- 1Department of Radiation Oncology, University of Freiburg - Medical Center, Freiburg, Germany
- 2German Cancer Consortium (DKTK) Partner Site Freiburg, German Cancer Research Center (dkfz), Heidelberg, Germany
- 3Department of Molecular Radiation Oncology, German Cancer Research Center (dkfz), Heidelberg, Germany
Mesenchymal stromal cells (MSCs) comprise a heterogeneous population of multipotent stromal cells that have gained attention for the treatment of irradiation-induced normal tissue toxicities due to their regenerative abilities. As the vast majority of studies focused on the effects of MSCs for photon irradiation-induced toxicities, little is known about the regenerative abilities of MSCs for particle irradiation-induced tissue damage or the effects of particle irradiation on the stem cell characteristics of MSCs themselves. MSC-based therapies may help treat particle irradiation-related tissue lesions in the context of cancer radiotherapy. As the number of clinical proton therapy centers is increasing, there is a need to decidedly investigate MSC-based treatments for particle irradiation-induced sequelae. Furthermore, therapies with MSCs or MSC-derived exosomes may also become a useful tool for manned space exploration or after radiation accidents and nuclear terrorism. However, such treatments require an in-depth knowledge about the effects of particle radiation on MSCs and the effects of MSCs on particle radiation-injured tissues. Here, the existing body of evidence regarding the particle radiobiology of MSCs as well as regarding MSC-based treatments for some typical particle irradiation-induced toxicities is presented and critically discussed.
Introduction
Mesenchymal stromal cells (MSCs) were first isolated from the human bone marrow by Friedenstein and colleagues in the late 1960s (1, 2), but have since been described in various other tissue types such as adipose and glandular tissues, brain and umbilical cord (3–6) and many other organs. As MSCs are a heterogeneous population that can only be characterized by combining molecular and functional traits, the International Society for Cellular Therapy (ISCT) proposed minimal defining criteria for MSCs in order to enhance comparability between the various studies: MSCs are required to adhere to plastic surfaces, exhibit a pattern of positive and (absent) negative surface markers and possess the ability to differentiate along the adipogenic, osteogenic and chondrogenic lineages (7). Currently, MSC-based treatments hold approval for several indications including graft-versus-host disease, Crohn’s-related enterocutaneous fistular disease, bone regeneration, osteoarthritis and cartilage repair (8–11). MSCs possess several characteristics that make them an attractive cell type for cell-based treatments: MSCs can be conveniently isolated and expanded; they are immune-privileged cells, therefore not requiring immunosuppression prior to application, and exhibit the ability to migrate to damaged tissues (12–15). Meta-analyses with more than 1000 patients showed the general safety of MSC-based treatments and did not reveal any severe adverse effects (16). As MSCs also home to tissues damaged by ionizing radiation, these cells came into focus as potential treatments for radiation-induced toxicities, for instance, radiation mucositis, pulmonary fibrosis and enteritis (17–20).
In the context of treating radiation-induced tissue injuries, the MESRIX trial provided a major step towards the routine usage of MSCs in radiation oncology. This randomized, placebo-controlled, double-blinded phase I/II study evaluated for the first time the efficacy of an MSC application for radiotherapy-induced tissue damage (21): Autologous adipose tissue-derived MSCs were transplanted into the submandibular salivary glands of patients with severe radiotherapy-related xerostomia at three or more years after treatment. Upon MSC administration, salivary flow rates were found significantly increased compared to the placebo group, and consistently, xerostomia symptoms decreased in MSC-treated but not in placebo-treated patients. Based on these encouraging results, a follow-up trial (NCT03874572) aims to validate these findings also for allogenic MSCs.
So far, most in vitro and in vivo studies have focused on the impact of photon irradiation on MSCs, and little is known about the particle radiobiology of these multipotent cells, which is a crucial step towards the usage of MSC-based therapies for particle radiation-associated tissue damage, e.g. after particle radiotherapy or during manned deep space flight (22–24).
Material and Methods
The databases PubMed, Google Scholar and Web of Science were screened for studies investigating the impact of MSC-based treatments for particle irradiation-induced toxicities as well as the influence of particle irradiation on MSCs by using the search terms mesenchymal stem cells/mesenchymal stromal cells in combination with protons, proton radiotherapy, helium ions, alpha particles, carbon ions, carbon ion radiotherapy, oxygen ions, iron ions, heavy ions and heavy ion radiotherapy, respectively.
Application of MSCs for the Attenuation of Typical Toxicities After Particle Radiation
Particle Radiotherapy in Cancer Treatment
Currently, heavy ion radiotherapy most commonly employs 12C carbon ions for patient treatment. Clinical feasibility and benefits of carbon ion radiotherapy have been studied in various malignancies such as intracranial tumors, head-and-neck cancers, lung cancer, gastrointestinal malignancies, prostate cancer, sarcomas, gynecological cancers and pediatric malignancies (25).
Head-and-Neck Tumors
Particle radiotherapy has been shown to be an effective treatment for salivary gland, nasopharyngeal and sinunasal carcinomas (25–29); however, bone- and mucosa-related toxicities are common, and no causative treatments have been licensed to date for these radiation-induced lesions.
Radiation-related mucositis is a common acute normal tissue toxicity and affects most patients receiving radiotherapy for head-and-neck malignancies. It considerably worsens patients’ quality of life, increases the risk for malnutrition and infection and can therefore result in treatment interruptions that may in turn deteriorate oncological outcomes. The incidence of severe mucositis after proton irradiation for head-and-neck squamous cell carcinomas ranges between 40 and 79% (30). The impact of both bone marrow- and adipose tissue-derived MSCs on radiation-induced mucositis has been examined in several preclinical studies (19, 31–33). In the study of Maria and colleagues, MSCs significantly attenuated radiation-induced oral mucositis in mice, as evident by reduced ulcer duration and ulcer size, leading to increased weight as well as improved hydration and nutritional status (31). Osteoradionecrosis constitutes another rare but severe chronic toxicity after head-and-neck radiotherapy and is characterized by chronically exposed bone structures that fail to heal within 90 days after irradiation. In the head-and-neck region, osteoradionecrosis commonly affects the mandibular bone, and the incidence of mandibular osteoradionecrosis is reported to be less than 10% in most studies after intensity-modulated radiotherapy, although the variation of incidence in the literature is wide (34). The pathophysiology of osteoradionecrosis is complex and is driven by chronic inflammation, hypovascularity, hypoxia and hypocellularity (35). Treatment aims at alleviating symptoms and may comprise antibiotic treatment, surgical debridement, reconstructive surgery and hyperbaric oxygen; however, management of mandibular osteoradionecrosis remains a clinical challenge (36). Although the incidence of mandibular or maxillary osteoradionecrosis may be lower after carbon ion radiotherapy compared to photon radiotherapy, there are several case reports describing severe cases of osteoradionecrosis after carbon ion treatment (37, 38). In a retrospective analysis, 3 of 63 patients developed grade 3 osteoradionecrosis after carbon ion radiotherapy of head-and-neck malignancies (39). Stem-cell based treatments using MSCs obtained from the bone marrow, adipose tissue or tonsils have been examined for osteoradionecrosis after photon irradiation in four in vivo studies and in two clinical case reports (40–46).
While it is conceivable that MSCs may exert their beneficial effects regarding immunomodulation and replacement of functional cells also after carbon ion irradiation, confirmative data for the treatment of particle radiation-induced mucositis and osteoradionecrosis are still lacking.
Prostate Cancer
In a post-hoc analysis including more than 1000 patients with prostate cancer receiving carbon ion radiotherapy within prospective phase II trials, the 10‐year rates of moderate-to-severe gastrointestinal and genitourinary toxicities were found to be 1.7% and 11.7%, respectively (47). Analyses of the SEER database comparing photon and proton radiotherapy for prostate cancer observed an increased risk for rectal bleeding in patients treated with particle radiation (48). MSCs have been successfully investigated for radiation-induced proctitis, cystitis and fistulas. For instance, four patients received allogeneic bone marrow-derived MSCs for hemorrhagic radiation-induced fistulizing colitis after a radiation oncology accident at a public hospital in France, in which prostate cancer patients were overdosed by up to 30% (49). While two patients exhibited a significant response regarding pain and hemorrhage, another patient experienced a relapse after 6 months that was responsive to a second MSC administration. Considering these encouraging results, a prospective phase II trial is currently evaluating the impact of MSC-based treatments for late severe gastrointestinal complications such as proctitis and cystitis (PRISME trial, NCT02814864). However, similar to the other discussed late sequelae here, there are no studies available that exclusively investigate MSC-based therapies after proton or carbon ion radiotherapy.
MSC Administration as a Treatment Against Acute Radiation Syndrome
The acute radiation syndrome (ARS) represents a complex clinical situation, consisting of the hematopoietic syndrome, the gastrointestinal syndrome and (only clinically relevant for doses exceeding 10 Gy) the cerebrovascular syndrome (50). ARS regularly occurs after radiation exposure of the whole body with doses exceeding 0.5-1 Gy over a short time period. Currently, the standard of care for ARS consists of mainly symptomatic measures, e.g. intravenous hydration, antiemetic and analgesics medication, antibiotic treatment, blood transfusions, application of hematopoietic growth factors and rarely stem cell transplantation. Depending on the extent of medical interventions, the LD50/60 (dose that kills 50% of the population within the first 60 days) ranges between 2.5 and 5 Gy. There are several scenarios in which a particle radiation-induced ARS may occur, e.g. during radiation accidents, nuclear terrorism or warfare and in deep space. The rising number of centers providing particle radiotherapy as well as the increasing usage of nuclear technology for medical purposes, industrial procedures and military functions emphasizes the importance of effective medical countermeasures to treat particle radiation-induced ARS.
Animal studies have shown beneficial effects of MSC-based treatments (including both bone marrow MSCs and MSC-derived exosomes) on the hematopoietic system in lethally irradiated mice (51–54). There are also several case reports showing both the feasibility and efficacy of MSC-based therapies for the treatment of tissue damage caused by radiation accidents (55–58). However, no studies have yet been reported that described the impact of MSC-based therapies on particle irradiation-induced ARS. MSCs from different tissues of origin (bone marrow and umbilical cord) have successfully been investigated for ARS treatment (51, 59). Besides its relevance for unintended radiation accidents, stem cell-based treatments including both MSCs and hematopoietic stem cells are discussed also in the case of nuclear terrorism (60). In this context, the United States Army Medical Research Institute of Chemical Defense (USAMRICD) has established production methods in order to have MSC-based treatments available for ARS (61).
Responses of MSCs to Particle Radiation Exposure
For photon radiotherapy, it has been demonstrated that MSCs from different tissues of origin (e.g. bone marrow, adipose tissue, umbilical cord) are relatively radioresistant and maintain their stem cell traits even after high radiation doses (23, 62, 63). A high anti-oxidative capacity, an effective DNA double-strand break repair, low levels of pro-apoptotic proteins (e.g. Bim and Puma) accompanied by high levels of anti-apoptotic proteins (e.g. Bcl-2 and Bcl-XL) have been reported to contribute to photon radioresistance of these multipotent stromal cells (64, 65). However, only limited data are available on the particle radiobiology of MSCs, and it remains unclear if the photon radioresistance can be extrapolated to radiation with protons or heavier particles. To date, proton radiation has not yet been systematically studied regarding the MSC radiobiology as it is believed to biologically resemble photon radiation. However, there are limited data available on the radiation effects of 12C and heavier particles such as 56Fe on MSCs.
Cellular Survival After Particle Irradiation
Work by our group demonstrated a relatively radioresistant phenotype of bone marrow-derived MSCs after 12C particle radiation (Table 1). The relative biological effectiveness (RBE) calculated at 10% clonogenic survival in bone marrow-derived MSCs ranged between 2.0 and 3.1 compared to photon radiation, underpinning the known heterogeneity of MSCs (69). In line with a radioresistant phenotype after particle radiation, apoptosis rates in bone marrow-MSCs remained low even after exposure to 4 Gy of 12C irradiation. However, no data are available for the influence of protracted courses of low-dose 12C radiotherapy on MSCs that may be more relevant in the space environment and also during radiation exposure in the time of cancer treatment. The different biological effects between photon and 56Fe irradiation on human bone marrow-MSCs have also been thoroughly characterized in vitro (68). While it was reported that neither photon nor 56Fe ions impaired the osteogenic differentiation capability of MSCs, 56Fe irradiation with 1 Gy resulted in a G2/M-phase arrest, which was more pronounced than after physically equivalent doses of photon irradiation. Microarray analyses showed that several genes playing a role in cell cycle progression including cyclin B1 and cyclin E2 were significantly downregulated after 0.1 Gy 56Fe ions, while only a marginal response was observed after photon radiation. Additionally, a more pronounced activation of p53 was found after 56Fe ion radiation than after photon treatment.
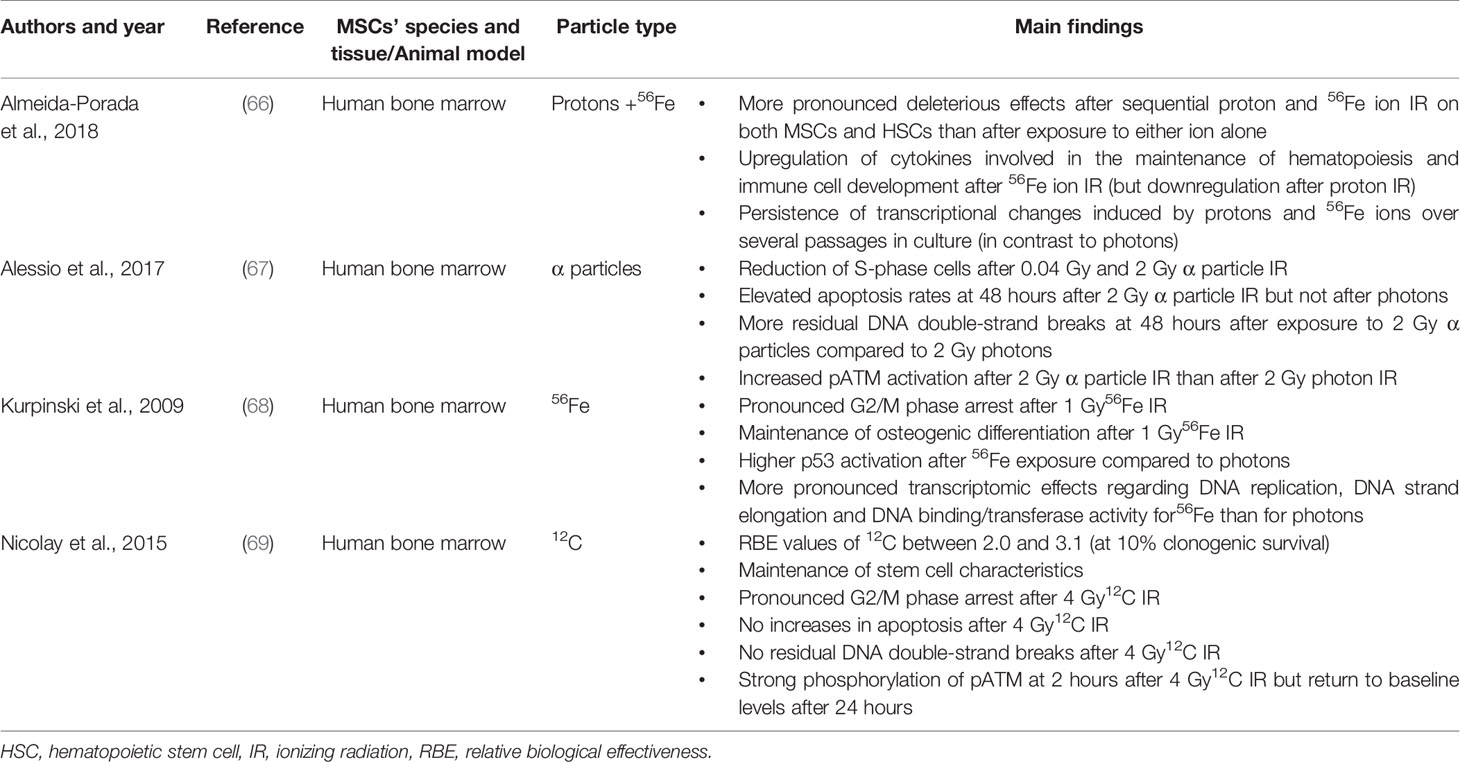
Table 1 Summary of preclinical studies that investigated the effects of different types of particle irradiation on MSCs.
While these analyses were based on 2D systems, some groups also investigated the effects of particle irradiation on adipose-derived stem cells in 3D sphere cultures based on agar coating (70). Both after photon and carbon ion radiation, radiation sensitivity was higher in 2D culture than in 3D culture, which could to some extent be related to the development of radioprotective tissue hypoxia inside of the 3D spheres. In this regard, one should take into consideration that particle irradiation can partly overcome the radioresistance caused by tissue hypoxia (71).
Stem Cell Characteristics After Particle Irradiation
The influence of particle radiation on the defining stem cell characteristics has been investigated both for 12C and 56Fe ions. After 12C irradiation, bone marrow-derived MSCs maintained their adhesive and cellular motility abilities independently of the MSC donor as well as their multi-lineage differentiation capability, which are all pre-requisites for the cells’ regenerative effects (69). Kurpinski et al. could show that the osteogenic differentiation potential of human bone marrow-derived MSCs was maintained after exposure to 1 Gy 56Fe ions. While undirected cellular motility as assessed by time-lapse microscopy remained unaffected after 12C irradiation, directed migration towards particle-irradiated hematopoietic cells has not been examined so far. For lighter alpha particles, the preservation of MSC functions seems to depend on the dose, and only higher doses (2 Gy) have been shown to inhibit the stemness capacity of bone marrow MSCs (67).
DNA Damage Repair After Particle Irradiation
As particle irradiation induces clustered and more complex DNA lesions than photon irradiation, many cell lines have been found to exhibit increased numbers of initial and residual DNA lesions after physically equivalent doses of particle radiation (72–74). However, γH2AX foci analyses in human bone marrow-derived MSCs revealed an effective DNA double strand-break repair after 4 Gy 12C irradiation as evident by low numbers of residual double strand-breaks and only temporary activation of the DNA double-strand break signaling pathways (69). This effective DNA double strand-break repair may also contribute to the reported low apoptosis rates after 12C irradiation in this study (69). However, in the study of Alessio, residual γH2AX levels were significantly higher after 2 Gy alpha particle irradiation than after 2 Gy photon irradiation in bone marrow-isolated MSCs (67). In line with these findings, the authors also observed increased pATM expression at 48 hours after 2 Gy alpha particle irradiation when compared to baseline levels, which was not observed after 2 Gy photon irradiation. Lower alpha particle doses of 0.04 Gy were found to result in an upregulation of pATM at 1 hour after exposure and a decline at later timepoints; additionally, there was no significant difference regarding the number of γH2AX-positive cells at 48 hours after 0.04 Gy alpha particle irradiation compared to unirradiated controls. In the study of Kurpinski and colleagues, pathway and network analyses of transcriptomic profiles revealed differential effects of 56Fe and photons on DNA replication, DNA strand elongation and DNA binding/transferase activity of human bone marrow-MSCs with a more pronounced effect of 56Fe ions on these pathways. Furthermore 1 Gy56Fe ions resulted in a stronger activation of p53 than 1 Gy photons.
MSC-Based Treatment Concepts for Space Radiation-Induced Toxicities
Galactic cosmic rays (GCR) and solar cosmic radiation (SCR) constitute the main components of space irradiation. With an proportion of about 90%, protons form by far the largest component of both GCR and SCR, followed by helium ions (about 10%), electrons and heavier ions (75). Solar particle events (SPEs) as part of SCR, are unpredictable events that occur when protons are accelerated during a flare or during a coronal mass ejection. Besides highly energetic protons and helium ions, SPEs include heavier charged (HZE) particles such as carbon, oxygen and iron ions. Long-term missions to the Mars that may last more than 2 years harbor the risk for considerable radiation exposures, as the shielding provided by the Earth’s magnetic field is absent. Therefore, the National Aeronautics and Space Administration (NASA) classifies the ARS caused by SPEs as a major obstacle to long-term manned space expeditions (76).
Besides higher doses encountered by SPEs, cumulative annual GCR particle doses inside a space craft amount to 176 ± 29 mGy based on measurements in the Mars Science Laboratory (77). In vivo data demonstrated that even low and protracted oxygen ion (16O) radiation doses of 0.1 Gy impair hematopoiesis (78), and the effective proton dose to reduce the whole blood cell count (WBC) by half was shown to be approximately 1 Gy (79).
A major step towards the usage of MSCs for space irradiation-induced toxicities was performed recently by Huang and colleagues, who demonstrated the feasibility to grow human bone marrow-MSC aboard the International Space Station (ISS) (80). The MSCs’ phenotype was observed unaltered during proliferation in space, and MSCs maintained their proliferative characteristics aboard ISS. Interestingly, space-expanded MSCs seem to exhibit pronounced immunosuppressive effects compared to MSCs grown on the Earth. At least in short-term space cultures, there were no signs for tumorigenic transformation and genomic instability in MSCs.
Recently, a Chinese study reported transcriptional changes of murine bone marrow cells after whole-body 12C irradiation with 2 Gy (81), that would mimic the acute bone marrow exposure during a severe SPE. 12C irradiation resulted in increased reactive oxygen species production, γH2AX foci and apoptosis levels in bone marrow cells. Significant alterations in genes belonging to the immune response, DNA damage repair, MAPK, TNF signaling and apoptosis pathways were observed in murine bone marrow cells after 2 Gy 12C irradiation.
A high-quality study simulating the combined effects of GCR and SPE on the interaction between human bone marrow MSCs and hematopoietic progenitor cells was conducted by Almeida-Porada (66). Interestingly, 56Fe ions upregulated many cytokines involved in hematopoiesis and immune cell development, whereas proton irradiation produced the opposite effect and downregulated key cytokines involved in these functions. Also very importantly, the transcriptional changes after proton and 56Fe ion irradiation were long-lasting and persisted over several passages.
It is important to consider that low-dose irradiation (≤ 0.1 Gy) has shown to have significantly different effects on MSCs and adipose-derived stem cells than higher radiation doses (24, 82, 83). Low dose irradiation was found to enhance proliferation and to increase the secretion of stem-cell factor (SCF) and GM-CSF, which may favor hematopoiesis (83). In the study of Yang et al., the pro-survival effects of low-dose irradiation on human bone marrow MSCs were mediated via several proteins involved in cell cycle control such as Rb, cyclin E, CDK1, and CDC25B (83). Following these findings, there are considerations to use low-dose irradiation to support large scale expansion as well as therapeutic effects of MSCs (82, 84). Unfortunately, to the best of our knowledge, no studies have reported effects of low-dose particle irradiation, especially protons, on the proliferation rate of MSCs. Additionally, protracted low-dose irradiation over several weeks to mimic GCR are complicated by the difficulty to long-term culture MSCs due to premature senescence and therefore limited passage numbers (85). In the case of prolonged MSC culturing, which may be necessary to expand autologous MSC of astronauts prior to space missions, one should be aware that the DNA repair capacity is lowered leading to more spontaneous and radiation-induced micronuclei (86).
In the space environment, the more convenient way would be the usage of commercially available allogenic off-the-shelf MSCs. Due to the low immunogenicity and immune-privileged behavior, allogenic MSC treatments are generally feasible without prior immune suppression, as demonstrated in large clinical trials (10, 87). In this regard, effective shielding of these off-the-shelf MSCs would be desirable to avoid damage prior to application in a case of SPE.
Conclusion
In general, MSC-based therapies hold promise for the treatment of irradiation-induced toxicities such as mucositis, osteoradionecrosis, proctitis or cystitis as typical sequelae after radiotherapy in the head-and-neck or pelvic region, respectively. So far, preclinical and clinical research has been conducted focussing on the effects of MSCs on photon-induced toxicities, wherefore the role of MSCs as cell therapy for particle irradiation-related adverse reactions in cancer treatment remains to be elucidated. Furthermore, MSC-based therapies may be used after nuclear accidents or during future manned space missions, although the evidence is very limited. In summary, many further efforts are needed in the future to fully examine the impact of particle irradiation on the regenerative abilities of MSCs and potential attenuating effects of MSCs on particle irradiation-induced normal tissue toxicities.
Author Contributions
AR, A-LG, and NN wrote and revised this mini-review. All authors contributed to the article and approved the submitted version.
Funding
AR was supported by the IMM-PACT-Program for Clinician Scientists, Department of Medicine II, Medical Center – University of Freiburg and Faculty of Medicine, University of Freiburg, funded by the Deutsche Forschungsgemeinschaft (DFG, German Research Foundation) – 413517907. We thank the European Space Agency (ESA) for their financial support of the project “Human mesenchymal stem cells as potential mitigators of bone marrow toxicity caused by spaceradiation” within the CORA-IBER program.
Conflict of Interest
The authors declare that the research was conducted in the absence of any commercial or financial relationships that could be construed as a potential conflict of interest.
References
1. Friedenstein AJ, Petrakova KV, Kurolesova AI, Frolova GP. Heterotopic of bone marrow. Analysis of precursor cells for osteogenic and hematopoietic tissues. Transplantation (1968) 6(2):230–47. doi: 10.1097/00007890-196803000-00009
2. Friedenstein AJ, Deriglasova UF, Kulagina NN, Panasuk AF, Rudakowa SF, Luria EA, et al. Precursors for fibroblasts in different populations of hematopoietic cells as detected by the in vitro colony assay method. Exp Hematol (1974) 2(2):83–92.
3. Zuk PA, Zhu M, Ashjian P, De Ugarte DA, Huang JI, Mizuno H, et al. Human adipose tissue is a source of multipotent stem cells. Mol Biol Cell (2002) 13(12):4279–95. doi: 10.1091/mbc.e02-02-0105
4. Bieback K, Kern S, Kluter H, Eichler H. Critical parameters for the isolation of mesenchymal stem cells from umbilical cord blood. Stem Cells (2004) 22(4):625–34. doi: 10.1634/stemcells.22-4-625
5. Paul G, Özen I, Christophersen NS, Reinbothe T, Bengzon J, Visse E, et al. The adult human brain harbors multipotent perivascular mesenchymal stem cells. PloS One (2012) 7(4):e35577. doi: 10.1371/journal.pone.0035577
6. Rotter N, Oder J, Schlenke P, Lindner U, Böhrnsen F, Kramer J, et al. Isolation and characterization of adult stem cells from human salivary glands. Stem Cells Dev (2008) 17(3):509–18. doi: 10.1089/scd.2007.0180
7. Dominici M, Le Blanc K, Mueller I, Slaper-Cortenbach I, Marini F, Krause D, et al. Minimal criteria for defining multipotent mesenchymal stromal cells. The International Society for Cellular Therapy position statement. Cytotherapy (2006) 8(4):315–7. doi: 10.1080/14653240600855905
8. Godoy JAP, Paiva RMA, Souza AM, Kondo AT, Kutner JM, Okamoto OK. Clinical Translation of Mesenchymal Stromal Cell Therapy for Graft Versus Host Disease. Front Cell Dev Biol (2019) 7:255–. doi: 10.3389/fcell.2019.00255
9. Morata-Tarifa C, del Mar Macías-Sánchez M, Gutiérrez-Pizarraya A, Sanchez-Pernaute R. Mesenchymal stromal cells for the prophylaxis and treatment of graft-versus-host disease—a meta-analysis. Stem Cell Res Ther (2020) 11(1):1–12. doi: 10.1186/s13287-020-01592-z
10. Panes J, Garcia-Olmo D, Van Assche G, Colombel JF, Reinisch W, Baumgart DC, et al. Expanded allogeneic adipose-derived mesenchymal stem cells (Cx601) for complex perianal fistulas in Crohn’s disease: a phase 3 randomised, double-blind controlled trial. Lancet (2016) 388(10051):1281–90. doi: 10.1016/S0140-6736(16)31203-X
11. Jo CH, Lee YG, Shin WH, Kim H, Chai JW, Jeong EC, et al. Intra-articular injection of mesenchymal stem cells for the treatment of osteoarthritis of the knee: a proof-of-concept clinical trial. Stem Cells (2014) 32(5):1254–66. doi: 10.1002/stem.1634
12. Ruhle A, Lopez Perez R, Zou B, Grosu AL, Huber PE, Nicolay NH. The Therapeutic Potential of Mesenchymal Stromal Cells in the Treatment of Chemotherapy-Induced Tissue Damage. Stem Cell Rev (2019) 15(3):356–73. doi: 10.1007/s12015-019-09886-3
13. Li L, Jiang J. Regulatory factors of mesenchymal stem cell migration into injured tissues and their signal transduction mechanisms. Front Med (2011) 5(1):33–9. doi: 10.1007/s11684-011-0114-1
14. Le Blanc K, Tammik C, Rosendahl K, Zetterberg E, Ringden O. HLA expression and immunologic properties of differentiated and undifferentiated mesenchymal stem cells. Exp Hematol (2003) 31(10):890–6. doi: 10.1016/S0301-472X(03)00110-3
15. De Miguel MP, Fuentes-Julian S, Blazquez-Martinez A, Pascual CY, Aller MA, Arias J, et al. Immunosuppressive properties of mesenchymal stem cells: advances and applications. Curr Mol Med (2012) 12(5):574–91. doi: 10.2174/156652412800619950
16. Lalu MM, McIntyre L, Pugliese C, Fergusson D, Winston BW, Marshall JC, et al. Safety of cell therapy with mesenchymal stromal cells (SafeCell): a systematic review and meta-analysis of clinical trials. PloS One (2012) 7(10):e47559. doi: 10.1371/journal.pone.0047559
17. Nicolay NH, Lopez Perez R, Debus J, Huber PE. Mesenchymal stem cells - A new hope for radiotherapy-induced tissue damage? Cancer Lett (2015) 366(2):133–40. doi: 10.1016/j.canlet.2015.06.012
18. Chang PY, Qu YQ, Wang J, Dong LH. The potential of mesenchymal stem cells in the management of radiation enteropathy. Cell Death Dis (2015) 6(8):e1840–e. doi: 10.1038/cddis.2015.189
19. Schmidt M, Haagen J, Noack R, Siegemund A, Gabriel P, Dorr W. Effects of bone marrow or mesenchymal stem cell transplantation on oral mucositis (mouse) induced by fractionated irradiation. Strahlenther Onkol (2014) 190(4):399–404. doi: 10.1007/s00066-013-0510-3
20. Zanoni M, Cortesi M, Zamagni A, Tesei A. The Role of Mesenchymal Stem Cells in Radiation-Induced Lung Fibrosis. Int J Mol Sci (2019) 20(16). doi: 10.3390/ijms20163876
21. Gronhoj C, Jensen DH, Vester-Glowinski P, Jensen SB, Bardow A, Oliveri RS, et al. Safety and Efficacy of Mesenchymal Stem Cells for Radiation-Induced Xerostomia: A Randomized, Placebo-Controlled Phase 1/2 Trial (MESRIX). Int J Radiat Oncol Biol Phys (2018) 101(3):581–92. doi: 10.1016/j.ijrobp.2018.02.034
22. Ruhle A, Perez RL, Glowa C, Weber KJ, Ho AD, Debus J, et al. Cisplatin radiosensitizes radioresistant human mesenchymal stem cells. Oncotarget (2017) 8(50):87809–20. doi: 10.18632/oncotarget.21214
23. Chen MF, Lin CT, Chen WC, Yang CT, Chen CC, Liao SK, et al. The sensitivity of human mesenchymal stem cells to ionizing radiation. Int J Radiat Oncol Biol Phys (2006) 66(1):244–53. doi: 10.1016/j.ijrobp.2006.03.062
24. Liang X, So YH, Cui J, Ma K, Xu X, Zhao Y, et al. The Low-dose Ionizing Radiation Stimulates Cell Proliferation via Activation of the MAPK/ERK Pathway in Rat Cultured Mesenchymal Stem Cells. J Radiat Res (2011) 52(3):380–6. doi: 10.1269/jrr.10121
25. Malouff TD, Mahajan A, Krishnan S, Beltran C, Seneviratne DS, Trifiletti DM. Carbon Ion Therapy: A Modern Review of an Emerging Technology. Front Oncol (2020) 10(82). doi: 10.3389/fonc.2020.00082
26. Akbaba S, Held T, Lang K, Forster T, Federspil P, Herfarth K, et al. Bimodal radiotherapy with active raster-scanning carbon ion radiotherapy and intensity-modulated radiotherapy in high-risk nasopharyngeal carcinoma results in excellent local control. Cancers (2019) 11(3):379. doi: 10.3390/cancers11030379
27. Akbaba S, Ahmed D, Lang K, Held T, Mattke M, Hoerner-Rieber J, et al. Results of a combination treatment with intensity modulated radiotherapy and active raster-scanning carbon ion boost for adenoid cystic carcinoma of the minor salivary glands of the nasopharynx. Oral Oncol (2019) 91:39–46. doi: 10.1016/j.oraloncology.2019.02.019
28. Jensen AD, Nikoghosyan AV, Lossner K, Haberer T, Jäkel O, Münter MW, et al. COSMIC: A Regimen of Intensity Modulated Radiation Therapy Plus Dose-Escalated, Raster-Scanned Carbon Ion Boost for Malignant Salivary Gland Tumors: Results of the Prospective Phase 2 Trial. Int J Radiat Oncol Biol Phys (2015) 93(1):37–46. doi: 10.1016/j.ijrobp.2015.05.013
29. Koto M, Hasegawa A, Takagi R, Sasahara G, Ikawa H, Mizoe J-e, et al. Feasibility of carbon ion radiotherapy for locally advanced sinonasal adenocarcinoma. Radiother Oncol (2014) 113(1):60–5. doi: 10.1016/j.radonc.2014.09.009
30. Beddok A, Vela A, Calugaru V, Tessonnier T, Kubes J, Dutheil P, et al. Proton therapy for head and neck squamous cell carcinomas: A review of the physical and clinical challenges. Radiother Oncol (2020) 147:30–9. doi: 10.1016/j.radonc.2020.03.006
31. Maria OM, Shalaby M, Syme A, Eliopoulos N, Muanza T. Adipose mesenchymal stromal cells minimize and repair radiation-induced oral mucositis. Cytotherapy (2016) 18(9):1129–45. doi: 10.1016/j.jcyt.2016.06.008
32. Elsaadany B, El Kholy S, El Rouby D, Rashed L, Shouman T. Effect of Transplantation of Bone Marrow Derived Mesenchymal Stem Cells and Platelets Rich Plasma on Experimental Model of Radiation Induced Oral Mucosal Injury in Albino Rats. Int J Dent (2017) 2017:8634540. doi: 10.1155/2017/8634540
33. Schmidt M, Piro-Hussong A, Siegemund A, Gabriel P, Dorr W. Modification of radiation-induced oral mucositis (mouse) by adult stem cell therapy: single-dose irradiation. Radiat Environ Biophys (2014) 53(4):629–34. doi: 10.1007/s00411-014-0552-7
34. Aarup-Kristensen S, Hansen CR, Forner L, Brink C, Eriksen JG, Johansen J. Osteoradionecrosis of the mandible after radiotherapy for head and neck cancer: risk factors and dose-volume correlations. Acta Oncol (2019) 58(10):1373–7. doi: 10.1080/0284186X.2019.1643037
35. Marx RE. Osteoradionecrosis: a new concept of its pathophysiology. J Oral Maxillofac Surg (1983) 41(5):283–8. doi: 10.1016/0278-2391(83)90294-X
36. Rice N, Polyzois I, Ekanayake K, Omer O, Stassen LF. The management of osteoradionecrosis of the jaws–a review. Surgeon (2015) 13(2):101–9. doi: 10.1016/j.surge.2014.07.003
37. Dell’Era V, Aluffi Valletti P, Garzaro G, Garzaro M. Maxillo-mandibular osteoradionecrosis following C-ion radiotherapy: Clinical notes and review of literature. Eur J Inflamm (2020) 18:2058739220934562. doi: 10.1177/2058739220934562
38. Oki M, Kanazaki A, Taniguchi H, Oki M, Kanazaki A, Taniguchi H. Osteoradionecrosis Following Carbon Ion Radiotherapy: Case History Report of a Soft Palate Defect. Int J Prosthod (2016) 29(5):448–50. doi: 10.11607/ijp.4836
39. Sasahara G, Koto M, Ikawa H, Hasegawa A, Takagi R, Okamoto Y, et al. Effects of the dose-volume relationship on and risk factors for maxillary osteoradionecrosis after carbon ion radiotherapy. Radiat Oncol (2014) 9(1):92. doi: 10.1186/1748-717X-9-92
40. Janus JR, Jackson RS, Lees KA, Voss SG, Wilson ZC, Remmes NB, et al. Human Adipose-Derived Mesenchymal Stem Cells for Osseous Rehabilitation of Induced Osteoradionecrosis: A Rodent Model. Otolaryngol Head Neck Surg (2017) 156(4):616–21. doi: 10.1177/0194599816688647
41. Park HS, Lee J, Kim JW, Kim HY, Jung SY, Lee SM, et al. Preventive effects of tonsil-derived mesenchymal stem cells on osteoradionecrosis in a rat model. Head Neck (2018) 40(3):526–35. doi: 10.1002/hed.25004
42. Jin IG, Kim JH, Wu HG, Kim SK, Park Y, Hwang SJ. Effect of bone marrow-derived stem cells and bone morphogenetic protein-2 on treatment of osteoradionecrosis in a rat model. J Craniomaxillofac Surg (2015) 43(8):1478–86. doi: 10.1016/j.jcms.2015.06.035
43. Xu J, Zheng Z, Fang D, Gao R, Liu Y, Fan Z, et al. Mesenchymal stromal cell-based treatment of jaw osteoradionecrosis in Swine. Cell Transplant (2012) 21(8):1679–86. doi: 10.3727/096368911X637434
44. Manimaran K, Sankaranarayanan S, Ravi VR, Elangovan S, Chandramohan M, Perumal SM. Treatment of osteoradionecrosis of mandible with bone marrow concentrate and with dental pulp stem cells. Ann Maxillofac Surg (2014) 4(2):189–92. doi: 10.4103/2231-0746.147130
45. Mendonça JJ, Juiz-Lopez P. Regenerative facial reconstruction of terminal stage osteoradionecrosis and other advanced craniofacial diseases with adult cultured stem and progenitor cells. Plast Reconstr Surg (2010) 126(5):1699–709. doi: 10.1097/PRS.0b013e3181f24164
46. Gundestrup AK, Lynggaard CD, Forner L, Heino TJ, Jakobsen KK, Fischer-Nielsen A, et al. Mesenchymal Stem Cell Therapy for Osteoradionecrosis of the Mandible: a Systematic Review of Preclinical and Human Studies. Stem Cell Rev Rep (2020) 16(6):1208–21. doi: 10.1007/s12015-020-10034-5
47. Kasuya G, Ishikawa H, Tsuji H, Haruyama Y, Kobashi G, Ebner DK, et al. Cancer-specific mortality of high-risk prostate cancer after carbon-ion radiotherapy plus long-term androgen deprivation therapy. Cancer Sci (2017) 108(12):2422–9. doi: 10.1111/cas.13402
48. Sheets NC, Goldin GH, Meyer AM, Wu Y, Chang Y, Stürmer T, et al. Intensity-modulated radiation therapy, proton therapy, or conformal radiation therapy and morbidity and disease control in localized prostate cancer. Jama (2012) 307(15):1611–20. doi: 10.1001/jama.2012.460
49. Tamarat R, Benderitter M. The Medical Follow-up of the Radiological Accident: Épinal 2006. Radiat Res (2019) 192(3):251–7. doi: 10.1667/RR15262.1
50. López M, Martín M. Medical management of the acute radiation syndrome. Rep Pract Oncol Radiother (2011) 16(4):138–46. doi: 10.1016/j.rpor.2011.05.001
51. Hu KX, Sun QY, Guo M, Ai HS. The radiation protection and therapy effects of mesenchymal stem cells in mice with acute radiation injury. Br J Radiol (2010) 83(985):52–8. doi: 10.1259/bjr/61042310
52. Lange C, Brunswig-Spickenheier B, Cappallo-Obermann H, Eggert K, Gehling UM, Rudolph C, et al. Radiation Rescue: Mesenchymal Stromal Cells Protect from Lethal Irradiation. PloS One (2011) 6(1):e14486. doi: 10.1371/journal.pone.0014486
53. Yang X, Balakrishnan I, Torok-Storb B, Pillai MM. Marrow Stromal Cell Infusion Rescues Hematopoiesis in Lethally Irradiated Mice despite Rapid Clearance after Infusion. Adv Hematol (2012) 2012:142530. doi: 10.1155/2012/142530
54. Alchinova I, Polyakova M, Yakovenko E, Medvedeva YS, Saburina I, Karganov MY. Effect of Extracellular Vesicles Formed by Multipotent Mesenchymal Stromal Cells on Irradiated Animals. Bull Exp Biol Med (2019) 166(4):574–9. doi: 10.1007/s10517-019-04394-3
55. Guo M, Dong Z, Qiao J, Yu C, Sun Q, Hu K, et al. Severe acute radiation syndrome: treatment of a lethally 60Co-source irradiated accident victim in China with HLA-mismatched peripheral blood stem cell transplantation and mesenchymal stem cells. J Radiat Res (2014) 55(2):205–9. doi: 10.1093/jrr/rrt102
56. Lataillade J, Doucet C, Bey E, Carsin H, Huet C, Clairand I, et al. New approach to radiation burn treatment by dosimetry-guided surgery combined with autologous mesenchymal stem cell therapy. Regen Med (2007) 2(5):785–94. doi: 10.2217/17460751.2.5.785
57. Chapel A, Francois S, Douay L, Benderitter M, Voswinkel J. New insights for pelvic radiation disease treatment: Multipotent stromal cell is a promise mainstay treatment for the restoration of abdominopelvic severe chronic damages induced by radiotherapy. World J Stem Cells (2013) 5(4):106–11. doi: 10.4252/wjsc.v5.i4.106
58. Chapel A, Francois S, Douay L, Benderitter M, Voswinkel J. Fifteen years of preclinical and clinical experiences about biotherapy treatment of lesions induced by accidental irradiation and radiotherapy. World J Stem Cells (2013) 5(3):68–72. doi: 10.4252/wjsc.v5.i3.68
59. Shim S, Lee SB, Lee J-g, Jang W-S, Lee S-J, Park S, et al. Mitigating effects of hUCB-MSCs on the hematopoietic syndrome resulting from total body irradiation. Exp Hematol (2013) 41(4):346–53.e2. doi: 10.1016/j.exphem.2013.01.002
60. Gale RP, Armitage JO. Are We Prepared for Nuclear Terrorism? N Engl J Med (2018) 378(13):1246–54. doi: 10.1056/NEJMsr1714289
61. Eaton EB Jr, Varney TR. Mesenchymal stem cell therapy for acute radiation syndrome: innovative medical approaches in military medicine. Mil Med Res (2015) 2:2. doi: 10.1186/s40779-014-0027-9
62. Nicolay NH, Sommer E, Lopez R, Wirkner U, Trinh T, Sisombath S, et al. Mesenchymal stem cells retain their defining stem cell characteristics after exposure to ionizing radiation. Int J Radiat Oncol Biol Phys (2013) 87(5):1171–8. doi: 10.1016/j.ijrobp.2013.09.003
63. Rühle A, Xia O, Perez RL, Trinh T, Richter W, Sarnowska A, et al. The Radiation Resistance of Human Multipotent Mesenchymal Stromal Cells Is Independent of Their Tissue of Origin. Int J Radiat Oncol Biol Phys (2018) 100(5):1259–69. doi: 10.1016/j.ijrobp.2018.01.015
64. Nicolay NH, Lopez Perez R, Saffrich R, Huber PE. Radio-resistant mesenchymal stem cells: mechanisms of resistance and potential implications for the clinic. Oncotarget (2015) 6(23):19366–80. doi: 10.18632/oncotarget.4358
65. Sugrue T, Brown JA, Lowndes NF, Ceredig R. Multiple facets of the DNA damage response contribute to the radioresistance of mouse mesenchymal stromal cell lines. Stem Cells (2013) 31(1):137–45. doi: 10.1002/stem.1222
66. Almeida-Porada G, Rodman C, Kuhlman B, Brudvik E, Moon J, George S, et al. Exposure of the Bone Marrow Microenvironment to Simulated Solar and Galactic Cosmic Radiation Induces Biological Bystander Effects on Human Hematopoiesis. Stem Cells Dev (2018) 27(18):1237–56. doi: 10.1089/scd.2018.0005
67. Alessio N, Esposito G, Galano G, De Rosa R, Anello P, Peluso G, et al. Irradiation of Mesenchymal Stromal Cells With Low and High Doses of Alpha Particles Induces Senescence and/or Apoptosis. J Cell Biochem (2017) 118(9):2993–3002. doi: 10.1002/jcb.25961
68. Kurpinski K, Jang DJ, Bhattacharya S, Rydberg B, Chu J, So J, et al. Differential effects of x-rays and high-energy 56Fe ions on human mesenchymal stem cells. Int J Radiat Oncol Biol Phys (2009) 73(3):869–77. doi: 10.1016/j.ijrobp.2008.10.002
69. Nicolay NH, Liang Y, Lopez Perez R, Bostel T, Trinh T, Sisombath S, et al. Mesenchymal stem cells are resistant to carbon ion radiotherapy. Oncotarget (2015) 6(4):2076–87. doi: 10.18632/oncotarget.2857
70. Rong --Y, Dong -P, Xiong --Y, Gang -X, Xin --Z, Man --X, et al. Irradiation Response of Adipose-derived Stem Cells under Three-dimensional Culture Condition. Biomed Environ Sci (2015) 28(- 8):549. doi: 10.3967/bes2015.079
71. Durante M, Debus J. Heavy Charged Particles: Does Improved Precision and Higher Biological Effectiveness Translate to Better Outcome in Patients? Semin Radiat Oncol (2018) 28(2):160–7. doi: 10.1016/j.semradonc.2017.11.004
72. Lopez Perez R, Nicolay NH, Wolf JC, Frister M, Schmezer P, Weber KJ, et al. DNA damage response of clinical carbon ion versus photon radiation in human glioblastoma cells. Radiother Oncol (2019) 133:77–86. doi: 10.1016/j.radonc.2018.12.028
73. Walenta S, Mueller-Klieser W. Differential Superiority of Heavy Charged-Particle Irradiation to X-Rays: Studies on Biological Effectiveness and Side Effect Mechanisms in Multicellular Tumor and Normal Tissue Models. Front Oncol (2016) 6(30). doi: 10.3389/fonc.2016.00030
74. Hartfiel S, Häfner M, Perez RL, Rühle A, Trinh T, Debus J, et al. Differential response of esophageal cancer cells to particle irradiation. Radiat Oncol (2019) 14(1):119. doi: 10.1186/s13014-019-1326-9
75. Kennedy AR. Biological Effects of Space Radiation and Development of Effective Countermeasures. Life Sci Space Res (2014) 1:10–43. doi: 10.1016/j.lssr.2014.02.004
76. Chancellor JC, Scott GBI, Sutton JP. Space Radiation: The Number One Risk to Astronaut Health beyond Low Earth Orbit. Life (Basel) (2014) 4(3):491–510. doi: 10.3390/life4030491
77. Zeitlin C, Hassler DM, Cucinotta FA, Ehresmann B, Wimmer-Schweingruber RF, Brinza DE, et al. Measurements of Energetic Particle Radiation in Transit to Mars on the Mars Science Laboratory. Science (2013) 340(6136):1080–4. doi: 10.1126/science.1235989
78. Wang Y, Chang J, Li X, Pathak R, Sridharan V, Jones T, et al. Low doses of oxygen ion irradiation cause long-term damage to bone marrow hematopoietic progenitor and stem cells in mice. PloS One (2017) 12(12):e0189466. doi: 10.1371/journal.pone.0189466
79. Sanzari JK, Cengel KA, Wan XS, Rusek A, Kennedy AR. Acute Hematological Effects in Mice Exposed to the Expected Doses, Dose-rates, and Energies of Solar Particle Event-like Proton Radiation. Life Sci Space Res (2014) 2:86–91. doi: 10.1016/j.lssr.2014.01.003
80. Huang P, Russell AL, Lefavor R, Durand NC, James E, Harvey L, et al. Feasibility, potency, and safety of growing human mesenchymal stem cells in space for clinical application. NPJ Microgravity (2020) 6:16. doi: 10.1038/s41526-020-0106-z
81. Liu F, Wang Z, Li W, Wei Y. Transcriptional response of murine bone marrow cells to total-body carbon-ion irradiation. Mutat Res Genet Toxicol Environ Mutagen (2019) 839:49–58. doi: 10.1016/j.mrgentox.2019.01.014
82. Schröder A, Kriesen S, Hildebrandt G, Manda K. First Insights into the Effect of Low-Dose X-Ray Irradiation in Adipose-Derived Stem Cells. Int J Mol Sci (2019) 20(23):6075. doi: 10.3390/ijms20236075
83. Yang L, Liu Z, Chen C, Cong X, Li Z, Zhao S, et al. Low-dose radiation modulates human mesenchymal stem cell proliferation through regulating CDK and Rb. Am J Transl Res (2017) 9(4):1914–21.
84. Guo W-Y, Wang G-J, Wang P, Chen Q, Tan Y, Cai L. Acceleration of Diabetic Wound Healing by Low-Dose Radiation is Associated with Peripheral Mobilization of Bone Marrow Stem Cells. Radiat Res (2010) 174(4):467–79. doi: 10.1667/RR1980.1
85. Estrada JC, Torres Y, Benguría A, Dopazo A, Roche E, Carrera-Quintanar L, et al. Human mesenchymal stem cell-replicative senescence and oxidative stress are closely linked to aneuploidy. Cell Death Dis (2013) 4(6):e691–e. doi: 10.1038/cddis.2013.211
86. Hladik D, Höfig I, Oestreicher U, Beckers J, Matjanovski M, Bao X, et al. Long-term culture of mesenchymal stem cells impairs ATM-dependent recognition of DNA breaks and increases genetic instability. Stem Cell Res Ther (2019) 10(1):218. doi: 10.1186/s13287-019-1334-6
Keywords: stem cell therapy, normal tissue toxicities, radiotherapy, particle irradiation, mesenchymal stem cells, mesenchymal stromal cells, space irradiation, radiation accidents
Citation: Rühle A, Grosu A-L and Nicolay NH (2021) The Particle Radiobiology of Multipotent Mesenchymal Stromal Cells: A Key to Mitigating Radiation-Induced Tissue Toxicities in Cancer Treatment and Beyond? Front. Oncol. 11:616831. doi: 10.3389/fonc.2021.616831
Received: 13 October 2020; Accepted: 23 March 2021;
Published: 12 April 2021.
Edited by:
Daniel Michael Trifiletti, Mayo Clinic Florida, United StatesReviewed by:
Igor Maiborodin, Institute of Chemical Biology and Fundamental Medicine (RAS), RussiaUmberto Galderisi, University of Campania Luigi Vanvitelli, Italy
Copyright © 2021 Rühle, Grosu and Nicolay. This is an open-access article distributed under the terms of the Creative Commons Attribution License (CC BY). The use, distribution or reproduction in other forums is permitted, provided the original author(s) and the copyright owner(s) are credited and that the original publication in this journal is cited, in accordance with accepted academic practice. No use, distribution or reproduction is permitted which does not comply with these terms.
*Correspondence: Nils H. Nicolay, bmlscy5uaWNvbGF5QHVuaWtsaW5pay1mcmVpYnVyZy5kZQ==