- 1Division of Radiotherapy and Imaging, The Institute of Cancer Research, London, United Kingdom
- 2Department of Radiotherapy, The Royal Marsden NHS Foundation Trust, London, United Kingdom
- 3Department of Radiation Oncology, Radboud University Medical Center, Nijmegen, Netherlands
- 4Department of Oncology, Odense University Hospital, Odense, Denmark
- 5Laboratory of Radiation Physics, Odense University Hospital, Odense, Denmark
- 6Department of Radiation Oncology, Washington University School of Medicine in St. Louis, St. Louis, MO, United States
Radiotherapy has an important role in the curative and palliative treatment settings for bladder cancer. As a target for radiotherapy the bladder presents a number of technical challenges. These include poor tumor visualization and the variability in bladder size and position both between and during treatment delivery. Evidence favors the use of magnetic resonance imaging (MRI) as an important means of tumor visualization and local staging. The availability of hybrid systems incorporating both MRI scanning capabilities with the linear accelerator (MR-Linac) offers opportunity for in-room and real-time MRI scanning with ability of plan adaption at each fraction while the patient is on the treatment couch. This has a number of potential advantages for bladder cancer patients. In this article, we examine the technical challenges of bladder radiotherapy and explore how magnetic resonance (MR) guided radiotherapy (MRgRT) could be leveraged with the aim of improving bladder cancer patient outcomes. However, before routine clinical implementation robust evidence base to establish whether MRgRT translates into improved patient outcomes should be ascertained.
Introduction
Bladder cancer is the ninth most common cancer diagnosis globally with over 390,000 new cases and over 150,000 deaths occurring each year (1). Muscle invasive bladder cancer (MIBC) makes up approximately 20% of patients at presentation. For these patients, cure is achieved through both effective local treatment and systemic treatment (2, 3).
Radical cystectomy has been the internationally accepted main stay of local treatment for MIBC (4). This requires removal of the bladder, which then necessitates a urinary diversion. Most commonly, this is in the form of an incontinent stoma (ileal conduit). Continent stomas and orthoptic neo-bladder reconstructions are feasible options for some patients. Despite this, continence and sexual function impact significantly on quality of life post-operatively (5–8). A highly selected proportion of patients may be suitable for partial cystectomy by virtue of having a unifocal tumor in a region of the bladder which then permits an adequately safe margin to be obtained without compromise to the bladder capacity. As less than 5% of patients meet these stringent criteria, removal of the whole bladder will be necessary for almost all patients. The clear absence of comparable functional organ substitutes following surgery means that bladder preservation with radiotherapy offers opportunity for cancer cure with organ preservation (3, 9, 10).
Concerns about oncologic equivalence and absence of randomised control data have driven underutilization of radical radiotherapy for the treatment of MIBC (11–13). However, when radiotherapy is used as part of a multi-modality strategy, it achieves similar survival outcomes to radical cystectomy (14, 15). The 5-year cancer-specific survival ranges from 50% to 82% (depending on initial stage), with 5-year overall survival of approximately 50%. Long-term bladder preservation is successfully achieved in up to two-thirds of patients (9). As a result, it would be accepted that patients should be offered opportunity to consider both modalities when either radical treatment would be suitable (3, 10, 16).
The aetiological association of bladder cancer with smoking means patients often have multiple comorbidities on a background of increasing frailty with advancing age that may restrict opportunity for either radical treatment options (17). For these patients, hypofractionated radiotherapy offers prospect for long-term disease and symptom control (18, 19).
In both the radical and palliative bladder radiotherapy settings, there remains opportunity to improve clinical outcomes further by overcoming some of the challenges that bladder radiotherapy poses. In this article, we examine the technical challenges of bladder radiotherapy and explore how magnetic resonance (MR) guided radiotherapy (MRgRT) could provide a solution for geometric and biologically adapted treatment delivery.
Current Role of MR Imaging in Bladder Cancer
The tumor staging of bladder cancer is contingent on accurately determining the presence of muscle invasion. Given the different treatment approaches for NMIBC and MIBC, establishing the correct tumor stage is critical in deciding the correct treatment strategy (3, 20). Although CT provides high spatial resolution allowing visualization of extra-vesical spread, it is not a reliable means of determining the extent of muscle involvement (21). It is limited both by inter-observer variability and inability to distinguish the muscle layers of the bladder (22, 23). As a result, the current standard means of diagnosing and staging MIBC remains performing a TURBT with the aim of ensuring bladder muscle is included in the specimen so that its involvement can be ascertained (3, 20, 24). However, TURBT remains imperfect as it risks under staging in 25%–50% of patients (25–27).
Magnetic resonance imaging (MRI) staging accuracy exceeds those reported for TURBT in terms of distinguishing between MIBC (≥T2) and NMIBC (≤T1) (28, 29). Three meta-analyses have evaluated the performance characteristics of multi-parametric MRI (mpMRI) for local tumor staging across approximately 5,000 patients. These studies reported similar results, with pooled sensitivity of 0.87 (95% Confidence interval, CI 0.82–0.91), 0.90 (95% CI 0.83–0.94), and 0.92 (95% CI 0.88–0.95), and specificity of 0.79 (95% CI 0.72–0.85), 0.87 (95% CI 0.78–0.93), and 0.88 (95% CI 0.77–0.94) (28–30).
A mpMRI examination for bladder cancer staging usually consists of a T2-weighted image (T2W) with diffusion-weighted image (DWI), or dynamic contrast enhancement (DCE) image (28–31). There is suggestion however that mpMRI using DWI is the optimal protocol for tumor staging of bladder cancer (29, 30). Figure 1 illustrates example image of a localized MIBC as evaluated on 1.5T MRI.
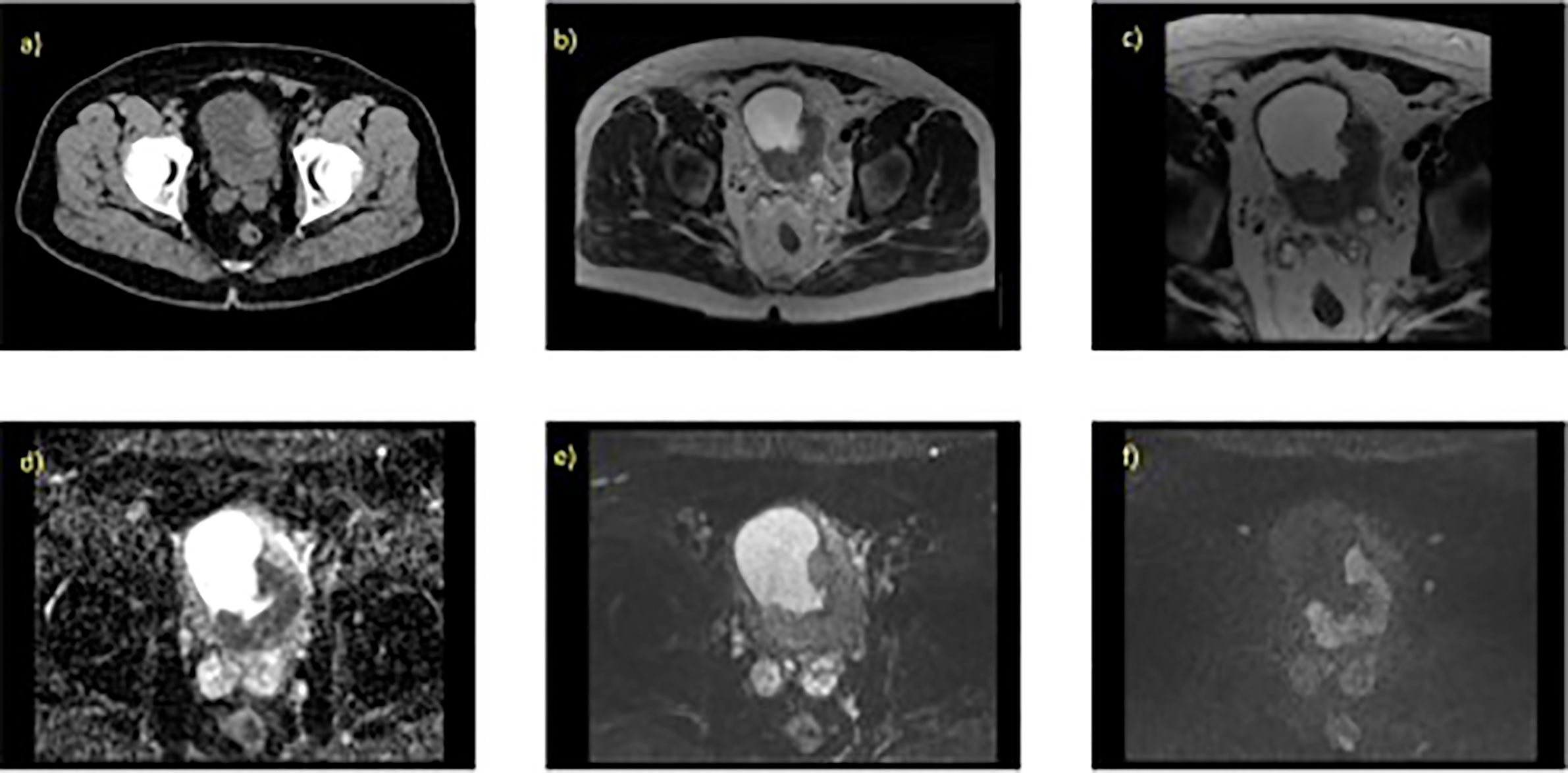
Figure 1 Localized MIBC as evaluated on T2W and DWI with the associated parameter settings for 1.5T MRI. 70 year old male with known T3 N0 M0 bladder cancer, tumour is present at the left ureteric orifice (extending posteriorlaterally) (A) contrast enhanced CT scan, axial slice through pelvis, (B) axial T2W (large field of view) showing hypo intense lesion, (C) axial T2W small field of view (D) corresponding ADC map, (E) axial DWI at b-value 0, (F) axial DWI at b-value 750.
In order to standardize the image acquisition, interpretation, and reporting of mpMRI for newly diagnosed bladder cancer, the Vesical Imaging-Reporting and Data System (VI-RADS) was developed in 2018 (31). This is a five-point qualitative scoring system of bladder tumors as seen on T2W, DWI, and DCE imaging, to determine the likelihood of muscle invasion. The final score is based on T2W imaging because of its high spatial resolution to evaluate the integrity of the muscle layer. Definitive muscular invasion is decided by the assessment of DWI and DCE. However, as DWI improves the accuracy of distinguishing MIBC, it is relied upon particularly when there is discordant scoring between T2W and DCE sequences (29, 31–33).
Multi-institutional studies applying VI-RADs scoring (1-5) to mpMRI interpretation to determine local staging demonstrates high sensitivity and specificity when a cut off score of ≥3 is used to describe likelihood muscle invasion (34–38). VI-RADS scoring also reflects good to excellent interobserver reporting agreement, with indices of agreement ranging between 0.73 and 0.92 (34–37). Despite this evidence, mpMRI has not yet established its place as recommended and preferred standard imaging for local bladder cancer staging in clinical guidance (3).
In prostate cancer mpMRI has been shown to identify those men who could safely avoid unnecessary biopsy with the aim of enabling detection of clinically significant disease (39). In bladder cancer, it is also hypothesized that mpMRI may also serve as a triage test prior to TURBT (40). The advantage this presents for MIBC patients is that it would potentially reduce delays to definitive treatment, avoids under staging on initial TURBT, and minimizes the risk of systemic circulating cancer cell dissemination occurring as a result of bladder perforation with TURBT (25, 26, 41, 42). The possibility that the TURBT may be completely avoided when suspicion of MIBC is high on mpMRI is being explored in a randomized phase 2/3 trial (BladderPath, ISRCTN reference number 35296862) (43). This trial aims to compare the standard diagnostic pathway consisting of flexible cystoscopy and biopsy, with imaging followed by TURBT versus a risk stratified imaging directed pathway whereby if on flexible cystoscopy there is clinical suspicion of possible MIBC, a biopsy is taken and patients proceed to mpMRI. If the mpMRI supports likelihood of NMIBC, patients then proceed to TURBT otherwise if the mpMRI supports likelihood of MIBC patients proceed to directly to treatment. Initial feasibility to randomize possible MIBC patients to a TURBT directed diagnostic pathway or mpMRI directed diagnostic pathway has been successfully demonstrated. The trial is ongoing to investigate how a mpMRI-driven diagnostic pathway impacts on time to correct therapy for MIBC and NMIBC and clinical progression-free survival (43).
Rationale for MR-Guided Online Adaptive Bladder Radiotherapy
MRI Improves Target Visualization
The uncertainties of using CT for bladder tumor staging also impact on the ability to reliably define the outer bladder wall and gross tumor volume (GTV) within the bladder. As a result, use of CT leads to significant inter-observer target delineation variability particularly at interfaces with neighboring structures such as small bowel or prostate, and in the presence of extra-vesical spread (44–47). Poor target delineation is a major source of systematic inaccuracies in radiotherapy (45). The improved soft tissue contrast of MRI may help address this.
The GTV visibility in bladder cancer however can be hampered after TURBT and good response to neo-adjuvant chemotherapy (46). Insertion of radio-opaque markers at cystoscopy to demarcate the visible tumor extension has been explored (48). Surgical clips or gold fiducial markers can be inserted at the borders of visible tumor or tumor bed via cystoscope (49–51). Although they provide excellent visualization on CT, these markers are prone to migration and fall out in up to 50% of cases following implantation (49, 52). Diathermy post insertion or gold seeds with micro-tines further improve retention rates but net marker losses (up to 18%) are still seen (50, 51). Metallic fiducial markers do not yield a signal on MRI and appear dark. By using T2*weighted sequences, the signal loss can be emphasized such that their position can be identified allowing them to be used to guide localization on MRI (53).
Iodized oil contrast (Lipiodol®), 0.25–0.50cc injected sub-epithelially into the bladder wall has also been used as an alternative fiducial marker (54–57). Its use is limited to patients with no history of contrast medium sensitivity or active thyroid disease (54, 58). It is not subject to the same frequency of marker loss, but the liquid nature of the contrast medium means intra- and extra-vesical spillage can occur (54–56). In circumstances of high concentration, this can lead to streak artefacts on CT (59, 60). Lipiodol is not visible on MRI.
Novel radiographic gel-like markers (BioXmark®) that are liquid, with low initial viscosity prior to and during injection but transforms into a highly viscous liquid to form a 3D gel-like shape have also been investigated (61). It produces signals void on MRI in phantom studies (62). Further work is in progress to assess this marker when used clinically for bladder MRI evaluation.
Adaptive Radiotherapy to Address Target Motion
The bladder is relatively mobile target subject to filling variation and deformation. It is fixed at the caudal pole and is abutted by the rectum or uterus posteriorly. Therefore, as the bladder volume increases non-uniform organ expansion generally occurs which is more pronounced in the cranial and anterior directions (47, 63–66). The magnitude of this change is rarely consistent or predictable (67, 68). Patient interventions such as drinking protocols, catheterization, dietary modifications, and laxatives have been explored but do not consistently reduce bladder target variation (60, 69, 70).
Inter-Fractional Motion Mitigation
In an attempt to compensate for both the variability of the bladder shape, and size between treatments (inter-fraction), historically large population-based margins (up to 1.5–2cm) have been applied to create the planning target volume (PTV). Despite the use of such large margins to address the bladder target positional uncertainties, without the adoption of soft tissue image guidance, geographical misses will occur at treatment delivery (71).
Pre-treatment, in-room three-dimensional volumetric soft tissue imaging provides anatomical information that can feedback into the plan and adapt dose delivery optimization (72). The overall aim of these adaptive radiotherapy strategies is to further improve the fidelity of dose delivered to target in order to reliably reduce the PTV so dose to normal tissues can also be reduced. In bladder cancer radiotherapy, two main adaptive approaches based on the wide availably of cone bean CT (CBCT) have seen drift into clinical practice based on reported dosimetric gains (68, 73).
The composite volume method is an offline adaptive radiotherapy approach that utilizes information from the verification CBCT acquired for the first 3–5 fractions to determine a patient specific internal target volume (ITV) informed by the maximal excursions of the bladder actually occurring. A smaller margin to account for remaining residual uncertainties is then applied to create a new PTV and plan. This solution adequately maintains bladder target coverage and reduces the PTV by approximately 40%–50% compared to population based PTV approach (74, 75). The main disadvantage is that patients can only benefit from the adaptive radiotherapy strategy after sufficient number of verification CBCTS images has been acquired. This presents limitations in its application to hypofractionated regimes because a significant proportion of treatment course would already have been delivered before a new plan can be created.
The alternative and more widely adopted method currently employed is to generate a library of patient specific treatment plans with varying PTV sizes (73). Using the CBCT acquired prior to each fraction, the anatomy is assessed to select the most appropriate plan that covers the bladder target with minimal normal tissue exposure. The library of plans can be created by applying either variable margins or by modeling the patient’s own bladder filling pattern using either serial planning CT scans or the verification CBCTs from the initial fractions (76–78). This solution also successfully maintains target coverage, and reduces the PTV by approximately 40% with subsequent reduction in normal tissue irradiation (79). The main disadvantage is that a discrete library created to cover the spectrum of interfraction variation means the individual conformity of the selected plan to the imaged bladder on the day can be relatively poor (80). It is also possible in some circumstances that none of the plans in the library encompass the imaged bladder target on the day (78, 80).
Modeled approaches in bladder cancer radiotherapy illustrate that by adopting an online replanning adaptive radiotherapy process, whereby the patient’s treatment plan is produced based on the actual anatomy seen while they are on the treatment couch would further improve target coverage and OAR sparing (81, 82). In work comparing standard single plan, with different adaptive strategies the volume of normal tissue receiving more than 95% of the prescribed dose was reduced to 66% (range 48%–100%) with library approach and to 41% (range 33%–50%) with daily re-optimization (81). Considerable normal tissue sparing potential therefore exists for bladder cancer patients with online re-optimization.
The availability of hybrid systems that incorporate both MRI scanning capabilities and linear accelerator (MR-Linac) allows an in-room, real-time MRI scan to be obtained immediately prior each fraction (83–85). As MRI yields superior soft-tissue contrast compared to CBCT it would be preferred means for accurate bladder target delineation and organs at risk (OARs), i.e., rectum and bowel identification to inform re-optimization at each fraction (86). Feasibility of these platforms to deliver an MR-informed fully online re-optimized new bladder plan at each fraction has been demonstrated (87, 88).
Intra-Fractional Motion Mitigation
Stochastic variation in the organ filling, deformation, and peristaltic motion means that changes will occur in the bladder target and OARs within the time scale of pre-treatment imaging and delivery of each individual treatment fraction. This necessitates additional consideration to determine the best means of accommodating for this motion in order to minimize risk of geographical miss.
The most common strategy in bladder cancer radiotherapy is to treat on an empty bladder and passively manage intra-fractional change by the application of a margin that will encompass the magnitude of motion likely to occur within the time frame of the workflow. For treatment delivery based on the CBCT adaptive solutions described above, intra-fraction margins ranging from 2 mm to 7 mm have been suggested (76, 77, 79, 81, 89, 90). This margin may also be influenced by treatment technique, as intensity modulated arc therapy (IMAT) is associated with faster delivery times than fixed field IMRT so facilitating use of smaller intra-fraction margins (91).
In a patient population who had serial MRI scans acquired at 2 minute intervals for up to 10-min post voiding, it was possible to demonstrate that the application of anisotropic margins (14 mm cranially and anteriorly, 9 mm posteriorly, and 5 mm in all other directions) successfully maintained target coverage as evaluated on the 10-min MRI scan for the entire treatment course (82). Target under dosing (≥D1cc <95% of the prescribed dose) was seen in 4% of fractions compared to 20% when a 5 mm isotropic margin was used (82).
Currently, treatment workflow times for utilization of an MRgRT online reoptimization approach are in the region of approximately 30–40 min (87, 92, 93). It has been successfully shown that an anisotropic margin of 15 mm applied cranially and anteriorly, 1 cm posteriorly, and 5 mm in all other directions will successfully maintain target coverage in 96.6% of fractions as assessed on the post treatment MRI scan (87). The mean conformity of the 95% isodose to the post treatment bladder target is 2.4 (range 1.5–3.6), suggesting the intra-fraction margin could be reliably reduced in some instances (87). While maintenance of target coverage throughout the fraction delivery is a priority, the potential gains of online re-optimization would be mitigated by the use of over-generous intra-fraction margins.
The alternative approach is to actively manage intra-fraction change with MR guided motion management. During beam on period, continuous MR imaging can be acquired for real-time motion monitoring, tracking, and or gating. A tracking slice is positioned to include a cross-sectional axis at the target volume of interest. A minimum tracking boundary or motion monitoring structure is set such that if a pre-specified proportion of the tracked target leaves this boundary, the beam will turn off (10). This allows extremes of anatomical changes to be detected while the target is being irradiated to minimize the risk of a geographical miss (10, 94).
MR guided tumor tracking has been successfully used on the MR-Linac for treatment of tumors of the upper abdomen and prostate (95–97). However, the challenge this presents for bladder cancer radiotherapy is that tracking alone is not necessarily a universal solution if the target is increasing in overall volume as occurs with whole bladder radiotherapy (65, 66). It raises the question then, could the tumor itself be tracked and could this region be safely prioritized over the uninvolved bladder.
Enabling Tumor-Focused Partial Bladder Irradiation
Tumor-focused partial bladder radiotherapy is attractive for two main clinical reasons: firstly, the reduced high dose opens the possibility that treatment-related toxicity could be reduced; and secondly, it opens the possibility for dose escalation to the tumor beyond limits currently determined by the whole bladder tolerance of 64-65Gy in 2Gy per fraction (98–100).
Whole bladder radiotherapy has been the accepted convention even in the presence of unifocal disease possibly because of the difficulty in identifying the tumor within the bladder on CT and the historical inaccuracies of treatment delivery described above. Nevertheless, evidence to date supports that partial bladder irradiation is likely to be safe (3, 101–103).
Bladder brachytherapy has been used for a highly select patient population with unifocal small lesions (≤50 mm) achieving similar outcomes to a matched population undergoing radical cystectomy (104). It is not widely accepted or recommended as an organ-conserving treatment option mainly because technical expertise is confined to highly specialized centers and no randomized control data is available (3, 101).
Randomized control trials of whole bladder versus tumor-focused partial bladder external beam radiotherapy have successfully demonstrated that tumor-focused partial bladder radiotherapy could be utilized with no adverse effect on local control (103, 105). However, these randomized controlled trials failed to show decrease treatment related toxicity (103, 105). A number of technical aspects are likely to have mitigated any benefit from a reduced high dose volume. Treatment was planned and delivered on an empty bladder. In addition, delineation of the tumor within the bladder using a planning CT scan would have invariably led to overestimating the GTV size (44–46). The subsequent isotropic 1.5 cm expansion margin around the GTV to generate the PTV boost volume from which a 3D conformal treatment plan was created would then leave very little additional normal tissue sparing compared to whole bladder treatment. Setup in the era of these trials was either to skin or bone and preceded soft tissue verification, so it can be assumed that with 1.5 cm margin target coverage may have only been approximately 60% (71). Dose unsuccessfully delivered to target would have resulted in unwanted normal tissue irradiation.
Many investigators have sought to overcome these challenges by using library of plans to deliver tumor-focused high dose radiotherapy on filled or partially filled bladder (80, 106, 107). The advantage of striving for a fuller bladder in these circumstances is that it reduces dose to the uninvolved bladder and provides greater opportunity for normal bladder sparing. Treatment delivered in these trials used either fixed field IMRT or IMAT. This improves conformity of radiation fields around the target volume, relative to 3D conformal techniques (91). In comparisons of clinical outcomes of bladder cancer radiotherapy, IMRT has been reported to significantly reduce acute CTCAE grade ≥2 diarrhoea compared to 3D conformal radiotherapy (56% versus 30%; p = 0.008) (108). Whether using library of plans to escalate tumor-focused dose translates into clinically meaningful outcomes will be evaluated in an international randomised phase II trial (RAIDER, NCT02447549) (109).
Dosimetric analysis of library of plans to deliver tumor-focused high dose radiotherapy reveals that although excellent target coverage can be achieved meeting normal bladder and bowel constraints, the high mean conformity of the 95% isodose of the selected plan to the tumor boost as seen on CBCT is 5.0 (SD 2.2, range 2.1–21.4) and the whole bladder is 3.5 (SD 1.0, range 1.7–8.9). This suggests large volume non-target irradiation is still occurring (80). The MR-Linac may therefore open opportunity for an online re-optimized tumor-focused partial bladder approach.
Successful tumor-focused partial bladder irradiation is dependent on ability to define GTV on both the planning CT and CBCT. Although CBCT allows reasonable discrimination of the bladder wall, visualization of the tumor itself is challenging (80, 110). As local recurrences occur most frequently at the original MIBC tumor site, correctly identifying the GTV becomes increasing critical particularly in the era of margin reduction (111). The superior soft tissue contrast of MRI may therefore enable more reliable tumor-focused partial bladder radiotherapy (Figure 2).
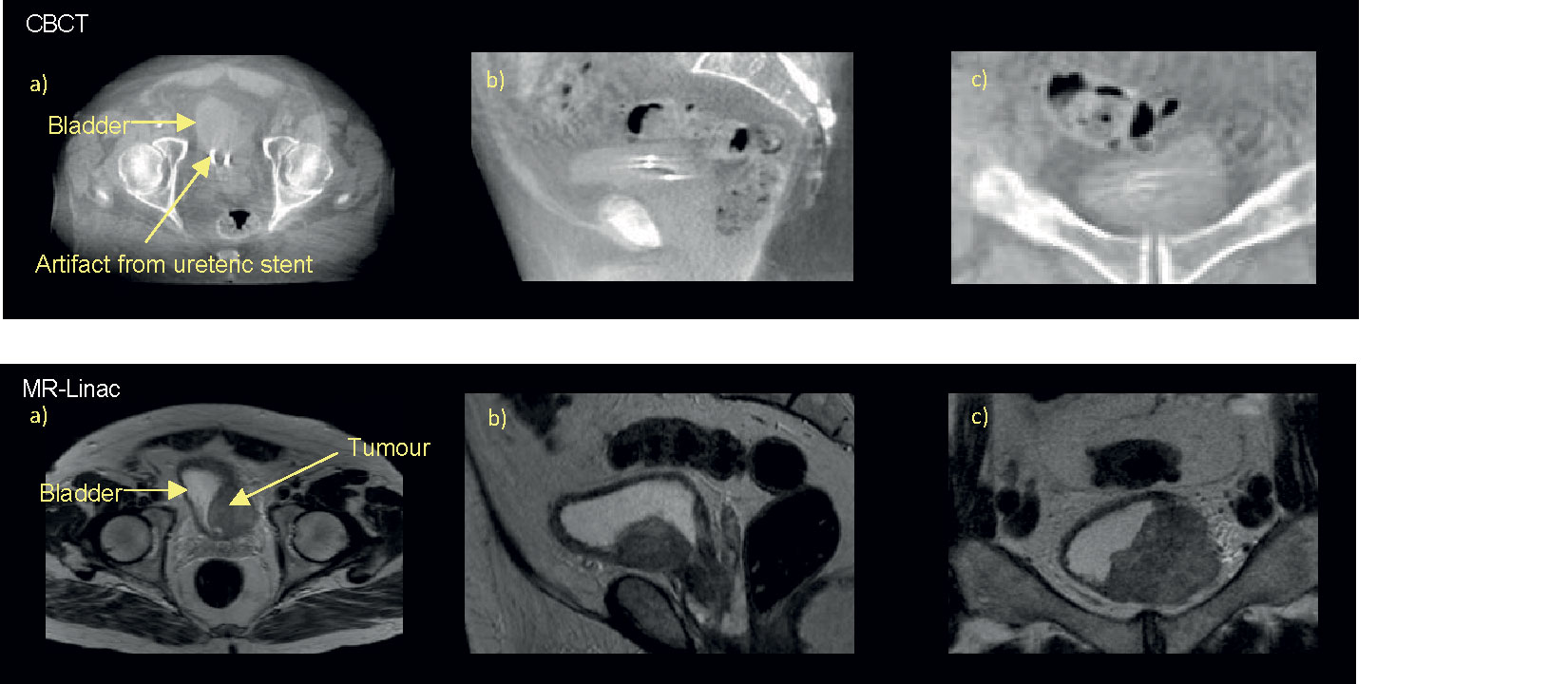
Figure 2 Online pre-treatment CBCT and MR (T2W) images. Bladder tumour at left bladder wall as seen on axial a), sagittal b), and coronal c) views of the pelvis on corresponding CBCT and T2W taken on the MR-Linac, here urine appears bright and tumour dark/hypointense.
The MR-Linac may also provide greater opportunity to assess how the tumor moves in relation to the filling status of the bladder to determine the most appropriate intra-fraction margins for partial bladder radiotherapy. Work to date suggests that the bladder tumor is relatively rigid and non-elastic compared to non-tumor-bearing bladder regions but this is based on CT interpretation (112).
Workflow Considerations for Bladder Treatment on the MR-Linac
An overview of the principal workflow components is presented in Figure 3. For treatment of the whole bladder on the MR-Linac, workflow time pressures are critical because of the anticipated intra-fraction volume increase. If workflow time could be reduced, the margins currently applied to accommodate for this change could also be reduced. Several considerations can assist with achieving this.
Ideally as little time as possible should be spent re-optimizing the daily treatment plan. This can be aided by generating a robust planning class solution from the outset to minimize the need for online modification and experimentation. This should be robust to the expected daily changes in anatomy that will occur.
Prior to starting treatment, a reference plan is created. A planning CT (CTplanning) and, or a simulation MR (MRplanning) scan is acquired with an empty bladder. This is achieved by asking the patient to void immediately prior to scanning. The CTplanning is used for density information and it is deformably registered to the MRplanning. It is also possible that at simulation serial images over time are acquired to estimate a “patient specific” intra-fraction bladder filling PTV margin.
When patients attend for treatment, they are asked to void their bladder immediately prior to set up. A session or pre-treatment MRI (MRsession) image is acquired on the MR-Linac which is registered to the planning reference image (CTplanning or MRplanning). The contours from the planning reference image are propagated to the MRsession image using deformable registration or segmented using artificial intelligence contouring algorithms (113). The contours are reviewed and corrected if necessary. To speed up the outlining time, more accurate delineation of OARs is limited to a 2 cm region around the target. The consequence of having less accurate contours is that, although the dose distribution will still be close to optimal, the reported dose statistics for these OARs will be less reflective of actual dose to these structures. This trade-off is made to balance the desire for accurate delineation and the fact that the OARs underlying those contours are continuously changing whilst they are being delineated.
A new plan with full re-optimization is created. For online bladder planning dose-volume metrics do not have to be used, instead focus can be placed on how rapidly the dose falls off away from the target. Here, the optimizer only considers the dose gradient in the region where the OAR abuts the target, and as such is not dependent on the overall OAR volume. This approach is also less sensitive to accurate delineation of the OARs, as only the approximate region where they border the target is needed.
During the optimization process, a fast T2W MRI (MRverfication) is acquired to confirm that appropriate target coverage is maintained either by reviewing the PTV coverage of the bladder or the isodose coverage of the bladder. If the bladder is not optimally covered then the plan can be shifted relative to the isocenter and dose recalculated on the MRsession (114, 115). If this maneuver would also not sufficiently cover the bladder target then it would be recommended that the patient is removed from the couch, voids their bladder, and are treated with the reference plan. Prior to the subsequent fraction patient factors contributing to rapid bladder filling, i.e., pre-treatment diuretic or excessive hydration should be explored and managed. It may also be necessary to review and increase the intra-fraction margin.
At treatment delivery, cine MR can be used to monitor bladder motion during beam on with the option that should the bladder move out of the PTV or the pre-defined motion monitoring structure, the treatment can be interrupted if required. A post treatment T2W MRI (MRpost) is acquired immediately following delivery for offline dose assessment of the treatment delivered. The difference between planned dose on MRsession and delivered dose as determined on MRpost could potentially be incorporated into the online adaption strategy and compensated for at the subsequent fractions, if clinically indicated.
Currently the time to deliver this workflow at best is between 15 and 27 min (personal communication, A Bertelsen & C Nyborg, Odense University Hospital, Denmark) but we have found the median total time for patients on the treatment couch is 39 min (range 33–48) (87). We expect that this will be reduced further with faster image acquisition, improvements in auto-contouring, increased computational ability for plan optimization and dose calculation, and the implementation of IMAT delivery techniques.
Beyond Geometric Adaption
MRI could be used to acquire biological information about the bladder tumor. This could provide opportunity to develop MRI informed biologically adapted radiotherapy approaches (116).
DWI is a functional imaging technique dependent on the inhibitory effect of cell membranes to the random motion of water molecules. The higher cellular density of tumors compared to normal tissue means they demonstrate higher signal intensity, i.e., restricted diffusion on MRI, reflected quantitatively in a low mean apparent diffusion coefficient value (ADC). Per pixel ADC throughout the tumor volume can be used to capture the regional heterogeneity known to exist within tumors which may have prognostic and predictive value (117–121). As the local relapse site following radiotherapy is at the site of the MIBC tumor, it is hypothesized that by escalating dose to the tumor region of highest cellularity, local control rates could be improved (111).
Following successful treatment, the ADC value increases, reflecting decrease in cellularity. In MIBC ADC change is an independent predictor of pathological response (122, 123). Given serial DWI acquisition on the MR-Linac is possible at each fraction, there is potential for monitoring ADC change throughout treatment with identification of early non-responders who may benefit from change in treatment approach (124). As such MRI offers opportunity for a response adapted radiotherapy delivery.
Tumor hypoxia in MIBC is a potential predictor of radiotherapy response with effective modification improving outcome (125, 126). MRI can be used to measure and map tumor hypoxia in a number of ways not otherwise possible on biopsy or serum surrogates (127, 128). Intrinsic susceptibility weighted or blood oxygenation level dependent MRI (BOLD), exploits the difference in magnetic susceptibility of oxyhaemoglobin and deoxyhaemoglobin to generate contrast and identify regions of hypoxia (129).
Visualization of tumor blood flow can be used as a surrogate to identify areas of hypoxia. DCE enables in vivo assessment of tumor blood flow and permeability using paramagnetic contrast agents. DCE has been shown to have ability to predict treatment response in MIBC following chemotherapy (130). Experimental models demonstrate the potential effectiveness of hypoxia informed boost dose delivery to increase tumor control (126). Future partial bladder radiotherapy approaches could therefore inform a mpMRI derived biological target volume. Given this volume is up to 45% smaller than an anatomically defined bladder GTV, it opens the possibility of further normal tissue sparing (131). As the volume of radiation influences the immunogenic potential of the tumor microenvironment, defining alternative meaningful target sub-volumes particularly with systemic immunotherapy warrants further evaluation (132, 133).
Conclusion
MRgRT heralds a paradigm shift for bladder cancer patients with potential gains to be had at the simulation, treatment delivery, and response assessment stages. Whether the closer integration of MRI into the bladder patient radiotherapy pathway translates into clinical gains for our patient population is still yet to be determined. A framework for clinical evaluation of MR-Linac technologies has been suggested (134, 135). We would strongly advocate participation in clinical trials to generate robust evidence base to prove our expectations (and hopes) of further improving bladder cancer patient outcomes with MRgRT.
Author Contributions
All authors meet at least of one the criteria recommended by the ICMJE. SH wrote the first manuscript draft. AH, BT, AB, IH, HM, CN, RS, and GS were all sub-section contributors. All authors contributed to the article and approved the submitted version.
Conflict of Interest
BCB reports personal fees from Mevion, personal fees from Mevion/Sanofi, outside the submitted work. SH reports non-financial support from Elekta (Elekta AB, Stockholm, Sweden), non-financial support from Merck Sharp & Dohme (MSD), personal fees and non-financial support from Roche outside the submitted work.
The remaining authors declare that the research was conducted in the absence of any commercial or financial relationships that could be construed as a potential conflict of interest.
Acknowledgments
AH, BT, IH, GS, and SH acknowledge this study represents independent research supported by the National Institute for Health Research (NIHR) Biomedical Research Centre at The Royal Marsden NHS Foundation Trust and The Institute of Cancer Research, London.
References
1. Jemal A, Bray F, Center MM, Ferlay J, Ward E, Forman D. Global cancer statistics. CA: Cancer J Clin (2011) 61(2):69–90. doi: 10.3322/caac.20107
2. Advanced Bladder Cancer Meta-analysis C. Neoadjuvant chemotherapy in invasive bladder cancer: update of a systematic review and meta-analysis of individual patient data advanced bladder cancer (ABC) meta-analysis collaboration. Eur Urol (2005) 48(2):202–205; discussion 205-206. doi: 10.1016/j.eururo.2005.04.006
3. Witjes JA, Bruins HM, Cathomas R, Comperat EM, Cowan NC, Gakis G, et al. European Association of Urology Guidelines on Muscle-invasive and Metastatic Bladder Cancer: Summary of the 2020 Guidelines. Eur Urol (2020) 79(1):82–104. doi: 10.1016/j.eururo.2020.03.055
4. Gakis G, Efstathiou J, Lerner SP, Cookson MS, Keegan KA, Guru KA, et al. ICUD-EAU International Consultation on Bladder Cancer 2012: Radical cystectomy and bladder preservation for muscle-invasive urothelial carcinoma of the bladder. Eur Urol (2013) 63(1):45–57. doi: 10.1016/j.eururo.2012.08.009
5. Pruthi RS, Nix J, McRackan D, Hickerson A, Nielsen ME, Raynor M, et al. Robotic-assisted laparoscopic intracorporeal urinary diversion. Eur Urol (2010) 57(6):1013–21. doi: 10.1016/j.eururo.2009.12.028
6. Kessler TM, Burkhard FC, Studer UE. Clinical indications and outcomes with nerve-sparing cystectomy in patients with bladder cancer. Urol Clin North Am (2005) 32(2):165–75. doi: 10.1016/j.ucl.2005.02.005
7. Hautmann RE, de Petriconi R, Gottfried HW, Kleinschmidt K, Mattes R, Paiss T. The ileal neobladder: complications and functional results in 363 patients after 11 years of followup. J Urol (1999) 161(2):422–427; discussion 427-428. doi: 10.1016/S0022-5347(01)61909-8
8. Caffo O, Fellin G, Graffer U, Luciani L. Assessment of quality of life after cystectomy or conservative therapy for patients with infiltrating bladder carcinoma. A survey by a self-administered questionnaire. Cancer (1996) 78(5):1089–97. doi: 10.1002/(SICI)1097-0142(19960901)78:5<1089::AID-CNCR20>3.0.CO;2-Y
9. Ploussard G, Daneshmand S, Efstathiou JA, Herr HW, James ND, Rodel CM, et al. Critical analysis of bladder sparing with trimodal therapy in muscle-invasive bladder cancer: a systematic review. Eur Urol (2014) 66(1):120–37. doi: 10.1016/j.eururo.2014.02.038
10. Dinh TT, Mitin T, Bagshaw HP, Hoffman KE, Hwang C, Karnes RJ, et al. Executive Summary of the American Radium Society Appropriate Use Criteria for Radiation Treatment of Node-Negative Muscle Invasive Bladder Cancer. Int J Radiat Oncol Biol Phys (2020) 27:S0360-3016(20)34448-5. doi: 10.1016/j.ijrobp.2020.10.031
11. Gray PJ, Fedewa SA, Shipley WU, Efstathiou JA, Lin CC, Zietman AL, et al. Use of potentially curative therapies for muscle-invasive bladder cancer in the United States: results from the National Cancer Data Base. Eur Urol (2013) 63(5):823–9. doi: 10.1016/j.eururo.2012.11.015
12. Huddart RA, Hall E, Lewis R, Birtle A, Group STM. Life and death of spare (selective bladder preservation against radical excision): reflections on why the spare trial closed. BJU Int (2010) 106(6):753–5. doi: 10.1111/j.1464-410X.2010.09537.x
13. Hafeez S, Lewis R, Griffin C, Hall E, Huddart R. Failing to Close the Gap Between Evidence and Clinical Practice in Radical Bladder Cancer Radiotherapy. (R Coll Radiol) (2021) 33(1):46–9. doi: 10.1016/j.clon.2020.07.001
14. Arcangeli G, Arcangeli S, Strigari L. A systematic review and meta-analysis of clinical trials of bladder-sparing trimodality treatment for muscle-invasive bladder cancer (MIBC). Crit Rev Oncol Hematol (2015) 94(1):105–15. doi: 10.1016/j.critrevonc.2014.11.007
15. Kulkarni GS, Hermanns T, Wei Y, Bhindi B, Satkunasivam R, Athanasopoulos P, et al. Propensity Score Analysis of Radical Cystectomy Versus Bladder-Sparing Trimodal Therapy in the Setting of a Multidisciplinary Bladder Cancer Clinic. J Clin Oncol (2017) 35(20):2299–305. doi: 10.1200/JCO.2016.69.2327
16. National Institute for Health and Clinical Excellence (NICE) Guidance. Bladder cancer: diagnosis and management. (2015). Available at: http://wwwniceorguk/guidance/ng2/evidence/full-guideline-3744109.
17. Hautmann RE, Gschwend JE, de Petriconi RC, Kron M, Volkmer BG. Cystectomy for transitional cell carcinoma of the bladder: results of a surgery only series in the neobladder era. J Urol (2006) 176(2):486–492; discussion 491-482. doi: 10.1016/j.juro.2006.03.038
18. Duchesne GM, Bolger JJ, Griffiths GO, Trevor Roberts J, Graham JD, Hoskin PJ, et al. A randomized trial of hypofractionated schedules of palliative radiotherapy in the management of bladder carcinoma: results of medical research council trial BA09. Int J Radiat Oncol Biol Phys (2000) 47(2):379–88. doi: 10.1016/S0360-3016(00)00430-2
19. Hafeez S, McDonald F, Lalondrelle S, McNair H, Warren-Oseni K, Jones K, et al. Clinical Outcomes of Image Guided Adaptive Hypofractionated Weekly Radiation Therapy for Bladder Cancer in Patients Unsuitable for Radical Treatment. Int J Radiat Oncol Biol Phys (2017) 98(1):115–22. doi: 10.1016/j.ijrobp.2017.01.239
20. Babjuk M, Burger M, Comperat EM, Gontero P, Mostafid AH, Palou J, et al. European Association of Urology Guidelines on Non-muscle-invasive Bladder Cancer (TaT1 and Carcinoma In Situ) - 2019 Update. Eur Urol (2019) 76(5):639–57. doi: 10.1016/j.eururo.2019.08.016
21. Paik ML, Scolieri MJ, Brown SL, Spirnak JP, Resnick MI. Limitations of computerized tomography in staging invasive bladder cancer before radical cystectomy. J Urol (2000) 163(6):1693–6. doi: 10.1016/S0022-5347(05)67522-2
22. MacVicar AD. Bladder cancer staging. BJU Int (2000) 86 Suppl 1:111–22. doi: 10.1046/j.1464-410X.2000.00589.x
23. Tritschler S, Mosler C, Tilki D, Buchner A, Stief C, Graser A. Interobserver Variability Limits Exact Preoperative Staging by Computed Tomography in Bladder Cancer. Urology (2012) 79(6):1317–21. doi: 10.1016/j.urology.2012.01.040
24. Mariappan P, Zachou A, Grigor KM, Edinburgh Uro-Oncology G. Detrusor muscle in the first, apparently complete transurethral resection of bladder tumour specimen is a surrogate marker of resection quality, predicts risk of early recurrence, and is dependent on operator experience. Eur Urol (2010) 57(5):843–9. doi: 10.1016/j.eururo.2009.05.047
25. Kulkarni GS, Hakenberg OW, Gschwend JE, Thalmann G, Kassouf W, Kamat A, et al. An updated critical analysis of the treatment strategy for newly diagnosed high-grade T1 (previously T1G3) bladder cancer. Eur Urol (2010) 57(1):60–70. doi: 10.1016/j.eururo.2009.08.024
26. Klaassen Z, Kamat AM, Kassouf W, Gontero P, Villavicencio H, Bellmunt J, et al. Treatment Strategy for Newly Diagnosed T1 High-grade Bladder Urothelial Carcinoma: New Insights and Updated Recommendations. Eur Urol (2018) 74(5):597–608. doi: 10.1016/j.eururo.2018.06.024
27. Gray PJ, Lin CC, Jemal A, Shipley WU, Fedewa SA, Kibel AS, et al. Clinical-pathologic stage discrepancy in bladder cancer patients treated with radical cystectomy: results from the national cancer data base. Int J Radiat Oncol Biol Phys (2014) 88(5):1048–56. doi: 10.1016/j.ijrobp.2014.01.001
28. Gandhi N, Krishna S, Booth CM, Breau RH, Flood TA, Morgan SC, et al. Diagnostic accuracy of magnetic resonance imaging for tumour staging of bladder cancer: systematic review and meta-analysis. BJU Int (2018) 122(5):744–53. doi: 10.1111/bju.14366
29. Woo S, Suh CH, Kim SY, Cho JY, Kim SH. Diagnostic performance of MRI for prediction of muscle-invasiveness of bladder cancer: A systematic review and meta-analysis. Eur J Radiol (2017) 95:46–55. doi: 10.1016/j.ejrad.2017.07.021
30. Huang L, Kong Q, Liu Z, Wang J, Kang Z, Zhu Y. The Diagnostic Value of MR Imaging in Differentiating T Staging of Bladder Cancer: A Meta-Analysis. Radiology (2018) 286(2):502–11. doi: 10.1148/radiol.2017171028
31. Panebianco V, Narumi Y, Altun E, Bochner BH, Efstathiou JA, Hafeez S, et al. Multiparametric Magnetic Resonance Imaging for Bladder Cancer: Development of VI-RADS (Vesical Imaging-Reporting And Data System). Eur Urol (2018) 74(3):294–306. doi: 10.1016/j.eururo.2018.04.029
32. Panebianco V, Barchetti F, de Haas RJ, Pearson RA, Kennish SJ, Giannarini G, et al. Improving Staging in Bladder Cancer: The Increasing Role of Multiparametric Magnetic Resonance Imaging. Eur Urol Focus (2016) 2(2):113–21. doi: 10.1016/j.euf.2016.04.010
33. Takeuchi M, Sasaki S, Ito M, Okada S, Takahashi S, Kawai T, et al. Urinary bladder cancer: diffusion-weighted MR imaging–accuracy for diagnosing T stage and estimating histologic grade. Radiology (2009) 251(1):112–21. doi: 10.1148/radiol.2511080873
34. Ueno Y, Takeuchi M, Tamada T, Sofue K, Takahashi S, Kamishima Y, et al. Diagnostic Accuracy and Interobserver Agreement for the Vesical Imaging-Reporting and Data System for Muscle-invasive Bladder Cancer: A Multireader Validation Study. Eur Urol (2019) 76(1):54–6. doi: 10.1016/j.eururo.2019.03.012
35. Kim SH. Validation of vesical imaging reporting and data system for assessing muscle invasion in bladder tumor. Abdominal Radiol (2020) 45(2):491–8. doi: 10.1007/s00261-019-02190-1
36. Makboul M, Farghaly S, Abdelkawi IF. Multiparametric MRI in differentiation between muscle invasive and non-muscle invasive urinary bladder cancer with vesical imaging reporting and data system (VI-RADS) application. Br J Radiol (2019) 92(1104). doi: 10.1259/bjr.20190401
37. Juri H, Narumi Y, Panebianco V, Osuga K. Staging of bladder cancer with multiparametric MRI. Br J Radiol (2020) 2020:20200116. doi: 10.1259/bjr.20200116
38. Luo C, Huang B, Wu Y, Chen J, Chen L. Use of Vesical Imaging-Reporting and Data System (VI-RADS) for detecting the muscle invasion of bladder cancer: a diagnostic meta-analysis. Eur Radiol (2020) 30(8):4606–14. doi: 10.1007/s00330-020-06802-z
39. Ahmed HU, El-Shater Bosaily A, Brown LC, Gabe R, Kaplan R, Parmar MK, et al. et al: Diagnostic accuracy of multi-parametric MRI and TRUS biopsy in prostate cancer (PROMIS): a paired validating confirmatory study. Lancet (2017) 389(10071):815–22. doi: 10.1016/S0140-6736(16)32401-1
40. Panebianco V, Narumi Y, Barchetti G, Montironi R, Catto JWF. Should We Perform Multiparametric Magnetic Resonance Imaging of the Bladder Before Transurethral Resection of Bladder? Time to Reconsider the Rules. Eur Urol (2019) 76(1):57–8. doi: 10.1016/j.eururo.2019.03.046
41. Engilbertsson H, Aaltonen KE, Björnsson S, Kristmundsson T, Patschan O, Rydén L, et al. Transurethral Bladder Tumor Resection Can Cause Seeding of Cancer Cells into the Bloodstream. J Urol (2015) 193(1):53–7. doi: 10.1016/j.juro.2014.06.083
42. Fahmy NM, Mahmud S, Aprikian AG. Delay in the Surgical Treatment of Bladder Cancer and Survival: Systematic Review of the Literature. Eur Urol (2006) 50(6):1176–82. doi: 10.1016/j.eururo.2006.05.046
43. James ND, Pirrie S, Liu W, Amir R, Gallagher J, Hughes A, et al. et al: Replacing TURBT with mpMRI for staging MIBC: Pilot data from the BladderPath study. J Clin Oncol (2020) 38(6_suppl):446–6. doi: 10.1200/JCO.2020.38.6_suppl.446
44. Weiss E, Hess CF. The impact of gross tumor volume (GTV) and clinical target volume (CTV) definition on the total accuracy in radiotherapy theoretical aspects and practical experiences. Strahlenther Onkol (2003) 179(1):21–30. doi: 10.1007/s00066-003-0976-5
45. Njeh CF. Tumor delineation: The weakest link in the search for accuracy in radiotherapy. J Med Phys (2008) 33(4):136–40. doi: 10.4103/0971-6203.44472
46. Logue JP, Sharrock CL, Cowan RA, Read G, Marrs J, Mott D. Clinical variability of target volume description in conformal radiotherapy planning. Int J Radiat Oncol Biol Phys (1998) 41(4):929–31. doi: 10.1016/S0360-3016(98)00148-5
47. Meijer GJ, Rasch C, Remeijer P, Lebesque JV. Three-dimensional analysis of delineation errors, setup errors, and organ motion during radiotherapy of bladder cancer. Int J Radiat Oncol Biol Phys (2003) 55(5):1277–87. doi: 10.1016/S0360-3016(02)04162-7
48. Chan MF, Cohen GN, Deasy JO. Qualitative evaluation of fiducial markers for radiotherapy imaging. Technol Cancer Res Treat (2015) 14(3):298–304. doi: 10.1177/1533034614547447
49. Hulshof MCCM, van Andel G, Bel A, Gangel P, van de Kamer JB. Intravesical markers for delineation of target volume during external focal irradiation of bladder carcinomas. Radiother Oncol (2007) 84(1):49–51. doi: 10.1016/j.radonc.2007.05.017
50. Mangar S, Thompson A, Miles E, Huddart R, Horwich A, Khoo V. A feasibility study of using gold seeds as fiducial markers for bladder localization during radical radiotherapy. Br J Radiol (2007) 80(952):279–83. doi: 10.1259/bjr/54321311
51. Garcia MM, Gottschalk AR, Brajtbord J, Konety BR, Meng MV, Roach M,3, et al. Endoscopic gold fiducial marker placement into the bladder wall to optimize radiotherapy targeting for bladder-preserving management of muscle-invasive bladder cancer: feasibility and initial outcomes. PloS One (2014) 9(3):e89754. doi: 10.1371/journal.pone.0089754
52. Poggi MM, Gant DA, Sewchand W, Warlick WB. Marker seed migration in prostate localization. Int J Radiat Oncol Biol Phys (2003) 56(5):1248–51. doi: 10.1016/S0360-3016(03)00328-6
53. Pathmanathan AU, Schmidt MA, Brand DH, Kousi E, van As NJ, Tree AC. Improving fiducial and prostate capsule visualization for radiotherapy planning using MRI. J Appl Clin Med Phys (2019) 20(3):27–36. doi: 10.1002/acm2.12529
54. Pos F, Bex A, Dees-Ribbers HM, Betgen A, van Herk M, Remeijer P. Lipiodol injection for target volume delineation and image guidance during radiotherapy for bladder cancer. Radiother Oncol (2009) 93(2):364–7. doi: 10.1016/j.radonc.2009.09.003
55. Sondergaard J, Olsen KO, Muren LP, Elstrom UV, Grau C, Hoyer M. A study of image-guided radiotherapy of bladder cancer based on lipiodol injection in the bladder wall. Acta Oncol (2010) 49(7):1109–15. doi: 10.3109/02841861003789491
56. Baumgarten AS, Emtage JB, Wilder RB, Biagioli MC, Gupta S, Spiess PE. Intravesical lipiodol injection technique for image-guided radiation therapy for bladder cancer. Urology (2014) 83(4):946–50. doi: 10.1016/j.urology.2013.09.058
57. Chai X, van Herk M, van de Kamer JB, Remeijer P, Bex A, Betgen A, et al. Behavior of lipiodol markers during image guided radiotherapy of bladder cancer. Int J Radiat Oncol Biol Phys (2010) 77(1):309–14. doi: 10.1016/j.ijrobp.2009.08.019
58. Freilich JM, Spiess PE, Biagioli MC, Fernandez DC, Shi EJ, Hunt DC, et al. Lipiodol as a fiducial marker for image-guided radiation therapy for bladder cancer. Int Braz J Urol Off J Braz Soc Urol (2014) 40(2):190–7. doi: 10.1590/S1677-5538.IBJU.2014.02.08
59. Dees-Ribbers HM, Pos FJ, Betgen A, Bex A, Hulshof MC, Remeijer P, et al. Fusion of planning CT and cystoscopy images for bladder tumor delineation: a feasibility study. Med Phys (2013) 40(5):051713. doi: 10.1118/1.4799842
60. Dees-Ribbers HM, Betgen A, Pos FJ, Witteveen T, Remeijer P, van Herk M. Inter- and intra-fractional bladder motion during radiotherapy for bladder cancer: a comparison of full and empty bladders. Radiother Oncol (2014) 113(2):254–9. doi: 10.1016/j.radonc.2014.08.019
61. de Ridder M, Gerbrandy LC, de Reijke TM, Hinnen KA, Hulshof M. BioXmark(R) liquid fiducial markers for image-guided radiotherapy in muscle invasive bladder cancer: a safety and performance trial. Br J Radiol 2020 (1111) 93:20200241. doi: 10.1259/bjr.20200241
62. Schneider S, Jolck RI, Troost EGC, Hoffmann AL. Quantification of MRI visibility and artifacts at 3T of liquid fiducial marker in a pancreas tissue-mimicking phantom. Med Phys (2018) 45(1):37–47. doi: 10.1002/mp.12670
63. Fokdal L, Honore H, Hoyer M, Meldgaard P, Fode K, von der Maase H. Impact of changes in bladder and rectal filling volume on organ motion and dose distribution of the bladder in radiotherapy for urinary bladder cancer. Int J Radiat Oncol Biol Phys (2004) 59(2):436–44. doi: 10.1016/j.ijrobp.2003.10.039
64. Pos FJ, Koedooder K, Hulshof M, van Tienhoven G, Gonzalez DG. Influence of bladder and rectal volume on spatial variability of a bladder tumor during radical radiotherapy. Int J Radiat Oncol Biol Phys (2003) 55(3):835–41. doi: 10.1016/S0360-3016(02)04158-5
65. Mangar SA, Scurr E, Huddart RA, Sohaib SA, Horwich A, Dearnaley DP, et al. Assessing intra-fractional bladder motion using cine-MRI as initial methodology for Predictive Organ Localization (POLO) in radiotherapy for bladder cancer. Radiother Oncol (2007) 85(2):207–14. doi: 10.1016/j.radonc.2007.04.037
66. McBain CA, Khoo VS, Buckley DL, Sykes JS, Green MM, Cowan RA, et al. Assessment of bladder motion for clinical radiotherapy practice using cine-magnetic resonance imaging. Int J Radiat Oncol Biol Phys (2009) 75(3):664–71. doi: 10.1016/j.ijrobp.2008.11.040
67. Turner SL, Swindell R, Bowl N, Marrs J, Brookes B, Read G, et al. Bladder movement during radiation therapy for bladder cancer: implications for treatment planning. Int J Radiat Oncol Biol Phys (1997) 39(2):355–60. doi: 10.1016/S0360-3016(97)00070-9
68. Lalondrelle S, Huddart R. Improving radiotherapy for bladder cancer: an opportunity to integrate new technologies. Clin Oncol (R Coll Radiol) (2009) 21(5):380–4. doi: 10.1016/j.clon.2009.03.005
69. Muren LP, Redpath AT, Lord H, McLaren D. Image-guided radiotherapy of bladder cancer: bladder volume variation and its relation to margins. Radiother Oncol (2007) 84(3):307–13. doi: 10.1016/j.radonc.2007.06.014
70. Miralbell R, Nouet P, Rouzaud M, Bardina A, Hejira N, Schneider D. Radiotherapy of bladder cancer: relevance of bladder volume changes in planning boost treatment. Int J Radiat Oncol Biol Phys (1998) 41(4):741–6. doi: 10.1016/S0360-3016(98)00131-X
71. Foroudi F, Pham D, Bressel M, Wong J, Rolfo A, Roxby P, et al. Bladder cancer radiotherapy margins: a comparison of daily alignment using skin, bone or soft tissue. Clin Oncol (R Coll Radiol) (2012) 24(10):673–81. doi: 10.1016/j.clon.2012.06.012
72. Yan D, Vicini F, Wong J, Martinez A. Adaptive radiation therapy. Phys Med Biol (1997) 42(1):123–32. doi: 10.1088/0031-9155/42/1/008
73. Bertholet J, Anastasi G, Noble D, Bel A, van Leeuwen R, Roggen T, et al. Patterns of practice for adaptive and real-time radiation therapy (POP-ART RT) part II: offline and online plan adaption for interfractional changes. Radiother Oncol (2020) 153:88–96. doi: 10.1016/j.radonc.2020.06.017
74. Tolan S, Kong V, Rosewall T, Craig T, Bristow R, Milosevic M, et al. Patient-specific PTV margins in radiotherapy for bladder cancer - a feasibility study using cone beam CT. Radiother Oncol (2011) 99(2):131–6. doi: 10.1016/j.radonc.2011.04.008
75. Pos FJ, Hulshof M, Lebesque J, Lotz H, van Tienhoven G, Moonen L, et al. Adaptive radiotherapy for invasive bladder cancer: a feasibility study. Int J Radiat Oncol Biol Phys (2006) 64(3):862–8. doi: 10.1016/j.ijrobp.2005.07.976
76. Burridge N, Amer A, Marchant T, Sykes J, Stratford J, Henry A, et al. Online adaptive radiotherapy of the bladder: small bowel irradiated-volume reduction. Int J Radiat Oncol Biol Phys (2006) 66(3):892–7. doi: 10.1016/j.ijrobp.2006.07.013
77. Lalondrelle S, Huddart R, Warren-Oseni K, Hansen VN, McNair H, Thomas K, et al. Adaptive-predictive organ localization using cone-beam computed tomography for improved accuracy in external beam radiotherapy for bladder cancer. Int J Radiat Oncol Biol Phys (2011) 79(3):705–12. doi: 10.1016/j.ijrobp.2009.12.003
78. Foroudi F, Pham D, Rolfo A, Bressel M, Tang CI, Tan A, et al. The outcome of a multi-centre feasibility study of online adaptive radiotherapy for muscle-invasive bladder cancer TROG 10.01 BOLART. Radiother Oncol (2014) 111(2):316–20. doi: 10.1016/j.radonc.2014.02.015
79. McDonald F, Lalondrelle S, Taylor H, Warren-Oseni K, Khoo V, McNair HA, et al. Clinical implementation of adaptive hypofractionated bladder radiotherapy for improvement in normal tissue irradiation. Clin Oncol (R Coll Radiol) (2013) 25(9):549–56. doi: 10.1016/j.clon.2013.06.001
80. Hafeez S, Warren-Oseni K, McNair HA, Hansen VN, Jones K, Tan M, et al. Prospective Study Delivering Simultaneous Integrated High-dose Tumor Boost (</=70 Gy) With Image Guided Adaptive Radiation Therapy for Radical Treatment of Localized Muscle-Invasive Bladder Cancer. Int J Radiat Oncol Biol Phys (2016) 94(5):1022–30. doi: 10.1016/j.ijrobp.2015.12.379
81. Vestergaard A, Muren LP, Sondergaard J, Elstrom UV, Hoyer M, Petersen JB. Adaptive plan selection vs. re-optimisation in radiotherapy for bladder cancer: a dose accumulation comparison. Radiother Oncol (2013) 109(3):457–62. doi: 10.1016/j.radonc.2013.08.045
82. Vestergaard A, Hafeez S, Muren LP, Nill S, Hoyer M, Hansen VN, et al. he potential of MRI-guided online adaptive re-optimisation in radiotherapy of urinary bladder cancer. Radiother Oncol (2016) 118(1):154–9. doi: 10.1016/j.radonc.2015.11.003
83. Hu Y, Rankine L, Green OL, Kashani R, Li HH, Li H, et al. Characterization of the onboard imaging unit for the first clinical magnetic resonance image guided radiation therapy system. Med Phys (2015) 42(10):5828–37. doi: 10.1118/1.4930249
84. Lagendijk JJ, Raaymakers BW, Raaijmakers AJ, Overweg J, Brown KJ, Kerkhof EM, et al. MRI/linac integration. Radiother Oncol (2008) 86(1):25–9. doi: 10.1016/j.radonc.2007.10.034
85. Hunt A, Hansen VN, Oelfke U, Nill S, Hafeez S. Adaptive Radiotherapy Enabled by MRI Guidance. Clin Oncol (R Coll Radiol) (2018) 30(11):711–9. doi: 10.1016/j.clon.2018.08.001
86. Noel CE, Parikh PJ, Spencer CR, Green OL, Hu Y, Mutic S, et al. Comparison of onboard low-field magnetic resonance imaging versus onboard computed tomography for anatomy visualization in radiotherapy. Acta Oncol (2015) 54(9):1474–82. doi: 10.3109/0284186X.2015.1062541
87. Hunt A, Hanson I, Dunlop A, Barnes H, Bower L, Chick J, et al. Feasibility of magnetic resonance guided radiotherapy for the treatment of bladder cancer. Clin Transl Radiat Oncol (2020) 25:46–51. doi: 10.1016/j.ctro.2020.09.002
88. Henke LE, Contreras JA, Green OL, Cai B, Kim H, Roach MC, et al. Magnetic Resonance Image-Guided Radiotherapy (MRIgRT): A 4.5-Year Clinical Experience. Clin Oncol (2018) 30(11):720–7. doi: 10.1016/j.clon.2018.08.010
89. Foroudi F, Wong J, Kron T, Rolfo A, Haworth A, Roxby P, et al. Online adaptive radiotherapy for muscle-invasive bladder cancer: results of a pilot study. Int J Radiat Oncol Biol Phys (2011) 81(3):765–71. doi: 10.1016/j.ijrobp.2010.06.061
90. Tuomikoski L, Collan J, Keyrilainen J, Visapaa H, Saarilahti K, Tenhunen M. Adaptive radiotherapy in muscle invasive urinary bladder cancer–an effective method to reduce the irradiated bowel volume. Radiother Oncol (2011) 99(1):61–6. doi: 10.1016/j.radonc.2011.02.011
91. Foroudi F, Wilson L, Bressel M, Haworth A, Hornby C, Pham D, et al. A dosimetric comparison of 3D conformal vs intensity modulated vs volumetric arc radiation therapy for muscle invasive bladder cancer. Radiat Oncol (2012) 7:111. doi: 10.1186/1748-717X-7-111
92. Acharya S, Fischer-Valuck BW, Kashani R, Parikh P, Yang D, Zhao T, et al. Online Magnetic Resonance Image Guided Adaptive Radiation Therapy: First Clinical Applications. Int J Radiat Oncol Biol Phys (2016) 94(2):394–403. doi: 10.1016/j.ijrobp.2015.10.015
93. Raaymakers BW, Jurgenliemk-Schulz IM, Bol GH, Glitzner M, Kotte A, van Asselen B, et al. First patients treated with a 1.5 T MRI-Linac: clinical proof of concept of a high-precision, high-field MRI guided radiotherapy treatment. Phys Med Biol (2017) 62(23):L41–50. doi: 10.1088/1361-6560/aa9517
94. Cusumano D, Dhont J, Boldrini L, Chiloiro G, Romano A, Votta C, et al. et al: Reliability of ITV approach to varying treatment fraction time: a retrospective analysis based on 2D cine MR images. Radiat Oncol (2020) 15(1):152. doi: 10.1186/s13014-020-01530-6
95. Tetar SU, Bruynzeel AME, Lagerwaard FJ, Slotman BJ, Bohoudi O, Palacios MA. Clinical implementation of magnetic resonance imaging guided adaptive radiotherapy for localized prostate cancer. Phys Imaging Radiat Oncol (2019) 9:69–76. doi: 10.1016/j.phro.2019.02.002
96. Henke L, Kashani R, Robinson C, Curcuru A, DeWees T, Bradley J, et al. Phase I trial of stereotactic MR-guided online adaptive radiation therapy (SMART) for the treatment of oligometastatic or unresectable primary malignancies of the abdomen. Radiother Oncol (2018) 126(3):519–26. doi: 10.1016/j.radonc.2017.11.032
97. Palacios MA, Bohoudi O, Bruynzeel AME, van Sornsen-de Koste JR, Cobussen P, Slotman BJ, et al. Role of daily plan adaptation in MR-guided stereotactic ablative radiotherapy for adrenal metastases. Int J Radiat Oncol Biol Phys (2018) 102(2):426–33. doi: 10.1016/j.ijrobp.2018.06.002
98. Efstathiou JA, Bae K, Shipley WU, Kaufman DS, Hagan MP, Heney NM, et al. Late pelvic toxicity after bladder-sparing therapy in patients with invasive bladder cancer: RTOG 89-03, 95-06, 97-06, 99-06. J Clin Oncol (2009) 27(25):4055–61. doi: 10.1200/JCO.2008.19.5776
99. Viswanathan AN, Yorke ED, Marks LB, Eifel PJ, Shipley WU. Radiation dose-volume effects of the urinary bladder. Int J Radiat Oncol Biol Phys (2010) 76(3 Suppl):S116–122. doi: 10.1016/j.ijrobp.2009.02.090
100. Emami B, Lyman J, Brown A, Coia L, Goitein M, Munzenrider JE, et al. Tolerance of normal tissue to therapeutic irradiation. Int J Radiat Oncol Biol Phys (1991) 21(1):109–22. doi: 10.1016/0360-3016(91)90171-Y
101. Mannion L, Bosco C, Nair R, Mullassery V, Enting D, Jones EL, et al. Overall survival, disease-specific survival and local recurrence outcomes in patients with muscle-invasive bladder cancer treated with external beam radiotherapy and brachytherapy: a systematic review. BJU Int (2020) 125(6):780–91. doi: 10.1111/bju.15047
102. Huddart RA, Hall E, Hussain SA, Jenkins P, Rawlings C, Tremlett J, et al. Randomized noninferiority trial of reduced high-dose volume versus standard volume radiation therapy for muscle-invasive bladder cancer: results of the BC2001 trial (CRUK/01/004). Int J Radiat Oncol Biol Phys (2013) 87(2):261–9. doi: 10.1016/j.ijrobp.2013.06.2044
103. Cowan RA, McBain CA, Ryder WD, Wylie JP, Logue JP, Turner SL, et al. Radiotherapy for muscle-invasive carcinoma of the bladder: results of a randomized trial comparing conventional whole bladder with dose-escalated partial bladder radiotherapy. Int J Radiat Oncol Biol Phys (2004) 59(1):197–207. doi: 10.1016/j.ijrobp.2003.10.018
104. Voskuilen CS, Bosschieter J, van Werkhoven E, Hendricksen K, Vis AN, Witteveen T, et al. Long-term survival and complications following bladder-preserving brachytherapy in patients with cT1-T2 bladder cancer. Radiother Oncol (2019) 141:130–6. doi: 10.1016/j.radonc.2019.09.026
105. Huddart R, Hall E, Hussein SA, Jenkins P, Rawlings C, Tremlett J, et al. Randomized noninferiority trial of reduced high-dose volume versus standard volume radiation therapy for muscle-invasive bladder cancer: results of the BC2001 trial (CRUK/01/004). Int J Radiat Oncol Biol Phys (2013) 87(2):261–9. doi: 10.1016/j.ijrobp.2013.06.2044. Erratum in: Int J Radiat Oncol Biol Phys. 2013 Dec 1;87(5):860
106. Meijer GJ, van der Toorn PP, Bal M, Schuring D, Weterings J, de Wildt M. High precision bladder cancer irradiation by integrating a library planning procedure of 6 prospectively generated SIB IMRT plans with image guidance using lipiodol markers. Radiother Oncol (2012) 105(2):174–9. doi: 10.1016/j.radonc.2012.08.011
107. Murthy V, Gupta P, Baruah K, Krishnatry R, Joshi A, Prabhash K, et al. Adaptive Radiotherapy for Carcinoma of the Urinary Bladder: Long-term Outcomes With Dose Escalation. Clin Oncol (R Coll Radiol) (2019) 31(9):646–52. doi: 10.1016/j.clon.2019.06.005
108. Sondergaard J, Holmberg M, Jakobsen AR, Agerbaek M, Muren LP, Hoyer M. A comparison of morbidity following conformal versus intensity-modulated radiotherapy for urinary bladder cancer. Acta Oncol (2014) 2014:1–8. doi: 10.3109/0284186X.2014.928418
109. Hafeez S, Webster A, Hansen VN, McNair HA, Warren-Oseni K, Patel E, et al. Protocol for tumour-focused dose-escalated adaptive radiotherapy for the radical treatment of bladder cancer in a multicentre phase II randomised controlled trial (RAIDER): radiotherapy planning and delivery guidance. BMJ Open (2020) 10(12):e041005. doi: 10.1136/bmjopen-2020-041005
110. Nishioka K, Shimizu S, Kinoshita R, Inoue T, Onodera S, Yasuda K, et al. Evaluation of inter-observer variability of bladder boundary delineation on cone-beam CT. Radiat Oncol (2013) 8:185. doi: 10.1186/1748-717X-8-185
111. Zietman AL, Grocela J, Zehr E, Kaufman DS, Young RH, Althausen AF, et al. Selective bladder conservation using transurethral resection, chemotherapy, and radiation: management and consequences of Ta, T1, and Tis recurrence within the retained bladder. Urology (2001) 58(3):380–5. doi: 10.1016/S0090-4295(01)01219-5
112. Lotz HT, Pos FJ, Hulshof MC, van Herk M, Lebesque JV, Duppen JC, et al. Tumor motion and deformation during external radiotherapy of bladder cancer. Int J Radiat Oncol Biol Phys (2006) 64(5):1551–8. doi: 10.1016/j.ijrobp.2005.12.025
113. Vandewinckele L, Claessens M, Dinkla A, Brouwer C, Crijns W, Verellen D, et al. Overview of artificial intelligence-based applications in radiotherapy: Recommendations for implementation and quality assurance. Radiother Oncol (2020) 153:55–66. doi: 10.1016/j.radonc.2020.09.008
114. Bol GH, Lagendijk JJ, Raaymakers BW. Virtual couch shift (VCS): accounting for patient translation and rotation by online IMRT re-optimization. Phys Med Biol (2013) 58(9):2989–3000. doi: 10.1088/0031-9155/58/9/2989
115. Winkel D, Bol GH, Kroon PS, van Asselen B, Hackett SS, Werensteijn-Honingh AM, et al. et al: Adaptive radiotherapy: The Elekta Unity MR-linac concept. Clin Transl Radiat Oncol (2019) 18:54–9. doi: 10.1016/j.ctro.2019.04.001
116. Hafeez S, Huddart R. Advances in bladder cancer imaging. BMC Med (2013) 11:104. doi: 10.1186/1741-7015-11-104
117. Afaq A, Koh DM, Padhani A, van As N, Sohaib SA. Clinical utility of diffusion-weighted magnetic resonance imaging in prostate cancer. BJU Int (2011) 108(11):1716–22. doi: 10.1111/j.1464-410X.2011.10256.x
118. Gerlinger M, Rowan AJ, Horswell S, Larkin J, Endesfelder D, Gronroos E, et al. Intratumor heterogeneity and branched evolution revealed by multiregion sequencing. N Engl J Med (2012) 366(10):883–92. doi: 10.1056/NEJMoa1113205
119. Barajas RF Jr., Rubenstein JL, Chang JS, Hwang J, Cha S. Diffusion-weighted MR imaging derived apparent diffusion coefficient is predictive of clinical outcome in primary central nervous system lymphoma. AJNR Am J Neuroradiol (2010) 31(1):60–6. doi: 10.3174/ajnr.A1750
120. Pope WB, Kim HJ, Huo J, Alger J, Brown MS, Gjertson D, et al. Recurrent glioblastoma multiforme: ADC histogram analysis predicts response to bevacizumab treatment. Radiology (2009) 252(1):182–9. doi: 10.1148/radiol.2521081534
121. Kyriazi S, Collins DJ, Messiou C, Pennert K, Davidson RL, Giles SL, et al. Metastatic ovarian and primary peritoneal cancer: assessing chemotherapy response with diffusion-weighted MR imaging–value of histogram analysis of apparent diffusion coefficients. Radiology (2011) 261(1):182–92. doi: 10.1148/radiol.11110577
122. Yoshida S, Koga F, Kobayashi S, Ishii C, Tanaka H, Tanaka H, et al. Role of diffusion-weighted magnetic resonance imaging in predicting sensitivity to chemoradiotherapy in muscle-invasive bladder cancer. Int J Radiat Oncol Biol Phys (2012) 83(1):e21–27. doi: 10.1016/j.ijrobp.2011.11.065
123. Hafeez S, Koh M, Sohaib A, Huddart R. PO-0734: Assessing response to radiotherapy with diffusion weighted MRI (DW-MRI) in muscle invasive bladder cancer (MIBC). Radiother Oncol (2014) 111, Supplement 1(0):S34. doi: 10.1016/S0167-8140(15)30852-5
124. Kooreman ES, van Houdt PJ, Keesman R, Pos FJ, van Pelt VWJ, Nowee ME, et al. ADC measurements on the Unity MR-linac - A recommendation on behalf of the Elekta Unity MR-linac consortium. Radiother Oncol (2020) 153:106–13. doi: 10.1016/j.radonc.2020.09.046
125. Hoskin PJ, Rojas AM, Bentzen SM, Saunders MI. Radiotherapy with concurrent carbogen and nicotinamide in bladder carcinoma. J Clin Oncol (2010) 28(33):4912–8. doi: 10.1200/JCO.2010.28.4950
126. Epel B, Maggio MC, Barth ED, Miller RC, Pelizzari CA, Krzykawska-Serda M, et al. Oxygen-Guided Radiation Therapy. Int J Radiat Oncol Biol Phys (2019) 103(4):977–84. doi: 10.1016/j.ijrobp.2018.10.041
127. O’Connor JP, Naish JH, Parker GJ, Waterton JC, Watson Y, Jayson GC, et al. Preliminary study of oxygen-enhanced longitudinal relaxation in MRI: a potential novel biomarker of oxygenation changes in solid tumors. Int J Radiat Oncol Biol Phys (2009) 75(4):1209–15. doi: 10.1016/j.ijrobp.2008.12.040
128. Price JM, Robinson SP, Koh DM. Imaging hypoxia in tumours with advanced MRI. Q J Nucl Med Mol Imaging Off Publ Ital Assoc Nucl Med (2013) 57(3):257–70.
129. Taylor NJ, Baddeley H, Goodchild KA, Powell ME, Thoumine M, Culver LA, et al. BOLD MRI of human tumor oxygenation during carbogen breathing. J Magn Reson Imaging (2001) 14(2):156–63. doi: 10.1002/jmri.1166
130. Nguyen HT, Jia G, Shah ZK, Pohar K, Mortazavi A, Zynger DL, et al. Prediction of chemotherapeutic response in bladder cancer using K-means clustering of dynamic contrast-enhanced (DCE)-MRI pharmacokinetic parameters. J Magn Reson Imaging (2015) 41(5):1374–82. doi: 10.1002/jmri.24663
131. Chan K, Warren-Oseni K, Abdel-Aty H, Dunlop A, McQuaid D, Koh M, et al. Normal tissue sparing with diffusion weighted MRI informed tumour boost in bladder radiotherapy. Radiother Oncol (2019) 133:S455–6. doi: 10.1016/S0167-8140(19)31284-8
132. Jagodinsky JC, Harari PM, Morris ZS. The Promise of Combining Radiation Therapy with Immunotherapy. Int J Radiat Oncol Biol Phys (2020) 108(1):6–16. doi: 10.1016/j.ijrobp.2020.04.023
133. Daro-Faye M, Kassouf W, Souhami L, Marcq G, Cury F, Niazi T, et al. Combined radiotherapy and immunotherapy in urothelial bladder cancer: harnessing the full potential of the anti-tumor immune response. World J Urol (2020) 11:1–13. doi: 10.1007/s00345-020-03440-4
134. de Mol van Otterloo SR, Christodouleas JP, Blezer ELA, Akhiat H, Brown K, Choudhury A, et al. The MOMENTUM Study: An International Registry for the Evidence-Based Introduction of MR-Guided Adaptive Therapy. Front Oncol (2020) 10(2234-943X(2234-943X (Print):1328. doi: 10.3389/fonc.2020.01328
Keywords: adaptive radiotherapy, bladder cancer, MR guided radiotherapy, MR-linac, MRI
Citation: Hijab A, Tocco B, Hanson I, Meijer H, Nyborg CJ, Bertelsen AS, Smeenk RJ, Smith G, Michalski J, Baumann BC and Hafeez S (2021) MR-Guided Adaptive Radiotherapy for Bladder Cancer. Front. Oncol. 11:637591. doi: 10.3389/fonc.2021.637591
Received: 03 December 2020; Accepted: 11 January 2021;
Published: 25 February 2021.
Edited by:
Vincenzo Valentini, Catholic University of the Sacred Heart, ItalyReviewed by:
Khaled Elsayad, University of Münster, GermanyMarco Krengli, Università del Piemonte Orientale, Italy
Copyright © 2021 Hijab, Tocco, Hanson, Meijer, Nyborg, Bertelsen, Smeenk, Smith, Michalski, Baumann and Hafeez. This is an open-access article distributed under the terms of the Creative Commons Attribution License (CC BY). The use, distribution or reproduction in other forums is permitted, provided the original author(s) and the copyright owner(s) are credited and that the original publication in this journal is cited, in accordance with accepted academic practice. No use, distribution or reproduction is permitted which does not comply with these terms.
*Correspondence: Shaista Hafeez, c2hhaXN0YS5oYWZlZXpAaWNyLmFjLnVr
†These authors share first authorship