- Division of Pathology, Department of Translational Research and New Technologies in Medicine and Surgery, University of Pisa, Pisa, Italy
The processes of recurrence and metastasis, through which cancer relapses locally or spreads to distant sites in the body, accounts for more than 90% of cancer-related deaths. At present there are very few treatment options for patients at this stage of their disease. The main obstacle to successfully treat advanced cancer is the cells' ability to change in ways that make them resistant to treatment. Understanding the cellular mechanisms that mediate this cancer cell plasticity may lead to improved patient survival. Epigenetic reprogramming, together with tumor microenvironment, drives such dynamic mechanisms favoring tumor heterogeneity, and cancer cell plasticity. In addition, the development of new approaches that can report on cancer plasticity in their native environment have profound implications for studying cancer biology and monitoring tumor progression. We herein provide an overview of recent advancements in understanding the mechanisms regulating cell plasticity and current strategies for their monitoring and therapy management.
Cancer Cell Plasticity: a New Level of Heterogeneity in a Tumor
Tumor heterogeneity can be inter-tumoral, if genetic variations are found among different patients with tumors of the same type, or intra-tumoral, involving different cancer cells in the same tumor. In particular, intra-tumor heterogeneity can be caused by genetic variation, modulation in the expression of a gene, transition among cellular states or environmental changes (1). Thus, it is easy to understand that intra-tumor heterogeneity drives cancer progression and represents the main cause of treatment failure (2).
Initially, two models were proposed to justify intra-tumor heterogeneity: the “clonal evolution” model and the “cancer stem-like cell” (CSC) model. The first contemplates differences among cancer cells due to stochastic alterations in genes; according to this theory, clones which gain a growth advantage are selected over time (3, 4). The second involves CSCs, a minority population of cancer cells with self-renewing capacity that initiates and maintains tumor growth, in contrast with the majority of the cancer cells which show a more differentiated phenotype (5–7). Lately, a third model has been proposed: the “CSC plasticity” model, where CSCs possess the capacity to move between stem and differentiated states. This shift may be caused by intrinsic cues such as genetic mutations and/or epigenetic modifications but also by extrinsic cues from the tumor microenvironment (inflammation, injury, senescence). In addition, the tumor-initiating potential is enhanced by the overexpression of transcription factors involved in the process of epithelial-to-mesenchymal transition (EMT) (8–10) and CSCs exhibit an induced EMT program (11). These data suggest that EMT is strictly linked to CSC features. Indeed, CSCs switch between epithelial and mesenchymal states and this process depends on both genetic mutations, epigenetic modifications and transcriptional modulation of cancer cells and signals provided by the tumor microenvironment through the mediation of growth factors, cytokines, cancer-associated fibroblasts (CAFs), tumor associated macrophages (TAMs) and hypoxia (12, 13) (Figure 1). These transitions promote metastasis at distant sites as well as drug resistance and, therefore, disease recurrence (14–16). In breast cancer cells co-expression of epithelial and mesenchymal genes promotes stemness inducing the formation of 3D-spheroid structures named “tumor-spheres” (17). Moreover, cells with intermediate state of EMT showed similar tumor-initiating potential when compared with fully differentiated mesenchymal cells in a mouse model of prostate cancer (18). Thus, we suppose that cancer cell stemness may be associated with a partial EMT phenotype and, indeed, cells which exhibit this intermediate EMT state possess a much more pronounced plasticity (19). According to this definition, the CSC plasticity model suggests that the two historical models of cancer heterogeneity, i.e., the clonal evolution model and the CSC model, are not mutually exclusive (1, 20–22). We believe that this third model suggests a new level of complexity in tumor heterogeneity concept.
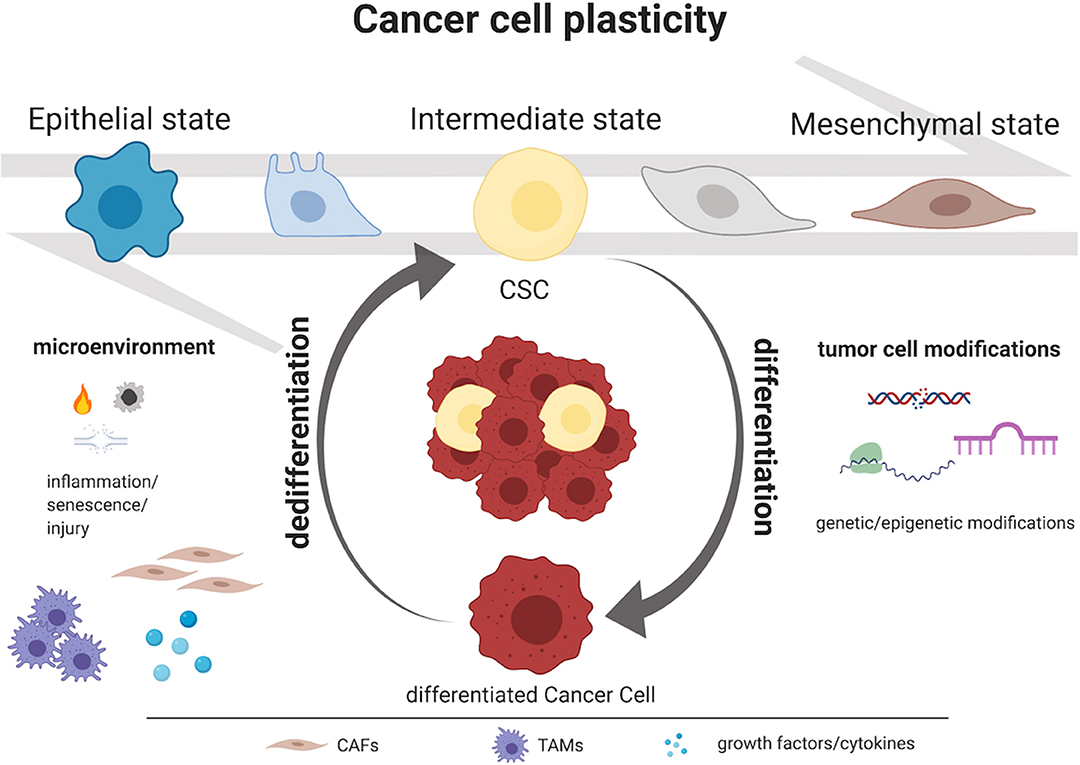
Figure 1. Mechanisms governing CSC plasticity model. Intra-tumor heterogeneity relies on the capacity to shift dynamically and reversibly between CSC and non-CSC/differentiated state. Tumor cell modifications as genetic and epigenetic alterations and microenvironment perturbations as inflammation, injury, and senescence represent the major causes of cancer cells plasticity. Moreover, CSCs exhibit an induced epithelial-to-mesenchymal transition (EMT) program and, particularly, they display an intermediate state of EMT. This process depends on both genetic mutations, epigenetic modifications and transcriptional modulation of cancer cells and signals provided by the tumor microenvironment (i.e., growth factors, cytokines, CAFs or TAMs). Created with BioRender.com.
Novel Approaches for Monitoring Tumor Cell Plasticity and Progression
Solid tumors are consisted of several sub-clonal cells populations, which compete in a Darwinian manner under the selective pressures of endogenous and exogenous factors, leading to the clonal evolution of a dominant subclone that will characterize the tumor's molecular landscape. Hence, it will be highly heterogeneous and will dynamically change during the disease progression, so longitudinal sampling is essential to define therapeutic strategies.
Currently, cancer molecular profile is evaluated through “solid biopsies” from primary tumor or metastatic nodule; however, this approach has several issues: (i) biopsies are not representative of the whole tumor mass; (ii) often tumor site is not accessible; (iii) frequently, biopsies cannot be serially performed; (iv) each metastasis could have a different genomic landscape; (v) finally, therapeutic selective pressure has to be considered too (23–28). To overcome these limitations the novel approach of “liquid biopsy” is gaining attention.
The rapid turnover of cancer cells results in the constant release into the bloodstream of: (i) cell-free circulating tumor DNA (ctDNA); (ii) tumor derived RNA (predominantly micro-RNAs and long-non-coding-RNA) (29); (iii) circulating tumor cells (CTCs); and (iv) extracellular vesicles (EVs) (sub-cellular structures with a membrane that contain nucleic acids and/or proteins) (30–33). This enables clinicians to repeatedly and non-invasively interrogate the dynamic evolution of human cancers.
CTCs are (probably) intravasated or passively spread from the primary and/or secondary tumor sites into the bloodstream, and could be responsible for the beginning of distant metastases.
In cancer patients CTCs can be isolated single or in clusters with other CTCs or with endothelial cells, platelets, leukocytes and fibroblasts, conferring them resistance to oxidative stress, and protection from the immune system (34). Their absolute number is really low (~1 CTC per 1 × 109 blood cells), especially in early cancer stage, and can vary between cancer types (34–36).
CTCs detection and isolation challenges are related to the high sensitivity and specificity required, and several factors still hamper standardized clinical application. Different approaches have been extensively investigated to isolate CTCs: (i) technologies such as density gradient stratification, membrane filtration, photoacoustic detection, dielectric mobility, and microfluidic separation are based on CTCs physical properties (density, size, mechanical plasticity, and dielectric mobility) that are different from those of other blood cells (37, 38). However, these techniques have low specificity (39), so new antibody-based functional assays have been developed: (ii) cytometric high-throughput imaging which provides the scanning of cells on slides; (iii) negative depletion of leucocytes and erythrocytes (Batch cell lysis, Microfluidic CTC-iChip, Immunomagnetic separation) (40) using specific antigens such as CD45 for leucocytes and glycophorin for erythrocytes; (iv) positive CTC enrichment by specific markers expressed on the cell surface (CellSearch, Magsweeper, Microfluidic CTC-Chip) such as epithelial cell adhesion molecule (EpCAM) (41) cytokeratins (CK8, CK18, CK19) (42) or tumor specific markers (TTF-1, PSA, HER-2 etc.) (43, 44). Nevertheless, no agreement has been reached on the specific antibodies to test. Indeed, EpCAM is usually lost during EMT, that sustains CTC migration, extravasation and apoptosis/anoikis resistance (45). Additionally, CTCs may develop a stem-like phenotype (46, 47). Hence, it is possible to find “commingling” CTCs that express epithelial, EMT or cancer stem cells phenotype; these CTCs have the highest plasticity potential and thus may represent CSCs (48). Different expression levels of stem cell markers such as CD24, CD44, CD133, ALDH, NANOG, OCT4, were found in ovarian (49), breast (50), and prostate CTCs (51). Remarkably, CTCs differentially express genes involved in oncogenic signaling pathways depending on their plasticity or stemness levels (52–54).
Finally, innovative developed approaches to CTCs/CSCs isolation are based on: (v) CTCs functional features such as protein secretion and cell migration (Epispot assay, Invasion assay) that allow the attachment of these cells to synthetic substrates co-treated with specific matching molecules (55); (vi) nanotechnology (Immunomagnetic nanobeads, Nanostructures substrates in microchip) (56, 57); (vii) the combination of surface/cytoplasmic markers, size and dielectrophoretic migration properties (DEPArray) (58).
Despite different approaches, in our opinion none of them completely satisfy the necessary requirements since low purity, loss of CTCs, and a narrow detection range still need to be tackled.
Finally, an additional central aspect to consider in the cancer plasticity is the complex network of epithelial-stromal cells interactions. Stroma undergoes, in parallel with the epithelial compartment, in a dynamic remodeling that may predict and explain several clinico-pathological features (59–63). To date, several in-vitro and in in-vivo models have been created and novel approaches have been used to study this interaction and its remodeling (64–66): genomic (scRNA-seq); protein translation and secretion (serial analysis of gene expression, antibody arrays and bead-based arrays, mass spectrometry and yeast, bacterial and mammalian secretion traps); autocrine, paracrine and long distance (cells co-culture, proximal culture); and directly in human tissue (multispectral imaging analysis). However, stroma characterization is still incomplete and fragmentary, also because of the difficulty to perform an “evolution tracking” of the whole stromal compartment.
Since malignancies development and progression are the result of these complex interactions, we believe that the treatment with chemotherapeutic agents against the cancer epithelial compartment combined with novel stroma-targeted therapies, may efficiently reduce cancer recurrence, also thank to the targeting and eradication of CSCs.
Clinical Relevance of Cancer Cell Plasticity: Limitations and New Opportunities
Though the presence of CTCs has been known since the 1869 (67), their clinical relevance was demonstrated only in 1994 (68). Despite their low number in the blood stream, they are related to clinical outcomes (34–36). In our opinion CTCs and CSCs may represent the key for early diagnosis, better prognostic stratification and a more accurate therapeutic response prediction; in addition, their concentration and pheno/genotyping could be easily measured and repeated over time. To date, however, only few authors tried to demonstrate advantages of liquid biopsy over the solid biopsies in cancer surveillance and follow-up (69, 70); this is also due to the important technical issues still to be overcome. In addition, according to recent insights, CSCs do not constitute an autonomous compartment; rather, they play an active role in the microsystem, constituted both by the epithelial and the stromal compartments; indeed several authors have demonstrated the mutual influences between CSCs and their microenvironment (71–74).
We think that one promising approach to eradicate CSCs may be to target the EMT (75): inhibitors of TGFβ-induced EMT as well as SRC, MEK, or ALK5 inhibitors have been tested (76, 77). Interestingly, also inflammatory cytokines—IL6 and IL8 in particular—may represent potential therapeutic targets of EMT: IL-6 acts as a direct regulator of breast CSCs (BCSCs) self-renewal (78) and high levels of IL-6 are demonstrated to be associated to poor clinical outcome (79); on the other hand, BCSCs have been successfully eradicated both in vitro and in animal models by blocking the IL-8 receptor CXCR1 (80). In addition, in patients with HER2 positive breast cancer, treatment with HER2 inhibitors decreased the content of BCSCs (81), suggesting that combination therapies that include HER2 targeting agents may overcome BCSCs resistance. Based on this knowledge, we believe that therapies targeting BCSCs represent an urgent need to prevent recurrence. Other authors have suggested to target also Notch, Hedgehog, Wnt and PI3K/Akt/mTOR pathways (82). Intriguingly recent evidences demonstrate that CSCs rely on mitochondrial biogenesis for their propagation (83). Lamb et al. previously demonstrated that the antibiotic doxycycline, in a known inhibitor of the 28S mitochondrial ribosome subunit, inhibits CSC propagation in vitro (84). In 2018 we performed a pilot clinical trial and demonstrated that doxycycline treatment decreases the expression of CSC markers in breast cancer tumor samples (85). We thus propose that selected antibiotics, in monotherapy or in combination, may be further studied as interesting drugs for the eradication of CSCs.
From now on, this review concentrates on specific issues concerning cancer cell plasticity in breast cancer, glioblastoma, and melanoma, which represent our expertise and, in our opinion, the most challenging models in this field. A detailed table is then provided reporting the latest knowledge in other tumor models.
CSC Plasticity in Breast Cancer
Breast cancer has been largely investigated in terms of its etiology (86–89) and still little is known on the mechanisms of its progression. Breast cancer cells commonly gain genetic and epigenetic modifications in their genome (90), contributing to its characteristic intra-tumor heterogeneity (91–96). Intra-tumor heterogeneity is strongly influenced by numerous factors from the tumor microenvironment: breast cancer cells are indeed under continuous selective pressure due to attacks by the immune system or administered therapies (97, 98). This supports breast cancer progression, conferring a competitive advantage to specific subclones (92).
In recent decades, a hierarchical organization has been proposed, where cancer cells with self-renew capacity, the so-called BCSCs, are postulated to be at the top of the tumor pyramid. Al-Hajj et al. in 2003 first isolated a population of BCSCs expressing high levels of CD44 and low levels of CD24 (CD44+CD24−/low) and capable to form tumors when injected into immune deficient mice (99). Since then, numerous studies have tested other biomarkers to sort BCSCs: among all, aldehyde dehydrogenase 1 (ALDH1) resulted to be a potentially useful alternative or complement to the CD44+CD24−/low phenotype, particularly in high grade and HER2 positive tumors (100). BCSCs not only possess high tumorigenic properties but represent the cells that mediate tumor metastasis. Indeed, the CD44+/CD24−/low phenotype is highly expressed in triple negative breast cancers (101, 102) and is associated to poor overall survival (103, 104); moreover, it has been reported among cancer cells spread into the bone marrow (105) or to the lung (106) of patients with breast cancer. At present, BCSCs are believed to enter the circulation and become CTCs: indeed, high expression levels of BCSC markers have been found in CTCs (107). Thanks to their capacity of anoikis resistance, CTCs with BCSC phenotype have the potential to seed metastatic lesions (108). Studies from liquid biopsy samples demonstrate that CTCs with a BCSC phenotype are enriched in the group with clinical disease progression (107).
A large number of studies also suggest that BCSCs display resistance to traditional cancer therapies (109–116). Cytotoxic chemotherapies target the bulk of the tumor composed of highly proliferative breast cancer cells and does not affect BCSCs that, over time, cause tumor relapse (81). In addition, genetic alterations may confer to BCSCs intrinsic chemoresistance, including modifications in proteins involved in the detoxification of chemotherapy agents (117). As reported above, BCSCs express high levels of ALDH1, that metabolizes cyclophosphamide, thus minimize its toxic effects (101). Also, tumor microenvironment plays a crucial role in BCSC chemoresistance: in hypoxic conditions, activation of hypoxia induced factors not only promotes the formation of new blood vessel but also a BCSCs quiescent phenotype (118, 119).
CSC Plasticity in Glioblastoma
Glioblastoma (GBM) is the most frequent and deadly glial tumor (120); it is morphologically (121) and molecularly (97, 122, 123) characterized by high intra- and inter- tumor heterogeneity, which may play a pivotal role in recurrence and therapy resistance (124, 125).
The Cancer Genome Atlas has identified four GBM molecular subtypes: proneural, neural, classical, and mesenchymal (126). However, it has been demonstrated how multiple molecular subtypes may co-exist in the same tumor mass (122) or how GBM presents hybrid states with the expression of a peculiar signature overlapping two molecular subtypes (127). The establishment and the constant evolution of this heterogeneity equilibrium are due to glioma stem cells (GSCs) (128) and can be influenced by cytotoxic therapies and other endogenous factors (129, 130). However, how GSC heterogeneity is determined still remains unclear; in-vitro studies have shown that GSCs preserve their capability for recapitulating their primary heterogeneity also after many cell divisions, and temozolomide (TMZ) does not influence this capacity (131, 132); though, the same cytotoxic drug is able to drive GSCs heterogeneity and further drug resistance (133).
GSCs' isolation and characterization are based on stem markers expression; therefore, their choice is fundamental. One of the first discovered marker was CD133 (134); however, its expression is highly variable (~20–60%) (135), and also CD133– cells have a clonogenic potential. Indeed, Chen et al. (136) divided GSCs into three subtypes based on malignant potential (MP): type 1 (high MP) and type 3 (mild MP) were CD133−; whereas, type 2 GSCs (moderate MP) were CD133+. An additional marker is CD15, which is more frequently expressed in GBM than CD133; CD15+ GSCs are more clonogenic, proliferative and tumorigenic (137). CD44 represents another reliable marker: indeed, CD44+ GSCs present high tumor-sphere forming and tumorigenic potential, and have the capability to restore the heterogeneity of the parental GBM (138). Furthermore, ALDH1A3+ GSCs, besides having the above mentioned features, express other stem cell markers, such as musashi and nestin, and are able to differentiate into several neural lineages (139, 140), and promote TMZ resistance (141).
Nevertheless, a clear-cut segregation of GBM cells between CSCs and non-CSCs is not possible yet; instead, it is more conceivable the ability of GBM cells to transit among states or the acquisition of intermediate or metastable cellular state, exhibiting a wide and continuous range of CSC signature (142, 143).
CSC Plasticity in Melanoma
Melanoma represents a significant challenge, with low curative rates (<10%) and poor prognosis (median survival: 6–9 months) in the metastatic stage (144–146). Aggressive melanoma has revealed to co-express specific genes and proteins of multiple cellular types, including embryonic stem cells and endothelial cells, underlying cell plasticity.
3D in vitro models demonstrated that melanoma cells are able to form perfusable, vasculogenic-like channels, a biological phenomenon called vasculogenic mimicry (VM) (147). The treatment with endostatin has proved no effect on the inhibition of melanoma VM (148), thus portraying aggressive melanoma as being able to survive by its own perfusion network (149).
On the other hand, a large number of molecular studies jointly revealed a strong stem signature in aggressive melanoma, with still unknown practical significance (150–152). In particular, Nodal, a signaling pathway active in embryonic development, was notably upregulated in more aggressive melanoma (153). The nodal family of proteins, are a subset of the TGFβ superfamily and cooperate to the pluripotency of human embryonic stem cells (154). This observation led researchers to recognize a commonality in the phenotype of aggressive melanoma, linking vascular, embryonic and cancer stem cell properties.
CSC Plasticity in Other Solid Tumors
Several authors have demonstrated how it is possible to isolate CSCs in most solid malignancies. However, several aspects and molecular features regarding cell stemness still remain uncovered; this means that even if most markers across different cancer are the same (Table 1), a common and reliable signature is still lacking, due to technical issues mostly. Nevertheless, in our opinion, a change in clinical trials approach may be of help to overcome this limitation. Indeed, the implementation of biobanks of fresh tissues and biological fluids may represent a precious source for the next future when new techniques and novel approaches will be introduced.
Conclusions and Future Directions
Future research studies will be needed in order to improve our understanding of the complex phenomenon of cancer cell plasticity. The recent insights on the role of plasticity in cancer progression and relapse highlights the need to develop new and combinatorial therapies, that aim to: (i) inhibit specific cell markers; (ii) interfere with stemness and EMT signaling pathways; (iii) affect also components of the tumor microenvironment.
Author Contributions
GF and AN wrote the paper. CS conceived the idea, supervised, and edited the manuscript. All authors discussed and commented on the manuscript.
Conflict of Interest
The authors declare that the research was conducted in the absence of any commercial or financial relationships that could be construed as a potential conflict of interest.
References
1. Meacham CE, Morrison SJ. Tumour heterogeneity and cancer cell plasticity. Nature. (2013) 501:328–37. doi: 10.1038/nature12624
2. Bedard PL, Hansen AR, Ratain MJ, Siu LL. Tumour heterogeneity in the clinic. Nature. (2013) 501:355–64. doi: 10.1038/nature12627
3. Nowell P. The clonal evolution of tumor cell populations. Science. (1976) 194:23–8. doi: 10.1126/science.959840
4. Baylin SB, Jones PA. A decade of exploring the cancer epigenome — biological and translational implications. Nat Rev Cancer. (2011) 11:726–34. doi: 10.1038/nrc3130
5. Easwaran H, Tsai H-C, Baylin Stephen B. Cancer epigenetics: tumor heterogeneity, plasticity of stem-like states, and drug resistance. Mol. Cell. (2014) 54:716–27. doi: 10.1016/j.molcel.2014.05.015
6. Xin H, Kong Y, Jiang X, Wang K, Qin X, Miao Z-H, et al. Multiz-drug–resistant cells enriched from chronic myeloid leukemia cells by doxorubicin possess tumor-initiating–cell properties. J Pharm Sci. (2013) 122:299–304. doi: 10.1254/jphs.13025FP
7. Duggal R, Minev B, Vescovi A, Szalay A. Cancer Stem Cell Models and Role in Drug Discovery. Stem Cells and Human Diseases. Dordrecht: Springer. (2011) p. 217–28. doi: 10.1007/978-94-007-2801-1_10
8. Mani SA, Guo W, Liao M-J, Eaton EN, Ayyanan A, Zhou AY, et al. The epithelial-mesenchymal transition generates cells with properties of stem cells. Cell. (2008) 133:704–15. doi: 10.1016/j.cell.2008.03.027
9. Morel A-P, Lièvre M, Thomas C, Hinkal G, Ansieau S, Puisieux A. Generation of breast cancer stem cells through epithelial-mesenchymal transition. PLoS ONE. (2008) 3:e2888. doi: 10.1371/journal.pone.0002888
10. Wellner U, Schubert J, Burk UC, Schmalhofer O, Zhu F, Sonntag A, et al. The EMT-activator ZEB1 promotes tumorigenicity by repressing stemness-inhibiting microRNAs. Nat Cell Biol. (2009) 11:1487–95. doi: 10.1038/ncb1998
11. Gupta PB, Chaffer CL, Weinberg RA. Cancer stem cells: mirage or reality? Nat Med. (2009) 15:1010–2. doi: 10.1038/nm0909-1010
12. Liu S, Cong Y, Wang D, Sun Y, Deng L, Liu Y, et al. Breast cancer stem cells transition between epithelial and mesenchymal states reflective of their normal counterparts. Stem Cell Rep. (2014) 2:78–91. doi: 10.1016/j.stemcr.2013.11.009
13. Beerling E, Seinstra D, de Wit E, Kester L, van der Velden D, Maynard C, et al. Plasticity between epithelial and mesenchymal states unlinks EMT from metastasis-enhancing stem cell capacity. Cell Rep. (2016) 14:2281–8. doi: 10.1016/j.celrep.2016.02.034
14. Ye X, Brabletz T, Kang Y, Longmore GD, Nieto MA, Stanger BZ, et al. Upholding a role for EMT in breast cancer metastasis. Nature. (2017) 547:E1–3. doi: 10.1038/nature22816
15. Scheel C, Weinberg RA. Cancer stem cells and epithelial–mesenchymal transition: concepts and molecular links. Semin Cancer Biol. (2012) 22:396–403. doi: 10.1016/j.semcancer.2012.04.001
16. La Ferla M, Lessi F, Aretini P, Pellegrini D, Franceschi S, Tantillo E, et al. ANKRD44 gene silencing: a putative role in trastuzumab resistance in Her2-like breast cancer. Front Oncol. (2019) 9:547. doi: 10.3389/fonc.2019.00547
17. Grosse-Wilde A, Fouquier d'Hérouël A, McIntosh E, Ertaylan G, Skupin A, Kuestner RE, et al. Stemness of the hybrid epithelial/mesenchymal state in breast cancer and its association with poor survival. PLoS ONE. (2015) 10:e0126522. doi: 10.1371/journal.pone.0126522
18. Ruscetti M, Quach B, Dadashian EL, Mulholland DJ, Wu H. Tracking and functional characterization of epithelial-mesenchymal transition and mesenchymal tumor cells during prostate cancer metastasis. Cancer Res. (2015) 75:2749–59. doi: 10.1158/0008-5472.CAN-14-3476
19. Pastushenko I, Brisebarre A, Sifrim A, Fioramonti M, Revenco T, Boumahdi S, et al. Identification of the tumour transition states occurring during EMT. Nature. (2018) 556:463–8. doi: 10.1038/s41586-018-0040-3
20. Rompolas P, Mesa KR, Greco V. Spatial organization within a niche as a determinant of stem-cell fate. Nature. (2013) 502:513–8. doi: 10.1038/nature12602
21. Merrell AJ, Stanger BZ. Adult cell plasticity in vivo: de-differentiation and transdifferentiation are back in style. Nat Rev Mol Cell Biol. (2016) 17:413–25. doi: 10.1038/nrm.2016.24
22. Ge Y, Fuchs E. Stretching the limits: from homeostasis to stem cell plasticity in wound healing and cancer. Nat Rev Genetics. (2018) 19:311–25. doi: 10.1038/nrg.2018.9
23. Siravegna G, Mussolin B, Buscarino M, Corti G, Cassingena A, Crisafulli G, et al. Clonal evolution and resistance to EGFR blockade in the blood of colorectal cancer patients. Nat Med. (2015) 795–801. doi: 10.1038/nm.3870
24. Diaz LA, Williams RT, Wu J, Kinde I, Hecht JR, Berlin J., et al. The molecular evolution of acquired resistance to targeted EGFR blockade in colorectal cancers. Nature. (2012) 486:537–40. doi: 10.1038/nature11219
25. Russo M, Siravegna G, Blaszkowsky LS, Corti G, Crisafulli G, Ahronian LG., et al. Tumor heterogeneity and lesion-specific response to targeted therapy in colorectal cancer. Cancer Discov. (2015) 6:147–53. doi: 10.1158/2159-8290.CD-15-1283
26. Murtaza M, Dawson SJ, Pogrebniak K, Rueda OM, Provenzano E, Grant J., et al. Multifocal clonal evolution characterized using circulating tumour DNA in a case of metastatic breast cancer. Nat Commun. (2015) 6:8760. doi: 10.1038/ncomms9760
27. Morelli MP, Overman MJ, Dasari A, Kazmi SM, Mazard T, Vilar E., et al. Characterizing the patterns of clonal selection in circulating tumor DNA from patients with colorectal cancer refractory to anti- EGFR treatment. Ann Oncol. (2015) 26:731–6. doi: 10.1093/annonc/mdv005
28. Indraccolo S, Lombardi G, Fassan M, Pasqualini L, Giunco S, Marcato R., et al. Genetic, epigenetic, and immunologic profiling of MMR-deficient relapsed glioblastoma. Clin Cancer Res. (2019) 25:1828–37. doi: 10.1158/1078-0432.CCR-18-1892
29. Salvianti F, Canu L, Poli G, Armignacco R, Scatena C, Cantini G, et al. New insights in the clinical and translational relevance of miR483-5p in adrenocortical cancer. Oncotarget. (2017) 8:65525–33. doi: 10.18632/oncotarget.19118
30. Fanelli GN, Gasparini P, Coati I, Cui R, Pakula H, Chowdhury B, et al. LONG-NONCODING RNAs in gastroesophageal cancers. Noncoding RNA Res. (2018) 3:195–212. doi: 10.1016/j.ncrna.2018.10.001
31. Del Re M, Bertolini I, Crucitta S, Fontanelli L, Rofi E, De Angelis C, et al. Overexpression of TK1 and CDK9 in plasma-derived exosomes is associated with clinical resistance to CDK4/6 inhibitors in metastatic breast cancer patients. Breast Cancer Res Treat. (2019) 178:57–62. doi: 10.1007/s10549-019-05365-y
32. Saraggi D, Galuppini F, Fanelli GN, Remo A, Urso EDL, Bao RQ, et al. MiR-21 up-regulation in ampullary adenocarcinoma and its pre-invasive lesions. Pathol Res Pract. (2018) 214:835–9. doi: 10.1016/j.prp.2018.04.018
33. Fassan M, Facchin S, Munari G, Fanelli GN, Lorenzon G, Savarino E. Noncoding RNAs as drivers of the phenotypic plasticity of oesophageal mucosa. World J Gastroenterol. (2017) 23:7653–6. doi: 10.3748/wjg.v23.i43.7653
34. Palmirotta R, Lovero D, Cafforio P, Felici C, Mannavola F, Pelle E, et al. Liquid biopsy of cancer: a multimodal diagnostic tool in clinical oncology. Ther Adv Med Oncol. (2018) doi: 10.1177/1758835918794630
35. Krebs MG, Metcalf RL, Carter L, Brady G, Blackhall FH, Dive C. Molecular analysis of circulating tumour cells — biology and biomarkers. Nat Rev Clin Oncol. (2014) 11:129–144. doi: 10.1038/nrclinonc.2013.253
36. Cristofanilli M, Budd GT, Ellis MJ, Stopeck A, Matera J, Miller MC., et al. Circulating tumor cells, disease progression, and survival in metastatic breast cancer. N Engl J Med. (2004) 351:781–91. doi: 10.1056/NEJMoa040766
37. Mazzini C, Pinzani P, Salvianti F, Scatena C, Paglierani M, Ucci F, et al. Circulating tumor cells detection and counting in uveal melanomas by a filtration-based method. Cancers. (2014) 6:323–32. doi: 10.3390/cancers6010323
38. Pinzani P, Scatena C, Salvianti F, Corsini E, Canu L, Poli G, et al. Detection of circulating tumor cells in patients with adrenocortical carcinoma: a monocentric preliminary study. J Clin Endocrinol Metab. (2013) 98:3731–8. doi: 10.1210/jc.2013-1396
39. Arya SK, Lim B, Rahman ARA. Enrichment, detection and clinical significance of circulating tumor cells. Lab Chip. (2013) 13:1995. doi: 10.1039/c3lc00009e
40. van der Toom EE, Verdone JE, Gorin MA, Pienta KJ. Technical challenges in the isolation and analysis of circulating tumor cells. Oncotarget. (2016) 7:62754–66. doi: 10.18632/oncotarget.11191
41. Alix-Panabières C, Pantel K. Challenges in circulating tumour cell research. Nat Rev Cancer. (2014) 14:623–31. doi: 10.1038/nrc3820
42. Schneck H, Gierke B, Uppenkamp F, Behrens B, Niederacher D, Stoecklein NH, et al. EpCAM-independent enrichment of circulating tumor cells in metastatic breast cancer. PLoS ONE. (2015) 10:e0144535. doi: 10.1371/journal.pone.0144535
43. Messaritakis I, Stoltidis D, Kotsakis A, Dermitzaki E-K, Koinis F, Lagoudaki E, et al. TTF-1- and/or CD56-positive circulating tumor cells in patients with small cell lung cancer (SCLC). Sci Rep. (2017) 7:45351. doi: 10.1038/srep45351
44. Beije N, Onstenk W, Kraan J, Sieuwerts AM, Hamberg P, Dirix LY, et al. Prognostic impact of HER2 and ER status of circulating tumor cells in metastatic breast cancer patients with a HER2-negative primary tumor. Neoplasia. (2016) 18:647–53. doi: 10.1016/j.neo.2016.08.007
45. Yadavalli S, Jayaram S, Manda SS, Madugundu AK, Nayakanti DS, Tan TZ, et al. Data-driven discovery of extravasation pathway in circulating tumor cells. Sci Rep. (2017) 7:43710. doi: 10.1038/srep43710
46. Correnti M, Raggi C. Stem-like plasticity and heterogeneity of circulating tumor cells: current status and prospect challenges in liver cancer. Oncotarget. (2016) 8:7094–115. doi: 10.18632/oncotarget.12569
47. Aktas B, Tewes M, Fehm T, Hauch S, Kimmig R, Kasimir-Bauer S. Stem cell and epithelial-mesenchymal transition markers are frequently overexpressed in circulating tumor cells of metastatic breast cancer patients. Breast Cancer Res. (2009) 11:R46. doi: 10.1186/bcr2333
48. Tam WL, Weinberg RA. The epigenetics of epithelial-mesenchymal plasticity in cancer. Nat Med. (2013) 19:1438–49. doi: 10.1038/nm.3336
49. Blassl C, Kuhlmann JD, Webers A, Wimberger P, Fehm T, Neubauer H. Gene expression profiling of single circulating tumor cells in ovarian cancer—establishment of a multi-marker gene panel. Mol Oncol. (2016) 10:1030–42. doi: 10.1016/j.molonc.2016.04.002
50. Powell AA, Talasaz AH, Zhang H, Coram MA, Reddy A, Deng G., et al. Single cell profiling of circulating tumor cells: transcriptional heterogeneity and diversity from breast cancer cell lines. PLoS ONE. (2012) 7: e33788. doi: 10.1371/journal.pone.0033788
51. Miyamoto DT, Lee RJ, Kalinich M, LiCausi JA, Zheng Y, Chen T., et al. An RNA-based digital circulating tumor cell signature is predictive of drug response and early dissemination in prostate cancer. Cancer Discov. (2018) 8:288–303. doi: 10.1158/2159-8290.CD-16-1406
52. Gorges TM, Kuske A, Röck K, Mauermann O, Müller V, Peine S., et al. Accession of tumor heterogeneity by multiplex transcriptome profiling of single circulating tumor cells. Clin Chem. (2016) 62:1504–1515. doi: 10.1373/clinchem.2016.260299
53. LoPiccolo J, Blumenthal GM, Bernstein WB, Dennis PA. Targeting the PI3K/Akt/mTOR pathway: effective combinations and clinical considerations. Drug Resist Updat. (2008) 11:32–50. doi: 10.1016/j.drup.2007.11.003
54. Yeung TM, Gandhi SC, Wilding JL, Muschel R, Bodmer WF. Cancer stem cells from colorectal cancer-derived cell lines. Proc Natl Acad Sci USA. (2010) 107:3722–7. doi: 10.1073/pnas.0915135107
55. Alix-Panabières C, Pantel K. Clinical applications of circulating tumor cells and circulating tumor dna as liquid biopsy. Cancer Discovery. (2016) 6:479–91. doi: 10.1158/2159-8290.CD-15-1483
56. Lin M, Chen J-F, Lu Y-T, Zhang Y, Song J, Hou S, et al. Nanostructure embedded microchips for detection, isolation, and characterization of circulating tumor cells. Acc Chem Res. (2014) 47:2941–50. doi: 10.1021/ar5001617
57. Ming Y, Li Y, Xing H, Luo M, Li Z, Chen J, et al. Circulating tumor cells: from theory to nanotechnology-based detection. Front Pharmacol. (2017) 8:35. doi: 10.3389/fphar.2017.00035
58. Abonnenc M, Manaresi N, Borgatti M, Medoro G, Fabbri E, Romani A, et al. Programmable interactions of functionalized single bioparticles in a dielectrophoresis-based microarray chip. Anal Chem. (2013) 85:8219–24. doi: 10.1021/ac401296m
59. Ting DT, Wittner BS, Ligorio M, Vincent Jordan N, Shah AM, Miyamoto DT., et al. Single-cell RNA sequencing identifies extracellular matrix gene expression by pancreatic circulating tumor cells. Cell Rep. (2014) 8:1905–18. doi: 10.1016/j.celrep.2014.08.029
60. Giuliano M, Shaikh A, Lo HC, Arpino G, De Placido S, Zhang XH, et al. Perspective on circulating tumor cell clusters: why it takes a village to metastasize. Cancer Res. (2018) 78:845–52. doi: 10.1158/0008-5472.CAN-17-2748
61. Li L, Cole J, Margolin DA. Cancer stem cell and stromal microenvironment. Ochsner J. (2013) 13:109–18. doi: 10.1002/9781118356203.ch21
62. Cazet AS, Hui MN, Elsworth BL, Wu SZ, Roden D, Chan CL, et al. Targeting stromal remodeling and cancer stem cell plasticity overcomes chemoresistance in triple negative breast cancer. Nat Commun. (2018) 9:2897. doi: 10.1038/s41467-018-05220-6
63. Park TS, Donnenberg VS, Donnenberg AD, Zambidis ET, Zimmerlin L. Dynamic interactions between cancer stem cells and their stromal partners. Curr Pathobiol Rep. (2014) 2:41–52. doi: 10.1007/s40139-013-0036-5
64. Krause S, Maffini MV, Soto AM, Sonnenschein C. A novel 3D in vitro culture model to study stromal-epithelial interactions in the mammary gland. Tissue Eng Part C Methods. (2008) 14:261–71. doi: 10.1089/ten.tec.2008.0030
65. Fang X, Sittadjody S, Gyabaah K, Opara EC, Balaji KC. Novel 3D co-culture model for epithelial-stromal cells interaction in prostate cancer. PLoS ONE. (2013) 8:e75187. doi: 10.1371/journal.pone.0075187
66. Lang SH, Stower M, Maitland NJ. In vitro modelling of epithelial and stromal interactions in non-malignant and malignant prostates. Br J Cancer. (2000) 82:990–7. doi: 10.1054/bjoc.1999.1029
67. Ashworth TR. A case of cancer in which cells similar to those in the tumours where seen in the blood after death. Australian Med J. (1869) 14:146–7.
68. Krebs MG, Hou JM, Ward TH, Blackhall FH, Dive C. Circulating tumour cells: their utility in cancer management and predicting outcomes. Ther Adv Med Oncol. (2010) 2:351–65. doi: 10.1177/1758834010378414
69. Sprenger T, Conradi LC, Beissbarth T, Ermert H, Homayounfar K, Middel P, et al. Enrichment of CD133-expressing cells in rectal cancers treated with preoperative radiochemotherapy is an independent marker for metastasis and survival. Cancer. (2013) 119:26–35. doi: 10.1002/cncr.27703
70. Lin S, Xu Y, Gan Z, Han K, Hu H, Yao Y, et al. Monitoring cancer stem cells: insights into clinical oncology. Onco Targets Ther. (2016) 9:731–40. doi: 10.2147/OTT.S96645
71. Alvarado AG, Thiagarajan PS, Mulkearns-Hubert EE, Silver DJ, Hale JS, Alban TJ, et al. Glioblastoma cancer stem cells evade innate immune suppression of self-renewal through reduced TLR4 expression. Cell Stem Cell. (2017) 20:450–61.e4. doi: 10.1016/j.stem.2016.12.001
72. Lathia JD, Mack SC, Mulkearns-Hubert EE, Valentim CL, Rich JN. Cancer stem cells in glioblastoma. Genes Dev. (2015) 29:1203–17. doi: 10.1101/gad.261982.115
73. Sundar SJ, Hsieh JK, Manjila S, Lathia JD, Sloan A. The role of cancer stem cells in glioblastoma. Neurosurg Focus. (2014) 37:E6. doi: 10.3171/2014.9.FOCUS14494
74. Turaga SM, Lathia JD. Adhering towards tumorigenicity: altered adhesion mechanisms in glioblastoma cancer stem cells. CNS Oncol. (2016) 5:251–9. doi: 10.2217/cns-2016-0015
75. Tsai JH, Yang J. Epithelial-mesenchymal plasticity in carcinoma metastasis. Genes Dev. (2013) 27:2192–206. doi: 10.1101/gad.225334.113
76. Reka AK, Kuick R, Kurapati H, Standiford TJ, Omenn GS, Keshamouni VG. Identifying inhibitors of epithelial-mesenchymal transition by connectivity map–based systems approach. J Thorac Oncol. (2011) 6:1784–92. doi: 10.1097/JTO.0b013e31822adfb0
77. Chua K-N, Sim W-J, Racine V, Lee S-Y, Goh BC, Thiery JP. A cell-based small molecule screening method for identifying inhibitors of epithelial-mesenchymal transition in carcinoma. PLoS ONE. (2012) 7:e33183. doi: 10.1371/journal.pone.0033183
78. Sansone P, Storci G, Tavolari S, Guarnieri T, Giovannini C, Taffurelli M, et al. IL-6 triggers malignant features in mammospheres from human ductal breast carcinoma and normal mammary gland. J Clin Invest. (2007) 117:3988–4002. doi: 10.1172/JCI32533
79. Korkaya H, Liu S, Wicha MS. Regulation of cancer stem cells by cytokine networks: attacking cancer's inflammatory roots. Clin Cancer Res. (2011) 17:6125–9. doi: 10.1158/1078-0432.CCR-10-2743
80. Ginestier C, Liu S, Diebel ME, Korkaya H, Luo M, Brown M, et al. CXCR1 blockade selectively targets human breast cancer stem cells in vitro and in xenografts. J Clin Invest. (2010) 120:485–97. doi: 10.1172/JCI39397
81. Li X, Lewis MT, Huang J, Gutierrez C, Osborne CK, Wu MF, et al. Intrinsic resistance of tumorigenic breast cancer cells to chemotherapy. J Natl Cancer Inst. (2008) 100:672–9. doi: 10.1093/jnci/djn123
82. Chiotaki R, Polioudaki H, Theodoropoulos PA. Cancer stem cells in solid and liquid tissues of breast cancer patients: characterization and therapeutic perspectives. Curr Cancer Drug Targets. (2015) 15:256–69. doi: 10.2174/1568009615666150211102503
83. Peiris-Pagès M, Martinez-Outschoorn UE, Pestell RG, Sotgia F, Lisanti MP. Cancer stem cell metabolism. Breast Cancer Res. (2016) 18:55. doi: 10.1186/s13058-016-0712-6
84. Lamb R, Ozsvari B, Lisanti CL, Tanowitz HB, Howell A, Martinez-Outschoorn UE, et al. Antibiotics that target mitochondria effectively eradicate cancer stem cells, across multiple tumor types: treating cancer like an infectious disease. Oncotarget. (2015) 6:4569–84. doi: 10.18632/oncotarget.3174
85. Scatena C, Roncella M, Di Paolo A, Aretini P, Menicagli M, Fanelli G, et al. Doxycycline, an inhibitor of mitochondrial biogenesis, effectively reduces cancer stem cells (CSCs) in early breast cancer patients: a clinical pilot study. Front Oncol. (2018) 8:452. doi: 10.3389/fonc.2018.00452
86. Fernandez SV, Russo J. Estrogen and xenoestrogens in breast cancer. Toxicol Pathol. (2009) 38:110–22. doi: 10.1177/0192623309354108
87. Naccarato AG, Lessi F, Zavaglia K, Scatena C, Hamad MAA, Aretini P, et al. Mouse mammary tumor virus (MMTV) - like exogenous sequences are associated with sporadic but not hereditary human breast carcinoma. Aging. (2019) 11:7236–41. doi: 10.18632/aging.102252
88. Mazzanti CM, Lessi F, Armogida I, Zavaglia K, Franceschi S, Hamad MAL, et al. Human saliva as route of inter-human infection for mouse mammary tumor virus. Oncotarget. (2015) 6:18355–63. doi: 10.18632/oncotarget.4567
89. Mazzanti CM, Al Hamad M, Fanelli G, Scatena C, Zammarchi F, Zavaglia K, et al. A mouse mammary tumor virus env-like exogenous sequence is strictly related to progression of human sporadic breast carcinoma. Am J Pathol. (2011) 179:2083–90. doi: 10.1016/j.ajpath.2011.06.046
90. Vogelstein B, Papadopoulos N, Velculescu VE, Zhou S, Diaz LA, Kinzler KW. Cancer genome landscapes. Science. (2013) 339:1546–58. doi: 10.1126/science.1235122
91. Marjanovic ND, Weinberg RA, Chaffer CL. Cell plasticity and heterogeneity in cancer. Clin Chem. (2012) 59:168–79. doi: 10.1373/clinchem.2012.184655
92. Prasetyanti PR, Medema JP. Intra-tumor heterogeneity from a cancer stem cell perspective. Mol Cancer. (2017) 16:41. doi: 10.1186/s12943-017-0600-4
93. Medema JP, Vermeulen L. Microenvironmental regulation of stem cells in intestinal homeostasis and cancer. Nature. (2011) 474:318–26. doi: 10.1038/nature10212
94. Junttila MR, de Sauvage FJ. Influence of tumour micro-environment heterogeneity on therapeutic response. Nature. (2013) 501:346–54. doi: 10.1038/nature12626
95. Lu P, Weaver VM, Werb Z. The extracellular matrix: a dynamic niche in cancer progression. J Cell Biol. (2012) 196:395–406. doi: 10.1083/jcb.201102147
96. Lessi F, Scatena C, Aretini P, Menicagli M, Franceschi S, Naccarato AG, et al. Molecular profiling of microinvasive breast cancer microenvironment progression. J Transl Med. (2019) 17:187. doi: 10.1186/s12967-019-1936-x
97. McGranahan N, Swanton C. Clonal heterogeneity and tumor evolution: past, present, and the future. Cell. (2017) 168:613–28. doi: 10.1016/j.cell.2017.01.018
98. Colak S, Medema JP. Cancer stem cells - important players in tumor therapy resistance. FEBS J. (2014) 281:4779–91. doi: 10.1111/febs.13023
99. Al-Hajj M, Wicha MS, Benito-Hernandez A, Morrison SJ, Clarke MF. Prospective identification of tumorigenic breast cancer cells. Proc Natl Acad Sci USA. (2003) 100:3983–8. doi: 10.1073/pnas.0530291100
100. Ricardo S, Vieira AF, Gerhard R, Leitao D, Pinto R, Cameselle-Teijeiro JF, et al. Breast cancer stem cell markers CD44, CD24 and ALDH1: expression distribution within intrinsic molecular subtype. J Clin Pathol. (2011) 64:937–46. doi: 10.1136/jcp.2011.090456
101. Croker AK, Allan AL. Inhibition of aldehyde dehydrogenase (ALDH) activity reduces chemotherapy and radiation resistance of stem-like ALDHhiCD44+ human breast cancer cells. Breast Cancer Res Treat. (2011) 133:75–87. doi: 10.1007/s10549-011-1692-y
102. Croker AK, Goodale D, Chu J, Postenka C, Hedley BD, Hess DA, et al. High aldehyde dehydrogenase and expression of cancer stem cell markers selects for breast cancer cells with enhanced malignant and metastatic ability. J Cell Mol Med. (2008) 13:2236–52. doi: 10.1111/j.1582-4934.2008.00455.x
103. Liu R, Wang X, Chen GY, Dalerba P, Gurney A, Hoey T, et al. The prognostic role of a gene signature from tumorigenic breast-cancer cells. N Engl J Med. (2007) 356:217–26. doi: 10.1056/NEJMoa063994
104. Honeth G, Bendahl P-O, Ringnér M, Saal LH, Gruvberger-Saal SK, Lövgren K, et al. The CD44+/CD24-phenotype is enriched in basal-like breast tumors. Breast Cancer Res. (2008) 10:R53. doi: 10.1186/bcr2108
105. Balic M, Lin H, Young L, Hawes D, Giuliano A, McNamara G, et al. Most early disseminated cancer cells detected in bone marrow of breast cancer patients have a putative breast cancer stem cell phenotype. Clin Cancer Res. (2006) 12:5615–21. doi: 10.1158/1078-0432.CCR-06-0169
106. Liu H, Patel MR, Prescher JA, Patsialou A, Qian D, Lin J, et al. Cancer stem cells from human breast tumors are involved in spontaneous metastases in orthotopic mouse models. Proc Natl Acad Sci USA. (2010) 107:18115–20. doi: 10.1073/pnas.1006732107
107. Baccelli I, Schneeweiss A, Riethdorf S, Stenzinger A, Schillert A, Vogel V., et al. Identification of a population of blood circulating tumor cells from breast cancer patients that initiates metastasis in a xenograft assay. Nat Biotechnol. (2013) 31:539–44. doi: 10.1038/nbt.2576
108. Batlle E, Clevers H. Cancer stem cells revisited. Nat Med. (2017) 23:1124–34. doi: 10.1038/nm.4409
109. Phillips HS, Kharbanda S, Chen R, Forrest WF, Soriano RH, Wu TD, et al. Molecular subclasses of high-grade glioma predict prognosis, delineate a pattern of disease progression, and resemble stages in neurogenesis. Cancer Cell. (2006) 9:157–73. doi: 10.1016/j.ccr.2006.02.019
110. Lagadec C, Vlashi E, Della Donna L, Meng Y, Dekmezian C, Kim K, et al. Survival and self-renewing capacity of breast cancer initiating cells during fractionated radiation treatment. Breast Cancer Res. (2010) 12:R13. doi: 10.1186/bcr2479
111. Karimi-Busheri F, Rasouli-Nia A, Mackey JR, Weinfeld M. Senescence evasion by MCF-7 human breast tumor-initiating cells. Breast Cancer Res. (2010) 12:R31. doi: 10.1186/bcr2583
112. Fillmore CM, Kuperwasser C. Human breast cancer cell lines contain stem-like cells that self-renew, give rise to phenotypically diverse progeny and survive chemotherapy. Breast Cancer Res. (2008) 10:R25. doi: 10.1186/bcr1982
113. Shafee N, Smith CR, Wei S, Kim Y, Mills GB, Hortobagyi GN, et al. Cancer stem cells contribute to cisplatin resistance in Brca1/p53-mediated mouse mammary tumors. Cancer Res. (2008) 68:3243–50. doi: 10.1158/0008-5472.CAN-07-5480
114. Diehn M, Cho RW, Lobo NA, Kalisky T, Dorie MJ, Kulp AN, et al. Association of reactive oxygen species levels and radioresistance in cancer stem cells. Nature. (2009) 458:780–3. doi: 10.1038/nature07733
115. Yu F, Yao H, Zhu P, Zhang X, Pan Q, Gong C, et al. let-7 regulates self-renewal and tumorigenicity of breast cancer cells. Cell. (2007) 131:1109–23. doi: 10.1016/j.cell.2007.10.054
116. Zielske SP, Spalding AC, Wicha MS, Lawrence TS. Ablation of breast cancer stem cells with radiation. Transl Oncol. (2011) 4:227–33. doi: 10.1593/tlo.10247
117. Hirschmann-Jax C, Foster AE, Wulf GG, Nuchtern JG, Jax TW, Gobel U, et al. A distinct “side population” of cells with high drug efflux capacity in human tumor cells. Proc Natl Acad Sci USA. (2004) 101:14228–33. doi: 10.1073/pnas.0400067101
118. Crowder SW, Balikov DA, Hwang Y-S, Sung H-J. Cancer stem cells under hypoxia as a chemoresistance factor in the breast and brain. Curr Pathobiol Rep. (2014) 2:33–40. doi: 10.1007/s40139-013-0035-6
119. Mimeault M, Batra SK. Hypoxia-inducing factors as master regulators of stemness properties and altered metabolism of cancer- and metastasis-initiating cells. J Cell Mol Med. (2013) 17:30–54. doi: 10.1111/jcmm.12004
120. Kirkpatrick JP, Laack NN, Shih HA, Gondi V. Management of GBM: a problem of local recurrence. J Neuro Oncol. (2017) 134:487–93. doi: 10.1007/s11060-016-2347-y
121. Jung CS, Unterberg AW, Hartmann C. Diagnostic markers for glioblastoma. Histol Histopathol. (2011) 26:1327–41. doi: 10.14670/HH-26.1327
122. Sottoriva A, Spiteri I, Piccirillo SGM, Touloumis A, Collins VP, Marioni JC, et al. Intratumor heterogeneity in human glioblastoma reflects cancer evolutionary dynamics. Proc Natl Acad Sci USA. (2013) 110:4009–14. doi: 10.1073/pnas.1219747110
123. Turtoi A, Musmeci D, Naccarato AG, Scatena C, Ortenzi V, Kiss R, et al. Sparc-like protein 1 is a new marker of human glioma progression. J Proteome Res. (2012) 11:5011–21. doi: 10.1021/pr3005698
124. Franceschi S, Lessi F, Aretini P, Ortenzi V, Scatena C, Menicagli M, et al. Cancer astrocytes have a more conserved molecular status in long recurrence free survival (RFS) IDH1 wild-type glioblastoma patients: new emerging cancer players. Oncotarget. (2018) 9:24014–27. doi: 10.18632/oncotarget.25265
125. Franceschi S, Mazzanti CM, Lessi F, Aretini P, Carbone FG, La Ferla M, et al. Investigating molecular alterations to profile short- and long-term recurrence-free survival in patients with primary glioblastoma. Oncol Lett. (2015) 10:3599–606. doi: 10.3892/ol.2015.3738
126. Verhaak RG, Hoadley KA, Purdom E, Wang V, Qi Y, Wilkerson MD, et al. Integrated genomic analysis identifies clinically relevant subtypes of glioblastoma characterized by abnormalities in PDGFRA, IDH1, EGFR, and NF1. Cancer Cell. (2010) 17:98–110. doi: 10.1016/j.ccr.2009.12.020
127. Meyer M, Reimand J, Lan X, Head R, Zhu X, Kushida M, et al. Single cell-derived clonal analysis of human glioblastoma links functional and genomic heterogeneity. Proc Natl Acad Sci USA. (2015) 112:851–6. doi: 10.1073/pnas.1320611111
128. Singh SK, Clarke ID, Hide T, Dirks PB. Cancer stem cells in nervous system tumors. Oncogene. (2004) 23:7267–73. doi: 10.1038/sj.onc.1207946
129. Baysan M, Woolard K, Bozdag S, Riddick G, Kotliarova S, Cam MC, et al. Micro-environment causes reversible changes in DNA methylation and mRNA expression profiles in patient-derived glioma stem cells. PLoS ONE. (2014) 9:e94045. doi: 10.1371/journal.pone.0094045
130. Bhat Krishna PL, Balasubramaniyan V, Vaillant B, Ezhilarasan R, Hummelink K, Hollingsworth F, et al. Mesenchymal differentiation mediated by NF-κB promotes radiation resistance in glioblastoma. Cancer Cell. (2013) 24:331–46. doi: 10.1016/j.ccr.2013.08.001
131. Brown DV, Filiz G, Daniel PM, Hollande F, Dworkin S, Amiridis S, et al. Expression of CD133 and CD44 in glioblastoma stem cells correlates with cell proliferation, phenotype stability and intra-tumor heterogeneity. PLOS ONE. (2017) 12:e0172791. doi: 10.1371/journal.pone.0172791
132. Sugimori M, Hayakawa Y, Boman BM, Fields JZ, Awaji M, Kozano H, et al. Discovery of power-law growth in the self-renewal of heterogeneous glioma stem cell populations. PLoS ONE. (2015) 10:e0135760. doi: 10.1371/journal.pone.0135760
133. Welker AM, Jaros BD, An M, Beattie CE. Changes in tumor cell heterogeneity after chemotherapy treatment in a xenograft model of glioblastoma. Neuroscience. (2017) 356:35–43. doi: 10.1016/j.neuroscience.2017.05.010
134. Uchida N, Buck DW, He D, Reitsma MJ, Masek M, Phan TV, et al. Direct isolation of human central nervous system stem cells. Proc Natl Acad Sci USA. (2000) 97:14720–5. doi: 10.1073/pnas.97.26.14720
135. Holmberg Olausson K, Maire CL, Haidar S, Ling J, Learner E, Nistér M, et al. Prominin-1 (CD133) defines both stem and non-stem cell populations in CNS development and gliomas. PLoS ONE. (2014) 9:e106694. doi: 10.1371/journal.pone.0106694
136. Chen R, Nishimura MC, Bumbaca SM, Kharbanda S, Forrest WF, Kasman IM, et al. A hierarchy of self-renewing tumor-initiating cell types in glioblastoma. Cancer Cell. (2010) 17:362–75. doi: 10.1016/j.ccr.2009.12.049
137. Son MJ, Woolard K, Nam D-H, Lee J, Fine HA. SSEA-1 is an enrichment marker for tumor-initiating cells in human glioblastoma. Cell Stem Cell. (2009) 4:440–52. doi: 10.1016/j.stem.2009.03.003
138. Jijiwa M, Demir H, Gupta S, Leung C, Joshi K, Orozco N, et al. CD44v6 regulates growth of brain tumor stem cells partially through the AKT-mediated pathway. PLoS ONE. (2011) 6:e24217. doi: 10.1371/journal.pone.0024217
139. Choi SA, Lee JY, Phi JH, Wang K-C, Park C-K, Park S-H, et al. Identification of brain tumour initiating cells using the stem cell marker aldehyde dehydrogenase. Eur J Cancer. (2014) 50:137–49. doi: 10.1016/j.ejca.2013.09.004
140. Rasper M, Schafer A, Piontek G, Teufel J, Brockhoff G, Ringel F, et al. Aldehyde dehydrogenase 1 positive glioblastoma cells show brain tumor stem cell capacity. Neuro Oncol. (2010) 12:1024–33. doi: 10.1093/neuonc/noq070
141. Schafer A, Teufel J, Ringel F, Bettstetter M, Hoepner I, Rasper M, et al. Aldehyde dehydrogenase 1A1–a new mediator of resistance to temozolomide in glioblastoma. Neuro Oncol. (2012) 14:1452–64. doi: 10.1093/neuonc/nos270
142. Chambers I, Silva J, Colby D, Nichols J, Nijmeijer B, Robertson M, et al. Nanog safeguards pluripotency and mediates germline development. Nature. (2007) 450:1230–4. doi: 10.1038/nature06403
143. Hayashi K, Lopes SMCdS, Tang F, Surani MA. Dynamic equilibrium and heterogeneity of mouse pluripotent stem cells with distinct functional and epigenetic states. Cell Stem Cell. (2008) 3:391–401. doi: 10.1016/j.stem.2008.07.027
144. Song X, Zhao Z, Barber B, Farr AM, Ivanov B, Novich M. Overall survival in patients with metastatic melanoma. Curr Med Res Opin. (2015) 31:987–91. doi: 10.1185/03007995.2015.1021904
145. Falanga A, Marchetti M, Massi D, Merelli B, Verzeroli C, Russo L, et al. Thrombophilic status may predict prognosis in patients with metastatic BRAFV600-mutated melanoma who are receiving BRAF inhibitors. J Am Acad Dermatol. (2016) 74:1254–6.e4. doi: 10.1016/j.jaad.2015.11.006
146. Massi D, Simi L, Sensi E, Baroni G, Xue G, Scatena C, et al. Immunohistochemistry is highly sensitive and specific for the detection of NRASQ61R mutation in melanoma. Mod Pathol. (2014) 28:487–97. doi: 10.1038/modpathol.2014.137
147. Maniotis AJ, Folberg R, Hess A, Seftor EA, Gardner LMG, Pe'er J, et al. Vascular channel formation by human melanoma cells in vivo and in vitro: vasculogenic mimicry. Am J Pathol. (1999) 155:739–52. doi: 10.1016/S0002-9440(10)65173-5
148. van der Schaft DWJ, Seftor REB, Seftor EA, Hess AR, Gruman LM, Kirschmann DA, et al. Effects of angiogenesis inhibitors on vascular network formation by human endothelial and melanoma cells. JNCI J Natl Cancer Inst. (2004) 96:1473–7. doi: 10.1093/jnci/djh267
149. Hendrix MJC, Seftor EA, Hess AR, Seftor REB. Vasculogenic mimicry and tumour-cell plasticity: lessons from melanoma. Nat Rev Cancer. (2003) 3:411–21. doi: 10.1038/nrc1092
150. Bittner M, Meltzer P, Chen Y, Jiang Y, Seftor E, Hendrix M, et al. Molecular classification of cutaneous malignant melanoma by gene expression profiling. Nature. (2000) 406:536–40. doi: 10.1038/35020115
151. Carr KM, Bittner M, Trent JM. Gene-expression profiling in human cutaneous melanoma. Oncogene. (2003) 22:3076–80. doi: 10.1038/sj.onc.1206448
152. Demou ZN, Hendrix MJC. Microgenomics profile the endogenous angiogenic phenotype in subpopulations of aggressive melanoma. J Cell Biochem. (2008) 105:562–73. doi: 10.1002/jcb.21855
153. Topczewska JM, Postovit L-M, Margaryan NV, Sam A, Hess AR, Wheaton WW, et al. Embryonic and tumorigenic pathways converge via nodal signaling: role in melanoma aggressiveness. Nat Med. (2006) 12:925–32. doi: 10.1038/nm1448
154. Schier AF, Shen MM. Nodal signalling in vertebrate development. Nature. (2000) 403:385–9. doi: 10.1038/35000126
155. Sun S, Wang Z. Head neck squamous cell carcinoma c-Met(+) cells display cancer stem cell properties and are responsible for cisplatin-resistance and metastasis. Int J Cancer. (2011) 129:2337–48. doi: 10.1002/ijc.25927
156. Rothenberger N, Stabile L. Hepatocyte growth factor/c-met signaling in head and neck cancer and implications for treatment. Cancers. (2017) 9:39. doi: 10.3390/cancers9040039
157. Chen C, Wei Y, Hummel M, Hoffmann TK, Gross M, Kaufmann AM, et al. Evidence for epithelial-mesenchymal transition in cancer stem cells of head and neck squamous cell carcinoma. PLoS ONE. (2011) 6:e16466. doi: 10.1371/journal.pone.0016466
158. Chen D, Wu M, Li Y, Chang I, Yuan Q, Ekimyan-Salvo M, et al. Targeting BMI1 + cancer stem cells overcomes chemoresistance and inhibits metastases in squamous cell carcinoma. Cell Stem Cell. (2017) 20:621–34.e6. doi: 10.1016/j.stem.2017.02.003
159. Ricci-Vitiani L, Lombardi DG, Pilozzi E, Biffoni M, Todaro M, Peschle C, et al. Identification and expansion of human colon-cancer-initiating cells. Nature. (2006) 445:111–5. doi: 10.1038/nature05384
160. Wang T, Lv M, Shen S, Zhou S, Wang P, Chen Y., et al. Cell-free microRNA expression profiles in malignant effusion associated with patient survival in non-small cell lung cancer. PLoS ONE. (2012) 7:e43268. doi: 10.1371/journal.pone.0043268
161. Maheswaran S, Sequist LV, Nagrath S, Ulkus L, Brannigan B, Collura CV, et al. Detection of mutations inEGFRin circulating lung-cancer cells. N Engl J Med. (2008) 359:366–77. doi: 10.1056/NEJMoa0800668
162. Chiou SH, Wang ML, Chou YT, Chen CJ, Hong CF, Hsieh WJ, et al. Coexpression of oct4 and nanog enhances malignancy in lung adenocarcinoma by inducing cancer stem cell-like properties and epithelial-mesenchymal transdifferentiation. Cancer Res. (2010) 70:10433–44. doi: 10.1158/0008-5472.CAN-10-2638
163. Dhar M, Wong J, Che J, Matsumoto M, Grogan T, Elashoff D, et al. Evaluation of PD-L1 expression on vortex-isolated circulating tumor cells in metastatic lung cancer. Sci Rep. (2018) 8:2592. doi: 10.1038/s41598-018-19245-w
164. Harada K, Pool Pizzi M, Baba H, Shanbhag ND, Song S, Ajani JA. Cancer stem cells in esophageal cancer and response to therapy. Cancer. (2018) 124:3962–4. doi: 10.1002/cncr.31697
165. Bekaii-Saab T, El-Rayes B. Identifying and targeting cancer stem cells in the treatment of gastric cancer. Cancer. (2017) 123:1303–12. doi: 10.1002/cncr.30538
166. Yin S, Li J, Hu C, Chen X, Yao M, Yan M, et al. CD133 positive hepatocellular carcinoma cells possess high capacity for tumorigenicity. Int J Cancer. (2007) 120:1444–50. doi: 10.1002/ijc.22476
167. Zhu Z, Hao X, Yan M, Yao M, Ge C, Gu J., et al. Cancer stem/progenitor cells are highly enriched in CD133+CD44+population in hepatocellular carcinoma. Int J Cancer. (2009) 126:2067–78. doi: 10.1002/ijc.24868
168. Yang ZF, Ngai P, Ho DW, Yu WC, Ng MNP, Lau CK, et al. Identification of local and circulating cancer stem cells in human liver cancer. Hepatology. (2008) 47:919–28. doi: 10.1002/hep.22082
169. Yang ZF, Ho DW, Ng MN, Lau CK, Yu WC, Ngai P, et al. Significance of CD90+ cancer stem cells in human liver cancer. Cancer Cell. (2008) 13:153–66. doi: 10.1016/j.ccr.2008.01.013
170. Di Carlo C, Brandi J, Cecconi D. Pancreatic cancer stem cells: perspectives on potential therapeutic approaches of pancreatic ductal adenocarcinoma. World J Stem Cells. (2018) 10:172–82. doi: 10.4252/wjsc.v10.i11.172
171. Steinert G, Schölch S, Niemietz T, Iwata N, García SA, Behrens B., et al. Immune escape and survival mechanisms in circulating tumor cells of colorectal cancer. Cancer Res. (2014) 74:1694–704. doi: 10.1158/0008-5472.CAN-13-1885
172. Huang EH, Hynes MJ, Zhang T, Ginestier C, Dontu G, Appelman H, et al. Aldehyde dehydrogenase 1 is a marker for normal and malignant human colonic Stem Cells (SC) and tracks SC overpopulation during colon tumorigenesis. Cancer Res. (2009) 69:3382–9. doi: 10.1158/0008-5472.CAN-08-4418
173. Miyamoto DT. RNA-Seq of single prostate CTCs implicates noncanonical Wnt signaling in antiandrogen resistance. Science. (2015) 349:1351–6. doi: 10.1126/science.aab0917
174. Peng S, Maihle NJ, Huang Y. Pluripotency factors Lin28 and oct4 identify a sub-population of stem cell-like cells in ovarian cancer. Oncogene. (2010) 29:2153–9. doi: 10.1038/onc.2009.500
175. Meirelles K, Benedict LA, Dombkowski D, Pepin D, Preffer FI, Teixeira J, et al. Human ovarian cancer stem/progenitor cells are stimulated by doxorubicin but inhibited by Mullerian inhibiting substance. Proc Natl Acad Sci USA. (2012) 109:2358–63. doi: 10.1073/pnas.1120733109
176. Siu MKY, Wong ESY, Kong DSH, Chan HY, Jiang L, Wong OGW, et al. Stem cell transcription factor NANOG controls cell migration and invasion via dysregulation of E-cadherin and FoxJ1 and contributes to adverse clinical outcome in ovarian cancers. Oncogene. (2012) 32:3500–9. doi: 10.1038/onc.2012.363
177. Di J, Duiveman-de Boer T, Zusterzeel PLM, Figdor CG, Massuger LFAG, Torensma R. The stem cell markers oct4a, Nanog and c-Myc are expressed in ascites cells and tumor tissue of ovarian cancer patients. Cell Oncol. (2013) 36:363–74. doi: 10.1007/s13402-013-0142-8
178. Belotte J, Fletcher NM, Alexis M, Morris RT, Munkarah AR, Diamond MP, et al. Sox2 gene amplification significantly impacts overall survival in serous epithelial Ovarian cancer. Reprod Sci. (2014) 22:38–46. doi: 10.1177/1933719114542021
179. Yan HC, Xu JUN, Fang LS, Qiu YY, Lin XM, Huang HX, et al. Ectopic expression of the WWOX gene suppresses stemness of human ovarian cancer stem cells. Oncol Lett. (2015) 9:1614–20. doi: 10.3892/ol.2015.2971
Keywords: cancer plasticity, stem cell, heterogeneity, recurrence, liquid biopsy
Citation: Fanelli GN, Naccarato AG and Scatena C (2020) Recent Advances in Cancer Plasticity: Cellular Mechanisms, Surveillance Strategies, and Therapeutic Optimization. Front. Oncol. 10:569. doi: 10.3389/fonc.2020.00569
Received: 30 November 2019; Accepted: 30 March 2020;
Published: 22 April 2020.
Edited by:
Dong-Hua Yang, St. John's University, United StatesCopyright © 2020 Fanelli, Naccarato and Scatena. This is an open-access article distributed under the terms of the Creative Commons Attribution License (CC BY). The use, distribution or reproduction in other forums is permitted, provided the original author(s) and the copyright owner(s) are credited and that the original publication in this journal is cited, in accordance with accepted academic practice. No use, distribution or reproduction is permitted which does not comply with these terms.
*Correspondence: Cristian Scatena, Y3Jpc3RpYW4uc2NhdGVuYSYjeDAwMDQwO3VuaXBpLml0