- 1Division of Hematology and Oncology, Department of Internal Medicine, University of Cincinnati College of Medicine, Cincinnati, OH, United States
- 2Department of Cancer Biology, University of Cincinnati College of Medicine, OH, United States
- 3Division of Inflammation Research, Center for Molecular Medicine, Jichi Medical University, Tochigi, Japan
- 4Institute for Advanced Biosciences, Keio University, Tsuruoka, Japan
- 5Department of Cell Biology and Center for Cell Dynamics, Johns Hopkins University School of Medicine, Baltimore, MD, United States
- 6Department of Neurosurgery, Brain Tumor Center at UC Gardner Neuroscience Institute, Cincinnati, OH, United States
Despite advances in targeted therapeutics and understanding in molecular mechanisms, metastasis remains a substantial obstacle for cancer treatment. Acquired genetic mutations and transcriptional changes can promote the spread of primary tumor cells to distant tissues. Additionally, recent studies have uncovered that metabolic reprogramming of cancer cells is tightly associated with cancer metastasis. However, whether intracellular metabolism is spatially and temporally regulated for cancer cell migration and invasion is understudied. In this review, we highlight the emergence of a concept, termed “membraneless metabolic compartmentalization,” as one of the critical mechanisms that determines the metastatic capacity of cancer cells. In particular, we focus on the compartmentalization of purine nucleotide metabolism (e.g., ATP and GTP) at the leading edge of migrating cancer cells through the uniquely phase-separated microdomains where dynamic exchange of nucleotide metabolic enzymes takes place. We will discuss how future insights may usher in a novel class of therapeutics specifically targeting the metabolic compartmentalization that drives tumor metastasis.
Introduction
Many metastatic processes require dynamic changes in cell motility—i.e., epithelial-mesenchymal transition (EMT), detachment of cells from the primary tumor; local invasion of the basement membrane; intravasation and extravasation; or invasion in a distant site (1, 2). Genetic mutations and changes in transcriptional landscape that increase metastatic capacity have been identified [reviewed in (3, 4)]. EMT is one key initiating step for metastasis, converting epithelial cancer (i.e., carcinoma) to highly motile and invasive mesenchymal cell phenotype, rendering spatial asymmetry that corresponds to the emergence of lamellipodial and filopodial membrane extensions at the leading edge (i.e., the front end) (5–7), via dynamic signaling components. For example, localized activation of RAS and PI3K at the leading edge promotes cellular polarization, directional cell migration, and random cell migration (8, 9). Recent studies have demonstrated that rewiring of metabolic pathways in cancer and cancer stem cells via oncogenic signaling and/or EMT is another key regulator for cell motility and metastasis [reviewed in (10)]. However, the mechanism of this spatiotemporal regulation of metabolic enzymes in migrating cells remained unclear until recently (11). In this review, we highlight an emerging concept of membraneless metabolic compartmentalization and its possible roles in tumor invasion and metastasis.
Membrane-Bound Organelles for Metabolic Compartmentalization
There are several critical roles for membrane-bound compartmentalization (12) (Figure 1A). For instance, metabolic compartmentalization within the peroxisome is crucial for sequestering toxic metabolites and for isolating the harsh conditions required for peroxisomal oxidative reactions from other more fragile cellular compartments (13). Sequestration of metabolic intermediates generally acts to preclude undesirable off-target enzymatic reactions and interference from other enzymatic pathways (14, 15) (Figure 1A); a network-based analysis conducted by Alam et al. suggests that the organelle-level compartmentalization of metabolic reactions relieves the inhibitory effect of unrestricted metabolite diffusion within cells by up to half (16). Additionally, changes in metabolic compartmentalization can evoke coordinated cellular responses such as apoptosis, in which case the release of cytochrome c from mitochondria into the cytosol initiates the apoptotic cascade (17) (Figure 1A).
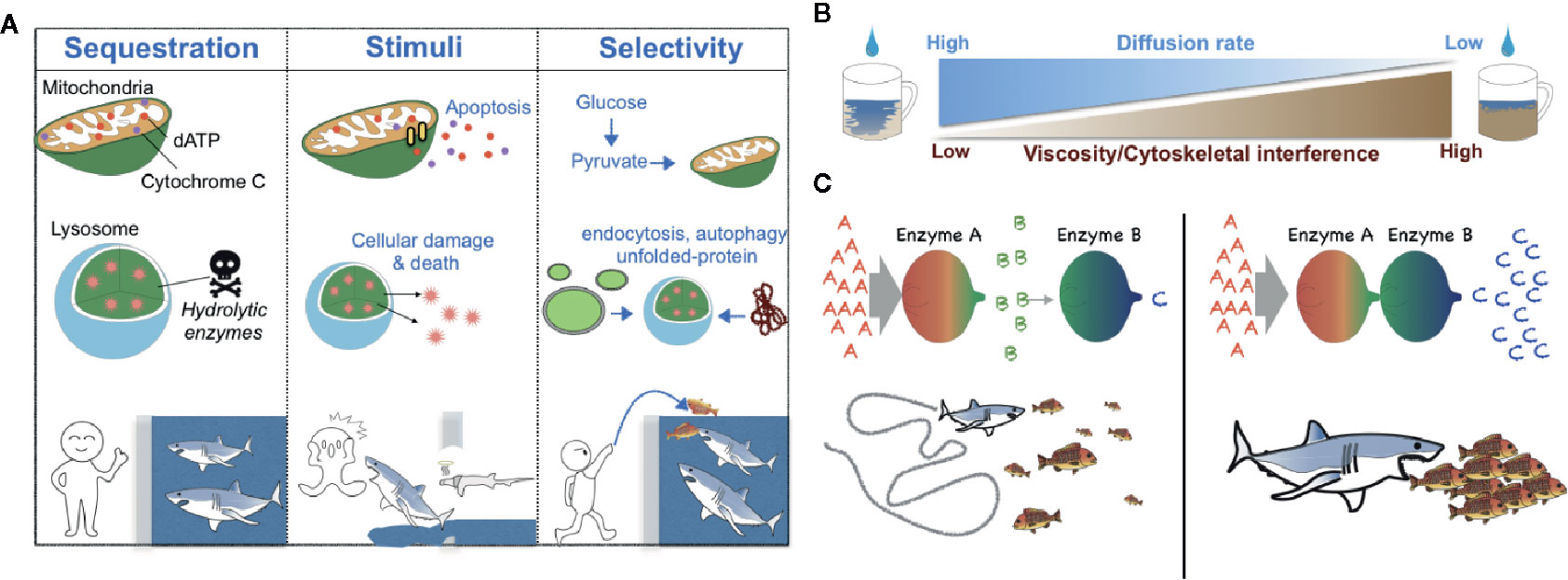
Figure 1 Schematic models of the biological roles of compartmentalization, viscosity, and local protein concentrations. (A) Metabolic compartmentalization by membrane-bound organelles confines metabolites to organelles to increase reaction efficiency and protect cellular contents—analogous to potential prey that may be protected from sharks by confining the predators to a shark tank (left). Release of organellar contents into the cytoplasm can elicit changes in cell fate (e.g., induction of an apoptotic program by cytochrome C and dATP or cellular damage mediated by lysosomal enzymes)—analogous to sharks that can either attack prey or themselves die when there is a breach in a shark tank (middle). Generally, the transportation of molecules into membrane-bound organelles is highly selective and regulated—analogous to sharks that may be fed without opening oneself up to the possibility of bodily harm by introducing prey into a shark tank from a distance (right). (B) A schematic diagram of the negative correlation between the fluid viscosity of a medium and the diffusion rate of metabolites within it. (C). A schematic diagram of the effect of the local concentration or relative proximity of enzymes belonging to the same metabolic pathway. When Enzymes A and B are spatially separated, Enzyme B can receive only small amounts of its substrate b, which is generated by the distant Enzyme A. Thus, Enzyme B produces only small amounts of its enzymatic product c—analogous to a shark that can catch only a relatively small number of fish when the fish are sparse (left). However, when Enzymes A and B are in close proximity, Enzyme B can receive much more of its substrate b and thus produce much more of its product c—analogous to a shark that can capture more fish when the fish are schooling (right).
Membraneless Compartments for Metabolic Processes
Cell biologists of the late 19th and early 20th centuries considered the cytosol to be merely a “bag of enzymes” that functioned within the limits of diffusion and in which metabolites and proteins were free to randomly diffuse throughout the cell (18). However, accumulating evidence now points to the ability of cells to generate metabolic compartmentalization even in the cytoplasm. The cell may accomplish this form of compartmentalization through several mechanisms that derive from the physical nature of the cytoplasm, which differs locally in parameters of fluid viscosity, resulting in differential local diffusion rates of metabolites and proteins and differential local rates of molecular interactions (Figure 1B). In a term first coined by Paul Srere in 1985 (19), membraneless multi-enzyme complexes are referred to as metabolons (20–22).
Local Diffusion Rate
The cytoplasm of the cell is a viscous solution of ions, macromolecules, and cytoskeletal proteins. A number of metabolites could experience very slow diffusion rates in certain cellular contexts (18, 23–25). The diffusion rate of small molecules such as ions is reported to be reduced by less than two-fold in cytoplasm-like conditions compared to water (26), whereas the diffusion of larger macromolecules like nucleotides is hindered by greater than three-fold (27, 28). Likewise, the diffusion of polypeptides such as green fluorescent protein (GFP) has been found to be slowed by 3-14 times in bacterial cell cytosols compared to diffusion in water (29–31) and molecules larger than 60 kDa travel half the distance of smaller molecules (32). Additionally, increased nonspecific associations of larger macromolecules (e.g., proteins) with other solutes in the cytoplasm (e.g., polymerized actin, microtubules) are an impediment to diffusion (33–38) (Figure 1B).
The anomalous diffusion of macromolecules can be slowed by a tight mesh structure of actins and microtubules (Figure 1), which causes rapid jumps in the solute’s trajectory as it passes between contiguous actins and tubulin fibers (39). Furthermore, cross-linked actin filaments can transit into a gel phase, thereby dramatically increasing the elastic and viscous properties of the cytoplasm (40). Lastly, diffusion rates in the cytoplasm can also be impeded dramatically at spatiotemporal locations where liquid-liquid phase separation occurs (41).
Local Protein Concentration
The increased local concentration of enzymes belonging to the same enzymatic pathway can protect highly labile metabolic intermediates via substrate channeling, which here acts as a mechanism to decrease reaction time (14, 22, 42) (Figure 1C). To achieve substrate channeling, cells control the proximity of metabolic enzymes in an elegant way and thereby allow for tunable metabolic reactions that can respond dynamically to the evolving cellular status.
Several enzymes involved in glycolysis [e.g., glyceraldehyde 3-phosphate dehydrogenase (GAPDH), fructose-bisphosphate aldolase (ALDA), and phosphofructokinase (PFK)] have been discovered to be bound to actin fibers in the cellular cytoplasm, thereby forming long chains of metabolic enzymes belonging to the same glycolytic pathway that can promote rapid formation of pathway intermediates (20, 25, 42–47). In Hudder et al. the authors documented the large percentage of proteins that are bound to the polymerized filamentous actin (F-actin) cytoskeleton of CHO cells (20). Cell permeabilization released ~12% of the total protein content within the cell. By contrast, pre-incubating the cells with latrunculin B, which sequesters monomeric actin and thus diminishes the F-actin cytoskeleton, followed by permeabilization led to the release of nearly 40% of the proteins. This suggests that large fractions of proteins were bound to actin fibers (20).
The Leading Edge as a Membraneless Organellar Compartment
The ruffling lamellipodium and actin-rich lamellum were described during the late 1960s in motile fibroblast cells in culture (48). As described in the previous section, the diffusion of nucleotides between the cytoplasm and the leading edge is likely hindered by the dense actin and microtubule mesh and the surrounding network of filament-bound macromolecules. Importantly, the formation and maintenance of a leading edge requires the input of purine nucleotides like guanosine 5’-triphosphate (GTP) and adenosine 5’-triphosphate (ATP).
The assembly of microtubules relies upon the binding of GTP to tubulin dimers, which are subsequently incorporated into the GTP-cap that resides at the plus-end of growing microtubules (49). Conversely, GTP hydrolysis results in GDP-bound tubulin dimers and its dissociation from the microtubules. Actin polymerization requires the binding of ATP to G-actin monomers, which may subsequently be incorporated into the barbed end of the growing F-actin polymeric chain. When ATP is hydrolyzed, the ADP-bound subunit conformation of the monomeric actin unit changes, leading to the dissociation of G-actin monomers from F-actin. In chick ciliary neuron culture, approximately 50% of global ATP hydrolysis is associated with the maintenance of the actin cytoskeleton (50).
The physical nature of the leading edge and the elaborated metabolic utility of purine nucleotides suggest a possible requirement for localized biosynthesis of ATP and GTP. Strikingly, the compartmentalization of an entire metabolic pathway within the leading edge was discovered in 2019 (11). In the following section, we will briefly introduce the GTP metabolic enzymes and their significance in cell motility.
GTP Biosynthetic Enzymes in Cancer Cell Dissemination
IMPDH
GTP and ATP can be synthesized through either the energy-saving salvage pathway or the de novo biosynthesis pathway (Figure 2A). The de novo biosynthetic process consumes glucose to synthesize the intermediate metabolite inosine 5’-monophosphate (IMP) after > 17 enzymatic steps. IMP is then used as a substrate by both the ATP-biosynthetic or GTP-biosynthetic branches of the de novo pathway. IMP dehydrogenase (IMPDH) is the rate-limiting, NAD+-dependent first step in GTP biosynthesis and commits IMP to the GTP pathway. IMPDH has been extensively studied in cancer biology since the 1950s (51–61), and is the bona fide target of the FDA-approved immunosuppressant mycophenolic acid (MPA) and its prodrug form mycophenolate mofetil (MMF, CellCept) (62), which is also used as an immunosuppressant in the clinic. There are two isotypes of IMPDH in humans, IMPDH isotype 1 (IMPDH1) and IMPDH isotype 2 (IMPDH2), of which IMPDH2 has been reported to be more highly upregulated in cancers (62–64).
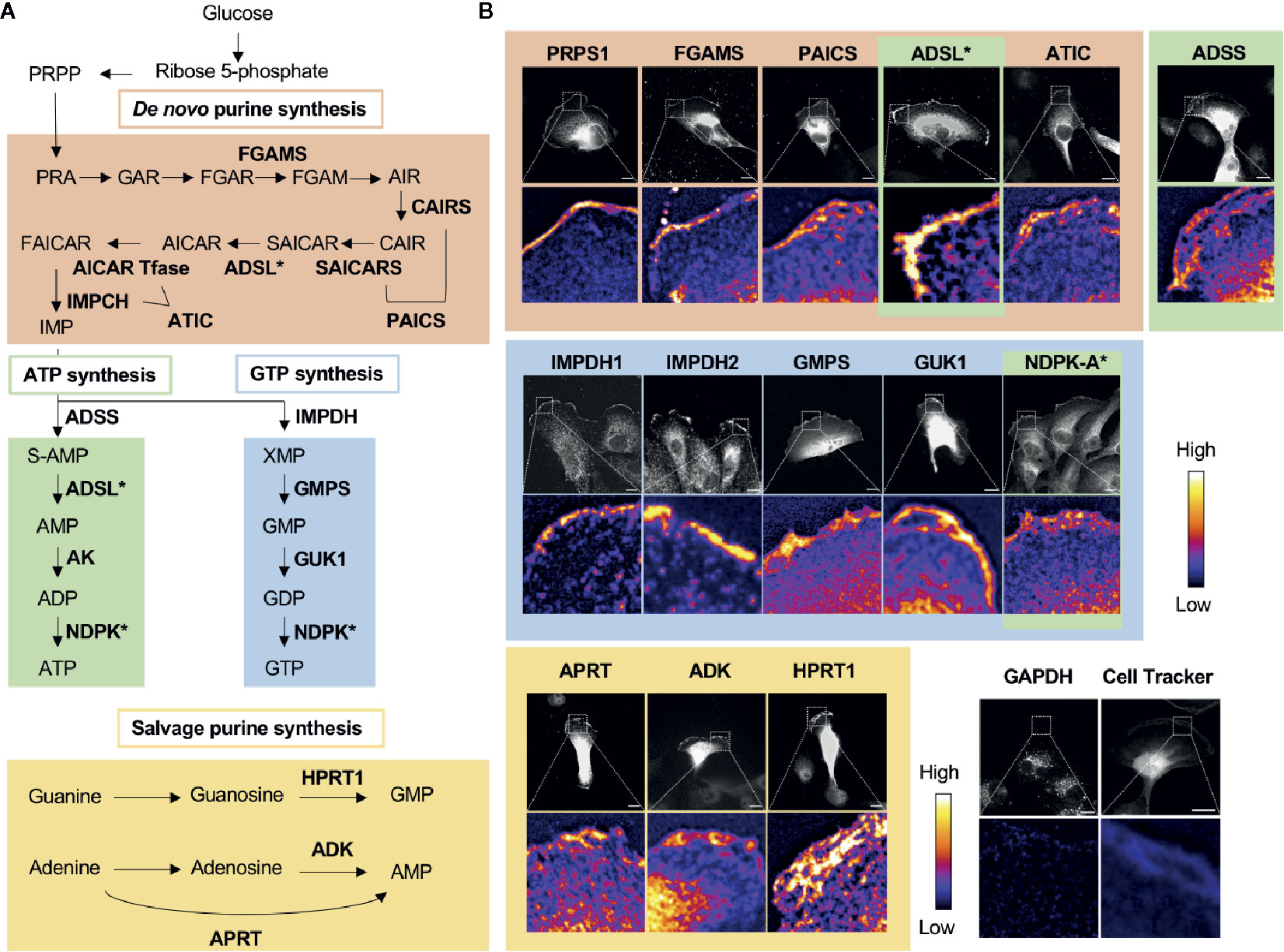
Figure 2 Nucleotide metabolic enzymes localize at the lamellipodial membrane. (A) Purine nucleotide biosynthesis schematic. The 9 enzymatic steps of de novo biosynthesis (orange), the ATP branch (green), GTP branch (blue), and selected salvage pathway enzymes (yellow). (B) Selected immunofluorescence staining images of purine biosynthetic enzymes (color scheme matches part A) localizing to the leading edge of migrating kidney cancer cells. Relative intensity map of immunofluorescence staining shown in bottom micrograph with intensity scale at the right. Immunofluorescence of GAPDH and fluorescence imaging of Cell Tracker dye, which stain intracellular proteins, show major signals in cytoplasm, which indicate that the localization of purine metabolic enzymes at the leading edge is specific. PRPS, phosphoribosyl pyrophosphate synthetase; FGAMS: phosphoribosyl formylglycinamidine synthase; PAICS, phosphoribosyl aminoimidazole succinocarboxamide synthetase; ADSL, adenylosuccinate lyase; ATIC, 5-amino-4-imidazolecarboxamide ribonucleotide transformylase/IMP cyclohydrolase; ADSS, adenylosuccinate synthase; AK, adenylate kinase; IMPDH, inosine-5′-monophosphate dehydrogenase; GMPS, GMP synthase; GUK1, guanylate kinase 1; NDPK, nucleoside-diphosphate kinase; APRT, adenine phosphoribosyltransferase; ADK, adenosine kinase; HPRT1, Hypoxanthine-guanine phosphoribosyltransferase; GAPDH, glyceraldehyde 3-phosphate dehydrogenase.
Several studies show the functional role of IMPDH enzyme in cell motility. In human fibroblasts, inhibiting IMPDH via MPA treatment was shown to lead to decreased adhesion and migration along with dysregulated cytoskeletal proteins (65). Similarly, MPA treatment led to a decrease in the migration and invasion of gastric cancer cells in vitro (66) and to a decrease in the EMT, in vitro migration, and metastatic seeding of prostate cancer cells (67). IMPDH inhibition was reported to decrease the fraction of GTP-bound RAC1, RHOA, and RHOC, the molecular switch proteins responsible for polarizing cells during migration (68), in melanoma cells (69). It is worth noting that many of these studies used long-term treatment with IMPDH inhibitor (e.g., over 16 h), which is likely to change both the transcriptional landscape and the phenotypic status (e.g., cell cycle arrest, apoptosis, or senescence) of the cellular targets. Nonetheless, although more careful analyses may be required to verify the direct effects of GTP on cancer cell motility, most of the evidence thus far points to a correlation between GTP biosynthesis and cell motility.
Enzymes Downstream of IMPDH
The enzymes that lie downstream of IMPDH during GTP biosynthesis, GMP synthase (GMPS) and nucleoside diphosphate kinase-A (NME1 or NDPK-A), a prominent member of the nucleoside diphosphate kinase family (70), have additionally been implicated in migration and metastasis. In human melanoma samples, increased GMPS protein expression was found in metastatic lesions compared to localized tumors, and the pharmacological inhibition of GMPS decreased melanoma cell proliferation and invasion, both in vitro and in vivo (71). By contrast, NDPK-A expression in tumors has long been controversial. The gene name NME1 [NM23/NDP kinase (non-metastatic clone 23)] (72) originated with the observed inverse correlation of its increased expression with decreased metastatic potential in mouse models and some human cancers (70, 73). However, the following studies showed that NDPK-A expression was correlated proportionally with metastasis in neuroblastomas (74) and renal cell carcinomas (75). Also, NDPK-A knockout in mice was observed to lead to diminished tumor formation in vivo and decreased lung colonization in xenograft models (76).
Metabolic Compartmentalization of Purine Biosynthetic Enzymes Within the Leading Edge of Highly Motile Cancer Cells
In 2019, we found the striking localization of de novo purine biosynthetic enzymes as well as IMPDH1 and IMPDH2 at the membrane of the leading edge in metastatic renal cell carcinoma cell lines (11) (Figure 2B). All three enzymes of the GTP biosynthetic branch after IMPDH—GMPS, guanylate kinase (GUK1), and NDPK-A—were enriched significantly at the leading edge. Interestingly, we found a substantially greater colocalization at the leading edge of the cells in comparison to the cell body. Additionally, an essential enzyme for the GTP salvage pathway, HPRT1, was also enriched at the leading edge. Thus, all the GTP biosynthetic enzymes responsible for making GTP from IMP were localized at the leading edge (Figure 2B).
While not all enzymes of the ATP biosynthetic pathway were tested, three of the four enzymes that act sequentially in the pathway to convert IMP to ATP were also found to enrich at the leading edge (Figure 2B). The ATP salvage enzymes APRT and ADK were also found to enrich at this location (11) (Figure 2B).
Given that all 16 enzymes analyzed localized to the leading edge and colocalized with IMPDH1 and/or IMPDH2, our data suggests the formation of a GTP- and possibly also an ATP-specific metabolic compartment—GTP and ATP metabolons, respectively—at the leading edge of the motile cancer cells. These compartments are expected to increase the local concentration of GTP and possibly also ATP via local, compartmentalized synthesis for availability to enhance actin polymerization, microtubule organization, and signaling. Consistent with this model, our recent cell biological and pathophysiological studies suggest a significant role for the non-membrane compartmentalization of purine metabolism at the leading edge in cell motility and the metastasis of certain types of cancers (manuscript in preparation).
Translocation of a GTP Biosynthetic Enzyme to the Leading Edge Depends on F-Actin
Actin polymerization is required for the translocation of several proteins such as PI3K to the leading edge and the subsequent local induction of leading edge signaling (7, 77–80). Interestingly and mechanistically importantly, the enrichment of IMPDH1 and IMPDH2 at the leading edge was reduced following the inhibition of actin polymerization by latrunculin B treatment. The data suggest that F-actin polymerization could provide a mechanistic basis by which purine biosynthetic enzymes localize to the leading edge. Just as binding to actin filaments has been shown to enhance flux through the glycolytic pathway, it would be interesting to determine whether the enzymes involved in purine biosynthesis can directly bind to F-actin fibers during translocation and whether this potential binding leads to enhanced flux through the metabolic pathway. A potentially interesting experiment is to use inhibitors for F-actin or tubulin and assess the ATP and GTP distribution using their designated biosensors (81, 82). Future studies should clarify how F-actin polymerization induces IMPDH localization at the leading edge as well as whether the leading edge localization of other purine metabolic enzymes utilizes this actin-dependent localization.
Heterogenous Purine Nucleotide Metabolism in Cell Membrane-Proximal Regions
ATP is one of the most abundant metabolites in a cell, ranging from 1 to 5 mM in mammalian cells (83). Intracellular distribution of ATP, detected by several types of genetic ATP biosensors (81), shows discrete ATP levels in organelles (84–87). There is also a report showing elevated ATP in the cortical region (88). Importantly, the turnover rate of ATP is increased at the leading edge via the activities of actin and tubulin polymerization (89), which is consistent with the previously noted high energy expenditure of cytoskeletal reorganization. Furthermore, very excitingly, the recently developed ratiometric fluorescent GTP biosensors have shown that the intracellular distribution of GTP is heterogeneous in SK-Mel-103 melanoma cells, with high GTP levels in the cytosol near certain regions of the cell membrane (82). Although further studies are required to eliminate potential confounding effects that might arise from the unintended pH sensitivity of these GTP biosensors, the provocative observation of high local GTP levels near some regions of the cell membrane, together with our identification of what is likely a GTP metabolon at the leading edge, suggest that elevated GTP levels specifically at the leading edge are highly probable.
Possible Existence of Other Metabolons at the Leading Edge
Our results show that purine salvage enzymes, such as HPRT1, are also localized at the leading edge, raising the possible existence of metabolons of salvage ATP and GTP biosynthesis at the leading edge. In addition to the GTP and ATP metabolons, we expect that there are likely additional metabolons formed at the leading edge. For example, some key enzymes of glycolysis, 6-phosphofructo-2-kinase/fructose-2,6-biphosphatase 3 (PFKFB3), and pyruvate kinase isozyme M2 (PKM2), localize to the leading edge membrane in macrophages and to cellular projections in migrating tip endothelial cells (90). In the report containing this data, although the existence of no other glycolytic enzyme at the leading edge was confirmed, the authors proposed that the localization of such ATP-generating enzymes at the leading edge might promote concentrated ATP production to fuel the polymerization of actin, with which these enzymes heavily colocalize (90–92). Although our own data show that GAPDH, a key rate-limiting enzyme for glycolysis (93), fails to enrich at the leading edge formation in kidney cancer cells (11), it is possible that GAPDH may either translocate to the leading edge under certain conditions or that its accumulation at the leading edge may not be necessary given its high general abundance in the cell. Regardless, it remains important to further verify the existence of a glycolytic metabolon at the leading edge.
Energy Homeostasis at the Leading Edge
Lastly, we highlight the possible links between homeostatic enzymes and leading edge activity. Adenylate kinase (AK)—which reversibly catalyzes the interconversion between 2ADP and 1ATP + 1AMP—is a critical enzyme for regulating cellular energy levels, and thus contributes to modulating the AMP-mediated response to stress signals (94, 95). In mouse embryonic fibroblasts, enforced localization of adenylate kinase 1 (AK1) to either focal contacts or the leading edge membrane significantly increases cell migration (96), supporting a notion of high metabolic turnover of ATP. Perhaps connecting to this observation, mitochondria have been shown to translocate to the leading edge through an AMPK-mediated mechanism to help sustain the local ATP:ADP ratio (97). The AMPK-dependent translocation of mitochondria to the leading edge suggests a locally enriched homeostatic system for ATP regeneration and recovery so that a high local ATP:ADP ratio may be maintained for robust leading edge activity. Currently, how AMPK induces the translocation of mitochondria remains largely unclear. Likewise, the dynamics of AMPK activation at the leading edge in relation to mitochondrial regulation remains unknown. Since AMPK is a known regulator of cell polarity (98), a possible model would be that downstream substrates of AMPK, which regulate cell polarity, may participate in the mitochondrial translocation. The use of AMPK biosensors may dissect the more detailed spatio and temporal regulation of AMPK activation and mitochondrial responses (99). Another potentially interesting experiment would be to compare the metabolic compartmentalization and ATP/GTP distribution in cells knocked out for AMPK or with mutations for the upstream kinase LKB1.
Concluding Remark
The importance of the discoveries highlighted in this text and the significance of the idea that metabolic compartmentalization may crucially fuel the leading edge during cell migration is currently underappreciated. Additional studies are badly needed to determine whether pharmacological inhibition of the purine biosynthetic enzymes is sufficient to decrease cancer cell migration and invasion. For instance, it will be of prime importance to investigate whether metabolic compartments form within invadopodia—membrane protrusions on the cell surface of tumor cells that mediate matrix cleavage for tumor invasion—and, if so, their functional significance in tumor invasion. Also, it will be critical to clarify the interplay between localized purine metabolism at the leading edge and molecules essential for leading edge functions, such as membrane type-I-matrix metalloproteinase (MT1-MMP) (100), Na+/H+ exchanger NHE1 (101), and RHO GTPases, to name a few. Such interactions, if they exist, would further induce coordinated changes promoting cell migration and metabolic responses—e.g., normoxic HIF1 upregulation by MT1-MMP and MINT3 pathway (102–104). With ongoing advances in sub-cellular biosensor technology and targeted therapeutics, our appreciation of the biological importance and therapeutic potential of the leading edge purine metabolon is only just beginning.
Author Contributions
KW and KC wrote the manuscript. RK projected to draw the illustration. TI overviewed. AS conceptualized, organized, and wrote the manuscript. All authors contributed to the article and approved the submitted version.
Funding
The work is supported in part by Emerson Collective Cancer Research Fund (645098 to TI), MTP UC-Brain Tumor Center grant, Ohio Cancer Research grant, R21NS100077, and R01NS089815 (AS).
Conflict of Interest
The authors declare that the research was conducted in the absence of any commercial or financial relationships that could be construed as a potential conflict of interest.
Acknowledgments
We thank Emily Dobbs, Robert DeRose, and Eric P. Smith for excellent editing.
References
1. Gupta GP, Massagué J. Cancer Metastasis: Building a Framework. Cell (2006) 127:679–95. doi: 10.1016/j.cell.2006.11.001
2. Fidler IJ. The pathogenesis of cancer metastasis: the “seed and soil” hypothesis revisited. Nat Rev Cancer (2003) 3:453–8. doi: 10.1038/nrc1098
3. Lambert AW, Pattabiraman DR, Weinberg RA. Emerging Biological Principles of Metastasis. Cell (2017) 168:670–91. doi: 10.1016/j.cell.2016.11.037
4. Nguyen DX, Massagué J. Genetic determinants of cancer metastasis. Nat Rev Genet (2007) 8:341–52. doi: 10.1038/nrg2101
5. Ridley AJ, Schwartz MA, Burridge K, Firtel RA, Ginsberg MH, Borisy G, et al. Cell Migration: Integrating Signals from Front to Back. Sci (80 ) (2003) 302:1704–9. doi: 10.1126/science.1092053
6. Devreotes P, Horwitz AR. Signaling networks that regulate cell migration. Cold Spring Harb Perspect Biol (2015) 7:1–16. doi: 10.1101/cshperspect.a005959
7. Yamaguchi H, Condeelis J. Regulation of the actin cytoskeleton in cancer cell migration and invasion. Biochim Biophys Acta Mol Cell Res (2007) 1773:642–52. doi: 10.1016/j.bbamcr.2006.07.001
8. Sasaki AT, Firtel RA. Finding the way: directional sensing and cell polarization through Ras signalling. Novartis Found Symp (2005) 269:73–91,223-230. doi: 10.1002/047001766X.ch8
9. Sasaki AT, Janetopoulos C, Lee S, Charest PG, Takeda K, Sundheimer LW, et al. G protein-independent Ras/PI3K/F-actin circuit regulates basic cell motility. J Cell Biol (2007) 178:185–91. doi: 10.1083/jcb.200611138
10. Elia I, Doglioni G, Fendt S-M. Metabolic Hallmarks of Metastasis Formation. Trends Cell Biol (2018) 28:673–84. doi: 10.1016/j.tcb.2018.04.002
11. Wolfe K, Kofuji S, Yoshino H, Sasaki M, Okumura K, Sasaki AT. Dynamic compartmentalization of purine nucleotide metabolic enzymes at leading edge in highly motile renal cell carcinoma. Biochem Biophys Res Commun (2019) 516:50–6. doi: 10.1016/j.bbrc.2019.05.190
12. Diekmann Y, Pereira-Leal JB. Evolution of intracellular compartmentalization. Biochem J (2012) 449:319–31. doi: 10.1042/BJ20120957
13. del Río LA, Sandalio LM, Palma JM, Bueno P, Corpas FJ. Metabolism of oxygen radicals in peroxisomes and cellular implications. Free Radic Biol Med (1992) 13:557–80. doi: 10.1016/0891-5849(92)90150-F
14. Gaertner FH. Unique catalytic properties of enzyme clusters. Trends Biochem Sci (1978) 3:63–5. doi: 10.1016/S0968-0004(78)94045-8
15. Hinzpeter F, Gerland U, Tostevin F. Optimal Compartmentalization Strategies for Metabolic Microcompartments. Biophys J (2017) 112:767–79. doi: 10.1016/j.bpj.2016.11.3194
16. Alam MT, Olin-Sandoval V, Stincone A, Keller MA, Zelezniak, Luisi BF, et al. The self-inhibitory nature of metabolic networks and its alleviation through compartmentalization. Nat Commun (2017) 8:1–13. doi: 10.1038/ncomms16018
17. Elmore S. Apoptosis: A Review of Programmed Cell Death. Toxicol Pathol (2007) 35:495–516. doi: 10.1080/01926230701320337
18. Welch GR. An analogical “field” construct in cellular biophysics: History and present status. Prog Biophys Mol Biol (1992) 57:71–128. doi: 10.1016/0079-6107(92)90005-Q
20. Hudder A, Nathanson L, Deutscher MP. Organization of Mammalian Cytoplasm. Mol Cell Biol (2003) 23:9318–26. doi: 10.1128/MCB.23.24.9318-9326.2003
21. Ovádi J, Saks V. On the origin of intracellular compartmentation and organized metabolic systems. Mol Cell Biochem (2003) 256:5–12. doi: 10.1023/B:MCBI.0000009855.14648.2c
23. Welch GR. On the role of organized multienzyme systems in cellular metabolism: A general synthesis. Prog Biophys Mol Biol (1978) 32:103–91. doi: 10.1016/0079-6107(78)90019-6
24. Saks VA, Ventura-Clapier R, Leverve X, Rossi A, Rigoulet M. What do we not know of cellular bioenergetics?–a general view on the state of the art. Mol Cell Biochem (1998) 184:3–9. doi: 10.1007/978-1-4615-5653-4_1
25. Saks V, Beraud N, Wallimann T. Metabolic Compartmentation – A System Level Property of Muscle Cells. Int J Mol Sci (2008) 9:751–67. doi: 10.3390/ijms9050751
26. Mastro AM, Babich MA, Taylor WD, Keith AD. Diffusion of a Small Molecule in the Cytoplasm of Mammalian Cells. Proc Natl Acad Sci (1984) 81:3414–8. doi: 10.1073/pnas.81.11.3414
27. Luby-Phelps K, Castle PE, Taylor DL, Lanni F. Hindered diffusion of inert tracer particles in the cytoplasm of mouse 3T3 cells. Proc Natl Acad Sci U S A (1987) 84:4910–3. doi: 10.1073/pnas.84.14.4910
28. Weiss M, Elsner M, Kartberg F, Nilsson T. Anomalous subdiffusion is a measure for cytoplasmic crowding in living cells. Biophys J (2004) 87:3518–24. doi: 10.1529/biophysj.104.044263
29. Mourão MA, Hakim JB, Schnell S. Connecting the dots: The effects of macromolecular crowding on cell physiology. Biophys J (2014) 107:2761–6. doi: 10.1016/j.bpj.2014.10.051
30. Parry BR, Surovtsev IV, Cabeen MT, O’Hern CS, Dufresne ER, Jacobs-Wagner C. The bacterial cytoplasm has glass-like properties and is fluidized by metabolic activity. Cell (2014) 156:183–94. doi: 10.1016/j.cell.2013.11.028
31. Sochacki KA, Shkel IA, Record MT, Weisshaar JC. Protein diffusion in the periplasm of E. coli under osmotic stress. Biophys J (2011) 100:22–31. doi: 10.1016/j.bpj.2010.11.044
32. McGuffee SR, Elcock AH. Diffusion, crowding & protein stability in a dynamic molecular model of the bacterial cytoplasm. PloS Comput Biol (2010) 6:1–18. doi: 10.1371/journal.pcbi.1000694
33. Minton AP. The Influence of Macromolecular Crowding and Macromolecular Confinement on Biochemical Reactions in Physiological Media. J Biol Chem (2002) 276:10577–80. doi: 10.1074/jbc.R100005200
34. Zhou H-X, Rivas G, Minton AP. Macromolecular Crowding and Confinement: Biochemical, Biophysical, and Potential Physiological Consequences. Annu Rev Biophys (2008) 37:375–97. doi: 10.1146/annurev.biophys.37.032807.125817
35. Dix JA, Verkman AS. Crowding Effects on Diffusion in Solutions and Cells. Annu Rev Biophys (2008) 37:247–63. doi: 10.1146/annurev.biophys.37.032807.125824
36. Kekenes-Huskey PM, Scott CE, Atalay S. Quantifying the Influence of the Crowded Cytoplasm on Small Molecule Diffusion. J Phys Chem B (2016) 120:8696–706. doi: 10.1021/acs.jpcb.6b03887
37. Verkman AS. Solute and macromolecule diffusion in cellular aqueous compartments. Trends Biochem Sci (2002) 27:27–33. doi: 10.1016/S0968-0004(01)02003-5
38. Biess A, Korkotian E, Holcman D. Barriers to diffusion in dendrites and estimation of calcium spread following synaptic inputs. PloS Comput Biol (2011) 7:1–14. doi: 10.1371/journal.pcbi.1002182
39. Wong IY, Gardel ML, Reichman DR, Weeks ER, Valentine MT, Bausch AR, et al. Anomalous diffusion probes microstructure dynamics of entangled F-actin networks. Phys Rev Lett (2004) 92:30–3. doi: 10.1103/PhysRevLett.92.178101
40. Weirich KL, Banerjee S, Dasbiswas K, Witten TA, Vaikuntanathan S, Gardel ML. Liquid behavior of cross-linked actin bundles. Proc Natl Acad Sci U S A (2017) 114:2131–6. doi: 10.1073/pnas.1616133114
41. Hyman AA, Weber CA, Jülicher F. Liquid-Liquid Phase Separation in Biology. Annu Rev Cell Dev Biol (2014) 30:39–58. doi: 10.1146/annurev-cellbio-100913-013325
42. Al-Habori M. Microcompartmentation, metabolic channelling and carbohydrate metabolism. Int J Biochem Cell Biol (1995) 27:123–32. doi: 10.1016/1357-2725(94)00079-Q
43. Coue M, Brenner SL, Spector I, Korn ED. Inhibition of actin polymerization by latrunculin A. FEBS Lett (1987) 213:316–8. doi: 10.1016/0014-5793(87)81513-2
44. Collin M. Interaction Between Glycolytic Enzymes and Cytomatrix. J Cell Biol (1984) 99:222–5. doi: 10.1083/jcb.99.1.222s
45. Real-Hohn A, Zancan P, Da Silva D, Martins ER, Salgado LT, Mermelstein CS, et al. Filamentous actin and its associated binding proteins are the stimulatory site for 6-phosphofructo-1-kinase association within the membrane of human erythrocytes. Biochimie (2010) 92:538–44. doi: 10.1016/j.biochi.2010.01.023
46. Menard L, Maughan D, Vigoreaux J. The structural and functional coordination of glycolytic enzymes in muscle: Evidence of a metabolon? Biology (2014) 3:623–44. doi: 10.3390/biology3030623
47. Arnold H, Pette D. Binding of Glycolytic Enzymes to Structure Proteins of the Muscle. Eur J Biochem (1968) 6:163–71. doi: 10.1111/j.1432-1033.1968.tb00434.x
48. Abercrombie M, Heaysman JEM, Pegrum SM. The locomotion of fibroblasts in culture. Exp Cell Res (1970) 62:389–98. doi: 10.1016/0014-4827(70)90570-7
49. Mandelkow EM, Mandelkow E, Milligan RA. Microtubule dynamics and microtubule caps: A time-resolved cryo-electron microscopy study. J Cell Biol (1991) 114:977–91. doi: 10.1083/jcb.114.5.977
50. Bernstein BW, Bamburg JR. Actin-ATP hydrolysis is a major energy drain for neurons. J Neurosci (2003) 23:1–6. doi: 10.1523/JNEUROSCI.23-01-00002.2003
51. Magasanik B, Moyed HS, Gehring LB. Enzymes essential for the biosynthesis of nucleic acid guanine; inosine 5’-phosphate dehydrogenase of aerobacter aerogenes. J Biol Chem (1957) 226:339–50.
52. Konno Y, Natsumeda Y, Nagai M, Yamaji Y, Ohno S, Suzuki K, et al. Expression of Human IMP Dehydrogenase Types I and II in Escherichia coli and Distribution in Human Normal Lymphocytes and Leukemic Cell Lines. J Biol Chem (1991) 266:506–9.
53. Floryk D, Thompson TC. Antiproliferative effects of AVN944, a novel inosine 5-monophosphate dehydrogenase inhibitor, in prostate cancer cells. Int J Cancer (2008) 123:2294–302. doi: 10.1002/ijc.23788
54. Nagai M, Natsumeda Y, Konno Y, Hoffman R, Irino S, Weber G, et al. Selective Up-Regulation of Type II Inosine 5 ‘ -Monophosphate Dehydrogenase Messenger RNA Expression in Human Leukemias. Cancer Res (1991) 51:3886–90.
55. Zou J, Han Z, Zhou L, Cai C, Luo H, Huang Y, et al. Elevated expression of IMPDH2 is associated with progression of kidney and bladder cancer. Med Oncol (2015) 32:373. doi: 10.1007/s12032-014-0373-1
56. Wang X, Yang K, Xie Q, Wu Q, Mack SC, Shi Y, et al. Purine synthesis promotes maintenance of brain tumor initiating cells in glioma. Nat Neurosci (2017) 20:661–73. doi: 10.1038/nn.4537
57. Collart FR, Chubb CB, Mirkin BL, Huberman E. Increased Inosine-5′-phosphate Dehydrogenase Gene Expression in Solid Tumor Tissues and Tumor Cell Lines. Cancer Res (1992) 52:5826–8. doi: 10.2172/10148922
58. Zhou L, Xia D, Zhu J, Chen Y, Chen G, Mo R, et al. Enhanced expression of IMPDH2 promotes metastasis and advanced tumor progression in patients with prostate cancer. Clin Transl Oncol (2014) 16:906–13. doi: 10.1007/s12094-014-1167-9
59. Fellenberg J, Kunz P, Sahr H, Depeweg D. Overexpression of Inosine 5 9 -Monophosphate Dehydrogenase Type II Mediates Chemoresistance to Human Osteosarcoma Cells. PloS One (2010) 5:1–8. doi: 10.1371/journal.pone.0012179
60. Proffitt RT, Pathak VK, Villacorte DG, Presant CA. Sensitive Radiochemical Assay for Inosine 5 ‘ -Monophosphate Dehydrogenase and Determination of Activity in Murine Tumor and Tissue Extracts. Cancer Res (1983) 43:1620–3.
61. Huang F, Ni M, Chalishazar MD, Huffman KE, Kim J, Cai L, et al. Inosine Monophosphate Dehydrogenase Dependence in a Subset of Small Cell Lung Cancers. Cell Metab (2018) 28:1–14. doi: 10.1016/j.cmet.2018.06.005
62. Naffouje R, Grover P, Yu H, Sendilnathan A, Wolfe K, Majd N, et al. Anti-tumor potential of IMP dehydrogenase inhibitors: A century-long story. Cancers (2019) 11:1–30. doi: 10.3390/cancers11091346
63. Kofuji S, Hirayama A, Otto Eberhardt A, Kawaguchi R, Sugiura Y, Sampetrean O, et al. IMP dehydrogenase-2 drives aberrant nucleolar activity and promotes tumorigenesis in glioblastoma. Nat Cell Biol (2019) 21:1003–14. doi: 10.1038/s41556-019-0363-9
64. Majd N, Sumita K, Yoshino H, Chen D, Terakawa J, Daikoku T, et al. A Review of the Potential Utility of Mycophenolate Mofetil as a Cancer Therapeutic. J Cancer Res (2014) 2014:1–12. doi: 10.1155/2014/423401
65. Morath C, Reuter H, Simon V, Krautkramer E, Muranyi W, Schwenger V, et al. Effects of mycophenolic acid on human fibroblast proliferation, migration and adhesion in vitro and in vivo. Am J Transplant (2008) 8:1786–97. doi: 10.1111/j.1600-6143.2008.02322.x
66. Dun B, Sharma A, Teng Y, Liu H, Purohit S, Xu H, et al. Mycophenolic acid inhibits migration and invasion of gastric cancer cells via multiple molecular pathways. PloS One (2013) 8:1–12. doi: 10.1371/journal.pone.0081702
67. Duan S, Huang W, Liu X, Liu X, Chen N, Xu Q, et al. IMPDH2 promotes colorectal cancer progression through activation of the PI3K/AKT/mTOR and PI3K/AKT/FOXO1 signaling pathways. J Exp Clin Cancer Res (2018) 37:1–16. doi: 10.1186/s13046-018-0980-3
68. Parri M, Chiarugi P. Rac and Rho GTPases in cancer cell motility control. Cell Commun Signal (2010) 8:23. doi: 10.1186/1478-811X-8-23
69. Wawrzyniak JA, Bianchi-Smiraglia A, Bshara W, Mannava S, Ackroyd J, Bagati A, et al. A Purine Nucleotide Biosynthesis Enzyme Guanosine Monophosphate Reductase Is a Suppressor of Melanoma Invasion. Cell Rep (2013) 5:493–507. doi: 10.1016/j.celrep.2013.09.015
70. Hartsough MT, Steeg PS. Nm23/nucleoside diphosphate kinase in human cancers. J Bioenerg Biomembr (2000) 32:301–8. doi: 10.1023/A:1005597231776
71. Bianchi-Smiraglia A, Wawrzyniak JA, Bagati A, Marvin EK, Ackroyd J, Moparthy S, et al. Pharmacological targeting of guanosine monophosphate synthase suppresses melanoma cell invasion and tumorigenicity. Cell Death Differ (2015) 22:1858–64. doi: 10.1038/cdd.2015.47
72. Steeg PS, Bevilacqua G, Kopper L, Thorgeirsson UP, Talmadge JE, Liotta LA, et al. Evidence for a Novel Gene Associated With Low Tumor Metastatic Potential2. JNCI J Natl Cancer Inst (1988) 80:200–4. doi: 10.1093/jnci/80.3.200
73. Leone A, Flatow U, King CR, Sandeen MA, Margulies IMK, Liotta LA, et al. Reduced Tumor Incidence, Metastatic Potential, and Cytokine Responsiveness of nm23-Transfected Melanoma Cells. Cell (1991) 65:25–35. doi: 10.1016/0092-8674(91)90404-M
74. Tan C-Y, Chang CL. NDPKA is not just a metastasis suppressor - be aware of its metastasis-promoting role in neuroblastoma. Lab Invest (2017) 00:1–9. doi: 10.1038/labinvest.2017.105
75. Park DS, Cho NH, Lee YT, Park C, Lee JM. Analysis of nm23 expression as a prognostic parameter in renal cell carcinoma. J Korean Med Sci (1995) 10:258–62. doi: 10.3346/jkms.1995.10.4.258
76. Wang Y, Leonard MK, Snyder DE, Fisher ML, Eckert RL. NME1 Drives Expansion of Melanoma Cells with Enhanced Tumor Growth and Metastatic Properties. Mol Cancer Res (2019) 17:1665–74. doi: 10.1158/1541-7786.MCR-18-0019
77. Sasaki AT, Chun C, Takeda K, Firtel RA. Localized Ras signaling at the leading edge regulates PI3K, cell polarity, and directional cell movement. J Cell Biol (2004) 167:505–18. doi: 10.1083/jcb.200406177
78. Sandilands E, Cans C, Fincham VJ, Brunton VG, Mellor H, Prendergast GC, et al. RhoB and actin polymerization coordinate Src activation with endosome-mediated delivery to the membrane. Dev Cell (2004) 7:855–69. doi: 10.1016/j.devcel.2004.09.019
79. Lambrechts A, Gevaert K, Cossart P, Vandekerckhove J, Van Troys M. Listeria comet tails: the actin-based motility machinery at work. Trends Cell Biol (2008) 18:220–7. doi: 10.1016/j.tcb.2008.03.001
80. Dandekar SN, Park JS, Peng GE, Onuffer JJ, Lim WA, Weiner OD. Actin dynamics rapidly reset chemoattractant receptor sensitivity following adaptation in neutrophils. Philos Trans R Soc B Biol Sci (2013) 368:1–11. doi: 10.1098/rstb.2013.0008
81. Rajendran M, Dane E, Conley J, Tantama M. Imaging Adenosine Triphosphate (ATP). Biol Bull (2016) 231:73–84. doi: 10.1086/689592
82. Bianchi-Smiraglia A, Rana MS, Foley CE, Paul LM, Lipchick BC, Moparthy S, et al. Internally ratiometric fluorescent sensors for evaluation of intracellular GTP levels and distribution. Nat Methods (2017) 14:1003–9. doi: 10.1038/nmeth.4404
83. Traut TW. Physiological concentrations of purines and pyrimidines. Mol Cell Biochem (1994) 140:1–22. doi: 10.1007/BF00928361
84. Imamura H, Huynh Nhat KP, Togawa H, Saito K, Iino R, Kato-Yamada Y, et al. Visualization of ATP levels inside single living cells with fluorescence resonance energy transfer-based genetically encoded indicators. Proc Natl Acad Sci USA (2009) 106:15651–6. doi: 10.1073/pnas.0904764106
85. Takaine M, Ueno M, Kitamura K, Imamura H, Yoshida S. Reliable imaging of ATP in living budding and fission yeast. J Cell Sci (2019) 132:1–11. doi: 10.1242/jcs.230649
86. Yaginuma H, Kawai S, Tabata KV, Tomiyama K, Kakizuka A, Komatsuzaki T, et al. Diversity in ATP concentrations in a single bacterial cell population revealed by quantitative single-cell imaging. Sci Rep (2014) 4:1–7. doi: 10.1038/srep06522
87. Shields LY, Mendelsohn BA, Nakamura K. Measuring ATP in Axons with FRET BT. In: Strack S, Usachev YM, editors. Techniques to Investigate Mitochondrial Function in Neurons. Springer New York: Humana Press (2017). p. 115–31. doi: 10.1007/978-1-4939-6890-9_6
88. Ozawa S, Ueda S, Imamura H, Mori K, Asanuma K, Yanagita M, et al. Glycolysis, but not Mitochondria, responsible for intracellular ATP distribution in cortical area of podocytes. Sci Rep (2015) 5:1–15. doi: 10.1038/srep18575
89. Suzuki R, Hotta K, Oka K. Spatiotemporal quantification of subcellular ATP levels in a single HeLa cell during changes in morphology. Sci Rep (2015) 5:1–9. doi: 10.1038/srep16874
90. De Bock K, Georgiadou M, Schoors S, Kuchnio A, Wong BW, Cantelmo AR, et al. Role of PFKFB3-driven glycolysis in vessel sprouting. Cell (2013) 154:651–63. doi: 10.1016/j.cell.2013.06.037
91. Semba H, Takeda N, Isagawa T, Sugiura Y, Honda K, Wake M, et al. HIF-1α-PDK1 axis-induced active glycolysis plays an essential role in macrophage migratory capacity. Nat Commun (2016) 7:1–10. doi: 10.1038/ncomms11635
92. Marelli-Berg FM, Jangani M. Metabolic regulation of leukocyte motility and migration. J Leukoc Biol (2018) 104:285–93. doi: 10.1002/JLB.1MR1117-472R
93. Liberti MV, Dai Z, Wardell SE, Baccile JA, Liu X, Gao X, et al. A Predictive Model for Selective Targeting of the Warburg Effect through GAPDH Inhibition with a Natural Product. Cell Metab (2017) 26:648–59.e8. doi: 10.1016/j.cmet.2017.08.017
94. Dzeja P, Terzic A. Adenylate kinase and AMP signaling networks: metabolic monitoring, signal communication and body energy sensing. Int J Mol Sci (2009) 10:1729–72. doi: 10.3390/ijms10041729
95. Dzeja PP, Zeleznikar RJ, Goldberg ND. Adenylate kinase: kinetic behavior in intact cells indicates it is integral to multiple cellular processes. Mol Cell Biochem (1998) 184:169–82. doi: 10.1007/978-1-4615-5653-4_13
96. Van Horssen R, Janssen E, Peters W, van de Pasch L, te Lindert MM, van Dommelen MMT, et al. Modulation of cell motility by spatial repositioning of enzymatic ATP/ADP Exchange capacity. J Biol Chem (2009) 284:1620–7. doi: 10.1074/jbc.M806974200
97. Cunniff B, McKenzie AJ, Heintz NH, Howe AK. AMPK activity regulates trafficking of mitochondria to the leading edge during cell migration and matrix invasion. Mol Biol Cell (2016) 27:2662–74. doi: 10.1091/mbc.e16-05-0286
98. Lee JH, Koh H, Kim M, Kim Y, Lee SY, Karess RE, et al. Energy-dependent regulation of cell structure by AMP-activated protein kinase. Nature (2007) 447:1017–20. doi: 10.1038/nature05828
99. Miyamoto T, Rho E, Sample V, Akano H, Magari M, Ueno T, et al. Compartmentalized AMPK signaling illuminated by genetically encoded molecular sensors and actuators. Cell Rep (2015) 11:657–70. doi: 10.1016/j.celrep.2015.03.057
100. Poincioux R, Lizárraga F, Chavrier P. Matrix invasion by tumour cells: A focus on MT1-MMP trafficking to invadopodia. J Cell Sci (2009) 122:3015–24. doi: 10.1242/jcs.034561
101. Schneider L, Stock C-M, Dieterich P, Jensen BH, Pedersen LB, Satir P, et al. The Na +/H + exchanger NHE1 is required for directional migration stimulated via PDGFR-α in the primary cilium. J Cell Biol (2009) 185:163–76. doi: 10.1083/jcb.200806019
102. Sakamoto T, Seiki M. A membrane protease regulates energy production in macrophages by activating hypoxia-inducible factor-1 via a non-proteolytic mechanism. J Biol Chem (2010) 285:29951–64. doi: 10.1074/jbc.M110.132704
103. Sakamoto T, Seiki M. Mint3 enhances the activity of hypoxia-inducible factor-1 (HIF-1) in macrophages by suppressing the activity of factor inhibiting HIF-1. J Biol Chem (2009) 284:30350–9. doi: 10.1074/jbc.M109.019216
Keywords: membraneless metabolic compartmentalization, leading edge, liquid-liquid phase separation, metabolon, purine biosynthesis, GTP-metabolism, cancer, metastasis
Citation: Wolfe K, Kamata R, Coutinho K, Inoue T and Sasaki AT (2020) Metabolic Compartmentalization at the Leading Edge of Metastatic Cancer Cells. Front. Oncol. 10:554272. doi: 10.3389/fonc.2020.554272
Received: 21 April 2020; Accepted: 29 September 2020;
Published: 02 November 2020.
Edited by:
Hongquan Zhang, Peking University Health Science Centre, ChinaCopyright © 2020 Wolfe, Kamata, Coutinho, Inoue and Sasaki. This is an open-access article distributed under the terms of the Creative Commons Attribution License (CC BY). The use, distribution or reproduction in other forums is permitted, provided the original author(s) and the copyright owner(s) are credited and that the original publication in this journal is cited, in accordance with accepted academic practice. No use, distribution or reproduction is permitted which does not comply with these terms.
*Correspondence: Atsuo T. Sasaki, YXRzdW8uc2FzYWtpQHVjLmVkdQ==