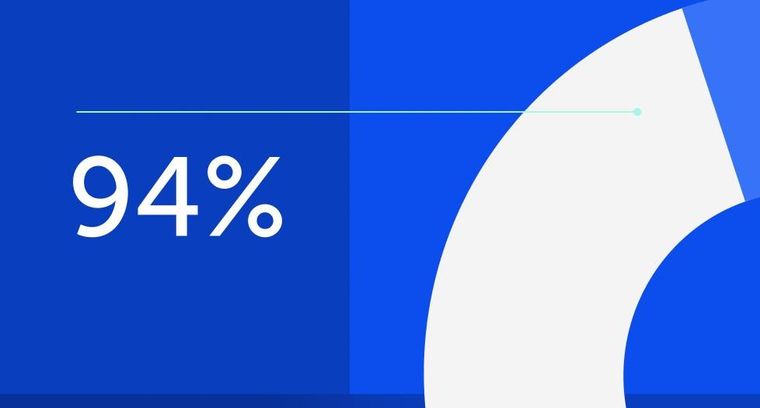
94% of researchers rate our articles as excellent or good
Learn more about the work of our research integrity team to safeguard the quality of each article we publish.
Find out more
REVIEW article
Front. Oncol., 25 May 2021
Sec. Radiation Oncology
Volume 11 - 2021 | https://doi.org/10.3389/fonc.2021.644400
The biological effects of radiation dose to organs at risk surrounding tumor target volumes are a major dose-limiting constraint in radiotherapy. This can mean that the tumor cannot be completely destroyed, and the efficacy of radiotherapy will be decreased. Thus, ways to reduce damage to healthy tissue has always been a topic of particular interest in radiotherapy research. Modern radiotherapy technologies such as helical tomotherapy (HT), intensity-modulated radiation therapy (IMRT), and proton radiotherapy can reduce radiation damage to healthy tissues. Recent outcomes of animal experiments show that FLASH radiotherapy (FLASH-RT) can reduce radiation-induced damage in healthy tissue without decreasing antitumor effectiveness. The very short radiotherapy time compared to that of conventional dose-rate radiotherapy is another advantage of FLASH-RT. The first human patient received FLASH-RT in Switzerland in 2018. FLASH-RT may become one of the main radiotherapy technologies in clinical applications in the future. We summarize the history of the development of FLASH-RT, its mechanisms, its influence on radiotherapy, and its future.
Radiotherapy is required by 60–70% of cancer patients during their treatment (1). Radiotherapy is the most widely used and effective anti-tumor therapy (2), but it can cause acute and late damage to healthy tissues. The dose delivered to the tumor is, hence, limited by the toxicity to nearby healthy tissue; this can mean that a tumor cannot be completely killed, and the efficacy of radiotherapy will be decreased. Thus, preventing or mitigating radiation-induced healthy tissue injury has always been a topic of particular interest in radiotherapy research. Modern radiotherapy technologies, such as intensity-modulated radiation therapy (IMRT), helical tomotherapy (HT), and proton radiotherapy can reduce radiation damage to healthy tissues. For example, IMRT reduces the incidence of grade 2–4 xerostomia in patients with head and neck cancers without compromising loco-regional control and overall survival (3). HT can also reduce the incidence of skin toxicity in breast cancer patients (4). The advantage of proton radiotherapy is that most of the energy is transferred to the position of the “Bragg peak,” and energy transfer outside of this point is very low (5). Thus, the radiation damage to healthy tissues outside the Bragg peak is relatively small, and proton therapy causes less radiation damage to healthy tissues than photon therapy when treating head and neck cancers, prostate cancer, and pediatric cancer, among others (6–8).
FLASH radiotherapy (FLASH-RT) is a novel radiotherapy technology defined as a single ultra-high dose-rate (≥ 40 Gy/s) radiotherapy. Compared with conventional dose-rate irradiation, FLASH irradiation is 400-fold more rapid than conventional irradiation (Figure 1). Recent animal experiments have shown that FLASH-RT can reduce radiation-induced damage in healthy tissues (9). In the first patient with T-cell cutaneous lymphoma who received FLASH-RT, the anti-tumor effect was rapid and long-lasting; moreover, only grade 1 epithelitis and grade 1 edema occurred in the soft tissues surrounding the tumor (10). In this first clinical use of FLASH-RT, the treatment time was only 90 ms. Compared with conventional dose-rate radiotherapy, the very short radiotherapy time is another advantage of FLASH-RT. Considering that FLASH-RT can reduce the damage to healthy tissue and the advantages of the short treatment time, we have reason to predict that FLASH radiotherapy may become one of the main radiotherapy technologies in clinical practice in the future.
In this paper, we summarize the history of development of FLASH-RT, its mechanism, its influence on radiotherapy, and its future.
Before 2014, FLASH-RT was referred to as the flash effect, which was first reported by Dewey and Boag in 1959. Ultra-high dose-rate 1.5-MV X-rays were used to irradiate a bacterium, Serratia marcescens (11). This study showed that S. marcescens in a nitrogen-oxygen mixture containing 1% oxygen is more radiosensitive than when in 100% nitrogen with normal dose-rate irradiation (1000 rads/min). However, when the ultra-high dose-rate (10-20 kilorads/2 µs) was used, S. marcescens in the same nitrogen-oxygen mixture showed lower radiosensitivity, corresponding to anaerobic irradiation. In summary, Dewey and Boag’s study outlined that ultra-high dose-rate irradiation can protect bacteria when compared to conventional dose-rate irradiation. Similar results were observed in mammalian cells in later studies. Town reported that when mammalian cells received the same dose ultra-high dose-rate (3.5×109 rad/s) irradiation, and the dose reached up to 1000 rads, one pulse had a higher survival rate than two pulses (12). Berry et al. showed similar results in hamster cells and HeLa cells using ultra-high dose-rate (1,000 rads for the 15-ns pulse) irradiation (13). A series of experiments showed that the flash effect is related to oxygen consumption (14–18). In 2014, Favaudon reported that using FLASH-RT to treat lung tumors can lead to a complete response and reduce the early and late toxicity affecting normal lung tissue; subsequently, FLASH-RT has become a topic of particular interest in radiation research (19). A series of studies have shown that FLASH-RT delivers dramatically reduced adverse side effects in the healthy tissue of mice (20–26), and this effect was greater in mini-pig and cat (27). Finally, a T-cell cutaneous lymphoma human patient received FLASH-RT, and long-lasting complete tumor response was achieved with fewer side effects than those expected with conventional radiotherapy (10). Table 1 summarizes the preclinical and clinical evidence supporting FLASH-RT.
The biological mechanism of FLASH-RT is very complex (Figure 2). Dewey and Boag first reported the effects of ultra-high dose-rate, which showed that hypoxia was induced following high dose-rate radiotherapy of bacteria (11). This phenomenon can be explained by local oxygen consumption because the rapid deposition of radiation energy occurs too fast to maintain sufficient oxygenation levels. A series of studies have shown that ultra-high dose-rate irradiation can induce the protection of mammalian cells through transient hypoxia (including cancer cells) (14–18). A recent study showed that hyperoxia can eliminate flash effects in mice (33). Another theory is that the number of DNA damage sites in FLASH-RT is less than that following conventional dose-rate irradiation. Some authors have shown that FLASH-RT causes fewer dicentric chromosomes to be formed than conventional dose-rate irradiation, and there was a difference in G2 cell cycle arrest after FLASH-RT and conventional dose-rate irradiation (34–37). Another study showed that radiation with short pulses leads to fewer late side effects in healthy tissue than conventional dose-rate irradiation (38). Recently, Kim et al. showed that myosin light chain activation plays an important role in the separation of biological effects between FLASH-RT and conventional dose-rate irradiation (39).
However, those studies did not demonstrate the same protective effect between healthy and tumor tissues in vivo. In vivo, FLASH-RT leads to differential responses between healthy and tumor tissues. Compared with conventional dose-rate irradiation, FLASH-RT can reduce radiation-induced lung fibrosis and has the same antitumor effectiveness in mice (19). Why are there differential responses between healthy and tumor tissues in vivo? A theory is that the different types of DNA damage caused by FLASH-RT and conventional dose-rate irradiation result in the differential responses of healthy and tumor tissue (33, 40, 41). Another theory is that solid tumors are hypoxic, so they will not be protected from the transient hypoxia caused by FLASH-RT, while healthy tissues will be, resulting in the differential effect (23, 33). In addition, some authors believe that cancer cells and normal cells have different abilities to scavenge hydrogen peroxide products. FLASH-RT instantaneously consumes oxygen in all local tissues and produces hydrogen peroxide products. Healthy tissue cells have a lower oxidant load and higher catalase reduction reserve capacity; therefore, healthy tissue can remove hydrogen peroxide products more easily than tumor tissue (42).
In general, previous studies have agreed that FLASH-RT leads to local oxygen consumption that is much faster than tissue oxygenation, which results in transient radiation-induced hypoxia. Interestingly, some researchers believed that FLASH-RT using carbon ions would improve therapeutic ratio with greater toxicity in the tumor due to the generation of oxygen at the spread-out Bragg peak (43). Hence, the mechanism behind the differential responses of healthy and tumor tissues remains unclear and the various explanatory hypotheses require more experimental verification (44).
FLASH-RT might potentially change the theories of radiobiology as follows. The first change may be in the five Rs of radiobiology: DNA repair, reoxygenation, repopulation, redistribution, and intrinsic radiosensitivity (45). The delivery time of FLASH-RT is too short for reoxygenation, repopulation, and redistribution to occur, or reoxygenation, repopulation, and redistribution may occur but cannot influence the effect of radiotherapy because FLASH-RT is performed only once. Therefore, FLASH-RT may be related to two Rs: DNA repair and intrinsic radiosensitivity. The second change may be the limit dose of healthy tissue because preclinical studies have confirmed that compared with conventional dose-rate irradiation, FLASH-RT needs a higher dose to cause the same degree of toxicity. As a result, when healthy tissue is irradiated in FLASH-RT, its α/β value will change. Conventional dose-rate irradiation (15 Gy) triggers lung fibrosis, but higher dose FLASH-RT (20 Gy) does not cause lung fibrosis after 36 weeks. Regarding skin toxicity, both 20 Gy and 15 Gy FLASH-RT do not exhibit macroscopic signs of cutaneous lesions, but 17-Gy conventional dose-rate irradiation can lead to severe cutaneous lesions within the irradiated field (19). Compared with conventional dose-rate radiotherapy, FLASH-RT also shows protective effects in other healthy tissues, including the brain and digestive tract (9, 16, 20, 21, 27, 46, 47). A series of animal experiments have shown that the tolerance of healthy tissues is increased; thus, when FLASH-RT is used, the α/β value of healthy tissues will differ from the current value using in conventional dose-rate irradiation. The third potential change is the comprehensive treatment strategy. For example, how can radiotherapy be combined with chemotherapy? Because FLASH-RT is performed only once for a very short time, concurrent chemoradiotherapy is not possible; only neoadjuvant and adjuvant chemotherapy can be administered. The fourth change may be the fraction of radiotherapy. If FLASH-RT technology is used in clinical practice and gradually develops, single fraction radiotherapy will be widely used to replace the current multiple fractions of radiotherapy.
Additional animal experiments are needed to prove that FLASH-RT can provide more protection to healthy tissues. Favaudon et al. reported that FLASH-RT has lower rates of acute and late pulmonary toxicity after thoracic irradiation of mice than conventional dose-rate irradiation at the same dose (19). However, the two groups were irradiated with different rays (FLASH-RT with 4.5 MeV electrons vs. conventional dose-rate irradiation with Cs-137 photons). The protective effect of FLASH-RT in Favaudon′s study may be due to the different physical characteristics of electrons and photons. Because the energy of 4.5 MeV of electrons is mainly deposited on the surface, FLASH-RT delivers a lower dose to deep tissue (lung) when compared with that with conventional dose-rate irradiation. In another study of the brains of mice, FLASH-RT was applied using X-ray (21). In this study, the shape of the radiation field was different, although the circular radiation field of FLASH-RT was equivalent in area to the square radiation field of conventional dose-rate radiotherapy. Future experiments should be designed to use the same conditions, for example, by using the same rays and same shape of radiation field. Moreover, other organs should also be examined in future experiments.
Different modified irradiation systems can produce FLASH dose-rates, including electron linear accelerators, synchrotron light sources, and proton accelerators. Electron beam radiotherapy could be a source of FLASH-RT. The two prototype linear accelerators were modified to generate 4.5 MeV and 6 MeV electron beams with dose-rates in the thousands of Gy/s (19). High dose-rate (18000 Gy/s) X-ray from a synchrotron light source can also produce a FLASH effect (21). The proton cyclotron can also produce high-energy rays with a dose-rate of more than 40 Gy/s for FLASH-RT (48). However, most of the equipment can only be used in animal experiments. Only one setup was used to treat a T-cell cutaneous lymphoma patient, and the radiotherapeutic fields of this setup were small. In the future, equipment should be modified to allow radiotherapy over larger fields that are suitable for treating cancer patients. Electrons, X-rays, and protons can be used for FLASH-RT; however, the electron beam is only suitable for superficial tumors. Protons are suitable for the treatment of deep tumors. The first proton FLASH-RT equipment has obtained investigational device exemption (IDE) for clinical trials from the US Food and Drug Administration (49). However, the equipment for proton FLASH-RT is expensive. Because X-ray equipment is widely used all over the world and cheaper than that required for proton therapy, it is also suitable for treating deep tumors. Therefore, in our opinion, future development in the equipment for FLASH-RT should be focused on modifying X-ray equipment.
Future FLASH-RT devices may need to have the function of multiple-field conformal radiation. Multiple-field conformal radiation can reduce healthy tissue toxicity compared to the use of a single regular field or two regular fields. Multiple-field conformal radiation can be achieved using mechanical gantry rotation and movement of the multileaf collimator. In a previous study, one-pulse FLASH-RT provided greater protection than two-pulse therapy at the same dose and dose-rate, so one pulse will be the best choice (13). The time for one pulse is too short for the movement of the multileaf collimator and mechanical gantry rotation, so a multi-mechanical gantry will be needed, and the multileaf collimator should be moved to the corresponding position in advance to form a conformal region. Equipment similar to the Gamma Knife can meet the above requirements, but it will be very different from the Gamma Knife. Based on the above principles, some researchers have developed a PHASER radiotherapy system that can provide highly conformal intensity modulated FLASH-RT for tumors (50). However, there is still a long way to go before it can be used clinically.
Furthermore, before FLASH-RT is used clinically, two problems need to be solved. First, because of the differences between animal models and humans, the FLASH effect should be confirmed in cancer patients. Acute and late toxicity in different organs should be monitored. Second, because FLASH-RT can be completed in a single sitting, the definitive irradiation dose for different cancers needs to be redefined. Radiation oncologists should rebalance the effect of irradiation and healthy tissue toxicity and then define the radical irradiation dose. This may require the treatment of many cancer patients and a long time before this is satisfactorily defined. It may take many years before FLASH-RT becomes a mainstay radiotherapy technology in clinical applications.
From 1959 to 2020, FLASH-RT has progressed from bench to bedside. FLASH-RT causes rapid depletion of oxygen, eliciting transient hypoxia. However, the exact mechanism of differential responses between healthy and tumor tissues is unclear. FLASH-RT may bring changes in radiobiology, limit radiation doses to healthy tissue, and promote new methods of combining radiotherapy with other antitumor treatments. Before FLASH-RT becomes the main radiotherapy technology in clinical application, more animal experiments are needed. The delivery should be modified to fit larger fields of radiotherapy, involve conformal radiation to multiple fields, redefine the dose limits to healthy tissue, and radical irradiation dose of cancer. In conclusion, for FLASH-RT, the road is tortuous but the future is bright.
BL and FG draft the manuscript, YY, DW, YZ, GF, and TD participated in the data review and collection for the study. XD conceived of the study, and participated in its design and coordination. All authors contributed to the article and approved the submitted version.
The authors declare that the research was conducted in the absence of any commercial or financial relationships that could be construed as a potential conflict of interest.
1. Baumann M, Krause M, Overgaard J, Debus J, Bentzen SM, Daartz J, et al. Radiation Oncology in the Era of Precision Medicine. Nat Rev Cancer (2016) 16:234–49. doi: 10.1038/nrc.2016.18
3. Marta GN, Silva V, de Andrade Carvalho H, de Arruda FF, Hanna SA, Gadia R, et al. Intensity-Modulated Radiation Therapy for Head and Neck Cancer: Systematic Review and Meta-Analysis. Radiother Oncol (2013) 110(1):9–15. doi: 10.1016/j.radonc.2013.11.010
4. Lancellotta V, Chierchini S, Perrucci E, Saldi S, Falcinelli L, Iacco M, et al. Skin Toxicity After Chest Wall/Breast Plus Level III-IV Lymph Nodes Treatment With Helical Tomotherapy. Cancer Invest (2018) 36(9):1–8. doi: 10.1080/07357907.2018.1545854
5. Newhauser WD, Zhang R. The Physics of Proton Therapy. Phys Med Biol (2015) 60:R155–209. doi: 10.1088/0031-9155/60/8/R155
6. Jakobi A, Bandurska-Luque A, Stützer K, Haase R, Löck S, Wack LJ, et al. Identification of Patient Benefit From Proton Therapy for Advanced Head and Neck Cancer Patients Based on Individual and Subgroup Normal Tissue Complication Probability Analysis. Int J Radiat Oncol Biol Phys (2015) 92:1165–74. doi: 10.1016/j.ijrobp.2015.04.031
7. Colaco RJ, Hoppe BS, Flampouri S, McKibben BT, Henderson RH, Bryant C, et al. Rectal Toxicity After Proton Therapy for Prostate Cancer: an Analysis of Outcomes of Prospective Studies Conducted At the University of Florida Proton Therapy Institute. Int J Radiat Oncol Biol Phys (2015) 91:172–81. doi: 10.1016/j.ijrobp.2014.08.353
8. Leroy R, Benahmed N, Hulstaert F, Van Damme N, De Ruysscher D. Proton Therapy in Children: A Systematic Review of Clinical Effectiveness in 15 Pediatric Cancers. Int J Radiat Oncol Biol Phys (2016) 95:267–78. doi: 10.1016/j.ijrobp.2015.10.025
9. Loo BW, Schuler E, Lartey FM, Rafat M, King GJ, Trovati S, et al. (P003) Delivery of Ultra-Rapid Flash Radiation Therapy and Demonstration of Normal Tissue Sparing After Abdominal Irradiation of Mice. Int J Radiat Oncol (2017) 98:E16. doi: 10.1016/j.ijrobp.2017.02.101
10. Bourhis J, Sozzi WJ, Jorge PG, Gaide O, Bailat C, Duclos F, et al. Treatment of a First Patient With FLASH-Radiotherapy. Radiother Oncol (2019) 139:18–22. doi: 10.1016/j.radonc.2019.06.019
11. Dewey DL, Boag JW. Modification of the Oxygen Effect When Bacteria Are Given Large Pulses of Radiation. Nature (1959) 183(4673):1450. doi: 10.1038/1831450a0
12. Town CD. Effect of High Dose-Rates on Survival of Mammalian Cells. Nature (1967) 215(5103):847–8. doi: 10.1038/215847a0
13. Berry RJ, Hall EJ, Forster DW, Storr TH, Goodman MJ. Survival of Mammalian Cells Exposed to X Rays At Ultra-High Dose-Rates. Br J Radiol (1969) 42(494):102–7. doi: 10.1259/0007-1285-42-494-102
14. Hornsey S, Bewley DK. Hypoxia in Mouse Intestine Induced by Electron Irradiation At High Dose-Rates. Int J Radiat Biol (1971) 19(5):479–83. doi: 10.1080/09553007114550611
15. Berry RJ, Stedeford JBH. Reproductive Survival of Mammalian Cells After Irradiation At Ultra-High Dose-Rates: Further Observations and Their Importance for Radiotherapy. Br J Radiol (1972) 45(531):171–7. doi: 10.1259/0007-1285-45-531-171
16. Field SB, Bewley DK. Effects of Dose-Rate on the Radiation Response of Rat Skin. Int J Radiat Biol Related Stud Phys Chem Med (1974) 26(3):259–267.17. doi: 10.1080/09553007414551221
17. Weiss H, Epp ER, Heslin JM, Ling CC, Santomasso A. Oxygen Depletion in Cells Irradiated At Ultra-High Dose-Rates and At Conventional Dose-Rates. Int J Radiat Biol Related Stud Phys Chem Med (1974) 26(1):17–29. doi: 10.1080/09553007414550901
18. Michaels HB, Epp ER, Ling CC, Peterson EC. Oxygen Sensitization of CHO Cells At Ultrahigh Dose Rates: Prelude to Oxygen Diffusion Studies. Radiat Res (1979) 76(3):510–21. doi: 10.2307/3574800
19. Favaudon V, Caplier L, Monceau V, Pouzoulet F, Sayarath M, Fouillade C, et al. Ultrahigh Dose-Rate Flash Irradiation Increases the Differential Response Between Normal and Tumor Tissue in Mice. Sci Trans Med (2014) 6(245):245ra93–245ra93. doi: 10.1126/scitranslmed.3008973
20. Montay-Gruel P, Petersson K, Jaccard M, Boivin G, Germond JF, Petit B, et al. Irradiation in a Flash: Unique Sparing of Memory in Mice After Whole Brain Irradiation With Dose Rates Above 100, Gy/s. Radiother Oncol (2017) 124(3):S0167814017303651. doi: 10.1016/j.radonc.2017.05.003
21. Pierre MG, Audrey B, Maud J, David P, Raphael S, Warren A, et al. X-Rays can Trigger the Flash Effect: Ultra-High Dose-Rate Synchrotron Light Source Prevents Normal Brain Injury After Whole Brain Irradiation in Mice. Radiother Oncol (2018) 129(3):S0167814018334546. doi: 10.1016/j.radonc.2018.08.016
22. Buonanno M, Grilj V, Brenner DJ. Biological Effects in Normal Cells Exposed to FLASH Dose Rate Protons. Radiother Oncol (2019) 139:51–55. doi: 10.1016/j.radonc.2019.02.009
23. Montay-Gruel P, Acharya MM, Petersson K, Alikhani L, Yakkala C, Allen BD, et al. Long-Term Neurocognitive Benefits of FLASH Radiotherapy Driven by Reduced Reactive Oxygen Species. Proc Natl Acad Sci USA (2019) 116(22):10943–51. doi: 10.1073/pnas.1901777116
24. Simmons DA, Lartey FM, Schüler E, Rafat M, King G, Kim A, et al. Reduced Cognitive Deficits After FLASH Irradiation of Whole Mouse Brain Are Associated With Less Hippocampal Dendritic Spine Loss and Neuroinflammation. Radiother Oncol (2019) 139:4–10. doi: 10.1016/j.radonc.2019.06.006
25. Chabi S, Van To TH, Leavitt R, Poglio S, Jorge PG, Jaccard M, et al. Ultra-High Dose Rate FLASH and Conventional Dose Rate Irradiation Differentially Affect Human Acute Lymphoblastic Leukemia and Normal Hematopoiesis. Int J Radiat Oncol Biol Phys (2020) 109(3):S0360–3016(20)34400-X. doi: 10.1016/j.ijrobp.2020.10.012
26. Montay-Gruel P, Acharya MM, Gonçalves Jorge P, Petit B, Petridis IG, Fuchs P, et al. Hypo-Fractionated FLASH-RT as an Effective Treatment Against Glioblastoma That Reduces Neurocognitive Side Effects in Mice. Clin Cancer Res (2020) 27(3):clincanres.0894.2020. doi: 10.1158/1078-0432
27. Vozenin MC, De Fornel P, Petersson K, Favaudon V, Jaccard M, Germond JF, et al. The Advantage of Flash Radiotherapy Confirmed in Mini-Pig and Cat-Cancer Patients. Clin Cancer Res (2019) 25(1):35–42. doi: 10.1158/1078-0432.CCR-17-3375
28. Venkatesulu BP, Sharma A, Pollard-Larkin JM, Sadagopan R, Symons J, Neri S, et al. Ultra High Dose Rate (35 Gy/Sec) Radiation Does Not Spare the Normal Tissue in Cardiac and Splenic Models of Lymphopenia and Gastrointestinal Syndrome. Sci Rep (2019) 9(1):17180. doi: 10.1038/s41598-019-53562-y
29. Fouillade C, Curras-Alonso S, Giuranno L, Quelennec E, Heinrich S, Bonnet-Boissinot S, et al. Flash Irradiation Spares Lung Progenitor Cells and Limits the Incidence of Radio-Induced Senescence. Clin Cancer Res (2020) 26(6):1497–506. doi: 10.1158/1078-0432.CCR-19-1440
30. Favaudon V, Fouillade C, Vozenin M-C. Radiothérapie « Flash » À Très Haut Debit De Dose: Un Moyen D’augmenter L’indice Thérapeutique Par Minimization Des Dommages Aux Tissus Sains? Cancer/Radiothérapie (2015) 19:526–31. doi: 10.1016/j.canrad.2015.04.006
31. Adrian G, Konradsson E, Lempart M, Bäck S, Ceberg C, Petersson K. The FLASH Effect Depends on Oxygen Concentration. Br J Radiol (2020) 93(1106):20190702. doi: 10.1259/bjr.20190702
32. Beyreuther E, Brand M, Hans S, Hideghéty K, Karsch L, Leßmann E, et al. Feasibility of Proton FLASH Effect Tested by Zebrafish Embryo Irradiation. Radiother Oncol (2019) 139:46–50. doi: 10.1016/j.radonc.2019.06.024
33. Bourhis J, Montay-Gruel P, Gonçalves Jorge P, Bailat C, Petit B, Ollivier J, et al. Clinical Translation of FLASH Radiotherapy: Why and How? Radiother Oncol (2019) 139:11–7. doi: 10.1016/j.radonc.2019.04.008
34. Prempree T, Michelsen A, Merz T. The Repair Time of Chromosome Breaks Induced by Pulsed X-Rays on Ultra-High Dose-Rate. Int J Radiat Biol Relat Stud Phys Chem Med (1969) 15:571–4. doi: 10.1080/09553006914550871
35. Schmid TE, Dollinger G, Hable V, Greubel C, Zlobinskaya O, Michalski D, et al. Relative Biological Effectiveness of Pulsed and Continuous 20 Mev Protons for Micronucleus Induction in 3D Human Reconstructed Skin Tissue. Radiother Oncol (2010) 95:66–72. doi: 10.1016/j.radonc.2010.03.010
36. Schmid TE, Dollinger G, Hable V, Greubel C, Zlobinskaya O, Michalski D, et al. The Effectiveness of 20 Mev Protons At Nanosecond Pulse Lengths in Producing Chromosome Aberrations in Human-Hamster Hybrid Cells. Radiat Res (2011) 175:719–27. doi: 10.1667/RR2465.1
37. Auer S, Hable V, Greubel C, Drexler GA, Schmid TE, Belka C, et al. Survival of Tumor Cells After Proton Irradiation With Ultra-High Dose Rates. Radiat Oncol (2011) 6:139. doi: 10.1186/1748-717X-6-139
38. Rong Y, Paliwal B, Howard SP, Welsh J. Treatment Planning for Pulsed Reduced Dose-Rate Radiotherapy in Helical Tomotherapy. In J Radiat Oncol Biol Phys (2011) 79:934–42. doi: 10.1016/j.ijrobp.2010.05.055
39. Kim YE, Gwak SH, Hong BJ, Oh JM, Choi HS, Kim MS, et al. Effects of Ultra-High Dose Rate FLASH Irradiation on the Tumor Microenvironment in Lewis Lung Carcinoma: Role of Myosin Light Chain. Int J Radiat Oncol Biol Phys (2020) 109(5):S0360–3016(20)34500-4. doi: 10.1016/j.ijrobp.2020.11.012
40. Fernet M, Ponette V, Deniaud-Alexandre E, Ménissier-DeMurcia J, De Murcia G, Giocanti N, et al. Poly(ADP-Ribose) Polymerase, a Major Determinant of Early Cell Response to Ionizing Radiation. Int J Radiat Biol (2000) 76:1621–9. doi: 10.1080/09553000050201118
41. Ponette V, Le Péchoux C, Deniaud-Alexandre E, Fernet M, Giocanti N, Tourbez H, et al. Hyperfast, Early Cell Response to Ionizing Radiation. Int J Radiat Biol (2000) 76:1233–43. doi: 10.1080/09553000050134465
42. Spitz DR, Buettner GR, Petronek MS, St-Aubin JJ, Flynn RT, Waldron TJ, et al. An Integrated Physico-Chemical Approach for Explaining the Differential Impact of Flash Versus Conventional Dose Rate Irradiation on Cancer and Normal Tissue Responses. Radiother Oncol (2019) 139:23–7. doi: 10.1016/j.radonc.2019.03.028
43. Zakaria AM, Colangelo NW, Meesungnoen J, Azzam EI, Plourde MÉ, Jay-Gerin JP. Ultra-High Dose-Rate, Pulsed (Flash) Radiotherapy With Carbon Ions: Generation of Early, Transient, Highly Oxygenated Conditions in the Tumor Environment. Radiat Res (2020) 194(6):587–93. doi: 10.1667/RADE-19-00015.1
44. Griffin RJ, Ahmed MM, Amendola B, Belyakov O, Bentzen SM, Butterworth KT, et al. Understanding High-Dose, Ultra-High Dose Rate, and Spatially Fractionated Radiation Therapy. Int J Radiat Oncol Biol Phys (2020) 107(4):766–78. doi: 10.1016/j.ijrobp.2020.03.028
45. Steel GG, McMillan TJ, Peacock JH. The 5Rs of Radiology. Int J Radiat Biol (1989) 56:1045–8. doi: 10.1080/09553008914552491
46. Hornsey S, Alper T. Unexpected Dose-Rate Effect in the Killing of Mice by Radiation. Nature (1966) 210:212–3. doi: 10.1038/210212a0
47. Hendry JH, Moore JV, Hodgson BW, Keene JP. The Constant Low Oxygen Concentration in All the Target Cells for Mouse Tail Radionecrosis. Radiat Res (1982) 92:172–81. doi: 10.2307/3575852
48. Patriarca A, Fouillade C, Auger M, Martin F, Pouzoulet F, Nauraye C, et al. Experimental Set-Up for FLASH Proton Irradiation of Small Animals Using a Clinical System. Int J Radiat Oncol Biol Phys (2018) 102:619–26. doi: 10.1016/j.ijrobp.2018.06.403
49. Varian Receives Investigational Device Exemption (IDE) and Prepares for First Ever Clinical Trial of FLASH Therapy. Available at: https://www.varian.com/about-varian/newsroom/press-releases/varian-receives-investigational-device-exemption-ide-and
Keywords: FLASH radiotherapy, history, mechanisms, future, conventional dose-rate radiotherapy
Citation: Lin B, Gao F, Yang Y, Wu D, Zhang Y, Feng G, Dai T and Du X (2021) FLASH Radiotherapy: History and Future. Front. Oncol. 11:644400. doi: 10.3389/fonc.2021.644400
Received: 21 December 2020; Accepted: 04 May 2021;
Published: 25 May 2021.
Edited by:
William Small, Jr., Loyola University Chicago, United StatesReviewed by:
Khaled Elsayad, University of Münster, GermanyCopyright © 2021 Lin, Gao, Yang, Wu, Zhang, Feng, Dai and Du. This is an open-access article distributed under the terms of the Creative Commons Attribution License (CC BY). The use, distribution or reproduction in other forums is permitted, provided the original author(s) and the copyright owner(s) are credited and that the original publication in this journal is cited, in accordance with accepted academic practice. No use, distribution or reproduction is permitted which does not comply with these terms.
*Correspondence: Xiaobo Du, ZHV4aWFvYm8yMDA1QDEyNi5jb20=
†These authors share first authorship
Disclaimer: All claims expressed in this article are solely those of the authors and do not necessarily represent those of their affiliated organizations, or those of the publisher, the editors and the reviewers. Any product that may be evaluated in this article or claim that may be made by its manufacturer is not guaranteed or endorsed by the publisher.
Research integrity at Frontiers
Learn more about the work of our research integrity team to safeguard the quality of each article we publish.