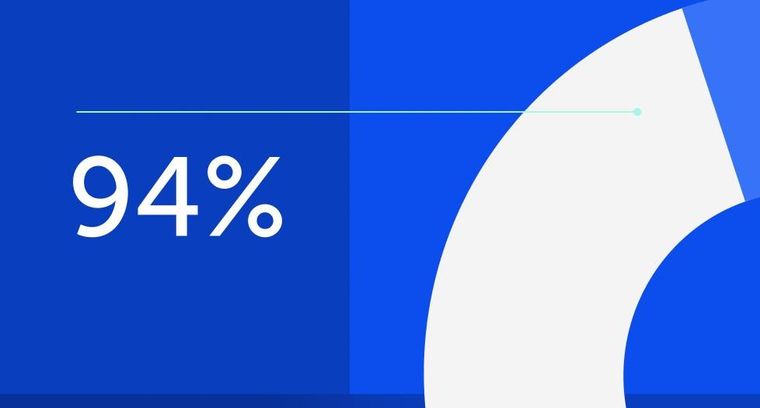
94% of researchers rate our articles as excellent or good
Learn more about the work of our research integrity team to safeguard the quality of each article we publish.
Find out more
REVIEW article
Front. Oncol., 23 July 2020
Sec. Cancer Molecular Targets and Therapeutics
Volume 10 - 2020 | https://doi.org/10.3389/fonc.2020.01182
The development of targeted medicine has greatly expanded treatment options and spurred new research avenues in cancer therapeutics, with monoclonal antibodies (mAbs) emerging as a prevalent treatment in recent years. With mixed clinical success, mAbs still hold significant shortcomings, as they possess limited tumor penetration, high manufacturing costs, and the potential to develop therapeutic resistance. However, the recent discovery of “nanobodies,” the smallest-known functional antibody fragment, has demonstrated significant translational potential in preclinical and clinical studies. This review highlights their various applications in cancer and analyzes their trajectory toward their translation into the clinic.
Just under 50 years ago, the “first generation” of therapeutic antibodies consisted of murine-derived, monoclonal antibodies (mAbs), with over 30 mAbs now approved by the Food and Drug Administration (FDA) for clinical use. Despite the clinical potential, their immunogenicity and large size (~150 kDa) became major detriments to their efficacy (1). This prompted the improved “second generation” of utilizing antibody fragments such as the antigen-binding fragment (Fab, ~50kDa) and single-chain variable fragment (scFv, ~30kDa); however, this approach remained limited by a short serum half-life and aggregation-induced immunogenicity (2).
The serendipitous discovery of heavy-chain only antibodies (HcAbs) in camelids sparked the most recent wave of “third generation” antibodies. Compared to conventional mAbs, HcAbs consist of just two heavy chains, with a single variable domain (VHH, ~15kDa) as the antigen-binding region. These nanoscale VHHs were coined the name “nanobodies” and could retain full antigen-binding potential upon isolation, establishing them as the smallest, naturally-derived antigen-binding fragment (3). Nanobodies have spurred the development of commercial companies and have been used in applications such as biosensing, affinity-capture, and protein crystallization; however, their most significant potential lies in therapeutics, especially for cancer. This review highlights how nanobodies have enhanced various cancer diagnostic tools and therapies, both alone, and synergistically. To conclude, an overview of nanobodies in cancer clinical trials is discussed, with an analysis on obstacles, and potential strategies to expedite their implementation as a translational cancer therapy.
Unlike other antibody fragments, nanobodies do not require extensive assembly or molecular optimization to create complex constructs. Possessing such a highly modular nature has propelled a wide array of nanobody-fusion molecules (Figure 1B). Although lacking a VL domain may seem detrimental to antigen binding, nanobodies have evolved to compensate, developing features that also enhance stability, diversity, and binding capacity. In general, antigen specificity is determined at the exposed ends of each variable domain through three peptide loops, or complementarity determining regions (CDRs). The CDR3 loop provides the most significant contribution to an antibody's specificity and diversity, and on average, nanobodies have a much greater CDR3 length compared to that of human VH domains, which strengthens their interactions with target antigens (4) (Figure 1A). Furthermore, their CDR3 regions can form finger-like projections that enable high-affinity binding to traditionally inaccessible cavity-like epitopes (5). Their CDR1 and CDR2 regions also aid in antigen binding, which enables greater paratope diversity than that of mAbs (6).
Figure 1. General nanobody structure and types of nanobodies. (A) Comparison of the monoclonal antibody (mAb) vs. heavy chain antibody (HcAb) to highlight the structural differences of their respective antigen binding regions. The VHH/Nanobody has a much longer CDR3 loop compared to that of the VH-VL domains in mAbs, providing antigen affinity and access to hidden epitopes. (B) A generalized overview of the types of engineered nanobodies to demonstrate how their high modularity enables various modifications. For enhanced antigen avidity, bivalent nanobodies can be created by connecting two identical nanobodies with a linker peptide. Biparatopic nanobodies are a fusion of two nanobodies targeting unique epitopes for the same antigen, with decreased dissociation from the target antigen. Bispecific nanobodies are composed of two nanobodies targeting different antigens and are often utilized as T cell engagers. Nanobodies can also be conjugated to other cancer therapies, nanoparticles, viral vectors, or to imaging agents for targeted tumor visualization. CDR3, complementarity-determining region 3; scFv, short-chain variable fragment; IL-2, Interleukin-2.
The inherent properties of nanobodies make them advantageous for cancer applications (Table 1). Their nanoscale dimensions enable deep penetration of tumors, with certain nanobodies able to cross the blood brain barrier (BBB) (7). Nanobodies also retain high affinity and specificity for their target antigens, with low off-target accumulation due to their hydrophilic regions (8). They're also unexpectedly robust due to their high refolding capacity, recovering from chemical denaturation with minimal damage to functionality, although a recent study suggests that thermal denaturation may be irreversible (9). Furthermore, they can tolerate high temperatures (60–80°C, several weeks at 37°C), elevated pressures (500–750 MPa), non-physiological pHs (3.0–9.0), and even the strongest of chemical denaturants (2–3 M guanidinium chloride, 6–8 M urea) (10). From a manufacturing standpoint, nanobodies are simple and inexpensive to produce. Lacking post-translational modifications, nanobodies can be synthesized through microbial systems, with the additional benefit of generating homogeneous products (11).
Much of the focus in cancer is placed on therapeutics, but the diagnostics of tumor imaging are just as critical, as visual knowledge of the tumor's antigen profile is needed to maximize therapeutic efficacy. A variety of imaging modalities are utilized in cancer diagnostics, and molecular imaging techniques have shown potential in improving existing techniques (Table 2). Molecular imaging utilizes a molecular probe that binds to a tumor antigen. Molecular imaging has been extensively explored with mAbs; however, their weak tumor penetration and longer serum half-life are significant obstacles in creating high-contrast images. Thus, nanobodies form quite suitable candidates, ensuring minimal non-target retention to create a high tumor-to-background ratio (T/B) shortly after administration.
The nuclear techniques of position emission tomography (PET) and single photon emission computed tomography (SPECT) comprise the majority of molecular imaging studies due to their quantitative output, high sensitivity, and clinical relevance. PET proves superior in sensitivity and spatial resolution; however, it's limited by a shorter imaging window and costly implementation. For tracking, nanobodies are tagged with a positron-emitting nuclide (e.g., 18F, 68Ga, 89Zr) for PET, and gamma-emitting nuclides (e.g., 99mTc) are used for SPECT.
Optical imaging, ultrasound, magnetic resonance imaging (MRI), and quantum dots have also been studied with nanobodies. In optical imaging, nanobodies are tagged with fluorescent dyes, and although the technique has weaker penetration, it offers the advantages of flexibility, simplicity, cost-effectiveness, and safety. Ultrasound imaging utilizes reflected sound waves from tissues, and nanobodies have been tagged to contrast agents, microbubbles, and nanobubbles. It is also a comparatively safer technique, but its applications are currently limited to systemic vasculature (12). MRI is a more expensive technique that utilizes strong magnetic fields to generate higher resolution images, and it has been paired with nanobody-coated superparamagnetic nanoparticles (13), magnetoliposomes (14), and fluorescent streptavidin (15) for detecting ovarian tumors. Quantum dots are fluorescent nanocrystals that have recently demonstrated tumor imaging potential for their adaptable properties, superior stability, and multiplex detection; however, their current implementation is limited by their low biocompatibility. Nanobody-conjugated quantum dots targeting carcinoembryonic antigen (CEA) (16), epidermal growth factor receptor vIII (EGFRvIII) (17), and cytotoxic T lymphocyte antigen-4 (CTLA-4) (18) have achieved enhanced targeting with minimal toxicity in vivo (17, 18).
Currently, the most advanced of nanobody probes target human epidermal growth factor receptor 2 (HER2) and are in clinical testing. In 2014, a phase I clinical trial tested a 68Ga-HER2 nanobody that could detect primary and metastatic tumors without adverse effects (19), leading to a phase II clinical trial (20). Other studies have assessed carbonic anhydrase IX (CAIX) and HER2-CAIX targeting for optical imaging (21). Notably, the HER2-CAIX combination synergistically enhanced the T/B ratio and could also detect lung metastases (22). Additionally, 89Zr-HER3 (23), 18F-HER2 (24), and 68Ga-NOTA-CD20 (25) nanobodies have demonstrated success in various tumor models. Pant et al. (26) developed a novel implementation of anti-EGFR-nanobody-dendritic polyglycerols (dPGs), demonstrating enhanced accumulation in vivo. 99mTc-EGFR (27), 99mTc-EGFR-cartilage oligomeric matrix protein (COMP) (28), 99mTc-dipeptidyl-peptidase-like protein 6 (DPP6) (29), 99mTc-mesothelin (30), and 131I-HER2 (31) nanobodies nanobody probes have also demonstrated high T/B ratios. Additionally, anti-EGFR nanobody probes have been utilized in dual-isotope SPECT (32) and optical imaging (33), with an enhanced T/B ratio vs. mAb-based probes (32, 33).
Tumor treatment resistance is often due to its intimate interactions with the surrounding tumor microenvironment (TME), an amalgam of extracellular matrix (ECM), angiogenesis, and infiltrating immune cells. This TME often accelerates tumor growth while repressing therapeutic efficacy; thus, its visualization is of paramount importance. Imaging tumor angiogenesis has been explored through targeting vascular cell adhesion molecule-1 (VCAM-1), a marker associated with metastasis and immune evasion, and anti-VCAM-1 nanobody-microbubbles have been used for ultrasound imaging of murine carcinomas (12). Nanobody probes targeting immune checkpoints (ICP) CTLA-4 and programmed death ligand 1 (PD-L1) (34–38) have been implemented in nuclear imaging with high T/B ratios (39, 40), and a phase I clinical study of the 99mTc–PD-L1 nanobody was recently completed (35). Notably, Lecocq et al. (41) developed the first anti-LAG-3 nanobodies for SPECT/CT imaging, demonstrating potential applications for detecting tumor-infiltrating immune cells.
In addition to visualizing the tumor's antigen profile, monitoring its immune infiltration regarding density, cell type, and activation levels, can be highly prognostic of a patient's therapeutic response. For monitoring T cell infiltration and activation, an anti-ADP-ribosyltransferase-2 (ART-2) nanobody demonstrated T cell tracking and unexpected therapeutic potential through ART-2 inhibition (42). A PEGylated 89Zr-CD8+ nanobody could track T cell response to ICP blockade, suggesting the utilization of imaged T cell distributions in predicting ICP therapy response (43). Another study revealed the myeloid compartment's role in PD-1 blockade response using PEGylated 89Zr-CD8+and 89Zr-CD11b+ nanobodies (44). Jailkhani et al. (45) developed a novel anti-EIIIB nanobody (splice variant of fibronectin) that enhanced detection of tumors, metastasis, and fibroses. The balance between anti-tumor and pro-tumor macrophages is another critical component that dictates the TME; thus, their targeting would be useful in illuminating overall macrophage polarization. The macrophage mannose receptor (MMR) is highly expressed in pro-tumor macrophages (46), and 99mTc-MMR (47), and 18F-MMR nanobodies (48) demonstrated specific targeting of MMR+ tumor associated macrophages (TAMs), with the 18F-MMR possessing a 20x lower kidney retention. Notably, preclinical validation of a 68Ga-NOTA-MMR nanobody had no observed toxicity, establishing its qualification for a phase I clinical trial (49). Opposite to MMR, (major histocompatibility complex class II) MHC-II expression is associated with anti-tumor macrophages and indicates effective antigen presentation to CD4+ T cells, with 64Cu-MHC-II (50), and 18F-MHC-II (40) nanobodies demonstrating good T/B ratios. Nanobody-based probes have also been designed to target antigen presenting cells (APCs). De Groeve et al. (51) created 99mTc-labeled nanobodies DC2.1 and DC1.8, mainly targeting myeloid and bone marrow-derived dendritic cells, respectively.
In 2007, Roovers et al. (52) published the first successful implementation of therapeutic nanobodies for solid tumors in vivo. Their anti-EGFR nanobody effectively delayed tumor growth (52), and they later developed a biparatopic version that superiorly reduced EGFR activation, with comparable potency to its mAb counterpart, cetuximab (53). Furthermore, variations have been developed against EGFR's dimer interface (54), EGFR-tyrosine kinase (55), and notably, nanobodies targeting EGFR-ectodomains could overcome the therapeutic resistance associated with mAbs (56). Notably, Rossotti et al. (57) reported DNA immunization-raised EGFR nanobodies with improved functionality compared to protein immunization-raised nanobodies. Nanobodies targeting EGF (58), HER2 (59, 60), CAIX (61), death receptor 5 (DR5) (62, 63), c-Met (64, 65), HGF (66), AgSK1 (67), mesothelin (68), proteasome activator complex PA28 (69), ephrin receptor A4 (EphA4) (70), CEA-cell adhesion molecule-6 (CEACAM6) (71), mitochondrial translation elongation factor (TUFM) (72), protein C receptor (73), Wnt receptors (LRP5/6) (74), and CD33 (75) have also demonstrated delayed tumor growth.
The inhibition of ICP pathways using mAbs as immune checkpoint inhibitors (ICIs) created a revolutionary breakthrough in the field of cancer therapeutics. Currently, antibodies targeting the molecules PD-1/PD-L1 and CTLA-4 have been FDA approved (76); however, their potency remains inconsistent, with minimal efficacy in most patients. Thus, the structural advantages of nanobodies show promise in enhancing ICIs. Various studies have created nanobody ICIs for PD-L1 (36, 77–81), enhancing anti-tumor efficacy when combined with its mAb counterpart, avelumab in vivo (36). Anti-CTLA-4 nanobodies have also demonstrated anti-tumor effects (39, 82); however, Ingram et al. (39) study suggest that an Fc domain may be needed for clinically-relevant potency. Homayouni et al. (83) developed the first nanobody targeting T-cell immunoglobulin and mucin domain 3 (TIM-3), demonstrating anti-proliferative effects in vitro. CD47 is another ICI target due to its involvement in both adaptive and innate immunity. However, because CD47 is also highly expressed in red blood cells, their clinical translation is stunted due to the high risks of anemia and hemagglutination (84). Anti-CD47 nanobodies have demonstrated improved therapeutic efficacy and synergistic potential with other ICIs (85, 86); furthermore, the fusion of an anti-CD47 nanobody with an anti-CD20 mAb showed high in vivo potency (87).
Nanobodies have also demonstrated potential in fighting tumor angiogenesis (Figure 2), a key accelerant of tumor growth and metastasis. The vascular endothelial growth factor (VEGF) and its receptors are well-established stimulants and thus ideal targets for inhibition. Monovalent and bivalent nanobodies blocked VEGF ligand binding (88, 89) while also inhibiting VEGF-activated proliferation in vivo (89). Additionally, conjugation to a proline-alanine-serine (PAS) sequence was reported to improve in vivo functionality and pharmacokinetics (90). An anti-VEGF receptor-2 (VEGFR2) nanobody demonstrated in vitro inhibition of capillary-like formation (91). Furthermore, nanobodies targeting delta-like ligand 4 (DLL4) (92) and CD3 (93) have demonstrated inhibition of neovascularization and tumor proliferation in vitro (92) and in vivo (93).
Figure 2. Nanobodies: targeting the tumor microenvironment. The synergistic potential of utilizing nanobodies to enhance tumor therapies targeting the tumor microenvironment. TAA, tumor associated antigen; DC, dendritic cell; MMR, mannose macrophage receptor; MHC-II, major histocompatibility complex-II; VEGF, vascular endothelial growth factor; VEGFR2, vascular endothelial growth factor receptor-2; IFN-γ, interferon- γ; IL-2, Interleukin-2; TNFα, tumor necrosis factor- α; IL-23, Interleukin-23; GCSFR, granulocyte colony-stimulating factor receptor; BiTE, bispecific T cell engager; CD16, cluster of differentiation-16; NK, natural killer; TRAIL, tumor necrosis factor- related apoptosis-inducing ligand; TCR, T-cell receptor; Treg, regulatory T cells; CAR, chimeric antigen receptor; UniCAR, universal CAR; TM, targeting module.
In addition to intrinsically therapeutic behavior, nanobodies can be utilized to augment the efficacy of other cancer therapies, especially in targeting the TME (Figure 2).
Antibodies targeting CD3, a receptor found in all T cells, were the first FDA-approved mAbs for clinical use; however, their initial systemic toxicity helped launch the development of bi-specific T-cell engagers (BiTEs). Smaller than mAbs, BiTEs are composed of two scFvs (one activates T cells, the other binds tumor antigens), and nanobody substitution has enabled more compact, enhanced BiTEs. HER2-scFvCD3 (94) and HER2-EGFR (95) BiTEs have been developed that can activate T cell-mediated, targeted tumor lysis both in vitro and in vivo (94, 95). Li et al. (96) created a BiTE composed of an anti-CEA nanobody and anti-CD3 Fab (“S-Fab”), with significant T-cell mediated cytotoxicity in vitro and in vivo. The S-Fab was PEGylated to extend its serum half-life and reported uncompromised anti-tumor activity (97). Various advancements have also been made in targeting CD3 (98), and anti-CD3 nanobodies have recently reported a targeted anti-tumor response in vivo (93, 99).
Similarly, bispecific light T-cell engagers (LiTEs) targeting EGFR and CD3 have demonstrated T cell-mediated tumor lysis with minimal cytotoxicity (100). The same group subsequently created the “ATTACK,” composed of three anti-EGFR nanobodies and an anti-CD3 scFv, with a 15-fold higher efficacy than their LiTEs (101). The group also developed 4-1BB-agonistic trimerbodies targeting EGFR (102) and CEA (103), with minimal off-tumor cytotoxicity in vitro (103) and in vivo (102).
The efficacy of chimeric antigen receptor (CAR) T cells has been established in blood-based malignancies; however, their solid tumor implementation has been limited by their inherent immunogenicity and large size of CARs. Various studies have demonstrated the efficacy of utilizing MUC-1 (104), CD7 (105), CD38 (106), VEGFR2 (107), prostate-specific membrane antigen (PSMA) (108, 109), glypican-2 (GPC2) (110), and T cell receptor (TCR)-like nanobody-CARs (111) in various tumor models. Bispecific nanobody-CARs targeting CD20 and HER2 have also been developed; however, experiments have yet to be performed in vivo (112). Xie et al. (113) created TME-targeting CAR T cells binding to PD-L1 or EIIIB, with significant tumor reduction in vivo and suggested potential in combination therapies. Additionally, anti-PD-L1/CTLA-4 nanobody-secreting CAR T cells have demonstrated enhanced anti-tumor response in vivo and indicate synergistic potential (114).
Studies have also evaluated the integration of nanobodies and the universal CAR (UniCAR) platform. Rather than recognizing tumor antigens, the UniCAR is activated by externally-administered “targeting modules” (TMs), which are UniCAR-activating epitopes linked to an antibody component that recognizes the target antigen, creating a “safety switch” that regulates an otherwise uncontrolled therapy (115). Albert et al. (116) created a nanobody-based TM that effectively retargets UniCAR T cells to induce EGFR+ tumor lysis, and they subsequently developed a bivalent version that could target low EGFR+ tumors in vivo (117).
Gamma-delta (γδ) T cells comprise 0.5–5% of all T-lymphocytes, and the Vγ9Vδ2 T subset has demonstrated therapeutic potential in various tumor models. In the context of nanobodies, a BiTE targeting the EGFR and Vγ9Vδ2 TCR stimulated T-cell mediated cytotoxicity against EGFR+ tumor cells in vivo (118). Compared to other anti-CD3-BiTEs, this removed the risk of activating pro-tumor cells such as regulatory T cells (Tregs), which heavily predominate the TME.
Natural killer (NK) cells possess both cytolytic and immunomodulatory abilities against tumor cells and have demonstrated clinical efficacy in blood-based malignancies. However, like T cell-based therapies, their potency remains stunted in solid tumors, particularly from limited tissue penetration and immunosuppression. To address this, studies have conjugated anti-CD16 nanobodies to nanobodies targeting CEA (119–122), MUC-1 (123), HER2 (124), or to Fabs targeting HER2 (125, 126) or GPC3 (127), with potent tumor growth suppression in vivo.
Dendritic cells (DCs) are the most potent of APCs and are promising targets for cancer vaccines, which build the anti-tumor response by prematurely delivering tumor antigen-mAb conjugates to APCs. Utilizing the penetration capacity and structural simplicity of nanobodies, studies have explored the implementation of nanobody-based antigen conjugates to enhance DC-based immunity. Some target DC surface proteins such as CD11b (128, 129), CD36 (128), and MHC-II (128, 130), and others have been designed to block ICPs CTLA-4 (131), and PD-L1 (132) to enhance DC-mediated T cell activation. Kwon et al. (133) developed a novel anti-MHC-II nanobody conjugated to cyclotides (cyclic, plant-derived peptides) that also demonstrated cyclotide-scaffold potential against constrained epitopes. Antigen delivery can also be achieved through viral transduction. Adeno-associated viruses (AAVs), adenoviruses (Ads), and lentiviruses (LVs) have been explored; however, their main limitation is their broad tropism (134). Thus, the surface fusion of nanobodies can increase their target specificity. Nanobody-LVs have shown specific DC transduction (135) in vitro, but a subsequent study suggested that broad tropism LVs may be more effective in inducing an anti-tumor response (136). Furthermore, Crowley et al. (137) developed nanobody-conjugated peptide vaccines targeting MHC-II for APC delivery, demonstrating enhanced vaccine-mediated CD8+ T cell activation in vivo.
Nanobody-AAVs have shown success in targeting antigens such as CD38, ARTC2.2, and P2X7, but further in vivo studies are needed (138). Viral vectors have also shown potential for targeting tumor vasculature, and Ahani et al. (139) developed anti-VEGFR2-LVs with comparable targeting to that of VEGF. Additionally, recombinant lambda (λ) bacteriophages have reported significant in vitro inhibition of HER2+ cell proliferation (140).
Despite the preclinical success of cytokine-based therapy, clinical studies have been met with subpar efficacy due to their narrow therapeutic window and short half-life. However, the incorporation of nanobodies could impart a new wave of improved cytokine therapies. An anti-PD-L1 nanobody fused to either interleukin-2 (IL-2) or interferon-γ (IFNγ) demonstrated in vivo efficacy in treatment-resistant pancreatic tumors (141). Similarly, an anti-CEA nanobody fused to IL-12 demonstrated amplified immune cell proliferation and antitumor activity in vivo (142). Furthermore, nanobodies designed to neutralize TNFα (143), IL-23 (144), granulocyte colony-stimulating factor receptor (G-CSF-R) (145), and transforming growth factor beta (TGF-β) (146) have demonstrated success in vitro (145), and in vivo (143, 144). Nanobodies have also been created to target chemokines, a class of chemotactic cytokines that directly impact tumor proliferation, angiogenesis, and metastasis. Nanobodies blocking protumor chemokines [CXCL10 (147)] or fused to anti-tumor chemokines [CCL21 (148)] have demonstrated functionality in microfluidic devices, but have yet to be tested in vivo. Antagonistic nanobodies for chemokine receptors such as ChemR23 (149), US28 (150), CCR7 (151), CXCR4 (152–154), and CXCR7 (154, 155) have also been developed. Smolarek et al. (156) developed the first nanobody inhibiting the Duffy antigen receptor for chemokines (DARC), but it has yet to be applied in tumor models. An anti-L-plastin nanobody was reported to augment T cell proliferation and IL-2 secretion, but this has also not been studied in tumors (157).
Several studies have explored utilizing nanobodies to deliver bacterial toxin pseudomonas exotoxin A (PE). PE and its fragments have been fused to anti-GPC3 (158, 159), anti-GPC2 (110), anti-VEGFR2 (160), anti-CD7 (161, 162), anti-HER2 (163), and anti-CD38 (164) nanobodies, enhancing cytotoxic effects in various tumor models. Notably, Cao et al. (165) developed an enhanced anti-HER2-PE toxin that improved both efficacy and the maximum tolerated dose. β-lactamases from Enterobacter cloacae also have established anti-tumor activity, and their enhanced potency after anti-CEA nanobody conjugation reflects potential in improving directed enzyme prodrug therapies (166). Massa et al. (167) conjugated anti-CD20 nanobodies to Salmonella bacteria carrying a drug-converting enzyme, demonstrating significant in vivo efficacy. L-DOS47 is a recently developed nanobody-urease enzyme conjugate targeting CEACAM6 (168) and is currently in phase I/II clinical trials. A similar anti-VEGFR2 nanobody conjugated to DOS-47 has been developed for angiogenesis inhibition (169). Vlaeminck et al. (170) developed an anti-MMR nanobody fused to an active form of second mitochondria-derived activator of caspase (tSMAC) to target TAMs, reporting upregulated macrophage caspase 3/7 activity in vitro. Fusion of anti-EGFR nanobodies have increased therapeutic efficacy of platinum prodrugs (171) and cucurmosin (172), and anti-MHC-II nanobodies fused to the drug DM1 have also exhibited significant targeting and tumor cytotoxicity (173).
Compared to nanobody-drug conjugation, using nanoparticles (NPs) as drug carriers offers benefits such as enhanced protection, bioavailability, and decreased immunogenicity, with enhanced targeting through nanobody conjugation. Wang et al. (174) created quantum-dot-based, anti-EGFR-nanobody micelles carrying aminoflavone, showing enhanced tumor regression in vivo. Additionally, anti-EGFR-nanobody micelles carrying doxorubicin (175, 176) and anti-EGFR-nanobody liposomes carrying kinase inhibitors (177) demonstrated enhanced anti-tumor efficacy in vivo (176, 177). Interestingly, empty anti-EGFR-nanobody liposomes could also downregulate in vivo EGFR expression, an effect that anti-EGFR-scFv liposomes were unable to induce (178). Co-delivery of simvastatin/gefitinib using anti-PD-L1-nanobody liposomes reversed tyrosine kinase inhibitor (TKI) resistance, addressing a major treatment obstacle in non-small-cell lung cancers (NSCLC) (179). Anti-CD8-nanobody-gold NPs carrying a TGF-β inhibitor demonstrated a 40-fold increase in CD8+ T cell uptake in vivo (180). Polymer-based NPs (181) composed of albumin (182, 183), and polyethyleneimine-PEG (184) demonstrated tumor proliferation inhibition. Notably, anti-HER2, saporin-loaded NPs demonstrated synergistic efficacy when paired with photochemical internalization (PCI) (185). Other explored implementations are nanobody-conjugated extracellular vesicles (186, 187), dendrimers (188), DNA nanoplatforms (189), and nanogels (190), but further studies are needed to characterize these modalities.
Another approach to improving drug uptake is the use of tumor penetrating peptides (TPPs), which can increase vasculature and tissue permeability through activating endocytic pathways (191). Various studies have conjugated nanobodies to TPPs to improve specificity and penetration. Anti-EGFR nanobodies fused to the “iRGD” TPP have demonstrated antitumor activity in vivo (192–195), while also showing synergy with chemotherapy drugs (193), T cells (194), silk fibroin nanoparticles (192), and nanoparticles containing gambogic acid (195). Additionally, anti-EGFR nanobodies conjugated to a lactoferrin sequence demonstrated enhanced combinatorial EGFR inhibition (196).
Stem cells have demonstrated great potential in cancer therapeutics due to their inherent tumor tropism and engineered ability to secrete various therapeutic agents, creating a customizable system for targeted tumor delivery. Van de Water et al. (197) developed therapeutic stem cells (SCs) that secreted either anti-EGFR nanobodies (“ENbs”) or proapoptotic ENbs conjugated to TNF-related apoptosis-inducing ligand (TRAIL) for application in GBMs. Notably, the ENb-TRAIL conjugates could significantly reduce tumor growth and invasion in vivo across a wide spectrum of GBMs with varying TRAIL resistances (197).
Targeted radiotherapy delivers a cytotoxic radionuclide-mAb conjugate to the tumor site, and α-particles are commonly used for their high biological efficacy (198). However, their short half-lives are incompatible with the large size, and slow tissue clearance of mAbs; thus, nanobodies hold potential as improved delivery vectors. 225Ac-HER2 (199) and 211At-HER2 (200) nanobody conjugates enhanced targeting in vivo and demonstrate the relevance of further exploring nanobody-delivered α-particle radiation therapy.
Another avenue of nanobody applications is photodynamic therapy (PDT), which uses a light-activated photosensitizer (PS) to kill tumor cells. mAbs have been used as conjugates to better direct the photosensitizing agent to the tumor site; however, their limitations hinder PDT efficacy and prolong patient photosensitivity (201), which could be circumvented with nanobodies. Anti-HER2 nanobodies conjugated to branched gold NPs could remove HER2+ cells upon 5 min of laser treatment (202), and anti-EGFR (203–205), anti-c-Met (205), and anti-U28 (206) nanobody-PS conjugates demonstrated targeted phototoxicity in vitro (203, 205, 206), and in vivo (203). Additionally, anti-EGFR nanobodies conjugated to a novel RuII polypyridyl complex reported EGFR-specific targeting (207).
Currently, most therapies are designed for extracellular markers, despite the fact that most tumor signaling is controlled intracellularly (208). Various nanobodies, or “intrabodies,” have been created for human papillomavirus (HPV) oncoproteins E6 (209), E7 (210), with E7 intrabody expression in HPV16+ cells significantly delaying their growth (210). Steels et al. (211) developed intrabodies targeting tumor suppressor p53's transactivation domain (TAD) and DNA-binding domain (DBD) (212). The p53-DBD nanobodies unexpectedly inhibited p53 transactivation (212), demonstrating that p53 mechanisms are still not fully understood. Additionally, intrabodies developed against B-cell receptor-associated protein 31 (BAP31), have demonstrated caspase-dependent tumor apoptosis in vivo (213).
Most studies have delivered nanobodies intravenously; however, their small size subjects them to rapid renal clearance, increasing the load/frequency needed to achieve clinically relevant efficacy. As it would be both impractical and wasteful to implement such a treatment regimen, a common approach has been to modify nanobodies to extend their serum half-life. PEGylation, fusion to anti-albumin nanobodies or Fc domains, and multimerization have demonstrated longer serum half-lives; however, the trade-offs are lower tumor penetration and additional manufacturing costs. Although not as highly explored, the utilization of nanobody-secreting carriers could circumvent such issues by ensuring both continuous and localized delivery. Notably, “programmable” bacteria that release CD47-targeting nanobodies in the TME increased tumor regression and metastatic inhibition in vivo (214). Similarly, Gurbatri et al. (215) engineered a probiotic system that could release anti-PD-L1 and anti-CTLA-4 nanobodies intratumorally, and a single dose reported efficacy comparable to mAbs in vivo, a potentiated systemic immune response, and synergistic potential with granulocyte-macrophage CSF (GM-CSF).
For intracellular delivery, nanobodies can also be encoded within viral vectors to produce intracellular-targeting nanobodies inside the tumor, but further in vivo studies are needed. Notably, the bacterial type III protein secretion system (T3SS) has been utilized to deliver nanobodies into tumor cells. Essentially a molecular syringe, the nanobodies are injected into the cytoplasm, and anti-EGFP (216), anti-amylase (217), and anti-GFP (217) nanobodies have demonstrated successful delivery in vitro (216, 217), and in vivo (216). Currently, the unspecific targeting hinders T3SS potential, but this could be addressed through conjugating nanobodies to the bacteria's surface. For imaging applications, most tumors can be visualized through intravenous delivery of nanobody-based probes. The same cannot be said for brain tumors, as the BBB significantly hinders their uptake. However, a recent study found that intra-arterial administration of nanobody imaging probes dramatically enhanced delivery regardless of BBB status (218), suggesting a potential avenue for circumventing BBB limitations.
Relative to the numerous avenues of nanobody applications, very few clinical trials have been completed for cancer (Table 3). This could be attributed to their relative infancy as a cancer therapeutic, heightened by the 2012 clinical trial of a tetravalent nanobody targeting DR5, which was terminated due to unanticipated hepatoxicity (NCT01529307). As mentioned previously, the completed phase I trial for the 68Ga-HER2 PET/CT nanobody spurred an ongoing phase II trial that will quantify the metastasis in breast carcinoma patients and assess repeatability (NCT03924466). The same group is currently recruiting for a phase II trial assessing 68Ga-NOTA-HER2 nanobody uptake in brain metastasis of breast carcinoma patients (NCT03331601), a phase I/II trial for 68Ga-NOTA-MMR-VHH2 nanobodies (NCT04168528), and a feasibility trial for anti-idiotypic nanobodies in multiple myeloma patients (NCT03956615). An early phase I trial for evaluating a 99mTc-HER2 nanobody is projected to finish in June 2020 (NCT04040686). Additionally, a completed phase I study assessing the [131I]-SGMIB-HER2 nanobody demonstrated no adverse effects after one intravenous dose (NCT02683083). The third completed clinical trial is an early phase I study of a 99mTc-PD-L1 nanobody for NSCLC patients (NCT02978196), which successfully visualized tumor uptake 2 h post-injection (35).
As for therapeutics, phase Ib/II and phase II trials are currently evaluating the safety and tolerability of L-DOS47 in combination with doxorubicin (NCT04203641) or vinorelbine/cisplatin (NCT03891173), respectively. Furthermore, trials testing the safety and tolerability of PD-L1 (NCT04034823, NCT03248843, NCT03101488) and CTLA-4 (NCT04126590) inhibitors are recruiting for breast and metastatic tumor patients. Nanobody-based immune cell therapies also have clinical trials in the recruiting phase. A phase I/II trial will assess the overall response rate to T cells expressing anti-mesothelin nanobodies fused to the endogenous TCR (NCT03907852). CD19/20 (NCT03881761) and B-cell maturation antigen (BCMA) CAR T cells (NCT03664661) will also be assessed in patients with refractory/relapsed B cell lymphoma.
Nanobodies uniquely possess the combined therapeutic advantages of mAbs and the targeting potential of nanoscale delivery. Their compact size enables enhanced tumor penetration and access to hidden and/or intracellular epitopes, while also granting high modularity for creating more complex nanobody-based constructs. Their robustness and manufacturing ease are favorable for large-scale production, and their superior paratope diversity allows an extensive arsenal for tumor antigen targeting. Although nanobodies could be portrayed as a superior version of mAbs, it is important to consider the implications of their differences. Nanobodies are subject to rapid renal clearance, and although this is desirable for imaging purposes, it limits their therapeutic lifetime and lowers the threshold for inducing renal toxicity (219), further limited if conjugated to cytotoxic loads. However, this could be combatted through infusing gelofusine or lysine (27), inserting charged residues in the nanobodies, and the aforementioned methods of extending serum half-life. Additionally, unlike mAbs, nanobodies lack an Fc region, and thus cannot directly initiate an Fc-mediated immune response.
As nanobodies are not naturally produced in humans, their therapeutic implementation brings into question their overall safety (Table 4). Nanobody sequence studies have revealed high similarity with human VH domains (220), and combined with their size, structure, and low agglutination, nanobodies possess low immunogenicity and are appropriate for human administration. Nonetheless, immunogenicity could be further minimized through “humanization,” which is generally accomplished through replacing various surface regions with human sequences. However, such replacements may compromise functionality, and more concerningly, humanization may decrease solubility, negating any immunogenicity-lowering effects (221). Currently, conflicting clinical results make it difficult to establish an immunogenic profile (222, 223), which may best be resolved through the completion of additional clinical trials.
Nanobody are versatile in that their applications extend across the full timeline of a cancer patient's treatment. Using nanobody-based imaging probes has shown improved visualization compared to traditional mAb-based probes. On their own, nanobodies can be utilized as targeted antagonists, ICIs, angiogenesis inhibitors, and as cytokine neutralizers or stimulants. Their synergy with existing cancer therapeutics is reflective of their promising potential to elevate cancer treatments well outside of their origins in antibody-based applications. Nanobodies can be conjugated to drugs, cytokines, NPs, TPPs, photosensitizers, and α-particles for enhanced delivery. Furthermore, they can augment immune cell-based therapies, improve viral vector delivery, and be secreted by engineered stem cells and bacteria. In light of these various applications, their greatest potential may be found in intracellular targeting. As evidenced by existing preclinical studies, the targeting of critical intracellular tumor antigens may be the next pivotal step to revolutionizing a new wave of cancer therapeutics.
EY and KS: literature review and interpretation, manuscript writing, and final approval of manuscript. KS: conception and design. Both authors contributed to the article and approved the submitted version.
This work was supported by NIH grant R01-CA201148 (KS) and by DoD grant LC180495.
KS owns equity in and is a member of the Board of Directors of AMASA Therapeutics, a company developing stem cell-based therapies for cancer. KS's interests were reviewed and are managed by Brigham and Women's Hospital and Partners HealthCare in accordance with their conflict of interest policies.
The remaining author declares that the research was conducted in the absence of any commercial or financial relationships that could be construed as a potential conflict of interest.
1. Liu JKH. The history of monoclonal antibody development - progress, remaining challenges and future innovations. Ann Med Surg. (2014) 3:113–6. doi: 10.1016/j.amsu.2014.09.001
2. Ewert S, Huber T, Honegger A, Plückthun A. Biophysical properties of human antibody variable domains. J Mol Biol. (2003) 325:531–53. doi: 10.1016/S0022-2836(02)01237-8
3. Hamers-Casterman C, Atarhouch T, Muyldermans S, Robinson G, Hammers C, Songa EB, et al. Naturally occurring antibodies devoid of light chains. Nature. (1993) 363:446–8. doi: 10.1038/363446a0
4. Desmyter A, Decanniere K, Muyldermans S, Wyns L. Antigen specificity and high affinity binding provided by one single loop of a camel single-domain antibody. J Biol Chem. (2001) 276:26285–90. doi: 10.1074/jbc.M102107200
5. De Genst E, Silence K, Decanniere K, Conrath K, Loris R, Kinne J, et al. Molecular basis for the preferential cleft recognition by dromedary heavy-chain antibodies. Proc Natl Acad Sci USA. (2006) 103:4586–91. doi: 10.1073/pnas.0505379103
6. Mitchell LS, Colwell LJ. Analysis of nanobody paratopes reveals greater diversity than classical antibodies. Protein Eng Des Sel. (2018) 31:267–75. doi: 10.1093/protein/gzy017
7. Muruganandam A, Tanha J, Narang S, Stanimirovic D. Selection of phage-displayed llama single-domain antibodies that transmigrate across human blood-brain barrier endothelium. FASEB J. (2002) 16:240–2. doi: 10.1096/fj.01-0343fje
8. Muyldermans S, Atarhouch T, Saldanha J, Barbosa JARG, Hamers R. Sequence and structure of vh domain from naturally occurring camel heavy chain immunoglobulins lacking light chains. Protein Eng Des Sel. (1994) 7:1129–35. doi: 10.1093/protein/7.9.1129
9. Kunz P, Zinner K, Mücke N, Bartoschik T, Muyldermans S, Hoheisel JD. The structural basis of nanobody unfolding reversibility and thermoresistance. Sci Rep. (2018) 8:7934. doi: 10.1038/s41598-018-26338-z
10. de Vos J, Devoogdt N, Lahoutte T, Muyldermans S, Muyldermans S. Camelid single-domain antibody-fragment engineering for (pre)clinical in vivo molecular imaging applications: Adjusting the bullet to its target. Expert Opin Biol Ther. (2013) 13:1149–60. doi: 10.1517/14712598.2013.800478
11. Arbabi-Ghahroudi M, Tanha J, MacKenzie R. Prokaryotic expression of antibodies. Cancer Metastasis Rev. (2005) 24:501–19. doi: 10.1007/s10555-005-6193-1
12. Hernot S, Unnikrishnan S, Du Z, Shevchenko T, Cosyns B, Broisat A, et al. Nanobody-coupled microbubbles as novel molecular tracer. J Control Release. (2012) 158:346–53. doi: 10.1016/j.jconrel.2011.12.007
13. Shahbazi-Gahrouei D, Abdolahi M. Detection of MUC1-expressing ovarian cancer by c595 monoclonal antibody-conjugated SPIONs using MR imaging. Sci World J. (2013) 2013:1–7. doi: 10.1155/2013/609151
14. Khaleghi S, Rahbarizadeh F, Ahmadvand D, Hosseini HRM. Anti-HER2 VHH targeted magnetoliposome for intelligent magnetic resonance imaging of breast cancer cells. Cell Mol Bioeng. (2017) 10:263–72. doi: 10.1007/s12195-017-0481-z
15. Prantner AM, Yin C, Kamat K, Sharma K, Lowenthal AC, Madrid PB, et al. Molecular imaging of mesothelin-expressing ovarian cancer with a human and mouse cross-reactive nanobody. Mol Pharm. (2018) 15:1403–11. doi: 10.1021/acs.molpharmaceut.7b00789
16. Sukhanova A, Even-Desrumeaux K, Kisserli A, Tabary T, Reveil B, Millot JM, et al. Oriented conjugates of single-domain antibodies and quantum dots: toward a new generation of ultrasmall diagnostic nanoprobes. Nanomed Nanotechnol Biol Med. (2012) 8:516–25. doi: 10.1016/j.nano.2011.07.007
17. Fatehi D, Baral TN, Abulrob A. In vivo imaging of brain cancer using epidermal growth factor single domain antibody bioconjugated to near-infrared quantum dots. J Nanosci Nanotechnol. (2014) 14:5355–62. doi: 10.1166/jnn.2014.9076
18. Wang W, Hou X, Yang X, Liu A, Tang Z, Mo F, et al. Highly sensitive detection of CTLA-4-positive T-cell subgroups based on nanobody and fluorescent carbon quantum dots. Oncol Lett. (2019) 18:109–16. doi: 10.3892/ol.2019.10320
19. Keyaerts M, Xavier C, Heemskerk J, Devoogdt N, Everaert H, Ackaert C, et al. Phase i study of 68Ga-HER2-Nanobody for PET/CT assessment of HER2 expression in breast carcinoma. J Nucl Med. (2016) 57:27–33. doi: 10.2967/jnumed.115.162024
20. Repeatability of 68-GaNOTA-Anti-HER2 VHH1 PET/CT in Breast Carcinoma Patients (VUBAR). (2019). Available online at: https://clinicaltrials.gov/ct2/show/NCT03924466 (accessed April 23, 2019).
21. van Brussel ASA, Adams A, Oliveira S, Dorresteijn B, El Khattabi M, Vermeulen JF, et al. Hypoxia-Targeting fluorescent nanobodies for optical molecular imaging of pre-invasive breast cancer. Mol Imaging Biol. (2016) 18:535–44. doi: 10.1007/s11307-015-0909-6
22. Kijanka MM, van Brussel ASA, van der Wall E, Mali WPTM, van Diest PJV, van Bergen en Henegouwen PMP, et al. Optical imaging of pre-invasive breast cancer with a combination of VHHs targeting CAIX and HER2 increases contrast and facilitates tumour characterization. EJNMMI Res. (2016) 6:14. doi: 10.1186/s13550-016-0166-y
23. Warnders FJ, Van Scheltinga AGT, Knuehl C, Van Roy M, De Vries EFJ, Kosterink JGW, et al. Human epidermal growth factor receptor 3-specific tumor uptake and bioDistribution of 89Zr-MSB0010853 visualized by real-time and noninvasive pet imaging. J Nucl Med. (2017) 58:1210–5. doi: 10.2967/jnumed.116.181586
24. Zhou Z, McDougald D, Devoogdt N, Zalutsky MR, Vaidyanathan G. Labeling single domain antibody fragments with fluorine-18 Using 2,3,5,6-tetrafluorophenyl 6-[ 18 F]fluoronicotinate resulting in high tumor-to-kidney ratios. Mol Pharm. (2019) 16:214–26. doi: 10.1021/acs.molpharmaceut.8b00951
25. Krasniqi A, D'Huyvetter M, Xavier C, Van der Jeught K, Muyldermans S, Van Der Heyden J, et al. Theranostic radiolabeled anti-CD20 sdAb for targeted radionuclide therapy of non-hodgkin lymphoma. Mol Cancer Ther. (2017) 16:2828–39. doi: 10.1158/1535-7163.MCT-17-0554
26. Pant K, Neuber C, Zarschler K, Wodtke J, Meister S, Haag R, et al. Active targeting of dendritic polyglycerols for diagnostic cancer imaging. Small. (2019) 16:1905013. doi: 10.1002/smll.201905013
27. Tchouate Gainkam LO, Caveliers V, Devoogdt N, Vanhove C, Xavier C, Boerman O, et al. Localization, mechanism and reduction of renal retention of technetium-99m labeled epidermal growth factor receptor-specific nanobody in mice. Contrast Media Mol Imaging. (2011) 6:85–92. doi: 10.1002/cmmi.408
28. Li C, Feng H, Xia X, Wang L, Gao B, Zhang Y, et al. 99mTc-labeled tetramer and pentamer of single-domain antibody for targeting epidermal growth factor receptor in xenografted tumors. J Label Compd Radiopharm. (2016) 59:305–12. doi: 10.1002/jlcr.3399
29. Balhuizen A, Massa S, Mathijs I, Turatsinze JV, De Vos J, Demine S, et al. A nanobody-based tracer targeting DPP6 for non-invasive imaging of human pancreatic endocrine cells. Sci Rep. (2017) 7:15130. doi: 10.1038/s41598-017-15417-2
30. Montemagno C, Cassim S, Trichanh D, Savary C, Pouyssegur J, Pagès G, et al. 99mTc-A1 as a novel imaging agent targeting mesothelin-expressing pancreatic ductal adenocarcinoma. Cancers. (2019) 11:1531. doi: 10.3390/cancers11101531
31. D'Huyvetter M, De Vos J, Xavier C, Pruszynski M, Sterckx YGJ, Massa S, et al. 131I-labeled anti-HER2 camelid sdAb as a theranostic tool in cancer treatment. Clin Cancer Res. (2017) 23:6616–28. doi: 10.1158/1078-0432.CCR-17-0310
32. Beltrán Hernández I, Rompen R, Rossin R, Xenaki KT, Katrukha EA, Nicolay K, et al. Imaging of tumor spheroids, dual-isotope SPECT, and autoradiographic analysis to assess the tumor uptake and distribution of different nanobodies. Mol Imaging Biol. (2019) 21:1079–88. doi: 10.1007/s11307-019-01320-x
33. Oliveira S, van Dongen GAMS, Walsum MS, Roovers RC, Stam JC, Mali W, et al. Rapid visualization of human tumor xenografts through optical imaging with a near-infrared fluorescent anti–epidermal growth factor receptor nanobody. Mol Imaging. (2012) 11:7290. doi: 10.2310/7290.2011.00025
34. Li D, Cheng S, Zou S, Zhu D, Zhu T, Wang P, et al. Immuno-PET imaging of 89 Zr labeled anti-PD-L1 domain antibody. Mol Pharm. (2018) 15:1674–81. doi: 10.1021/acs.molpharmaceut.8b00062
35. Xing Y, Chand G, Liu C, Cook GJR, O'Doherty J, Zhao L, et al. Early phase I study of a 99mTc-labeled anti-programmed death ligand-1 (PD-L1) single-domain antibody in SPECT/CT assessment of PD-L1 expression in non-small cell lung cancer. J Nucl Med. (2019) 60:1213–20. doi: 10.2967/jnumed.118.224170
36. Broos K, Lecocq Q, Xavier C, Bridoux J, Nguyen TT, Corthals J, et al. Evaluating a single domain antibody targeting human PD-L1 as a nuclear imaging and therapeutic agent. Cancers. (2019) 11:872. doi: 10.3390/cancers11060872
37. Broos K, Keyaerts M, Lecocq Q, Renmans D, Nguyen T, Escors D, et al. Non-invasive assessment of murine PD-L1 levels in syngeneic tumor models by nuclear imaging with nanobody tracers. Oncotarget. (2017) 8:41932–46. doi: 10.18632/oncotarget.16708
38. Lv G, Sun X, Qiu L, Sun Y, Li K, Liu Q, et al. PET imaging of tumor PD-L1 expression with a highly specific nonblocking single-domain antibody. J Nucl Med. (2020) 61:117–22. doi: 10.2967/jnumed.119.226712
39. Ingram JR, Blomberg OS, Rashidian M, Ali L, Garforth S, Fedorov E, et al. Anti-CTLA-4 therapy requires an Fc domain for efficacy. Proc Natl Acad Sci USA. (2018) 115:3912–7. doi: 10.1073/pnas.1801524115
40. Rashidian M, Keliher EJ, Dougan M, Juras PK, Cavallari M, Wojtkiewicz GR, et al. Use of 18F-2-fluorodeoxyglucose to label antibody fragments for immuno-positron emission tomography of pancreatic cancer. ACS Cent Sci. (2015) 1:142–7. doi: 10.1021/acscentsci.5b00121
41. Lecocq Q, Zeven K, De Vlaeminck Y, Martens S, Massa S, Goyvaerts C, et al. Noninvasive imaging of the immune checkpoint LAG-3 using nanobodies, from development to pre-clinical use. Biomolecules. (2019) 9:548. doi: 10.3390/biom9100548
42. Bannas P, Well L, Lenz A, Rissiek B, Haag F, Schmid J, et al. In vivo near-infrared fluorescence targeting of T cells: Comparison of nanobodies and conventional monoclonal antibodies. Contrast Media Mol Imaging. (2014) 9:135–42. doi: 10.1002/cmmi.1548
43. Rashidian M, Ingram JR, Dougan M, Dongre A, Whang KA, LeGall C, et al. Predicting the response to CTLA-4 blockade by longitudinal noninvasive monitoring of CD8 T cells. J Exp Med. (2017) 214:2243–55. doi: 10.1084/jem.20161950
44. Rashidian M, LaFleur MW, Verschoor VL, Dongre A, Zhang Y, Nguyen TH, et al. Immuno-PET identifies the myeloid compartment as a key contributor to the outcome of the antitumor response under PD-1 blockade. Proc Natl Acad Sci USA. (2019) 116:16971–80. doi: 10.1073/pnas.1905005116
45. Jailkhani N, Ingram JR, Rashidian M, Rickelt S, Tian C, Mak H, et al. Noninvasive imaging of tumor progression, metastasis, and fibrosis using a nanobody targeting the extracellular matrix. Proc Natl Acad Sci USA. (2019) 116:14181–90. doi: 10.1073/pnas.1817442116
46. Ezekowitz RA, Gordon S. Alterations of surface properties by macrophage activation: expression of receptors for Fc and mannose-terminal glycoproteins and differentiation antigens. Contemp Top Immunobiol. (1984) 13:33–56. doi: 10.1007/978-1-4757-1445-6_2
47. Movahedi K, Schoonooghe S, Laoui D, Houbracken I, Waelput W, Breckpot K, et al. Nanobody-based targeting of the macrophage mannose receptor for effective in vivo imaging of tumor-associated macrophages. Cancer Res. (2012) 72:4165–77. doi: 10.1158/0008-5472.CAN-11-2994
48. Blykers A, Schoonooghe S, Xavier C, D'Hoe K, Laoui D, D'Huyvetter M, et al. PET imaging of macrophage mannose receptor-expressing macrophages in tumor stroma using 18F-radiolabeled camelid single-domain antibody fragments. J Nucl Med. (2015) 56:1265–71. doi: 10.2967/jnumed.115.156828
49. Xavier C, Blykers A, Laoui D, Bolli E, Vaneyken I, Bridoux J, et al. Clinical translation of [68Ga]Ga-NOTA-anti-MMR-sdAb for PET/CT imaging of protumorigenic macrophages. Mol Imaging Biol. (2019) 21:898–906. doi: 10.1007/s11307-018-01302-5
50. Van Elssen CHMJ, Rashidian M, Vrbanac V, Wucherpfennig KW, El Habre Z, Sticht J, et al. Noninvasive imaging of human immune responses in a human xenograft model of graft-versus-host disease. J Nucl Med. (2017) 58:1003–8. doi: 10.2967/jnumed.116.186007
51. De Groeve K, Deschacht N, De Koninck C, Caveliers V, Lahoutte T, Devoogdt N, et al. Nanobodies as tools for in vivo imaging of specific immune cell types. J Nucl Med. (2010) 51:782–9. doi: 10.2967/jnumed.109.070078
52. Roovers RC, Laeremans T, Huang L, De Taeye S, Verkleij AJ, Revets H, et al. Efficient inhibition of EGFR signalling and of tumour growth by antagonistic anti-EGFR Nanobodies. Cancer Immunol Immunother. (2007) 56:303–17. doi: 10.1007/s00262-006-0180-4
53. Roovers RC, Vosjan MJWD, Laeremans T, El Khoulati R, De Bruin RCG, Ferguson KM, et al. A biparatopic anti-EGFR nanobody efficiently inhibits solid tumour growth. Int J Cancer. (2011) 129:2013–24. doi: 10.1002/ijc.26145
54. Zhu H, Zhao L, Li Z, Wen B, Qiu C, Liu M, et al. Preparation and characterization of humanized nanobodies targeting the dimer interface of epidermal growth factor receptor (EGFR). Protein Expr Purif. (2019) 157:57–62. doi: 10.1016/j.pep.2019.02.003
55. Tabtimmai L, Suphakun P, Srisook P, Kiriwan D, Phanthong S, Kiatwuthinon P, et al. Cell-penetrable nanobodies (transbodies) that inhibit the tyrosine kinase activity of EGFR leading to the impediment of human lung adenocarcinoma cell motility and survival. J Cell Biochem. (2019) 120:18077–87. doi: 10.1002/jcb.29111
56. Tintelnot J, Baum N, Schultheiß C, Braig F, Trentmann M, Finter J, et al. Nanobody targeting of epidermal growth factor receptor (EGFR) ectodomain variants overcomes resistance to therapeutic EGFR antibodies. Mol Cancer Ther. (2019) 18:823–33. doi: 10.1158/1535-7163.MCT-18-0849
57. Rossotti MA, Henry KA, van Faassen H, Tanha J, Callaghan D, Hussack G, et al. Camelid single-domain antibodies raised by DNA immunization are potent inhibitors of EGFR signaling. Biochem J. (2019) 476:39–50. doi: 10.1042/BCJ20180795
58. Guardiola S, Varese M, Sánchez-Navarro M, Vincke C, Teixidó M, García J, et al. Blocking EGFR activation with anti-EGF nanobodies via two distinct molecular recognition mechanisms. Angew Chem Int Ed. (2018) 57:13843–7. doi: 10.1002/anie.201807736
59. Wang H, Wang Y, Xiao Z, Li W, Dimitrov D, Chen W. Human domain antibodies to conserved epitopes on her2 potently inhibit growth of HER2-overexpressing human breast cancer cells in vitro. Antibodies. (2019) 8:25. doi: 10.3390/antib8010025
60. Hussack G, Raphael S, Lowden MJ, Henry KA. Isolation and characterization of camelid single-domain antibodies against HER2. BMC Res Notes. (2018) 11:866. doi: 10.1186/s13104-018-3955-8
61. Araste F, Ebrahimizadeh W, Rasooli I, Rajabibazl M, Mousavi Gargari SL. A novel VHH nanobody against the active site (the CA domain) of tumor-associated, carbonic anhydrase isoform IX and its usefulness for cancer diagnosis. Biotechnol Lett. (2014) 36:21–8. doi: 10.1007/s10529-013-1340-1
62. Huet HA, Growney JD, Johnson JA, Li J, Bilic S, Ostrom L, et al. Multivalent nanobodies targeting death receptor 5 elicit superior tumor cell killing through efficient caspase induction. MAbs. (2014) 6:1560–70. doi: 10.4161/19420862.2014.975099
63. Sadeghnezhad G, Romão E, Bernedo-Navarro R, Massa S, Khajeh K, Muyldermans S, et al. Identification of new DR5 agonistic nanobodies and generation of multivalent nanobody constructs for cancer treatment. Int J Mol Sci. (2019)20:4818. doi: 10.3390/ijms20194818
64. Slørdahl TS, Denayer T, Moen SH, Standal T, Børset M, Ververken C, et al. Anti-c-MET nanobody ® - a new potential drug in multiple myeloma treatment. Eur J Haematol. (2013) 91:399–410. doi: 10.1111/ejh.12185
65. Godar M, Morello V, Sadi A, Hultberg A, De Jonge N, Basilico C, et al. Dual anti-idiotypic purification of a novel, native-format biparatopic anti-MET antibody with improved in vitro and in vivo efficacy. Sci Rep. (2016) 6:31621. doi: 10.1038/srep31621
66. Vosjan MJWD, Vercammen J, Kolkman JA, Stigter-Van Walsum M, Revets H, Van Dongen GAMS. Nanobodies targeting the hepatocyte growth factor: potential new drugs for molecular cancer therapy. Mol Cancer Ther. (2012) 11:1017–25. doi: 10.1158/1535-7163.MCT-11-0891
67. Rashidi SK, Gargari SLM, Ebrahimizadeh W. Targeting colorectal cancer cell lines using nanobodies; AgSK1as a potential target. Iran J Biotechnol. (2017) 15:78–86. doi: 10.15171/ijb.1472
68. Tang Z, Feng M, Gao W, Phung Y, Chen W, Chaudhary A, et al. A human single-domain antibody elicits potent antitumor activity by targeting an epitope in mesothelin close to the cancer cell surface. Mol Cancer Ther. (2013) 12:416–26. doi: 10.1158/1535-7163.MCT-12-0731
69. Sánchez-Martín D, Martínez-Torrecuadrada J, Teesalu T, Sugahara KN, Alvarez-Cienfuegos A, Ximénez-Embún P, et al. Proteasome activator complex PA28 identified as an accessible target in prostate cancer by in vivo selection of human antibodies. Proc Natl Acad Sci USA. (2013) 110:13791–6. doi: 10.1073/pnas.1300013110
70. Schoonaert L, Rué L, Roucourt B, Timmers M, Little S, Chávez-Gutiérrez L, et al. Identification and characterization of nanobodies targeting the EphA4 receptor. J Biol Chem. (2017) 292:11452–65. doi: 10.1074/jbc.M116.774141
71. Cheng TM, Murad YM, Chang CC, Yang MC, Baral TN, Cowan A, et al. Single domain antibody against carcinoembryonic antigen-related cell adhesion molecule 6 (CEACAM6) inhibits proliferation, migration, invasion and angiogenesis of pancreatic cancer cells. Eur J Cancer. (2014) 50:713–21. doi: 10.1016/j.ejca.2012.07.019
72. Samec N, Jovcevska I, Stojan J, Zottel A, Liovic M, Myers MP, et al. Glioblastoma-specific anti-TUFM nanobody for in-vitro immunoimaging and cancer stem cell targeting. Oncotarget. (2018) 9:17282–99. doi: 10.18632/oncotarget.24629
73. Wang D, Hu X, Liu C, Jia Y, Bai Y, Cai C, et al. Protein C receptor is a therapeutic stem cell target in a distinct group of breast cancers. Cell Res. (2019) 29:832–45. doi: 10.1038/s41422-019-0225-9
74. Fenderico N, van Scherpenzeel RC, Goldflam M, Proverbio D, Jordens I, Kralj T, et al. Anti-LRP5/6 VHHs promote differentiation of Wnt-hypersensitive intestinal stem cells. Nat Commun. (2019) 10:365. doi: 10.1038/s41467-018-08172-z
75. Romão E, Krasniqi A, Maes L, Vandenbrande C, Sterckx YGJ, Stijlemans B, et al. Identification of nanobodies against the acute myeloid leukemia marker cd33. Int J Mol Sci. (2020) 21:310. doi: 10.3390/ijms21010310
76. Rotte A. Combination of CTLA-4 and PD-1 blockers for treatment of cancer. J Exp Clin Cancer Res. (2019) 38:1–12. doi: 10.1186/s13046-019-1259-z
77. Zhang F, Wei H, Wang X, Bai Y, Wang P, Wu J, et al. Structural basis of a novel PD-L1 nanobody for immune checkpoint blockade. Cell Discov. (2017) 3:17004. doi: 10.1038/celldisc.2017.4
78. Sun X, Yan X, Zhuo W, Gu J, Zuo K, Liu W, et al. PD-l1 nanobody competitively inhibits the formation of the PD-1/PD-l1 complex: Comparative molecular dynamics simulations. Int J Mol Sci. (2018) 19:1984. doi: 10.3390/ijms19071984
79. Xian Z, Ma L, Zhu M, Li G, Gai J, Chang Q, et al. Blocking the PD-1-PD-L1 axis by a novel PD-1 specific nanobody expressed in yeast as a potential therapeutic for immunotherapy. Biochem Biophys Res Commun. (2019) 519:267–73. doi: 10.1016/j.bbrc.2019.08.160
80. Wen B, Zhao L, Wang Y, Qiu C, Xu Z, Huang K, et al. Nanobodies targeting the interaction interface of programmed death receptor 1 (PD-1)/PD-1 ligand 1 (PD-1/PD-L1). Prep Biochem Biotechnol. (2019) 50:252–9. doi: 10.1080/10826068.2019.1692217
81. Li S, Jiang K, Wang T, Zhang W, Shi M, Chen B, et al. Nanobody against PDL1. Biotechnol Lett. (2020) 42:727–36. doi: 10.1007/s10529-020-02823-2
82. Wan R, Liu A, Hou X, Lai Z, Li J, Yang N, et al. Screening and antitumor effect of an anti-CTLA-4 nanobody. Oncol Rep. (2018) 39:511–8. doi: 10.3892/or.2017.6131
83. Homayouni V, Ganjalikhani-Hakemi M, Rezaei A, Khanahmad H, Behdani M, Lomedasht FK. Preparation and characterization of a novel nanobody against T-cell immunoglobulin and mucin-3 (TIM-3). Iran J Basic Med Sci. (2016) 19:1201–8. doi: 10.15171/ijb.1427
84. Sikic BI, Lakhani N, Patnaik A, Shah SA, Chandana SR, Rasco D, et al. First-in-human, first-in-class phase I trial of the anti-CD47 antibody Hu5F9-G4 in patients with advanced cancers. J Clin Oncol. (2019) 37:946–53. doi: 10.1200/JCO.18.02018
85. Ingram JR, Blomberg OS, Sockolosky JT, Ali L, Schmidt FI, Pishesha N, et al. Localized CD47 blockade enhances immunotherapy for murine melanoma. Proc Natl Acad Sci USA. (2017) 114:10184–9. doi: 10.1073/pnas.1710776114
86. Sockolosky JT, Dougan M, Ingram JR, Ho CCM, Kauke MJ, Almo SC, et al. Durable antitumor responses to CD47 blockade require adaptive immune stimulation. Proc Natl Acad Sci USA. (2016) 113:E2646–54. doi: 10.1073/pnas.1604268113
87. Ma L, Zhu M, Gai J, Li G, Chang Q, Qiao P, et al. Preclinical development of a novel CD47 nanobody with less toxicity and enhanced anti-cancer therapeutic potential. J Nanobiotechnol. (2020) 18:12. doi: 10.1186/s12951-020-0571-2
88. Farajpour Z, Rahbarizadeh F, Kazemi B, Ahmadvand D. A nanobody directed to a functional epitope on VEGF, as a novel strategy for cancer treatment. Biochem Biophys Res Commun. (2014) 446:132–6. doi: 10.1016/j.bbrc.2014.02.069
89. Sadeghi A, Behdani M, Muyldermans S, Habibi-Anbouhi M, Kazemi-Lomedasht F. Development of a mono-specific anti-VEGF bivalent nanobody with extended plasma half-life for treatment of pathologic neovascularization. Drug Test Anal. (2019) 12:92–100. doi: 10.1002/dta.2693
90. Khodabakhsh F, Norouzian D, Vaziri B, Ahangari Cohan R, Sardari S, Mahboudi F, et al. Development of a novel nano-sized anti-VEGFA nanobody with enhanced physicochemical and pharmacokinetic properties. Artif Cells Nanomed Biotechnol. (2018) 46:1402–14. doi: 10.1080/21691401.2017.1369426
91. Behdani M, Zeinali S, Khanahmad H, Karimipour M, Asadzadeh N, Azadmanesh K, et al. Generation and characterization of a functional nanobody against the vascular endothelial growth factor receptor-2; angiogenesis cell receptor. Mol Immunol. (2012) 50:35–41. doi: 10.1016/j.molimm.2011.11.013
92. Baharlou R, Tajik N, Behdani M, Shokrgozar MA, Tavana V, Kazemi-Lomedasht F, et al. An antibody fragment against human delta-like ligand-4 for inhibition of cell proliferation and neovascularization. Immunopharmacol Immunotoxicol. (2018) 40:368–74. doi: 10.1080/08923973.2018.1505907
93. Khatibi AS, Roodbari NH, Majidzade-A K, Yaghmaei P, Farahmand L. Tumor-suppressing and anti-angiogenic activities of a recombinant anti-CD3ϵ nanobody in breast cancer mice model. Immunotherapy. (2019) 11:1555–67. doi: 10.2217/imt-2019-0068
94. Xing J, Lin L, Li J, Liu J, Zhou C, Pan H, et al. BiHC, a T-cell–engaging bispecific recombinant antibody, has potent cytotoxic activity against her2 tumor cells. Transl Oncol. (2017) 10:780–5. doi: 10.1016/j.tranon.2017.07.003
95. Ahn S, Li J, Sun C, Gao K, Hirabayashi K, Li H, et al. Cancer immunotherapy with T cells carrying bispecific receptors that mimic antibodies. Cancer Immunol Res. (2019) 7:773–83. doi: 10.1158/2326-6066.CIR-18-0636
96. Li L, He P, Zhou C, Jing L, Dong B, Chen S, et al. A novel bispecific antibody, S-Fab, induces potent cancer cell killing. J Immunother. (2015) 38:350–6. doi: 10.1097/CJI.0000000000000099
97. Pan H, Liu J, Deng W, Xing J, Li Q, Wang Z. Site-specific PEGylation of an anti-CEA/CD3 bispecific antibody improves its antitumor efficacy. Int J Nanomedicine. (2018) 13:3189–201. doi: 10.2147/IJN.S164542
98. Kuhn C, Weiner HL. Therapeutic anti-CD3 monoclonal antibodies: from bench to bedside. Immunotherapy. (2016) 8:889–906. doi: 10.2217/imt-2016-0049
99. Moradi-Kalbolandi S, Sharifi-K A, Darvishi B, Majidzadeh-A K, jalili N, Sadeghi S, et al. Evaluation the potential of recombinant anti-CD3 nanobody on immunomodulatory function. Mol Immunol. (2020) 118:174–81. doi: 10.1016/j.molimm.2019.12.017
100. Mølgaard K, Harwood SL, Compte M, Merino N, Bonet J, Alvarez-Cienfuegos A, et al. Bispecific light T-cell engagers for gene-based immunotherapy of epidermal growth factor receptor (EGFR)-positive malignancies. Cancer Immunol Immunother. (2018) 67:1251–60. doi: 10.1007/s00262-018-2181-5
101. Harwood SL, Alvarez-Cienfuegos A, Nuñez-Prado N, Compte M, Hernández-Pérez S, Merino N, et al. ATTACK, a novel bispecific T cell-recruiting antibody with trivalent EGFR binding and monovalent CD3 binding for cancer immunotherapy. Oncoimmunology. (2018) 7:e1377874. doi: 10.1080/2162402X.2017.1377874
102. Compte M, Harwood SL, Muñoz IG, Navarro R, Zonca M, Perez-Chacon G, et al. A tumor-targeted trimeric 4-1BB-agonistic antibody induces potent anti-tumor immunity without systemic toxicity. Nat Commun. (2018) 9:4809. doi: 10.1038/s41467-018-07195-w
103. Mikkelsen K, Harwood SL, Compte M, Merino N, Mølgaard K, Lykkemark S, et al. Carcinoembryonic antigen (CEA)-specific 4-1BB-costimulation induced by CEA-targeted 4-1BB-agonistic trimerbodies. Front Immunol. (2019) 10:1791. doi: 10.3389/fimmu.2019.01791
104. Bakhtiari SHA, Rahbarizadeh F, Hasannia S, Ahmadvand D, Iri-Sofla FJ, Rasaee MJ. Anti-MUC1 nanobody can redirect T-body cytotoxic effector function. Hybridoma. (2009) 28:85–92. doi: 10.1089/hyb.2008.0079
105. You F, Wang Y, Jiang L, Zhu X, Chen D, Yuan L, et al. A novel CD7 chimeric antigen receptor-modified NK-92MI cell line targeting T-cell acute lymphoblastic leukemia. Am J Cancer Res. (2019) 9:64–78.
106. An N, Hou YN, Zhang QX, Li T, Zhang QL, Fang C, et al. Anti-multiple myeloma activity of nanobody-based anti-CD38 chimeric antigen receptor T cells. Mol Pharm. (2018) 15:4577–88. doi: 10.1021/acs.molpharmaceut.8b00584
107. Hajari Taheri F, Hassani M, Sharifzadeh Z, Behdani M, Arashkia A, Abolhassani M. T cell engineered with a novel nanobody-based chimeric antigen receptor against VEGFR2 as a candidate for tumor immunotherapy. IUBMB Life. (2019) 71:1259–67. doi: 10.1002/iub.2019
108. Hassani M, Hajari Taheri F, Sharifzadeh Z, Arashkia A, Hadjati J, van Weerden WM, et al. Construction of a chimeric antigen receptor bearing a nanobody against prostate a specific membrane antigen in prostate cancer. J Cell Biochem. (2019) 120:10787–95. doi: 10.1002/jcb.28370
109. Hassani M, Hajari Taheri F, Sharifzadeh Z, Arashkia A, Hadjati J, van Weerden WM, et al. Engineered jurkat cells for targeting prostate-specific membrane antigen on prostate cancer cells by nanobody-based chimeric antigen receptor. Iran Biomed J. (2020) 24:81–8. doi: 10.29252/ibj.24.2.81
110. Li N, Fu H, Hewitt SM, Dimitrov DS, Ho M. Therapeutically targeting glypican-2 via single-domain antibody-based chimeric antigen receptors and immunotoxins in neuroblastoma. Proc Natl Acad Sci USA. (2017) 114:E6623–31. doi: 10.1073/pnas.1706055114
111. Zhang G, Wang L, Cui H, Wang X, Zhang G, Ma J, et al. Anti-melanoma activity of T cells redirected with a TCR-like chimeric antigen receptor. Sci Rep. (2014) 4:3571. 10.1038/srep03571. doi: 10.1038/srep03571
112. De Munter S, Ingels J, Goetgeluk G, Bonte S, Pille M, Weening K, et al. Nanobody based dual specific CARs. Int J Mol Sci. (2018) 19:403. doi: 10.3390/ijms19020403
113. Xie YJ, Dougan M, Jailkhani N, Ingram J, Fang T, Kummer L, et al. Nanobody-based CAR T cells that target the tumor microenvironment inhibit the growth of solid tumors in immunocompetent mice. Proc Natl Acad Sci USA. (2019) 116:7624–31. doi: 10.1073/pnas.1817147116
114. Xie YJ, Dougan M, Ingram JR, Pishesha N, Fang T, Momin N, et al. Improved anti-tumor efficacy of chimeric antigen receptor T cells that secrete single-domain antibody fragments. Cancer Immunol Res. (2020) 8:canimm.0734.2019. doi: 10.1158/2326-6066.CIR-19-0734
115. Cartellieri M, Feldmann A, Koristka S, Arndt C, Loff S, Ehninger A, et al. Switching CAR T cells on and off: a novel modular platform for retargeting of T cells to AML blasts. Blood Cancer J. (2016) 6:e458. doi: 10.1038/bcj.2016.61
116. Albert S, Arndt C, Feldmann A, Bergmann R, Bachmann D, Koristka S, et al. A novel nanobody-based target module for retargeting of T lymphocytes to EGFR-expressing cancer cells via the modular UniCAR platform. Oncoimmunology. (2017) 6:e1287246. doi: 10.1080/2162402X.2017.1287246
117. Albert S, Arndt C, Koristka S, Berndt N, Bergmann R, Feldmann A, et al. From mono- to bivalent: improving theranostic properties of target modules for redirection of UniCAR T cells against EGFR-expressing tumor cells in vitro and in vivo. Oncotarget. (2018) 9:25597–616. doi: 10.18632/oncotarget.25390
118. de Bruin RCG, Veluchamy JP, Lougheed SM, Schneiders FL, Lopez-Lastra S, Lameris R, et al. A bispecific nanobody approach to leverage the potent and widely applicable tumor cytolytic capacity of Vγ9Vδ2-T cells. Oncoimmunology. (2017) 7:e1375641. doi: 10.1080/2162402X.2017.1375641
119. Zhao Y, Li Y, Wu X, Li L, Liu J, Wang Y, et al. Identification of anti-CD16a single domain antibodies and their application in bispecific antibodies. Cancer Biol Ther. (2019) 21:72–80. doi: 10.1080/15384047.2019.1665953
120. Dong B, Zhou C, He P, Li J, Chen S, Miao J, et al. A novel bispecific antibody, BiSS, with potent anti-cancer activities. Cancer Biol Ther. (2016) 17:364–70. doi: 10.1080/15384047.2016.1139266
121. Li J, Zhou C, Dong B, Zhong H, Chen S, Li Q, et al. Single domain antibody-based bispecific antibody induces potent specific anti-tumor activity. Cancer Biol Ther. (2016) 17:1231–9. doi: 10.1080/15384047.2016.1235659
122. Rozan C, Cornillon A, Petiard C, Chartier M, Behar G, Boix C, et al. Single-domain antibody-based and linker-free bispecific antibodies targeting fcgriii induce potent antitumor activity without recruiting regulatory T cells. Mol Cancer Ther. (2013) 12:1481–91. doi: 10.1158/1535-7163.MCT-12-1012
123. Li Y, Zhou C, Li J, Liu J, Lin L, Li L, et al. Single domain based bispecific antibody, Muc1-Bi-1, and its humanized form, Muc1-Bi-2, induce potent cancer cell killing in muc1 positive tumor cells. PLoS ONE. (2018) 13:E0191024. doi: 10.1371/journal.pone.0191024
124. Turini M, Chames P, Bruhns P, Baty D, Kerfelec B. A FcγRIII-engaging bispecific antibody expands the range of HER2-expressing breast tumors eligible to antibody therapy. Oncotarget. (2014) 5:5304–19. doi: 10.18632/oncotarget.2093
125. Deng W, Liu J, Pan H, Li L, Zhou C, Wang X, et al. A bispecific antibody based on pertuzumab fab has potent antitumor activity. J Immunother. (2018) 41:1–8. doi: 10.1097/CJI.0000000000000200
126. Li A, Xing J, Li L, Zhou C, Dong B, He P, et al. A single-domain antibody-linked fab bispecific antibody her2-S-Fab has potent cytotoxicity against her2-expressing tumor cells. AMB Express. (2016) 6:32. doi: 10.1186/s13568-016-0201-4
127. Wang Y, Liu J, Pan H, Xing J, Wu X, Li Q, et al. A GPC3-targeting bispecific antibody, GPC3-S-Fab, with potent cytotoxicity. J Vis Exp. (2018) 12:57588. doi: 10.3791/57588
128. Duarte JN, Cragnolini JJ, Swee LK, Bilate AM, Bader J, Ingram JR, et al. Generation of immunity against pathogens via single-domain antibody–antigen constructs. J Immunol. (2016) 197:4838–47. doi: 10.4049/jimmunol.1600692
129. Woodham AW, Cheloha RW, Ling J, Rashidian M, Kolifrath SC, Mesyngier M, et al. Nanobody–antigen conjugates elicit HPV-specific antitumor immune responses. Cancer Immunol Res. (2018) 6:870–80. doi: 10.1158/2326-6066.CIR-17-0661
130. Fang T, Van Elssen CHMJ, Duarte JN, Guzman JS, Chahal JS, Ling J, et al. Targeted antigen delivery by an anti-class II MHC VHH elicits focused αmUC1(Tn) immunity. Chem Sci. (2017) 8:5591–7. doi: 10.1039/C7SC00446J
131. Tang Z, Mo F, Liu A, Duan S, Yang X, Liang L, et al. A nanobody against cytotoxic t-lymphocyte associated antigen-4 increases the anti-tumor effects of specific cd8+ T cells. J Biomed Nanotechnol. (2019) 15:2229–39. doi: 10.1166/jbn.2019.2859
132. Broos K, Lecocq Q, De Keersmaecker B, Raes G, Corthals J, Lion E, et al. Single domain antibody-mediated blockade of programmed death-ligand 1 on dendritic cells enhances CD8 T-cell activation and cytokine production. Vaccines. (2019) 7:85. doi: 10.3390/vaccines7030085
133. Kwon S, Duarte JN, Li Z, Ling JJ, Cheneval O, Durek T, et al. Targeted delivery of cyclotides via conjugation to a nanobody. ACS Chem Biol. (2018) 13:2973–80. doi: 10.1021/acschembio.8b00653
134. Hauck B, Xiao W. Characterization of tissue tropism determinants of adeno-associated virus type 1. J Virol. (2003) 77:2768–74. doi: 10.1128/JVI.77.4.2768-2774.2003
135. Goyvaerts C, Dingemans J, De Groeve K, Heirman C, Van Gulck E, Vanham G, et al. Targeting of human antigen-presenting cell subsets. J Virol. (2013) 87:11304–8. doi: 10.1128/JVI.01498-13
136. Goyvaerts C, De Vlaeminck Y, Escors D, Lienenklaus S, Keyaerts M, Raes G, et al. Antigen-presenting cell-targeted lentiviral vectors do not support the development of productive T-cell effector responses: implications for in vivo targeted vaccine delivery. Gene Ther. (2017) 24:370–5. doi: 10.1038/gt.2017.30
137. Crowley SJ, Bruck PT, Bhuiyan MA, Mitchell-Gears A, Walsh MJ, Zhangxu K, et al. Neoleukin-2 enhances anti-tumour immunity downstream of peptide vaccination targeted by an anti-MHC class II VHH. Open Biol. (2020) 10:190235. doi: 10.1098/rsob.190235
138. Eichhoff AM, Börner K, Albrecht B, Schäfer W, Baum N, Haag F, et al. Nanobody-enhanced targeting of AAV gene therapy vectors. Mol Ther Methods Clin Dev. (2019) 15:211–20. doi: 10.1016/j.omtm.2019.09.003
139. Ahani R, Roohvand F, Cohan RA, Etemadzadeh MH, Mohajel N, Behdani M, et al. Sindbis virus-pseudotyped lentiviral vectors carrying VEGFR2-specific nanobody for potential transductional targeting of tumor vasculature. Mol Biotechnol. (2016) 58:738–47. doi: 10.1007/s12033-016-9973-7
140. Shoae-Hassani A, Mortazavi-Tabatabaei SA, Sharif S, Madadi S, Rezaei-Khaligh H, Verdi J. Recombinant λ bacteriophage displaying nanobody towards third domain of HER-2 epitope inhibits proliferation of breast carcinoma SKBR-3 cell line. Arch Immunol Ther Exp. (2013) 61:75–83. doi: 10.1007/s00005-012-0206-x
141. Dougan M, Ingram JR, Jeong HJ, Mosaheb MM, Bruck PT, Ali L, et al. Targeting cytokine therapy to the pancreatic tumor microenvironment using PD-L1–specific VHHs. Cancer Immunol Res. (2018) 6:389–401. doi: 10.1158/2326-6066.CIR-17-0495
142. Liu Y, Wang Y, Xing J, Li Y, Liu J, Wang Z. A novel multifunctional anti-CEA-IL15 molecule displays potent antitumor activities. Drug Des Devel Ther. (2018) 12:2645–54. doi: 10.2147/DDDT.S166373
143. Ji X, Peng Z, Li X, Yan Z, Yang Y, Qiao Z, et al. Neutralization of TNFα in tumor with a novel nanobody potentiates paclitaxel-therapy and inhibits metastasis in breast cancer. Cancer Lett. (2017) 386:24–34. doi: 10.1016/j.canlet.2016.10.031
144. Desmyter A, Spinelli S, Boutton C, Saunders M, Blachetot C, de Haard H, et al. Neutralization of human interleukin 23 by multivalent nanobodies explained by the structure of cytokine–nanobody complex. Front Immunol. (2017) 8:884. doi: 10.3389/fimmu.2017.00884
145. Bakherad H, Gargari SLM, Sepehrizadeh Z, Aghamollaei H, Taheri RA, Torshabi M, et al. Identification and in vitro characterization of novel nanobodies against human granulocyte colony-stimulating factor receptor to provide inhibition of G-CSF function. Biomed Pharmacother. (2017) 93:245–54. doi: 10.1016/j.biopha.2017.06.025
146. Henry KA, Hussack G, Collins C, Zwaagstra JC, Tanha J, Mackenzie CR. Isolation of TGF-β-neutralizing single-domain antibodies of predetermined epitope specificity using next-generation DNA sequencing. Protein Eng Des Sel. (2016) 29:439–43. doi: 10.1093/protein/gzw043
147. Sadeghian-Rizi T, Behdani M, Khanahmad H, Sadeghi HM, Jahanian-Najafabadi A. Generation and characterization of a functional nanobody against inflammatory chemokine CXCL10, as a novel strategy for the treatment of multiple sclerosis. CNS Neurol Disord Drug Targets. (2019) 18:141–8. doi: 10.2174/1871527317666181114134518
148. Fang T, Li R, Li Z, Cho J, Guzman JS, Kamm RD, et al. Remodeling of the tumor microenvironment by a chemokine/anti-pd-l1 nanobody fusion protein. Mol Pharm. (2019) 16:2838–44. doi: 10.1021/acs.molpharmaceut.9b00078
149. Peyrassol X, Laeremans T, Gouwy M, Lahura V, Debulpaep M, Van Damme J, et al. Development by genetic immunization of monovalent antibodies (nanobodies) behaving as antagonists of the human chemr23 receptor. J Immunol. (2016) 196:2893–901. doi: 10.4049/jimmunol.1500888
150. Heukers R, Fan TS, de Wit RH, van Senten JR, De Groof TWM, Bebelman MP, et al. The constitutive activity of the virally encoded chemokine receptor US28 accelerates glioblastoma growth. Oncogene. (2018) 37:4110–21. doi: 10.1038/s41388-018-0255-7
151. Jakobs BD, Spannagel L, Purvanov V, Allmen EU, Von Matti C, Legler DF. Engineering of nanobodies recognizing the human chemokine receptor CCR7. Int J Mol Sci. (2019) 20:2597. doi: 10.3390/ijms20102597
152. Van Hout A, Klarenbeek A, Bobkov V, Doijen J, Arimont M, Zhao C, et al. CXCR4-targeting nanobodies differentially inhibit CXCR4 function and HIV entry. Biochem Pharmacol. (2018) 158:402–12. doi: 10.1016/j.bcp.2018.10.015
153. Griffiths K, Dolezal O, Cao B, Nilsson SK, See HB, Pfleger KDG, et al. i-bodies, human single domain antibodies that antagonize chemokine receptor CXCR4. J Biol Chem. (2016) 291:12641–57. doi: 10.1074/jbc.M116.721050
154. Bobkov V, Arimont M, Zarca A, de Groof TWM, van der Woning B, de Haard H, et al. Antibodies targeting chemokine receptors CXCR4 and ACKR3. Mol Pharmacol. (2019) 96:753–64. doi: 10.1124/mol.119.116954
155. Maussang D, Mujić-Delić A, Descamps FJ, Stortelers C, Vanlandschoot P, Stigter-Van Walsum M, et al. Llama-derived single variable domains (nanobodies) directed against chemokine receptor CXCR7 reduce head and neck cancer cell growth in vivo. J Biol Chem. (2013) 288:29562–72. doi: 10.1074/jbc.M113.498436
156. Smolarek D, Hattab C, Hassanzadeh-Ghassabeh G, Cochet S, Gutiérrez C, De Brevern AG, et al. A recombinant dromedary antibody fragment (VHH or nanobody) directed against human duffy antigen receptor for chemokines. Cell Mol Life Sci. (2010) 67:3371–87. doi: 10.1007/s00018-010-0387-6
157. De Clercq S, Zwaenepoel O, Martens E, Vandekerckhove J, Guillabert A, Gettemans J. Nanobody-induced perturbation of LFA-1/L-plastin phosphorylation impairs MTOC docking, immune synapse formation and T cell activation. Cell Mol Life Sci. (2013) 70:909–22. doi: 10.1007/s00018-012-1169-0
158. Fleming BD, Urban DJ, Hall M, Longerich T, Greten T, Pastan I, et al. The engineered anti-GPC3 immunotoxin, HN3-ABD-T20, produces regression in mouse liver cancer xenografts via prolonged serum retention. Hepatology. (2019) 71:1696–711. doi: 10.1002/hep.30949
159. Wang C, Gao W, Feng M, Pastan I, Ho M. Construction of an immunotoxin, HN3-mPE24, targeting glypican-3 for liver cancer therapy. Oncotarget. (2017) 8:32450–60. doi: 10.18632/oncotarget.10592
160. Behdani M, Zeinali S, Karimipour M, Khanahmad H, Schoonooghe S, Aslemarz A, et al. Development of VEGFR2-specific nanobody pseudomonas exotoxin a conjugated to provide efficient inhibition of tumor cell growth. N Biotechnol. (2013) 30:205–9. doi: 10.1016/j.nbt.2012.09.002
161. Yu Y, Li J, Zhu X, Tang X, Bao Y, Sun X, et al. Humanized CD7 nanobody-based immunotoxins exhibit promising anti-T-cell acute lymphoblastic leukemia potential. Int J Nanomed. (2017) 12:1969–83. doi: 10.2147/IJN.S127575
162. Tang J, Li J, Zhu X, Yu Y, Chen D, Yuan L, et al. Novel CD7-specific nanobody-based immunotoxins potently enhanced apoptosis of CD7-positive malignant cells. Oncotarget. (2016) 7:34070–83. doi: 10.18632/oncotarget.8710
163. Sokolova E, Guryev E, Yudintsev A, Vodeneev V, Deyev S, Balalaeva I. HER2-specific recombinant immunotoxin 4D5scFv-PE40 passes through retrograde trafficking route and forces cells to enter apoptosis. Oncotarget. (2017) 8:22048–58. doi: 10.18632/oncotarget.15833
164. Li T, Qi S, Unger M, Hou YN, Deng QW, Liu J, et al. Immuno-targeting the multifunctional CD38 using nanobody. Sci Rep. (2016) 6:27055. doi: 10.1038/srep27055
165. Cao L, Li Q, Tong Z, Xing Y, Xu K, Yijia Wang J, et al. HER2-specific immunotoxins constructed based on single-domain antibodies and the improved toxin PE24X7. Int J Pharm. (2020) 574:118939. doi: 10.1016/j.ijpharm.2019.118939
166. Cortez-Retamozo V, Backmann N, Senter PD, Wernery U, De Baetselier P, Muyldermans S, et al. Efficient cancer therapy with a nanobody-based conjugate. Cancer Res. (2004) 64:2853–7. doi: 10.1158/0008-5472.CAN-03-3935
167. Massa PE, Paniccia A, Monegal A, De Marco A, Rescigno M. Salmonella engineered to express CD20-targeting antibodies and a drug-converting enzyme can eradicate human lymphomas. Blood. (2013) 122:705–14. doi: 10.1182/blood-2012-12-474098
168. Tian B, Wong WY, Hegmann E, Gaspar K, Kumar P, Chao H. Production and characterization of a camelid single domain antibody-urease enzyme conjugate for the treatment of cancer. Bioconjug Chem. (2015) 26:1144–55. doi: 10.1021/acs.bioconjchem.5b00237
169. Tian B, Wong WY, Uger MD, Wisniewski P, Chao H. National Research Council of Canada (NRC)Development and characterization of a camelid single domain antibody– urease conjugate that targets vascular endothelial growth factor receptor 2. Front Immunol. (2017) 8:956. doi: 10.3389/fimmu.2017.00956
170. De Vlaeminck Y, Lecocq Q, Giron P, Heirman C, Geeraerts X, Bolli E, et al. Single-domain antibody fusion proteins can target and shuttle functional proteins into macrophage mannose receptor expressing macrophages. J Control Release. (2019) 299:107–20. doi: 10.1016/j.jconrel.2019.02.023
171. Huang H, Wu T, Shi H, Wu Y, Yang H, Zhong K, et al. Modular design of nanobody-drug conjugates for targeted-delivery of platinum anticancer drugs with an MRI contrast agent. Chem Commun. (2019) 55:5175–8. doi: 10.1039/C9CC01391A
172. Deng C, Xiong J, Gu X, Chen X, Wu S, Wang Z, et al. Novel recombinant immunotoxin of EGFR specific nanobody fused with cucurmosin, construction and antitumor efficiency in vitro. Oncotarget. (2017) 8:38568–80. doi: 10.18632/oncotarget.16930
173. Fang T, Duarte JN, Ling J, Li Z, Guzman JS, Hidde P, et al. Structurally-defined αMHC-II nanobody-drug conjugates: therapeutic and imaging platforms for B-cell lymphoma. Angew Chem Int Ed Engl. (2016) 55:2416–20. doi: 10.1002/anie.201509432
174. Wang Y, Wang Y, Chen G, Li Y, Xu W, Gong S. Quantum-dot-based theranostic micelles conjugated with an anti-EGFR NANOBODY FOR TRIPLE-NEGATIVE BREAST CANCER THERAPY. ACS Appl Mater Interfaces. (2017) 9:30297–305. doi: 10.1021/acsami.7b05654
175. Costa SA, Mozhdehi D, Dzuricky MJ, Isaacs FJ, Brustad EM, Chilkoti A. Active targeting of cancer cells by nanobody decorated polypeptide micelle with bio-orthogonally conjugated drug. Nano Lett. (2019) 19:247–54. doi: 10.1021/acs.nanolett.8b03837
176. Talelli M, Oliveira S, Rijcken CJF, Pieters EHE, Etrych T, Ulbrich K, et al. Intrinsically active nanobody-modified polymeric micelles for tumor-targeted combination therapy. Biomaterials. (2013) 34:1255–60. doi: 10.1016/j.biomaterials.2012.09.064
177. van der Meel R, Oliveira S, Altintas I, Heukers R, Pieters EHE, van Bergen en Henegouwen PMP, et al. Inhibition of tumor growth by targeted anti-EGFR/IGF-1R nanobullets depends on efficient blocking of cell survival pathways. Mol Pharm. (2013) 10:3717–27. doi: 10.1021/mp400212v
178. Oliveira S, Schiffelers RM, van der Veeken J, van der Meel R, Vongpromek R, van Bergen en Henegouwen PMP, et al. Downregulation of EGFR by a novel multivalent nanobody-liposome platform. J Control Release. (2010) 145:165–75. doi: 10.1016/j.jconrel.2010.03.020
179. Yin W, Yu X, Kang X, Zhao Y, Zhao P, Jin H, et al. Remodeling tumor-associated macrophages and neovascularization overcomes egfrt790m-associated drug resistance by pd-l1 nanobody-mediated codelivery. Small. (2018) 14:e1802372. doi: 10.1002/smll.201802372
180. Yang YSS, Moynihan KD, Bekdemir A, Dichwalkar TM, Noh MM, Watson N, et al. Targeting small molecule drugs to T cells with antibody-directed cell-penetrating gold nanoparticles. Biomater Sci. (2019) 7:113–24. doi: 10.1039/C8BM01208C
181. Debets MF, Leenders WPJ, Verrijp K, Zonjee M, Meeuwissen SA, Otte-Höller I, et al. Nanobody-Functionalized polymersomes for tumor-vessel targeting. Macromol Biosci. (2013) 13:938–45. doi: 10.1002/mabi.201300039
182. Heukers R, Altintas I, Raghoenath S, De Zan E, Pepermans R, Roovers RC, et al. Targeting hepatocyte growth factor receptor (Met) positive tumor cells using internalizing nanobody-decorated albumin nanoparticles. Biomaterials. (2014) 35:601–10. doi: 10.1016/j.biomaterials.2013.10.001
183. Altintas I, Heukers R, Van Der Meel R, Lacombe M, Amidi M, Van Bergen En Henegouwen PMP, et al. Nanobody-albumin nanoparticles (NANAPs) for the delivery of a multikinase inhibitor 17864 to EGFR overexpressing tumor cells. J Control Release. (2013) 165:110–8. doi: 10.1016/j.jconrel.2012.11.007
184. Saqafi B, Rahbarizadeh F. Polyethyleneimine-polyethylene glycol copolymer targeted by anti-HER2 nanobody for specific delivery of transcriptionally targeted tBid containing construct. Artif Cells Nanomed Biotechnol. (2019) 47:501–11. doi: 10.1080/21691401.2018.1549063
185. Martínez-Jothar L, Beztsinna N, Van Nostrum CF, Hennink WE, Oliveira S. Selective cytotoxicity to HER2 positive breast cancer cells by saporin-loaded nanobody-targeted polymeric nanoparticles in combination with photochemical internalization. Mol Pharm. (2019) 16:1633–47. doi: 10.1021/acs.molpharmaceut.8b01318
186. Kooijmans SAA, Aleza CG, Roffler SR, van Solinge WW, Vader P, Schiffelers RM. Display of GPI-anchored anti-EGFR nanobodies on extracellular vesicles promotes tumour cell targeting. J Extracell Vesicles. (2016) 5:31053. doi: 10.3402/jev.v5.31053
187. Zhao C, Busch DJ, Vershel CP, Stachowiak JC. Multifunctional transmembrane protein ligands for cell-specific targeting of plasma membrane-derived vesicles. Small. (2016) 12:3837–48. doi: 10.1002/smll.201600493
188. Reshadmanesh A, Rahbarizadeh F, Ahmadvand D, Jafari Iri Sofla F. Evaluation of cellular and transcriptional targeting of breast cancer stem cells via anti-HER2 nanobody conjugated PAMAM dendrimers. Artif Cells Nanomed Biotechnol. (2018) 46:S105–15. doi: 10.1080/21691401.2018.1489269
189. Wu T, Liu J, Liu M, Liu S, Zhao S, Tian R, et al. A nanobody-conjugated DNA nanoplatform for targeted platinum-drug delivery. Angew Chemie Int Ed. (2019) 58:14224–8. doi: 10.1002/anie.201909345
190. Nuhn L, Bolli E, Massa S, Vandenberghe I, Movahedi K, Devreese B, et al. Targeting protumoral tumor-associated macrophages with nanobody-functionalized nanogels through strain promoted azide alkyne cycloaddition ligation. Bioconjug Chem. (2018) 29:2394–405. doi: 10.1021/acs.bioconjchem.8b00319
191. Ruoslahti E. Tumor penetrating peptides for improved drug delivery. Adv Drug Deliv Rev. (2017) 110–111:3–12. doi: 10.1016/j.addr.2016.03.008
192. Bian X, Wu P, Sha H, Qian H, Wang Q, Cheng L, et al. Anti-EGFR-iRGD recombinant protein conjugated silk fibroin nanoparticles for enhanced tumor targeting and antitumor efficiency. Onco Targets Ther. (2016) 9:3153–62. doi: 10.2147/OTT.S100678
193. Sha H, Zou Z, Xin K, Bian X, Cai X, Lu W, et al. Tumor-penetrating peptide fused EGFR single-domain antibody enhances cancer drug penetration into 3D multicellular spheroids and facilitates effective gastric cancer therapy. J Control Release. (2015) 200:188–200. doi: 10.1016/j.jconrel.2014.12.039
194. Zhu A, Sha H, Su S, Chen F, Wei J, Meng F, et al. Bispecific tumor-penetrating protein anti-EGFR-iRGD efficiently enhances the infiltration of lymphocytes in gastric cancer. Am J Cancer Res. (2018) 8:91–105.
195. Zhang Z, Qian H, Huang J, Sha H, Zhang H, Yu L, et al. Anti-EGFR-iRGD recombinant protein modified biomimetic nanoparticles loaded with gambogic acid to enhance targeting and antitumor ability in colorectal cancer treatment. Int J Nanomed. (2018) 13:4961–75. doi: 10.2147/IJN.S170148
196. van Lith SAM, van den Brand D, Wallbrecher R, van Duijnhoven SMJ, Brock R, Leenders WPJ. A conjugate of an anti-epidermal growth factor receptor (EGFR) VHH and a cell-penetrating peptide drives receptor internalization and blocks EGFR activation. ChemBioChem. (2017) 18:2390–4. doi: 10.1002/cbic.201700444
197. Van De Water JAJM, Bagci-Onder T, Agarwal AS, Wakimoto H, Roovers RC, Zhu Y, et al. Therapeutic stem cells expressing variants of EGFR-specific nanobodies have antitumor effects. Proc Natl Acad Sci USA. (2012) 109:16642–7. doi: 10.1073/pnas.1202832109
198. Larson SM, Carrasquillo JA, Cheung NKV, Press OW. Radioimmunotherapy of human tumours. Nat Rev Cancer. (2015) 15:347–60. doi: 10.1038/nrc3925
199. Pruszynski M, D'Huyvetter M, Bruchertseifer F, Morgenstern A, Lahoutte T. Evaluation of an anti-her2 nanobody labeled with 225 ac for targeted α-particle therapy of cancer. Mol Pharm. (2018) 15:1457–66. doi: 10.1021/acs.molpharmaceut.7b00985
200. Choi J, Vaidyanathan G, Koumarianou E, Kang CM, Zalutsky MR. Astatine-211 labeled anti-HER2 5F7 single domain antibody fragment conjugates: radiolabeling and preliminary evaluation. Nucl Med Biol. (2018) 56:10–20. doi: 10.1016/j.nucmedbio.2017.09.003
201. Fernandes SRG, Fernandes R, Sarmento B, Pereira PMR, Tomé JPC. Photoimmunoconjugates: novel synthetic strategies to target and treat cancer by photodynamic therapy. Org Biomol Chem. (2019) 17:2579–93. doi: 10.1039/C8OB02902D
202. Van de Broek B, Devoogdt N, D'Hollander A, Gijs HL, Jans K, Lagae L, et al. Specific cell targeting with nanobody conjugated branched gold nanoparticles for photothermal therapy. ACS Nano. (2011) 5:4319–28. doi: 10.1021/nn1023363
203. Van Driel PBAA, Boonstra MC, Slooter MD, Heukers R, Stammes MA, Snoeks TJA, et al. EGFR targeted nanobody-photosensitizer conjugates for photodynamic therapy in a pre-clinical model of head and neck cancer. J Control Release. (2016) 229:93–105. doi: 10.1016/j.jconrel.2016.03.014
204. Driehuis E, Spelier S, Beltrán Hernández I, de Bree RM, Willems S, Clevers H, et al. Patient-derived head and neck cancer organoids recapitulate egfr expression levels of respective tissues and are responsive to EGFR-targeted photodynamic therapy. J Clin Med. (2019) 8:1880. doi: 10.3390/jcm8111880
205. Heukers R, Mashayekhi V, Ramirez-Escudero M, de Haard H, Verrips T, van Bergen en Henegouwen P, et al. VHH-photosensitizer conjugates for targeted photodynamic therapy of met-overexpressing tumor cells. Antibodies. (2019) 8:26. doi: 10.3390/antib8020026
206. De Groof TWM, Mashayekhi V, Fan TS, Bergkamp ND, Toraño JS, Van Senten JR, et al. Nanobody-Targeted photodynamic therapy selectively kills viral GPCR-expressing glioblastoma cells. Mol Pharm. (2019) 16:3145–56. doi: 10.1021/acs.molpharmaceut.9b00360
207. Karges J, Jakubaszek M, Mari C, Zarschler K, Goud B, Stephan H, et al. Synthesis and characterization of an epidermal growth factor receptor-selective ruii polypyridyl–nanobody conjugate as a photosensitizer for photodynamic therapy. ChemBioChem. (2019) 21:531–42. doi: 10.1002/cbic.201900419
208. Noguchi T, Kato T, Wang L, Maeda Y, Ikeda H, Sato E, et al. Intracellular tumor-associated antigens represent effective targets for passive immunotherapy. Cancer Res. (2012) 72:1672–82. doi: 10.1158/0008-5472.CAN-11-3072
209. Togtema M, Hussack G, Dayer G, Teghtmeyer MR, Raphael S, Tanha J, et al. Single-domain antibodies represent novel alternatives to monoclonal antibodies as targeting agents against the human papillomavirus 16 E6 protein. Int J Mol Sci. (2019) 20:2088. doi: 10.1101/388884
210. Li S, Zhang W, Jiang K, Shan H, Shi M, Chen B, et al. Nanobody against the E7 oncoprotein of human papillomavirus 16. Mol Immunol. (2019) 109:12–9. doi: 10.1016/j.molimm.2019.02.022
211. Steels A, Verhelle A, Zwaenepoel O, Gettemans J. Intracellular displacement of p53 using transactivation domain (p53 TAD) specific nanobodies. MAbs. (2018) 10:1045–59. doi: 10.1080/19420862.2018.1502025
212. Steels A, Vannevel L, Zwaenepoel O, Gettemans J. Nb-induced stabilisation of p53 in HPV-infected cells. Sci Rep. (2019) 9:12680. doi: 10.1038/s41598-019-49061-9
213. Chen J, Guo H, Jiang H, Namusamba M, Wang C, Lan T, et al. A BAP31 intrabody induces gastric cancer cell death by inhibiting p27 kip1 proteasome degradation. Int J Cancer. (2019) 144:2051–62. doi: 10.1002/ijc.31930
214. Chowdhury S, Castro S, Coker C, Hinchliffe TE, Arpaia N, Danino T. Programmable bacteria induce durable tumor regression and systemic antitumor immunity. Nat Med. (2019) 25:1057–63. doi: 10.1038/s41591-019-0498-z
215. Gurbatri CR, Lia I, Vincent R, Coker C, Castro S, Treuting PM, et al. Engineered probiotics for local tumor delivery of checkpoint blockade nanobodies. Sci Transl Med. (2020) 12:eaax0876. doi: 10.1126/scitranslmed.aax0876
216. Ittig SJ, Schmutz C, Kasper CA, Amstutz M, Schmidt A, Sauteur L, et al. A bacterial type III secretion-based protein delivery tool for broad applications in cell biology. J Cell Biol. (2015) 211:913–31. doi: 10.1083/jcb.201502074
217. Blanco-Toribio A, Muyldermans S, Frankel G, Fernández LÁ. Direct injection of functional single-domain antibodies from E. coli into human cells. PLoS ONE. (2010) 5:e15227. doi: 10.1371/journal.pone.0015227
218. Lesniak WG, Chu C, Jablonska A, Behnam Azad B, Zwaenepoel O, Zawadzki M, et al. PET imaging of distinct brain uptake of a nanobody and similarly-sized PAMAM dendrimers after intra-arterial administration. Eur J Nucl Med Mol Imaging. (2019) 46:1940–51. doi: 10.1007/s00259-019-04347-y
219. Suzuki T, Yamaguchi H, Ogura J, Kobayashi M, Yamada T, Iseki K. Megalin contributes to kidney accumulation and nephrotoxicity of colistin. Antimicrob Agents Chemother. (2013) 57:6319–24. doi: 10.1128/AAC.00254-13
220. Klarenbeek A, El Mazouari K, Desmyter A, Blanchetot C, Hultberg A, de Jonge N, et al. Camelid Ig V genes reveal significant human homology not seen in therapeutic target genes, providing for a powerful therapeutic antibody platform. MAbs. (2015) 7:693–706. doi: 10.1080/19420862.2015.1046648
221. Conrath K, Vincke C, Stijlemans B, Schymkowitz J, Decanniere K, Wyns L, et al. Antigen binding and solubility effects upon the veneering of a camel VHH in framework-2 to mimic a VH. J Mol Biol. (2005) 350:112–25. doi: 10.1016/j.jmb.2005.04.050
222. Papadopoulos KP, Isaacs R, Bilic S, Kentsch K, Huet HA, Hofmann M, et al. Unexpected hepatotoxicity in a phase I study of TAS266, a novel tetravalent agonistic nanobody® targeting the DR5 receptor. Cancer Chemother Pharmacol. (2015) 75:887–95. doi: 10.1007/s00280-015-2712-0
Keywords: cancer, therapeutics, imaging, immunetherapy, nanobodies
Citation: Yang EY and Shah K (2020) Nanobodies: Next Generation of Cancer Diagnostics and Therapeutics. Front. Oncol. 10:1182. doi: 10.3389/fonc.2020.01182
Received: 02 March 2020; Accepted: 10 June 2020;
Published: 23 July 2020.
Edited by:
Wafik S. El-Deiry, Brown University, United StatesReviewed by:
Pierpaolo Correale, Azienda Ospedaliera Bianchi-Melacrino-Morelli, ItalyCopyright © 2020 Yang and Shah. This is an open-access article distributed under the terms of the Creative Commons Attribution License (CC BY). The use, distribution or reproduction in other forums is permitted, provided the original author(s) and the copyright owner(s) are credited and that the original publication in this journal is cited, in accordance with accepted academic practice. No use, distribution or reproduction is permitted which does not comply with these terms.
*Correspondence: Khalid Shah, a3NoYWhAYndoLmhhcnZhcmQuZWR1
Disclaimer: All claims expressed in this article are solely those of the authors and do not necessarily represent those of their affiliated organizations, or those of the publisher, the editors and the reviewers. Any product that may be evaluated in this article or claim that may be made by its manufacturer is not guaranteed or endorsed by the publisher.
Research integrity at Frontiers
Learn more about the work of our research integrity team to safeguard the quality of each article we publish.