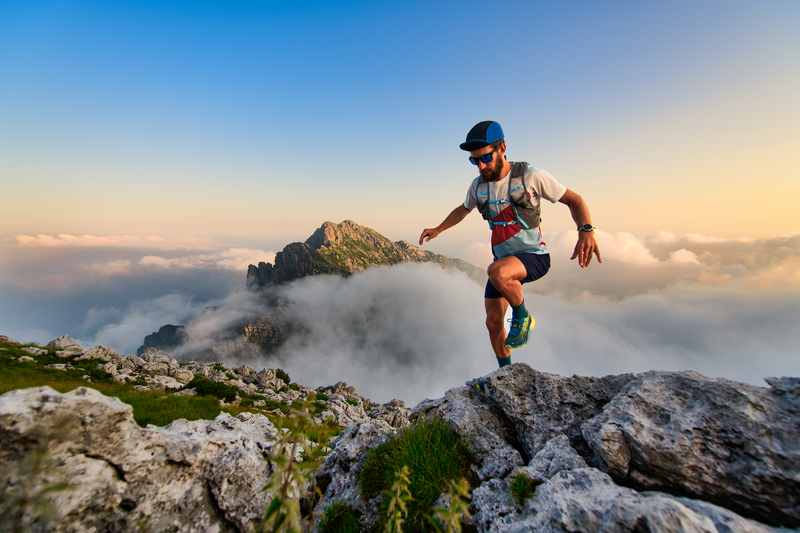
94% of researchers rate our articles as excellent or good
Learn more about the work of our research integrity team to safeguard the quality of each article we publish.
Find out more
REVIEW article
Front. Oncol. , 12 May 2020
Sec. Skin Cancer
Volume 10 - 2020 | https://doi.org/10.3389/fonc.2020.00597
Melanoma is the most aggressive malignant skin tumor and arises from melanocytes. The resistance of melanoma cells to various treatments results in rapid tumor growth and high mortality. As a local therapeutic modality, photodynamic therapy has been successfully applied for clinical treatment of skin diseases. Photodynamic therapy is a relatively new treatment method for various types of malignant tumors in humans and, compared to conventional treatment methods, has fewer side effects, and is more accurate and non-invasive. Although several in vivo and in vitro studies have shown encouraging results regarding the potential benefits of photodynamic therapy as an adjuvant treatment for melanoma, its clinical application remains limited owing to its relative inefficiency. This review article discusses the use of photodynamic therapy in melanoma treatment as well as the latest progress made in deciphering the mechanism of tolerance. Lastly, potential targets are identified that may improve photodynamic therapy against melanoma cells.
Melanoma is a highly aggressive malignant tumor that originates from melanocytes, and its progression is difficult to predict. Treatment of melanoma continues to face serious challenges, resulting in an increased annual global incidence of 3% (1). Melanoma typically occurs in the skin, but can also develop in other tissues that originate from pigmented neural crest (NC) cells, including the eyes, nasal cavity, anal canal, digestive tract, and genitourinary tract (2, 3). Although melanoma accounts for only 2% of all skin cancer cases, it is responsible for 80% of deaths from dermatologic cancers (4). Hence, the recent increase in morbidity and mortality due to melanoma is a matter of concern for global human health.
Current guideline-based therapies for patients with melanoma include surgery, radiotherapy, chemotherapy, immunotherapy, and targeted therapy (5). Although the treatment of patients with early stage melanoma is effective, the 5-year survival rate for advanced melanoma is only 16%, which is related to the low sensitivity to conventional treatment procedures (6). Photodynamic therapy (PDT) has been successfully used to treat patients with non-melanoma skin cancer (7), esophageal cancer (8), head and neck cancer (9), breast cancer (10), and lung cancer (11). In recent years, several in vitro and in vivo studies have been conducted to examine the efficacy of PDT for melanoma treatment; the results for which indicate that PDT may prove to be a promising adjuvant treatment for melanoma patients.
Although PDT has been successfully used in the treatment of cancer and non-neoplastic diseases, its use in the treatment of patients with melanoma has been limited owing to low response rates and unsatisfactory efficiency (12, 13). This article reviews the studies on PDT treatment of melanoma and other tumors and summarizes the effects (Figures 1, 2) as well as the potential mechanisms for tolerance (Figure 3) of PDT for the treatment of melanoma patients.
Figure 1. Effector mechanisms during photodynamic therapy of melanoma. The ground state photosensitizer (PS) is activated by irradiation with appropriate wavelength light to produce singlet state. Reactive oxygen species (ROS), the main cytotoxic components, can cause death of tumor cells by apoptosis (①) and induce the damage of the tumor vascular system (②). In addition, photodynamic therapy may also activate immune responses against tumors by affecting the secretion of inflammatory factor (IL-6, IL-1, and TNF-α), HSPs (heat shock proteins) and DAMPs (damage associated molecular patterns) (③), and exosomes (④). Moreover, exosomes induced by photodynamic therapy (PDT) might play an important role in inhibitory regulation of EMT (epithelial-mesenchymal transition) in melanoma cells (⑤).
Figure 2. Effector mechanisms leading to necrosis after photodynamic therapy of melanoma. PDT may induce DNA damage and swelling of organelles, leading to necrosis of melanoma cells. PDT may also activate the RIPK1 pathway to promote the phosphorylation of downstream RIPK3, make the phosphorylation of RIPK3 merge with MLKL, and form RIPK1-RIPK3-MLKL complex, namely necrotizing corpuscles.
Figure 3. Resistance mechanisms during photodynamic therapy of melanoma. Photosensitizers cannot be effectively excited by near-infrared (NIR) in PDT for melanoma, melanin granules and autophagy could be the main contributors to this resistance. First, visible light can be absorbed by melanin in melanoma cell (①), leading to diminishment of photothermal effect induced by PS and decrease in production of ROS and singlet oxygen, then resulting in the inhibition of immune response in tumor microenvironment (②) and apoptosis blocking (③) of melanoma cell. Only in the near-infrared conditions, PS can play an even greater role in PDT treatment of melanoma. Second, subcellular organelle damage induced by ROS in PDT treatment can enhance autophagy to maintain cell homeostasis against apoptosis, which ultimately leads to the resistance to PDT treatment in melanoma (④).
PDT is a novel non-invasive therapeutic technique for malignant tumors. The clinical results of PDT for cancer treatment show that it is efficacious in the treatment of early stage cancer that of head and neck tumors and basal cell carcinomas, for which complete remission may be achieved, which subsequently prolongs the survival time of patients with inoperable carcinoma (14, 15). The use of photosensitizers (PSs) can selectively target diseased tissues and improve the efficiency of photoinitiation. These PSs are activated by specific wavelength lasers and can trigger photochemical reactions that precisely target the tumor while reducing damage to the surrounding normal tissue. Therefore, PDT is considered to induce minimal toxicity to normal tissues and negligible systemic side effects, while significantly reducing long-term morbidity, offering positive cosmetic/esthetic outcomes, and protecting organ function (16, 17).
PDT combines photosensitizers, oxygen molecules, and light stimulation to treat tumors. Excited state singlet oxygen (1O2) serves as the primary cytotoxic material in PDT. Molecular oxygen in this state functions as a highly active reactive oxygen species (ROS) that oxidizes biological substrates (18, 19). The singlet oxygen or ROS produced within the cell membrane can cause photo-oxidative damage to proteins and lipids within the photosensitive binding site, and induce oxidative damage in the target cells, ultimately causing apoptosis, necrosis, and tumor vasculature damage. Furthermore, ROS can induce an inflammatory response to stimulate antitumor immune responses. These mechanisms, summarized in Figure 1, may lead to long-term tumor control through antitumor effects on primary/metastatic tumors (20, 21).
PDT plays an important role in cellular necrosis or apoptosis induced by light damage. The treatment method varies according to the PS aggregation site. Apoptosis is a form of programmed cell death, and apoptosis-induced tumor cell death has become one of the primary targets in tumor therapy. Alternatively, dysregulated cellular apoptosis may result in uncontrolled proliferation of melanoma cells (22). During treatment, the accumulation of PSs in the mitochondria and endoplasmic reticulum can cause oxidative stress-induced apoptosis (23). Therefore, PDT can induce apoptosis in melanoma cells, which might play a key role in inhibiting their proliferation and metastasis.
Studies have confirmed that PDT promotes apoptosis in the melanoma cell lines, A375 and UCT mel-1, by both the intrinsic and extrinsic apoptotic pathways (24, 25). PDT-induced apoptosis in melanoma cells occurs via three key signaling pathways. (1) In the intrinsic pathway, cellular death occurs through mitochondria-mediated signaling pathways involving DNA damage, p53 activation, p53-mediated inhibition of antiapoptotic proteins such as Bcl-2 (26–28), and kinases involved in cell proliferation and survival, such as B-Raf (29, 30). PSs can also induce damage in the mitochondria and lysosomes (31) by inhibiting production of MMP-9, Bcl-2, and Bcl-xL, while upregulating the expression of apoptotic related proteins Bax and PARP, and promoting activation of death receptor pathways. Moreover, PDT has been shown to induce intrinsic apoptosis by inducing increased levels of ER stress and activation of caspase cascade pathways (32). When PDT doses are increased beyond sublethal damage, stress response pathways become activated, including the ER stress pathway, with disruption of Ca2+ homeostasis and unfolding of protein. These responses lead to either apoptosis or autophagic cell death, dependent on the availability of Bax/Bak (33); (2) The extrinsic pathway is initiated by oligomeric death ligands, including tumor necrosis factor (TNF)-related apoptosis-inducing ligand (TRAIL) or CD95L (34); (3) Cytotoxic T lymphocytes (CTL) and natural killer (NK) cells release granzyme B, a serine protease, into the target cell to induce apoptosis (35). These cellular apoptosis pathways converge at a common terminal stage and rely on the disintegration of the cellular matrix by cysteine protease caspases. Specifically, caspase 9 is involved in the intrinsic pathway and caspase 8 in the extrinsic, both of which ultimately lead to activation of downstream caspase 3 in the execution pathway (36–38). Caspase−3,−8,−9 are released in PDT-treated melanoma cells (24, 25, 39), resulting in initiation of a series of cascades that induce irreversible apoptosis (40, 41).
PDT can also induce cellular necrosis upon accumulation of PSs in the cell membrane and lysosomes (see Figure 2). Necrosis is a caspase-8-independent cell death pathway that requires synergistic activation of receptor-interacting protein 1 (RIP1) and receptor-interacting protein 3 (RIP3) kinases (42). Unlike protein-driven cell apoptosis, necrotic apoptosis is mediated by a cascade of kinase signaling that activates (automatic) phosphorylation of RIPK1, RIPK3, and MLKL, which then form solubilized pores in the plasma membrane, leading to rapid plasma membrane rupture and inflammatory responses through the release of damage-associated molecular patterns and cytokines (43, 44). The necrosis pathway has been shown to be related to a variety of tumor types, including melanoma, pancreatic adenocarcinoma, and certain hematological malignancies (45). Mohammadalipour et al. demonstrated that a low dose of nitrogen-doped titanium dioxide (N-TiO2) nanoparticles (NPs) (1–100 μg/ml) stimulated autophagy flow response of non-toxic A375 cells. However, their light-activation can impede autophagosome-lysosome fusion and resulting in an increase at the basal ROS level. Therefore, PDT with N-TiO2 NPs leads to the blockade of autophagy flux and ultimately the occurrence of necroptosis in melanoma A375 cells (46). Additional factors that contribute to PDT-induced cellular necrosis include PS localization, exposure to light, and hypoxia-induced glucose deprivation. Furthermore, Thibaut et al. (47) demonstrated that PDT can induce necrosis in a murine melanoma B16-A45 cells signal pathway that involves activated caspase. Moreover, apoptosis and necrosis often use the same initiation signal pathway that involves activated caspase. Therefore, these are the major pathways that lead to melanoma cell death. Learning how to decrease apoptotic resistance of melanoma cells to improve PDT efficacy could be an important research goal for the future.
Tumor vasculature provides oxygen and nutrition to support the continuous growth of tumors and is a major pathway for metastasis (48). Therefore, antiangiogenesis treatment strategies are expected to have a strong therapeutic effect on various tumors. Antiangiogenic PDT can induce endothelial cell injury, vasoconstriction, release of coagulation factors, platelet aggregation, vascular rupture, vascular occlusion, blood flow stagnation, and hemorrhaging (49).
Lisnjak et al. (50) have demonstrated that PDT can significantly reduce the serum concentration of vascular endothelial growth factor (VEGF) as well as the metastatic transmission rate, while inducing changes to the vasculature of tumor tissues in lung carcinoma-bearing C57BL/6 mice. These results suggest that inhibition of tumor vasculature formation is an antitumor effect of PDT. In particular, the increased proliferation of vascular endothelial cells in the process of intravascular angiogenesis may lead to excessive accumulation of protoporphyrin-IX (PpIX), a potent photosensitizer, and selective enhancement of the photodynamic action on angiogenic endothelial cells in tumor tissues (51, 52). Schuitmaker et al. investigated the efficiency of the new PSs bacteriochlorin a (BCA) mediated PDT on Greene hamster melanoma implanted in the anterior eye. Following BCA-PDT, blood vessels and intracellular spaces were enlarged and clotting was immediately observed with swollen erythrocytes. Fused inner and outer membranes of mitochondria resulted in mitochondria damage that was confirmed by electron microscopy. With the passage of time, the degree of tissue and cell damage increased. At 24 h, nearly complete necrosis was observed at the treatment site. It was postulated that direct mitochondrial and vascular injury induced by BCA-PDT may account for the immediate cause of tumor necrosis (53). Similarly, Zilberstein et al. tested the effects of bacteriochlorophyll-serine (Bchl-Ser)-PDT in Nude CD1 mice bearing malignant M2R melanotic melanoma xenografts (76–212 mm3). Primary vascular damage with occlusive thrombi, hemorrhage, and tumor necrosis were confirmed by histopathology. Moreover, the treatment protocol was short and effective with a cure rate of over 80% (54).
Furthermore, studies have demonstrated that PDT induced vascular damage in other tumors, the mechanisms for which may translate to similar effects in melanoma. For example, ALA-PDT in a coculture of human umbilical vein endothelial cells (HUVECs) and human bladder carcinoma cell line had an antiangiogenic and antitumor effect, most notably when in combination with deferoxamine, which increased accumulation of PpIX (55). Karwicka demonstrated that treatment focused on vascular destruction (V-PDT) can lead to highly effective long-term antitumor responses mediated by strong blood supply deprivation in vivo. Further, 67% of Lewis lung carcinoma (LLC) bearing mice treated with V-PDT exhibited complete regression without relapse for over 1 year (56). Similarly, an in vivo study performed by Inoue et al. (55) showed that antiangiogenic PDT is effective for tumor tissues and does not significantly affect angiogenesis in normal tissue surrounding tumors in lung carcinoma-bearing C57BL/6 mice.
Although there is limited direct evidence demonstrating that PDT damages blood vessels in melanoma tumors, specifically, the results of the aforementioned studies do offer a theoretical basis for this hypothesis. Antiangiogenic PDT may function to disrupt or damage the tumor vasculature of melanoma; therefore, the combination of antiangiogenic PDT with radiochemotherapy may be clinically effective in relieving symptoms and improving the survival rate of patients with melanoma. The optimal approach to tumor and vascular targeting of PDT can disrupt melanoma and endothelial tumor cells and activate the immune response, thereby improving overall efficacy (16, 57).
Immune tolerance in the tumor microenvironment reduces the tumor killing capacity of immune cells and promotes tumor cell growth (58). Hence, reversion of immunosuppression in the tumor microenvironment is currently an exciting area in tumor immunotherapy research. Preclinical studies have demonstrated that PDT enhances host antitumor immune responses in lung cancer and non-melanoma skin cancer, at the treatment site by inducing oxidative stress, which can trigger the release of proinflammatory factors such as TNF, interleukin (IL)-6, IL-1, heat shock proteins (HSPs), complement proteins, and metabolites (17, 59). Innate immune cells including monocytes/macrophages, neutrophils, and dendritic cells may then be recruited to the treatment site by these inflammatory cytokines, which then function to kill tumor cells. Tumor vasculature also changes significantly upon PDT-induced inflammation. Adhesion molecules (intracellular adhesion molecules-1, vascular cell adhesion molecules-1, and selectins), which were found to be overexpressed following PDT, can recruit neutrophils and other inflammatory cells to tumor sites and convert the tumor vascular endothelium from a non-thrombotic, non-adhesive barrier between blood and tumor tissue to a pro-adhesive surface permitting infiltration of blood constituents (60). As a result of increased permeability, inflammatory cells have been shown to readily enter the vasculature after which the innate immune cells infiltrate the subcutaneous FsaR fibrosarcoma tumors in syngeneic C3H/HeN mice (61).
Acute inflammatory responses are associated with the development of adaptive antitumor immunity and thus, can protect the host organism in an antigen-specific manner. Previous studies have confirmed that PDT primarily activates dendritic cells (DCs) to enhance adaptive antitumor immunity (62, 63); and damage-associated molecular-pattern molecules (DAMPs)/cell death-associated molecular-pattern molecules (CDAMPs) that become released from dying tumor cells may be involved in this process. HSP70, a key member of the HSP complex, is released after PDT and binds to tumor cytoplasmic antigens in a stable concomitant complex (64). Thereafter, HSP-tumor antigen complexes bind to risk signal receptors and are recognized by Toll-like receptors 2 and 4 on the surface of the DCs (65). In turn, these induce activation of DCs and the release of proinflammatory cytokines.
Previous studies have demonstrated that the expression and secretion of high mobility group box 1 (HMGB1) in mouse colon cancer cells (66), cutaneous squamous cell carcinoma (SCC) cells (67), LLC cells (68), and cervical cancer cells (69) are significantly elevated following PDT. Extracellular HMGB1 can activate macrophages and DCs, and recruit neutrophils, using various receptors. Korbelik et al. (68) suggested that PDT-treated LLC cells release signals to induce production of HMGB1 by macrophages and other immune cells. These signals may then promote an antitumor immune response. Furthermore, a clinical study has suggested that the percentages of mature DCs increases in the blood of patients treated with 5-aminolevulinic acid-mediated PDT (ALA-PDT). Moreover, ALA-PDT significantly downregulated miR-34a and upregulated HMGB1 expression levels in cervical cancer tissues (69). PDT was also reported to induce a further increase in the number of regulatory T cells and NK cells and upregulate HMGB1 expression in the peripheral blood of patients with head and neck squamous cell carcinoma (HNSCC) (70). Cumulatively, these results suggest that PDT induces HMGB1 expression and is a crucial pathway for activating antitumor immunity.
Several proinflammation cytokines and DAMPS induced by PDT in the tumor microenvironment play a critical role in activating DCs. Mature DCs migrate to lymph nodes in large numbers and upregulate the expression of major histocompatibility complex (MHC)-I, MHC-II, and costimulatory molecules CD80 and CD86 (71). These changes enable DCs to express the antigen peptide-MHC complex on their cell surface and enhance activation of CD4+ T helper cells and CD8+ CTLs, thus triggering an adaptive immune response against tumor antigens (72).
Recent studies have shown that immune checkpoint inhibitors against programmed death 1 (PD-1) and programmed death ligand 1 (PD-L1) are well-established leading immunomodulatory agents that act in specific pathways involved in the adaptive immune suppression of tumor tissues. The focus of these studies was initially placed on targeting cancers that were considered to be immunogenic, including melanoma, renal, and lung cancers; however, subsequently the application was expanded to include other cancers such as Hodgkin lymphoma, urothelial, as well as head and neck cancer (73). Wang et al. (74) demonstrated a multifunctional acid-activated micro-micelle that enhances a PDT-driven tumor immune response by inhibiting the expression of PD-L1 in melanoma cells. This micelle not only enhances ROS-induced and PD-L1 knockout antitumor immune characteristics but also stimulates the immune response by promoting cytokine secretion and lymphocyte proliferation, and effectively inhibits B16-F10 melanoma tumor growth. Due to the immune response and immunologic memory induced by PDT, pulmonary metastasis of transplanted B16-F10 melanoma xenograft tumors was also inhibited in the in vivo study. These studies have comprehensively demonstrated that PDT-induced antitumor immunity plays an important role in the treatment of melanoma.
Additional studies have shown that PDT combined immunotherapy induces potent systemic antitumor immunity in mice and should be evaluated for the treatment of human cancer. Saji et al. showed that although PDT and (intratumorally injection of naïve dendritic cells) IT-DC were not effective on their own, PDT combined with IT-DC eradicated both CT26 and B16 tumors in a significant proportion of animals, and prolonged the survival of mice with tumors that were not cured. Most importantly, PDT combined with IT-DC treatment at a single tumor site resulted in tumor regression at distant sites, including multiple lung metastases (75).
In situ photoimmunotherapy (ISPI), which combines photodynamic therapy with immunological stimulation induction, is a promising modality for the treatment of metastatic melanoma (76). A continued local application of topical imiquimod (a Toll-like receptor 7 agonist) in combination with indocyanine green-PDT has been used to treat late stage melanoma patients. In one study, 11 patients received ISPI in one or multiple 6-week treatment cycles applied to a 200-cm2 site, which often contained multiple cutaneous metastases. The treatment included local application of topical imiquimod, injection of indocyanine green (ICG), and a 805 nm laser for local irradiation. All patients completed at least one treatment cycle. The result shows that complete response was observed in six patients, five patients were alive at the time of last follow-up and the probability of 12-month overall survival was 70% (77). Therefore, ISPI not only produces a complete local response but also demonstrates an effective immune response against metastatic nodules. Furthermore, injecting dendritic cells (DCs) into a tumor can stimulate an immune response, which, combined with local PDT, induces a striking antitumor effect with potent systemic antitumor immunity. In fact, PDT + IT-DC eradicated both CT26 and B16 tumors in a significant proportion of animals and prolonged the survival of mice in which the tumors were not cured (75). PDT creates a favorable microenvironment for the acquisition of tumor antigen and the activation of DC, which reduces the need for tumor antigen loading in vitro by DCs (78). These studies show that these combined treatments can sometimes induce strong and durable tumor specific immunity that results in destruction of targeted tumors as well as initiation of systemic antitumor immune response.
Therefore, PDT combined with immunostimulatory agents seems to show great promise and could change the current therapeutic strategy for melanoma treatment. However, to improve its efficacy, further investigations on the precise antitumor immune mechanism elicited by PDT in melanoma treatment must be completed.
Exosomes are a type of extracellular particle that can mediate the communication between cells. They contain cellular components such as microRNAs, mRNAs, proteins, and DNA. Exosomes have been a subject of recent investigation to study the mechanisms of tumorigenesis and tumor progression, including tumor metastasis, angiogenesis, antitumor immunity, and tumor immunological escape (79–81). Two studies have demonstrated that PDT can regulate exosome secretion by tumor cells. ALA-PDT induced the production of exosomes with high levels of HMGB1, which in turn promoted DC maturation in the peripheral blood of ALA-PDT-treated patients with cervical cancer (69). Moreover, exosomes induced by PDT treatment were involved in the regulation of epithelial-mesenchymal transition (EMT) of tumor cells. Analysis of the exosomes obtained from the plasma of nine HNSCC patients (three in stage pT1, one in stage pT3, and five in stage pT4), all of whom benefited from positive clinical outcomes following treatment, on day 7 or 4–6 weeks after PDT treatment confirmed that the PDT-mediated secretion of exosomes contained E-cadherin, and restored epithelial morphology and epithelial cell adhesion molecule (EpCAM) expression in tumor cells. Further, the exosomes of these patients exhibited downregulated expression of mesenchymal genes and inhibited proliferation, migration, and invasion of the recipient tumor cells in vitro (82). These studies suggest that PDT can potentially induce antitumor immune responses and inhibit tumor migration by regulating exosome secretion. However, the key components of exosomes involved in PDT-mediated tumor suppression are currently unknown, which may be a hot topic of future studies.
Melanin can absorb ultraviolet (UV) and visible light, which can prevent ultraviolet radiation (UVR) to protect the skin (83). The melanin pigment is synthesized in melanocytes by tyrosinase that is integrated into an organelle named melanosome (84, 85). After synthesis, melanin is transported into the surrounding keratinocytes of the epidermis (86).
Tyrosinase (TYR) is a key enzyme that produces melanin from melanocytes. Tyrosinase-related proteins (TRP-1, TRP-2) convert tyrosine to dopamine and dopamine to dopamine quinone in a two-step enzymatic reaction catalyzed by tyrosine hydroxylase and dopamine oxidase, respectively. The resulting quinone is used to synthesize pheomelanin and eumelanin (87). Melanogenesis can be induced by a variety of paracrine cytokines, including α-melanocyte-stimulating hormone (α-MSH), endothelin 1 (ET-1), nitric oxide, adreno-cortico-tropic-hormone (ACTH), prostaglandins, thymidine dinucleotides, and histamines, upon exposure to ultraviolet B (UVB) (88). These factors activate pigment-related proteins such as microphthalmia-associated transcription factor (MITF), tyrosinase (TYR), tyrosinase-related protein-1 (TRP-1), and tyrosinase-related protein-2 (TRP-2) (89). MITF, in particular, plays a key role in melanogenesis by regulating melanocyte differentiation, pigmentation, proliferation, and survival. In the absence of radiation, melanocytes are exposed to eumelanin that causes DNA damage by inducing DNA strand breakage. In addition, melanin may damage DNA through a Fenton reaction (90), and can prevent the access of DNA repair enzymes to the DNA damaged sites. Furthermore, DNA damage can cause melanocytic mutation and increase melanin production (91). Moderate to high levels of pigmentation have been observed in melanoma tumors, and the melanin content in cells may be directly proportional to the degree of cell differentiation and inversely proportional to cell growth (92).
The singlet oxygen produced by PDT can reduce natural oxidation of melanin and DNA damage caused by melanin (93). PDT has been suggested to reduce the melanin content and tyrosinase activity in melanocytes, but not to affect cell survival (94). Studies have shown that melanin can scavenge ROS, such as singlet oxygen, hydroxyl radicals, and superoxide anions (95). These studies indicate that melanin protects pigmented cells from oxidative stress, changes cell metabolism, induces immune suppression and mutagenesis of tumor microenvironment, thus protecting malignant melanocytes from various treatments. Currently, it is believed that the inhibition of melanogenesis by immunotherapy, radiotherapy, chemotherapy, and PDT can reduce the incidence of melanoma deterioration (96).
In order to avoid an adverse reduction in PDT efficacy due to the absorption of light by melanin, combination with depigmentation may be necessary. In vivo studies demonstrated that combining hypericin-mediated PDT with depigmentation agents, such as tyrosinase inhibitors (kojic acid) or phenyl thiourea, significantly increases ROS production and decreases viability of MEL-1 cells to a similar extent as that of A375 cells, suggesting that this treatment increases susceptibility of melanoma cells (97, 98). Further, melanoma cells were treated by photobleaching in combination with PDT and 420-nm violet light (99), and the results showed that the bleaching effect of violet light on melanoma cells significantly increased their sensitivity to PDT. Therefore, a drug with the ability to inhibit melanin production or induce depigmentation would be an important component in the therapeutic arsenal to treat melanoma more effectively.
Autophagy is a basic physiological process that relies on lysosomal pathways to degrade cytoplasmic proteins and organelles to maintain cellular homeostasis. Interestingly, autophagy elicits opposing effects depending on the needs of the cells. For instance, it can serve as a type II programmed cell death pathway if necessary, while eliciting cytoprotective effects in other instances. In the advanced stage of melanoma and many other types of tumors, autophagy serves as a resistance mechanism and occurs as a tumor cell pro-survival mechanism (100, 101). Several studies have shown that chemotherapy, radiotherapy, immunotherapy, and PDT can upregulate the expression of autophagy-related proteins such as ATG4, ATG5, ATG12, and Beclin-1, thereby increasing tumor resistance (102–106). Marino et al. demonstrated that melanoma cells can survive in an acidic environment by upregulating autophagy; meanwhile, inhibition of ATG5 can reduce survival of melanoma cells (107). Similarly, Mehnert et al. established a mouse melanoma model by deleting ATG7 and PTEN gene in melanoma cells (108), which significantly inhibited tumor growth and prolonged the survival of mice. In this model, dabrafenib combined with ATG7 antagonistic therapy significantly inhibited the growth of melanoma (109). Martin et al. (110) also demonstrated that the combination of chemotherapy with autophagy and mitogen extracellular signal-regulated kinase (MEK) inhibition can enhance the melanoma cell killing effect of chemotherapeutic drugs. Based on these studies, the induction of autophagy may serve as a resistance mechanism to PDT for melanoma treatment.
After PDT treatment of tumor cells, autophagy is activated through the cell-related pressure sensor, and the intracellular components or organelles are transported to lysosomes for decomposition and reuse to offset the alarms of their environment and to respond to the cytotoxic effect. Tumor cells need to control and adapt to the redox imbalance caused by ROS produced following PDT. The redox homeostasis is closely related to the occurrence, progression, and metastasis of tumors (111). PDT-induced autophagy provides a protective mechanism for breast cancer cells, osteosarcoma cells, HeLa cells, and colon and rectum cancer stem cells. Inhibition of autophagy can enhance the photodynamic tumor cell killing effect (112–114). On the other hand, PDT-induced autophagy can also lead to the dissociation of Bcl-2 and Beclin-1, reduce Bax/Bak protein levels, and inhibit caspase-8 through induction of apoptosis. However, these results suggest that autophagy-induced cell death can only occur in tumor cells with defective apoptosis (115).
In addition to directly destroying cancer cells, PDT seems to influence other indirect killing mechanisms by, for instance, regulating innate antitumor immune activity and damaging tumor vasculature (116). Autophagy is fundamental for cell survival and for the proper function of immune cells and endothelial cells; however, its role in determining melanoma resistance to PDT is still unknown (117–119). Therefore, further studies on understanding the molecular mechanism of PDT-induced autophagy in melanoma resistance will be of significance to improve the development of future combinatorial strategies.
PSs accumulated intracellularly are the first step in PDT. Given the role of singlet oxygen and other ROS in tissue damage inflicted by PDT, the most commonly used PSs are dependent on the molecular oxygen tissue concentrations. Using PSs in PDT allows for selective tumor targeting due to the intracellular metabolism, and are, therefore, important for effective PDT treatment of malignant tumors. However, melanoma patients do not seem to have benefited significantly from PDT using the PSs and treatment protocols available today. There are two possible reasons that might account for this. First, the high melanin content in melanoma cells absorbs visible spectral radiation, especially in the blue region, which reduces the light available for absorption by the PS and reduces PDT efficacy (120). PSs with absorption in the near-infrared (NIR) range may be more suitable for PDT of melanoma cells. Second, tumor targeting and PS accumulation may not be fully effective with current PSs. Therefore, in this article, we will now identify and describe PSs potentially available for melanoma treatment.
Generally, PSs for PDT are divided into three generations, some of which have been investigated for melanoma treatment (Table 1). Porfimer sodium, the first-generation PS, mediated PDT on melanotic and amelanotic malignant melanoma in athymic nude mice and was effective against amelanotic but not melanotic melanoma (121–124). There are many types of second generation PSs, such as porphyrin derivatives (PD), phthalocyanines, biomimetic dyes, and polycyclic quinone. 5-ALA is one of the PD used for non-melanoma clinical treatment. Many in vitro studies revealed that 5-ALA-PDT effectively inhibits the activity of melanoma cell lines and triggers cellular apoptosis (24, 31, 125–129). However, when 5-ALA was used for cutaneous melanoma treatment in vivo, it did not significantly inhibit tumor progression (24). The effect of 5-ALA seems to be related to the dose. Our unpublished data showed that only high doses of 5-ALA (10 mM) significantly inhibited autophagy in melanoma cells. As reviewed in Table 1, methylene blue (MB) is a cationic dye derived from the phenothiazine family. It exhibits strong broad-spectrum red light absorption (550–700 nm, maximum absorption at 664 nm) and high affinity for melanocytes, which helps selective absorbance of this PS in cutaneous melanoma. MB-PDT and irradiation with a 664-nm light hindered tumor growth and prolonged survival in a B16F10 pigmented mouse melanoma model (163, 164).
The third generation PSs have demonstrated improved tumor treatment characteristics, such as higher biocompatibility, stronger tumor targeting capacity, higher ROS generation rate, and longer wavelength absorption, compared to the first- and second generation PSs. This was achieved by incorporating the PS into a nanocarrier and by using peptides or antibodies for selective delivery of PSs to tumors. The second generation PSs combined with carrier can circumvent the skin barrier and improve selective delivery to melanoma cells (120, 146, 149, 150, 165). The use of nanocarriers can improve the activity of photosensitizing agents through preferential accumulation of the carrier at the tumor site and facilitating slow, controlled release of the PS (166). Nanocarriers function by binding to the target molecules of overexpressed receptors in tumor cells, leading to enhanced uptake of PS that have been conjugated with peptides, aptamers, and antibody fragments. Additionally, PS-coated upconversion nanoparticles were found to trigger ROS production under 980 nm NIR excitation and showed great promise for PDT (167). Rationally designed DNA nanosponges were reported to load and deliver PSs effectively, target tumors precisely, and effectively relieve hypoxia-associated resistance to enhance the efficacy of PDT (168). In general, the application of nanotechnology in PDT aims to improve water hydrophobic drug compatibility/PS, protect against drug degradation, produce a sustained release of drugs, improve drug bioavailability (169), increase tumor selectivity, and allow improved treatment of deep tumor infiltration depth, so as to increase therapeutic efficacy and reduce adverse side effects (170–172).
It has been found that micelles, liposomes, and metal oxide enable passive targeting of tumors through enhanced permeability and retention (EPR) effects to improve the efficacy of PDT (173, 174). Many of these PS delivery systems have been used in melanoma treatment (175, 176). For example, nitrogen-doped titanium dioxide, polyethylene glycol-polyaspartate-modified rose bengal-loaded magnetic mesoporous silica (RB-MMSNs), Titanium-dioxide-nanoparticle-gold-nanocluster-graphene (TAG), doxorubicin, which is an anticancer agent (DOX/PheoA-ALG NPs), and POP micelles have all been shown to increase PDT efficacy against B16F10 melanoma in vivo and to stimulate and enhance immunological responses (152–155).
NIR light absorption PSs also improve the photodynamic effect. P-nitrophenyl-pD-glucopyranoside (PNPG), a new class of PS, exhibits large absorption peak in the NIR spectrum. The encouraging results have revealed that PNPG can effectively target CD44-overexpressing cancer cells and selectively kill B16 cells when exposed to NIR light (808 nm) after modified with hyaluronic acid (HA) and polyethylene glycol diamine (PEG) (158). The ICG-mediated PDT with a broader irradiation range (600–1,600 nm) was studied previously. The authors found that NIR radiation was most effective in inducing B16F10 cell apoptosis and G0/G1 cell cycle arrest in vitro (156, 157). In addition, Tookad1 (177), naphthalocyanines (160), PcNP@Drug (161), and Platinum(II) Ring-Fused Chlorin (162) were reported to kill melanoma cells and suppress malignant melanoma tumor growth upon exposure to NIR light (700–1,000 nm) in a pigmented melanoma model. Therefore, third generation PSs for melanoma treatment are currently in the spotlight of the PDT research field.
Branching out from in-depth research on PDT, new treatments have emerged such as sonodynamic therapy (SDT) or photothermal therapy (PTT), which have advantages similar to PDT, including tumor selectivity, minimal invasiveness, and ability to enhanced PS activation without the need for direct access to the tumor site.
SDT represents an emerging approach that offers the possibility of non-invasively eradicating solid tumors in a site directed manner. It involves the sensitization of target tissues with a non-toxic sensitizing chemical agent and subsequent exposure of the sensitized tissues to relatively low intensity ultrasound. Because ultrasound has stronger tissue penetration ability, this method has advantages over similar alternative methods (such as PDT), thus showing more concentrated therapeutic effect on the lesions. Many experiments have confirmed that SDT has obvious killing effect on tumor cells at home and abroad. Jin et al. (178) compared the efficacy of ALA-PDT and SDT in a squamous cell carcinoma (A431) cell line as well as the ability of these treatments to reduce the size of A431 ectopic tumors in mice. Similarly, the relative efficacy of Rose Bengal-PDT and SDT was investigated in a B16-melanoma cell line and in a B16 ectopic tumor model. The results tested no statistically significant difference in efficacy between ALA -PDT or SDT in the non-melanoma model; however, Rose Bengal-SDT was significantly more efficacious than PDT in the melanoma. This difference in efficacy was due to the pigmentation of the melanoma cells that effectively filtered the excitation light preventing it from activating the sensitizer, while the use of ultrasound avoids this problem (179). Harada et al. also demonstrated the induction of the melanoma cell (C32) apoptosis by the combination of TiO2 nanoparticles and ultrasound (US). Meanwhile, in vivo results showed significant inhibition of C32 solid tumors in mice growth in groups treated with TiO2 and US (TiO2-SDT) (180). ALA-SDT showed synergistic antitumor effects in malignant melanoma by constituting a positive feedback loop of p53-miR-34a-Sirt1 axis (181). In addition, there are several new sonosensitizer-mediated sonodynamic therapies that result in complete regression of melanoma, such as chloroaluminum phthalocyanine disulfonate (ClAlPcS2), a nickel ferrite/carbon nanocomposite (NiFeO/C), redox/enzyme/ultrasound responsive chondroitin sulfate-chlorin e6-lipoic acid nanoplatform loading docetaxel (136, 182, 183). These studies suggest that SDT may be more effective than PDT in treating hyperpigmented melanoma.
PTT is an emerging approach for tumor treatment. Under NIR illumination, the photothermal conversion materials can convert light energy into heat energy to kill tumor cells. The damaged tumor cells can evoke efficient antitumor immune response and promote the necrosis and apoptosis of tumor cells. PTT provides a precise and minimally invasive alternative for cancer treatment. It is effective in controlling metastatic cancer. Xu et al. (184) developed the GNS-TAT-Cy5 nanoprobe which can serve as a precise theranostic platform via regulating the photothermal dose and achieved regulation and detection of apoptosis related to caspase-3 for melanoma. Zhang et al. (185) demonstrated the potential application of a piTRL-mediated immuno-photothermal therapy against melanoma and its metastases in a study in vivo. Alvi et al. (186) described a convenient method to synthesize a new type of superparamagnetic up conversion nanoprobes, which possesses high biocompatibility and can be used in imaging-guided photothermal therapy for the treatment of malignant melanoma. PTT has advantages similar to PDT, such as high specificity, minimal invasiveness, and precise spatial-temporal selectivity. PTT penetrates deeper into the tissue, making it more effective in treating melanoma.
Although the question of whether PDT can be used in the clinical treatment of melanoma still remains unanswered, some reports on clinical application of PDT for melanoma treatment are available. For instance, Barbazetto et al. reported results of PDT in four patients with choroidal melanoma. Results showed PDT led to tumor regression in two patients (one tumor decreased in size and remained stable for 18 months; another tumor exhibited no growth for 11 months), melanomas in the other patients continued to grow, eventually requiring surgical intervention (187). Interestingly, another study used the same protocol on a patient with choroidal amelanotic melanoma and reported complete resolution of the lesion, leaving a flat, atrophic chorioretinal scar. Thirteen months after her last treatment, she remained asymptomatic with no signs of recurrence (188).
Alternatively, Campbell et al. tested the effectiveness of PDT with verteporfin on nine patients with posteriorly located amelanotic choroidal melanomas, one of which contained a pigmented portion. The basal diameters of the tumors ranged from 4 to 16 mm and heights ranged from 1.3 to 5.7 mm. Treatment was repeated until the melanoma adopted a flat appearance or its height reached a stable end point. Combination therapy resulted in complete tumor regression with no recurrence in eight patients during a follow-up period between 34 and 81 months. However, one case in this study presented with two local recurrences, one at 21 months and the other at 34 months (189). Similarly, O'Day et al. reported that initial tumor regression was achieved in 36 of 41 (88%) patients with choroidal amelanotic melanoma (no distant metastasis) following an initial course of PDT. However, recurrent disease occurred in 44% of these cases with a mean follow-up of 3.5 years. Moreover, primary treatment failure occurred in 12% of patients (190). Turkoglu et al. also examined the effects of PDT on 12 patients with eye melanoma; 10 of whom demonstrating amelanotic and two presented with a lightly pigmented appearance. Results showed complete tumor regression of small amelanotic choroidal melanoma in 67% of patients at a mean of 5 years (191). Similarly, Fabian et al. (192) reported PDT to be a safe and efficient treatment modality for small pigmented posterior pole choroidal melanoma, achieving short-term tumor control in 80% of patients by 6-month follow-up after three PDT sessions. Cumulatively, these studies suggest that PDT may be an effective therapy strategy for choroid melanoma, with no major effect on visual acuity. Alternatively, Sheleg demonstrated the effectiveness of Ce6-PDT on skin metastases of pigmented melanoma (193). Above all, PDT may induce tumor regression in a significant proportion of melanoma.
Although PDT has proven to be relatively safe for use in clinical treatment, minor side effects have been reported, the most common of which is local swelling, pain, and a burning sensation (194, 195). PDT may also elicit side effects such as skin rash, as was reported in two patients with early gastric cancer (196). Furthermore, PDT treatment of esophageal cancer may cause mild esophageal stenosis (197). Nevertheless, an increasing number of studies suggest that PDT appears to be a non-invasive, relatively simple method that can be performed on an outpatient basis. It has also demonstrated reproducible results in basal-cell carcinoma, cervical intraepithelial neoplasia, and cervical human papilloma virus (HPV) infection cases, while causing minimal side effects (15, 198, 199).
Despite the efficacy of surgical treatment for early stage melanoma, appropriate diagnosis of this condition is often difficult, which causes delay in treatment. Melanoma is often not diagnosed until intermediate or late stages, which translates to poor prognosis, recurrence, and low survival rate. Therefore, the discovery of new adjuvant treatments is an important and valuable subject in the field of melanoma research.
PDT is a promising therapeutic strategy for tumor treatment. Significant breakthroughs in basic research have indicated that PDT can provide substantial benefits in the treatment of advanced stage melanoma. Overall, this review has summarized efficacy and resistance mechanisms of melanoma during PDT treatment, and described new adjuvant therapeutic approaches. The synthesis of new PSs that absorb NIR light may improve the efficacy of PDT treatment. Moreover, PDT combined with autophagy inhibitors, immunotherapy, or melanogenesis inhibitors might be a better treatment to overcome melanoma resistance and achieve better therapeutic effects. However, for optimal safety and efficacy, it will be very important to understand the molecular mechanisms of these combination therapies. For example, tumor-derived exosomes induced by PDT might be a double-edged sword for melanoma treatment. At present, what we can definitely say is that further research on ways to exploit PDT for melanoma treatment should continue to be an important focus of future research.
Y-GL and HZ contributed substantially to the overall concept of this review, revised the article, and they gave the approval of the final version for publishing. X-YL and L-CT wrote the manuscript. L-WD and XL prepared the figures. W-QZ and X-XS prepared the table.
This research was supported by the Natural Science Foundation of China (81672694) and Medical innovation of graduate students in Chongqing (CYS19368).
The authors declare that the research was conducted in the absence of any commercial or financial relationships that could be construed as a potential conflict of interest.
1. Tripp MK, Watson M, Balk SJ, Swetter SM, Gershenwald JE. State of the science on prevention and screening to reduce melanoma incidence and mortality: the time is now. CA Cancer J Clin. (2016) 66:460–80. doi: 10.3322/caac.21352
2. Larribere L, Utikal J. Stem cell-derived models of neural crest are essential to understand melanoma progression and therapy resistance. Front Mol Neurosci. (2019) 12:111. doi: 10.3389/fnmol.2019.00111
3. Motofei IG. Melanoma and autoimmunity: spontaneous regressions as a possible model for new therapeutic approaches. Melanoma Res. (2019) 29:231–6. doi: 10.1097/CMR.0000000000000573
4. US Preventive Services Task Force, Grossman DC, Curry SJ, Owens DK, Barry MJ, Caughey AB, et al. Behavioral counseling to prevent skin cancer: US preventive services task force recommendation statement. JAMA. (2018) 319:1134–42. doi: 10.1001/jama.2018.1623
5. US Preventive Services Task Force, Bibbins-Domingo K, Grossman DC, Curry SJ, Davidson KW, Ebell M, et al. Screening for skin cancer: US preventive services task force recommendation statement. JAMA. (2016) 316:429–35. doi: 10.1001/jama.2016.8465
6. Chen DJ, Li XS, Zhao H, Fu Y, Kang HR, Yao FF, et al. Dinitrophenyl hapten with laser immunotherapy for advanced malignant melanoma: a clinical study. Oncol Lett. (2017) 13:1425–31. doi: 10.3892/ol.2016.5530
7. Lu YG, Wang YY, Yang YD, Zhang XC, Gao Y, Yang Y, et al. Efficacy of topical ALA-PDT combined with excision in the treatment of skin malignant tumor. Photodiagnosis Photodyn Ther. (2014) 11:122–6. doi: 10.1016/j.pdpdt.2014.02.006
8. Yano T, Muto M, Minashi K, Iwasaki J, Kojima T, Fuse N, et al. Photodynamic therapy as salvage treatment for local failure after chemoradiotherapy in patients with esophageal squamous cell carcinoma: a phase II study. Int J Cancer. (2012) 131:1228–34. doi: 10.1002/ijc.27320
9. Lou PJ, Jager HR, Jones L, Theodossy T, Bown SG, Hopper C. Interstitial photodynamic therapy as salvage treatment for recurrent head and neck cancer. Br J Cancer. (2004) 91:441–6. doi: 10.1038/sj.bjc.6601993
10. Isola V, Pece A, Pierro L. Photodynamic therapy with verteporfin of choroidal malignancy from breast cancer. Am J Ophthalmol. (2006) 142:885–7. doi: 10.1016/j.ajo.2006.06.008
11. Simone CB II, Cengel KA. Photodynamic therapy for lung cancer and malignant pleural mesothelioma. Semin Oncol. (2014) 41:820–30. doi: 10.1053/j.seminoncol.2014.09.017
12. Brown SB, Brown EA, Walker I. The present and future role of photodynamic therapy in cancer treatment. Lancet Oncol. (2004) 5:497–508. doi: 10.1016/S1470-2045(04)01529-3
13. Dummer R, Hauschild A, Pentheroudakis G. Cutaneous malignant melanoma: ESMO clinical recommendations for diagnosis, treatment and follow-up. Ann Oncol. (2009) 20(Suppl. 4):129–31. doi: 10.1093/annonc/mdp152
14. Ahn PH, Finlay JC, Gallagher-Colombo SM, Quon H, O'Malley BW Jr, Weinstein GS, et al. Lesion oxygenation associates with clinical outcomes in premalignant and early stage head and neck tumors treated on a phase 1 trial of photodynamic therapy. Photodiagnosis Photodyn Ther. (2018) 21:28–35. doi: 10.1016/j.pdpdt.2017.10.015
15. Li X, Tan L, Kou H, Zhang J, Wang Y, Li G, et al. Ocular preservation through limited tumor excision combined with ALA-PDT in patients with periocular basal cell carcinoma. Photodiagnosis Photodyn Ther. (2019) 27:291–4. doi: 10.1016/j.pdpdt.2019.06.016
16. Baldea I, Filip AG. Photodynamic therapy in melanoma–an update. J Physiol Pharmacol. (2012) 63:109–18.
17. Castano AP, Mroz P, Hamblin MR. Photodynamic therapy and anti-tumour immunity. Nat Rev Cancer. (2006) 6:535–45. doi: 10.1038/nrc1894
18. Kim MM, Ghogare AA, Greer A, Zhu TC. On the in vivo photochemical rate parameters for PDT reactive oxygen species modeling. Phys Med Biol. (2017) 62:R1–48. doi: 10.1088/1361-6560/62/5/R1
19. Lim ME, Lee YL, Zhang Y, Chu JJ. Photodynamic inactivation of viruses using upconversion nanoparticles. Biomaterials. (2012) 33:1912–20. doi: 10.1016/j.biomaterials.2011.11.033
20. Biteghe FN, Davids LM. A combination of photodynamic therapy and chemotherapy displays a differential cytotoxic effect on human metastatic melanoma cells. J Photochem Photobiol B. (2017) 166:18–27. doi: 10.1016/j.jphotobiol.2016.11.004
21. Anzengruber F, Avci P, de Freitas LF, Hamblin MR. T-cell mediated anti-tumor immunity after photodynamic therapy: why does it not always work and how can we improve it? Photochem Photobiol Sci. (2015) 14:1492–509. doi: 10.1039/c4pp00455h
22. de Unamuno B, Palanca S, Botella R. Update on melanoma epigenetics. Curr Opin Oncol. (2015) 27:420–6. doi: 10.1097/CCO.0000000000000217
23. Reiners JJ Jr, Agostinis P, Berg K, Oleinick NL, Kessel D. Assessing autophagy in the context of photodynamic therapy. Autophagy. (2010) 6:7–18. doi: 10.4161/auto.6.1.10220
24. Cai J, Zheng Q, Huang H, Li B. 5-aminolevulinic acid mediated photodynamic therapy inhibits survival activity and promotes apoptosis of A375 and A431 cells. Photodiagnosis Photodyn Ther. (2018) 21:257–62. doi: 10.1016/j.pdpdt.2018.01.004
25. Kleemann B, Loos B, Scriba TJ, Lang D, Davids LM. St. John's wort (Hypericum perforatum L.) photomedicine: hypericin-photodynamic therapy induces metastatic melanoma cell death. PLoS ONE. (2014) 9:e103762. doi: 10.1371/journal.pone.0103762
26. Roos WP, Kaina B. DNA damage-induced cell death: from specific DNA lesions to the DNA damage response and apoptosis. Cancer Lett. (2013) 332:237–48. doi: 10.1016/j.canlet.2012.01.007
27. Naumann SC, Roos WP, Jost E, Belohlavek C, Lennerz V, Schmidt CW, et al. Temozolomide- and fotemustine-induced apoptosis in human malignant melanoma cells: response related to MGMT, MMR, DSBs, and p53. Br J Cancer. (2009) 100:322–33. doi: 10.1038/sj.bjc.6604856
28. Beck D, Niessner H, Smalley KS, Flaherty K, Paraiso KH, Busch C, et al. Vemurafenib potently induces endoplasmic reticulum stress-mediated apoptosis in BRAFV600E melanoma cells. Sci Signal. (2013) 6:ra7. doi: 10.1126/scisignal.2003057
29. Shao Y, Aplin AE. BH3-only protein silencing contributes to acquired resistance to PLX4720 in human melanoma. Cell Death Diff. (2012) 19:2029–39. doi: 10.1038/cdd.2012.94
30. Selimovic D, Porzig BB, El-Khattouti A, Badura HE, Ahmad M, Ghanjati F, et al. Bortezomib/proteasome inhibitor triggers both apoptosis and autophagy-dependent pathways in melanoma cells. Cell Signal. (2013) 25:308–18. doi: 10.1016/j.cellsig.2012.10.004
31. Tsai T, Ji HT, Chiang PC, Chou RH, Chang WS, Chen CT. ALA-PDT results in phenotypic changes and decreased cellular invasion in surviving cancer cells. Lasers Surg Med. (2009) 41:305–15. doi: 10.1002/lsm.20761
32. Kong F, Zou H, Liu X, He J, Zheng Y, Xiong L, et al. miR-7112-3p targets PERK to regulate the endoplasmic reticulum stress pathway and apoptosis induced by photodynamic therapy in colorectal cancer CX-1 cells. Photodiagnosis Photodyn Ther. (2020) 29:101663. doi: 10.1016/j.pdpdt.2020.101663
33. Krammer B, Verwanger T. Molecular response to hypericin-induced photodamage. Curr Med Chem. (2012) 19:793–8. doi: 10.2174/092986712799034842
34. Hao C, Beguinot F, Condorelli G, Trencia A, Van Meir EG, Yong VW, et al. Induction and intracellular regulation of tumor necrosis factor-related apoptosis-inducing ligand (TRAIL) mediated apotosis in human malignant glioma cells. Cancer Res. (2001) 61:1162–70.
35. Nowis D, Makowski M, Stoklosa T, Legat M, Issat T, Golab J. Direct tumor damage mechanisms of photodynamic therapy. Acta Biochim Pol. (2005) 52:339–52.
36. Elmore S. Apoptosis: a review of programmed cell death. Toxicol Pathol. (2007) 35:495–516. doi: 10.1080/01926230701320337
37. Thompson CB. Apoptosis in the pathogenesis and treatment of disease. Science. (1995) 267:1456–62. doi: 10.1126/science.7878464
38. Leibowitz B, Yu J. Mitochondrial signaling in cell death via the Bcl-2 family. Cancer Biol Ther. (2010) 9:417–22. doi: 10.4161/cbt.9.6.11392
39. Chiang PC, Chou RH, Chien HF, Tsai T, Chen CT. Chloride intracellular channel 4 involves in the reduced invasiveness of cancer cells treated by photodynamic therapy. Lasers Surg Med. (2013) 45:38–47. doi: 10.1002/lsm.22112
40. Taylor RC, Cullen SP, Martin SJ. Apoptosis: controlled demolition at the cellular level. Nat Rev Mol Cell Biol. (2008) 9:231–41. doi: 10.1038/nrm2312
41. Hellwig CT, Passante E, Rehm M. The molecular machinery regulating apoptosis signal transduction and its implication in human physiology and pathophysiologies. Curr Mol Med. (2011) 11:31–47. doi: 10.2174/156652411794474400
42. Hanna-Addams S, Liu S, Liu H, Chen S, Wang Z. CK1α, CK1δ, and CK1ε are necrosome components which phosphorylate serine 227 of human RIPK3 to activate necroptosis. Proc Natl Acad Sci USA. (2020) 117:1962–70. doi: 10.1073/pnas.1917112117
43. Galluzzi L, Kepp O, Chan FK, Kroemer G. Necroptosis: mechanisms and relevance to disease. Annu Rev Pathol. (2017) 12:103–30. doi: 10.1146/annurev-pathol-052016-100247
44. Wang H, Sun L, Su L, Rizo J, Liu L, Wang LF, et al. Mixed lineage kinase domain-like protein MLKL causes necrotic membrane disruption upon phosphorylation by RIP3. Mol Cell. (2014) 54:133–46. doi: 10.1016/j.molcel.2014.03.003
45. Seifert L, Miller G. Molecular pathways: the necrosome-A target for cancer therapy. Clin Cancer Res. (2017) 23:1132–6. doi: 10.1158/1078-0432.CCR-16-0968
46. Mohammadalipour Z, Rahmati M, Khataee A, Moosavi MA. Differential effects of N-TiO2 nanoparticle and its photo-activated form on autophagy and necroptosis in human melanoma A375 cells. J Cell Physiol. (2020) 28:1–14. doi: 10.1002/jcp.29479
47. Thibaut S, Bourre L, Hernot D, Rousset N, Lajat Y, Patrice T. Effects of BAPTA-AM, forskolin, DSF and Z.VAD.fmk on PDT-induced apoptosis and m-THPC phototoxicity on B16 cells. Apoptosis. (2002) 7:99–106. doi: 10.1023/a:1014350128251
48. Shi W, Yin Y, Wang Y, Zhang B, Tan P, Jiang T, et al. A tissue factor-cascade-targeted strategy to tumor vasculature: a combination of EGFP-EGF1 conjugation nanoparticles with photodynamic therapy. Oncotarget. (2017) 8:32212–27. doi: 10.18632/oncotarget.12922
49. Firczuk M, Nowis D, Golab J. PDT-induced inflammatory and host responses. Photochem Photobiol Sci. (2011) 10:653–63. doi: 10.1039/c0pp00308e
50. Lisnjak IO, Kutsenok VV, Polyschuk LZ, Gorobets OB, Gamaleia NF. Effect of photodynamic therapy on tumor angiogenesis and metastasis in mice bearing lewis lung carcinoma. Exp Oncol. (2005) 27:333–5.
51. Folkman J. Fighting cancer by attacking its blood supply. Sci Am. (1996) 275:150–4. doi: 10.1038/scientificamerican0996-150
52. Wyld L, Burn JL, Reed MW, Brown NJ. Factors affecting aminolaevulinic acid-induced generation of protoporphyrin IX. Br J Cancer. (1997) 76:705–12. doi: 10.1038/bjc.1997.450
53. Schuitmaker JJ, Vrensen GF, van Delft JL, de Wolff-Rouendaal D, Dubbelman TM, de Wolf A. Morphologic effects of bacteriochlorin a and light in vivo on intraocular melanoma. Invest Ophthalmol Vis Sci. (1991) 32:2683–8.
54. Zilberstein J, Schreiber S, Bloemers MC, Bendel P, Neeman M, Schechtman E, et al. Antivascular treatment of solid melanoma tumors with bacteriochlorophyll-serine-based photodynamic therapy. Photochem Photobiol. (2001) 73:257–66. doi: 10.1562/0031-86552001073<0257:atosmt>2.0.co2
55. Inoue K, Fukuhara H, Kurabayashi A, Furihata M, Tsuda M, Nagakawa K, et al. Photodynamic therapy involves an antiangiogenic mechanism and is enhanced by ferrochelatase inhibitor in urothelial carcinoma. Cancer Sci. (2013) 104:765–72. doi: 10.1111/cas.12147
56. Karwicka M, Pucelik B, Gonet M, Elas M, Dabrowski JM. Effects of photodynamic therapy with redaporfin on tumor oxygenation and blood flow in a lung cancer mouse model. Sci Rep. (2019) 9:12655. doi: 10.1038/s41598-019-49064-6
57. Baldea I, Giurgiu L, Teacoe ID, Olteanu DE, Olteanu FC, Clichici S, et al. Photodynamic therapy in melanoma - where do we stand? Curr Med Chem. (2018) 25:5540–63. doi: 10.2174/0929867325666171226115626
58. Binnewies M, Roberts EW, Kersten K, Chan V, Fearon DF, Merad M, et al. Understanding the tumor immune microenvironment (TIME) for effective therapy. Nat Med. (2018) 24:541–50. doi: 10.1038/s41591-018-0014-x
59. Wachowska M, Muchowicz A, Golab J. Targeting epigenetic processes in photodynamic therapy-induced anticancer immunity. Front Oncol. (2015) 5:176. doi: 10.3389/fonc.2015.00176
60. Pazos M, Nader HB. Effect of photodynamic therapy on the extracellular matrix and associated components. Braz J Med Biol Res. (2007) 40:1025–35. doi: 10.1590/s0100-879x2006005000142
61. Korbelik M. PDT-associated host response and its role in the therapy outcome. Lasers Surg Med. (2006) 38:500–8. doi: 10.1002/lsm.20337
62. Muchowicz A, Wachowska M, Stachura J, Tonecka K, Gabrysiak M, Wolosz D, et al. Inhibition of lymphangiogenesis impairs antitumour effects of photodynamic therapy and checkpoint inhibitors in mice. Eur J Cancer. (2017) 83:19–27. doi: 10.1016/j.ejca.2017.06.004
63. Ji J, Zhang Y, Chen WR, Wang X. DC vaccine generated by ALA-PDT-induced immunogenic apoptotic cells for skin squamous cell carcinoma. Oncoimmunology. (2016) 5:e1072674. doi: 10.1080/2162402X.2015.1072674
64. Korbelik M, Sun J, Cecic I. Photodynamic therapy-induced cell surface expression and release of heat shock proteins: relevance for tumor response. Cancer Res. (2005) 65:1018–26.
65. Terai K, Mochizuki N. Heat shock proteins regulates cardiomyocytes via toll-like receptors. Circ J. (2011) 75:2322–3. doi: 10.1253/circj.cj-11-0647
66. Tanaka M, Kataoka H, Yano S, Sawada T, Akashi H, Inoue M, et al. Immunogenic cell death due to a new photodynamic therapy (PDT) with glycoconjugated chlorin (G-chlorin). Oncotarget. (2016) 7:47242–51. doi: 10.18632/oncotarget.9725
67. Wang X, Ji J, Zhang H, Fan Z, Zhang L, Shi L, et al. Stimulation of dendritic cells by DAMPs in ALA-PDT treated SCC tumor cells. Oncotarget. (2015) 6:44688–702. doi: 10.18632/oncotarget.5975
68. Korbelik M, Zhang W, Merchant S. Involvement of damage-associated molecular patterns in tumor response to photodynamic therapy: surface expression of calreticulin and high-mobility group box-1 release. Cancer Immunol Immunother. (2011) 60:1431–7. doi: 10.1007/s00262-011-1047-x
69. Jin Y, Guan Z, Wang X, Wang Z, Zeng R, Xu L, et al. ALA-PDT promotes HPV-positive cervical cancer cells apoptosis and DCs maturation via miR-34a regulated HMGB1 exosomes secretion. Photodiagnosis Photodyn Ther. (2018) 24:27–35. doi: 10.1016/j.pdpdt.2018.08.006
70. Theodoraki MN, Lorenz K, Lotfi R, Furst D, Tsamadou C, Jaekle S, et al. Influence of photodynamic therapy on peripheral immune cell populations and cytokine concentrations in head and neck cancer. Photodiagnosis Photodyn Ther. (2017) 19:194–201. doi: 10.1016/j.pdpdt.2017.05.015
71. Reginato E, Wolf P, Hamblin MR. Immune response after photodynamic therapy increases anti-cancer and anti-bacterial effects. World J Immunol. (2014) 4:1–11. doi: 10.5411/wji.v4.i1.1
72. Maeding N, Verwanger T, Krammer B. Boosting tumor-specific immunity using PDT. Cancers. (2016) 8:91. doi: 10.3390/cancers8100091
73. Constantinidou A, Alifieris C, Trafalis DT. Targeting programmed cell death−1 (PD-1) and ligand (PD-L1): a new era in cancer active immunotherapy. Pharmacol Ther. (2019) 194:84–106. doi: 10.1016/j.pharmthera.2018.09.008
74. Wang D, Wang T, Liu J, Yu H, Jiao S, Feng B, et al. Acid-activatable versatile micelleplexes for PD-L1 blockade-enhanced cancer photodynamic immunotherapy. Nano Lett. (2016) 16:5503–13. doi: 10.1021/acs.nanolett.6b01994
75. Saji H, Song W, Furumoto K, Kato H, Engleman EG. Systemic antitumor effect of intratumoral injection of dendritic cells in combination with local photodynamic therapy. Clin Cancer Res. (2006) 12:2568–74. doi: 10.1158/1078-0432.CCR-05-1986
76. Naylor MF, Chen WR, Teague TK, Perry LA, Nordquist RE. In situ photoimmunotherapy: a tumour-directed treatment for melanoma. Br J Dermatol. (2006) 155:1287–92. doi: 10.1111/j.1365-2133.2006.07514.x
77. Li X, Naylor MF, Le H, Nordquist RE, Teague TK, Howard CA, et al. Clinical effects of in situ photoimmunotherapy on late-stage melanoma patients: a preliminary study. Cancer Biol Ther. (2010) 10:1081–7. doi: 10.4161/cbt.10.11.13434
78. Broady R, Yu J, Levings MK. Pro-tolerogenic effects of photodynamic therapy with TH9402 on dendritic cells. J Clin Apher. (2008) 23:82–91. doi: 10.1002/jca.20162
79. Whiteside TL. Exosomes and tumor-mediated immune suppression. J Clin Invest. (2016) 126:1216–23. doi: 10.1172/JCI81136
80. Pascual G, Avgustinova A, Mejetta S, Martin M, Castellanos A, Attolini CS, et al. Targeting metastasis-initiating cells through the fatty acid receptor CD36. Nature. (2017) 541:41–45. doi: 10.1038/nature20791
82. Theodoraki MN, Yerneni SS, Brunner C, Theodorakis J, Hoffmann TK, Whiteside TL. Plasma-derived exosomes reverse epithelial-to-mesenchymal transition after photodynamic therapy of patients with head and neck cancer. Oncoscience. (2018) 5:75–87. doi: 10.18632/oncoscience.410
83. Amico-Ruvio SA, Paganelli MA, Myers JM, Popescu GK. Ifenprodil effects on GluN2B-containing glutamate receptors. Mol Pharmacol. (2012) 82:1074–81. doi: 10.1124/mol.112.078998
84. Kim NH, Choi SH, Kim CH, Lee CH, Lee TR, Lee AY. Reduced MiR-675 in exosome in H19 RNA-related melanogenesis via MITF as a direct target. J Invest Dermatol. (2014) 134:1075–82. doi: 10.1038/jid.2013.478
85. Lee AY, Noh M. The regulation of epidermal melanogenesis via cAMP and/or PKC signaling pathways: insights for the development of hypopigmenting agents. Arch Pharm Res. (2013) 36:792–801. doi: 10.1007/s12272-013-0130-6
86. Tsatmali M, Ancans J, Thody AJ. Melanocyte function and its control by melanocortin peptides. J Histochem Cytochem. (2002) 50:125–33. doi: 10.1177/002215540205000201
87. Pillaiyar T, Manickam M, Jung SH. Downregulation of melanogenesis: drug discovery and therapeutic options. Drug Discov Today. (2017) 22:282–98. doi: 10.1016/j.drudis.2016.09.016
88. Speeckaert R, Van Gele M, Speeckaert MM, Lambert J, van Geel N. The biology of hyperpigmentation syndromes. Pigment Cell Melanoma Res. (2014) 27:512–24. doi: 10.1111/pcmr.12235
89. Reissmann M, Ludwig A. Pleiotropic effects of coat colour-associated mutations in humans, mice and other mammals. Semin Cell Dev Biol. (2013) 24:576–86. doi: 10.1016/j.semcdb.2013.03.014
90. Bohm M, Hill HZ. Ultraviolet B, melanin and mitochondrial DNA: photo-damage in human epidermal keratinocytes and melanocytes modulated by alpha-melanocyte-stimulating hormone. F1000Res. (2016) 5:881. doi: 10.12688/f1000research.8582.1
91. Smit NP, van Nieuwpoort FA, Marrot L, Out C, Poorthuis B, van Pelt H, et al. Increased melanogenesis is a risk factor for oxidative DNA damage–study on cultured melanocytes and atypical nevus cells. Photochem Photobiol. (2008) 84:550–5. doi: 10.1111/j.1751-1097.2007.00242.x
92. Kadekaro AL, Wakamatsu K, Ito S, Abdel-Malek ZA. Cutaneous photoprotection and melanoma susceptibility: reaching beyond melanin content to the frontiers of DNA repair. Front Biosci. (2006) 11:2157–73. doi: 10.2741/1958
93. Pellosi MC, Suzukawa AA, Scalfo AC, Di Mascio P, Martins Pereira CP, de Souza Pinto NC, et al. Effects of the melanin precursor 5,6-dihydroxy-indole-2-carboxylic acid (DHICA) on DNA damage and repair in the presence of reactive oxygen species. Arch Biochem Biophys. (2014) 557:55–64. doi: 10.1016/j.abb.2014.05.024
94. Kim SK, Oh SJ, Park SY, Kim WJ, Kim YS, Kim YC. Photodynamic therapy inhibits melanogenesis through paracrine effects by keratinocytes and fibroblasts. Pigment Cell Melanoma Res. (2018) 31:277–86. doi: 10.1111/pcmr.12658
95. Korytowski W, Kalyanaraman B, Menon IA, Sarna T, Sealy RC. Reaction of superoxide anions with melanins: electron spin resonance and spin trapping studies. Biochim Biophys Acta. (1986) 882:145–53.
96. Slominski A, Paus R, Mihm MC. Inhibition of melanogenesis as an adjuvant strategy in the treatment of melanotic melanomas: selective review and hypothesis. Anticancer Res. (1998) 18:3709–15.
97. Sharma KV, Davids LM. Depigmentation in melanomas increases the efficacy of hypericin-mediated photodynamic-induced cell death. Photodiagnosis Photodyn Ther. (2012) 9:156–63. doi: 10.1016/j.pdpdt.2011.09.003
98. Sharma KV, Bowers N, Davids LM. Photodynamic therapy-induced killing is enhanced in depigmented metastatic melanoma cells. Cell Biol Int. (2011) 35:939–44. doi: 10.1042/CBI20110103
99. Ma LW, Nielsen KP, Iani V, Moan J. A new method for photodynamic therapy of melanotic melanoma – effects of depigmentation with violet light photodynamic therapy. J Environ Pathol Toxicol Oncol. (2007) 26:165–72. doi: 10.1615/jenvironpatholtoxicoloncol.v26.i3.10
100. Ndoye A, Weeraratna AT. Autophagy- an emerging target for melanoma therapy. F1000Res. (2016) 5:8347.1. doi: 10.12688/f1000research.8347.1
101. Sheen JH, Zoncu R, Kim D, Sabatini DM. Defective regulation of autophagy upon leucine deprivation reveals a targetable liability of human melanoma cells in vitro and in vivo. Cancer Cell. (2011) 19:613–28. doi: 10.1016/j.ccr.2011.03.012
102. Ge J, Chen Z, Huang J, Chen J, Yuan W, Deng Z, et al. Upregulation of autophagy-related gene-5 (ATG-5) is associated with chemoresistance in human gastric cancer. PLoS ONE. (2014) 9:e110293. doi: 10.1371/journal.pone.0110293
103. Limpert AS, Lambert LJ, Bakas NA, Bata N, Brun SN, Shaw RJ, et al. Autophagy in cancer: regulation by small molecules. Trends Pharmacol Sci. (2018) 39:1021–32. doi: 10.1016/j.tips.2018.10.004
104. Piya S, Andreeff M, Borthakur G. Targeting autophagy to overcome chemoresistance in acute myleogenous leukemia. Autophagy. (2017) 13:214–15. doi: 10.1080/15548627.2016.1245263
105. Garcia-Fernandez M, Karras P, Checinska A, Canon E, Calvo GT, Gomez-Lopez G, et al. Metastatic risk and resistance to BRAF inhibitors in melanoma defined by selective allelic loss of ATG5. Autophagy. (2016) 12:1776–90. doi: 10.1080/15548627.2016.1199301
106. Levy JM, Thompson JC, Griesinger AM, Amani V, Donson AM, Birks DK, et al. Autophagy inhibition improves chemosensitivity in BRAF(V600E) brain tumors. Cancer Discov. (2014) 4:773–80. doi: 10.1158/2159-8290.CD-14-0049
107. Marino ML, Pellegrini P, Di Lernia G, Djavaheri-Mergny M, Brnjic S, Zhang X, et al. Autophagy is a protective mechanism for human melanoma cells under acidic stress. J Biol Chem. (2012) 287:30664–76. doi: 10.1074/jbc.M112.339127
108. Paunovic V, Kosic M, Djordjevic S, Zugic A, Djalinac N, Gasic U, et al. Marrubium vulgare ethanolic extract induces proliferation block, apoptosis, and cytoprotective autophagy in cancer cells in vitro. Cell Mol Biol. (2016) 62:108–14.
109. Xie X, Koh JY, Price S, White E, Mehnert JM. Atg7 overcomes senescence and promotes growth of Brafv600e-driven melanoma. Cancer Discov. (2015) 5:410–23. doi: 10.1158/2159-8290.CD-14-1473
110. Martin S, Dudek-Peric AM, Maes H, Garg AD, Gabrysiak M, Demirsoy S, et al. Concurrent MEK and autophagy inhibition is required to restore cell death associated danger-signalling in Vemurafenib-resistant melanoma cells. Biochem Pharmacol. (2015) 93:290–304. doi: 10.1016/j.bcp.2014.12.003
111. Duan X, Chen B, Cui Y, Zhou L, Wu C, Yang Z, et al. Ready player one? autophagy shapes resistance to photodynamic therapy in cancers. Apoptosis. (2018) 23:587–606. doi: 10.1007/s10495-018-1489-0
112. Tu P, Huang Q, Ou Y, Du X, Li K, Tao Y, et al. Aloe-emodin-mediated photodynamic therapy induces autophagy and apoptosis in human osteosarcoma cell line MG63 through the ROS/JNK signaling pathway. Oncol Rep. (2016) 35:3209–15. doi: 10.3892/or.2016.4703
113. Dos Santos AF, Terra LF, Wailemann RA, Oliveira TC, Gomes VM, Mineiro MF, et al. Methylene blue photodynamic therapy induces selective and massive cell death in human breast cancer cells. BMC Cancer. (2017) 17:194. doi: 10.1186/s12885-017-3179-7
114. Wei MF, Chen MW, Chen KC, Lou PJ, Lin SY, Hung SC, et al. Autophagy promotes resistance to photodynamic therapy-induced apoptosis selectively in colorectal cancer stem-like cells. Autophagy. (2014) 10:1179–92. doi: 10.4161/auto.28679
115. Levine B, Kroemer G. Autophagy in aging, disease and death: the true identity of a cell death impostor. Cell Death Diff. (2009) 16:1–2. doi: 10.1038/cdd.2008.139
116. Kawczyk-Krupka A, Wawrzyniec K, Musiol SK, Potempa M, Bugaj AM, Sieron A. Treatment of localized prostate cancer using WST-09 and WST-11 mediated vascular targeted photodynamic therapy-a review. Photodiagnosis Photodyn Ther. (2015) 12:567–74. doi: 10.1016/j.pdpdt.2015.10.001
117. Li CF, Sun JX, Gao Y, Shi F, Pan YK, Wang YC, et al. Clinorotation-induced autophagy via HDM2-p53-mTOR pathway enhances cell migration in vascular endothelial cells. Cell Death Dis. (2018) 9:147. doi: 10.1038/s41419-017-0185-2
118. Riffelmacher T, Richter FC, Simon AK. Autophagy dictates metabolism and differentiation of inflammatory immune cells. Autophagy. (2018) 14:199–206. doi: 10.1080/15548627.2017.1362525
119. Gao Y, Zhu H, Yang F, Wang Q, Feng Y, Zhang C. Glucocorticoid-activated IRE1alpha/XBP-1s signaling: an autophagy-associated protective pathway against endotheliocyte damage. Am J Physiol Cell Physiol. (2018) 315:C300–9. doi: 10.1152/ajpcell.00009.2018
120. Gomaa I, Sebak A, Afifi N, Abdel-Kader M. Liposomal delivery of ferrous chlorophyllin: a novel third generation photosensitizer for in vitro PDT of melanoma. Photodiagnosis Photodyn Ther. (2017) 18:162–70. doi: 10.1016/j.pdpdt.2017.01.186
121. Nowak-Sliwinska P, Karocki A, Elas M, Pawlak A, Stochel G, Urbanska K. Verteporfin, photofrin II, and merocyanine 540 as PDT photosensitizers against melanoma cells. Biochem Biophys Res Commun. (2006) 349:549–55. doi: 10.1016/j.bbrc.2006.08.060
122. Saczko J, Kulbacka J, Chwilkowska A, Drag-Zalesiniska M, Wysocka T, Lugowski M, et al. The influence of photodynamic therapy on apoptosis in human melanoma cell line. Folia Histochem Cytobiol. (2005) 43:129–32.
123. Ferrario A, Rucker N, Wong S, Luna M, Gomer CJ. Survivin, a member of the inhibitor of apoptosis family, is induced by photodynamic therapy and is a target for improving treatment response. Cancer Res. (2007) 67:4989–95. doi: 10.1158/0008-5472.CAN-06-4785
124. Chang CJ, Yu JS, Wei FC. In vitro and in vivo photosensitizing applications of photofrin in malignant melanoma cells. Chang Gung Med J. (2008) 31:260–7.
125. Lang K, Bolsen K, Stahl W, Ruzicka T, Sies H, Lehmann P, et al. The 5-aminolevulinic acid-induced porphyrin biosynthesis in benign and malignant cells of the skin. J Photochem Photobiol B. (2001) 65:29–34. doi: 10.1016/s1011-1344(01)00242-1
126. Haddad R, Kaplan O, Greenberg R, Siegal A, Skornick Y, Kashtan H. Photodynamic therapy of murine colon cancer and melanoma using systemic aminolevulinic acid as a photosensitizer. Int J Surg Investig. (2000) 2:171–8.
127. Krestyn E, Kolarova H, Bajgar R, Tomankova K. Photodynamic properties of ZnTPPS(4), ClAlPcS(2) and ALA in human melanoma G361 cells. Toxicol In Vitro. (2010) 24:286–91. doi: 10.1016/j.tiv.2009.08.015
128. Frank J, Lornejad-Schafer MR, Schoffl H, Flaccus A, Lambert C, Biesalski HK. Inhibition of heme oxygenase-1 increases responsiveness of melanoma cells to ALA-based photodynamic therapy. Int J Oncol. (2007) 31:1539–45.
129. Ickowicz Schwartz D, Gozlan Y, Greenbaum L, Babushkina T, Katcoff DJ, Malik Z. Differentiation-dependent photodynamic therapy regulated by porphobilinogen deaminase in B16 melanoma. Br J Cancer. (2004) 90:1833–41. doi: 10.1038/sj.bjc.6601760
130. Schmitt F, Govindaswamy P, Zava O, Suss-Fink G, Juillerat-Jeanneret L, Therrien B. Combined arene ruthenium porphyrins as chemotherapeutics and photosensitizers for cancer therapy. J Biol Inorg Chem. (2009) 14:101–9. doi: 10.1007/s00775-008-0427-y
131. Serra A, Pineiro M, Santos CI, Gonsalves AM, Abrantes M, Laranjo M, et al. In vitro photodynamic activity of 5, 15-bis(3-hydroxyphenyl)porphyrin and its halogenated derivatives against cancer cells. Photochem Photobiol. (2010) 86:206–12. doi: 10.1111/j.1751-1097.2009.00622.x
132. Nackiewicz J, Kliber-Jasik M, Skonieczna M. A novel pro-apoptotic role of zinc octacarboxyphthalocyanine in melanoma me45 cancer cell's photodynamic therapy (PDT). J Photochem Photobiol B. (2019) 190:146–53. doi: 10.1016/j.jphotobiol.2018.12.002
133. Ndhundhuma IM, Abrahamse H. Susceptibility of in vitro melanoma skin cancer to photoactivated hypericin versus aluminium(III) phthalocyanine chloride tetrasulphonate. Biomed Res Int. (2017) 2017:5407012. doi: 10.1155/2017/5407012
134. Decreau R, Richard MJ, Verrando P, Chanon M, Julliard M. Photodynamic activities of silicon phthalocyanines against achromic M6 melanoma cells and healthy human melanocytes and keratinocytes. J Photochem Photobiol B. (1999) 48:48–56. doi: 10.1016/S1011-1344(99)00008-1
135. Barge J, Decreau R, Julliard M, Hubaud JC, Sabatier AS, Grob JJ, et al. Killing efficacy of a new silicon phthalocyanine in human melanoma cells treated with photodynamic therapy by early activation of mitochondrion-mediated apoptosis. Exp Dermatol. (2004) 13:33–44. doi: 10.1111/j.0906-6705.2004.00147.x
136. Kolarova H, Tomankova K, Bajgar R, Kolar P, Kubinek R. Photodynamic and sonodynamic treatment by phthalocyanine on cancer cell lines. Ultrasound Med Biol. (2009) 35:1397–404. doi: 10.1016/j.ultrasmedbio.2009.03.004
137. Kolarova H, Nevrelova P, Bajgar R, Jirova D, Kejlova K, Strnad M. In vitro photodynamic therapy on melanoma cell lines with phthalocyanine. Toxicol In Vitro. (2007) 21:249–53. doi: 10.1016/j.tiv.2006.09.020
138. Yue J, Liang L, Shen Y, Guan X, Zhang J, Li Z, et al. Investigating dynamic molecular events in melanoma cell nucleus during photodynamic therapy by SERS. Front Chem. (2018) 6:665. doi: 10.3389/fchem.2018.00665
139. Oh DS, Kim H, Oh JE, Jung HE, Lee YS, Park JH, et al. Intratumoral depletion of regulatory T cells using CD25-targeted photodynamic therapy in a mouse melanoma model induces antitumoral immune responses. Oncotarget. (2017) 8:47440–53. doi: 10.18632/oncotarget.17663
140. Ji Y, Zhao J, Chu CC. Biodegradable nanocomplex from hyaluronic acid and arginine based poly(ester amide)s as the delivery vehicles for improved photodynamic therapy of multidrug resistant tumor cells: an in vitro study of the performance of chlorin e6 photosensitizer. J Biomed Mater Res A. (2017) 105:1487–99. doi: 10.1002/jbm.a.35982
141. Lee CH, Lai PS, Lu YP, Chen HY, Chai CY, Tsai RK, et al. Real-time vascular imaging and photodynamic therapy efficacy with micelle-nanocarrier delivery of chlorin e6 to the microenvironment of melanoma. J Dermatol Sci. (2015) 80:124–32. doi: 10.1016/j.jdermsci.2015.08.005
142. Santos GMP, Oliveira S, Monteiro JCS, Fagnani SR, Sampaio FP, Correia NA, et al. ROS-induced autophagy reduces B16F10 melanoma cell proliferative activity. Lasers Med Sci. (2018) 33:1335–40. doi: 10.1007/s10103-018-2489-6
143. Rice L, Wainwright M, Phoemix DA. Phenothiazine photosensitizers. III. Activity of methylene blue derivatives against pigmented melanoma cell lines. J Chemother. (2000) 12:94–104. doi: 10.1179/joc.2000.12.1.94
144. Davids LM, Kleemann B, Cooper S, Kidson SH. Melanomas display increased cytoprotection to hypericin-mediated cytotoxicity through the induction of autophagy. Cell Biol Int. (2009) 33:1065–72. doi: 10.1016/j.cellbi.2009.06.026
145. Davids LM, Kleemann B, Kacerovska D, Pizinger K, Kidson SH. Hypericin phototoxicity induces different modes of cell death in melanoma and human skin cells. J Photochem Photobiol B. (2008) 91:67–76. doi: 10.1016/j.jphotobiol.2008.01.011
146. Li Z, Wang C, Deng H, Wu J, Huang H, Sun R, et al. Robust photodynamic therapy using 5-ALA-incorporated nanocomplexes cures metastatic melanoma through priming of CD4+CD8+ double positive T cells. Adv Sci. (2019) 6:1802057. doi: 10.1002/advs.201802057
147. Lin MW, Huang YB, Chen CL, Wu PC, Chou CY, Wu PC, et al. A formulation study of 5-aminolevulinic encapsulated in DPPC liposomes in melanoma treatment. Int J Med Sci. (2016) 13:483–9. doi: 10.7150/ijms.15411
148. Shivashankarappa A, Sanjay KR. Photodynamic therapy on skin melanoma and epidermoid carcinoma cells using conjugated 5-aminolevulinic acid with microbial synthesised silver nanoparticles. J Drug Target. (2019) 27:434–41. doi: 10.1080/1061186X.2018.1531418
149. Clemente N, Miletto I, Gianotti E, Invernizzi M, Marchese L, Dianzani U, et al. Verteporfin-loaded mesoporous silica nanoparticles inhibit mouse melanoma proliferation in vitro and in vivo. J Photochem Photobiol B. (2019) 197:111533. doi: 10.1016/j.jphotobiol.2019.111533
150. Freitas LF, Hamblin MR, Anzengruber F, Perussi JR, Ribeiro AO, Martins VCA, et al. Zinc phthalocyanines attached to gold nanorods for simultaneous hyperthermic and photodynamic therapies against melanoma in vitro. J Photochem Photobiol B. (2017) 173:181–6. doi: 10.1016/j.jphotobiol.2017.05.037
151. Goto PL, Siqueira-Moura MP, Tedesco AC. Application of aluminum chloride phthalocyanine-loaded solid lipid nanoparticles for photodynamic inactivation of melanoma cells. Int J Pharm. (2017) 518:228–41. doi: 10.1016/j.ijpharm.2017.01.004
152. Zeni PF, Santos DPD, Canevarolo RR, Yunes JA, Padilha FF, Junior R, et al. Photocatalytic and cytotoxic effects of nitrogen-doped TiO2 nanoparticles on melanoma cells. J Nanosci Nanotechnol. (2018) 18:3722–8. doi: 10.1166/jnn.2018.14621
153. Zhan J, Ma Z, Wang D, Li X, Li X, Le L, et al. Magnetic and pH dual-responsive mesoporous silica nanocomposites for effective and low-toxic photodynamic therapy. Int J Nanomedicine. (2017) 12:2733–48. doi: 10.2147/IJN.S127528
154. Cheng Y, Chang Y, Feng Y, Liu N, Sun X, Feng Y, et al. Simulated sunlight-mediated photodynamic therapy for melanoma skin cancer by titanium-dioxide-nanoparticle-gold-nanocluster-graphene heterogeneous nanocomposites. Small. (2017) 13:1603935. doi: 10.1002/smll.201603935
155. Zhang C, Shi G, Zhang J, Niu J, Huang P, Wang Z, et al. Redox- and light-responsive alginate nanoparticles as effective drug carriers for combinational anticancer therapy. Nanoscale. (2017) 9:3304–14. doi: 10.1039/c7nr00005g
156. Urbanska K, Romanowska-Dixon B, Matuszak Z, Oszajca J, Nowak-Sliwinska P, Stochel G. Indocyanine green as a prospective sensitizer for photodynamic therapy of melanomas. Acta Biochim Pol. (2002) 49:387–91.
157. Mamoon AM, Gamal-Eldeen AM, Ruppel ME, Smith RJ, Tsang T, Miller LM. In vitro efficiency and mechanistic role of indocyanine green as photodynamic therapy agent for human melanoma. Photodiagnosis Photodyn Ther. (2009) 6:105–16. doi: 10.1016/j.pdpdt.2009.05.002
158. Jiang BP, Zhang L, Guo XL, Shen XC, Wang Y, Zhu Y, et al. Poly(N-phenylglycine)-based nanoparticles as highly effective and targeted near-infrared photothermal therapy/photodynamic therapeutic agents for malignant melanoma. Small. (2017) 13:1602496. doi: 10.1002/smll.201602496
159. Gross S, Gilead A, Scherz A, Neeman M, Salomon Y. Monitoring photodynamic therapy of solid tumors online by BOLD-contrast MRI. Nat Med. (2003) 9:1327–31. doi: 10.1038/nm940
160. Camerin M, Rello-Varona S, Villanueva A, Rodgers MA, Jori G. Metallo-naphthalocyanines as photothermal sensitisers for experimental tumours: in vitro and in vivo studies. Lasers Surg Med. (2009) 41:665–73. doi: 10.1002/lsm.20846
161. Tham HP, Xu K, Lim WQ, Chen H, Zheng M, Thng TGS, et al. Microneedle-assisted topical delivery of photodynamically active mesoporous formulation for combination therapy of deep-seated melanoma. ACS Nano. (2018) 12:11936–48. doi: 10.1021/acsnano.8b03007
162. Pereira NA, Laranjo M, Casalta-Lopes J, Serra AC, Pineiro M, Pina J, et al. Platinum(II) ring-fused chlorins as near-infrared emitting oxygen sensors and photodynamic agents. ACS Med Chem Lett. (2017) 8:310–5. doi: 10.1021/acsmedchemlett.6b00476
163. Chen Y, Zheng W, Li Y, Zhong J, Ji J, Shen P. Apoptosis induced by methylene-blue-mediated photodynamic therapy in melanomas and the involvement of mitochondrial dysfunction revealed by proteomics. Cancer Sci. (2008) 99:2019–27. doi: 10.1111/j.1349-7006.2008.00910.x
164. Wagner M, Suarez ER, Theodoro TR, Machado Filho CD, Gama MF, Tardivo JP, et al. Methylene blue photodynamic therapy in malignant melanoma decreases expression of proliferating cell nuclear antigen and heparanases. Clin Exp Dermatol. (2012) 37:527–33. doi: 10.1111/j.1365-2230.2011.04291.x
165. Germic N, Frangez Z, Yousefi S, Simon HU. Regulation of the innate immune system by autophagy: monocytes, macrophages, dendritic cells and antigen presentation. Cell Death Differ. (2019) 26:715–27. doi: 10.1038/s41418-019-0297-6
166. Shanmugapriya K, Kang HW. Engineering pharmaceutical nanocarriers for photodynamic therapy on wound healing: review. Mater Sci Eng C Mater Biol Appl. (2019) 105:110110. doi: 10.1016/j.msec.2019.110110
167. Wu J, Du S, Wang Y. Photosensitizer coated upconversion nanoparticles for triggering reactive oxygen species under 980 nm near-infrared excitation. J Mater Chem B. (2019) 7:7306–13. doi: 10.1039/c9tb01629e
168. Pan M, Jiang Q, Sun J, Xu Z, Zhou Y, Zhang L, et al. Programming DNA nanoassembly for enhanced photodynamic therapy. Angew Chem Int Ed Engl. (2019) 132:24381. doi: 10.1002/anie.201912574
169. Jia X, Jia L. Nanoparticles improve biological functions of phthalocyanine photosensitizers used for photodynamic therapy. Curr Drug Metab. (2012) 13:1119–22. doi: 10.2174/138920012802850074
170. Huang YY, Sharma SK, Dai T, Chung H, Yaroslavsky A, Garcia-Diaz M, et al. Can nanotechnology potentiate photodynamic therapy? Nanotechnol Rev. (2012) 1:111–46. doi: 10.1515/ntrev-2011-0005
171. Monge-Fuentes V, Muehlmann LA, de Azevedo RB. Perspectives on the application of nanotechnology in photodynamic therapy for the treatment of melanoma. Nano Rev. (2014) 5:24381. doi: 10.3402/nano.v5.24381
172. Wu M, Ding Y, Li L. Recent progress in the augmentation of reactive species with nanoplatforms for cancer therapy. Nanoscale. (2019) 11:19658–83. doi: 10.1039/c9nr06651a
173. Rajkumar S, Prabaharan M. Theranostics based on iron oxide and gold nanoparticles for imaging- guided photothermal and photodynamic therapy of cancer. Curr Top Med Chem. (2017) 17:1858–71. doi: 10.2174/1568026617666161122120537
174. Gamaleia NF, Shton IO. Gold mining for PDT: great expectations from tiny nanoparticles. Photodiagnosis Photodyn Ther. (2015) 12:221–31. doi: 10.1016/j.pdpdt.2015.03.002
175. Wang C, Cheng L, Liu Z. Upconversion nanoparticles for photodynamic therapy and other cancer therapeutics. Theranostics. (2013) 3:317–30. doi: 10.7150/thno.5284
176. Liu K, Jiang X, Hunziker P. Carbohydrate-based amphiphilic nano delivery systems for cancer therapy. Nanoscale. (2016) 8:16091–156. doi: 10.1039/c6nr04489a
177. Luoma H, Turtola L, Collan Y, Meurman J, Helminen S. The effect of a bicarbonate-phosphate combination, without and with fluoride, on the growth and dental caries in the rat. Caries Res. (1972) 6:183–92. doi: 10.1159/000259789
178. Jin ZH, Miyoshi N, Ishiguro K, Umemura S, Kawabata K, Yumita N, et al. Combination effect of photodynamic and sonodynamic therapy on experimental skin squamous cell carcinoma in C3H/HeN mice. J Dermatol. (2000) 27:294–306. doi: 10.1111/j.1346-8138.2000.tb02171.x
179. Hashemi MM, Holden BS, Coburn J, Taylor MF, Weber S, Hilton B, et al. Proteomic Analysis of resistance of gram-negative bacteria to chlorhexidine and impacts on susceptibility to colistin, antimicrobial peptides, and ceragenins. Front Microbiol. (2019) 10:210. doi: 10.3389/fmicb.2019.00210
180. Harada Y, Ogawa K, Irie Y, Endo H, Feril LB Jr, Uemura T, et al. Ultrasound activation of TiO2 in melanoma tumors. J Control Release. (2011) 149:190–5. doi: 10.1016/j.jconrel.2010.10.012
181. Hu Z, Fan H, Lv G, Zhou Q, Yang B, Zheng J, et al. 5-Aminolevulinic acid-mediated sonodynamic therapy induces anti-tumor effects in malignant melanoma via p53-miR-34a-Sirt1 axis. J Dermatol Sci. (2015) 79:155–62. doi: 10.1016/j.jdermsci.2015.04.010
182. Gorgizadeh M, Azarpira N, Lotfi M, Daneshvar F, Salehi F, Sattarahmady N. Sonodynamic cancer therapy by a nickel ferrite/carbon nanocomposite on melanoma tumor: in vitro and in vivo studies. Photodiagnosis Photodyn Ther. (2019) 27:27–33. doi: 10.1016/j.pdpdt.2019.05.023
183. Liu M, Khan AR, Ji J, Lin G, Zhao X, Zhai G. Crosslinked self-assembled nanoparticles for chemo-sonodynamic combination therapy favoring antitumor, antimetastasis management and immune responses. J Control Release. (2018) 290:150–64. doi: 10.1016/j.jconrel.2018.10.007
184. Xu P, Ning P, Wang J, Qin Y, Liang F, Cheng Y. Precise control of apoptosis via gold nanostars for dose dependent photothermal therapy of melanoma. J Mater Chem B. (2019) 7:6934–44. doi: 10.1039/c9tb01956a
185. Zhang D, Wu T, Qin X, Qiao Q, Shang L, Song Q, et al. Intracellularly generated immunological gold nanoparticles for combinatorial photothermal therapy and immunotherapy against tumor. Nano Lett. (2019) 19:6635–46. doi: 10.1021/acs.nanolett.9b02903
186. Alvi SB, Appidi T, Deepak BP, Rajalakshmi PS, Minhas G, Singh SP, et al. The “nano to micro” transition of hydrophobic curcumin crystals leading to in situ adjuvant depots for Au-liposome nanoparticle mediated enhanced photothermal therapy. Biomater Sci. (2019) 7:3866–75. doi: 10.1039/c9bm00932a
187. Barbazetto IA, Lee TC, Rollins IS, Chang S, Abramson DH. Treatment of choroidal melanoma using photodynamic therapy. Am J Ophthalmol. (2003) 135:898–9. doi: 10.1016/s0002-9394(02)02222-5
188. Donaldson MJ, Lim L, Harper CA, Mackenzie J, Campbell GW. Primary treatment of choroidal amelanotic melanoma with photodynamic therapy. Clin Exp Ophthalmol. (2005) 33:548–9. doi: 10.1111/j.1442-9071.2005.01083.x
189. Campbell WG, Pejnovic TM. Treatment of amelanotic choroidal melanoma with photodynamic therapy. Retina. (2012) 32:1356–62. doi: 10.1097/IAE.10.1097/IAE.0b013e31822c28ec
190. O'Day RF, Pejnovic TM, Isaacs T, Muecke JS, Glasson WJ, Campbell WG. Australian and New Zealand study of photodynamic therapy in choroidal amelanotic melanoma. Retina. (2019) 19:972–6. doi: 10.1097/IAE.0000000000002520
191. Turkoglu EB, Pointdujour-Lim R, Mashayekhi A, Shields CL. Photodynamic therapy as primary treatment for small choroidal melanoma. Retina. (2019) 39:1319–25. doi: 10.1097/IAE.0000000000002169
192. Fabian ID, Stacey AW, Papastefanou V, Al Harby L, Arora AK, Sagoo MS, et al. Primary photodynamic therapy with verteporfin for small pigmented posterior pole choroidal melanoma. Eye. (2017) 31:519–28. doi: 10.1038/eye.2017.22
193. Sheleg SV, Zhavrid EA, Khodina TV, Kochubeev GA, Istomin YP, Chalov VN, et al. Photodynamic therapy with chlorin e(6) for skin metastases of melanoma. Photodermatol Photoimmunol Photomed. (2004) 20:21–6. doi: 10.1111/j.1600-0781.2004.00078.x
194. Kang HK, Yun JH, Son YM, Roh JY, Lee JR. Photodynamic therapy for bowen's disease of the vulva area. Ann Dermatol. (2014) 26:241–5. doi: 10.5021/ad.2014.26.2.241
195. Lecluse LL, Spuls PI. Photodynamic therapy versus topical imiquimod versus topical fluorouracil for treatment of superficial basal-cell carcinoma: a single blind, non-inferiority, randomised controlled trial: a critical appraisal. Br J Dermatol. (2015) 172:8–10. doi: 10.1111/bjd.13460
196. Ell C, Gossner L, May A, Schneider HT, Hahn EG, Stolte M, et al. Photodynamic ablation of early cancers of the stomach by means of mTHPC and laser irradiation: preliminary clinical experience. Gut. (1998) 43:345–9. doi: 10.1136/gut.43.3.345
197. Messmann H, Szeimies RM, Baumler W, Knuchel R, Zirngibl H, Scholmerich J, et al. Enhanced effectiveness of photodynamic therapy with laser light fractionation in patients with esophageal cancer. Endoscopy. (1997) 29:275–80. doi: 10.1055/s-2007-1004189
198. Hu YE, Dai SF, Wang B, Qu W, Gao JL. Therapeutic effects of topical 5-aminolevulinic acid photodynamic therapy. Pak J Med Sci. (2016) 32:961–4. doi: 10.12669/pjms.324.9634
Keywords: photodynamic therapy, melanoma, apoptosis, autophagy, tumor immunity
Citation: Li X-Y, Tan L-C, Dong L-W, Zhang W-Q, Shen X-X, Lu X, Zheng H and Lu Y-G (2020) Susceptibility and Resistance Mechanisms During Photodynamic Therapy of Melanoma. Front. Oncol. 10:597. doi: 10.3389/fonc.2020.00597
Received: 28 December 2019; Accepted: 01 April 2020;
Published: 12 May 2020.
Edited by:
Joshua Arbesman, Cleveland Clinic, United StatesReviewed by:
Edward V. Maytin, Cleveland Clinic, United StatesCopyright © 2020 Li, Tan, Dong, Zhang, Shen, Lu, Zheng and Lu. This is an open-access article distributed under the terms of the Creative Commons Attribution License (CC BY). The use, distribution or reproduction in other forums is permitted, provided the original author(s) and the copyright owner(s) are credited and that the original publication in this journal is cited, in accordance with accepted academic practice. No use, distribution or reproduction is permitted which does not comply with these terms.
*Correspondence: Yuan-Gang Lu, c2tpbjUxNUAxNjMuY29t; Hong Zheng, emllY29lQDE2My5jb20=
Disclaimer: All claims expressed in this article are solely those of the authors and do not necessarily represent those of their affiliated organizations, or those of the publisher, the editors and the reviewers. Any product that may be evaluated in this article or claim that may be made by its manufacturer is not guaranteed or endorsed by the publisher.
Research integrity at Frontiers
Learn more about the work of our research integrity team to safeguard the quality of each article we publish.