication in this journal is cited, in accordance with accepted academic practice. No use, distribution or reproduction is permitted which does not comply with these terms.
*Correspondence: Yunchun Zhao, NTUxNTA1OUB6anUuZWR1LmNu; Caihong Zheng, Y2h6aGVuZ0B6anUuZWR1LmNu
†These authors have contributed equally to this work and share first authorship