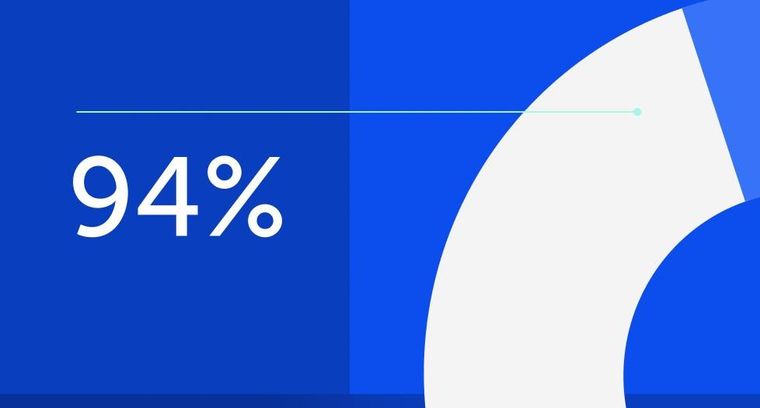
94% of researchers rate our articles as excellent or good
Learn more about the work of our research integrity team to safeguard the quality of each article we publish.
Find out more
REVIEW article
Front. Oncol., 29 October 2020
Sec. Pediatric Oncology
Volume 10 - 2020 | https://doi.org/10.3389/fonc.2020.566822
This article is part of the Research TopicRecent Advances in Pediatric Cancer Predisposition SyndromesView all 12 articles
Medulloblastoma is the most common malignant brain tumor in children. In addition to sporadic cases, medulloblastoma may occur in association with cancer predisposition syndromes. This review aims to provide a complete description of inherited cancer syndromes associated with medulloblastoma. We examine their epidemiological, clinical, genetic, and diagnostic features and therapeutic approaches, including their correlation with medulloblastoma. Furthermore, according to the most recent molecular advances, we describe the association between the various molecular subgroups of medulloblastoma and each cancer predisposition syndrome. Knowledge of the aforementioned conditions can guide pediatric oncologists in performing adequate cancer surveillance. This will allow clinicians to promptly diagnose and treat medulloblastoma in syndromic children, forming a team with all specialists necessary for the correct management of the other various manifestations/symptoms related to the inherited cancer syndromes.
Medulloblastoma (MB) is the most frequent malignant tumor of the central nervous system (CNS) in childhood, representing 15–20% of all CNS neoplasms (1). It mainly affects the pediatric age with a 10-fold higher frequency than in adults (2). Children are diagnosed generally between 2 and 8 years old (median of 6 years old), with 50% of cases occurring in children under 5 years old and with a male/female ratio of 2:1 (3).
Clinical manifestations are initially related to intracranial hypertension and to the tumor’s mass effect in the posterior fossa, including headaches, nausea, vomiting, ataxia, other motor deficits, and visual impairment. MB diagnosis is suspected based on neuroimaging of the brain and spine. Disease staging is established on magnetic resonance imaging (MRI) and cerebrospinal fluid (CSF) cytology (4), with about 35% of cases being metastatic at diagnosis (5).
Histological classification of MB distinguishes four variants: classic (68–80%); desmoplastic/nodular (7%), with a more favorable prognosis in children under 5 years old; MB with extensive nodularity (3%), generally found in young patients and sometimes associated with nevoid basal cell carcinoma syndrome; and large cell/anaplastic (10–22%), characterized by a more aggressive clinical behavior (6).
Treatment of MB is based on surgical resection, chemotherapy, and cranio-spinal irradiation (CSI). Due to the severe adverse effects of CSI, such as neurocognitive disability, endocrine dysfunction, impaired growth, infertility, and increased risk of secondary malignancies, great effort has been dedicated to reduce, differ, or omit radiation therapy, especially in children <3–5 years of age.
Among genetic defects, MYC amplification is the most recurrent and is associated with a worse prognosis (7–9).
A risk stratification based on histopathological subtype, age at diagnosis, staging, residual disease, MYC status, and molecular subgrouping allows a distinction of low-, average-, and high-risk patients (10). For low- and average-risk patients (characterized by age over three years old, absence of metastatic and/or residual disease, histotype other than anaplastic, absence of MYC amplification and/or TP53 mutations), 5-year overall survival (OS) is between 75% to over 90% (11–14), while high-risk patients show 5-year OS around 50–75% (11, 15–19).
More recently, four molecular MB subgroups have been identified and included in the 2016 WHO Classification of Tumors of the Central Nervous System (20): MBWNT, MBSHH, Group 3, and Group 4 (21). Molecular subgrouping reflects developmental aspects of the tumors’ cell of origin and has been shown to have prognostic significance.
Cancer predisposition syndromes’ importance has increasingly been recognized in pediatric neuro-oncology. According to Waszak et al. germline mutations in cancer predisposition genes account for about 5–6% of medulloblastoma diagnoses (22). Constitutional genetic defects are expected to result in deregulation of specific molecular pathways, leading to tumor development. Despite the significant amount of previous knowledge on inherited conditions predisposing to MB and the extensive molecular characterization of these tumors, limited attention has been given in the literature to their interconnection.
The main purpose of this review is to describe the association of cancer predisposition syndromes with MB molecular subgroups, including epidemiological, clinical, genetic, diagnostic, and therapeutic implications.
The authors conducted a literature search describing the issue of CNS tumors and cancer predisposition syndromes. Research studies were selected based on research topics (“cancer predisposition syndrome,” “brain tumor genetics,” “brain tumor cancer predisposition syndrome,” “medulloblastoma predisposition syndromes,” “medulloblastoma in childhood”) found in PubMed considering the last 10 years until April 2020. These studies were classified according to their relevance. In the selected studies the data were carefully evaluated, and they are described in detail and discussed in the following sections. The association between the different cancer predisposition syndromes described below and the related molecular subgroups of MB is summarized in Figure 1. The main cancer predisposition syndromes associated to pediatric MB and their related molecular, pathological, clinical, and prognostic features are summarized in Table 1.
Figure 1 Correlations between cancer predisposition syndromes and MB subtypes. (A) In Gorlin syndrome both PTCH1 and SUFU mutations have been associated to MB-SHH subgroup. Vismodegib and Sonidegib are selective antagonists of the transmembrane activator Smoothened (SMO). (B) In Li-Fraumeni syndrome los of TP53 finctions results in increased risk of developing MB-SHH subtype. (C) In Turcot syndrome, twotypes have been distinguished: Type 1 genetically related to the mutationof the mismatch repair genes and Type 2 related to APC mutation that are more commonly associated with MB-WNT subtype. (D) Pathogenic germline mutations in BRCA2, PALB2, GPR161, and ELP genes have been recently associated to an increased risk of developing different MB subtypes. (E) In Rubenstein-Taybi syndrome mutations in CREEBP and EP300 genes predispose to MB Group 3 onset.
Table 1 Cancer predisposition syndromes associated to pediatric medulloblastoma and their related molecular, pathological, clinical, and prognostic features.
Main features of MB subgroups are:
● Wingless (WNT) accounts for about 10% of diagnoses and is found mainly in girls with a peak between 10 and 12 years of age. The most common histological variant is classic. Approximately 85–90% of MBWNT harbor somatic mutations in exon 3 of Catenin beta 1 (CTNNB1), which causes stabilization and nuclear accumulation of β-catenin leading to uncontrolled activation of WNT signaling (23, 30). Patients with MBWNT without CTNNB1 mutations can harbor a mutant APC tumor suppressor gene, which is involved in the ubiquitination and consequently degradation of β-catenin (22). MBWNT have a low tendency to metastasize and patients under 16 years of age have an excellent prognosis. Therefore, some ongoing clinical trials, PNET5 and SJMB12, are currently investigating de-escalation of therapy (19).
● Sonic hedgehog (SHH) accounts for about 30% of all MB diagnoses and has a bimodal distribution, with peaks in children <3 years of age and in young adults >16 years of age (21). This subgroup affects both sexes almost equally with a slight predominance in males among infants (31). The histological variant is frequently desmoplastic/nodular. MBs-SHH harbor germline or somatic mutations in genes involved in SHH signaling pathway, leading to its constitutive activation, such as deletions or loss-of-function alterations in Patched 1 (PTCH1) (43% of patients) or Suppressor of fused (SUFU) (10%), activating mutations in Smoothened (SMO) (9%), amplification of GLI1/GLI2 (9%) or MYCN (7%) (23, 32). More recently, four SHH subtypes have been identified (SHHα, SHHβ, SHHγ, SHHδ) with distinct biological and clinical features (33). Older children with MBSHH can harbor germline or somatic Tumor Protein 53 (TP53) mutations, associated with a poor prognosis (25, 32).
● Group 3 accounts for about 25–28% of all MB diagnoses and is exclusively found in childhood, with a male sex predominance. It is associated with metastatic disease at diagnosis and with large cell/anaplastic histological variant. About 17% of Group 3 MBs harbor MYC amplification. Among MB subgroups, Group 3 is characterized by the poorest prognosis, especially in the presence of metastatic disease, isochromosome 17q, and MYC amplification (19).
● Group 4 is the most common MB molecular subgroup, accounting for about 35% of diagnoses. It is mostly found in males and more frequently associated to classic histological variant. It is characterized by an overall intermediate prognosis; however, a subset of patients with either chromosome 11 loss or 17 gain have an excellent prognosis (19).
Gorlin syndrome (GS) (OMIM #109400), also known as Gorlin-Goltz syndrome, or nevoid basal cell carcinoma syndrome (NBCCS), or basal cell nevus syndrome (BCNS), was first described by Gorlin and Goltz in 1960 (34). The incidence of GS reported is about 1 in 15.000 births (35) and is equal between males and females (36). The prevalence varies from 1:30,000 to 1:256,000 based on different reports (37–40). Prevalence data could be even greater since milder cases of GS could remain undiagnosed (41, 42).
GS is characterized by the onset of multiple jaw keratocysts, most frequent in the second decade of life, and/or basal cell carcinomas (BCCs), generally starting from the third decade. Sixty percent of all patients have a recognizable phenotype. More than 100 features have been associated with GS, and the most representative are listed in Table 2 (39, 40, 43).
Heterozygous germline mutations leading to the aberrant activation of SHH signaling are involved in GS, most frequently PTCH1, followed by SUFU. PTCH1 and SUFU mutations work at different levels by disabling SHH pathway signaling, which is normally active during brain development, thus promoting proliferation and inhibiting apoptosis (24, 44–47).
In 1963 Herzberg and Wiskemann first described the association between GS and MB that has been also confirmed by various published studies (48).
In the first large population based study of GS, Evans et al. investigated the incidence of GS in 173 consecutive cases of MB in the North-West of England between 1954 and 1989; they observed a 5% incidence of GS in MB patients with less than 5 years of age, conversely, the incidence of MB in the GS population considered in this study was 3.6% (49). The mean age at MB diagnosis was 2 years in GS patients, earlier than that described in the general population with sporadic MB (38). The desmoplastic/nodular and the extensive nodularity subtypes of MB are the most frequently described (50, 51). The risk of MB in subjects with germline mutations of PTCH1 reported in a large series of 115 individuals with related GS-PTCH1 was <2%, while individuals with GS and SUFU germline mutations presented an approximately 20 times higher risk (33%) (24).
Many individuals with GS are only recognized in adulthood. However, there are clinical signs that could appear early and guide the diagnosis, such as the presence of odontogenic keratocysts in children <20 years of age, basal cell carcinomas in persons <20 years of age, palmar or plantar pits, lamellar calcification of the falx cerebri, and MB with desmoplastic histology in combination with other major or minor criteria (52). Current diagnostic criteria for GS are summarized in Table 3. Diagnosis can be made if 2 major or 1 major and 2 minor criteria are fulfilled (36).
Surveillance protocols for individuals affected by GS have been proposed by several authors. As suggested in the consensus statement from the first international colloquium on GS, all individuals with GS should perform annually an assessment with a geneticist. A dermatological evaluation is also recommended annually until the first basal cell carcinoma is found, and then every 6 months. Baseline digital Panorex of jaw should be performed starting from the age of 3 years (or as soon as tolerated) and repeated annually before the detection of a first jaw cyst, and then every 6 months (until no jaw cyst for 2 years or until the age of 21).
A baseline echocardiographic evaluation is recommended to exclude cardiac fibromas; in females a pelvic ultrasound for fibromas is also recommended, starting from puberty.
A baseline spine film should be performed at age 1 or at time of diagnosis, and if a skeletal anomaly is found, it must be repeated every 6 months, or sooner if necessary. A routine developmental screening, including an assessment of vision, hearing, and speech, is recommended annually.
Annual brain MRI with contrast has been recommended until the age of 8 (52).
However, Smith and colleagues recently described the risk stratification of MB development between PTCH1 and SUFU mutation carriers, recommending the performance of brain MRI only for patients carrying SUFU mutation (24).
Expert consensus recommendations for tumor surveillance of gene carrier and family members were proposed in 2016 based on a literature review and discussion in the AACR Childhood Cancer Predisposition Workshop held in Boston, Massachusetts, in October 2016 (see Table 4) (53).
Vismodegib and Sonidegib are selective antagonists of the SHH pathway that act by binding to the transmembrane activator SMO, inhibiting the activation of the downstream SHH pathway.
Vismodegib is the first SHH pathway inhibitor approved by U.S. Food and Drug Administration (FDA) in 2012 and by European Medicines Agency in 2013 for the treatment of advanced or metastatic basal cell carcinomas (54, 55).
Sonidegib is approved by the FDA in adult patients for the treatment of locally advanced recurrent basal-cell carcinomas after radiation or surgery or for patients that cannot undergo surgery or radiotherapy (56).
A systemic review and meta-analysis about phase I and phase II Sonidegib and Vismodegib clinical trials highlighted that they are both well tolerated and with anti-tumor activity in MBSHH. The efficacy of Sonidegib was better than Vismodegib in pediatric MBSHH; however, this has been observed in 3 pediatric patients and further studies are needed for a reliable result (57).
Since SHH signaling has a crucial role during development, along with reports of younger patients treated with SMO inhibitors that show various growth plate complications, their use is not recommended in skeletally immature patients (58).
Li-Fraumeni Syndrome (LFS) (OMIM #151623) is one of the most aggressive cancer predisposition syndromes, first described in 1969 by Frederick Li and Joseph Fraumeni Jr (59). LFS is a rare autosomal dominantly inherited disorder caused by germline mutation of TP53, the “guardian of the genome” (60–62). Loss of p53 function in affected individuals is responsible for an increased risk of developing various solid and hematologic cancers (63). LFS has an estimated prevalence of 1 in 5,000 to 1 in 20,000 (64, 65). However, according to Andrade et al., prevalence estimates of the LFS could be higher (1 in 3,555–5,476), reflecting the complexity linked to a wide phenotype and a variable penetrance (66).
TP53 gene is located at chromosome 17p13.1 and is composed by 14 coding exons: 10 encode TP53 protein, one a non-coding exon, and three alternative exons (67). TP53 acts as a tumor suppressor gene: in unstressed cells TP53 is unstable and, after exposure to genotoxic stressors, it accumulates and induces the expression of various target genes involved in the regulation of critical cellular processes (growth suppression, apoptosis, DNA repair). Various mechanisms have been proposed to explain how the mutated TP53 protein contributes to tumor formation, including loss of TP53 tumor suppressor function and consequently the dysregulation of its target genes, the “dominant negative” effect in which the mutated TP53 protein inhibits wild-type TP53 protein and the “gain-of-function effect” in which the altered TP53 protein acquires new oncogenic properties.
Both children and adults affected by LFS have an increased risk of developing multiple primary tumors (68). The most frequent six “core” cancers, their relative prevalence estimates, and other less frequent types of tumor reported in LFS are summarized in Table 5 (60, 69, 70). Considering all ages, the most frequent tumor reported in LFS families is breast cancer, with a median age at onset of 33 years in females (65, 70–73). Soft tissue sarcomas and osteosarcoma are the most common tumors in children and adolescents with LFS (65, 70, 74). The most common type of CNS tumors is glioblastoma/astrocytoma (65, 71). Choroid plexus carcinomas (CPC) are more tightly associated with LFS since 45–100% of children with CPC show a germline TP53 mutation (65, 75–78).
Although MB has been described in families with LFS, its prevalence in TP53 carriers is not well known (79). About 5–10% of MBs present TP53 mutations; however, most of these are somatic and only 1% of MBs have been associated with germline TP53 mutations (22, 23, 80–82).
The correlation between TP53 mutation (both somatic and germline) and MB molecular subgroup has been investigated. In 2013, Zhukowa et al. analyzed a cohort of 397 individuals affected by MB (age 1.1 to 45 years) and reported a TP53 mutation almost exclusively in WNT and SHH subgroups while it was virtually absent in subgroups 3 and 4. They described a high difference in age distribution between MBSHH/TP53 mutated, which are almost exclusively between ages 5 and 18 years, and MBSHH/TP53 wild-type, that showed a bimodal distribution with peaks before 9 and after 18 years of age. Another interesting fact was that all individuals with TP53 germline mutation, therefore affected by LFS, had MBSHH, and no germline mutations were observed in MBWNT/TP53 mutated. For individuals with TP53 mutant tumors, a dramatic association between biologic subgroups and survival was observed. Patients with MBSHH/TP53 mutated showed a lower 5-year OS than those MBSHH without TP53 alteration (41% +/- 9% vs 81% +/- 5% respectively); on the contrary, individuals with MBWNT/TP53 mutated showed an almost similar 5-year OS than those MBWNT without TP53 alteration (90% +/- 9% vs 97% +/- 3% respectively), demonstrating that TP53 mutation status is much more crucial in the SHH subgroup. Within the limitation of the small cohort, no significant difference was observed between LFS children with MBSHH and MBSHH with somatic mutations of TP53 (25).
The original definition of LFS requires one individual with a sarcoma diagnosed under the age of 45 that has at least one first-degree relative (parent, sibling, or child) with a cancer of any kind diagnosed under the age of 45 and a third family member who is either a first- or second-degree relative in the same parental lineage (grandparent, aunt, uncle, niece, nephew, or grandchild) with any cancer diagnosed under the age of 45, or a sarcoma at any age (83, 84). The finding of TP53 mutations that did not fully respect classical criteria for LFS diagnosis led to the formulation of revised Chompret criteria. Individuals who meet classic and/or revised Chompret diagnostic criteria (Appendix A) should undergo TP53 genetic testing (65, 68, 71, 85).
Cancer screening in LFS individuals is challenging due to the wide range of associated tumors. Villani et al. in a prospective observational follow-up study of a comprehensive clinical surveillance protocol identified 89 carriers of TP53 pathogenic variants in 39 unrelated families and divided them in two groups: carriers who accepted surveillance (45%) and carriers who did not accept (55%); 21% of patients crossed over from the non-surveillance to the surveillance group for a total of 66% patients undergoing surveillance for a median of 32 months (86). Over an 11-year period, they identified 40 asymptomatic tumors in 32% of individuals who underwent surveillance and 60 symptomatic neoplasms in 88% patients who initially declined surveillance. The authors highlighted a significant survival advantage in individuals who underwent surveillance reporting 5-year OS of 88.8% in patients with the surveillance group and 59.6% in patients in the non-surveillance group. The Villani et al. 2016 version of the surveillance protocol for children with germline TP53 pathogenic variants is summarized in Table 6 (86). According to Ballinger et al. baseline whole-body magnetic resonance imaging can be used to identify early tumors in a highly cancer-prone population such as LFS patients, although further studies are needed (87).
Table 6 Villani et al. 2016 version of the surveillance protocol for children (birth to age 18 years) with germline TP53 pathogenic variants.
Currently, there is no targetable therapy against tumors of LFS patients available. Generally, it is recommended to avoid use of DNA-damaging agents such as ionizing radiation in order to reduce the risk of secondary tumors with the exception of high grade CNS tumors. Notably, CNS tumor patients with LFS tend to show an overall worse outcome when compared to patients with the same CNS tumors but without TP53 alteration (78, 88, 89). Even though no guidelines exist, LFS patients should be subjected to physical examination annually with particular attention to neurologic functions. Radiologic approaches without ionizing radiation such as whole-body MRI are currently under investigation (81, 86).
Turcot syndrome (TS) is defined by the association of colorectal cancer (CRC) and primary brain tumors and is one of the clinical manifestations of the mismatch repair cancer syndrome (OMIM # 276300). The first clinical report of the association of primary brain tumor and colorectal polyposis dates back to 1949 by Crail et al. (90). Ten years later Jacques Turcot described two siblings both affected by adenomatous colorectal polyposis and a malignant tumor of CNS, suggesting a common origin for this association (91). Two types of TS are known in literature. Type 1 (TS1) is characterized by the association between hereditary non-polyposis colorectal cancer (HNPCC), also called Lynch syndrome (LS), genetically related to the mutation of the mismatch repair (MMR) genes and CNS tumor (most frequently glioma). Type 2 (TS2) is characterized by the association of brain tumor and colorectal cancer due to familial adenomatous polyposis (FAP), caused by the mutation of the adenomatous polyposis coli (APC) gene, a suppressor gene in the long arm of chromosome 5 (92). Up to 10% of all CRC are inherited and among them a small number, commonly HNPCC or FAP, would be TS (93). Brain tumors in TS are mainly glioblastomas, associated with MMR genes mutations (TS1), and MB, associated with APC gene mutations (TS2).
There is a strong association between TS1 and LS. Lynch syndrome is caused by heterozygous germline mutations, inherited in an autosomal-dominant manner, in any of the MMR genes (MLH1; MSH2, MSH6, PMS1; PMS2), which are involved in DNA repair pathway. Unlike LS, TS1 is caused by homozygous mutations in the aforementioned genes (94, 95).
TS1 can clinically manifest with both gastrointestinal (diarrhea, constipation, and/or a positive fecal occult blood test) and neurological symptoms depending on which tumor arises first (95). Lynch syndrome is characterized by an average age of onset that is earlier than in sporadic cases (45 vs 63 years) and by CRC that develops most frequently proximal to splenic flexure and can often be synchronous and metachronous (94). Regarding the development of extracolonic cancers the most frequent are represented by carcinoma of the endometrium, ovary, stomach, small bowel, pancreas, hepatobiliary tract, brain, upper uroepithelial tract, sebaceous adenomas and carcinomas, and multiple keratoacanthomas (94). TS1 patients may have skin signs such as café-au-lait spots, resembling type 1 neurofibromatosis, which instead are not reported in TS2 patients (95).
MB cases within TS1 are less frequently described than those reported in the setting of TS2, while gliomas are the most frequently reported brain tumors in TS1 (96–99).
In 2007, Scott et al. described a 13-year-old girl with two colonic carcinomas and MB diagnosed at the age of 7 years caused by constitutional biallelic mutations in the mismatch repair gene MSH6, the first case of MB reported in literature that was caused by the aforementioned biallelic alteration (100). Another report by Lindsay et al. described a 12-year-old with colonic adenocarcinoma and classic MB due to biallelic deletion in PMS2 gene (101). To our knowledge, a correlation between TS1 and various subgroups of MB has not yet been highlighted.
Some aspects should be considered in TS1 diagnosis: individuals with TS1 are offspring of consanguineous in 20% of cases, with no family history of brain tumors or colon; in TS1 polyps are larger and less numerous than in TS2; in TS1 skin lesions are café-au-lait spots while in TS2 they resemble epidermal cysts (95).
According to the American College of Gastroenterology all newly diagnosed CRCs should be studied for MMR deficiency with immunohistochemical testing for the MLH1, MSH2, MSH6, PMS2 proteins and/or with testing for microsatellite instability. Individuals with a history of a tumor that is suspected to be determined by MMR deficiency, a known family mutation associated with LS, or a risk ≥5% of LS obtained with risk prediction models should undergo genetic testing: discovering LS may sometimes be the first step toward diagnosing TS1 (102).
Cancer surveillance guidelines for patients at risk of or affected by LS have been published while, to our knowledge, no specific guidelines regarding the brain tumor surveillance in patients with TS1 have been established (102).
Immunotherapeutic agents such as checkpoint inhibitors have been used in children with biallelic MMR deficiency glioblastoma multiforme, with encouraging results in some studies (26, 103). Checkpoint inhibitors seems to be effective in patients whose tumors harbor a high mutation load, resulting in the expression of neoantigens that act as a target for immunotherapy. Checkpoint inhibitors, through different mechanisms, activate T cells that recognize cancer cells as foreign by destroying them. Nivolumab is an anti-programmed death-1 (PD-1) directed checkpoint inhibitor, approved for the treatment of non-small-cell lung cancer and melanoma, and is being tested in various adult and pediatric tumors (103). Ipilimumab is an anti-cytotoxic T lymphocyte-associated protein 4 (CTLA-4) approved for the treatment of advanced melanoma and renal cell carcinoma and is also under clinical investigation in multiple adult and pediatric cancers (26). To our knowledge, there are no studies that have demonstrated the effectiveness of checkpoint inhibitors in children with MB, and therefore in those associated with MMR deficiency. Nivolumab and Ipilimumab are currently under investigation in a phase II trial of pediatric patients with high-grade CNS malignancies, including medulloblastoma (NCT03130959) (104).
APC mutation is generally inherited with an autosomal dominant manner for the development of FAP, while TS2 seems to require a biallelic loss of the APC gene (92, 105). Indeed, in patients with a germ-line alteration of APC, inactivation of the second copy of the gene seems to be crucial for brain tumor development.
Clinical findings are those typically associated with colorectal cancer and brain tumors, which can occur at different times. Patients with TS2 tend to develop a number of polyps, around thousands, and they frequently manifest gastrointestinal symptoms (similar to those mentioned for TS1). Either before or after the polyps are found, various neurological symptoms and signs can arise, depending on the location of the tumor: headache, vomiting, visual and/or hearing problems, and sensorimotor deficits. In TS2 patients brain tumors can occur without polyposis, and this could be explained by the hypothesis that affected individuals die before adenomatous polyps have time to develop. Skin lesions can also occur in patients with TS2 and are most commonly epidermal cysts.
About 40% of patients with TS develop MB (95). According to Hamilton et al., the relative risk of MB in patients with FAP was 92 times higher than in the general population (92). Surun et al. in their multicentric retrospective review of 12 patients, treated between 1988 and 2018 for MB with an identified or highly suspected APC germline pathogenic variant, described some recurrent features such as a constant classic histopathology, a frequent lateral location, and a predominant nonmetastatic status. They highlighted a strong correlation between APC-mutated MB and WNT subgroup, demonstrating their excellent outcome, as indeed have wild-type-MBWNT (106). An international multicenter study by Waszak et al., which included 1022 patients with MB, highlighted a close association between APC germline mutations and WNT subgroup; in this study germline APC mutations were found in five (71%) of seven CTNNB1-wild type MBWNT cases, representing 7.6% of all MBWNT, which together with the counterpart constituted by somatic mutations of CTNNB1 (89.4%), account for 97% of all MBWNT (22).
A key point in the diagnosis of TS2 patients is represented by family history. Individuals who have one or both parents with CRC diagnosed at an early age should be monitored for pre-cancerous colorectal polyps. According to the American College of Gastroenterology an individual with a history of ≥ 10 colorectal adenomatous polyps, or suggestive extracolonic manifestations, without a family history of an underlying pathogenic mutation, should be referred for genetic testing. In addition, the referral for genetic testing is also indicated for relatives of an individual with a known pathogenic mutation in order to establish the presence or absence of that specific mutation and to understand whether the relatives should be considered at-risk subjects (102).
The identification of family history of FAP and/or APC gene mutations may allow the clinician to perform surveillance in order to promptly identify the possible appearance of a brain tumor. An early diagnosis can allow an earlier treatment. However, it seems there is no advantage in terms of cost-effectiveness since not all individuals who present a CRC at an early age then develop a brain tumor and inversely (27, 95). Cancer surveillance guidelines for patients with FAP have been published, while, to our knowledge, no specific guidelines regarding brain tumor surveillance in patients with TS2 have been established (95, 102, 107).
There is currently no targeted therapy available against tumors arising in the setting of TS2.
Pathogenic germline mutations in BRCA2, PALB2, GPR161, and ELP genes have been recently associated with an increased risk of developing MB.
The international multicenter study by Waszak et al. identified germline BRCA2 mutations in 11 (1%) of 1022 patients with MB, 10 children and one adult, with a median age at diagnosis of 5.7 years (22). They observed compound heterozygosity at BRCA2 in 4 (36%) of 11 patients, of which all developed MBSHH and showed a worse Progression-Free Survival (PFS) and OS (25% at 5 years, respectively) compared to patients with heterozygous germline BRCA2 mutations, which instead showed a 100% OS and PFS, without secondary neoplasms. Germline mutations in BRCA2, compared with 53105 controls, were associated with increased risk of MBSHH and MBGroup3/4 (22).
BRCA2 biallelic mutations are known to be responsible for Fanconi Anemia (FA). The association of FA with MB has been described in literature (108). FA is a syndrome characterized by a chromosomal instability associated with congenital anomalies, bone marrow failure, and an increased risk of developing acute myeloid leukemia, myelodysplastic syndrome, and a number of solid tumors. It is a genetically and phenotypically heterogeneous disorder, inherited with an autosomal recessive pattern (rarely X-linked). We reported a novel BRCA2 mutation (c.2944_2944delA.) in a 35-month-old female with FA and diagnosis of two distinct MBs that had been previously treated for a nephroblastoma at the age of 15 months. Genetic testing on the patient’s DNA extracted from both peripheral blood and MB cells revealed the presence of compound heterozygosis for BRCA2 frameshift mutations. Molecular analysis showed a MBSHH for both the first- and the second-diagnosed MB. However differences in localization, more aggressive histology, and distinct gene expression pattern led to hypothesize a second distinct tumor rather than a distant relapse from the first one (109). The identification of SHH subgroup in FA patients may play a crucial role for their treatment with the use of targeted therapies, especially in these individuals extremely sensitive to conventional treatments.
In 2016 we described a case report of a 7-year-old girl with a classic histotype MBWNT and whose family history was negative for cancer (28). After six years of complete remission from MB the patient developed a secondary glioblastoma. Genetic testing for cancer predisposition syndromes was performed despite a negative family history for neoplasms, and we identified a maternal inherited heterozygous germline BRCA2 mutation, an unusual finding, since cases described in literature were non-WNT subgroups and, to our knowledge, this was the first case of BRCA2-mutated MBWNT reported so far.
Waszak et al. also reported pathogenic heterozygous germline PALB2 mutations in five (<1%) of 1022 patients with MB, of which there were 3 with MBSHH, 1 with MBGroup3, and 1 with MBGroup4. Five-year OS and PFS for patients with germline PALB2 mutations was 75% (22).
Interestingly, a correlation was described between germline BRCA2 and PALB2 mutations and homologous recombination repair deficiency (HRD)-like mutation spectrum, specifically for pediatric MBSHH (89% of cases), revealing HRD as potential biomarker for cancer predisposition in this subgroup (22). Furthermore, the association between germline BRCA2 and PALB2 with HRD-like mutation spectrum can be exploited to evaluate the susceptibility to combination therapies with PARP inhibitors.
Germline G protein-coupled receptor 161 (GPR161) mutations have recently been described by Begemann et al. as variants predisposing to pediatric MB (110). GPR161 is located on chromosome 1q24.2 and is involved in various aspects of embryonic development, including granule cell proliferation (111, 112). Proliferation of granule cells in cerebellum is regulated by SHH ligand and becomes abnormal when SHH-signaling pathway is constitutively activated. GPR161 acts as a SHH-pathway suppressor and its loss of function causes MB development (113). The frequency of germline GPR161 mutations in the general population is about 6 in 10,000 individuals (110). GPR161 biallelic inactivation, most frequently by copy-neutral loss of heterozygosity of chromosome 1q in individuals with heterozygous germline mutation, in the absence of other driver somatic events, has been associated with early TP53-wild-type-MBSHH development (110). According to Begemann et al., overall prevalence of germline GPR161 mutations among pediatric (age<18 years) and infant (age<4 years) patients with MBSHH was 3.4% and 5.5%, respectively (110). Copy-neutral loss of heterozygosity of chromosome 1q was never reported in GPR161 wild-type MBSHH; therefore, it can be considered a molecular feature (110).
Germline loss of function (LOF) variants in ELP1 have recently been identified in strong association with MBin pediatric age (114). ELP1 is a molecule that is part of the Elongator Complex, involved in epitranscriptomic tRNA modifications, whose main function is to modify wobble base uridines in the anticodon loop of tRNAs in order to ensure a correct translational elongation (29, 115–117). The loss of even a single subunit causes the dysregulation of the Elongator Complex with consequent proteome instability. The cerebellum is described as the site of greatest ELP1 expression during brain development (118, 119). According to Waszak et al., three consecutive mutational events are probably required for the development of ELP1-associated MBSHH: a heterozygous germline ELP1LOF variant; somatic biallelic inactivation of ELP1 with monoallelic inactivation of PTCH1 via loss of chromosome arm 9q and biallelic inactivation of the residual PTCH1 allele via a somatic mutation or focal deletion (114). Interestingly, Waszak et al. found a strong association between germline LOF variants in ELP1 and MBSHH subgroup, especially with SHHα subtype (114). Patients with ELP1-associated MBSHH showed a median age at diagnosis of 6.3 years, older than patients with MBSHH and germline SUFU or PTCH1 LOF variants and younger than those with MBSHH and germline TP53 mutations. These patients most frequently presented a desmoplastic nodular histotype and showed a favorable clinical outcome with 92% 5-year OS (114).
Rubinstein-Taybi syndrome (RSTS) is an extremely rare genetic disease, with an incidence of 1 in 100,000 to 125,000 live births, characterized by intellectual disability, unusual behavior, postnatal growth retardation, and multiple congenital anomalies, most frequently of the face and distal limbs (120, 121). RSTS is caused by a heterozygous mutation in cyclic-AMP regulated enhancer binding protein (CREBBP) gene, a transcriptional co-activator gene on chromosome 16p13.3, in about 60% of affected individuals (122), a submicroscopic deletion on chromosome 16p13.3 in about 10% of individuals (RSTS1, OMIM #180849) (123), alteration of E1A binding protein p300 (EP300) on chromosome 22q13.2 in about 5–10% of individuals (RSTS2, OMIM #613684) (124, 125). CREBBP gene and EP300 genes act as transcriptional co-activators and are involved in DNA repair, cellular growth, differentiation, apoptosis, and tumor suppression (126). According to Boot et al. that reviewed the literature from 1963 to 2017, a total of 132 tumors have been reported in 115 individuals with RSTS and MB was the second most frequent CNS neoplasm with 6 reported cases, after meningioma (121). However, an increased risk for malignant tumors in RSTS could not be confirmed given the small numbers of affected individuals reported in literature, and additional studies are warranted.
With the advent of next generation sequencing (NGS) and implementation of genetic testing for adult cancer predisposition syndromes into routine clinical practice, cancer genetics research has extended the use of molecular testing for tumor and germline analysis in pediatric cancer patients. Molecular diagnosis of cancer predisposition syndromes can influence cancer screening initiation or frequency, to either prevent or detect cancer at an earlier and more treatable stage, and directly impact treatment decisions. However, even if medulloblastoma can be associated with rare hereditary cancer predisposition syndromes, screening guidelines for genetic counseling and testing of pediatric patients are not available (23). For genetic testing of cancer predisposition syndromes, different approaches are being used, and, currently, most molecular diagnostics laboratories that offer NGS are performing targeted gene panel testing or clinical whole exome sequencing (WES), more rarely whole genome sequencing (WGS). A multi-gene panel usually includes high and moderate penetrance genes and, sometimes, some low or of yet unknown risk genes, offering the advantage of identifying germline pathogenic variants in genes that would normally not be tested based on the patient’s diagnosis. However, it is possible that variants in genes not included in the panels contribute to the cancer risk and WES or WGS can be used to explore other genetic basis of familial syndromes in a more extensive way, permitting to identify new high- and moderate-risk genes of cancer predisposition. Genome-wide approaches generate huge amounts of genetic data and it remains a challenge to interpret the identified variants. Such data interpretation needs close collaboration among molecular geneticists, bioinformaticians, and clinicians. However, as sequencing costs are decreasing and computer and technological resources are expanding, genome-wide analysis in clinical practice will become more common.
MB is the most frequent malignant CNS tumor in children, and additionally to the sporadic form, MB can occur in association with a cancer predisposition syndrome. Knowledge of the clinical findings, etiopathogenic basis, and diagnostic criteria of each syndrome described in this review allow the pediatrician to make a correct diagnosis, start cancer surveillance, and suspect precociously a MB on its onset, providing a prompt treatment. Conversely, when MB is diagnosed, the correct identification/detection of a cancer predisposition syndrome can allow the clinician to make a more appropriate and complete management of treatment involving several medical specialists in a multidisciplinary team. The molecular studies conducted in the last years have evidenced an association between the various cancer predisposition syndromes and the different MB subgroups. Knowing these relationships can help further clarify the difference not only from a biological point of view but also in prognostic terms. Notably, the extremely poor outcome of MBSHH in children expressing germline TP53 mutations has already been reported. Based on the findings described by Waszak et al., pediatric MBSHH development could be explained by a high genetic predisposition (about 40%); therefore, the effort to carry out genetic testing and surveillance program for affected patients and families in this subgroup becomes even more crucial.
According to Waszak et al. we suggest that patients with MBSHH should be tested for germline TP53 (when older than 3 years), SUFU and PTCH1 mutations (when younger than 3 years), and if negative, also for germline mutations in BRCA2 and PALB2. Furthermore, we suggest that patients with MBSHH should be tested for germline ELP1 mutations, especially those presenting outside of infancy, and for germline GPR161 mutations, particularly those presenting in infancy. We suggest, also, genetic counselling for germline APC mutations in children with MBWNT.
Considering that only 5–6% of MB are associated with cancer predisposition syndromes, our current knowledge is probably still limited. Given the importance that the recognition of a cancer predisposition syndrome can have in the management of a child with MB, we suggest to extend genetic testing also in patients with family history for cancer and/or finding of a dysmorphic phenotype. Knowledge of the associations between molecular subgroups and cancer predisposition syndromes can also be useful in clarifying the differences in terms of therapeutic vulnerability, guiding the development of new targeted therapies. Finally, the comprehension of these biological and molecular differences can help to further improve cancer surveillance measures, with the aim of guaranteeing the best quality of care for the patients.
RC and GB equally contributed to this manuscript. EM, AP, ZMB, FN, GC, EP, EA, MR, ML, and AC contributed to the finishing of the work. FN provided the figure. AM, ACar, EF, LB, and FL revised it critically for important intellectual content. All authors finally approved the version to be published and agreed to be accountable for all aspects of the work in ensuring that questions related to the accuracy or integrity of any part of the work are appropriately investigated and resolved. All authors contributed to the article and approved the submitted version.
The authors declare that the research was conducted in the absence of any commercial or financial relationships that could be construed as a potential conflict of interest.
The authors thank Megan Eckley for helping in the English final version and the Association “Il Laboratorio di Chiara”.
The Supplementary Material for this article can be found online at: https://www.frontiersin.org/articles/10.3389/fonc.2020.566822/full#supplementary-material
1. RARECAREnet. http://www.rarecarenet.eu/rarecarenet/.
2. Kombogiorgas D. The Medulloblastoma Book. In: Capitolo 1: introduction to medulloblastoma. New York: Nova Science Publishers, Inc. (2014). p. 1–12.
3. Gupta N, Banerjee A, Haas-Kogan D. Chapter 5: embryonal tumors. In:. Pediatric CNS Tumors. Springer (2016). p. 93–104.
4. Koeller K, Rushing E. From the archives of the AFIP: medulloblastoma: a comprehensive review with radiologic-pathologic correlation. Radiographics (2003) 23(6):1613–37. doi: 10.1148/rg.236035168
5. Fouladi M, Gajjar A, Boyett JM, Walter AW, Thompson SJ, Merchant TE, et al. Comparison of CSF cytology and spinal magnetic resonance imaging in the detection of leptomeningeal disease in pediatric medulloblastoma or primitive neuroectodermal tumor. J Clin Oncol (1999) 17(10):3234–7. doi: 10.1200/JCO.1999.17.10.3234
6. Louis DN, Ohgaki H, Wiestler OD, Cavenee WK, Burger PC, Jouvet A, et al. The 2007 WHO classification of tumours of the central nervous system. Acta Neuropathologica (2007) 114(2):97–109. doi: 10.1007/s00401-007-0243-4
7. De Haas T, Hasselt N, Troost D, Caron H, Popovic M, Zadravec-Zaletel L, et al. Molecular risk stratification of medulloblastoma patients based on immunohistochemical analysis of MYC, LDHB, and CCNB1 expression. Clin Cancer Res (2008) 14(13):4154–60. doi: 10.1158/1078-0432.CCR-07-4159
8. Park AK, Lee SJ, Phi JH, Wang KC, Kim DG, Cho BK, et al. Prognostic classification of pediatric medulloblastoma based on chromosome 17p loss, expression of MYCC and MYCN, and Wnt pathway activation. Neuro Oncol (2012) 14(2):203–14. doi: 10.1093/neuonc/nor196
9. Roussel MF, Robinson GW. Role of MYC in medulloblastoma. Cold SpringHarb Perspect Med (2013) 3(11):a014308. doi: 10.1101/cshperspect.a014308
10. Ramaswamy V, Remke M, Bouffet E, Bailey S, Clifford SC, Doz F, et al. Risk stratification of childhood medulloblastoma in the molecular era: the current consensus. Acta Neuropathol (2016) 131(6):821–31. doi: 10.1007/s00401-016-1569-6
11. Gajjar A, Chintagumpala M, Ashley D, Kellie S, Kun LE, Merchant TE, et al. Risk-adapted craniospinal radiotherapy followed by high-dose chemotherapy and stem-cell rescue in children with newly diagnosed medulloblastoma (St Jude Medulloblastoma-96): long-term results from a prospective, multicenter trial. Lancet Oncol (2006) 7(10):813–20. doi: 10.1016/S1470-2045(06)70867-1
12. Oyharcabal-Bourden V, Kalifa C, Gentet JC, Frappaz D, Edan C, Chastagner P, et al. Standard-risk medulloblastoma treated by adjuvant chemotherapy followed by reduced-dose craniospinal radiation therapy: A French Society of Pediatric Oncology study. J Clin Oncol (2005) 23(21):4726–34. doi: 10.1200/JCO.2005.00.760
13. Packer RJ, Gajjar A, Vezina G, Rorke-Adams L, Burger PC, Robertson PL, et al. Phase III study of craniospinal radiation therapy followed by adjuvant chemotherapy for newly diagnosed average-risk medulloblastoma. J Clin Oncol (2006) 24(25):4202–8. doi: 10.1200/JCO.2006.06.4980
14. Lannering B, Rutkowski S, Doz F, Pizer B, Gustafsson G, Navajas A, et al. Hyperfractionated versus conventional radiotherapy followed by chemotherapy in standard-risk medulloblastoma: Results from the randomized multicenter HIT-SIOP PNET 4 trial. J Clin Oncol (2012) 30(26):3187–93. doi: 10.1200/JCO.2011.39.8719
15. Gandola L, Massimino M, Cefalo G, Solero C, Spreafico F, Pecori E, et al. Hyperfractionated accelerated radiotherapy in the Milan strategy for metastatic medulloblastoma. J Clin Oncol (2009) 27(4):566–71. doi: 10.1200/JCO.2008.18.4176
16. Jakacki R, Burger P, Zhou T, Holmes EJ, Kocak M, Onar A, et al. Outcome of children with metastatic medulloblastoma treated with carboplatin during craniospinal radiotherapy: a Children’s Oncology Group Phase I/II study. J Clin Oncol (2012) 30(21):2648–53. doi: 10.1200/JCO.2011.40.2792
17. Kool M, Korshunov A, Remke M, Jones DTW, Schlanstein M, Northcott PA, et al. Molecular subgroups of medulloblastoma: An international meta-analysis of transcriptome, genetic aberrations, and clinical data of WNT, SHH, Group 3, and Group 4 medulloblastomas. Acta Neuropathol (2012) 123(4):473–84. doi: 10.1007/s00401-012-0958-8
18. Korshunov A, Remke M, Kool M, Hielscher T, Northcott PA, Williamson D, et al. Biological and clinical heterogeneity of MYCN-amplified medulloblastoma. Acta Neuropathol (2012) 123(4):515–27. doi: 10.1007/s00401-011-0918-8
19. Shih DJH, Northcott PA, Remke M, Korshunov A, Ramaswamy V, Kool M, et al. Cytogenetic prognostication within medulloblastomasubgroups. J Clin Oncol (2014)32(9):886–96. doi: 10.1200/JCO.2013.50.9539
20. Louis DN, Perry A, Reifenberger G, von Deimling A, Figarella-Branger D, Cavenee WK, et al. The 2016 World Health Organization Classification of Tumors of the Central Nervous System: a summary. Acta Neuropathologica (2016) 131(6):803–20. doi: 10.1007/s00401-016-1545-1
21. Taylor MD, Northcott PA, Korshunov A, Remke M, Cho YJ, Clifford SC, et al. Molecular subgroups of medulloblastoma: The current consensus. Acta Neuropathol (2012) 123(4):465–72. doi: 10.1007/s00401-011-0922-z
22. Waszak SM, Northcott PA, Buchhalter I, Robinson GW, Sutter C, Groebner S, et al. Spectrum and prevalence of genetic predisposition in medulloblastoma: a retrospective genetic study and prospective validation in a clinical trial cohort. Lancet Oncol (2018) 19(6):785–98. doi: 10.1016/S1470-2045(18)30242-0
23. Northcott PA, Buchhalter I, Morrissy AS, Hovestadt V, Weischenfeldt J, Ehrenberger T, et al. The whole-genome landscape of medulloblastomasubtypes. Nature (2017) 547(7663):311–7. doi: 10.1038/nature22973
24. Smith MJ, Beetz C, Williams SG, Bhaskar SS, O’Sullivan J, Anderson B, et al. Germline mutations in SUFU cause Gorlin syndrome-associated childhood medulloblastoma and redefine the risk associated with PTCH1 mutations. J Clin Oncol (2014) 32(36):4155–61. doi: 10.1200/JCO.2014.58.2569
25. Zhukova N, Ramaswamy V, Remke M, Pfaff E, Shih DJH, Martin DC, et al. Subgroup-specific prognostic implications of TP53 mutation inmedulloblastoma. J Clin Oncol (2013) 31(23):2927–35. doi: 10.1200/JCO.2012.48.5052
26. Larouche V, Atkinson J, Albrecht S, Al E. Sustained complete response of recurrent glioblastoma to combined checkpoint inhibition in a young patient with constitutional mismatch. Pediatr Blood Cancer (2018) 65(12):e27389. doi: 10.1002/pbc.27389
27. Bruwer Z, Algar U, Vorster A, Fieggen K, Davidson A, Goldberg P, et al. Predictive genetic testing in children: Constitutional mismatch repair deficiency cancer predisposing syndrome. J Genet Couns (2014) 23(2):147–55. doi: 10.1007/s10897-013-9659-2
28. Di Giannatale A, Carai A, Cacchione A, Marrazzo A, Dell’Anna VA, Colafati GS, et al. Anomalous vascularization in a Wnt medulloblastoma: A case report. BMC Neurol (2016) 16:103. doi: 10.1186/s12883-016-0632-1
29. Dauden MI, Kosinski J, Kolaj-Robin O, Desfosses A, Ori A, Faux C, et al. Architecture of the yeast Elongator complex. EMBO Rep (2017) 18(2):264–79. doi: 10.15252/embr.201643353
30. Gilbertson R. Medulloblastoma: signalling a change in treatment. Lancet Oncol (2004) 5(4):209–18. doi: 10.1016/S1470-2045(04)01424-X
31. Gajjar AJ, Robinson GW. Medulloblastoma—translating discoveries from the bench to the bedside. Nat Rev Clin Oncol (2014) 11(12):714–22. doi: 10.1038/nrclinonc.2014.181
32. Kool M, Jones DTW, Jäger N, Northcott PA, Pugh TJ, Hovestadt V, et al. Genome sequencing of SHH medulloblastoma predicts genotype-related response to smoothened inhibition. Cancer Cell (2014) 25(3):393–405.
33. Northcott PA, Hielscher T, Dubuc A, MacK S, Shih D, Remke M, et al. Pediatric and adult sonic hedgehog medulloblastomas are clinically and molecularly distinct. Acta Neuropathol (2011) 122(2):231–40. doi: 10.1007/s00401-011-0846-7
34. Gorlin R, Goltz R. Multiple nevoid basal-cell epithelioma, jaw cysts and bifid rib. A syndrome. N Engl J Med (1960) 5(262):908–12. doi: 10.1056/NEJM196005052621803
35. Evans DG, Howard E, Giblin C, Clancy T, Spencer H, Huson SM, et al. Birth incidence and prevalence of tumor-prone syndromes: Estimates from a UK family genetic register service. Am J Med Genet Part A (2010) 152A(2):327–32. doi: 10.1002/ajmg.a.33139
36. Jones EA, Sajid MI, Shenton A, Evans DG. Basal Cell Carcinomas in Gorlin Syndrome: A Review of 202 Patients. J Skin Cancer (2011) 2011:217378. doi: 10.1155/2011/217378
37. Endo M, Fujii K, Sugita K, Saito K, Kohno Y, Miyashita T. Nationwide survey of nevoid basal cell carcinoma syndrome in Japan revealing the low frequency of basal cell carcinoma. Am J Med Genet Part A (2012) 158A(2):351–7. doi: 10.1002/ajmg.a.34421
38. Evans DGR, Ladusans EJ, Rimmer S, Burnell LD, Thakker N, Farndon PA. Complications of the naevoid basal cell carcinoma syndrome: Results of a population based study. J Med Genet (1993) 30(6):460–4. doi: 10.1136/jmg.30.6.460
39. Kimonis VE, Goldstein AM, Pastakia B, Yang ML, Kase R, Digiovanna JJ, et al. Clinical manifestations in 105 persons with nevoid basal cell carcinoma syndrome. Am J Med Genet (1997) 69(3):299–308. doi: 10.1002/(SICI)1096-8628(19970331)69:3<299::AID-AJMG16>3.0.CO;2-M
40. Shanley S, Ratcliffe J, Hockey A, Haan E, Oley C, Ravine D, et al. Nevoid basal cell carcinoma syndrome: Review of 118 affected individuals. Am J Med Genet (1994) 50(3):282–90. doi: 10.1002/ajmg.1320500312
41. Thomas N, Vinod V, George A, Varghese A. Gorlin–Goltz syndrome: An often missed diagnosis. Ann Maxillofac Surg (2016) 6(1):120–4. doi: 10.4103/2231-0746.186148
42. Visioli F, Martins CAM, Heitz C, Rados PV, Sant’Ana Filho M. Is Nevoid Basal Cell Carcinoma Syndrome Really So Rare?: Proposal for an Investigative Protocol Based on a Case Series. J Oral Maxillofac Surg (2010) 68(4):903–8. doi: 10.1016/j.joms.2009.03.032
43. Ragge N, Salt A, Collin J, Michalski A, Farndon PA. Gorlin syndrome: The PTCH gene links ocular developmental defects and tumour formation. Br J Ophthalmol (2005) 89(8):988–91. doi: 10.1136/bjo.2004.061390
44. Farndon PA, Del Mastro RG, Kilpatrick MW, Evans DRG. Location of gene for Gorlin syndrome. Lancet (1992) 339(8793):581–2. doi: 10.1016/0140-6736(92)90868-4
45. Hahn H, Wicking C, Zaphiropoulos PG, Gailani MR, Shanley S, Chidambaram A, et al. Mutations of the human homolog of drosophila patched in the nevoid basal cell carcinoma syndrome. Cell (1996) 85(6):841–51. doi: 10.1016/S0092-8674(00)81268-4
46. Johnson RL, Rothman AL, Xie J, Goodrich LV, Bare JW, Bonifas JM, et al. Human homolog of patched, a candidate gene for the basal cell nevus syndrome. Science (1996) 272(5268):1668–71. doi: 10.1126/science.272.5268.1668
47. Pastorino L, Ghiorzo P, Nasti S, Battistuzzi L, Cusano R, Marzocchi C, et al. Identification of a SUFU germline mutation in a family with Gorlin syndrome. Am J Med Genet A (2009) 149A(7):1539–43. doi: 10.1002/ajmg.a.32944
48. Herzberg J, Wiskemann A. Die funfte Phakomatose. Basal-zellnaevus mit familiarer Belastung und Medulloblastom. Dermatologica (1963) 126:106–23. doi: 10.1159/000254913
49. Evans DGR, Farndon PA, Burnell LD, Rao Gattamaneni H, Birch JM. The incidence of Gorlin syndrome in 173 consecutive cases of medulloblastoma. Br J Cancer (1991) 64(5):959–61. doi: 10.1038/bjc.1991.435
50. Amlashi SFA, Riffaud L, Brassier G, Morandi X. Nevoid basal cell carcinoma syndrome: Relation with desmoplastic medulloblastoma in infancy: A population-based study and review of the literature. Cancer (2003) 98(3):618–24. doi: 10.1002/cncr.11537
51. Garrè ML, Cama A, Bagnasco F, Morana G, Giangaspero F, Brisigotti M, et al. Medulloblastoma variants: Age-dependent occurrence and relation to gorlin syndrome-a new clinical perspective. Clin Cancer Res (2009) 15(7):2463–71. doi: 10.1158/1078-0432.CCR-08-2023
52. Bree AF, Shah MR. Consensus statement from the first international colloquium on basal cell nevus syndrome (BCNS). Am J Med Genet A (2011) 155A(9):2091–7. doi: 10.1002/ajmg.a.34128
53. Foulkes WD, Kamihara J, Evans DGR, Brugières L, Bourdeaut F, Molenaar JJ, et al. Cancer surveillance in Gorlin syndrome and rhabdoid tumor predisposition syndrome. Clin Cancer Res (2017) 23(12):e62–7. doi: 10.1158/1078-0432.CCR-17-0595
54. Sekulic A, Migden MR, Oro AE, Dirix L, Lewis KD, Hainsworth JD, et al. Efficacy and safety of vismodegib in advanced basal-cell carcinoma. N Engl J Med (2012) 366(23):2171–9. doi: 10.1056/NEJMoa1113713
55. Tang JY, Ally MS, Chanana AM, Mackay-Wiggan JM, Aszterbaum M, Lindgren JA, et al. Inhibition of the hedgehog pathway in patients with basal-cell nevus syndrome: final results from the multicentre, randomised, double-blind, placebo-controlled, phase 2 trial. Lancet Oncol (2016) 17(12):1720–31. doi: 10.1016/S1470-2045(16)30566-6
56. Jain S, Song R, Xie J. Sonidegib: Mechanism of action, pharmacology, and clinical utility for advanced basal cell carcinomas. OncoTargets Ther (2017) 10:1645–53. doi: 10.2147/OTT.S130910
57. Li Y, Song Q, Day BW. Phase I and phase II sonidegib and vismodegib clinical trials for the treatment of paediatric and adult MB patients: a systemic review and meta-analysis. Acta Neuropathol Commun (2019) 7(1):123. doi: 10.1186/s40478-019-0773-8
58. Robinson GW, Kaste SC, Chemaitilly W, Bowers DC, Laughton S, Smith A, et al. Irreversible growth plate fusions in children with medulloblastoma treated with a targeted hedgehog pathway inhibitor. Oncotarget (2017) 8(41):69295–302. doi: 10.18632/oncotarget.20619
59. Li FP, Fraumeni JJ. Rhabdomyosarcoma in children: epidemiologic study and identification of a familial cancer syndrome. J Natl Cancer Inst (1969) 43(6):1365–73.
60. Malkin D, Li FP, Strong LC, Fraumeni JF, Nelson CE, Kim DH, et al. Germ line p53 mutations in a familial syndrome of breast cancer, sarcomas, and other neoplasms. Science (1990) 250(4985):1233–8. doi: 10.1126/science.1978757
61. Srivastava S, Zou Z, Pirollo K, Blattner W, Chang EH. Germ-line transmission of a mutated p53 gene in a cancer-prone family with Li-Fraumeni syndrome. Nature (1990) 348(6303):747–9. doi: 10.1038/348747a0
62. Evans DG, Birch JM, Narod SA. Is CHEK2 a cause of the Li-Fraumeni syndrome? J Med Gen (2008) 45(1):63–4. doi: 10.1136/jmg.2007.054700
63. Mai PL, Best AF, Peters JA, DeCastro R, Khincha PP, Loud JT, et al. Risks of first and subsequent cancers among TP53 mutation-carriers in the NCI LFS cohort. Cancer (2016) 122(23):3673–81. doi: 10.1002/cncr.30248
64. Lalloo F, Varley J, Ellis D, Moran A, O’Dair L, Pharoah P, et al. Prediction of pathogenic mutations in patients with early-onset breast cancer by family history. Lancet (2003) 361(9363):1101–2. doi: 10.1016/S0140-6736(03)12856-5
65. Gonzalez KD, Noltner KA, Buzin CH, Gu D, Wen-Fong CY, Nguyen VQ, et al. Beyond li fraumeni syndrome: Clinical characteristics of families with p53 germline mutations. J Clin Oncol (2009) 27(8):1250–6. doi: 10.1200/JCO.2008.16.6959
66. de Andrade KC, Frone MN, Wegman-Ostrosky T, Khincha PP, Kim J, Amadou A, et al. Variable population prevalence estimates of germline TP53 variants: A gnomAD-based analysis. Hum Mutation (2019) 40(1):97–105. doi: 10.1002/humu.23673
67. Hainaut P, Pfeifer GP. Somatic TP53 mutations in the era of genome sequencing. Cold Spring Harb Perspect Med (2016) 6(11):a026179. doi: 10.1101/cshperspect.a026179
68. Ruijs MWG, Verhoef S, Rookus MA, Pruntel R, Van Der Hout AH, Hogervorst FBL, et al. TP53 germline mutation testing in 180 families suspected of Li-Fraumeni syndrome: Mutation detection rate and relative frequency of cancers in different familial phenotypes. J Med Genet (2010) 47(6):421–8. doi: 10.1136/jmg.2009.073429
69. Nichols KE, Malkin D, Garber JE, Fraumeni J, Li FP. Germ-line p53 mutations predispose to a wide spectrum of early-onset cancers. Cancer Epidemiol Biomarkers Prev (2001) 10(2):83–7.
70. Valdez JM, Nichols KE, Kesserwan C. Li-Fraumeni syndrome: a paradigm for the understanding of hereditary cancer predisposition. Br J Haematol (2017) 176(4):539–52. doi: 10.1111/bjh.14461
71. Bougeard G, Renaux-Petel M, Flaman JM, Charbonnier C, Fermey P, Belotti M, et al. Revisiting Li-Fraumeni syndrome from TP53 mutation carriers. J Clin Oncol (2015) 33(21):2345–52. doi: 10.1200/JCO.2014.59.5728
72. Masciari S, Dillon DA, Rath M, Robson M, Weitzel JN, Balmana J, et al. Breast cancer phenotype in women with TP53 germline mutations: A Li-Fraumeni syndrome consortium effort. Breast Cancer Res Treat (2012) 133(3):1125–30. doi: 10.1007/s10549-012-1993-9
73. Melhem-Bertrandt A, Bojadzieva J, Ready KJ, Obeid E, Liu DD, Gutierrez-Barrera AM, et al. Early onset HER2-positive breast cancer is associated with germline TP53 mutations. Cancer (2012) 118(4):908–13. doi: 10.1002/cncr.26377
74. Ognjanovic S, Olivier M, Bergemann TL, Hainaut P. Sarcomas in TP53 germline mutation carriers: A review of the IARC TP53 database. Cancer (2012) 118(5):1387–96. doi: 10.1002/cncr.26390
75. Custodio G, Taques GR, Figueiredo BC, Gugelmin ES, Figueiredo MMO, Watanabe F, et al. Increased incidence of choroid plexus carcinoma due to the germline TP53 R337H mutation in southern Brazil. PloS One (2011) 6(3):e18015. doi: 10.1371/journal.pone.0018015
76. Seidinger AL, Mastellaro MJ, Paschoal Fortes F, Godoy Assumpção J, Aparecida Cardinalli I, Aparecida Ganazza M, et al. Association of the highly prevalent TP53 R337H mutation with pediatric choroid plexus carcinoma and osteosarcoma in Southeast Brazil. Cancer (2011) 117(10):2228–35. doi: 10.1002/cncr.25826
77. Krutilkova V, Trkova M, Fleitz J, Gregor V, Novotna K, Krepelova A, et al. Identification of five new families strengthens the link between childhood choroid plexus carcinoma and germline TP53 mutations. Eur J Cancer (2005) 41(11):1597–603. doi: 10.1016/j.ejca.2005.01.026
78. Tabori U, Shlien A, Baskin B, Levitt S, Ray P, Alon N, et al. TP53 alterations determine clinical subgroups and survival of patients with choroid plexus tumors. J Clin Oncol (2010) 28(12):1995–2001. doi: 10.1200/JCO.2009.26.8169
79. Pearson ADJ, Craft AW, Ratcliffe JM, Birch JM, Morris-Jones P, Roberts DF. Two families with the Li-Fraumeni cancer family syndrome. J Med Genet (1982) 19(5):362–5. doi: 10.1136/jmg.19.5.362
80. Schwalbe EC, Lindsey JC, Nakjang S, Crosier S, Smith AJ, Hicks D, et al. Novel molecular subgroups for clinical classification and outcome prediction in childhood medulloblastoma: a cohort study. Lancet Oncol (2017) 18(7):958–71. doi: 10.1016/S1470-2045(17)30243-7
81. Villani A, Tabori U, Schiffman J, Shlien A, Beyene J, Druker H, et al. Biochemical and imaging surveillance in germline TP53 mutation carriers with Li-Fraumeni syndrome: A prospective observational study. Lancet Oncol (2011) 12(6):559–67. doi: 10.1016/S1470-2045(11)70119-X
82. Tabori U, Baskin B, Shago M, Alon N, Taylor MD, Ray PN, et al. Universal poor survival in children with medulloblastoma harboring somatic TP53 mutations. J Clin Oncol (2010) 28(8):1345–50. doi: 10.1200/JCO.2009.23.5952
83. Kratz CP, Achatz MI, Brugieres L, Frebourg T, Garber JE, Greer MLC, et al. Cancer screening recommendations for individuals with Li-Fraumeni syndrome. Clin Cancer Res (2017) 23(11):e38–45. doi: 10.1158/1078-0432.CCR-17-0408
84. Li FP, Fraumeni JF, Mulvihill JJ, Blattner WA, Dreyfus MG, Tucker MA, et al. A Cancer Family Syndrome in Twenty-four Kindreds. Cancer Res (1988) 48(18):5358–62.
85. Chompret A, Abel A, Stoppa-Lyonnet D, Brugières L, Pagès S, Feunteun J, et al. Sensitivity and predictive value of criteria for p53 germline mutation screening. J Med Genet (2001) 38(1):43–7. doi: 10.1136/jmg.38.1.43
86. Villani A, Shore A, Wasserman JD, Stephens D, Kim RH, Druker H, et al. Biochemical and imaging surveillance in germline TP53 mutation carriers with Li-Fraumeni syndrome: 11 year follow-up of a prospective observational study. Lancet Oncol (2016) 17(9):1295–305. doi: 10.1016/S1470-2045(16)30249-2
87. Ballinger ML, Best A, Mai PL, Khincha PP, Loud JT, Peters JA, et al. Baseline Surveillance in Li-Fraumeni Syndrome Using Whole-BodyMagnetic Resonance Imaging: A Meta-analysis. JAMA Oncol(2017) 3(12):1634–39. doi: 10.1001/jamaoncol.2017.1968
88. Michaeli O, Tabori U. Pediatric high grade gliomas in the context of cancer predisposition syndromes. J Korean Neurosurg Soc (2018) 61(3):319–32. doi: 10.3340/jkns.2018.0031
89. Tabori U, Laberge A, Ellezam B, Carret A-S. Cancer Predisposition in Children with Brain Tumors: PediatricNeuro-oncology. New York: Springer (2015). p. 69–89.
90. Crail HW. Multiple primary malignancies arising in the rectum, brain, andthyroid; report of a case. U S Nav Med Bull (1949) 49(1):123–8.
91. Turcot J, Després JP, St. Pierre F. Malignant tumors of the central nervous system associated with familial polyposis of the colon - Report of two cases. Dis Colon Rectum (1959) 2:465–8. doi: 10.1007/BF02616938
92. Hamilton SR, Liu B, Parsons RE, Papadopoulos N, Jen J, Powell SM, et al. The molecular basis of turcot’s syndrome. N Engl J Med (1995) 332(13):839–47. doi: 10.1056/NEJM199503303321302
93. Haggar FA, Boushey RP. Colorectal cancer epidemiology: Incidence, mortality, survival, and risk factors. Clin Colon Rectal Surg (2009) 22(4):191–7. doi: 10.1055/s-0029-1242458
94. Lynch HT, De la Chapelle A. Hereditary colorectal cancer. New Engl J Med (2003) 348:919–32. doi: 10.1056/NEJMra012242
95. Khattab A, Monga D. Turcot Syndrome. StatPearls [Internet] Treasure Isl StatPearls Publ (2020). Jan.
96. Dupuis M, Verellen-Dumoulin C. Gastrointestinal polyposis and nonpolyposissyndromes. N Engl J Med (1995) 332(22):1518. doi: 10.1056/NEJM199506013322215
97. Giunti L, Cetica V, Ricci U, Giglio S, Sardi I, Paglierani M, et al. Type A microsatellite instability in pediatric gliomas as an indicator of Turcot syndrome. Eur J Hum Genet (2009) 17(7):919–27. doi: 10.1038/ejhg.2008.271
98. Toledano H, Goldberg Y, Kedar-Barnes I, Baris H, Porat RM, Shochat C, et al. Homozygosity of MSH2 c.1906G→C germline mutation is associated with childhood colon cancer, astrocytoma and signs of Neurofibromatosis type I. Familial Cancer. Springer (2009).
99. Bougeard G, Charbonnier F, Moerman A, Martin C, Ruchoux MM, Drouot N, et al. Early-onset brain tumor and lymphoma in MSH2-deficient children. Am J Hum Genet (2003) 72(1):213–6. doi: 10.1086/345297
100. Scott RH, Pritchard-Jones K, Rahman N, Mansour S, Kumar D, MacSweeney F. Medulloblastoma, acute myelocytic leukemia and colonic carcinomas in a child with biallelic MSH6 mutations. Nat Clin Pract Oncol (2007) 4(2):130–4. doi: 10.1038/ncponc0719
101. Lindsay H, Jubran RF, Wang L, Kipp BR, May WA. Simultaneous colonic adenocarcinoma and medulloblastoma in a 12-year-old with biallelic deletions in PMS2. J Pediatr (2013) 163(2):601–3. doi: 10.1016/j.jpeds.2013.03.007
102. Syngal S, Brand RE, Church JM, Giardiello FM, Hampel HL, Burt RW. ACG clinical guideline: Genetic testing and management of hereditary gastrointestinal cancer syndromes. Am J Gastroenterol (2015) 110(2):223–62. doi: 10.1038/ajg.2014.435
103. Bouffet E, Larouche V, Campbell B, Merico D, de Borja R, Aronson M, et al. Immune checkpoint inhibition for hypermutant glioblastoma multiforme resulting from germline biallelic mismatch repair deficiency. J Clin Oncol (2016) 34(19):2206–11. doi: 10.1200/JCO.2016.66.6552
104. Kabir TF, Kunos CA, Villano JL, Villano JL, Chauhan A. Immunotherapy for medulloblastoma: current perspectives. Immunotargets Ther (2020) 9:57–77. doi: 10.2147/ITT.S198162
105. Bussey H. Familial polyposis coli: family studies, histopathology, differentialdiagnosis, and results of treatment. Vol. 10. Baltimore: Balt Johns Hopkins Univ Press (1975).
106. Surun A, Varlet P, Brugières L, Lacour B, Faure-Conter C, Leblond P, et al. Medulloblastomas associated with an APC germline pathogenic variant share the good prognosis of CTNNB1-mutated medulloblastomas. Neuro Oncol (2020) 22(1):128–38. doi: 10.1093/neuonc/noz154
107. Vasen HFA, Möslein G, Alonso A, Aretz S, Bernstein I, Bertario L, et al. Guidelines for the clinical management of familial adenomatous polyposis (FAP). Gut (2008) 57(5):704–13. doi: 10.1136/gut.2007.136127
108. Tischkowitz MD, Chisholm J, Gaze M, Michalski A, Rosser EM. Medulloblastoma as a First Presentation of Fanconi Anemia. J Pediatr Hematol Oncol (2004) 26(1):52–5. doi: 10.1097/00043426-200401000-00016
109. Miele E, Mastronuzzi A, Po A, Carai A, Alfano V, Serra A, et al. Characterization of medulloblastoma in Fanconi Anemia: A novel mutation in the BRCA2 gene and SHH molecular subgroup. Biomark Res (2015) 3:13. doi: 10.1186/s40364-015-0038-z
110. Begemann M, Waszak S, Robinson G, Jäger N, Sharma T, Knopp C, et al. Germline GPR161 mutations predispose to pediatric medulloblastoma. J Clin Oncol (2020) 38(1):43–50. doi: 10.1200/JCO.19.00577
111. Leung T, Humbert J, Stauffer A, Giger KE, Chen H, Tsai HJ, et al. The orphan G protein-coupled receptor 161 is required for left-right patterning. Dev Biol (2008) 323(1):31–40. doi: 10.1016/j.ydbio.2008.08.001
112. Hwang S, White K, Somatilaka B, Shelton JM, Richardson JA, Mukhopadhyay S. The G protein-coupled receptor Gpr161 regulates forelimb formation, limb patterning and skeletal morphogenesis in a primary cilium-dependent manner. Development (2018) 145(1):dev154054. doi: 10.1242/dev.154054
113. Mukhopadhyay S, Wen X, Ratti N, Loktev A, Rangell L, Scales SJ, et al. The ciliary G-protein-coupled receptor Gpr161 negatively regulates the sonic hedgehog pathway via cAMP signaling. Cell (2013) 152(1-2):210–23. doi: 10.1016/j.cell.2012.12.026
114. Waszak SM, Robinson GW, Gudenas BL, Smith KS, Forget A, Kojic M, et al. Germline Elongator mutations in Sonic Hedgehog medulloblastoma. Nature (2020) 580(7803):396–401. doi: 10.1038/s41586-020-2164-5
115. Hawer H, Hammermeister A, Ravichandran KE, Glatt S, Schaffrath R, Klassen R. Roles of elongator dependent tRNA modification pathways in neurodegeneration and cancer. Genes (Basel) (2018) 10(1):19. doi: 10.3390/genes10010019
116. Johansson MJO, Xu F, Byström AS. Elongator—a tRNA modifying complex that promotes efficient translational decoding. Biochim Biophys Acta Gene Regul Mech (2018) 1861(4):401–8. doi: 10.1016/j.bbagrm.2017.11.006
117. Setiaputra DT, Cheng DT, Lu S, Hansen JM, Dalwadi U, Lam CH, et al. Molecular architecture of the yeast Elongator complex reveals an unexpected asymmetric subunit arrangement. EMBO Rep (2017) 18(2):280–91. doi: 10.15252/embr.201642548
118. Miller JA, Ding SL, Sunkin SM, Smith KA, Ng L, Szafer A, et al. Transcriptional landscape of the prenatal humanbrain. Nature (2014) 508(7495):199–206. doi: 10.1038/nature13185
119. Carter RA, Bihannic L, Rosencrance C, Hadley JL, Tong Y, Phoenix TN, et al. A Single-Cell Transcriptional Atlas of the Developing Murine Cerebellum. Curr Biol (2018) 28(18):2910–20.e2. doi: 10.1016/j.cub.2018.07.062
120. Rubinstein J, Taybi H. Broad Thumbs and Toes and Facial Abnormalities: A Possible Mental Retardation Syndrome. Am J Dis Child (1963) 105:588–608. doi: 10.1001/archpedi.1963.02080040590010
121. Boot M, van Belzen M, Overbeek L, Al E. Benign and malignant tumors in Rubinstein-Taybi syndrome. Am J Med Genet A (2018) 176(3):597–608. doi: 10.1002/ajmg.a.38603
122. Petrij F, Giles R, Dauwerse H, Al E. Rubinstein-Taybi Syndrome Caused by Mutations in the Transcriptional Co-Activator CBP. Nature (1995) 376(6538):348–51. doi: 10.1038/376348a0
123. Hennekam R. Rubinstein-Taybi Syndrome. Eur J Hum Genet (2006) 14(9):981–5. doi: 10.1038/sj.ejhg.5201594
124. Fergelot P, Van Belzen M, Van Gils J, Al E. Phenotype and genotype in 52 patients with Rubinstein-Taybi syndromecaused by EP300 mutations. Am J Med Genet A (2016) 170(12):3069–82. doi: 10.1002/ajmg.a.37940
125. Roelfsema J, White S, Ariyürek Y, Al E. Genetic Heterogeneity in Rubinstein-Taybi Syndrome: Mutations in Both the CBP and EP300 Genes Cause Disease. Am J Hum Genet (2005) 76(4):572–80. doi: 10.1086/429130
126. Goodman R, Smolik S. CBP/p300 in cell growth, transformation, and development. Genes Dev (2000) 14(13):1553–77.
Classic diagnostic criteria
A proband with Sarcoma diagnosed under the age of 45 years
AND
A first degree relative with any cancer under 45 years
AND
Another first or second degree relative with either cancer under 45 years or a sarcoma at any age
Chompret diagnostic criteria (revised)
A proband with an LFS spectrum tumor (soft tissue sarcoma, osteosarcoma, brain tumors, pre-menopausal breast cancer, adrenal cortical carcinoma, leukemia, lung bronchoalveolar cancer) before 46 years
AND one of the following criteria:
At least one first- or second-degree relative with an LFS tumor (except breast cancer, if the proband has breast cancer) before 56 years or with multiple primary tumors
OR
A proband with multiple primary tumors (except multiple breast tumors), two of which belong to the LFS tumor spectrum and the first of which occurred before 46 years
OR
A proband with adrenal cortical carcinoma or choroid plexus carcinoma or embryonal anaplastic subtype rhabdomyosarcoma independent of the family history
OR
Breast cancer before the age of 31 years
Keywords: pediatric brain tumors, cancer predisposition, hereditary neoplastic syndromes, cancer syndromes, medulloblastoma, cancer genes
Citation: Carta R, Del Baldo G, Miele E, Po A, Besharat ZM, Nazio F, Colafati GS, Piccirilli E, Agolini E, Rinelli M, Lodi M, Cacchione A, Carai A, Boccuto L, Ferretti E, Locatelli F and Mastronuzzi A (2020) Cancer Predisposition Syndromes and Medulloblastoma in the Molecular Era. Front. Oncol. 10:566822. doi: 10.3389/fonc.2020.566822
Received: 28 May 2020; Accepted: 30 September 2020;
Published: 29 October 2020.
Edited by:
Zoltan Patay, St. Jude Children's Research Hospital, United StatesReviewed by:
Joseph Louis Lasky, Cure 4 The Kids, United StatesCopyright © 2020 Carta, Del Baldo, Miele, Po, Besharat, Nazio, Colafati, Piccirilli, Agolini, Rinelli, Lodi, Cacchione, Carai, Boccuto, Ferretti, Locatelli and Mastronuzzi. This is an open-access article distributed under the terms of the Creative Commons Attribution License (CC BY). The use, distribution or reproduction in other forums is permitted, provided the original author(s) and the copyright owner(s) are credited and that the original publication in this journal is cited, in accordance with accepted academic practice. No use, distribution or reproduction is permitted which does not comply with these terms.
*Correspondence: Angela Mastronuzzi, YW5nZWxhLm1hc3Ryb251enppQG9wYmcubmV0
Disclaimer: All claims expressed in this article are solely those of the authors and do not necessarily represent those of their affiliated organizations, or those of the publisher, the editors and the reviewers. Any product that may be evaluated in this article or claim that may be made by its manufacturer is not guaranteed or endorsed by the publisher.
Research integrity at Frontiers
Learn more about the work of our research integrity team to safeguard the quality of each article we publish.