- 1Guangdong Immune Cell Therapy Engineering and Technology Research Center, Center for Protein and Cell-Based Drugs, Institute of Biomedicine and Biotechnology, Shenzhen Institutes of Advanced Technology, Chinese Academy of Sciences, Shenzhen, China
- 2University of Chinese Academy of Sciences, Beijing, China
The attachment of cells to the extracellular matrix (ECM) is the hallmark of structure–function stability and well-being. ECM detachment in localized tumors precedes abnormal dissemination of tumor cells culminating in metastasis. Programmed cell death (PCD) is activated during tumorigenesis to clear off ECM-detached cells through “anoikis.” However, cancer cells develop several mechanisms for abrogating anoikis, thus promoting their invasiveness and metastasis. Specific factors, such as growth proteins, pH, transcriptional signaling pathways, and oxidative stress, have been reported as drivers of anoikis resistance, thus enhancing cancer proliferation and metastasis. Recent studies highlighted the key contributions of metabolic pathways, enabling the cells to bypass anoikis. Therefore, understanding the mechanisms driving anoikis resistance could help to counteract tumor progression and prevent metastasis. This review elucidates the dynamics employed by cancer cells to impede anoikis, thus promoting proliferation, invasion, and metastasis. In addition, the authors have discussed other metabolic intermediates (especially amino acids and nucleotides) that are less explored, which could be crucial for anoikis resistance and metastasis.
Introduction
Cell–cell adherence and their interaction with the extracellular matrix (ECM) are imperative for the growth and survival of cells. The ECM is a three-dimensional scaffold that provides the required critical biochemical and mechanical signals for tissue formation, growth, differentiation, and homeostasis (1). It is composed of varying biomolecules, such as glycosaminoglycans, proteoglycans, and fibrous proteins (1, 2). Adhesion receptors, such as integrin, cadherin, selectin, and immunoglobulin-like cell adhesion molecules, mediate the attachment of cells to the ECM (3). The meshwork between the biomolecules and cell adhesion molecules in the ECM regulates homeostasis. Aberrations in the ECM often lead to severe dysfunction and even death, both at the microscopic and organism levels, which have been implicated in diverse mammalian diseases, including cancer (4, 5).
Cell death is a vital occurrence as it facilitates the development and differentiation of tissues, clearance of harmful or damaged cells, and maintenance of homeostasis (6, 7). Some studies had highlighted that cell death a regulated event and categorized it as programmed cell death (PCD) and non-PCD based on the biochemical and molecular signaling activities within and without cell milieu (8–10). PCD includes both apoptotic and non-apoptotic cell death. Apoptotic cell death (apoptosis and anoikis) mainly occurs through the activation of caspase while non-apoptotic cell deaths are independent of caspase (9, 10). Furthermore, non-apoptotic cell death includes entosis, methuosis, paraptosis, mitotopsis, parthanatos, ferroptosis, pyroptosis, netosis, necrosis, and autophagic cell death as mentioned in the previous studies (9, 10). On the other hand, non-PCD, also known as accidental cell death, is a type of death resulting from an unpredicted or unforeseen cell injury (8, 9).
The disruption in cell–cell attachment or cell-ECM attachment leads to a form of apoptotic cell death, which is termed as “anoikis” (11). Anoikis is a mechanism for the elimination of misplaced or detached cells under physiological or pathological conditions, which facilitate tissue homeostasis (12). Anoikis in cancer cells retards metastasis of cells to other sites; however, this is often not the case due to cancer cells becoming anoikis resistant. In addition to cancer, anoikis occurs in other pathological conditions such as diabetes and cardiovascular disorders (13). It ensures clearance of detached cells and this could be detrimental if excessively activated in diabetes and cardiovascular disease (14, 15). The initiation of anoikis is facilitated through the intrinsic (mitochondrial approach) and extrinsic (death receptor approach) caspase activation similar to the apoptotic cascade of endonucleases activation, DNA damage, and cell death (11, 16). Anoikis resistance occurs occasionally when detached cells circumvent death signaling pathway, enabling survival of cells as a result of several biochemical and molecular alterations within the cell milieu. These changes are the hallmark of invasiveness, metastasis, therapy resistance, and relapse of cancer cells.
Cancer is the second leading cause of death (17), most of which is not due to localized tumors but a result of metastasizing cells within the tumor mass. Cancer cells metastasize after successive processes of detachment from one another or the ECM, migrating to distal points, promoting reattachment, and proliferation in the new site (18). Cancer cells employ several mechanisms for abrogating anoikis, thus promoting their invasiveness and metastasis. Cellular acidosis and the changes in reactive oxygen species (ROS) generation in cancer cells had a tremendous impact in promoting anoikis resistance (19–21). These changes promote oncogenic signals that induce pro-survival pathways, leading to stemness, proliferation, and invasion (19–21). Similarly, the expression of long non-coding RNA (lncRNA) correlated with anoikis resistance and metastasis in cancer (22, 23). Recent studies highlighted the role of metabolic alteration and signaling pathways driving metabolic processes as an alternative approach to target anoikis resistance in cancer cells (24–26). While nutrient uptake by cells is necessary to supply the energy required for cellular growth, abnormal metabolism in patients with cancer could lead to anoikis resistance and promote therapy resistance. Therefore, a holistic understanding of several mechanisms underlying anoikis resistance could help in delaying tumor progression and prevent metastasis.
This review aims to explore the current knowledge on apoptosis, the regulators of anoikis resistance [such as pH, oxidative stress, growth factors (GFs), cancer stem cells (CSCs), and lncRNA], the role of signaling pathways as “drivers” of anoikis resistance, and the relevance of targeting metabolism to ameliorate tumor metastasis. The authors also shared their perspective on potential targets for ameliorating metastasis and their insights on current therapies that sensitize cancer cells to anoikis.
Anoikis: A Particular Type of Apoptosis
Biochemical and environmental changes within and outside the cell can activate cell death signals. Apoptosis is a form of regulated cell death involved in terminating the cell function to ensure homeostasis, normal growth, and the elimination of potential hazards (6, 7). Cell shrinkage, cell blebbing (plasma membrane protrusion), karyorrhexis (nucleus fragmentation), pyknosis (irreversible condensation of chromatin), and DNA fragmentation are some features of cells undergoing apoptosis (27–29). Prior to the aforementioned features, apoptosis can be initiated through intrinsic, extrinsic, or perforin/granzyme A/B pathways (16). All apoptosis initiation pathways are largely caspase dependent except granzyme A, which induces damage to the mitochondria as a committed step for apoptosis following cellular stress (30). Perforin/granzyme is a cytotoxic lymphocyte-mediated process. Granzyme B impaired tumor spread, migration, and invasion in human cancer cell lines while it induced anoikis in endothelial cells (ECs) through the modulation of cell adhesion (31). The authors identified that granzme B cleaved some ECM proteins, including laminin, fibronectin, and vitronectin, to induce detachment, anoikis, and inhibition of the migration (31).
The intrinsic pathway of apoptosis is a cellular stress-induced mitochondrial event. These stress signals could lead to permeabilization of the mitochondria or its swelling through the formation of membrane pores and the release of apoptotic proteins, such as cytochrome c, second mitochondria-derived activator of caspases (Smac/DIABLO), and serine protease (16). These proteins activate caspases for apoptosis in the mitochondria. Cytochrome c binds to an apoptotic protease activating factor-1 (Apaf-1) to form an apoptosome complex, which induces death signal spiraling pro-caspase-9 to caspase-9 and activates the effector caspase-3 and effector caspase-7. Smac/DIABLO and serine proteases promote apoptosis by antagonizing the inhibitors of apoptosis to further enhance the activity of effector caspase-3, effector caspase-7, and effector caspase-9 for killing the cells (32).
The extrinsic approach is mediated by tumor necrosis factors (TNFs) and the first apoptosis signal (Fas)-ligand (16). TNF binds to a TNF receptor (TNFR1) and propels the activation of caspase-8 for the cleavage of other effector caspases to activate apoptosis through TNF receptor-associated death domain (TRADD) and Fas-associated death domain (FADD) (16). Fas-ligand binds to an Apo-1 or CD 95-a transmembrane protein of the TNF family to form a death-inducing signaling complex (DISC). DISC activates caspase-8, which in turn activates effector caspase-3 and effector caspase-7 for cell death (33).
Anoikis is essentially an apoptotic event, which is activated to clear detached cells (34), and is a form of regulated cell death that utilizes both the intrinsic and extrinsic pathways. Similar to apoptosis, pro-apoptotic events are activated in the mitochondria or DISC formation to propel the activity of effector caspases for elimination of detached cells and restoration of tissue homeostasis.
Regulators of Anoikis Resistance
The evasion of anoikis in detached cells from localized tumors results in uncontrolled growth of these cancer cells in other sites. Studies have identified several factors, such as cell adhesion molecules, growth proteins, oxidative stress, stemness, autophagy, non-coding RNAs, and signaling pathways, for modulating anoikis resistance (Figures 1, 2). In the present study, we discuss some of these factors and their roles in mediating anoikis resistance, which are summarized in Table 1.
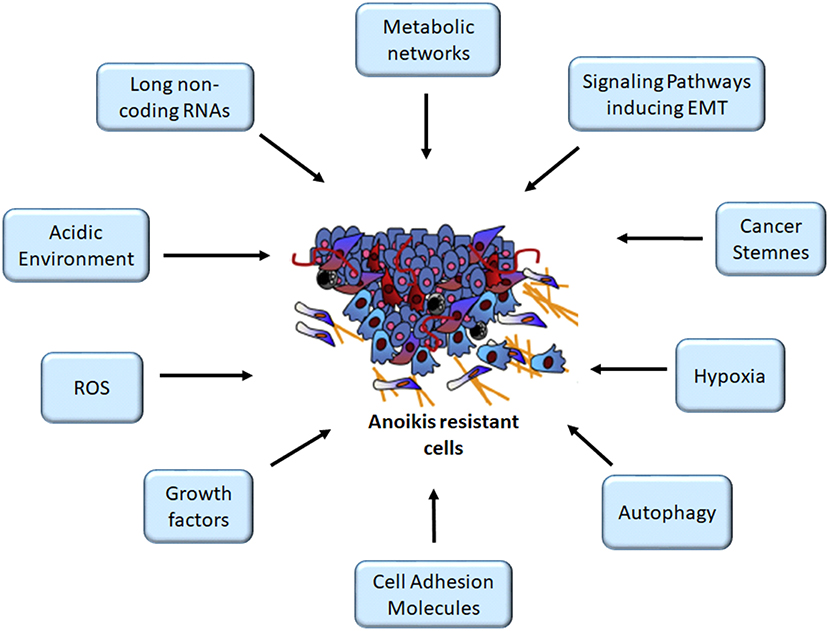
Figure 1. Mediators of anoikis-resistant cells. The figure depicts several factors altering anoikis mechanism, thus enhancing cancer cell survival following detachment, an important criterion for the initiation of metastasis cascade. ROS, reactive oxygen species. Image for anoikis resistant cells was adapted from (11).
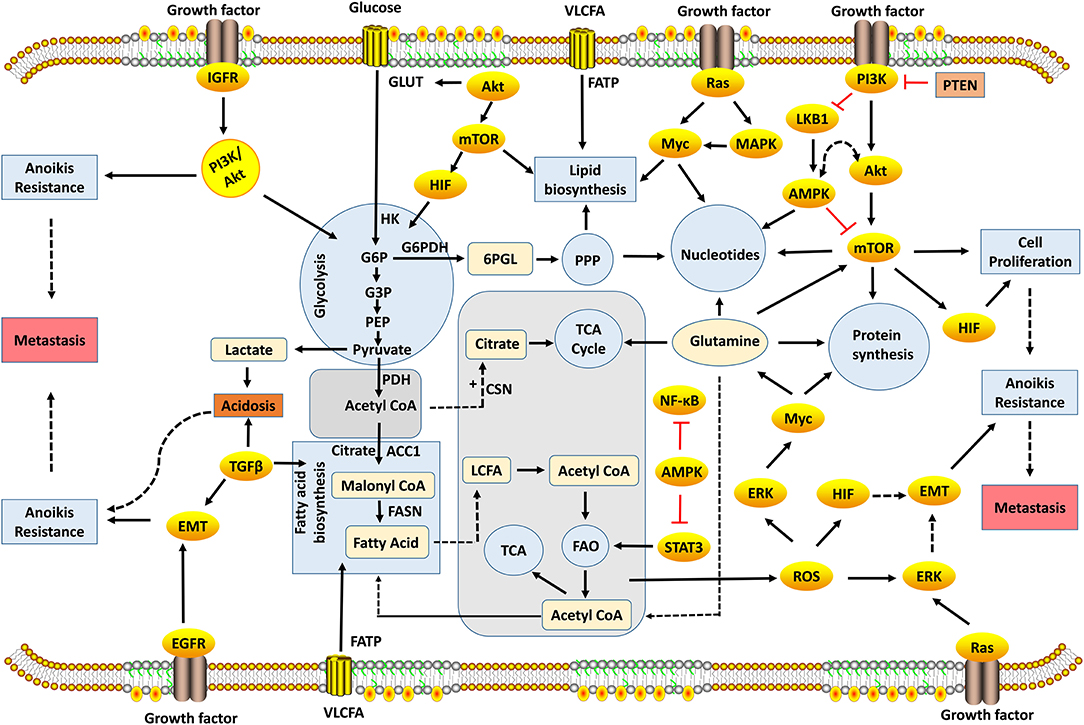
Figure 2. Metabolic and signaling networks involved in anoikis-resistance mechanism. Several metabolic intermediates generate the energy required for cellular growth and survival; oncogenic activation of signaling pathways reprogram cellular metabolism to promote anoikis resistance. Metabolic pathways occur in different compartments, which involve the biosynthesis or degradation of biomolecules to supply energy needs of the cell. Glycolysis, PPP, protein, fatty acid, and nucleotide biosynthesis take place in the cytosol, while TCA and FAO occur in the mitochondria. The decarboxylation of pyruvate to acetyl-CoA takes place in the mitochondrial matrix. These metabolic pathways are interrelated with acetyl-CoA, which acts a key substrate for connecting these pathways. Metabolism is regulated by rate-limiting enzymes that allow the cell to maintain cellular homeostasis. However, the dysregulation of genes encoding these enzymes leads to metabolic perturbation that drives anoikis resistance preceding tumor metastasis. 6PGL, 6-Phosphogluconolactone; AMPK, Adenosine Monophosphate Kinase; ACC1, Acetyl Carboxylase 1; ATP, Adenosine Triphosphate; CSN, Citrate Synthase; EGFR, Epidermal Growth Factor Receptors; EMT, Epithelial-Mesenchymal Transition; ERK, Extracellular-Signal-Regulated Kinase; FAO, Fatty Acid Oxidation; FASN, Fatty Acid Synthase; FATP, Fatty Acid Transport Proteins; G3P, Glyceraldehyde-3-Phosphate; G6P, Glucose-6-Phosphate; G6PDH, Glucose-6-Phosphate Dehydrogenase; GLUT, Glucose Transporters; HIF, Hypoxia-Inducible Factors; HK, Hexokinase; IGFR, Insulin-Like Growth Factor-1 Receptor; LKB1, Liver Kinase B1; MAPK, Mitogen-Activated Protein Kinase; mTOR, Mammalian Target of Rapamycin; NF-κB, Nuclear Factor-Kappa B; PDH, Pyruvate Dehydrogenase; PEP, Phosphoenolpyruvate; PI3K, Phosphatidylinositol 3-Kinase; PPP, Pentose Phosphate Pathway; PTEN, Phosphatase and Tensin; ROS, Reactive Oxygen Species; STAT3, Signal Transducer and Activator of Transcription 3; TCA, Tricarboxylic Acid; TGF-β, Transforming Growth Factor-Beta; VLCFA, Very Long-Chain Fatty Acid.
Cell Adhesion Molecules
The role of adhesion molecules in ECM stabilization is critical for anoikis, and changes in these molecules could confer anoikis resistance, which is a prerequisite for metastasis. A recent study on laryngeal squamous cell carcinoma reported that E-cadherin and N-cadherin, which are markers of an epithelial-mesenchymal transition (EMT—a transformation predisposing cells to adopt an anoikis-resistance phenotype) were downregulated and upregulated, respectively, thus contributing to tumor progression (35). Integrin αvβ3 is involved in the transduction of antiapoptotic signals by facilitating anchorage-independent growth (AIG) and metastatic potential of pancreatic cancer through the activation of cSrc in a focal adhesion kinase (FAK)—independent manner (36). Head and neck squamous cell carcinoma (HNSCC)—expressing high-integrin β1 was correlated with metastatic potential, a low survival in patients by modulating metalloproteinase-2 (MMP-2) activation (37). Similarly, a study reported that a docetaxel resistant, high-integrin α2β1-expressing prostate cancer cell possessed an increased invasive migratory capability but a retarded proliferation. However, low α2β1-expressing prostate cancer cells had higher proliferation and compact spheroids with less migration potential (38). These authors demonstrated that high-integrin α2β1 invasive capability was a result of activation of the p38-MAPK-MMP-1 signaling pathways. Similarly, the treatment of a breast cancer cell line (MCF-7) with lectin, a glycoprotein, induced anoikis through the reduction of integrin and the impairment of integrin-FAK signaling (102).
Kang et al. reported that soluble E-selectin promoted anoikis resistance and migration through its interaction with CD44 in breast cancer cell lines [melanoma differentiation-associated (MDA)-MB-231 and MDA-MB-468 cell lines] to promote shear-resistant adhesion to EC and the circulation of tumor cells, thus promoting metastasis (39). High E-selectin with no significant changes in intercellular adhesion molecule-1 (ICAM-1) and vascular cell adhesion molecule-1 (VCAM-1) were observed in the circulation of EC-expressing B-cell lymphoma-2 (Bcl-2) (40). Employing neutralizing antibodies against E-selectin, ICAM-1 and VCAM-1 revealed that anti-E-selectin was able to block tumor cell binding, while anti-ICAM-1 and anti-VCAM-1 failed to significantly block tumor cell binding (40). This study showed that the coculturing of tumor cells with Bcl-2-expressing EC conferred anoikis resistance on the tumor cells via the activation of E-selectin-FAK signaling. However, blocking of the E-selectin or FAK restored anoikis sensitivity in cancer cells cocultured with EC (40). On the other hand, Roland et al. studied a positive correlation of ICAM-1 expression with cancer cell malignancy (103). Cell surface proteoglycans (such as the Syndecan family) participated in cell adhesion (104), anoikis-resistant EC was showed to upregulate Syndecan-4 in comparison to the parent EC through an increased heparanase expression (105). Perlecan, a heparan sulfate proteoglycan in the ECM, is involved in cascades of the metastasis process (106). Treatment of anoikis-resistant EC with trastuzumab (an anticancer drug) significantly reduced Syndecan-4 and perlecan expression, which impaired cell proliferation and invasion (107). The roles of some adhesion molecules in mediating anoikis resistance and metastasis were previously reviewed by other authors (106, 108, 109). However, further elucidation of the interactions of different adhesion molecules is inherent in understanding the dynamics of ECM reconstruction in modulating anoikis signals to better curb tumor metastasis.
pH
The body homeostasis is dependent on the maintenance of physiological pH for different cells, tissues, and organs. Changes in pH were implicated in diseases (110). A recent study on melanoma identified that low extracellular pH promoted anoikis resistance through an increased N-cadherin expression with an enhanced migrative ability through the activation of epidermal growth factor receptor (EGFR) and Akt pathways (42). Nevertheless, the involvement of acidic-adapted melanoma cells in the metastatic cascade is yet to be elucidated (42). Another study reported that the culturing of cervical, pharynx, and colorectal cancer cell lines in an acidic environment showed an increase in lipid droplet formation, which facilitated anoikis resistance and the survival of cancer cells (43). Conversely, the significance of buffering acidity in solid tumor-bearing mice via oral administration of bicarbonate in combination with an immune checkpoint blockade or adoptive cell therapy in different cancer models was reported to improve the treatment outcome and survival of the mice model (111). Taken together, these studies suggest that the acidic environment stimulates anoikis resistance and tumor progression (Figure 1). Proper management of pH in solid tumors could be a promising approach to circumvent anoikis resistance. Therefore, future research can focus on the manipulation of pH to regulate the transformations conferring anoikis resistance in tumor cells.
Reactive Oxygen Species
The production of ROS by tumor cells is indicative of oxidative stress. There are contrasting views on the role of ROS during tumor growth, and it is yet to be fully understood how alteration in oxidative stress could contribute to anoikis. Two independent studies reported the generation of ROS as a result of the upregulation of NADPH oxidase 4 (NOX4) in gastric and lung cancer cells grown in suspension; this was observed to induce anoikis resistance and metastasis via EGFR signaling (44, 45). A recent study revealed an elevated ROS in CSCs as a metastatic trait to enhance EMT and the invasiveness of various previously studied cancer cell lines (19). On the contrary, low ROS content was observed in glioblastoma cultured in suspension with a concomitant increase in the expression of hypoxia-inducible factor (HIF) and was observed to be anoikis resistant (46). Likewise, two independent groups reported that the upregulation of manganese superoxide dismutase (an antioxidant that mops up ROS) promoted anoikis resistance and tumor metastasis in mammary epithelial cells and nasopharyngeal carcinoma, which suggested a high-level ROS capable of sensitizing the cells to anoikis upon their detachment (47, 48). The role of reactive radicals in cancer cannot be overemphasized and still requires further investigation in cancer from different origins to determine the specific effects of varying (low, moderate, or high) levels of ROS generated inside and outside the tumor environment. This may help to elucidate the overall ROS involvement in regulating anoikis resistance, thus providing novel means of targeting these species for better cancer therapy.
Growth Factors
Growth factors are regulatory molecules, mostly proteins or steroids, capable of initiating cell growth, proliferation, differentiation, inflammation, immunity, tissue healing and survival. The activity of GFs is accomplished by the interaction of GFs with target receptors, which could facilitate or incapacitate their functions. GF receptors are transmembrane proteins that bind to a specific GF and convey signals to the intracellular space. The receptors include EGFR, insulin-like growth factor (IGF) receptor, vascular endothelial growth factor (VEGF) receptor, fibroblast GF (FGF) receptor, platelet-derived GF receptor, and neurotropin receptor (112). For instance, epidermal growth factor (EGF) is a ligand of EGFR, a proto-oncogene that activates downstream kinases, such as FAK, to regulate cell adhesion site dynamics, thus promoting cancer growth, migration, and metastasis (49). IGF receptors include IGF-1 receptor (IGF1R), IGF-2 receptor (IGF2R), and insulin receptor (INSR). IGF/IGF1R mediated anoikis resistance in estrogen-responsive breast cancer via the signaling of a phosphoinositide-3-kinase (PI3k)/Akt pathway (Figure 2) (50). The expression of IGF receptors was implicated in the progression, metastasis, and therapy resistance of cancer (113, 114). The expression of VEGF-A and its receptor (VEGFR2/KDR) in epithelial ovarian cancer facilitated anoikis resistance, and the targeting of VEGF-A using a neutralizing antibody or KDR using a specific inhibitor sensitized the cells to anoikis (51). The signaling of FGF receptor-19 (FGFR19) and FGFR4 enabled the survival of breast cancer cells lines co-expressing them via the activation of PI3K/Akt pathway, targeting FGFR4/FGF19-sensitized cancer cells to death (52).
Brain-derived neurotrophic factor (BDNF) is a member of the neurotropin family, which is critical for neuron development and regeneration (115). The BDNF interaction with its receptor tyrosine kinase B (TrkB) was associated with anoikis resistance in cervical cancer (54). BDNF/TrkB expression was high in the anoikis-resistant model and promoted proliferation via the phosphorylation of P13k/Akt pathway (54). The upregulation of TrkB was also reported to promote EMT and anoikis resistance in endometrial carcinoma (55). Also, the silencing of TrkB in anoikis-resistant renal cell carcinoma abrogated proliferation, invasion, and sensitized cells to chemotherapy (sorafenib) through inactivation of the PI3K/Akt pathway and MEK/ERK signaling pathway (56). In addition to specific receptors, GFs also interact with the ECM to implement their functions (116). FGF is secreted into extracellular space where the cells bind to heparin-like glycosaminoglycan in the ECM to affect the localization of FGF and signal transduction in target cells (117). EGFR–integrin interaction was shown to inhibit anoikis by regulating Bim, a pro-apoptotic protein during cell detachment from the ECM (57). Also, the loss of hepatocellular carcinoma receptor protein 1 (HCRP-1) upregulated EGFR activity in modulating the expression of Bim, thus facilitating anoikis resistance and metastasis of colon cancer (58). In the same vein, a reduced pre-mRNA splicing factor 4 kinase (PRP4K) upregulated EGFR, thus facilitating anoikis resistance and metastasis (59). A different study reported that EGF induced the expression of angiopoietin-like-4 (ANGPTL4) protein in HNSCC, thereby favoring anoikis resistance (60). EGF regulated the expression of MMP-1 via integrin β1/c-Jun signaling, thus enabling the invasiveness and metastasis of HNSCC (60). As previously discussed, the NOX4-induced ROS generation in the cancer-activated EGFR signaling fostered anoikis resistance and metastasis (44, 45). Under an acidic environment, the upregulation of transforming growth factor-beta 2 (TGF-β2) induced anoikis resistance by facilitating EMT and lipid metabolism (Figure 2 and Table 1) (43). Also, TGF-β stimulated FGFR isoform switch to facilitate EMT and cancer progression (118). The exposure of pancreatic adenocarcinoma to platelet-derived GF-BB was recently reported to promote anoikis resistance and cell migration through EMT (53). The interaction of GFs with pro-survival signaling pathways mediated anoikis resistance to promote oncogenic transformation and metastasis in bone sarcoma (119). The activity of GFs is important for changes in the ECM skeleton, which is capable of culminating in anoikis attenuation, uncontrolled growth, metastasis, and relapse in some cases. Melosky discussed the treatment strategies for EGFR mutations in non-small cell lung cancer (NSCLC) and highlighted the existing EGFR mutations with an emphasis to first, second, and third lines of treatment plans in NSCLC (120). Although this study only focused on EGFR mutations and therapies in NSCLC, future studies can explore a similar approach for EGFR mutant cancers or other GFs driving oncogenic transformations to improve the current therapies (Figure 2). Other authors had reviewed the role of GFs and their receptors in mediating cancer (121–123). Therefore, the evaluation and targeting of aberrantly expressed growth proteins interacting with oncogenic markers may improve understanding of the impact of GFs on anoikis resistance and cancer metastasis.
Long Non-coding RNA
Long non-coding RNA (lncRNA) is composed of more than 200 nucleotides with no capability of being translated into proteins and is classified based on their location, mechanism of action, and the effects on DNA sequences. LncRNAs play diverse roles in epigenetic modification, protein synthesis, RNA transport, molecular signal regulation, among others, and have been implicated to be involved in anoikis resistance of cancer cells stemming toward metastatic phenotypes (124–126).
The antisense non-coding RNA in the INK4 locus (ANRIL) expression was found to be positively correlated with glioma grade in the tissues examined by Dai et al., and silencing of ANRIL in U251 cell lines induced anoikis and impaired cell proliferation (22). Similarly, metastasis-associated lung adenocarcinoma transcript 1 (MALAT1) was identified to be highly expressed in ovarian cancer cells, thereby promoting EMT, anchorage independence growth, and metastasis (61). The blockade of MALAT1 sensitized the cells to anoikis and inhibited the invasive potential (61).
Several lncRNAs were identified to be abnormally expressed in bladder cancer (23). However, LINC00958 and LINC01296 were upregulated, and their expression was observed to be correlated with anoikis-resistance fostering oncogenic signals, which were incumbent to the progression of bladder cancer (23). Although there are a myriad of lncRNAs (127), of which a few are associated with anoikis, it is, therefore, pertinent to explore how other aberrantly expressed lncRNAs which are yet to be identified in various cancers could contribute to anoikis or its resistance, thus furthering strategies to target cancer metastasis.
Cancer Stemness and Autophagy
Cancer stem cells exhibit characteristics of both stem cell and cancer cell. In addition to self-renewal and differentiation capacities of stem cells, CSCs can become tumors when transplanted into an animal model. The stem cells from MDA-MB-453 and MDA-MB-231 were reported to be anoikis resistant while non-stem bearing cells were sensitive to anoikis (128). Coculturing of stem-like cells with non-stem-like cells conferred anoikis resistance on non-stem-like cells (128). A different study reported that an amplified expression of CD44+/CD24− and aldehyde dehydrogenase 1 (ALDH1) in breast cancer cell line conferred stemness; which induced anoikis resistance through an increase in the phosphorylation of signal transducers and activators of transcription 3 (STAT3). The treatment with salinomycin, a selective agent, for targeting CSCs significantly reduced CD44+/CD24−, ALDH1 activity and STAT3 expression thus sensitized the cells to anoikis (62). Some studies reported the involvement of EMT in stemness of various cancers (129, 130), thereby facilitating aggressive tumor proliferation, anoikis resistance, and metastasis. Nevertheless, a genomic study reported three classes of CSCs: (i) glia CSCs with a high proliferation potential but repressed EMT markers; (ii) breast CSCs with high EMT signatures but less proliferative potential; and (iii) ovarian, prostate, and colon CSCs inhibited proliferative functions and varied EMT markers (131). Conversely, an experimental study reported the upregulation of MDA-9/syntenin in glioma stem cells fostered defensive autophagy and anoikis resistance (63). The blockade of MDA-9 promoted cell death through a reduced activation of the Bcl-2 and EGFR signaling (63). A recent study reported that low chaperone-mediated autophagy (CMA) enhanced the renewal of embryonic stem (ES) cell; however, CMA is upregulated at the onset of ES differentiation. The increase in CMA lowered intracellular α-ketoglutarate (α-KG) as CMA targeted isocitrate dehydrogenase 1/2 (enzymes decarboxylating isocitrate to yield α-KG) for lysosomal degradation, which in turn reduced the pluripotency and stemness of ES (64).
Despite the available literature highlighting the contribution of CSCs to tumorigenesis, a lot remains to be demystified. Future studies could consider understanding how various autophagy processes impact metabolism, thus mediating cancer stemness, anoikis resistance, and metastasis to improve the current therapy and overall survival of patients with cancer.
Signaling Pathways as Drivers of Anoikis Resistance
The survival of all living organisms from unicellular to multicellular organism anchors on a cascade of signaling processes that involve the storage of energy and renewal of building blocks for cellular needs. Cancer cells are capable of altering metabolism based on the availability of nutrients and transcriptional factors, the level of free radicals or antioxidants, and activities of immune cells, thereby favoring energy needed for proliferation, anoikis resistance, and metastasis. We have discussed below some of the signaling pathways driving metabolic changes in cancer cells as targets for anoikis resistance among other factors (Figures 1, 2). An overview of the signaling pathways contributing to anoikis resistance is summarized in Table 1.
Phosphoinositide-3-Kinase/Akt
The Phosphoinositide-3-kinase/Akt pathway is a crucial intracellular signaling pathway involved in cell proliferation and survival, migration, immune, and metabolic processes (132). Anomaly in this pathway is involved in the onset and progression of diverse diseases, including cancer, diabetes, neurological, and immunological disorders (133). PI3K is a family of enzyme that catalyzes the phosphorylation of phosphoinositide; its products can bind to other signaling kinases such as Akt and phosphoinositide-dependent protein kinase-1 to activate survival pathways (Figure 2).
Akt belongs to AMP/GMP kinases and protein kinase C-related family, and it can inhibit the activities of pro-apoptotic mediators, Bad, pro-caspase-9, and Fas-ligand. The target of rapamycin (TOR) is a central protein to life, and its mammal prototype called mTOR is an important regulator of protein synthesis and ribosome biogenesis. The upstream regulators of TOR include available nutrients, GFs, and their receptors to facilitate protein synthesis and other downstream processes necessary for life (134, 135). However, cancer cells exhibit an upsurge in this signaling pathway as it avails the signaling of oncogenic intermediates essential for continuous proliferation (135). Notably, phosphatase-tensin (PTEN), a negative regulator of the PI3K/Akt/mTOR pathway, acts as a tumor suppressor that inhibits growth and enhances cell sensitivity to anoikis (Figure 2) (65).
The elevated activity of PI3K/Akt is considered as a marker of cancer invasiveness (136). Similarly, activation of this pathway positively correlated with anoikis resistance in osteosarcoma (66, 67). A study reported activation of the PI3K/Akt pathway and the RAF/MEK/ERK pathway through caveolin 1 induced overexpression of IGF1R, thus promoting anchorage independence of hepatocellular carcinoma (HCC) (68). Also, PI3K/Akt activation through the downregulation of miR-133a-3p in prostate cancer was reported to promote anoikis resistance and bone metastasis (69). In addition, Akt signaling induced by stem cell-derived IL-8 promoted anchorage independence and pulmonary metastasis of osteosarcoma (70).
A cross talk exists between PI3K/Akt signaling and the MAPK/ERK signaling to promote cell survival, proliferation, and migration (137). The MAPK/ERK pathway, also known as the Ras-Raf-MEK-ERK pathway, plays a critical role in the initiation of cascades of events and conveys extracellular signals to intracellular targets (138). Anoikis-resistant ECs increased expression of RAS/ERK and PI3k/Akt, which facilitated ECM remodeling and invasiveness. Targeting either pathways impaired the proliferation, invasiveness, and sensitizated cells to anoikis (139). Similarly, upregulation of the MAPK/ERK pathway was reported in ECM-detached hepatoma although blockade of either ERK or PI3K/Akt had no significant effect in the sensitization of tumor cells to anoikis (71). On the contrary, a combined inhibition of both PI3K/Akt and ERK pathways sensitized the hepatoma to anoikis (71). The overexpression of Syndecan-2 in AIG melanoma cells was also reported to activate the PI3K/Akt and ERK signaling, hence promoting tumor invasiveness (41). This indicates some of the roles ECM components play in altering signaling pathways to promote anchorage independence and cancer cell invasive ability to circumvent anoikis. Mason et al. reported that oncogenic Ras enhanced the survival of ECM-detached cells with the aid of ATP generation in a PI3K and serum and glucocorticoid-regulated kinase-1 (SGK-1) dependent, but Akt independent, manner (26). The oncogenic Ras signaling promoted anoikis resistance by inhibiting the PH domain and leucine-rich repeat-containing protein phosphatase 1 (PHLPP1), which promotes anoikis through the activation of p38 MAPK. Despite the available literature on the role of PI3K in cancer, both at the basic research level and the clinical level (140), future studies on how anchorage-independence triggers its activation in various cancers are needed for better therapeutic strategies.
Adenosine Monophosphate-Activated Protein Kinase
Adenosine monophosphate-activated protein kinase (AMPK) is a crucial cellular ATP and a nutrient sensor for regulating glucose, lipid, and cholesterol metabolic pathways to ensure the maintenance of energy homeostasis and nutrient availability as prompted by the level of AMP-ATP in the tissues (Figure 2) (141). An increased level of AMP allosterically activates AMPK, whereas an increased ATP level deactivates AMPK. AMPK can also be activated via phosphorylation by liver kinase B1 (LKB1) (Figure 2), calcium/calmodulin-dependent protein kinase 2, and TGF-β-activated kinase 1 (TAK1) (142).
Adenosine monophosphate-activated protein kinase is considered neither an ally nor an adversary in the progression of cancer (143), and the upregulation of PI3K/Akt pathway with concomitant inhibition of PTEN in most cancers leads to LKB1 impairment in the activation of AMPK for its tumor suppression role (144). Anoikis resistance was reported in LKB1-deficient lung cancer with an elevated glutamate dehydrogenase 1 (GDH1) that is associated with an increased α-KG, leading to CamKK2-mediated AMPK activation and the repression of mTOR activity (72). Other studies reported AMPK activation in cells following detachment from the ECM, thus facilitating anoikis resistance, and subsequent inhibition of AMPK restored the sensitivity to anoikis (73–75).
The detachment of cells from the ECM in breast cancer triggered activation of AMPK with concomitant repression of Akt phosphorylation, which enabled cell survival, leading to anoikis resistance and cell migration (76). However, once the cells were reattached, there was a decrease in the expression of AMPK that was mediated by Akt upregulation. This suggests a possible feedback loop between AMPK activity and Akt activity in the regulation of anoikis resistance and tumor metastatic potential capable of being explored in various cancers (Figure 2). On the contrary, AMPK activation using metformin in cholangiocarcinoma cell lines sensitized cells to anoikis via the inhibition of nuclear translocation, NF-κB and STAT3 (77). Hence, there is a need for additional studies to elucidate the role of AMPK in anoikis resistance and metastasis, especially for tumors with an aberrant AMPK basal expression, which might be a biomarker for diagnosis and guide in the choice of a targeted therapy.
Hypoxia-Inducible Factors
Hypoxia-inducible factors are a family of proteins that are involved in response to oxygen unavailability (hypoxia) within the cell (145). Glioblastoma cultured in a poly hydroxyethyl methacrylate pre-coated dish presented with cell–cell detachment, spheroid formation, an upregulated HIF activity, a low ROS, and considerable resistance to anoikis (46). Silencing either HIF1α or HIF2α subjected HIF1α-targeted cells to anoikis but with no such effect recorded in HIF2α-targeted cells, thus elucidating the role of HIF1α in anoikis resistance (46).
The regulation of hypoxia-induced ANGPTL4 by HIF-1 enabled gastric cancer cell lines to achieve anoikis resistance and favor tumor growth (78). A study on HIF1 was reported to promote anoikis resistance through inhibition of integrinα5 in gastric cancer, and silencing of HIF1 sensitized the cells to anchorage dependence, impaired spheroid formation, increased the expression of integrinα5, and induced anoikis (79). HIF-1 was reported to be required by the oncogene ERBB2 for the dissemination of mammary gland tumors, anoikis resistance, and metastasis (80). The inhibition of HIF-1 in ERBB2 cells impaired ERK activity and Akt activity via the induction of pro-anoikis protein Bim (80). Taken together, the implication of the HIF family in promoting cancer development and anoikis resistance cannot be overemphasized as a result of the hypoxic environment. Nevertheless, studies and therapies targeted at manipulating the oxygen level in tumors may be an alternate path to potentiate death signals to overcome cancer proliferation and increase anoikis sensitivity.
Signal Transducers and Activators of Transcription
A family of STAT includes STAT1, STAT2, STAT3, STAT4, STAT5a, STAT5b, and STAT6, which are located in the cytoplasm and are activated by cytokines (GFs, interferon, and interleukin), hormones, and ligands for their signal transduction in the nucleus (146, 147). In addition to their transcriptional function, anomalies of the STAT family had been established to increase susceptibility to various diseases and their progression (148). STAT5a was reported to play a significant role in the normal morphology of mouse prostate, and STAT5a null mice had epithelial cell deformation that elucidate the involvement of STAT5a in normal prostate formation (149).
Targeting STAT4 through siRNA intervention intensified anoikis in ovarian cancer, thereby impaired cell migration and invasion potential (81). The authors demonstrated that the overexpression of STAT4 heightened metastasis and reduced survival of tumor-bearing mice through the stimulation of cancer-associated fibroblasts (CAFs) from normal fibroblasts triggered by Wnt7a signaling (81).
STAT3 expression was reported to be upregulated in anoikis-resistant human pancreatic cancer cells (82). Silencing of STAT3 activity propelled anoikis; however, the overexpression of IL-6 and STAT3 enhanced anoikis resistance and protected the cells from piplartine treatment. Piplartine-pretreated cells failed to initiate tumor formation and metastasis, when injected subcutaneously or intravenously respectively, whereas non-treated anoikis-resistant cells had a high STAT3 expression and developed lung metastasis (82). The loss of Erbin, a ErbB2 (v-erb-b2 avian erythroblastic leukemia viral oncogene homolog 2) interacting protein activity in cervical cancer, was reported to upsurge STAT3 phosphorylation, thus facilitating anoikis resistance and tumor invasiveness in vitro and in vivo (83). Also, silencing MMP-2 in glioma cell lines impaired α5, β1 integrin, IL-6 signaling, and STAT3 phosphorylation, thus hindering the proliferation and growth of glioma (84). IL-6 stimulation of STAT3 was impaired as a result of salinomycin treatment on triple-negative breast cancer (TNBC) cells cultured in suspension, which inhibited stemness, MMP-9, and MMP-2 expression, leading to anoikis (62). The role of STAT family invariably in altering the ECM architecture is imperative to facilitating anoikis resistance and cancer progression (Figure 2). Future studies could elaborate on STAT family activities to proffer more effective strategies for cancer treatment.
Rho GTPase
Rho GTPase belongs to the guanosine-5′-triphosphate (GTP) -binding protein family, a subfamily of the Ras superfamily that regulates diverse cellular activities, such as transcriptional processes, membrane trafficking, cell adhesion, cytoskeleton organization, and cell growth (150, 151). Guanine nucleotide exchange factors (GEF) and GTPase-activating proteins (GAP) are regulators of the Rho GTPase family (152). Rho GTPases are signaling proteins that regulate the catalysis and facilitate hydrolysis of GTP to GDP (guanosine diphosphate) and are active or inactive when bound to GTP or GDP respectively. Rho GTPases bind effector proteins [such as Rho-associated kinases 1 and 2, Rho-associated protein kinases (ROCKs) 1 and 2] and play a crucial role in reorganizing the actin cytoskeleton to promote cell migration (150, 153). Rac1, a Rho GTPase, was reported to enhance the transcription of estrogen receptor-alpha (ERα) in breast cancer. Targeting Rac1 pharmacologically inhibited estrogen-induced cell proliferation and attenuated the ERα transcriptional activity (85). Roc-A, a phytochemical, was reported to restrict cancer cell migration by inhibiting Rho GTPases, namely RhoA, Rac1, and Cdc42 activity, thereby changed cell morphology (86). Another study showed that the overexpression of ROCK 2 in colon carcinoma cells which were inoculated into immunocompromised mice altered the epithelial morphology and promoted tumor cell invasiveness into the surrounding tissues (154). Also, targeting ROCK 1 and ROCK 2 blocked medulloblastoma proliferation through the impairment of EMT, TGF-β, and TNFα via NFkβ signaling (87), which suggests that blockade of ROCK signaling could be a target to impair anoikis resistance and metastasis. Although the role of Rho GTPase in cancer was reviewed (150, 155), future studies are required to understand how targeting Rho GTPase can lead to the development of novel therapeutic agents for anoikis-resistant and metastasizing tumor cells.
Targeting Metabolic Pathways to Ameliorate Tumor Metastases
The detachment of cells from the ECM is dependent on metabolic alterations (156), and metabolism describes all reactions involving the conversions and interrelationships of various biomolecules to achieve a balanced redox state, thereby supplying energy to support cell survival. The consequences of normal metabolism are healthy growth and development, while abnormal metabolism underlies diseases, including cancer. Reprogrammed metabolism is a characteristic of cancer cells for enhancing cell proliferation, migration, and invasion. Cancer cells can combine the normal pathway of glucose oxidation to yield pyruvate and employ aerobic glycolysis, whereby there is an increase in glucose uptake for lactate production, thus known as the Warburg effect (157). The glucose oxidation via either paths makes tremendous intermediates and ATP available for the synthesis of various biomolecules, which are used by cancer cells for their magnificent meshwork to minimize ROS production and to suppress host immunity, thus combating anoikis signals from detaching cells (158, 159). In addition to glucose, other biomolecules of significant importance for cell growth and anoikis resistance include proteins, lipids, and nucleotides (Figure 2). In this section, we discuss evidence from studies on the anoikis-resistance mechanism in relation to metabolic perturbation, an emerging regulatory path for targeting anoikis that remains to be fully explored (refer Table 1).
Glucose Metabolism
The oxidation of glucose molecule to pyruvate occurring in the cytosol commences with the phosphorylation of glucose to glucose-6-phosphate (G6P) using hexokinase (Figure 2). This G6P is further converted into pyruvate in multi-catalyzed reaction steps to yield two molecules of ATP. This stepwise oxidation of glucose to pyruvate is termed glycolysis. Under extreme exercise conditions and in specific tissues (such as muscles, the brain, and the liver) with a limited oxygen supply, pyruvate can be rapidly converted to lactate by lactate dehydrogenase to meet the energy needs (160). On the other hand, oxidative phosphorylation (OXPHOS) generates about 36 ATPs per molecule of G6P. While the G6P-lactate pathway only generates about two molecules of ATP, it occurs spontaneously and efficiently, thus enabling both paths accumulate enough ATP needed for cancer cell growth and proliferation (161). The G6P-lactate reactions account for a good proportion of ATP used by cancer cells for their proliferative machinery, the Warburg effect. This enables cancer cells to circumvent production of ROS from the OXPHOS path, which is detrimental to the process of dysregulation of diverse signaling pathways culminating in acidosis of the tumor environment, uncontrolled cell growth, anoikis resistance, migration, and metastasis (42, 162). Highly invasive TNBC variant was demonstrated to exhibit metabolic perturbation as a result of utilizing glucose through the glycolytic path for its energy requirement in comparison to its less invasive variant; targeting these variants with 2-deoxy-glucose impaired glycolysis and sensitized more aggressive cell lines to anoikis (88).
Glycolysis-impaired cancer cells still survive, proliferate, and metastasize; cyclic adenosine protein kinase A (PKA) was identified to confer anoikis resistance in cancer cells in the presence of glucose starvation (89). The hyperactivation of PKA in anoikis-resistant cells stimulated autophagy and glutamine metabolism as alternate pathways for the energy needed through the abrogation of endoplasmic reticulum stress. The blockade of PKA activity impaired cancer cell survival and sensitized the cells to anoikis (89).
In addition to glycolysis, pentose phosphate pathway (PPP) is another pathway for glucose metabolism, called the hexose monophosphate shunt or the phosphogluconate pathway, which also takes place in the cytosol (Figure 2) with few similar intermediates to glycolysis, such as fructose-6-phosphate (F6P) and glyceraldehyde-3-phosphate (G3P) (163). PPP reaction is in two phases: first is the irreversible oxidative phase in which G6P is oxidatively decarboxylated to ribulose-5-phosphate (Ru5P) and nicotinamide adenine dinucleotide phosphate (NADPH); and the second is the reversible non-oxidative phase through which the Ru5P can be isomerized into ribose-5-phosphate (R5P), which either serves as a nucleotide precursor or is metabolized to yield F6P and G3P following the concerted enzymatic steps (164). Active division of cells require PPP to meet ribose-phosphate needs for DNA synthesis. Considering an intense metabolic requirement in cancer cells, PPP is a requisite for the proliferation of cancer cells to combat oxidative stress and to generate metabolites for various biosynthetic pathways (165).
Reduced nicotinamide adenine dinucleotide phosphate (NADPH), is an electron donor for cellular reactions such as nucleic acid, fatty acid, and steroids synthesis (166). The NADP+/NADPH ratio regulates glucose-6-phosphate dehydrogenase (G6PDH) activity in the cell. Cancer cells with a high proliferative activity have additional requirements for NADPH, glutathione (to mop free radicals from glutathione peroxidase activities), and G6PDH to supply DNA needs of pentose phosphates (164, 167), which highlights the importance of considering PPP intermediates as a potential target that could sensitize cancer cells to anoikis in future studies.
The growth of MDA-MB-231 cells in anchorage-independent conditions had increased ATP generation through fatty acid oxidation (FAO); it was observed that the glucose present in the cell was used to fuel PPP for redox homeostasis. The enhanced PPP activity subsequently fostered anoikis resistance in these cells (90). The treatment of MDA-MB-231 cells with a synthesized flavonoid (GL-V9) led to enhanced AMPK-activated lipid oxidation, ROS generation, limited PPP, and an increased sensitivity to anoikis (90). However, Mason et al. reported serum and glucocorticoid kinase-1 (SGK-1) role in energy availability for ECM detached cells, they elucidated it promoted increased glucose uptake via upregulated GLUT1 expression and PPP for increased ATP generation thus enabling anoikis resistance and survival (91).
Overall, the dysregulated glucose metabolism predisposes cancer cells to adopt resistance to anoikis and metastatic phenotypes (Figures 1, 2). Future studies could give considerable attention to elucidate glucose metabolic changes in various cancers for better therapeutic choices to improve the outcome of patients.
Protein Metabolism
Similar to other biomolecules, protein is turned over in anabolism and catabolism to meet the carbon and nitrogen needs of the cell via its amino acid building blocks. These processes provide intermediates for several metabolic pathways to enhance cell growth, including cancer proliferation. The coiled-coil domain-containing protein 178 (CCDC178) is an 867 amino acid polypeptide that is mutated in several human cancers including gastric cancer (168) and HCC (169). CCDC178 was reported to be overexpressed in HCC and promoted metastasis by mediating anoikis resistance via activation of the ERK/MAPK signaling pathway (24). However, the depletion of CCDC178 inhibited ERK activation, which underlies the role of CCDC178 as a protein oncogene involved in anoikis resistance and cancer metastasis; therefore, identifying the potential of targeting this polypeptide in cancer treatment.
Recently, Kodama et al. reported an increased expression of c-Myc from the growth of cells in anchorage-independent conditions (92). The elevated expression of c-Myc correlated with the induction of phosphoribosyl pyrophosphate amidotransferase (PPAT), an enzyme responsible for the transfer of γ-nitrogen from glutamine to 5-phosphoribosyl pyrophosphate (PRPP), a key rate-limiting enzyme in the nucleotide biosynthetic pathway. A meta-analysis of most human cancers (considered by these authors) revealed a high ratio of PPAT/Glutaminase 1 (GLS1), which correlated with cancer prognosis. The depletion in PPAT suppressed growth in cancer cell lines cultured in 2D, 3D, and tumor progression in vivo, while its overexpression conferred malignancy (92). However, the overexpression of GLS1 impaired AIG, whereas its depletion, as reported in another study, did not affect tumor growth (170). These studies highlighted a shift in the glutamine metabolism that is capable of modulating AIG, anoikis resistance, and malignancy. Also, Weber discussed the changes in cancer metabolism at various stages of cancer progression (171). The author highlighted that an increase in glutamate metabolism in ECM-detached cells generated glycine and creatine necessary for de novo purine synthesis and ATP (171).
In glioblastoma, glutaminolysis was found to occur via the upregulation of glutamate dehydrogenase 1 (GDH1) (25). GDH1 catalyzes the conversion of glutamate into α-KG, thereby promoting the anaplerotic entry of glutamine intermediates to the TCA cycle (172). The knockdown of GDH1 impaired AIG by decreasing colony formation, cell proliferation, and tumor growth in vivo (25). Mechanistically, it was identified that EGFR phosphorylates ELK1 (a transcriptional activator in response to MEK/ERK pathway) to enhance GDH1 transcription and glutamine metabolism (25). Exploring the dynamics of glutamine metabolism in various cancers could be a promising approach to hamper the transformations fueling AIG, anoikis resistance, and metastasis.
Altogether, there is a need for future studies to elucidate how regulating protein metabolism impacts anoikis or its resistance.
Fatty Acid Metabolism
Lipids are heterogeneous groups of biomolecules, which include fats, waxes, phospholipids, glycolipids, amino lipids, acyl lipids, and derived lipids. They are essential parts of diets, vitamins, hormones, cell membranes, and transport vesicles (173, 174). Lipids supply energy to the cells in a fasting state, supply essential nutrients for growth, provide thermal insulation outside the organs, coordinate different systems, provide a structural barrier support for cell membrane, and are involved in the transduction of signals (175, 176).
Acetyl-CoA is a central molecule for cellular metabolism, it is an intermediate from various biochemical processes (Figure 2), and acts as the building block for the biosynthesis of fatty acids and cholesterol and the end product of their oxidation (177). The synthesis of fatty acid is carried out in the cytosol to yield palmitate. Acetyl-CoA is carboxylated to malonyl-CoA in the presence of ATP, biotin, and bicarbonate, while acetyl CoA carboxylase, the rate-limiting enzyme, catalyzes this reaction (Figure 2). This step is followed by multi-catalyzed reactions via fatty acid synthase (FASN) complex with NADPH providing reducing equivalents for the production of palmitate which can be further esterified to yield complex lipids (178). In addition to the synthesis of de novo fatty acid, the requirement of cellular lipid can be achieved through exogenous fatty acid and amino acid uptake (179, 180).
Fatty acid oxidation, on the other hand, takes place in the mitochondria, and carnitine palmitoyltransferase 1 (CPT1) catalyzes the committed step of FAO. The cyclic cleavage of fatty acid from the carboxyl end of the chain is catalyzed by a fatty acid oxidase. Acetyl-CoA, the end product of FAO, enters the TCA cycle and the electron transport chain generate about 106 ATP and other metabolic intermediates per molecule of oxidized palmitate (177, 181).
Anomalies in lipid metabolism are associated with various diseases, including cancer (177, 182). Elevated FASN protein was found in the biopsy tissues of osteosarcoma patients presenting with lung metastasis in comparison with healthy individuals (183). It was reported that an increased expression of FASN mediated anoikis resistance and metastasis (94).
Some studies had highlighted FAO as a promoter of anoikis resistance and cancer progression (184, 185). Wang et al. reported the activation of FAO in colorectal cancer cells with an increased expression of CPT1, which correlated with anoikis resistance and metastasis in vitro and in vivo (95). Primary and metastatic tissues obtained from patients showed an elevated CPT1 in metastatic sites in comparison to the primary site corroborating the contribution of FAO to metastasis (95).
Similarly, breast cancer-expressing high Aldo-keto reductase family 1 member B10 (AKR1B10high) was reported to prefer FAO over glycolysis by utilizing exogenous fatty acids, thus favoring AIG and lung metastasis (96). However, the blockade of FAO in the AKR1B10high cells impaired AIG and metastasis (96). Also, the utilization of FAO by TNBC enhanced metastatic relapse via depletion of cytoplasmic lipid droplets mediated by CUB-domain-containing protein 1 (97). Another study elucidated that accumulated lipid droplets enhanced anoikis resistance and invasiveness of cancer cells (43). Mechanistically, TGF-β2 signaling enabled fatty acid uptake for the storage of neutral lipids or catabolism of lipids to meet cellular ATP needs (Figure 2) (43).
Overall cancer cells utilization of lipid for cellular metabolism is mediated by factors such as; the presence of exogenous fatty acids (96), hormones signaling (186), adipocytes (187, 188), lipid droplets (97, 189), fatty acid receptors-transporters (190), energy status and intermediates from various metabolic paths (191). These factors determine the metabolic path activated or repressed based on cellular needs. Therefore, a holistic consideration of these factors is imperative for the exploration of lipid metabolism to enhance anoikis and improve the survival of patients with metastatic cancer.
Nucleotide Metabolism
Nucleotides are biomolecules composed of nucleic acids as their building blocks, which are required for DNA and RNA synthesis. They are the major components of many coenzymes, and serve as the donors of phosphoryl groups found in some sugars or lipids. Nitrogen needs of the purine and pyrimidine synthesis are obtained from glutamine (Figure 2). Glutamine required for cellular growth can be either synthesized endogenously or obtained from the diet. Synthetic purine and pyrimidine analogs have been used as chemotherapeutic agents for cancer and other diseases (192). A recent study showed that high expression of glutamine synthase, an enzyme involved in the synthesis of glutamine, supports nucleotide metabolism, thereby leading to cancer cell survival (193). However, the role of nucleotide metabolism in mediating anoikis resistance is yet to be fully deciphered.
Rho-specific guanine nucleotide exchange factor (Rgnef) activated downstream of integrin in a complex with FAK, controls the migration of normal and tumor cells (194). In advanced-stage colon cancer patients, elevated expression, of Rgnef promoted tumor progression through its interaction with FAK (98, 99). Also, proteogenomic characterization of high-grade serous ovarian cancer cells revealed that the activation of RhoA pathway enhanced tumor metastasis and correlated with an impairment in the patient's survival (100). Recently, increased Rgnef expression was reported in the late-stage serous ovarian cancer, which was linked to tumor progression and a decrease in patient's survival (101). The deletion of Rgnef decreased the primary and metastatic tumor burden, elevated cellular ROS, inhibited AIG, thus reducing spheroid formation, and promoted anoikis (101). It is important to state that small molecules blocking oncogenic Rho signaling are in the early phase of development (195) and could be a promising approach for future studies aimed at regulating nucleotide-enhancing tumor metastasis.
Perspective on Therapy to Target Anoikis Resistance
Since anoikis resistance is not required for survival by healthy cells, this occurrence is an attribute of metastatic cancer cells and, therefore, denotes a possible therapeutic target. However, the comprehensive mechanisms involved in anoikis resistance are not completely understood, and there are currently no anoikis resistance-targeting drugs available. We have discussed some mediators of anoikis resistance and how studies targeted the mediators to promote sensitivity to anoikis and hindered metastasis. Early diagnosis is important to identify and characterize specific anoikis-resistance markers, thereby targeting the same to improve anoikis and to prevent metastasis. Hypoxia and low pH are some of the major attributes of tumor milieu, enhancing anoikis evasion and therapy resistance. Several studies have shown that the therapeutic potential of small molecules (such as salinomycin) induced ROS production in cancer cells to inhibit anoikis resistance, invasiveness, migration, and metastasis (62, 90, 196–198). This suggested that unexplored drugs, which demonstrate their pharmacological targets by inducing oxidative stress capable of restricting AIG in cancer cells without affecting normal cells, may be considered for future therapies. In addition, an acidic environment is critical for the proliferation of solid tumors and has been reported to reprogram the metabolic landscape of the tumor microenvironment, thus promoting resistance to anoikis and metastasis (42). To circumvent the acidic microenvironment, the administration of bicarbonate for reducing cellular acidosis could be combined with other available therapies for the treatment of patients with metastatic cancer (111). Some pharmacological agents (such as metformin, piplartine, and 2-deoxy-d-glucose) that promote anoikis through the regulation of oncogenic or metabolic signaling pathways were reported, but the drugs are not specific for targeting anoikis-resistant cells.
Discussion and Perspectives
Despite the available literature on the potential mediators of anoikis resistance preceding tumor metastasis, metabolic processes remain an emerging area of research that is currently less explored to enhance anoikis. UDP-glucose ceramide glucosyltransferase (UGCG), a key enzyme in glycosphingolipid metabolism, is overexpressed in metastatic breast cancer cells and augmented glutamine uptake for cellular energy metabolism (199). An increased expression of GLS enhanced oxidative stress response and glutamine oxidation to provide the required energy for cell proliferation (199). Metastatic breast cancer-overexpressing UGCG indicated that glutamine could be a precursor of other amino acid syntheses, such as aspartate and proline (199). An elevated proline biosynthesis contributed to tumor progression (200). Similarly, it was shown that Myc-dependent proline synthesis derived from glutamine mediated glucose oxidation via an increased extracellular acidification rate (ECAR) in human B lymphoma (P493) cells (200).
Another study reported that asparagine bioavailability correlated with the metastatic potential of patients with breast cancer (93). Genetic ablation of asparagine synthetase (ASNS), treatment with L-asparaginase, or restriction of dietary asparagine decreased metastasis without altering growth in the primary site whereas overexpression of ASNS or increased dietary asparagine reversed this effect. Similarly, the alteration in asparagine availability affected the invasiveness of cancer cells, which was associated with the regulation of EMT proteins (93). Thus, targeting asparagine metabolism could be a promising approach to prevent metastatic cancer cells and promote anoikis. Branched-chain amino acids (BCAAs), especially valine, leucine, and isoleucine, are essential amino acids obtained from the diet. Cancer cells have an enhanced ability for BCAA uptake (201). Also, BCAA aminotransferases (BCATs), the enzymes catalyzing the first step in the catabolic pathway of BCAAs, are overexpressed in cancer cells (202, 203). It was demonstrated that highly malignant pancreatic ductal adenocarcinoma (PDAC) cells relied on BCAA for its survival and proliferation, as well as a carbon source, to enhance lipogenesis in the cells (204). Genetic blockade of BCAT2 suppresses PDAC cell proliferation in vitro and in vivo. Considering these findings, exploring the regulation of BCAA catabolic pathways may enhance anoikis and improve the therapy for metastatic cancer cells.
Dietary methionine, an essential amino acid in one-carbon metabolism, has been reported to alter tissue metabolism (205, 206), and its depletion or restriction has been proposed to have an anticancer effect (207, 208). More recently, dietary methionine restriction altered methionine and sulfur metabolism in murine colorectal cancer via enhanced sensitivity to chemo- and radiotherapy (209). The restriction of methionine from the diet of healthy individuals had a profound impact on methionine levels and systemic metabolism. The methionine restriction affected metabolites of methylation, TCA cycle, amino acid, and nucleotide metabolism (210). These suggest regulating methionine dietary intake or systemic level in anoikis resistant or metastasizing tumor cells could be a promising approach to promote anoikis.
The upregulation of guanosine nucleotide was reported in a subtype of small-cell lung cancer (SCLC) (210). The tumor from this SCLC subtype expressed an increased guanosine biosynthetic enzyme known as inosine monophosphate dehydrogenase 1 and 2 (IMPDH1 and IMPDH2). Also, this subtype overexpressed Myc, which is a transcriptional target of IMPDH (211, 212), and targeting IMPDH drastically reduced tumor growth (210). In addition, Valvezan et al. identified that IMPDH inhibitor selectively targeted the cell and tumor models of tuberous sclerosis cells (TSCs) in an mTORC1-dependent manner (213). It was observed that mTORC1 promoted anabolic cell growth and proliferation via the regulation of ribosome biogenesis and nucleotide synthesis to sustain the anabolic balance, which indicated an increased dependence on the nucleotide synthesis in cells having an activated mTORC1. We suggest that the IMPDH inhibitors could be repurposed for the treatment of anoikis-resistant or metastatic cancer cells-overexpressing Myc. Phosphoribosyl-pyrophosphate synthetase 2 (PRPS2), a rate-limiting enzyme, in the purine synthesis was identified to promote the elevation of nucleotide biosynthesis in Myc-transformed cells (214). PRPS2 connected the nucleotide and protein biosynthesis through a specific cis-regulatory element within the PRPS2 5′ UTR. The strategies to block the PRPS2 expression or interfere with an Myc-dependent translational control hindered the availability of PPP and nucleotide intermediates, which led to a reduced nucleotide production and consequently slowed tumor initiation and proliferation without affecting the normal cell survival (214). The Myc oncogene remains undruggable but PRPS2 as an enzyme is considered a constituent of a druggable genome (215). Therefore, the drugs specifically targeting PRPS2 or other enzymes involved in nucleotide biosynthesis may be important for metabolic reprogramming in anoikis-evading cancer cells.
Gene expression is closely regulated at various levels, which includes transcription, posttranscription, translation, and posttranslation. Recently, a key nucleotide component, N6-Methyladenosine (m6A), emerged as a new layer of regulatory mechanism, which modulates gene expression in living organisms (216). Some studies showed the existence of m6A on several types of RNA [such as mRNA, rRNA, miRNA, lncRNA, circular RNA (circRNA), and small nuclear RNA (snRNA)], which was constantly regulated during normal development and disease states (inclusive of cancer) (216–218). The dysregulation in the m6A modification was reported in cancer pathogenesis and its response or resistance to drug treatment (219–221). Considering the critical role of RNA modification in cancer, there is a need for better understanding of the underlying mechanisms of these modifications and exploring such potential therapeutic targets to circumvent anoikis resistance.
Concluding Remarks
A few studies had shown several factors that confer the survival of cancer cells after detachment of cells from the ECM. These factors enable cancer cells to evade anoikis, thus supporting the formation of metastasis in secondary sites. Recent evidence revealed that aberrations in the ECM (5) are mediated by specific upregulated metabolic genes or intermediates, and transcriptional signaling pathways that drive acidic environment, EMT, and oncogenic processes (Figures 1, 2). Importantly, metabolism reprogramming, a hallmark of cancer, is critical to ECM detachment; however, our knowledge from literature is limited on the impact of manipulating a single metabolic pathway or the overall metabolic pathways in metastasis. A holistic approach of maintaining ECM stability to facilitate pro-apoptotic pathways for the eradication of detached cells may be relevant for enabling anoikis and achieving a successful therapy. Hence, a better understanding of the metabolic changes enhancing anoikis resistance could unravel novel approaches to target metastasis, the leading cause of cancer-related deaths.
Author Contributions
FA and GZ conceived the idea. FA wrote and revised the manuscript. GZ and XW reviewed and supervised the writing process. AA contributed significantly to the writing and figures design. LA and DY gave helpful suggestions throughout the writing. All authors contributed to the article and approved the submitted version.
Funding
This study was supported by the National Key R&D Program of China (2019YFA0906100), the Shenzhen Basic Science Research Project (JCYJ20190807164001762), the Special Funds for Major Science and Technology of Guangdong Province (2019B020201014), and a Nanshan pilot team project (LHTD20160004).
Conflict of Interest
XW is a member of the advisory board of the BinDeBioTech Co., Ltd.
The remaining authors declare that the research was conducted in the absence of any commercial or financial relationships that could be construed as a potential conflict of interest.
Acknowledgments
FA was sponsored by the Chinese Government Scholarship Council and the University of Chinese Academy of Sciences (UCAS) for international students.
References
1. Frantz C, Stewart KM, Weaver VM. The extracellular matrix at a glance. J Cell Sci. (2010) 123:4195–200. doi: 10.1242/jcs.023820
2. Kusindarta DL, Wihadmadyatami H. The role of extracellular matrix in tissue regeneration. In: Abdel hay El-Sayed Kaoud H., editor. Tissue Regeneration. London: Intechopen Limited. (2018). p. 65.
3. Ruggero Pardi MD. Signal transduction by adhesion receptors. In: Neitzel J, Rasband M., editor. Scitable. Cambridge, MA: Nature Education (2010). 3:38
4. Iozzo RV, Gubbiotti MA. Extracellular matrix: the driving force of mammalian diseases. Matrix Biol. (2018) 71–2:1–9. doi: 10.1016/j.matbio.2018.03.023
5. Sonbol HS. Extracellular matrix remodeling in human disease. J Microscopy Ultrastruct. (2018) 6:123–8. doi: 10.4103/JMAU.JMAU_4_18
6. Wu Y-S, Chen S-N. Apoptotic cell: linkage of inflammation and wound healing. Front Pharmacol. (2014) 5:1. doi: 10.3389/fphar.2014.00001
7. Green DR, Llambi F. Cell death signaling. Cold Spring Harb Perspect Biol. (2015) 7:a006080. doi: 10.1101/cshperspect.a006080
8. Galluzzi L, Vitale I, Aaronson SA, Abrams JM, Adam D, Agostinis P, et al. Molecular mechanisms of cell death: recommendations of the nomenclature committee on cell death 2018. Cell Death Different. (2018) 25:486–541. doi: 10.1038/s41418-017-0012-4
9. Yan G, Elbadawi M, Efferth T. Multiple cell death modalities and their key features (review). World Acad Sci J. (2020) 2:39–48. doi: 10.3892/wasj.2020.40
10. Tait SWG, Ichim G, Green DR. Die another way – non-apoptotic mechanisms of cell death. J Cell Sci. (2014) 127:2135–44. doi: 10.1242/jcs.093575
11. Paoli P, Giannoni E, Chiarugi P. Anoikis molecular pathways and its role in cancer progression. Biochim Biophys Acta. (2013) 1833:3481–98. doi: 10.1016/j.bbamcr.2013.06.026
12. Kim Y-N, Koo KH, Sung JY, Yun U-J, Kim H. Anoikis resistance: an essential prerequisite for tumor metastasis. Int J Cell Biol. (2012) 2012:306879. doi: 10.1155/2012/306879
13. Taddei M, Giannoni E, Fiaschi T, Chiarugi P. Anoikis: an emerging hallmark in health and diseases. J Pathol. (2012) 226:380–93. doi: 10.1002/path.3000
14. Dobler D, Ahmed N, Song L, Eboigbodin KE, Thornalley PJ. Increased dicarbonyl metabolism in endothelial cells in hyperglycemia induces anoikis and impairs angiogenesis by RGD and GFOGER motif modification. Diabetes. (2006) 55:1961–9. doi: 10.2337/db05-1634
15. Ortiz-Muñoz G, Houard X, Martín-Ventura J-L, Ishida BY, Loyau S, Rossignol P, et al. HDL antielastase activity prevents smooth muscle cell anoikis, a potential new antiatherogenic property. FASEB J. (2009) 23:3129–39. doi: 10.1096/fj.08-127928
16. Elmore S. Apoptosis: a review of programmed cell death. Toxicol Pathol. (2007) 35:495–516. doi: 10.1080/01926230701320337
17. Bray F, Ferlay J, Soerjomataram I, Siegel RL, Torre LA, Jemal A. Global cancer statistics 2018: GLOBOCAN estimates of incidence and mortality worldwide for 36 cancers in 185 countries. CA Cancer J Clin. (2018) 68:394–424. doi: 10.3322/caac.21492
18. Guan X. Cancer metastases: challenges and opportunities. Acta Pharm Sin B. (2015) 5:402–18. doi: 10.1016/j.apsb.2015.07.005
19. Wang C, Shao L, Pan C, Ye J, Ding Z, Wu J, et al. Elevated level of mitochondrial reactive oxygen species via fatty acid β-oxidation in cancer stem cells promotes cancer metastasis by inducing epithelial–mesenchymal transition. Stem Cell Res Ther. (2019) 10:175. doi: 10.1186/s13287-019-1265-2
20. Vander Linden C, Corbet C. Therapeutic targeting of cancer stem cells: integrating and exploiting the acidic niche. Front Oncol. (2019) 9:159. doi: 10.3389/fonc.2019.00159
21. Hu P, Li S, Tian N, Wu F, Hu Y, Li D, et al. Acidosis enhances the self-renewal and mitochondrial respiration of stem cell-like glioma cells through CYP24A1-mediated reduction of vitamin D. Cell Death Dis. (2019) 10:25. doi: 10.1038/s41419-018-1242-1
22. Dai W, Tian C, Jin S. Effect of lncRNA ANRIL silencing on anoikis and cell cycle in human glioma via microRNA-203a. OncoTargets Ther. (2018) 11:5103–9. doi: 10.2147/OTT.S169809
23. Seitz AK, Christensen LL, Christensen E, Faarkrog K, Ostenfeld MS, Hedegaard J, et al. Profiling of long non-coding RNAs identifies LINC00958 and LINC01296 as candidate oncogenes in bladder cancer. Sci Rep. (2017) 7:395. doi: 10.1038/s41598-017-00327-0
24. Hu X, Zhao Y, Wei L, Zhu B, Song D, Wang J, et al. CCDC178 promotes hepatocellular carcinoma metastasis through modulation of anoikis. Oncogene. (2017) 36:4047–59. doi: 10.1038/onc.2017.10
25. Yang R, Li X, Wu Y, Zhang G, Liu X, Li Y, et al. EGFR activates GDH1 transcription to promote glutamine metabolism through MEK/ERK/ELK1 pathway in glioblastoma. Oncogene. (2020) 39:1–12. doi: 10.1038/s41388-020-1199-2
26. Mason JA, Davison-Versagli CA, Leliaert AK, Pape DJ, McCallister C, Zuo J, et al. Oncogenic Ras differentially regulates metabolism and anoikis in extracellular matrix-detached cells. Cell Death Different. (2016) 23:1271–82. doi: 10.1038/cdd.2016.15
27. Coleman ML, Sahai EA, Yeo M, Bosch M, Dewar A, Olson MF. Membrane blebbing during apoptosis results from caspase-mediated activation of ROCK I. Nat Cell Biol. (2001) 3:339–45. doi: 10.1038/35070009
28. Núñez R, Sancho-Martínez SM, Novoa JML, López-Hernández FJ. Apoptotic volume decrease as a geometric determinant for cell dismantling into apoptotic bodies. Cell Death Different. (2010) 17:1665–71. doi: 10.1038/cdd.2010.96
29. Ernest NJ, Habela CW, Sontheimer H. Cytoplasmic condensation is both necessary and sufficient to induce apoptotic cell death. J Cell Sci. (2008) 121:290. doi: 10.1242/jcs.017343
30. Martinvalet D, Zhu P, Lieberman J. Granzyme A induces caspase-independent mitochondrial damage, a required first step for apoptosis. Immunity. (2005) 22:355–70. doi: 10.1016/j.immuni.2005.02.004
31. Buzza MS, Zamurs L, Sun J, Bird CH, Smith AI, Trapani JA, et al. Extracellular matrix remodeling by human granzyme B via cleavage of vitronectin, fibronectin, and laminin. J Biol Chem. (2005) 280:23549–58. doi: 10.1074/jbc.M412001200
32. Ola MS, Nawaz M, Ahsan H. Role of Bcl-2 family proteins and caspases in the regulation of apoptosis. Mol Cell Biochem. (2011) 351:41–58. doi: 10.1007/s11010-010-0709-x
33. Wajant H. The Fas signaling pathway: more than a paradigm. Science. (2002) 296:1635–6. doi: 10.1126/science.1071553
34. Guadamillas MC, Cerezo A, del Pozo MA. Overcoming anoikis – pathways to anchorage-independent growth in cancer. J Cell Sci. (2011) 124:3189–97. doi: 10.1242/jcs.072165
35. Zhu G-J, Song P-P, Zhou H, Shen X-H, Wang J-G, Ma X-F, et al. Role of epithelial-mesenchymal transition markers E-cadherin, N-cadherin, β-catenin and ZEB2 in laryngeal squamous cell carcinoma. Oncol Lett. (2018) 15:3472–81. doi: 10.3892/ol.2018.7751
36. Desgrosellier JS, Barnes LA, Shields DJ, Huang M, Lau SK, Prévost N, et al. An integrin αvβ3–c-Src oncogenic unit promotes anchorage-independence and tumor progression. Nat Med. (2009) 15:1163–9. doi: 10.1038/nm.2009
37. Wang D, Müller S, Amin AR, Huang D, Su L, Hu Z, et al. The pivotal role of integrin β1 in metastasis of head and neck squamous cell carcinoma. Clin Cancer Res. (2012) 18:4589–99. doi: 10.1158/1078-0432.CCR-11-3127
38. Ojalill M, Parikainen M, Rappu P, Aalto E, Jokinen J, Virtanen N, et al. Integrin α2β1 decelerates proliferation, but promotes survival and invasion of prostate cancer cells. Oncotarget. (2018) 9:32435–47. doi: 10.18632/oncotarget.25945
39. Kang S-A, Blache CA, Bajana S, Hasan N, Kamal M, Morita Y, et al. The effect of soluble E-selectin on tumor progression and metastasis. BMC Cancer. (2016) 16:331. doi: 10.1186/s12885-016-2391-1
40. Yadav A, Kumar B, Yu J-G, Old M, Teknos TN, Kumar P. Tumor-associated endothelial cells promote tumor metastasis by chaperoning circulating tumor cells and protecting them from anoikis. PLoS ONE. (2015) 10:e0141602. doi: 10.1371/journal.pone.0141602
41. Tseng T, Uen W, Tseng J, Lee S. Enhanced chemosensitization of anoikis-resistant melanoma cells through syndecan-2 upregulation upon anchorage independency. Oncotarget. (2017) 8:61528–37. doi: 10.18632/oncotarget.18616
42. Peppicelli S, Ruzzolini J, Bianchini F, Andreucci E, Nediani C, Laurenzana A, et al. Anoikis resistance as a further trait of acidic-adapted melanoma cells. J Oncol. (2019) 2019:8340926. doi: 10.1155/2019/8340926
43. Corbet C, Bastien E, Santiago de Jesus JP, Dierge E, Martherus R, Vander Linden C, et al. TGFβ2-induced formation of lipid droplets supports acidosis-driven EMT and the metastatic spreading of cancer cells. Nat Commun. (2020) 11:454. doi: 10.1038/s41467-019-14262-3
44. Du S, Miao J, Zhu Z, Xu E, Shi L, Ai S, et al. NADPH oxidase 4 regulates anoikis resistance of gastric cancer cells through the generation of reactive oxygen species and the induction of EGFR. Cell Death Dis. (2018) 9:948. doi: 10.1038/s41419-018-0953-7
45. Kim H, Sung JY, Park E-K, Kho S, Koo KH, Park S-Y, et al. Regulation of anoikis resistance by NADPH oxidase 4 and epidermal growth factor receptor. Br J Cancer. (2017) 116:370–81. doi: 10.1038/bjc.2016.440
46. Maurer GD, Brucker DP, Steinbach JP. Loss of cell-matrix contact increases hypoxia-inducible factor-dependent transcriptional activity in glioma cells. Biochem Biophys Res Commun. (2019) 515:77–84. doi: 10.1016/j.bbrc.2019.05.115
47. Kamarajugadda S, Cai Q, Chen H, Nayak S, Zhu J, He M, et al. Manganese superoxide dismutase promotes anoikis resistance and tumor metastasis. Cell Death Dis. (2013) 4:e504. doi: 10.1038/cddis.2013.20
48. Li S, Mao Y, Zhou T, Luo C, Xie J, Qi W, et al. Manganese superoxide dismutase mediates anoikis resistance and tumor metastasis in nasopharyngeal carcinoma. Oncotarget. (2016) 7:32408–20. doi: 10.18632/oncotarget.8717
49. Long W, Yi P, Amazit L, LaMarca HL, Ashcroft F, Kumar R, et al. SRC-3Delta4 mediates the interaction of EGFR with FAK to promote cell migration. Mol Cell. (2010) 37:321–32. doi: 10.1016/j.molcel.2010.01.004
50. Luey BC, May FEB. Insulin-like growth factors are essential to prevent anoikis in oestrogen-responsive breast cancer cells: importance of the type I IGF receptor and PI3-kinase/Akt pathway. Mol Cancer. (2016) 15:8. doi: 10.1186/s12943-015-0482-2
51. Sher I, Adham SA, Petrik J, Coomber BL. Autocrine VEGF-A/KDR loop protects epithelial ovarian carcinoma cells from anoikis. Int J Cancer. (2009) 124:553–61. doi: 10.1002/ijc.23963
52. Tiong KH, Tan BS, Choo HL, Chung FF.-L, Hii L.-W, Tan SH, et al. Fibroblast growth factor receptor 4 (FGFR4) and fibroblast growth factor 19 (FGF19) autocrine enhance breast cancer cells survival. Oncotarget. (2016) 7:57633–50. doi: 10.18632/oncotarget.9328
53. Li T, Guo T, Liu H, Jiang H, Wang Y. Platelet-derived growth factor-BB mediates pancreatic cancer malignancy via regulation of the Hippo/Yes-associated protein signaling pathway. Oncol Rep. (2021) 45:83–94. doi: 10.3892/or.2020.7859
54. Yuan Y, Ye HQ, Ren QC. Proliferative role of BDNF/TrkB signaling is associated with anoikis resistance in cervical cancer. Oncol Rep. (2018) 40:621–34. doi: 10.3892/or.2018.6515
55. Bao W, Qiu H, Yang T, Luo X, Zhang H, Wan X. Upregulation of TrkB promotes epithelial-mesenchymal transition and anoikis resistance in endometrial carcinoma. PLOS ONE. (2013) 8:e70616. doi: 10.1371/journal.pone.0070616
56. Zhang P, Xing Z, Li X, Song Y, Zhao J, Xiao Y, et al. Tyrosine receptor kinase B silencing inhibits anoikis-resistance and improves anticancer efficiency of sorafenib in human renal cancer cells. Int J Oncol. (2016) 48:1417–25. doi: 10.3892/ijo.2016.3356
57. Reginato MJ, Mills KR, Paulus JK, Lynch DK, Sgroi DC, Debnath J, et al. Integrins and EGFR coordinately regulate the pro-apoptotic protein Bim to prevent anoikis. Nat Cell Biol. (2003) 5:733–40. doi: 10.1038/ncb1026
58. Chen F, Zhang L, Wu J, Huo F, Ren X, Zheng J, et al. HCRP-1 regulates EGFR–AKT–BIM-mediated anoikis resistance and serves as a prognostic marker in human colon cancer. Cell Death Dis. (2018) 9:1176. doi: 10.1038/s41419-018-1217-2
59. Corkery DP, Clarke LE, Gebremeskel S, Salsman J, Pinder J, Le Page C, et al. Loss of PRP4K drives anoikis resistance in part by dysregulation of epidermal growth factor receptor endosomal trafficking. Oncogene. (2018) 37:174–84. doi: 10.1038/onc.2017.318
60. Liao YH, Chiang KH, Shieh JM, Huang CR, Shen CJ, Huang WC, et al. Epidermal growth factor-induced ANGPTL4 enhances anoikis resistance and tumour metastasis in head and neck squamous cell carcinoma. Oncogene. (2017) 36:2228–42. doi: 10.1038/onc.2016.371
61. Gordon MA, Babbs B, Cochrane DR, Bitler BG, Richer JK. The long non-coding RNA MALAT1 promotes ovarian cancer progression by regulating RBFOX2-mediated alternative splicing. Mol Carcinog. (2019) 58:196–205. doi: 10.1002/mc.22919
62. An H, Kim JY, Oh E, Lee N, Cho Y, Seo JH. Salinomycin promotes anoikis and decreases the CD44+/CD24- stem-like population via inhibition of STAT3 activation in MDA-MB-231 cells. PLoS ONE. (2015) 10:e0141919. doi: 10.1371/journal.pone.0141919
63. Talukdar S, Pradhan AK, Bhoopathi P, Shen X-N, August LA, Windle JJ, et al. MDA-9/Syntenin regulates protective autophagy in anoikis-resistant glioma stem cells. Proc Natl Acad Sci USA. (2018) 115:5768–73. doi: 10.1073/pnas.1721650115
64. Xu Y, Zhang Y, García-Cañaveras JC, Guo L, Kan M, Yu S, et al. Chaperone-mediated autophagy regulates the pluripotency of embryonic stem cells. Science. (2020) 369:397–403. doi: 10.1126/science.abb4467
65. Lu Y, Lin Y.-Z, LaPushin R, Cuevas B, Fang X, Yu SX, et al. The PTEN/MMAC1/TEP tumor suppressor gene decreases cell growth and induces apoptosis and anoikis in breast cancer cells. Oncogene. (1999) 18:7034–45. doi: 10.1038/sj.onc.1203183
66. Zhao G-S, Zhang Q, Cao Y, Wang Y Lv.-F, Zhang Z.-S, et al. High expression of ID1 facilitates metastasis in human osteosarcoma by regulating the sensitivity of anoikis via PI3K/AKT depended suppression of the intrinsic apoptotic signaling pathway. Am J Transl Res. (2019) 11:2117–39.
67. Díaz-Montero CM, Wygant JN, McIntyre BW. PI3-K/Akt-mediated anoikis resistance of human osteosarcoma cells requires Src activation. Eur J Cancer. (2006) 42:1491–500. doi: 10.1016/j.ejca.2006.03.007
68. Tang W, Feng X, Zhang S, Ren Z, Liu Y, Yang B, et al. Caveolin-1 confers resistance of hepatoma cells to anoikis by activating IGF-1 pathway. Cell Physiol Biochem. (2015) 36:1223–36. doi: 10.1159/000430292
69. Tang Y, Pan J, Huang S, Peng X, Zou X, Luo Y, et al. Downregulation of miR-133a-3p promotes prostate cancer bone metastasis via activating PI3K/AKT signaling. J Exp Clin Cancer Res. (2018) 37:160. doi: 10.1186/s13046-018-0813-4
70. Du L, Han X.-g, Tu B, Wang M.-q, Qiao H, Zhang S.-h, et al. CXCR1/Akt signaling activation induced by mesenchymal stem cell-derived IL-8 promotes osteosarcoma cell anoikis resistance and pulmonary metastasis. Cell Death Dis. (2018) 9:714. doi: 10.1038/s41419-018-0745-0
71. Cao L, Zhang Z, Han L, Du J, Liang X, Liu Y, et al. Mitogen-activated protein kinase pathway is pivotal for anoikis resistance in metastatic hepatoma cells. Mol Med Rep. (2014) 9:1121–7. doi: 10.3892/mmr.2014.1952
72. Jin L, Chun J, Pan C, Kumar A, Zhang G, Ha Y, et al. The PLAG1-GDH1 axis promotes anoikis resistance and tumor metastasis through CamKK2-AMPK signaling in LKB1-deficient lung cancer. Mol Cell. (2018) 69:87–99.e87. doi: 10.1016/j.molcel.2017.11.025
73. Guo P, Qiu Y, Ma X, Li T, Ma X, Zhu L, et al. Tripartite motif 31 promotes resistance to anoikis of hepatocarcinoma cells through regulation of p53-AMPK axis. Exp Cell Res. (2018) 368:59–66. doi: 10.1016/j.yexcr.2018.04.013
74. Ng TL, Leprivier G, Robertson MD, Chow C, Martin MJ, Laderoute KR, et al. The AMPK stress response pathway mediates anoikis resistance through inhibition of mTOR and suppression of protein synthesis. Cell Death Different. (2012) 19:501–10. doi: 10.1038/cdd.2011.119
75. Hindupur SK, Balaji SA, Saxena M, Pandey S, Sravan GS, Heda N, et al. Identification of a novel AMPK-PEA15 axis in the anoikis-resistant growth of mammary cells. Breast Cancer Res. (2014) 16:420. doi: 10.1186/s13058-014-0420-z
76. Saha M, Kumar S, Bukhari S, Balaji SA, Kumar P, Hindupur SK, et al. AMPK-Akt double-negative feedback loop in breast cancer cells regulates their adaptation to matrix deprivation. Cancer Res. (2018) 78:1497–510. doi: 10.1158/0008-5472.CAN-17-2090
77. Saengboonmee C, Seubwai W, Cha'on U, Sawanyawisuth K, Wongkham S, Wongkham C. Metformin exerts antiproliferative and anti-metastatic effects against cholangiocarcinoma cells by targeting STAT3 and NF-κB. Anticancer Res. (2017) 37:115–23. doi: 10.21873/anticanres.11296
78. Baba K, Kitajima Y, Miyake S, Nakamura J, Wakiyama K, Sato H, et al. Hypoxia-induced ANGPTL4 sustains tumour growth and anoikis resistance through different mechanisms in scirrhous gastric cancer cell lines. Sci Rep. (2017) 7:11127. doi: 10.1038/s41598-017-11769-x
79. Rohwer N, Welzel M, Daskalow K, Pfander D, Wiedenmann B, Detjen K, et al. Hypoxia-inducible factor 1α mediates anoikis resistance via suppression of α5 integrin. Cancer Res. (2008) 68:10113–20. doi: 10.1158/0008-5472.CAN-08-1839
80. Whelan KA, Schwab LP, Karakashev SV, Franchetti L, Johannes GJ, Seagroves TN, et al. The oncogene HER2/neu (ERBB2) requires the hypoxia-inducible factor HIF-1 for mammary tumor growth and anoikis resistance. J Biol Chem. (2013) 288:15865–77. doi: 10.1074/jbc.M112.426999
81. Zhao L, Ji G, Le X, Luo Z, Wang C, Feng M, et al. An integrated analysis identifies STAT4 as a key regulator of ovarian cancer metastasis. Oncogene. (2017) 36:3384–96. doi: 10.1038/onc.2016.487
82. Fofaria NM, Srivastava SK. STAT3 induces anoikis resistance, promotes cell invasion and metastatic potential in pancreatic cancer cells. Carcinogenesis. (2014) 36:142–50. doi: 10.1093/carcin/bgu233
83. Hu Y, Chen H, Duan C, Liu D, Qian L, Yang Z, et al. Deficiency of Erbin induces resistance of cervical cancer cells to anoikis in a STAT3-dependent manner. Oncogenesis. (2013) 2:e52. doi: 10.1038/oncsis.2013.18
84. Kesanakurti D, Chetty C, Dinh DH, Gujrati M, Rao JS. Role of MMP-2 in the regulation of IL-6/Stat3 survival signaling via interaction with α5β1 integrin in glioma. Oncogene. (2013) 32:327–40. doi: 10.1038/onc.2012.52
85. Rosenblatt AE, Garcia MI, Lyons L, Xie Y, Maiorino C, Désiré L, et al. Inhibition of the Rho GTPase, Rac1, decreases estrogen receptor levels and is a novel therapeutic strategy in breast cancer. Endocr Relat Cancer. (2011) 18:207. doi: 10.1677/ERC-10-0049
86. Becker MS, Müller PM, Bajorat J, Schroeder A, Giaisi M, Amin E, et al. The anticancer phytochemical rocaglamide inhibits Rho GTPase activity and cancer cell migration. Oncotarget. (2016) 7:51908–21. doi: 10.18632/oncotarget.10188
87. Dyberg C, Andonova T, Olsen TK, Brodin B, Kool M, Kogner P, et al. Inhibition of Rho-associated kinase suppresses medulloblastoma growth. Cancers. (2020) 12:73. doi: 10.3390/cancers12010073
88. O'Neill S, Porter RK, McNamee N, Martinez VG, O'Driscoll L. 2-Deoxy-D-glucose inhibits aggressive triple-negative breast cancer cells by targeting glycolysis and the cancer stem cell phenotype. Sci Rep. (2019) 9:3788. doi: 10.1038/s41598-019-39789-9
89. Palorini R, Votta G, Pirola Y, De Vitto H, De Palma S, Airoldi C, et al. Protein kinase A activation promotes cancer cell resistance to glucose starvation and anoikis. PLoS Genet. (2016) 12:e1005931. doi: 10.1371/journal.pgen.1005931
90. Yang L, He Z, Yao J, Tan R, Zhu Y, Li Z, Guo Q, et al. Regulation of AMPK-related glycolipid metabolism imbalances redox homeostasis and inhibits anchorage independent growth in human breast cancer cells. Redox Biol. (2018) 17:180–91. doi: 10.1016/j.redox.2018.04.016
91. Mason JA, Cockfield JA, Pape DJ, Meissner H, Sokolowski M, White TC, et al. SGK1 signaling promotes glucose metabolism and survival in extracellular matrix detached cells. bioRxiv [Preprint]. (2020). doi: 10.2139/ssrn.3570572
92. Kodama M, Oshikawa K, Shimizu H, Yoshioka S, Takahashi M, Izumi Y, et al. A shift in glutamine nitrogen metabolism contributes to the malignant progression of cancer. Nat Commun. (2020) 11:1320. doi: 10.1038/s41467-020-15136-9
93. Knott SRV, Wagenblast E, Khan S, Kim SY, Soto M, Wagner M, et al. Asparagine bioavailability governs metastasis in a model of breast cancer. Nature. (2018) 554:378–81. doi: 10.1038/nature25465
94. Sun T, Zhong X, Song H, Liu J, Li J, Leung F, et al. Anoikis resistant mediated by FASN promoted growth and metastasis of osteosarcoma. Cell Death Dis. (2019) 10:298. doi: 10.1038/s41419-019-1532-2
95. Wang Y-n, Zeng Z-l, Lu J, Wang Y, Liu Z-x, He M-m, et al. CPT1A-mediated fatty acid oxidation promotes colorectal cancer cell metastasis by inhibiting anoikis. Oncogene. (2018) 37:6025–40. doi: 10.1038/s41388-018-0384-z
96. van Weverwijk A, Koundouros N, Iravani M, Ashenden M, Gao Q, Poulogiannis G, et al. Metabolic adaptability in metastatic breast cancer by AKR1B10-dependent balancing of glycolysis and fatty acid oxidation. Nat Commun. (2019) 10:2698. doi: 10.1038/s41467-019-10592-4
97. Wright HJ, Hou J, Xu B, Cortez M, Potma EO, Tromberg BJ, et al. CDCP1 drives triple-negative breast cancer metastasis through reduction of lipid-droplet abundance and stimulation of fatty acid oxidation. Proc Natl Acad Sci USA. (2017) 114:E6556–65. doi: 10.1073/pnas.1703791114
98. Yu HG, Nam JO, Miller NL, Tanjoni I, Walsh C, Shi L, et al. p190RhoGEF (Rgnef) promotes colon carcinoma tumor progression via interaction with focal adhesion kinase. Cancer Res. (2011) 71:360–70. doi: 10.1158/0008-5472.CAN-10-2894
99. Masià-Balagué M, Izquierdo I, Garrido G, Cordomí A, Pérez-Benito L, Miller NL, et al. Gastrin-stimulated Gα13 activation of Rgnef protein (ArhGEF28) in DLD-1 colon carcinoma cells. J Biol Chem. (2015) 290:15197–209. doi: 10.1074/jbc.M114.628164
100. Zhang H, Liu T, Zhang Z, Payne SH, Zhang B, McDermott JE, et al. Integrated proteogenomic characterization of human high-grade serous ovarian cancer. Cell. (2016) 166:755–65. doi: 10.1016/j.cell.2016.05.069
101. Kleinschmidt EG, Miller NLG, Ozmadenci D, Tancioni I, Osterman CD, Barrie AM, et al. Rgnef promotes ovarian tumor progression and confers protection from oxidative stress. Oncogene. (2019) 38:6323–37. doi: 10.1038/s41388-019-0881-8
102. Saranya J, Shilpa G, Raghu KG, Priya S. Morus alba leaf lectin (MLL) sensitizes MCF-7 cells to anoikis by inhibiting fibronectin mediated integrin-FAK signaling through Ras and activation of P38 MAPK. Front Pharmacol. (2017) 8:34. doi: 10.3389/fphar.2017.00034
103. Roland CL, Harken AH, Sarr MG, Barnett CC Jr. ICAM-1 expression determines malignant potential of cancer. Surgery. (2007) 141:705–7. doi: 10.1016/j.surg.2007.01.016
104. Woods A., Couchman JR. Syndecans: synergistic activator of cell adhesion. Trends Cell Biol. (1998) 8:189–92. doi: 10.1016/S0962-8924(98)01244-6
105. Carneiro BR, Pernambuco Filho PCA, Mesquita APdS, da Silva DS, Pinhal MAS, Nader HB, et al. Acquisition of anoikis resistance up-regulates syndecan-4 expression in endothelial cells. PLoS ONE. (2014) 9:e116001. doi: 10.1371/journal.pone.0116001
106. Elgundi Z, Papanicolaou M, Major G, Cox TR, Melrose J, Whitelock JM, et al. Cancer metastasis: the role of the extracellular matrix and the heparan sulfate proteoglycan perlecan. Front Oncol. (2020) 9:1482. doi: 10.3389/fonc.2019.01482
107. Onyeisi JOS, Castanho de Almeida Pernambuco Filho P, de Araujo Lopes S, Nader HB, Lopes CC. Heparan sulfate proteoglycans as trastuzumab targets in anoikis-resistant endothelial cells. J Cell Biochem. (2019) 120:13826–40. doi: 10.1002/jcb.28656
108. Zhong X, Rescorla FJ. Cell surface adhesion molecules and adhesion-initiated signaling: understanding of anoikis resistance mechanisms and therapeutic opportunities. Cell Signal. (2012) 24:393–401. doi: 10.1016/j.cellsig.2011.10.005
109. Aksorn N, Chanvorachote P. Integrin as a molecular target for anti-cancer approaches in lung cancer. Anticancer Res. (2019) 39:541–8. doi: 10.21873/anticanres.13146
110. Hagihara H, Catts VS, Katayama Y, Shoji H, Takagi T, Huang FL, et al. Decreased brain pH as a shared endophenotype of psychiatric disorders. Neuropsychopharmacology. (2018) 43:459–68. doi: 10.1038/npp.2017.167
111. Pilon-Thomas S, Kodumudi KN, El-Kenawi AE, Russell S, Weber AM, Luddy K, et al. Neutralization of tumor acidity improves antitumor responses to immunotherapy. Cancer Res. (2016) 76:1381–90. doi: 10.1158/0008-5472.CAN-15-1743
112. Weiner HL. The role of growth factor receptors in central nervous system development and neoplasia. Neurosurgery. (1995) 37:179–94. doi: 10.1097/00006123-199508000-00001
113. Yuan J, Yin Z, Tao K, Wang G, Gao J. Function of insulin-like growth factor 1 receptor in cancer resistance to chemotherapy (review). Oncol Lett. (2018) 15:41–7. doi: 10.3892/ol.2017.7276
114. Simpson A, Petnga W, Macaulay VM, Weyer-Czernilofsky U, Bogenrieder T. Insulin-like growth factor (IGF) pathway targeting in cancer: role of the IGF axis and opportunities for future combination studies. Targeted Oncol. (2017) 12:571–97. doi: 10.1007/s11523-017-0514-5
115. Miranda M, Morici JF, Zanoni MB, Bekinschtein P. Brain-derived neurotrophic factor: a key molecule for memory in the healthy and the pathological brain. Front Cell Neurosci. (2019) 13:363. doi: 10.3389/fncel.2019.00363
116. Taipale J, Keski-Oja J. Growth factors in the extracellular matrix. FASEB J. (1997) 11:51–9. doi: 10.1096/fasebj.11.1.9034166
117. Powers CJ, McLeskey SW, Wellstein A. Fibroblast growth factors, their receptors and signaling. Endocr Relat Cancer. (2000) 7:165–97. doi: 10.1677/erc.0.0070165
118. Shirakihara T, Horiguchi K, Miyazawa K, Ehata S, Shibata T, Morita I, et al. TGF-β regulates isoform switching of FGF receptors and epithelial-mesenchymal transition. EMBO J. (2011) 30:783–95. doi: 10.1038/emboj.2010.351
119. Zhu L, McManus MM, Hughes DP. Understanding the biology of bone sarcoma from early initiating events through late events in metastasis and disease progression. Front Oncol. (2013) 3:230. doi: 10.3389/fonc.2013.00230
120. Melosky B. Current treatment algorithms for patients with metastatic non-small cell, non-squamous lung cancer. Front Oncol. (2017) 7:38. doi: 10.3389/fonc.2017.00038
121. Hartmann S, Bhola NE, Grandis JR. HGF/met signaling in head and neck cancer: impact on the tumor microenvironment. Clin Cancer Res. (2016) 22:4005–13. doi: 10.1158/1078-0432.CCR-16-0951
122. Tiash S, Chowdhury EH. Growth factor receptors: promising drug targets in cancer. J Cancer Metastasis Treat. (2015) 1:190–200. doi: 10.4103/2394-4722.163151
123. Roane BM, Arend RC, Birrer MJ. Review: targeting the transforming growth factor-beta pathway in ovarian cancer. Cancers. (2019) 11:668. doi: 10.3390/cancers11050668
124. Kornienko AE, Guenzl PM, Barlow DP, Pauler FM. Gene regulation by the act of long non-coding RNA transcription. BMC Biol. (2013) 11:59. doi: 10.1186/1741-7007-11-59
125. Yuan J.-h, Yang F, Wang F, Ma J.-z, Guo Y.-j, Tao Q.-f, et al. A long noncoding RNA activated by TGF-β promotes the invasion-metastasis cascade in hepatocellular carcinoma. Cancer Cell. (2014) 25:666–81. doi: 10.1016/j.ccr.2014.03.010
126. Jiang M.-C, Ni J.-J, Cui W.-Y, Wang B.-Y, Zhuo W. Emerging roles of lncRNA in cancer and therapeutic opportunities. Am J Cancer Res. (2019) 9:1354–66.
127. Fukunaga T, Iwakiri J, Ono Y, Hamada M. LncRRIsearch: a web server for lncRNA-RNA interaction prediction integrated with tissue-specific expression and subcellular localization data. Front Genet. (2019) 10:462. doi: 10.3389/fgene.2019.00462
128. Kim S.-Y, Hong S.-H, Basse PH, Wu C, Bartlett DL, Kwon YT, et al. Cancer stem cells protect non-stem cells from anoikis: bystander effects. J Cell Biochem. (2016) 117:2289–301. doi: 10.1002/jcb.25527
129. Mani SA, Guo W, Liao M-J, Eaton EN, Ayyanan A, Zhou AY, et al. The epithelial-mesenchymal transition generates cells with properties of stem cells. Cell. (2008) 133:704–15. doi: 10.1016/j.cell.2008.03.027
130. Yang L, Li N, Xue Z, Liu L.-R, Li J, Huang X, et al. Synergistic therapeutic effect of combined PDGFR and SGK1 inhibition in metastasis-initiating cells of breast cancer. Cell Death Different. (2020) 27:2066–80. doi: 10.1038/s41418-019-0485-4
131. Hsu C.-L, Chung F.-H, Chen C.-H, Hsu T.-T, Liu S.-M, Chung D.-S, et al. Genotypes of cancer stem cells characterized by epithelial-to-mesenchymal transition and proliferation related functions. Sci Rep. (2016) 6:32523. doi: 10.1038/srep32523
132. Xie Y, Shi X, Sheng K, Han G, Li W, Zhao Q, et al. PI3K/Akt signaling transduction pathway, erythropoiesis and glycolysis in hypoxia (review). Mol Med Rep. (2019) 19:783–91. doi: 10.3892/mmr.2018.9713
133. Fruman DA, Chiu H, Hopkins BD, Bagrodia S, Cantley LC, Abraham RT. The PI3K pathway in human disease. Cell. (2017) 170:605–35. doi: 10.1016/j.cell.2017.07.029
134. Gentilella A, Kozma SC, Thomas G. A liaison between mTOR signaling, ribosome biogenesis and cancer. Biochim Biophys Acta. (2015) 1849:812–20. doi: 10.1016/j.bbagrm.2015.02.005
135. Porta C, Paglino C, Mosca A. Targeting PI3K/Akt/mTOR signaling in cancer. Front Oncol. (2014) 4:64. doi: 10.3389/fonc.2014.00064
136. Vara JÁF, Casado E, de Castro J, Cejas P, Belda-Iniesta C, González-Barón M. PI3K/Akt signalling pathway and cancer. Cancer Treat Rev. (2004) 30:193–204. doi: 10.1016/j.ctrv.2003.07.007
137. Mendoza MC, Er EE, Blenis J. The Ras-ERK and PI3K-mTOR pathways: cross-talk and compensation. Trends Biochem Sci. (2011) 36:320–8. doi: 10.1016/j.tibs.2011.03.006
138. Guo YJ, Pan WW, Liu SB, Shen ZF, Xu Y, Hu LL. ERK/MAPK signalling pathway and tumorigenesis. Exp Ther Med. (2020) 19:1997–2007. doi: 10.3892/etm.2020.8454
139. de Sousa Mesquita AP, de Araújo Lopes S, Pernambuco Filho PCA, Nader HB, Lopes CC. Acquisition of anoikis resistance promotes alterations in the Ras/ERK and PI3K/Akt signaling pathways and matrix remodeling in endothelial cells. Apoptosis. (2017) 22:1116–37. doi: 10.1007/s10495-017-1392-0
140. Yang J, Nie J, Ma X, Wei Y, Peng Y, Wei X. Targeting PI3K in cancer: mechanisms and advances in clinical trials. Mol Cancer. (2019) 18:26. doi: 10.1186/s12943-019-0954-x
141. Hardie DG. AMP-activated protein kinase: an energy sensor that regulates all aspects of cell function. Genes Dev. (2011) 25:1895–908. doi: 10.1101/gad.17420111
142. Shackelford DB, Shaw RJ. The LKB1-AMPK pathway: metabolism and growth control in tumour suppression. Nat Rev Cancer. (2009) 9:563–75. doi: 10.1038/nrc2676
143. Vara-Ciruelos D, Russell FM, Hardie DG. The strange case of AMPK and cancer: Dr Jekyll or Mr Hyde? (†). Open Biol. (2019) 9:190099. doi: 10.1098/rsob.190099
144. Hawley Simon A, Ross Fiona A, Gowans Graeme J, Tibarewal P, Leslie Nicholas R, Hardie DG. Phosphorylation by Akt within the ST loop of AMPK-α1 down-regulates its activation in tumour cells. Biochem J. (2014) 459:275–87. doi: 10.1042/BJ20131344
145. Ziello JE, Jovin IS, Huang Y. Hypoxia-inducible factor (HIF)-1 regulatory pathway and its potential for therapeutic intervention in malignancy and ischemia. Yale J Biol Med. (2007) 80:51–60.
146. Haan S, Kortylewski M, Behrmann I, Müller-Esterl W, Heinrich PC, Schaper F. Cytoplasmic STAT proteins associate prior to activation. Biochem J. (2000) 345:417–21. doi: 10.1042/bj3450417
147. Lim CP, Cao X. Structure, function, and regulation of STAT proteins. Mol BioSyst. (2006) 2:536–50. doi: 10.1039/b606246f
148. Yang C, Mai H, Peng J, Zhou B, Hou J, Jiang D. STAT4: an immunoregulator contributing to diverse human diseases. Int J Biol Sci. (2020) 16:1575–85. doi: 10.7150/ijbs.41852
149. Nevalainen MT, Ahonen TJ, Yamashita H, Chandrashekar V, Bartke A, Grimley PM, et al. Epithelial defect in prostates of Stat5a-null mice. Lab Investig. (2000) 80:993–1006. doi: 10.1038/labinvest.3780105
150. Clayton NS, Ridley AJ. Targeting Rho GTPase signaling networks in cancer. Front Cell Dev Biol. (2020) 8:222. doi: 10.3389/fcell.2020.00222
151. Van Aelst L, D'Souza-Schorey C. Rho GTPases and signaling networks. Genes Dev. (1997) 11:2295–322. doi: 10.1101/gad.11.18.2295
153. Phuyal S, Farhan H. Multifaceted Rho GTPase signaling at the endomembranes. Front Cell Dev Biol. (2019) 7:127. doi: 10.3389/fcell.2019.00127
154. Croft DR, Sahai E, Mavria G, Li S, Tsai J, Lee WMF, et al. Conditional ROCK activation in vivo induces tumor cell dissemination and angiogenesis. Cancer Res. (2004) 64:8994–9001. doi: 10.1158/0008-5472.CAN-04-2052
155. Zubor P, Dankova Z, Kolkova Z, Holubekova V, Brany D, Mersakova S, et al. Rho GTPases in gynecologic cancers: in-depth analysis toward the paradigm change from reactive to predictive, preventive, and personalized medical approach benefiting the patient and healthcare. Cancers. (2020) 12:1292. doi: 10.3390/cancers12051292
156. Schafer ZT, Grassian AR, Song L, Jiang Z, Gerhart-Hines Z, Irie HY, et al. Antioxidant and oncogene rescue of metabolic defects caused by loss of matrix attachment. Nature. (2009) 461:109–13. doi: 10.1038/nature08268
157. Jose C, Bellance N, Rossignol R. Choosing between glycolysis and oxidative phosphorylation: a tumor's dilemma? Biochim Biophys Acta Bioenerget. (2011) 1807:552–61. doi: 10.1016/j.bbabio.2010.10.012
158. DeBerardinis RJ, Chandel NS. Fundamentals of cancer metabolism. Sci Adv. (2016) 2:e1600200. doi: 10.1126/sciadv.1600200
159. Davison CA, Durbin SM, Thau MR, Zellmer VR, Chapman SE, Diener J, et al. Antioxidant enzymes mediate survival of breast cancer cells deprived of extracellular matrix. Cancer Res. (2013) 73:3704–15. doi: 10.1158/0008-5472.CAN-12-2482
160. Rogatzki MJ, Ferguson BS, Goodwin ML, Gladden LB. Lactate is always the end product of glycolysis. Front Neurosci. (2015) 9:22. doi: 10.3389/fnins.2015.00022
161. Caneba CA, Bellance N, Yang L, Pabst L, Nagrath D. Pyruvate uptake is increased in highly invasive ovarian cancer cells under anoikis conditions for anaplerosis, mitochondrial function, and migration. Am J Physiol Endocrinol Metab. (2012) 303:E1036–52. doi: 10.1152/ajpendo.00151.2012
162. Cairns RA, Harris IS, Mak TW. Regulation of cancer cell metabolism. Nat Rev Cancer. (2011) 11:85–95. doi: 10.1038/nrc2981
163. Cho ES, Cha YH, Kim HS, Kim NH, Yook JI. The pentose phosphate pathway as a potential target for cancer therapy. Biomol Ther. (2018) 26:29–38. doi: 10.4062/biomolther.2017.179
164. Patra KC, Hay N. The pentose phosphate pathway and cancer. Trends Biochem Sci. (2014) 39:347–54. doi: 10.1016/j.tibs.2014.06.005
165. Rais B, Comin B, Puigjaner J, Brandes JL, Creppy E, Saboureau D, et al. Oxythiamine and dehydroepiandrosterone induce a G1 phase cycle arrest in Ehrlich's tumor cells through inhibition of the pentose cycle. FEBS Lett. (1999) 456:113–8. doi: 10.1016/S0014-5793(99)00924-2
166. Riganti C, Gazzano E, Polimeni M, Aldieri E, Ghigo D. The pentose phosphate pathway: an antioxidant defense and a crossroad in tumor cell fate. Free Radical Biol Med. (2012) 53:421–36. doi: 10.1016/j.freeradbiomed.2012.05.006
167. Zhelev Z, Aoki I, Gadjeva V, Nikolova B, Bakalova R, Saga T. Tissue redox activity as a sensing platform for imaging of cancer based on nitroxide redox cycle. Eur J Cancer. (2013) 49:1467–78. doi: 10.1016/j.ejca.2012.10.026
168. Zhang J, Huang J, Chen Y, Yuan F, Zhang H, Yan F, et al. Whole genome and transcriptome sequencing of matched primary and peritoneal metastatic gastric carcinoma. Sci Rep. (2015) 5:13750. doi: 10.1038/srep15309
169. Huang J, Deng Q, Wang Q, Li K.-Y, Dai J.-H, Li N, et al. Exome sequencing of hepatitis B virus–associated hepatocellular carcinoma. Nat Genet. (2012) 44:1117. doi: 10.1038/ng.2391
170. Davidson SM, Papagiannakopoulos T, Olenchock BA, Heyman JE, Keibler MA, Luengo A, et al. Environment impacts the metabolic dependencies of Ras-driven non-small cell lung cancer. Cell Metab. (2016) 23:517–28. doi: 10.1016/j.cmet.2016.01.007
171. Weber GF. Time and circumstances: cancer cell metabolism at various stages of disease progression. Front Oncol. (2016) 6:257. doi: 10.3389/fonc.2016.00257
172. Wang Y.-Q, Wang H.-L, Xu J, Tan J, Fu L.-N, Wang J.-L, et al. Sirtuin5 contributes to colorectal carcinogenesis by enhancing glutaminolysis in a deglutarylation-dependent manner. Nat Commun. (2018) 9:545. doi: 10.1038/s41467-018-02951-4
173. Pomorski T, Hrafnsdottir S, Devaux PF, van Meer G. Lipid distribution and transport across cellular membranes. Semin Cell Dev Biol. (2001) 12:139–48. doi: 10.1006/scdb.2000.0231
174. van Meer G, Voelker DR, Feigenson GW. Membrane lipids: where they are and how they behave. Nat Rev Mol Cell Biol. (2008) 9:112–24. doi: 10.1038/nrm2330
175. Efeyan A, Comb WC, Sabatini DM. Nutrient-sensing mechanisms and pathways. Nature. (2015) 517:302–10. doi: 10.1038/nature14190
176. Holthuis JC, Menon AK. Lipid landscapes and pipelines in membrane homeostasis. Nature. (2014) 510:48–57. doi: 10.1038/nature13474
177. Yan D, Adeshakin AO, Xu M, Afolabi LO, Zhang G, Chen YH, et al. Lipid metabolic pathways confer the immunosuppressive function of myeloid-derived suppressor cells in tumor. Front Immunol. (2019) 10:1399. doi: 10.3389/fimmu.2019.01399
178. Cheng C, Geng F, Cheng X, Guo D. Lipid metabolism reprogramming and its potential targets in cancer. Cancer Commun. (2018) 38:27. doi: 10.1186/s40880-018-0301-4
179. Hagberg CE, Falkevall A, Wang X, Larsson E, Huusko J, Nilsson I, et al. Vascular endothelial growth factor B controls endothelial fatty acid uptake. Nature. (2010) 464:917–21. doi: 10.1038/nature08945
180. Krall AS, Xu S, Graeber TG, Braas D, Christofk HR. Asparagine promotes cancer cell proliferation through use as an amino acid exchange factor. Nat Commun. (2016) 7:11457. doi: 10.1038/ncomms11457
181. Lochner M, Berod L, Sparwasser T. Fatty acid metabolism in the regulation of T cell function. Trends Immunol. (2015) 36:81–91. doi: 10.1016/j.it.2014.12.005
182. Rosen ED, Spiegelman BM. What we talk about when we talk about fat. Cell. (2014) 156:20–44. doi: 10.1016/j.cell.2013.12.012
183. Liu ZL, Wang G, Peng AF, Luo QF, Zhou Y, Huang SH. Fatty acid synthase expression in osteosarcoma and its correlation with pulmonary metastasis. Oncol Lett. (2012) 4:878–82. doi: 10.3892/ol.2012.862
184. Pacilli A, Calienni M, Margarucci S, D'Apolito M, Petillo O, Rocchi L, et al. Carnitine-acyltransferase system inhibition, cancer cell death, and prevention of Myc-induced lymphomagenesis. J Natl Cancer Inst. (2013) 105:489–98. doi: 10.1093/jnci/djt030
185. Carracedo A, Weiss D, Leliaert AK, Bhasin M, de Boer VC, Laurent G, et al. A metabolic prosurvival role for PML in breast cancer. J Clin Invest. (2012) 122:3088–100. doi: 10.1172/JCI62129
186. Stoykova GE, Schlaepfer IR. Lipid metabolism and endocrine resistance in prostate cancer, and new opportunities for therapy. Int J Mol Sci. (2019) 20:2626. doi: 10.3390/ijms20112626
187. Nieman KM, Romero IL, Van Houten B, Lengyel E. Adipose tissue and adipocytes support tumorigenesis and metastasis. Biochim Biophys Acta. (2013) 1831:1533–41. doi: 10.1016/j.bbalip.2013.02.010
188. Tan Y, Lin K, Zhao Y, Wu Q, Chen D, Wang J, et al. Adipocytes fuel gastric cancer omental metastasis via PITPNC1-mediated fatty acid metabolic reprogramming. Theranostics. (2018) 8:5452–68. doi: 10.7150/thno.28219
189. Cruz ALS, Barreto EdA, Fazolini NPB, Viola JPB, Bozza PT. Lipid droplets: platforms with multiple functions in cancer hallmarks. Cell Death Dis. (2020) 11:105. doi: 10.1038/s41419-020-2297-3
190. Pascual G, Avgustinova A, Mejetta S, Martín M, Castellanos A, Attolini CS-O, et al. Targeting metastasis-initiating cells through the fatty acid receptor CD36. Nature. (2017) 541:41–5. doi: 10.1038/nature20791
191. Lee H, Zandkarimi F, Zhang Y, Meena JK, Kim J, Zhuang L, et al. Energy-stress-mediated AMPK activation inhibits ferroptosis. Nat Cell Biol. (2020) 22:225–34. doi: 10.1038/s41556-020-0461-8
192. Lane AN, Fan TW. Regulation of mammalian nucleotide metabolism and biosynthesis. Nucl Acids Res. (2015) 43:2466–85. doi: 10.1093/nar/gkv047
193. Fu S, Li Z, Xiao L, Hu W, Zhang L, Xie B, et al. Glutamine synthetase promotes radiation resistance via facilitating nucleotide metabolism and subsequent DNA damage repair. Cell Rep. (2019) 28:1136–43.e1134. doi: 10.1016/j.celrep.2019.07.002
194. Miller NL, Kleinschmidt EG, Schlaepfer DD. RhoGEFs in cell motility: novel links between Rgnef and focal adhesion kinase. Curr Mol Med. (2014) 14:221–34. doi: 10.2174/1566524014666140128110339
195. Diviani D, Raimondi F, Del Vescovo CD, Dreyer E, Reggi E, Osman H, et al. Small-molecule protein-protein interaction inhibitor of oncogenic Rho signaling. Cell Chem Biol. (2016) 23:1135–46. doi: 10.1016/j.chembiol.2016.07.015
196. Hu B, Zhang T, An HM, Zheng JL, Yan X, Huang XW. Herbal formula YGJDSJ inhibits anchorage-independent growth and induces anoikis in hepatocellular carcinoma Bel-7402 cells. BMC Complement Altern Med. (2018) 18:17. doi: 10.1186/s12906-018-2083-2
197. Saleem MZ, Nisar MA, Alshwmi M, Din SRU, Gamallat Y, Khan M, et al. Brevilin A inhibits STAT3 signaling and induces ROS-dependent apoptosis, mitochondrial stress and endoplasmic reticulum stress in MCF-7 breast cancer cells. OncoTargets Ther. (2020) 13:435–50. doi: 10.2147/OTT.S228702
198. Yang Y, Zhang Y, Wang L, Lee S. Levistolide A induces apoptosis via ROS-mediated ER stress pathway in colon cancer cells. Cell Physiol Biochem. (2017) 42:929–38. doi: 10.1159/000478647
199. Schömel N, Hancock SE, Gruber L, Olzomer EM, Byrne FL, Shah D, et al. UGCG influences glutamine metabolism of breast cancer cells. Sci Rep. (2019) 9:15665. doi: 10.1038/s41598-019-52169-7
200. Liu W, Hancock CN, Fischer JW, Harman M, Phang JM. Proline biosynthesis augments tumor cell growth and aerobic glycolysis: involvement of pyridine nucleotides. Sci Rep. (2015) 5:17206. doi: 10.1038/srep17206
201. Hattori A, Tsunoda M, Konuma T, Kobayashi M, Nagy T, Glushka J, et al. Cancer progression by reprogrammed BCAA metabolism in myeloid leukaemia. Nature. (2017) 545:500–4. doi: 10.1038/nature22314
202. Mayers JR, Torrence ME, Danai LV, Papagiannakopoulos T, Davidson SM, Bauer MR, et al. Tissue of origin dictates branched-chain amino acid metabolism in mutant Kras-driven cancers. Science. (2016) 353:1161–5. doi: 10.1126/science.aaf5171
203. Tönjes M, Barbus S, Park YJ, Wang W, Schlotter M, Lindroth AM, et al. BCAT1 promotes cell proliferation through amino acid catabolism in gliomas carrying wild-type IDH1. Nat Med. (2013) 19:901–8. doi: 10.1038/nm.3217
204. Lee JH, Cho Y-R, Kim JH, Kim J, Nam HY, Kim SW, et al. Branched-chain amino acids sustain pancreatic cancer growth by regulating lipid metabolism. Exp Mol Med. (2019) 51:1–11. doi: 10.1038/s12276-019-0350-z
205. Mentch SJ, Mehrmohamadi M, Huang L, Liu X, Gupta D, Mattocks D, et al. Histone methylation dynamics and gene regulation occur through the sensing of one-carbon metabolism. Cell Metab. (2015) 22:861–73. doi: 10.1016/j.cmet.2015.08.024
206. Lees EK, Banks R, Cook C, Hill S, Morrice N, Grant L, et al. Direct comparison of methionine restriction with leucine restriction on the metabolic health of C57BL/6J mice. Sci Rep. (2017) 7:9977. doi: 10.1038/s41598-017-10381-3
207. Hens JR, Sinha I, Perodin F, Cooper T, Sinha R, Plummer J, et al. Methionine-restricted diet inhibits growth of MCF10AT1-derived mammary tumors by increasing cell cycle inhibitors in athymic nude mice. BMC Cancer. (2016) 16:349. doi: 10.1186/s12885-016-2367-1
208. Sharma B, Singh S, Kanwar SS. L-methionase: a therapeutic enzyme to treat malignancies. BioMed Res Int. (2014) 2014:506287. doi: 10.1155/2014/506287
209. Gao X, Sanderson SM, Dai Z, Reid MA, Cooper DE, Lu M, et al. Dietary methionine influences therapy in mouse cancer models and alters human metabolism. Nature. (2019) 572:397–401. doi: 10.1038/s41586-019-1437-3
210. Huang F, Ni M, Chalishazar MD, Huffman KE, Kim J, Cai L, et al. Inosine monophosphate dehydrogenase dependence in a subset of small cell lung cancers. Cell Metab. (2018) 28:369–82.e365. doi: 10.1016/j.cmet.2018.06.005
211. Liu YC, Li F, Handler J, Huang CR, Xiang Y, Neretti N, et al. Global regulation of nucleotide biosynthetic genes by c-Myc. PLoS ONE. (2008) 3:e2722. doi: 10.1371/journal.pone.0002722
212. Stine ZE, Walton ZE, Altman BJ, Hsieh AL, Dang CV. MYC, metabolism, and cancer. Cancer Discov. (2015) 5:1024–39. doi: 10.1158/2159-8290.CD-15-0507
213. Valvezan AJ, Turner M, Belaid A, Lam HC, Miller SK, McNamara MC, et al. mTORC1 couples nucleotide synthesis to nucleotide demand resulting in a Targetable metabolic vulnerability. Cancer Cell. (2017) 32:624–38.e625. doi: 10.1016/j.ccell.2017.09.013
214. Cunningham JT, Moreno MV, Lodi A, Ronen SM, Ruggero D. Protein and nucleotide biosynthesis are coupled by a single rate-limiting enzyme, PRPS2, to drive cancer. Cell. (2014) 157:1088–103. doi: 10.1016/j.cell.2014.03.052
215. Finan C, Gaulton A, Kruger FA, Lumbers RT, Shah T, Engmann J, et al. The druggable genome and support for target identification and validation in drug development. Sci Transl Med. (2017) 9:eaag1166. doi: 10.1126/scitranslmed.aag1166
216. Huang H, Weng H, Chen J. m(6)A modification in coding and non-coding RNAs: roles and therapeutic implications in cancer. Cancer Cell. (2020) 37:270–88. doi: 10.1016/j.ccell.2020.02.004
217. Deng X, Su R, Feng X, Wei M, Chen J. Role of N(6)-methyladenosine modification in cancer. Curr Opin Genet Dev. (2018) 48:1–7. doi: 10.1016/j.gde.2017.10.005
218. Maity A, Das B. N6-methyladenosine modification in mRNA: machinery, function and implications for health and diseases. FEBS J. (2016) 283:1607–30. doi: 10.1111/febs.13614
219. Lan Q, Liu PY, Haase J, Bell JL, Hüttelmaier S, Liu T. The critical role of RNA m(6)A methylation in cancer. Cancer Res. (2019) 79:1285–92. doi: 10.1158/0008-5472.CAN-18-2965
220. Liu J, Ren D, Du Z, Wang H, Zhang H, Jin Y. m(6)A demethylase FTO facilitates tumor progression in lung squamous cell carcinoma by regulating MZF1 expression. Biochem Biophys Res Commun. (2018) 502:456–64. doi: 10.1016/j.bbrc.2018.05.175
Keywords: ECM detachment, anoikis, anoikis resistance, metabolism, tumor metastasis
Citation: Adeshakin FO, Adeshakin AO, Afolabi LO, Yan D, Zhang G and Wan X (2021) Mechanisms for Modulating Anoikis Resistance in Cancer and the Relevance of Metabolic Reprogramming. Front. Oncol. 11:626577. doi: 10.3389/fonc.2021.626577
Received: 06 November 2020; Accepted: 08 February 2021;
Published: 29 March 2021.
Edited by:
Yong Teng, Augusta University, United StatesReviewed by:
Helena Bonciani Nader, Federal University of São Paulo, BrazilMariafrancesca Scalise, University of Calabria, Italy
Copyright © 2021 Adeshakin, Adeshakin, Afolabi, Yan, Zhang and Wan. This is an open-access article distributed under the terms of the Creative Commons Attribution License (CC BY). The use, distribution or reproduction in other forums is permitted, provided the original author(s) and the copyright owner(s) are credited and that the original publication in this journal is cited, in accordance with accepted academic practice. No use, distribution or reproduction is permitted which does not comply with these terms.
*Correspondence: Xiaochun Wan, eGMud2FuQHNpYXQuYWMuY24=; Guizhong Zhang, Z3ouemhhbmdAc2lhdC5hYy5jbg==