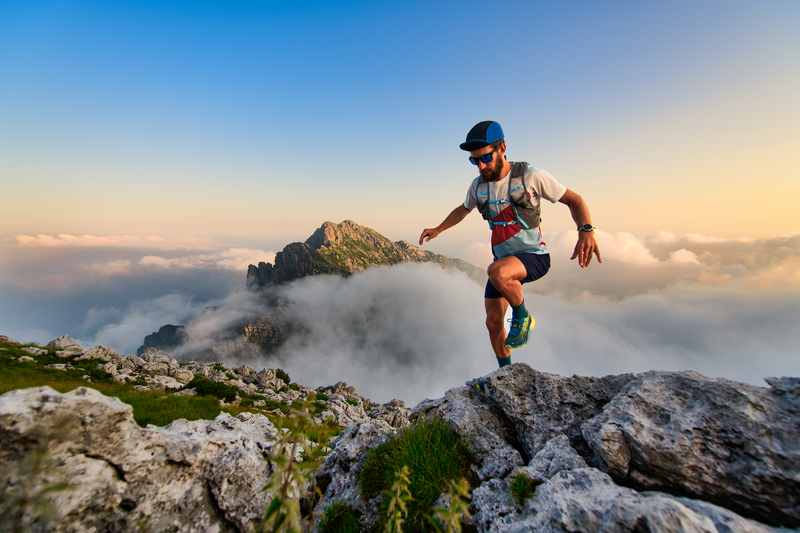
95% of researchers rate our articles as excellent or good
Learn more about the work of our research integrity team to safeguard the quality of each article we publish.
Find out more
REVIEW article
Front. Oncol. , 07 April 2020
Sec. Cancer Metabolism
Volume 10 - 2020 | https://doi.org/10.3389/fonc.2020.00499
This article is part of the Research Topic Role of Metabolic Remodeling in Cancer-Associated Epithelial-Mesenchymal Transition View all 8 articles
The epithelial-mesenchymal transition (EMT) represents a biological program during which epithelial cells lose their cell identity and acquire a mesenchymal phenotype. EMT is normally observed during organismal development, wound healing and tissue fibrosis. However, this process can be hijacked by cancer cells and is often associated with resistance to apoptosis, acquisition of tissue invasiveness, cancer stem cell characteristics, and cancer treatment resistance. It is becoming evident that EMT is a complex, multifactorial spectrum, often involving episodic, transient or partial events. Multiple factors have been causally implicated in EMT including transcription factors (e.g., SNAIL, TWIST, ZEB), epigenetic modifications, microRNAs (e.g., miR-200 family) and more recently, long non-coding RNAs. However, the relevance of metabolic pathways in EMT is only recently being recognized. Importantly, alterations in key metabolic pathways affect cancer development and progression. In this review, we report the roles of key EMT factors and describe their interactions and interconnectedness. We introduce metabolic pathways that are involved in EMT, including glycolysis, the TCA cycle, lipid and amino acid metabolism, and characterize the relationship between EMT factors and cancer metabolism. Finally, we present therapeutic opportunities involving EMT, with particular focus on cancer metabolic pathways.
In recent years, it has been progressively realized that cell identity is highly dynamic, as most notably demonstrated by Yamanaka et al., by reprogramming fully differentiated fibroblasts into induced pluripotent stem cells with the induction of four transcription factors (TFs) (1). Stem cells can renew themselves while maintaining their multipotency or can differentiate to a less potent cell type. During development and embryogenesis, a cascade of epigenetic and transcriptional programs is employed to ensure the differentiation of multipotent progenitor cells. Epithelial to mesenchymal transition (EMT) represents a cell biological program, during which epithelial cells progressively miss their cell identity and morphology and increasingly acquire mesenchymal characteristics (2, 3). The converse route is recognized as mesenchymal to epithelial transition (MET). EMT and MET are both mediated through a cascade of transcriptional and epigenetic changes and are physiologically observed during organismal development, tissue healing, homeostasis, as well as during fibrosis. However, the same processes can be hijacked by tumor cells during cancer development (4). Indeed, several analogies have been drawn between organismal development and tumorigenesis (5).
Epithelial cells exhibit cell-cell junctions, apico-basal polarity and limited migratory potential, and they can be identified using a multitude of cell surface markers, most notably E-cadherin, but also cytokeratins, occluding, and claudins (6, 7). In contrast, mesenchymal cells are characterized by front rear polarity and a migratory phenotype. In addition, during cancer development they display resistance to apoptosis, stem cell characteristics and tissue invasiveness (2, 8, 9). Mesenchymal cells can be identified by N-cadherin, Fibronectin and Vimentin cell surface markers (6). The EMT/MET model proposes that the migratory phenotype of cancer cells is acquired during EMT, enabling the invasion of other tissues, while MET potentiates the settlement of cancer cells at the new site (6).
Current work has demonstrated that EMT is not a binary state procedure, during which cells can either have a mesenchymal or an epithelial identity. Instead, EMT is a dynamic spectrum and reversible process and cells can be found at any locale on that spectrum, often sharing certain epithelial and mesenchymal characteristics (10–15). Most importantly, cells considered to be in a hybrid epithelial/mesenchymal state are more apoptosis-resistant and have higher tumor-initiating potential (15–17) Technological advances in CRISPR-Cas9 genome editing and decreasing costs in single cell sequencing have potentiated important breakthroughs in EMT. Firstly, single cell sequencing has revealed the extensive variability in gene expression and cell identity during EMT, both in development (18) and cancer (14, 19). Secondly, recent studies have demonstrated partial and transient EMT events with cells found across the continuum along the EMT spectrum (19–22). Thirdly, the variability and complexity in the expression patterns of multiple EMT and MET factors across disparate cell types and conditions is being appreciated and novel players in the EMT process are being discovered (14, 18, 19, 21, 22). CRISPR-Cas9 screens coupled with single cell RNA sequencing have identified novel EMT-associated factors and have provided evidence that specific signaling pathways control the EMT via discrete, regulatory checkpoints (21).
Cancer cells are known to adapt their metabolism to meet their high needs for energy and synthesis of biomolecules including proteins, lipids and nucleic acids (23, 24). Tumor cells are usually characterized by the Warburg effect, that is, the production of ATP mostly from glycolysis and not oxidative phosphorylation, even in conditions with high availability of oxygen (25). However, a multitude of key metabolic pathways are involved in the metabolic adaptations of cancer cells, with accumulating evidence for the importance of these pathways in EMT. Most notable among them are glycolysis, the TCA cycle, lipid and amino acid metabolism, which directly contribute to EMT, cancer cell survival, cancer invasiveness and metastasis. Although the regulation of these metabolic pathways was considered to be largely known, it seems that recent advances in our capacity to measure specific metabolites at the cell level and especially in the cancer state have shed new light on their modulation and intertwining with EMT transcriptional regulation (26, 27). As epithelial cancer cells acquire mesenchymal features during the EMT process, their metastatic potential increases. As a result, they should be able to penetrate the extracellular matrix, enter the blood stream and finally grow in a different tissue. All these steps require a continuous supply of nutrients to the cells that is provided through the blood stream and by metabolic reprogramming of the cells (28). More evidence is accumulating that this metabolic reprogramming is a highly regulated process by transcription factors that are known to be involved in EMT (29).
The requirement of EMT for metastasis may rely on the cancer type and there is ongoing contention regarding its role in metastasis, which may also be context-dependent and transient. There is substantial evidence supporting the notion that EMT is a driver during cancer metastasis in certain cancer types (30–37). Additionally, the induction of MET and the down-regulation of EMT TFs at the site of metastasis, supports the colonization of the metastatic cells (38–40). Metastasis accounts for an estimated 90% of cancer-associated deaths (41), reinforcing the importance of intervention at EMT. Various signaling molecules can activate the EMT process, including epidermal growth factor (EGF), fibroblast growth factor (FGF), hepatocyte growth factor (HGF), transforming growth factor β (TGFβ), β-catenin–dependent canonical and β-catenin–independent non-canonical WNT signaling, bone morphogenetic protein (BMP), Sonic Hedgehog (SHH) and the Notch signaling pathway, among others (12, 42–46). EMT transcription factors, epigenetic alterations, microRNAs, post-translational modifications, and metabolic reprogramming orchestrate the transition. In this review, we delve into each of them from a molecular and cellular viewpoint and summarize recent advances and changes in our understanding.
EMT-TFs represent master TFs that coordinate the EMT process. The most widely studied TFs among them are TWIST1, TWIST2, SNAIL1, SNAIL2, ZEB1, and ZEB2 (12), all of which directly inhibit the expression of E-cadherin and promote the transition to a mesenchymal state. The consequence of their expression is the suppression of the epithelial phenotype and the associated loss of epithelial cell surface biomarkers. A common feature among EMT-TFs is their physiological roles in embryogenesis and organismal development, as well as their reappearance in cancer cells during cancer development and progression. The expression of EMT-TFs can be overlapping and they can form networks, yet their functions are usually distinct. They are activated through signaling cascades and promote the transcriptional program switching. EMT-TFs have clinical relevance in metastasis and their expression correlates with poor clinical outcomes in cancer (6, 47, 48).
The SNAIL sub-family within the larger SNAG domain family of Zinc finger TFs in humans is composed of three members, namely SNAIL1, SNAIL2 (also known as SLUG), and SNAIL3, which act as transcriptional repressors (49). The number of SNAIL members varies by species and they are usually associated with mesoderm development and differentiation (50) and wound healing (51). SNAIL1 and SNAIL2 have important and widely studied roles in the EMT process, whereas SNAIL3 is a paralogue of SNAIL1 and SNAIL2 with distinct and divergent functions (52). SNAIL1 and SNAIL2 downregulate the expression of a number of target genes in relation to EMT, most notably E-cadherin, but also claudins, occludin, PALS1 and PATJ (53, 54). Both SNAIL1 and SNAIL2 bind directly to the E-cadherin promoter at E-box sequences to inhibit its expression (55). SNAIL1 interacts with chromatin remodeling factors to exert its repressor activity at the E-cadherin promoter (56). It also alters the polarity of epithelial cells by inhibiting the expression of Crumbs3, which is essential for epithelial morphogenesis (57). In addition to mediating the EMT process, SNAIL members promote cell survival, block the cell cycle and inhibit the apoptotic process, with roles in the induction of a metastatic phenotype and the acquisition of cancer stem cell features (8, 58). In support to that, circulating tumor cells from hepatocellular carcinomas express roughly 10 times more SNAIL1 mRNA (59), while transient SNAIL2 and SOX9 induction increases the metastatic ability of mammary gland cells (60).
A plethora of general and cell-type specific signals can activate SNAIL TFs. TGFβ1 induces SNAIL1 expression in a number of cell types including hepatocytes, palate, epithelial and mesothelial cells (61, 62). TGFβ2 induces SNAIL1 expression during hair follicle morphogenesis (63) and SNAIL2 expression during heart development (64). BMP4 induces SNAIL2 expression during neural crest development (65). Snail genes are up-regulated in multiple cancer types and they are associated with poor prognosis, including breast and ovarian cancers for SNAIL1 (66, 67) and colorectal cancers for SNAIL2 (68). In pancreatic and thyroid cancers and their metastases Snail genes are upregulated (69, 70). Furthermore, SNAIL TFs promote cancer recurrence (71) and resistance to cancer treatments (72). In turn, SNAIL1 can induce changes in the metabolism of glucose and can control the dependence of cancer cells to glycolysis relative to the pentose phosphate pathway (73), indicating the link between EMT factors, metabolism and cancer cell survival (Figure 1).
Figure 1. Interplay of glycolysis pathway with EMT factors. Enzymes are depicted in yellow font, EMT-related factors are depicted in red font. → denotes induction; ⊣ denotes inhibition. Yellow dots indicate intermediate reactions that are not depicted.
Phosphorylation of SNAIL proteins is crucial for their localization and their functionality in the cell (74). Glycogen Synthase Kinase-3 (GSK3) is a kinase that phosphorylates SNAIL1 resulting in its nuclear export and degradation (75). GSK3 can inhibit the EMT process by targeting SNAIL1 across multiple cell types (76). GSK3's activity contrasts that of p21-activated kinase (PAK1) which phosphorylates SNAIL1 resulting in its nuclear localization and the activation of EMT (77). Indeed, SNAIL1 expression and activation induces fibrosis in kidney and EMT (78). Importantly, de-phosphorylation of SNAIL proteins by Small C-terminal domain phosphatase (SCP) can also affect their localization and activity (79). Stabilization of SNAIL1 by nuclear factor kappa B (NF-kB) is mediated through prevention of its phosphorylation by GSK3 (80). Therefore, multiple post-translational regulators of SNAIL proteins control their functions and are putative targets for intervention.
TWIST1 and TWIST2 belong to the family of basic helix loop helix (bHLH) TFs. TWIST proteins are structurally similar and bind to E-box DNA response elements to repress or activate transcription (81). They have important physiological roles during embryonic morphogenesis, wound healing and tissue fibrosis (82, 83). In contrast, they are not expressed, or are expressed at extremely low levels, in most cell types after embryogenesis with the exception of certain precursor cell types (84), suggesting that they could be an attractive target for therapeutics or cancer biomarker development.
Expression of TWIST TFs can induce the EMT process and they are upregulated during cancer development (85, 86) and progression to metastasis (87). TWIST TFs are also associated with worse patient prognosis (87). Increasing the expression of TWIST1 is directly associated with tumor invasion and metastasis and mediates the loss of E-cadherin, a key epithelial marker. It also increases the expression of the mesenchymal markers Fibronectin, N-cadherin and Vimentin, leading to the reduction of cell adhesion and the promotion of cellular motility (87, 88). TWIST proteins also promote a cancer stem cell phenotype (89). TWIST activity can be modulated via post-translational modifications, such as phosphorylation. TWIST1 phosphorylation by MAP kinase stabilizes the protein and promotes breast cancer cell invasiveness and EMT (90). Similarly, AKT-mediated phosphorylation of TWIST results in increased invasiveness (91). In contrast, phosphorylation by IKKβ results in the degradation of TWIST (92).
The role of TWIST in metabolism has been mainly described in adipose tissue and has been associated with increased inflammation and insulin resistance (93). Its role in cancer metabolism has not been elucidated but it seems to be activated by asparagine and promote EMT (Figure 4) (94).
Another member of the bHLH EMT-TF group is Transcription Factor 3 (TCF3 or E2A) which produces two splice variants, E47 and E12 (95). TCF3 can induce the EMT process by inhibiting the expression of E-cadherin (96). Finally, E2.2 (also known as TCF4) is not a master EMT-TF, but it can induce a full EMT and represses E-cadherin expression indirectly, through complex, functional and hierarchical interactions with EMT factors (97, 98). Indeed, E2.2 is upregulated in cells overexpressing SNAIL1, SNAIL2, or E47 and after inhibition of E2.2 expression, the EMT is maintained, when driven by SNAIL1 and E47 (97).
The ZEB family in humans comprises ZEB1 (or δEF1) and ZEB2 (or SIP1), which are zinc finger TFs (99). ZEB TFs bind at bipartite E-boxes using their zinc-finger domains (100). Both ZEBs actively repress epithelial cell markers, and activate the expression of mesenchymal biomarkers, thus, mediating EMT (101). During physiological conditions, they are primarily expressed in the CNS, heart, skeletal muscle and hematopoietic cells. ZEB1 and ZEB2 can, in part, compensate for each other (102). Nevertheless, in lymphocytes, ZEB1 is mainly found in the thymus during T-lymphocyte development; whereas, ZEB2 is found primarily in the spleen during B-lymphocyte development (102), indicating differences in expression and functionality. The two ZEB TFs can even function antagonistically (103). ZEB2 knockout mice are embryonically lethal (104), indicating that ZEB1 cannot fully compensate for the developmental functions of ZEB2 in its absence.
Multiple signaling molecules control ZEB1 and ZEB2 expression. For instance, estrogen signaling cascades can induce ZEB1 expression (105). Similarly, TGFβ and Wnt/β-catenin signaling are activators of ZEB1 (106). Also, SNAIL1 and TWIST1 cooperatively control ZEB1 expression levels (107). In turn, ZEB1 suppresses multiple genes being involved in the generation and maintenance of epithelial cell polarity, including CDH1, Lgl2, PATJ, and Crumbs3 (108). ZEB1/2 expression in epithelial cells results in EMT and a mesenchymal phenotype, promoting invasion, metastatic dissemination and de-differentiation to a cancer stem cell state (109). The expression of ZEB1 associates with poor clinical outcome in solid tumors (110), including those of the breast (111), colorectum (112) or pancreas (113). ZEB2 expression also associates with poor prognosis and survival in different cancer types (114–116). ZEB1/2 and the miR-200 family expression levels are anti-correlated, with a double-negative feedback loop between them, which is described in the section of microRNAs. Post-translational modifications of both TFs can also modulate their expression levels, an example being the phosphorylation of ZEB1 (117) and the SUMOylation of ZEB2 [reviewed in (118)].
From a metabolic point of view, ZEB1 has been recently described to be a central component of adipogenesis (119) in non-cancer cell studies but ZEB1/2 have been more extensively studied in the context of cancer cell metabolism and appear to affect glycolysis (120) (Figure 1), to be affected by TCA cycle byproducts and drive EMT (121) (Figure 2), and to divert glycosphingolipid metabolism (122) (Figure 3).
Figure 2. Crosstalk of TCA cycle with EMT factors. Enzymes are depicted in yellow font, EMT-related factors are depicted in red font. → denotes induction; ⊣ denotes inhibition.
Figure 3. Fatty acid metabolism and EMT factors. Enzymes are depicted in yellow font, EMT-related factors are depicted in red font. → denotes induction; ⊣ denotes inhibition.
In addition to the thoroughly studied canonical EMT-TFs, a number of other TFs are also implicated in EMT. For instance, Krüppel-like factor 8 (KLF8) promotes EMT in breast (123, 124), ovarian (125) and gastric cancer cell lines (126). In particular, KLF8 resulted in the acquisition of mesenchymal features including enhanced motility, changes in cell morphology and direct inhibition of E-cadherin expression by modulating its promoter (123).
Paired-related homeobox 1 (PRRX1) overexpression activates EMT in certain cancers, including those of the stomach (127), colorectum (128), pancreas (129) or breast (39), and promotes a migratory and invasive phenotype. However, at a later stage of the metastatic process, its expression must be stopped, to promote MET, metastatic colonization and an epithelial phenotype with stem cell features (39). In particular, two isoforms of PRRX1, PRRX1a, and PRRX1b, have distinct functions in EMT and MET in pancreatic ductal adenocarcinoma (36). PRRX1b promotes de-differentiation, invasiveness and EMT; whereas, PRRX1a is involved in differentiation and MET (36).
Forkhead box C2 (FOXC2) is a TF that can induce EMT and thus, indirectly inhibit the expression of E-cadherin (130). Under physiological conditions, FOXC2 plays a role during in embryogenesis, affecting angiogenesis and the development of the muscles, kidney and urinary tract (131), while it also has distinct functionalities in adipocytes (132). Importantly, FOXC2 has roles in the metastatic process through EMT activation in breast, prostate and ovarian cancers (133–136).
Goosecoid (GSC) can indirectly inhibit E-cadherin (130) and is overexpressed in many breast cancers and metastases (137). Another TF, LBX1 (Ladybird homeobox 1) can up-regulate the expression of ZEB1/2 and SNAIL1, promoting cell migration and invasiveness in breast cancer (138). Finally, NF-kB induces EMT by regulating the expression of EMT-TFs, while its inhibition abolishes the metastatic potential of mammary epithelial cells (139).
All these non-canonical TFs that play a role of EMT have not been studied very thoroughly in the very specific content of cancer cell metabolism but some of them like FOXC2 (140) and PRRX1 (141) have established roles in adipocyte metabolism. However, their interaction with ZEB, SNAIL, TGFβ that are major players of EMT means that they can indirectly affect EMT and possibly relevant metabolic processes.
OVOL1 and OVOL2 are zinc-finger TFs involved in the maintenance of the epithelial state (142) and the suppression of the EMT (143). OVOL2 and ZEB1 act as mutual repressors of each other (11). Grainy-head like 2 (GRHL2) is involved in the establishment of epithelial identity (144) and the suppression of EMT (145, 146). It has been shown that GRHL2 suppresses EMT by inhibition of P300, which is required for EMT (146). It also antagonizes TGFβ-induced EMT in gastric cancer (147). Furthermore, knock down of GRHL2 and OVOL2 increases the collective cell migration (148). The pioneer TFs, FOXA1, and FOXA2, are transcriptional activators of epithelial genes, including E-cadherin expression (149). Both FOXA1 and FOXA2 are regulators and antagonists of the EMT and can be down-regulated by SNAIL1, resulting in the inactivation of enhancers at key epithelial genes (150). The TF GATA3 promotes the epithelial phenotype and inhibits metastasis in breast cancer (151). Thus, in addition to the TFs that are EMT inducers there is an opposing set of EMT suppressors. This set of transcription factors that suppress MET are not well described for their metabolic effects with the exception of a study that shows FOXA1 to reduce lipid accumulation in human hepatocytes (152). Their crosstalk though with ZEB1 or SNAIL can be relevant in an indirect way to cancer cell metabolism.
microRNAs (miRNAs) are small (18–24 nt long), non-coding RNAs that can post-transcriptionally fine-tune gene expression by targeting the 3′UTR of mRNA transcripts, leading to their destabilization and degradation. They are initially transcribed by RNA polymerase II into a pri-miRNA and processed by DROSHA to generate the pre-miRNA, which is subsequently exported from the nucleus and processed by DICER to mature miRNA (153). The mature miRNA interacts with the RNA-induced silencing complex (RISC) to target and cleave complementary mRNA molecules. About one third of human genes are recognized and targeted by miRNAs (154), indicating their pervasive regulatory control. Different miRNAs have been found to either promote or inhibit the EMT process through a multitude of functions. To date, over 130 different miRNAs have been implicated in EMT regulation (155) through combinatorial control networks (45). Among miRNA targets, there are multiple EMT-TFs, including SNAIL, TWIST and ZEB1/2 (156). microRNAs have also been described to regulate various metabolic processes including but not not limited to glucose and lipid metabolism in non-cancer cells (157) and have been described to participate in the regulation of metabolic pathways in cancer cells (158).
The miR-200 family members include miR-200a, miR-200b, miR-200c, miR-141, and miR-429, which are clustered in two polycistronic pri-miRNA loci, found in chromosomes 1 and 12, in humans. They can inhibit EMT by targeting the mRNA molecules of EMT promoting factors, resulting in their transcript degradation or translational repression. The miR-200 members share many of their targets, due to the high sequence homology between them in their seed region. Their overexpression leads to an increased E-cadherin expression, the maintenance of the epithelial phenotype and the inhibition of EMT (159). The miR-200 members target ZEB1/2, both of which repress E-cadherin (160–162). Their expression in cell lines results in MET, with the acquisition of epithelial cell morphology, phenotype and biomarkers and the loss of mesenchymal features, including the migratory phenotype and mesenchymal-associated biomarkers (159). ZEB1 can also inhibit the expression of miR-141 and miR-200c, increasing the complexity of this interaction network (160). As a result, ZEB1 and the miR-200 family are components of a mutual inhibition circuit. In cancer, the miR-200 family can suppress metastasis (163). The roles of miR-200 family in the suppression of EMT have been extensively studied in lung cancers (164). The miR-200 family also regulates multiple signaling cascades, including the WNT and Notch pathways (165, 166). TP53 is the most frequently mutated cancer gene among most cancer types and acts as a tumor suppressor (167). ZEB1 and ZEB2 expression can be downregulated by TP53, which activates miR-200 and miR-192, which in turn repress ZEB1 and ZEB2, resulting in EMT inhibition (168). miR-200 family has been reported to regulate pancreatic β cell survival in type 2 diabetes (169) and to be downregulated in high-fat diet-induced obesity in murine adipose tissue (170). In cancer cells it seems to be directly or indirectly intertwined with glycolysis (Figure 1) and TCA cycle metabolic pathways (Figure 2) (171, 172).
Other miRNAs can also regulate EMT, apart from the miR-200 family. For example, miR-9 and miR-10b can directly inactivate E-cadherin expression, promoting cell motility and metastasis (173–177). In breast cancer, miR-10b expression in otherwise non-metastatic tumors, promotes metastasis and correlates with clinical outcome (178). Also, silencing of miR-10b inhibits metastasis (179), suggesting its value as a putative therapeutic target. MYC and MYCN activate miR-9 inducing EMT in breast cancer and its expression is correlated with MYCN gene amplification in neuroblastoma (173). In addition, miR-29b and miR-30a inhibit the expression of SNAIL1 (156, 180). In prostate cancer, miR-29b levels are decreased and in prostate cancer cells its expression upregulates epithelial markers and downregulates mesenchymal markers (180). miR-34 and SNAIL1 both negatively control the expression of each other (181). During TGFβ-induced EMT, SNAIL1 suppresses the expression of miR-34. In breast cancer, miR-203 and SNAIL1 also negatively control the expression levels of each other (182). Similarly, there is a double-negative feedback loop between SNAIL2 on one hand, and miR-1 and miR-200b on the other (183). miR-21 has an EMT-promoting activity and is overexpressed in many cancers. It can up-regulate PTEN which in turn phosphorylates EMT factors to inhibit the EMT process (184). miR-23b targets ZEB1 (185). miR-424 is upregulated early during TWIST1- or SNAIL-driven EMT with roles in promoting the mesenchymal transitioning, without altering epithelial attributes (186). miR-205 family downregulates the expression of ZEB1/2 and in conjunction with the miR-200 members, it promotes MET (161). As a result of the above, multiple miRNAs are involved in the regulation of EMT across different cancer types and with a multitude of targets. Even though there is evidence that most of these described miRNAs play some roles in metabolic processes in normal cells, there is no concluding evidence that these, with the exception of miR-200, play specific roles in cancer cell metabolism with relevance to EMT process. Consequently, in the sections on EMT and metabolism below miR-200 is discussed more extensively and is depicted in summary Figures 1, 2.
Long non-coding RNAs (lncRNAs), i.e., non-coding RNAs of >200 nucleotides in length, are also involved in a plethora of biological processes, including EMT (187, 188). Hundreds of lncRNAs are deregulated during EMT (187), either promoting (189–192) or inhibiting it (193–196). Among their functions in EMT control, they can regulate signaling pathways including that of TGFβ (197), they can function as competing endogenous RNAs (ceRNAs) for miRNAs (198) or influence the expression of EMT-associated genes, including EMT master TFs (198–200). A 5′UTR intron at ZEB2 mRNA contains an internal ribosome entry site, which is required for its expression. ZEB2-AS1 lncRNA prevents the splicing of the 5′UTR intron, and enables the production of ZEB2 protein, which then inhibits E-cadherin expression (199). In breast cancer cells, UCA1 lncRNA promotes EMT through the activation of the Wnt/β-catenin signaling pathway (201). Its knock-down induces E-cadherin expression and it also reduces the mesenchymal characteristics of the cells and their invasiveness (201). H19 lncRNA is activated by hypoxia and TGFβ and promotes EMT. In particular, it inhibits E-cadherin expression, increasing the invasiveness of cancer cells and acts as a ceRNA for miR-138 and miR-200a (202, 203). These selected examples demonstrate the plethora and diversity in lncRNA functionalities relevant to EMT. Our understanding of the roles of lncRNAs is rapidly advancing. Expression levels of disparate lncRNAs are being investigated as clinical biomarkers of cancer diagnosis and prognosis (188) and could harbor clinical opportunities for intervention in EMT. Ofcourse, the research on the role of lncRNAs on metabolism is expanding (204) but currently there is not a lot of studies (205) linking them with cancer cell metabolism and specifically the ones that are related with the EMT process.
Metabolic changes during tumor development, of which the most thoroughly described mechanism has been the Warburg effect that facilitates the production of energy mostly from glycolysis and less from oxidative phosphorylation (206), potentiate the aggressive proliferation of cancer cells. However, it is important to note that cancer cells do not exclusively use glycolysis for energy production (207, 208) and studies have shown that oxidative phosphorylation promotion through enhanced mitochondrial biogenesis (209) or function (210) can also promote tumorigenesis progression and EMT. A key work (211) has shown that mesenchymal-like cancer cell lines exhibit a common metabolic gene signature that includes genes related to nucleotide, lipid, amino acid, glycan, carbon, and redox metabolism, and on top of that, known TFs affecting the EMT process, are co-expressed (up- or down-regulated) with these genes. Other individual studies focusing on specific cancers or cell-lines, also point to the same direction. In the following sections we summarize the most important metabolic pathways and their main players that are changing along with EMT, as well as how these can be potentially regulated by known EMT-driving TFs and other regulators. We also discuss if and how these metabolic processes can have an effect on EMT per se.
Fructose-1,6-biphosphatase 1 (FBP1) is an enzyme that hydrolyzes fructose 1,6-bisphosphate to fructose 6-phosphate and inorganic phosphate and regulates gluconeogenesis. SNAIL1 was found to directly represses the expression of FBP1 in two luminal breast cancer cells lines (212) and this led to enhancement of glycolytic flux, impaired oxygen consumption and reduced reactive oxygen species (ROS) production. FBP1 repression appears to occur due to de novo DNA methylation of its promoter. It is also interesting that ectopic overexpression of FBP1 in the SNAIL1-overexpressing cell lines inhibited the initiation of EMT and abrogated the downregulation of E-cadherin that is required for EMT (212). Downregulation of FBP1 has been shown to be a poor prognostic factor in gastric cancer (213) and in aggressive glioblastomas (214), as well indicating the importance of this finding.
Phosphofructokinase 1 (PFK1) is an important glycolytic enzyme that has the opposite function of FBP1; it catalyzes the conversion of fructose 6-phosphate to fructose 1,6-bisphosphate. Its increased expression facilitates the glycolytic flux and it is usually induced under hypoxic conditions as part of a wider transcriptional response induced by hypoxia-inducible factor 1 (HIF-1) (215, 216). Increased HGF signaling has been shown to lead to increased PFK1 activity and to EMT in a human hepatocarcinoma cell line (217). However, in cases where nutrients from cancer cells are depleted, glycolysis is no longer the “preferred” pathway for these cells and the glycolytic flux is diverted to pentose phosphate pathway (PPP) and PFK is repressed. SNAIL, a key EMT-TF has been described to repress PFKP, a major isoform of PFK1 (73). In breast cancer cell lines, under conditions of limited nutrients, it promotes PPP that generates NADPH, a reducing equivalent, and precursors for the synthesis of fatty acids, amino acids and nucleotides (73). In this way, the “stressed” cancer cells can survive in conditions of nutrient deprivation and its metastatic potential increases.
Hexokinases are enzymes that phosphorylate glucose to produce glucose-6-phosphate, which is the first step in most glucose pathways, including glycolysis. Hexokinase 2 (HK2) is the major isoform that is overexpressed in cancers (218) and its depletion can ameliorate the outcomes in a model of hepatocellular carcinoma (219). There are some indications that, under hypoxic conditions, the overexpression of HK2 can facilitate EMT (220). Another hexokinase isoform (HK3) has recently been described to be associated with EMT in colorectal cancers (221). Exact molecular mechanisms have not been described and these data are mainly based on association studies.
Pyruvate Dehydrogenase Kinase 4 (PDK4) is located in the mitochondrial matrix and inhibits the pyruvate dehydrogenase complex via phosphorylation. Thus, it inhibits the conversion of pyruvate to acetyl-CoA decreasing the metabolites flux to tricarboxylic acid cycle, down-regulating aerobic respiration and promoting glycolysis and fat metabolism. PDK4 has been described to have oncogenic roles in human colon cancer cells (222) and its increased levels to be related with aggressiveness and chemoresistance in bladder cancer (223). However, low PDK4 levels were found to be associated with poorer prognosis in a series of non-small cell lung cancer samples (194).
Pyruvate kinase (PK) catalyzes the transfer of a phosphoryl group from phosphoenolpyruvate to ADP, generating ATP and pyruvate, which is actually the last step of glycolysis. A splice variant of PK, PKM2, is expressed in fetal tissues and cancers (224) and has been shown to be part of EMT in human colorectal cancer cells. Specifically, PKM2 translocates in the nucleus during EMT where it represses E-cadherin transcription by interacting with TGFβ-induced factor homeobox 2 (TGIF2) (225). This role of PKM2 can be described as non-canonical, as it does not refer directly to the classic role of this enzyme (catalysis of glycolysis) but it also shows that metabolism-related enzymes can acquire alternative functions in cancer cells that may be critical in the fate of cancer cells. Of course there are several instances where it is shown that PKM2 expression is enhanced in cancers and favors the glycolytic pathway and potentially the metastatic potential such as in pancreatic ductal adenocarcinoma tissues and cell lines (226).
Enolase 1 (ENO1) catalyzes the conversion of 2-phosphoglycerate to phosphoenolpyruvate and it is usually overexpressed in cancers, such as those in head and neck or lung (227, 228). Lung adenocarcinomas show increased ENO1 expression and its silencing represses EMT in the relevant cell lines models (229). Proteomic analysis in gastric cancer cells has revealed that ENO1 is central to a protein-protein interaction network that regulates tumor growth and metastasis (230).
Phosphoglucose isomerase (PGI) converts glucose-6-phosphate to fructose-6-phosphate. Interestingly this protein can also be secreted by cancer cells and act as a cytokine (autocrine motility factor; AMF) promoting migration, invasion and metastasis (231). PGI has also been shown to promote EMT in breast cancer cells by repressing miR-200 and inducing ZEB1/2 (171) and silencing of PGI expression promotes mesenchymal to epithelial transition in human lung fibrosarcoma cells (232).
Aldolase A (ALDOA) catalyzes the conversion of fructose-1,6-bisphosphate to glyceraldehyde 3-phosphate and dihydroxyacetone phosphate. ALDOA is usually overexpressed in cancers and it is usually associated with poor prognosis (233). Downregulation of ALDOA in squamous lung carcinoma lines led to reduced expression of mesenchymal markers (234). Its overexpression in colon cancers is also associated with worse outcomes and also leads to EMT as shown by RNA-seq based transcriptomics analysis (235). Supportive of this role of ALDOA are other studies showing that silencing of ALDOA increased E-cadherin (epithelial marker) and decreased N-cadherin (mesenchymal marker) in pancreatic cancer (236) and bladder cancer cell lines (237).
Glucose transporters 1 and 3 (GLUT1, GLUT3) facilitate the entrance of glucose in cells in an insulin-independent manner. GLUT1 is expressed at different levels in all tissues and mostly in fetal tissues while GLUT3 is most abundant in neurons. Cancer cells usually overexpress GLUT1 and GLUT3 to facilitate the uptake of glucose independent of insulin levels, and high levels of GLUT1 and GLUT3 are usually associated with poor prognosis (238, 239). In laryngeal cancer cells GLUT1 expression correlated with Vimentin and N-cadherin expression that are markers of EMT (240). GLUT3 has been found overexpressed in mesenchymal cells of non-small cell lung cancer and ZEB1 can induce GLUT3 expression in these cancer cells (241), indicating that GLUT3 is an important component of EMT. In Figure 1 we briefly summarize the role of the glycolytic pathway in the EMT process.
Fumarate hydratase (FH) converts fumarate to malate. Loss of functions mutations of FH lead to leiomyomatosis, renal cancer and pheochromocytomas (172, 242). Accumulation of fumarate, due to these mutations, can lead to EMT in renal cancer cells. Specifically, fumarate can inhibit Ten-Eleven translocation (TET)-mediated demethylation of the regulatory region of miR-200. Hence, fumarate can ultimately inhibit miR-200 family expression and thus, abrogate miR-200-mediated EMT suppression (172).
Succinate dehydrogenase (SDH) catalyzes the oxidation of succinate to fumarate. Loss of function mutations of SDH are found in paragangliomas, gastric stroma tumors and pulmonary chondromas (243, 244). Metastatic pheochromocytomas and paragangliomas with reduced SDH expression due to SDHB mutations, show an EMT signature based on transcriptomics analysis and increased SNAIL 1/2 protein expression (245). Interestingly, breast cancer cell lines undergoing EMT show reduced SDH expression and hepatocellular carcinoma cell lines with reduced SDH expression show increased expression of EMT markers (246), indicating there may be a link between EMT and SDH (247) with molecular mechanisms that warrant further investigation. There is a hint that accumulation of succinate due to SDH mutations can induce EMT with a similar mechanism with fumarate (172).
Isocitrate dehydrogenases (IDH) catalyze the conversion of isocitrate to α-ketoglutarate. Three isoforms exist in humans: IDH1 and IDH2 which are NADP+ dependent, and are unrelated to IDH3. IDH1 and IDH2 catalyze reversible reactions while the reaction catalyzed by IDH3 is not reversible and is subject to allosteric modifiers (248). Mutations of IDH1 and IDH2 have been described in cancers and specifically in gliomas (249) and leukemia (250). IDH1 and IDH2 mutations render the enzymes to mainly produce 2-hydroxyglutarate, instead of α-ketoglutarate. Accumulation of 2-hydroxyglutarate leads to an EMT phenotype that is dependent on upregulation of ZEB1 and downregulation of miR-200 family (121). In colorectal cancer cell lines 2-hydroxyglutarate increased ZEB-1 expression by trimethylation of histone H3 lysine 4 of the promoter region of ZEB1 (251). In Figure 2 a brief visual summary of the main TCA cycle interactions with EMT is provided.
De novo lipogenesis is the synthesis of fatty acids from non-lipid precursors (mostly carbohydrates in the form of acetyl-CoA). Ultimately, the fatty acids are esterified to glycerol to form triglycerides. Cancer cells usually show increased lipogenesis (252) and this is the reason why lipogenesis has been proposed as a target for cancer treatment. Little is known though for the role of lipogenesis genes in EMT. We summarize below the current knowledge regarding them.
Acetyl-CoA carboxylase (ACC) catalyzes the carboxylation of acetyl-CoA to malonyl-CoA. Two ACC isoforms exist: ACC1, that is found in cytoplasm and regulates de novo lipogenesis; and ACC2, that is found at the mitochondrion membrane and mainly regulates fatty acid oxidation. ACC1 has been found to be overexpressed in cancers, such as those of the breast (253) and liver (254), and blocking of ACC1 has been shown to reduce lung tumor growth in mice (255). However, there is limited data on the role of ACC1 in EMT, with the exception of a relatively recent paper on breast cancer cells, that suggested an alternative non-canonical role for ACC1 in EMT. Specifically, it was shown that leptin and TGFβ can inhibit the activity of ACC1 through AMPK-phosphorylation of ACC1 at Ser79 and promote EMT (256). It was suggested that this effect should be mediated by accumulation of acetyl-CoA because of ACC1 inhibition and by the concomitant increased acetylation of SMAD2 that mediates the TGFβ-induced EMT. It, thus, seems that even though ACC1 expression is found increased in some cancers including breast cancer, it does not necessarily mean that it would be a good treatment approach to silence ACC1 as it can increase metastatic potential by favoring EMT.
Fatty acid synthase (FASN) is a multifunctional protein with its main function being the synthesis of palmitate from acetyl-CoA and malonyl-CoA. In some cancers, a fusion of FASN and Estrogen receptor α (ER-a) genes has been described that may play a role in estrogen signaling (257). Overexpression of FASN has been described in cancers like gastrointestinal stromal tumors (257), breast (258), ovarian (259), and lung cancers (260). It has been proposed that enhanced FASN expression in cisplatin-resistant non-small cell lung cancer cells promotes EMT through TGFβ signaling (260). Other smaller studies have suggested that FASN may mediate EMT, but they have not provided any mechanistic insight (261).
Stearoyl-CoA desaturase-1 (SCD-1) is an enzyme anchored in endoplasmic reticulum that catalyzes the formation of monounsaturated fatty acids (oleate and palmitoleate from stearoyl-CoA and palmitoyl-CoA, respectively). SCD-1 is overexpressed in cancers such as lung adenocarcinoma and its increased expression correlates with poor prognosis (262). Silencing of SCD-1 in breast cancer cells has led to impairment of their EMT-like behavior and to decreased nuclear localization of β-catenin, a known EMT mediator (263). ATP citrate lyase (ACLY) converts mitochondrially-derived citrate into acetyl-CoA and oxaloacetate providing the acetyl-CoA necessary for lipogenesis. ACLY is usually overexpressed in cancers (264, 265) and has been shown to promote EMT phenotypes in colon cancer cells at least partly through β-catenin signaling (266). Inhibition of ACLY has been proven effective to prevent EMT induced by ambient fine particulate matter (PM2.5) (267) and to reverse EMT phenotype in a lung adenocarcinoma cell line (268).
Acyl-CoA Synthetase Long Chain Family Member (ACSL) catalyzes the formation of fatty acyl-CoA from fatty acids and isoforms 1, 3, and 4 are more often overexpressed in cancer cells and specifically in colorectal (269), breast (270) and prostate cancers (271). Each isoform uses different substrates e.g., ACSL1 uses oleate and linoleate, ACSL3 uses myristate, palmitate, arachidonate and eicosapentaenoate and ACSL4 arachidonate (272). This activation of fatty acids by ACSL is a necessary step for the synthesis of cellular lipids as well as the β-oxidation. In colon cancer cells increased expression of ACSL1 and ACSL4 is associated with EMT features of these cells (273). The mechanism is not clear but one report suggests that this offers a metabolic advantage in the cancer cells by making them more energy efficient and by increasing the expression of SNAIL2 and N-cadherin (274).
Last, the metabolism of complex lipids and specifically glycosphingolipids has been shown to affect EMT. GM3 synthase converts lactosyl ceramide to a simple ganglioside called GM3 which is known to promote EMT by interacting with TGFβ receptors (275). It has been shown that ZEB1 can induce GM3 synthase gene by binding to its promoter and by repressing the micro-RNA mediated suppression of GM3 synthase in human lung cancer cells (122). Further work is needed to evaluate the importance of this regulation of glycosphingolipids metabolism in EMT in various types of cancer. Figure 3 summarizes the main lipid metabolism pathways that interact with EMT process.
Amino acid metabolism is essential for the maintenance of cellular homeostasis. In cancer cells there is an increased need for nitrogen for biosynthetic reactions, amino acids are consumed quickly and there is increased demand for non-essential amino acids that exceeds the supply (276). It is also impressive that in most cancer cells glutamine is the second highest nutrient in demand after glucose (277). Herein, we focus on the amino acids of glutamine, asparagine and cystine that have been described to be at least partly implicated in EMT in cancers.
Glutamine is the most abundant amino acid in serum and is highly consumed by many cancer cells. It provides also the major source of α-ketoglutarate in glutamine-dependent cancer cells to be used in TCA cycle through a process called glutaminolysis (278). Glutaminases 1 and 2 (cytosolic GLS1, mitochondrial GLS2) catalyze the hydrolysis of glutamine to glutamate and ammonia. GLS1 can be induced by TGFβ and Wnt and can promote EMT in a SNAIL-dependent manner while silencing of GLS1 prevents EMT (279). In contrast with GLS1 which is ubiquitously expressed, GLS2 is mainly expressed in brain, liver and pancreas and is inversely associated with EMT in breast cancer and hepatocellular carcinoma cells (279, 280). Interestingly, GLS2 levels are inversely correlated with GLS1 levels in breast cancer and it seems that at least in breast cancer cells GLS2 downregulation is the result and not the driver of EMT; silencing of FOXC2 led to increased levels of GLS2, did not affect GLS1 levels and led to inhibition of EMT (280). These observations mean that there may be required a fine tuning of glutaminolysis in different compartments of the cancer cells, reflected by the GLS1/GLS2 ratio, to support EMT and interfering with this can be a promising method for EMT inhibition.
Asparagine is a non-essential amino acid in humans and its abundance has been associated with EMT and the metastatic potential of breast cancer cells. Increased intake of asparagine with diet or increased asparagine synthetase activity led to increased incidence of metastases whereas reduced diet intake of asparagine or decreased asparagine synthetase activity or treatment with L-asparaginase reduced metastatic potential without affecting the growth of the primary tumor (281). Asparagine can become an essential amino acid in cases of glutamine deprivation in the tumor microenvironment so as to maintain protein synthesis and cell proliferation (282). Proteins that are upregulated during EMT have a ~20% higher asparagine content (281). Thus, it is rational to hypothesize that reduced asparagine availability would inhibit EMT at least at the translational level. However, it not clear how asparagine can transcriptionally regulate EMT genes like TWIST or N-cadherin (94). Further investigation is warranted to unravel all the potential mechanisms of asparagine's contribution to EMT.
Cystines formed by the oxidation of two cysteine molecules and their link by a disulfide bond. It is the main circulating form of cysteine that can be uptaken by cells. Cancer cells can become “addicted” to cysteine (283) and their reliance on cystine may be associated with EMT (284). Overexpression of miR-200c, that inhibits EMT, in cystine-addicted breast cancer cells resulted in these cells being less vulnerable to cystine deprivation (284). This is an indication that cystine can become an essential amino-acid during EMT at least in breast cancer cells but it is not known if and how it can affect EMT. Figure 4 summarizes briefly the interplay of amino-acid metabolism with EMT process.
Figure 4. Points of convergence between amino acid metabolism and EMT. Enzymes are depicted in yellow font, EMT-related factors are depicted in red font. → denotes induction.
Cancer is highly prevalent and remains a leading cause of death. The identification of novel treatments for the primary tumor as well as the discovery of potent inhibitors of metastasis is imperative. EMT and partial EMT can confer metastatic and stem cell properties to tumor cells (13, 285) and are correlated with the clinical outcome for cancer patients across multiple cancer types. It has also been demonstrated that EMT is linked to drug treatment resistance for multiple drugs (286, 287) and to multidrug resistance phenotype (288). EMT could be an attractive target to halt invasive and potentially metastatic cancer cells and to address treatment resistance. As a result, research in the development of EMT inhibitors for combinatorial cancer treatments is pivotal. In this section, we discuss multiple promising EMT targets including targets that link metabolic pathways with EMT.
The activity of EMT-TFs can be modulated by a number of kinases, which are therefore putative targets for intervention. In pancreatic cells, SB431542 blocks TGFβ-induced EMT by targeting the activity of TGFβ receptor kinase (289). In breast cancer cells, AG1478 targets EGFβ receptor kinase to halt EMT induction (290). Another small molecule inhibitor, BGB324 (also known as R428), blocks Axl kinase and inhibits metastasis (291). The signaling pathways involved in EMT are also putative therapeutic targets. TGFβ has been a target in several cancer types with Fresolimumab (GC-1008), which is a monoclonal antibody, in trials targeting EMT (292–294). Notch-2 is a signaling factor that promotes EMT. In pancreatic cancer, inactivation of Notch-2 by γ-secretase inhibitor IX resulted in selective inhibition of EMT (295). The mesenchymal phenotype of cancer cells has been the target of multiple additional intervention strategies. Withaferin A promotes the degradation of Vimentin (296) and can halt the migratory and invasive properties of cancer cells, therefore inhibiting the metastatic process. Antibody development against mesenchymal factors is also being pursued. For instance, an antibody raised against N-cadherin inhibits prostate cancer growth and metastasis (297). Certain miRNAs can halt the EMT induction while others promote it. In pancreatic cancer, miR-200 and let-7 upregulation by the natural compounds 3,3'-diindolylmethane and isoflavone results in a partial reversal of the EMT phenotype (298). Targeted inhibition of miR-21 has also been pursued with the development of a small molecule inhibitor, AC1MMYR2, which reverses the EMT process (299). Also, recent studies are unraveling the contribution of different lncRNAs in EMT regulation and have indicated that many lncRNAs could be utilized as clinical biomarkers such as prognostic and diagnostic biomarkers of metastasis and as potential therapeutic targets to inhibit cancer metastasis (188, 300).
Targeting metabolic pathways important for EMT has also been considered an alternative means of halting the EMT process (301). However, as most of these metabolic pathways are also essential for the survival of non-cancer cells, it is important to target as specifically as possible a certain pathway and ideally focus the treatment on the tissue and cells of interest and of course on the specific type of cancer. The metabolic pathways we describe herein in the context of EMT (glycolysis, TCA cycle, lipid metabolism, amino acid metabolism) have also been described as targets for treatment of cancer (302) and thus we could make use of the knowledge on targeting these pathways and affect the metabolism-dependent EMT. Specifically, glycolysis can be targeted at various levels (303, 304). For example, small molecule inhibitors of GLUT1 like fasentin (305), HK2 inhibitors like 3-bromopyruvate, and lonidamine (306) and PKM2 inhibitors (307) could be good candidate substances to be tested for EMT inhibition purposes. TCA cycle enzymes like IDH have been targeted in leukemias with inhibitors (e.g., AGI-6780) (308) and could also be the focus of studies on EMT. Lipogenesis inhibitors such as specific ACC1 inhibitors (309), SCD1 inhibitors (310) and ACLY inhibitors (311). Furthermore, methods to interfere with amino acid metabolism and specifically glutamine, asparagine and cystine would require even more fine tuning and targeted approaches given the differential role these amino acids may play in the original cancer and in the existing metastasis (94).
Last, the ideal scenario of intervening in the connection between EMT and cancer and especially in the possible hybrid mesenchymal-epithelial states of cancer cells warrants further investigations. Such research studies have shown for example that it is more effective to suppress TGFβ-driven EMT through targeting elements of the feedback loop between SMAD mediators of TGFβ signaling and EMT components in parallel and this intervention also inhibits these highly metastatic “hybrid” cancer cells (312). A combination of bioinformatics analyses (313) and single-cell sequencing studies (314) along with clinical cohorts of specific cancers (208) will be instrumental to unravel critical targets of EMT pathways and metabolism in parallel for the maximal effect on inhibiting EMT, haltering cancer progression and avoiding the formation of hybrid-state cancer cells.
In this review, we summarize the current knowledge on: (a) main molecular players underlying the EMT process; specifically transcription factors SNAIL, TWIST, ZEB, other non-canonical transcription factors and non-coding RNAs, and (b) the major metabolic pathways associated with EMT (glycolysis, TCA cycle, lipid and amino acid metabolism). We also review how these pathways can crosstalk with the molecular players. It is gradually becoming evident that there is a network of factors being affected by (and affecting) the metabolism of the pre-cancer or cancer cells, which change the fate of the cells through EMT, with major implications in cancer development. In Figures 1–4 we summarize the points where factors that affect EMT interplay with metabolic pathways. It is important to note that the data we summarize here emanate from studies on various cancer cell types that are noted in each instance. Thus, although cancers share certain common pathways, researchers should be cautious not to extrapolate results from one cancer type to another, but perform similar studies to their cancer of interest, before arriving to a conclusion. The metabolic features of cancer cells are an expanding field of study, as they are distinct from non-cancerous cells and could harbor therapeutic opportunities for intervention in EMT. Of course, suppressing EMT is an emerging prospect of preventing metastases, but it needs to be taken into consideration that this process represents a dynamic spectrum and once cancerous cells invade a tissue, they can undergo MET (315). Therefore, interference with the EMT process could promote metastasis if not targeted specifically at the site of origin.
IG-S, DC, and AZ conceived the study and wrote the review. VK contributed to writing and discussion. AZ supervised this work and paid the publication fees.
The authors declare that the research was conducted in the absence of any commercial or financial relationships that could be construed as a potential conflict of interest.
The authors would like to acknowledge Mr. George Georgoulias for assistance with referencing.
1. Takahashi K, Yamanaka S. Induction of pluripotent stem cells from mouse embryonic and adult fibroblast cultures by defined factors. Cell. (2006) 126:663–76. doi: 10.1016/j.cell.2006.07.024
2. Kalluri R, Weinberg RA. The basics of epithelial-mesenchymal transition. J Clin Invest. (2009) 119:1420–8. doi: 10.1172/JCI39104
3. Lamouille S, Xu J, Derynck R. Molecular mechanisms of epithelial-mesenchymal transition. Nat Rev Mol Cell Biol. (2014) 15:178–96. doi: 10.1038/nrm3758
4. Derynck R, Weinberg RA. EMT and cancer: more than meets the eye. Dev Cell. (2019) 49:313–6. doi: 10.1016/j.devcel.2019.04.026
5. Manzo G. Similarities between embryo development and cancer process suggest new strategies for research and therapy of tumors: a new point of view. Front Cell Dev Biol. (2019) 7:20. doi: 10.3389/fcell.2019.00020
6. Thiery JP, Acloque H, Huang RYJ, Nieto MA. Epithelial-mesenchymal transitions in development and disease. Cell. (2009) 139:871–90. doi: 10.1016/j.cell.2009.11.007
7. Zeisberg M, Neilson EG. Biomarkers for epithelial-mesenchymal transitions. J Clin Invest. (2009) 119:1429–37. doi: 10.1172/JCI36183
8. Mani SA, Guo W, Liao MJ, Eaton EN, Ayyanan A, Zhou AY, et al. The epithelial-mesenchymal transition generates cells with properties of stem cells. Cell. (2008) 133:704–15. doi: 10.1016/j.cell.2008.03.027
9. Morel AP, Lièvre M, Thomas C, Hinkal G, Ansieau S, Puisieux A. Generation of breast cancer stem cells through epithelial-mesenchymal transition. PLoS ONE. (2008) 3:e2888. doi: 10.1371/journal.pone.0002888
10. Jordan NV, Johnson GL, Abell AN. Tracking the intermediate stages of epithelial-mesenchymal transition in epithelial stem cells and cancer. Cell Cycle. (2011) 10:2865–73. doi: 10.4161/cc.10.17.17188
11. Hong T, Watanabe K, Ta CH, Villarreal-Ponce A, Nie Q, Dai X. An Ovol2-Zeb1 mutual inhibitory circuit governs bidirectional and multi-step transition between epithelial and mesenchymal states. PLoS Comp Biol. (2015) 11:e1004569. doi: 10.1371/journal.pcbi.1004569
12. Nieto MA, Huang RY-J, Rebecca Jackson AA, Jean Thiery PP. EMT: 2016. Cell. (2016) 166:21–45. doi: 10.1016/j.cell.2016.06.028
13. Lambert AW, Pattabiraman DR, Weinberg RA. Emerging biological principles of metastasis. Cell. (2017) 168:670–91. doi: 10.1016/j.cell.2016.11.037
14. Cook DP, Vanderhyden BC. Ovarian cancer and the evolution of subtype classifications using transcriptional profiling. Biol Reprod. (2019) 101:645–58. doi: 10.1093/biolre/ioz099
15. Kroger C, Afeyan A, Mraz J, Eaton EN, Reinhardt F, Khodor YL, et al. Acquisition of a hybrid E/M state is essential for tumorigenicity of basal breast cancer cells. Proc Natl Acad Sci USA. (2019) 116:7353–62. doi: 10.1073/pnas.1812876116
16. Jolly MK, Boareto M, Huang B, Jia D, Lu M, Ben-Jacob E, et al. Implications of the hybrid epithelial/mesenchymal phenotype in metastasis. Front Oncol. (2015) 5:155. doi: 10.3389/fonc.2015.00155
17. Pastushenko I, Brisebarre A, Sifrim A, Fioramonti M, Revenco T, Boumahdi S, et al. Identification of the tumour transition states occurring during EMT. Nature. (2018) 556:463–8. doi: 10.1038/s41586-018-0040-3
18. Dong J, Hu Y, Fan X, Wu X, Mao Y, Hu B, et al. Single-cell RNA-seq analysis unveils a prevalent epithelial/mesenchymal hybrid state during mouse organogenesis. Genome Biol. (2018) 19:31. doi: 10.1186/s13059-018-1416-2
19. Puram SV, Tirosh I, Parikh AS, Patel AP, Yizhak K, Gillespie S, et al. Single-cell transcriptomic analysis of primary and metastatic tumor ecosystems in head and neck cancer. Cell. (2017) 171:1611−24.e24. doi: 10.1016/j.cell.2017.10.044
20. van Dijk D, Sharma R, Nainys J, Yim K, Kathail P, Carr AJ, et al. Recovering gene interactions from single-cell data using data diffusion. Cell. (2018) 174:716−29.e27. doi: 10.1016/j.cell.2018.05.061
21. McFaline-Figueroa JL, Hill AJ, Qiu X, Jackson D, Shendure J, Trapnell C. A pooled single-cell genetic screen identifies regulatory checkpoints in the continuum of the epithelial-to-mesenchymal transition. Nat Genet. (2019) 51:1389–98. doi: 10.1038/s41588-019-0489-5
22. Sha Y, Haensel D, Gutierrez G, Du H, Dai X, Nie Q. Intermediate cell states in epithelial-to-mesenchymal transition. Phys Biol. (2019) 16:021001. doi: 10.1088/1478-3975/aaf928
23. Lazar MA, Birnbaum MJ. De-meaning of metabolism. Science. (2012) 336:1651–2. doi: 10.1126/science.1221834
24. Baenke F, Peck B, Miess H, Schulze A. Hooked on fat: the role of lipid synthesis in cancer metabolism and tumour development. Dis Model Mech. (2013) 6:1353–63. doi: 10.1242/dmm.011338
26. Li L, Li W. Epithelial-mesenchymal transition in human cancer: comprehensive reprogramming of metabolism, epigenetics, and differentiation. Pharmacol Ther. (2015) 150:33–46. doi: 10.1016/j.pharmthera.2015.01.004
27. Bhowmik SK, Ramirez-Pena E, Arnold JM, Putluri V, Sphyris N, Michailidis G, et al. EMT-induced metabolite signature identifies poor clinical outcome. Oncotarget. (2015) 6:42651–60. doi: 10.18632/oncotarget.4765
28. Sciacovelli M, Frezza C. Metabolic reprogramming and epithelial-to-mesenchymal transition in cancer. FEBS J. (2017) 284:3132–44. doi: 10.1111/febs.14090
29. Kang H, Kim H, Lee S, Youn H, Youn B. Role of metabolic reprogramming in epithelial-mesenchymal transition (EMT). Int J Mol Sci. (2019) 20:2042. doi: 10.3390/ijms20082042
30. Tarin D. The fallacy of epithelial mesenchymal transition in neoplasia. Cancer Res. (2005) 65:5996–6000. doi: 10.1158/0008-5472.CAN-05-0699
31. Christiansen JJ, Rajasekaran AK. Reassessing epithelial to mesenchymal transition as a prerequisite for carcinoma invasion and metastasis. Cancer Res. (2006) 66:8319–26. doi: 10.1158/0008-5472.CAN-06-0410
32. Tsai JH, Donaher JL, Murphy DA, Chau S, Yang J. Spatiotemporal regulation of epithelial-mesenchymal transition is essential for squamous cell carcinoma metastasis. Cancer Cell. (2012) 22:725–36. doi: 10.1016/j.ccr.2012.09.022
33. Tran HD, Luitel K, Kim M, Zhang K, Longmore GD, Tran DD. Transient SNAIL1 expression is necessary for metastatic competence in breast cancer. Cancer Res. (2014) 74:6330–40. doi: 10.1158/0008-5472.CAN-14-0923
34. Fischer KR, Durrans A, Lee S, Sheng J, Li F, S.Wong TC, et al. Epithelial-to-mesenchymal transition is not required for lung metastasis but contributes to chemoresistance. Nature. (2015) 527:472–6. doi: 10.1038/nature15748
35. Zheng X, Carstens JL, Kim J, Scheible M, Kaye J, Sugimoto H, et al. Epithelial-to-mesenchymal transition is dispensable for metastasis but induces chemoresistance in pancreatic cancer. Nature. (2015) 527:525–30. doi: 10.1038/nature16064
36. Takano S, Reichert M, Bakir B, Das KK, Nishida T, Miyazaki M, et al. Prrx1 isoform switching regulates pancreatic cancer invasion and metastatic colonization. Genes and Dev. (2016) 30:233–47. doi: 10.1101/gad.263327.115
37. Reichert M, Bakir B, Moreira L, Pitarresi JR, Feldmann K, Simon L, et al. Regulation of epithelial plasticity determines metastatic organotropism in pancreatic cancer. Dev Cell. (2018) 45:696−711.e8. doi: 10.1016/j.devcel.2018.05.025
38. Celià-Terrassa T, Meca-Cortés Ó, Mateo F, De Paz AM, Rubio N, Arnal-Estapé A, et al. Epithelial-mesenchymal transition can suppress major attributes of human epithelial tumor-initiating cells. J Clin Invest. (2012) 122:1849–68. doi: 10.1172/JCI59218
39. Ocaña OH, Córcoles R, Fabra Á, Moreno-Bueno G, Acloque H, Vega S, et al. Metastatic colonization requires the repression of the epithelial-mesenchymal transition inducer Prrx1. Cancer Cell. (2012) 22:709–24. doi: 10.1016/j.ccr.2012.10.012
40. Stankic M, Pavlovic S, Chin Y, Brogi E, Padua D, Norton L, et al. TGF-β‘-Id1 signaling opposes twist1 and promotes metastatic colonization via a mesenchymal-to-epithelial transition. Cell Rep. (2013) 5:1228–42. doi: 10.1016/j.celrep.2013.11.014
41. Chaffer CL, Weinberg RA. A perspective on cancer cell metastasis. Science. (2011) 331:1559–64. doi: 10.1126/science.1203543
42. Ito M, Yang Z, Andl T, Cui C, Kim N, Millar SE, et al. Wnt-dependent de novo hair follicle regeneration in adult mouse skin after wounding. Nature. (2007) 447:316–20. doi: 10.1038/nature05766
43. Nieto MA. The ins and outs of the epithelial to mesenchymal transition in health and disease. Ann Rev Cell Dev Biol. (2011) 27:347–76. doi: 10.1146/annurev-cellbio-092910-154036
44. Bielefeld KA, Amini-Nik S, Alman BA. Cutaneous wound healing: recruiting developmental pathways for regeneration. Cell Mol Life Sci. (2013) 70:2059–81. doi: 10.1007/s00018-012-1152-9
45. Zaravinos A. The regulatory role of MicroRNAs in EMT and cancer. J Clin. (2015) 2015:865816. doi: 10.1155/2015/865816
46. Stone RC, Pastar I, Ojeh N, Chen V, Liu S, Garzon KI, et al. Epithelial-mesenchymal transition in tissue repair and fibrosis. Cell Tissue Res. (2016) 365:495–506. doi: 10.1007/s00441-016-2464-0
47. Polyak K, Weinberg RA. Transitions between epithelial and mesenchymal states: acquisition of malignant and stem cell traits. Nat Rev Cancer. (2009) 9:265–73. doi: 10.1038/nrc2620
48. Peinado H, Olmeda D, Cano A. Snail, ZEB and bHLH factors in tumour progression: an alliance against the epithelial phenotype? Nat Rev Cancer. (2007). 7:415–28. doi: 10.1038/nrc2131
49. Hemavathy K, Ashraf SI, Ip YT. Snail/slug family of repressors: slowly going into the fast lane of development and cancer. Gene. (2000) 257:1–12. doi: 10.1016/S0378-1119(00)00371-1
50. Barrallo-Gimeno A, Nieto MA. The snail genes as inducers of cell movement and survival: implications in development and cancer. Development. (2005) 132:3151–61. doi: 10.1242/dev.01907
51. Arnoux V, Nassour M, L'Helgoualc'h A, Hipskind RA, Savagner P. Erk5 controls Slug expression and keratinocyte activation during wound healing. Mol Biol Cell. (2008) 19:4738–49. doi: 10.1091/mbc.e07-10-1078
52. Gras B, Jacqueroud L, Wierinckx A, Lamblot C, Fauvet F, Lachuer J, et al. Snail family members unequally trigger EMT and thereby differ in their ability to promote the neoplastic transformation of mammary epithelial cells. PLoS ONE. (2014) 9:e92254. doi: 10.1371/journal.pone.0092254
53. Whiteman EL, Liu CJ, Fearon ER, Margolis B. The transcription factor snail represses Crumbs3 expression and disrupts apico-basal polarity complexes. Oncogene. (2008) 27:3875–9. doi: 10.1038/onc.2008.9
54. Aigner K, Dampier B, Descovich L, Mikula M, Sultan A, Schreiber M, et al. The transcription factor ZEB1 (δEF1) promotes tumour cell dedifferentiation by repressing master regulators of epithelial polarity. Oncogene. (2007) 26:6979–88. doi: 10.1038/sj.onc.1210508
55. Batlle E, Sancho E, Francí C, Domínguez D, Monfar M, Baulida J, et al. The transcription factor Snail is a repressor of E-cadherin gene expression in epithelial tumour cells. Nat Cell Biol. (2000) 2:84–9. doi: 10.1038/35000034
56. Herranz N, Pasini D, Díaz VM, Francí C, Gutierrez A, Dave N, et al. Polycomb complex 2 is required for E-cadherin repression by the Snail1 transcription factor. Mol Cell Biol. (2008) 28:4772–81. doi: 10.1128/MCB.00323-08
57. Whiteman EL, Fan S, Harder JL, Walton KD, C.-Liu J, Soofi A, et al. Crumbs3 is essential for proper epithelial development and viability. Mol Cell Biol. (2014) 34:43–56. doi: 10.1128/MCB.00999-13
58. Yook JI, Li XY, Ota I, Hu C, Kim HS, Kim NH, et al. A Wnt-Axin2-GSK3β cascade regulates snail1 activity in breast cancer cells. Nat Cell Biol. (2006) 8:1398–406. doi: 10.1038/ncb1508
59. Min AL, Choi JY, Woo HY, Kim JD, Kwon JH, Bae SH, et al. High expression of Snail mRNA in blood from hepatocellular carcinoma patients with extra-hepatic metastasis. Clin Exp Metastasis. (2009) 26:759–67. doi: 10.1007/s10585-009-9275-6
60. Guo W, Keckesova Z, Donaher JL, Shibue T, Tischler V, Reinhardt F, et al. Slug and Sox9 cooperatively determine the mammary stem cell state. Cell. (2012) 148:1015–28. doi: 10.1016/j.cell.2012.02.008
61. Margetts PJ, Bonniaud P, Liu L, Hoff CM, Holmes CJ, West-Mays JA, et al. Transient overexpression of TGF-β1 induces epithelial mesenchymal transition in the rodent peritoneum. J Am Soc Nephrol. (2005) 16:425–36. doi: 10.1681/ASN.2004060436
62. Martínez-Álvarez C, Blanco MJ, Pérez R, Rabadán MA, Aparicio M, Resel E, et al. Snail family members and cell survival in physiological and pathological cleft palates. Dev Biol. (2004) 265:207–18. doi: 10.1016/j.ydbio.2003.09.022
63. Jamora C, Lee P, Kocieniewski P, Azhar M, Hosokawa R, Chai Y, et al. A signaling pathway involving TGF-β2 and snail in hair follicle morphogenesis. PLoS Biol. (2005) 3:e11. doi: 10.1371/journal.pbio.0030011
64. Romano LA, Runyan RB. Slug is an essential target of TGFbeta2 signaling in the developing chicken heart 10.1006/dbio.2000.9750 S0012-1606(00)99750-1 [pii]. Dev Biol. (2000) 223:91–102. doi: 10.1006/dbio.2000.9750
65. Dickinson ME, M.Selleck AJ, McMahon AP, Bronner-Fraser M. Dorsalization of the neural tube by the non-neural ectoderm. Development. (1995) 121:2099–106.
66. Blechschmidt K, Sassen S, Schmalfeldt B, Schuster T, Höfler H, Becker KF. The E-cadherin repressor Snail is associated with lower overall survival of ovarian cancer patients. Br J Cancer. (2008) 98:489–95. doi: 10.1038/sj.bjc.6604115
67. Fujita N, Jaye DL, Kajita M, Geigerman C, Moreno CS, Wade PA. MTA3, a Mi-2/NuRD complex subunit, regulates an invasive growth pathway in breast cancer. Cell. (2003) 113:207–19. doi: 10.1016/S0092-8674(03)00234-4
68. Shioiri M, Shida T, Koda K, Oda K, Seike K, Nishimura M, et al. Slug expression is an independent prognostic parameter for poor survival in colorectal carcinoma patients. Br J Cancer. (2006) 94:1816–22. doi: 10.1038/sj.bjc.6603193
69. Hardy RG, Vicente-Dueñas C, Gonzâlez-Herrero I, Anderson C, Flores T, Hughes S, et al. Snail family transcription factors are implicated in thyroid carcinogenesis. Am J Pathol. (2007) 171:1037–46. doi: 10.2353/ajpath.2007.061211
70. Hotz B, Arndt M, Dullat S, Bhargava S, Buhr HJ, Hotz HG. Epithelial to mesenchymal transition: expression of the regulators snail, slug, and twist in pancreatic cancer. Clin Cancer Res. (2007) 13:4769–76. doi: 10.1158/1078-0432.CCR-06-2926
71. Bruyere F, Namdarian B, Corcoran NM, Pedersen J, Ockrim J, Voelzke BB, et al. Snail expression is an independent predictor of tumor recurrence in superficial bladder cancers. Urol Oncol. (2010) 28:591–6. doi: 10.1016/j.urolonc.2008.11.005
72. Kurrey NK, Jalgaonkar SP, Joglekar AV, Ghanate AD, Chaskar PD, Doiphode RY, et al. Snail and slug mediate radioresistance and chemoresistance by antagonizing p53-mediated apoptosis and acquiring a stem-like phenotype in ovarian cancer cells. Stem Cells. (2009) 27:2059–68. doi: 10.1002/stem.154
73. Kim NH, Cha YH, Lee J, Lee SH, Yang JH, Yun JS, et al. Snail reprograms glucose metabolism by repressing phosphofructokinase PFKP allowing cancer cell survival under metabolic stress. Nat Commun. (2017) 8:14374. doi: 10.1038/ncomms14374
74. Dominguez D, Montserrat-Sentis B, Virgos-Soler A, Guaita S, Grueso J, Porta M, et al. Phosphorylation regulates the subcellular location and activity of the snail transcriptional repressor. Mol Cell Biol. (2003) 23:5078–89. doi: 10.1128/MCB.23.14.5078-5089.2003
75. Jong IY, Li XY, Ota I, Fearon ER, Weiss SJ. Wnt-dependent regulation of the E-cadherin repressor snail. J Biol Chem. (2005) 280:11740–8. doi: 10.1074/jbc.M413878200
76. Bachelder RE, Yoon SO, Franci C, García De Herreros A, Mercurio AM. Glycogen synthase kinase-3 is an endogenous inhibitor of Snail transcription: Implications for the epithelial - Mesenchymal transition. J Cell Biol. (2005) 168:29–33. doi: 10.1083/jcb.200409067
77. Yang Z, Rayala S, Nguyen D, Vadlamudi RK, Chen S, Kumar R. Pak1 phosphorylation of Snail, a master regulator of epithelial-to- mesenchyme transition, modulates Snail's subcellular localization and functions. Cancer Res. (2005) 65:3179–84. doi: 10.1158/0008-5472.CAN-04-3480
78. Boutet A, De Frutos CA, Maxwell PH, Mayol MJ, Romero J, Nieto MA. Snail activation disrupts tissue homeostasis and induces fibrosis in the adult kidney. EMBO J. (2006) 25:5603–13. doi: 10.1038/sj.emboj.7601421
79. Wu Y, Mark Evers B, Zhou BP. Small C-terminal domain phosphatase enhances snail activity through dephosphorylation. J Biol Chem. (2009) 284:640–8. doi: 10.1074/jbc.M806916200
80. Wu Y, Deng J, Rychahou PG, Qiu S, Evers BM, Zhou BP. Stabilization of snail by NF-κB is required for inflammation-induced cell migration and invasion. Cancer Cell. (2009) 15:416–28. doi: 10.1016/j.ccr.2009.03.016
81. Franco HL, Casasnovas J, Rodríguez-Medina JR, Cadilla CL. Redundant or separate entities? - Roles of Twist1 and Twist2 as molecular switches during gene transcription. Nucleic Acids Res. (2011) 39:1177–86. doi: 10.1093/nar/gkq890
82. Soldatov R, Kaucka M, Kastriti ME, Petersen J, Chontorotzea T, Englmaier L, et al. Spatiotemporal structure of cell fate decisions in murine neural crest. Science. (2019) 364:aas9536. doi: 10.1126/science.aas9536
83. Yeo SY, Lee KW, Shin D, An S, Cho KH, Kim SH. A positive feedback loop bi-stably activates fibroblasts. Nat Commun. (2018) 9:3016. doi: 10.1038/s41467-018-05274-6
84. Qin Q, Xu Y, He T, Qin C, Xu J. Normal and disease-related biological functions of Twist1 and underlying molecular mechanisms. Cell Res. (2012) 22:90–106. doi: 10.1038/cr.2011.144
85. Norozi F, Ahmadzadeh A, Shahjahani M, Shahrabi S, Saki N. Twist as a new prognostic marker in hematological malignancies. Clin Transl Oncol. (2016) 18:113–24. doi: 10.1007/s12094-015-1357-0
86. Nuti SV, Mor G, Li P, Yin G. TWIST and ovarian cancer stem cells: implications for chemoresistance and metastasis. Oncotarget. (2014) 5:7260–71. doi: 10.18632/oncotarget.2428
87. Yang J, Mani SA, Weinberg RA. Exploring a new twist on tumor metastasis. Cancer Res. (2006) 66:4549–52. doi: 10.1158/0008-5472.CAN-05-3850
88. Meng J, Chen S, Han JX, Qian B, Wang XR, Zhong WL, et al. Twist1 regulates vimentin through Cul2 circular RNA to promote EMT in hepatocellular carcinoma. Cancer Res. (2018) 78:4150–62. doi: 10.1158/0008-5472.CAN-17-3009
89. Wang SS, Jiang J, Liang XH, Tang YL. Links between cancer stem cells and epithelial- mesenchymal transition. Onco Targets Ther. (2015) 8:2973–80. doi: 10.2147/OTT.S91863
90. Hong J, Zhou J, Fu J, He T, Qin J, Wang L, et al. Phosphorylation of serine 68 of twist1 by MAPKs stabilizes twist1 protein and promotes breast cancer cell invasiveness. Cancer Res. (2011) 71:3980–90. doi: 10.1158/0008-5472.CAN-10-2914
91. Tang H, Massi D, Hemmings BA, Mandalà M, Hu Z, Wicki A, et al. AKT-ions with a TWIST between EMT and MET. Oncotarget. (2016) 7:62767–77. doi: 10.18632/oncotarget.11232
92. Zhong J, Ogura K, Wang Z, Inuzuka H. Degradation of the transcription factor Twist, an oncoprotein that promotes cancer metastasis. Discov Med. (2013) 15:7–15.
93. Dobrian AD. A tale with a Twist: a developmental gene with potential relevance for metabolic dysfunction and inflammation in adipose tissue. Front Endocrinol. (2012) 3:108. doi: 10.3389/fendo.2012.00108
94. Luo M, Brooks M, Wicha MS. Asparagine and Glutamine: Co-conspirators Fueling Metastasis. Cell Press. (2018) pp. 947-949. doi: 10.1016/j.cmet.2018.04.012
95. Sun XH, Baltimore D. An inhibitory domain of E12 transcription factor prevents DNA binding in E12 homodimers but not in E12 heterodimers. Cell. (1991) 64:459–70. doi: 10.1016/0092-8674(91)90653-G
96. Cubillo E, Diaz-Lopez A, Cuevas EP, Moreno-Bueno G, Peinado H, Montes A, et al. E47 and Id1 Interplay in Epithelial-Mesenchymal Transition. PLoS ONE. (2013) 8: doi: 10.1371/journal.pone.0059948
97. Sobrado VR, Moreno-Bueno G, Cubillo E, Holt LJ, Nieto MA, Portillo F, et al. The class I bHLH factors E2-2A and E2-2B regulate EMT. Journal of Cell Science. (2009) 122:1014–24. doi: 10.1242/jcs.028241
98. Cano A, Portillo F. An emerging role for class I bHLH E2-2 proteins in EMT regulation and tumour progression. Taylor and Francis Inc. (2010) pp. 56-60. doi: 10.4161/cam.4.1.9995
99. Gheldof A, Hulpiau P, Van Roy F, De Craene B, Berx G. Evolutionary functional analysis and molecular regulation of the ZEB transcription factors. (2012). pp. 2527-2541. doi: 10.1007/s00018-012-0935-3
100. Eger A, Aigner K, Sonderegger S, Dampier B, Oehler S, Schreiber M, et al. DeltaEF1 is a transcriptional repressor of E-cadherin and regulates epithelial plasticity in breast cancer cells. Oncogene. (2005) 24:2375–85. doi: 10.1038/sj.onc.1208429
101. Zhang P, Sun Y, Ma L. ZEB1: at the crossroads of epithelial-mesenchymal transition, metastasis and therapy resistance. Landes Biosci. (2015)14:481–7. doi: 10.1080/15384101.2015.1006048
102. Postigo AA, Dean DC. Differential expression and function of members of the zfh-1 family of zinc finger/homeodomain repressors. Proc Natl Acad Sci USA. (2000) 97:6391–6. doi: 10.1073/pnas.97.12.6391
103. Postigo AA, Depp JL, Taylor JJ, Kroll KL. Regulation of Smad signaling through a differential recruitment of coactivators and corepressors by ZEB proteins. EMBO J. (2003) 22:2453–62. doi: 10.1093/emboj/cdg226
104. De Putte TV, Maruhashi M, Francis A, Nelles L, Kondoh H, Huylebroeck D, et al. Mice lacking Zfhx1b, the gene that codes for Smad-interacting protein-1, reveal a role for multiple neural crest cell defects in the etiology of hirschsprung disease-mental retardation syndrome. Am J Human Genet. (2003) 72:465–70. doi: 10.1086/346092
105. Dillner NB, Sanders MM. The zinc finger/homeodomain protein deltaEF1 mediates estrogen-specific induction of the ovalbumin gene. Mol Cell Endocrinol. (2002) 192:85–91. doi: 10.1016/S0303-7207(02)00088-6
106. Heldin CH, Vanlandewijck M, Moustakas A. Regulation of EMT by TGFβ in cancer. FEBS Lett. (2012) 586:1959–70. doi: 10.1016/j.febslet.2012.02.037
107. Dave N, Guaita-Esteruelas S, Gutarra S, Frias À, Beltran M, Peiró S, et al. García De Herreros. Functional cooperation between snail1 and twist in the regulation of ZEB1 expression during epithelial to mesenchymal transition. J Biol Chem. (2011) 286:12024–32. doi: 10.1074/jbc.M110.168625
108. Spaderna S, Schmalhofer O, Wahlbuhl M, Dimmler A, Bauer K, Sultan A, et al. The transcriptional repressor ZEB1 promotes metastasis and loss of cell polarity in cancer. Cancer Res. (2008) 68:537–44. doi: 10.1158/0008-5472.CAN-07-5682
109. Chaffer CL, Marjanovic ND, Lee T, Bell G, Kleer CG, Reinhardt F, et al. XPoised chromatin at the ZEB1 promoter enables breast cancer cell plasticity and enhances tumorigenicity. Cell. (2013) 154:61. doi: 10.1016/j.cell.2013.06.005
110. Chen B, Chen B, Zhu Z, Ye W, Zeng J, Liu G, et al. Prognostic value of ZEB-1 in solid tumors: a meta-analysis. BMC Cancer. (2019) 19:635. doi: 10.1186/s12885-019-5830-y
111. Jang MH, Kim HJ, Kim EJ, Chung YR, Park SY. Expression of epithelial-mesenchymal transition-related markers in triple-negative breast cancer: ZEB1 as a potential biomarker for poor clinical outcome. Human Pathol. (2015) 46:1267–74. doi: 10.1016/j.humpath.2015.05.010
112. Zhang GJ, Zhou T, Tian HP, Liu ZL, Xia SS. High expression of ZEB1 correlates with liver metastasis and poor prognosis in colorectal cancer. Oncol Lett. (2013) 5:564–8. doi: 10.3892/ol.2012.1026
113. Kurahara H, Takao S, Maemura K, Mataki Y, Kuwahata T, Maeda K, et al. Epithelial-mesenchymal transition and mesenchymal-epithelial transition via regulation of ZEB-1 and ZEB-2 expression in pancreatic cancer. J Surg Oncol. (2012) 105:655–61. doi: 10.1002/jso.23020
114. Prislei S, Martinelli E, Zannoni GF, Petrillo M, Filippetti F, Mariani M, et al. Role and prognostic significance of the epithelial-mesenchymal transition factor ZEB2 in ovarian cancer. Oncotarget. (2015) 6:18966–79. doi: 10.18632/oncotarget.3943
115. Chen H, Lu W, Huang C, Ding K, Xia D, Wu Y, et al. Prognostic significance of ZEB1 and ZEB2 in digestive cancers: a cohort-based analysis and secondary analysis. Oncotarget. (2017) 8:31435–48. doi: 10.18632/oncotarget.15634
116. Li MZ, Wang JJ, Yang SB, Li WF, Xiao LB, He YL, et al. ZEB2 promotes tumor metastasis and correlates with poor prognosis of human colorectal cancer. Am J Transl Res. (2017) 9:2838–51.
117. Llorens MC, Lorenzatti G, Cavallo NL, Vaglienti MV, Perrone AP, Carenbauer AL, et al. Phosphorylation regulates functions of ZEB1 transcription factor. J Cell Physiol. (2016) 231:2205–17. doi: 10.1002/jcp.25338
118. Bogachek MV, De Andrade JP, Weigel RJ. Regulation of epithelial-mesenchymal transition through sumoylation of transcription factors. Cancer Res. (2015) 75:11–5. doi: 10.1158/0008-5472.CAN-14-2824
119. Gubelmann C, Schwalie PC, Raghav SK, Roder E, Delessa T, Kiehlmann E, et al. Identification of the transcription factor ZEB1 as a central component of the adipogenic gene regulatory network. Elife. (2014) 3:e03346. doi: 10.7554/eLife.03346
120. Pouyafar A, Heydarabad MZ, Abdolalizadeh J, Zade JA, Rahbarghazi R, Talebi M. Modulation of lipolysis and glycolysis pathways in cancer stem cells changed multipotentiality and differentiation capacity toward endothelial lineage. Cell Biosci. (2019) 9:30. doi: 10.1186/s13578-019-0293-z
121. Grassian AR, Lin F, Barrett R, Liu Y, Jiang W, Korpal M, et al. Isocitrate dehydrogenase (IDH) mutations promote a reversible ZEB1/MicroRNA (miR)-200-dependent epithelial-mesenchymal transition (EMT). J Biol Chem. (2012) 287:42180–94. doi: 10.1074/jbc.M112.417832
122. Mathow D, Chessa F, Rabionet M, Kaden S, Jennemann R, Sandhoff R, et al. Zeb1 affects epithelial cell adhesion by diverting glycosphingolipid metabolism. EMBO Rep. (2015) 16:321–31. doi: 10.15252/embr.201439333
123. Wang X, Zheng M, Liu G, Xia W, McKeown-Longo PJ, Hung MC, et al. Krüppel-like factor 8 induces epithelial to mesenchymal transition and epithelial cell invasion. Cancer Res. (2007) 67:7184–93. doi: 10.1158/0008-5472.CAN-06-4729
124. Wang X, Lu H, Urvalek AM, Li T, Yu L, Lamar J, et al. KLF8 promotes human breast cancer cell invasion and metastasis by transcriptional activation of MMP9. Oncogene. (2011) 30:1901–11. doi: 10.1038/onc.2010.563
125. Lu H, Wang X, Urvalek AM, Li T, Xie H, Yu L, et al. Transformation of human ovarian surface epithelial cells by Krüppel-like factor 8. Oncogene. (2014) 33:10–8. doi: 10.1038/onc.2012.545
126. Zhang H, Zhang H, Liu L, Wang Y, Zhao G, Xie R, et al. KLF8 involves in TGF-beta-induced EMT and promotes invasion and migration in gastric cancer cells. J Cancer Res Clin Oncol. (2013) 139:1033–42. doi: 10.1007/s00432-012-1363-3
127. Guo J, Fu Z, Wei J, Lu W, Feng J, Zhang S. PRRX1 promotes epithelial-mesenchymal transition through the Wnt/β-catenin pathway in gastric cancer. Med Oncol. (2015) 32:1–12. doi: 10.1007/s12032-014-0393-x
128. Takahashi Y, Sawada G, Kurashige J, Uchi R, Matsumura T, Ueo H, et al. Paired related homoeobox 1, a new EMT inducer, is involved in metastasis and poor prognosis in colorectal cancer. Br J Cancer. (2013) 109:307–11. doi: 10.1038/bjc.2013.339
129. Reichert M, Takano S, Von Burstin J, Kim SB, Lee JS, Ihida-Stansbury K, et al. The Prrx1 homeodomain transcription factor plays a central role in pancreatic regeneration and carcinogenesis. Genes Dev. (2013) 27:288–300. doi: 10.1101/gad.204453.112
130. Yang J, Weinberg RA. Epithelial-mesenchymal transition: at the crossroads of development and tumor metastasis. Dev Cell. (2008) 14:818–29. doi: 10.1016/j.devcel.2008.05.009
131. Kume T, Deng K, Hogan BLM. Minimal phenotype of mice homozygous for a null mutation in the forkhead/winged helix gene, Mf2. Mol Cell Biol. (2000) 20:1419–25. doi: 10.1128/MCB.20.4.1419-1425.2000
132. Cederberg A, Gronning LM, Ahrén B, Taskén K, Carlsson P, Enerbäck S. FOXC2 is a winged helix gene that counteracts obesity, hypertriglyceridemia, and diet-induced insulin resistance. Cell. (2001) 106:563–73. doi: 10.1016/S0092-8674(01)00474-3
133. Mani SA, Yang J, Brooks M, Schwaninger G, Zhou A, Miura N, et al. Mesenchyme Forkhead 1 (FOXC2) plays a key role in metastasis and is associated with aggressive basal-like breast cancers. Proc Natl Acad Sci USA. (2007) 104:10069–74. doi: 10.1073/pnas.0703900104
134. Hollier BG, Tinnirello AA, Werden SJ, Evans KW, Taube JH, Sarkar TR, et al. FOXC2 expression links epithelial-mesenchymal transition and stem cell properties in breast cancer. Cancer Res. (2013) 73:1981–92. doi: 10.1158/0008-5472.CAN-12-2962
135. Liu B, Han SM, Tang XY, Han LI, Li CZ. Overexpressed FOXC2 in ovarian cancer enhances the epithelial-to- mesenchymal transition and invasion of ovarian cancer cells. Oncol Rep. (2014) 31:2545–54. doi: 10.3892/or.2014.3119
136. Paranjape AN, Soundararajan R, Werden SJ, Joseph R, Taube JH, Liu H, et al. Inhibition of FOXC2 restores epithelial phenotype and drug sensitivity in prostate cancer cells with stem-cell properties. Oncogene. (2016) 35:5963–76. doi: 10.1038/onc.2015.498
137. Hartwell KA, Muir B, Reinhardt F, Carpenter AE, Sgroi DC, Weinberg RA. The Spemann organizer gene. Goosecoid, promotes tumor metastasis. Proc Natl Acad Sci USA. (2006) 103:18969–74. doi: 10.1073/pnas.0608636103
138. Yu M, Smolen GA, Zhang J, Wittner B, Schott BJ, Brachtel E, et al. A developmentally regulated inducer of EMT, LBX1, contributes to breast cancer progression. Genes Dev. (2009) 23:1737–42. doi: 10.1101/gad.1809309
139. Pires BRB, Mencalha AL, Ferreira GM, De Souza WF, Morgado-Díaz JA, Maia AM, et al. NF-kappaB is involved in the regulation of EMT genes in breast cancer cells. PLoS ONE. (2017) 12:e0169622. doi: 10.1371/journal.pone.0169622
140. Lidell ME, Seifert EL, Westergren R, Heglind M, Gowing A, Sukonina V, et al. The adipocyte-expressed forkhead transcription factor Foxc2 regulates metabolism through altered mitochondrial function. Diabetes. (2011) 60:427–35. doi: 10.2337/db10-0409
141. Du B, Cawthorn WP, Su A, Doucette CR, Yao Y, Hemati N, et al. The transcription factor paired-related homeobox 1 (Prrx1) inhibits adipogenesis by activating transforming growth factor-beta (TGFbeta) signaling. J Biol Chem. (2013) 288:3036–47. doi: 10.1074/jbc.M112.440370
142. Lee B, Villarreal-Ponce A, Fallahi M, Ovadia J, Sun P, Yu QC, et al. Transcriptional mechanisms link epithelial plasticity to adhesion and differentiation of epidermal progenitor cells. Dev Cell. (2014) 29:47–58. doi: 10.1016/j.devcel.2014.03.005
143. Roca H, Hernandez J, Weidner S, McEachin RC, Fuller D, Sud S, et al. Transcription factors OVOL1 and OVOL2 induce the mesenchymal to epithelial transition in human cancer. PLoS ONE. (2013) 8:e76773. doi: 10.1371/journal.pone.0076773
144. Werth M, Walentin K, Aue A, Schönheit J, Wuebken A, Pode-Shakked N, et al. The transcription factor grainyhead-like 2 regulates the molecular composition of the epithelial apical junctional complex. Development. (2010) 137:3835–45. doi: 10.1242/dev.055483
145. Cieply B, Farris J, Denvir J, Ford HL, Frisch SM. Epithelial-mesenchymal transition and tumor suppression are controlled by a reciprocal feedback loop between ZEB1 and Grainyhead-like-2. Cancer Res. (2013) 73:6299–309. doi: 10.1158/0008-5472.CAN-12-4082
146. Cieply B, Riley Iv P, Pifer PM, Widmeyer J, Addison JB, Ivanov AV, et al. Suppression of the epithelial-mesenchymal transition by grainyhead-like-2. Cancer Res. (2012) 72:2440–53. doi: 10.1158/0008-5472.CAN-11-4038
147. Xiang J, Fu X, Ran W, Wang Z. Grhl2 reduces invasion and migration through inhibition of TGFβ-induced EMT in gastric cancer. Oncogenesis. (2017) 6:e284. doi: 10.1038/oncsis.2016.83
148. Jolly MK, Tripathi SC, Jia D, Mooney SM, Celiktas M, Hanash SM, et al. Stability of the hybrid epithelial/mesenchymal phenotype. Oncotarget. (2016) 7:27067–84. doi: 10.18632/oncotarget.8166
149. Song Y, Washington MK, Crawford HC. Loss of FOXA1/2 is essential for the epithelial-to-mesenchymal transition in pancreatic cancer. Cancer Res. (2010) 70:2115–25. doi: 10.1158/0008-5472.CAN-09-2979
150. Jägle S, Busch H, Freihen V, Beyes S, Schrempp M, Boerries M, et al. SNAIL1-mediated downregulation of FOXA proteins facilitates the inactivation of transcriptional enhancer elements at key epithelial genes in colorectal cancer cells. PLoS Genet. (2017) 13:e1007109. doi: 10.1371/journal.pgen.1007109
151. Yan W, Cao QJ, Arenas RB, Bentley B, Shao R. GATA3 inhibits breast cancer metastasis through the reversal of epithelial-mesenchymal transition. J Biol Chem. (2010) 285:14042–51. doi: 10.1074/jbc.M110.105262
152. Moya M, Benet M, Guzman C, Tolosa L, Garcia-Monzon C, Pareja E, et al. Foxa1 reduces lipid accumulation in human hepatocytes and is down-regulated in nonalcoholic fatty liver. PLoS ONE. (2012) 7:e30014. doi: 10.1371/journal.pone.0030014
153. Bartel DP. MicroRNAs: genomics, biogenesis, mechanism, and function. Cell. (2004) 116:281–97. doi: 10.1016/S0092-8674(04)00045-5
154. Lewis BP, Burge CB, Bartel DP. Conserved seed pairing, often flanked by adenosines, indicates that thousands of human genes are microRNA targets. Cell. (2005) 120:15–20. doi: 10.1016/j.cell.2004.12.035
155. Cursons J, Pillman KA, Scheer KG, Gregory PA, Foroutan M, Hediyeh-Zadeh S, et al. Combinatorial targeting by microRNAs co-ordinates post-transcriptional control of EMT. Cell Syst. (2018) 7:77–91.e7. doi: 10.1016/j.cels.2018.05.019
156. Zhang J, Ma L. MicroRNA control of epithelial-mesenchymal transition and metastasis. Cancer Metastasis Rev. (2012) 31:653–62. doi: 10.1007/s10555-012-9368-6
157. Lynn FC. Meta-regulation: microRNA regulation of glucose and lipid metabolism. Trends Endocrinol Metab. (2009) 20:452–9. doi: 10.1016/j.tem.2009.05.007
158. Chen B, Li H, Zeng X, Yang P, Liu X, Zhao X, et al. Roles of microRNA on cancer cell metabolism. J Transl Med. (2012) 10:228. doi: 10.1186/1479-5876-10-228
159. Korpal M, Lee ES, Hu G, Kang Y. The miR-200 family inhibits epithelial-mesenchymal transition and cancer cell migration by direct targeting of E-cadherin transcriptional repressors ZEB1 and ZEB2. J Biol Chem. (2008) 283:14910–4. doi: 10.1074/jbc.C800074200
160. Burk U, Schubert J, Wellner U, Schmalhofer O, Vincan E, Spaderna S, et al. A reciprocal repression between ZEB1 and members of the miR-200 family promotes EMT and invasion in cancer cells. EMBO Rep. (2008) 9:582–9. doi: 10.1038/embor.2008.74
161. Gregory PA, Bert AG, Paterson EL, Barry SC, Tsykin A, Farshid G, et al. The miR-200 family and miR-205 regulate epithelial to mesenchymal transition by targeting ZEB1 and SIP1. Nat Cell Biol. (2008) 10:593–601. doi: 10.1038/ncb1722
162. Park SM, Gaur AB, Lengyel E, Peter ME. The miR-200 family determines the epithelial phenotype of cancer cells by targeting the E-cadherin repressors ZEB1 and ZEB2. Genes Dev. (2008) 22:894–907. doi: 10.1101/gad.1640608
163. Gibbons DL, Lin W, Creighton CJ, Rizvi ZH, Gregory PA, Goodall GJ, et al. Contextual extracellular cues promote tumor cell EMT and metastasis by regulating miR-200 family expression. Genes Dev. (2009) 23:2140–51. doi: 10.1101/gad.1820209
164. Wu SG, Chang TH, Liu YN, Shih JY. MicroRNA in lung cancer metastasis. Cancers. (2019) 11:265. doi: 10.3390/cancers11020265
165. Saydam O, Shen Y, Würdinger T, Senol O, Boke E, James MF, et al. Downregulated microRNA-200a in meningiomas promotes tumor growth by reducing E-cadherin and activating the Wnt/beta-catenin signaling pathway. Mol Cell Biol. (2009) 29:5923–40. doi: 10.1128/MCB.00332-09
166. Brabletz S, Bajdak K, Meidhof S, Burk U, Niedermann G, Firat E, et al. The ZEB1/miR-200 feedback loop controls Notch signalling in cancer cells. EMBO J. (2011) 30:770–82. doi: 10.1038/emboj.2010.349
167. Hollstein M, Sidransky D, Vogelstein B, Harris CC. p53 mutations in human cancers. Science. (1991) 253:49–53. doi: 10.1126/science.1905840
168. Kim T, Veronese A, Pichiorri F, Lee TJ, Jeon YJ, Volinia S, et al. p53 regulates epithelial-mesenchymal transition through microRNAs targeting ZEB1 and ZEB2. J Exp Med. (2011) 208:875–83. doi: 10.1084/jem.20110235
169. Belgardt BF, Ahmed K, Spranger M, Latreille M, Denzler R, Kondratiuk N, et al. The microRNA-200 family regulates pancreatic beta cell survival in type 2 diabetes. Nat Med. (2015) 21:619–27. doi: 10.1038/nm.3862
170. Chartoumpekis DV, Zaravinos A, Ziros PG, Iskrenova RP, Psyrogiannis AI, Kyriazopoulou VE, et al. Differential expression of microRNAs in adipose tissue after long-term high-fat diet-induced obesity in mice. PLoS ONE. (2012) 7:e34872. doi: 10.1371/journal.pone.0034872
171. Ahmad A, Aboukameel A, Kong D, Wang Z, Sethi S, Chen W, et al. Phosphoglucose isomerase/autocrine motility factor mediates epithelial-mesenchymal transition regulated by miR-200 in breast cancer cells. Cancer Res. (2011) 71:3400–09. doi: 10.1158/0008-5472.CAN-10-0965
172. Clark GR, Sciacovelli M, Gaude E, Walsh DM, Kirby G, Simpson MA, et al. Germline FH mutations presenting with pheochromocytoma. J Clin Endocrinol Metab. (2014) 99:E2046–50. doi: 10.1210/jc.2014-1659
173. Ma L, Young J, Prabhala H, Pan E, Mestdagh P, Muth D, et al. MiR-9, a MYC/MYCN-activated microRNA, regulates E-cadherin and cancer metastasis. Nat Cell Biol. (2010) 12:247–56. doi: 10.1038/ncb2024
174. Gwak JM, Kim HJ, Kim EJ, Chung YR, Yun S, Seo AN, et al. MicroRNA-9 is associated with epithelial-mesenchymal transition, breast cancer stem cell phenotype, and tumor progression in breast cancer. Breast Cancer Res Treat. (2014) 147:39–49. doi: 10.1007/s10549-014-3069-5
175. Song Y, Li J, Zhu Y, Dai Y, Zeng T, Liu L, et al. MicroRNA-9 promotes tumor metastasis via repressing E-cadherin in esophageal squamous cell carcinoma. Oncotarget. (2014) 5:11669–80. doi: 10.18632/oncotarget.2581
176. Zhang J, Xu L, Yang Z, Lu H, Hu D, Li W, et al. MicroRNA-10b indicates a poor prognosis of non-small cell lung cancer and targets E-cadherin. Clin Transl Oncol. (2015) 17:209–14. doi: 10.1007/s12094-014-1213-7
177. Zhang Y, Liao RB, Hu LL, Tong BX, Hao TF, Wu HJ. The microRNA miR-10b as a potentially promising biomarker to predict the prognosis of cancer patients: A meta-analysis. Oncotarget. (2017) 8:104543–51. doi: 10.18632/oncotarget.21428
178. Ma L, Teruya-Feldstein J, Weinberg RA. Tumour invasion and metastasis initiated by microRNA-10b in breast cancer. Nature. (2007) 449:682–8. doi: 10.1038/nature06174
179. Ma L, Reinhardt F, Pan E, Soutschek J, Bhat B, Marcusson EG, et al. Therapeutic silencing of miR-10b inhibits metastasis in a mouse mammary tumor model. Nat Biotechnol. (2010) 28:341–7. doi: 10.1038/nbt.1618
180. Ru P, Steele R, Newhall P, Phillips NJ, Toth K, Ray RB. miRNA-29b suppresses prostate cancer metastasis by regulating epithelial-mesenchymal transition signaling. Mol Cancer Ther. (2012) 11:1166–73. doi: 10.1158/1535-7163.MCT-12-0100
181. Siemens H, Jackstadt R, Hünten S, Kaller M, Menssen A, Götz U, et al. miR-34 and SNAIL form a double-negative feedback loop to regulate epithelial-mesenchymal transitions. Cell Cycle. (2011) 10:4256–71. doi: 10.4161/cc.10.24.18552
182. Moes M, Le Béchec A, Crespo I, Laurini C, Halavatyi A, Vetter G, et al. A novel network integrating a mirna-203/snai1 feedback loop which regulates epithelial to mesenchymal transition. PLoS ONE. (2012) 7:e35440. doi: 10.1371/journal.pone.0035440
183. Liu YN, Yin JJ, Abou-Kheir W, Hynes PG, Casey OM, Fang L, et al. MiR-1 and miR-200 inhibit EMT via Slug-dependent and tumorigenesis via Slug-independent mechanisms. Oncogene. (2013) 32:296–306. doi: 10.1038/onc.2012.58
184. Si ML, Zhu S, Wu H, Lu Z, Wu F, Mo YY. miR-21-mediated tumor growth. Oncogene. (2007) 26:2799–803. doi: 10.1038/sj.onc.1210083
185. Majid S, Dar AA, Saini S, Deng G, Chang I, Greene K, et al. MicroRNA-23b functions as a tumor suppressor by regulating zeb1 in bladder cancer. PLoS ONE. (2013) 8:e67686. doi: 10.1371/journal.pone.0067686
186. Drasin DJ, Guarnieri AL, Neelakantan D, Kim J, Cabrera JH, Wang CA, et al. TWIST1-induced miR-424 reversibly drives mesenchymal programming while inhibiting tumor initiation. Cancer Res. (2015) 75:1908–21. doi: 10.1158/0008-5472.CAN-14-2394
187. Richards EJ, Zhang G, Li ZP, Permuth-Wey J, Challa S, Li Y, et al. Erratum: long non-coding RNAs (LncRNA) regulated by transforming growth factor (TGF) β. LncRNA-hit-mediated TGF-induced epithelial to mesenchymal transition in mammary epithelia. J Biol Chem. (2015) 290:6857–67. doi: 10.1074/jbc.A114.610915
188. Gugnoni M, Ciarrocchi A. Long noncoding RNA and epithelial mesenchymal transition in cancer. Int J Mol Sci. (2019) 20:E1924. doi: 10.3390/ijms20081924
189. Flockhart RJ, Webster DE, Qu K, Mascarenhas N, Kovalski J, Kretz M, et al. BRAFV600E remodels the melanocyte transcriptome and induces BANCR to regulate melanoma cell migration. Genome Res. (2012) 22:1006–14. doi: 10.1101/gr.140061.112
190. Guo F, Parker Kerrigan BC, Yang D, Hu L, Shmulevich I, Sood AK, et al. Post-transcriptional regulatory network of epithelial-to-mesenchymal and mesenchymal-to-epithelial transitions. J Hematol Oncol. (2014) 7:19. doi: 10.1186/1756-8722-7-19
191. Lou KX, Li ZH, Wang P, Liu Z, Chen Y, Wang XL, et al. Long non-coding RNA BANCR indicates poor prognosis for breast cancer and promotes cell proliferation and invasion. Eur Rev Med Pharmacol Sci. (2018) 22:1358–65. doi: 10.26355/eurrev_201803_14479
192. Wang Y, Gu J, Lin X, Yan W, Yang W, Wu G. lncRNA BANCR promotes EMT in PTC via the Raf/MEK/ERK signaling pathway. Oncol Lett. (2018) 15:5865–70. doi: 10.3892/ol.2018.8017
193. Huang JF, Guo YJ, Zhao CX, Yuan SX, Wang Y, Tang GN, et al. Hepatitis B virus X protein (HBx)-related long noncoding RNA (lncRNA) down-regulated expression by HBx (Dreh) inhibits hepatocellular carcinoma metastasis by targeting the intermediate filament protein vimentin. Hepatology. (2013) 57:1882–92. doi: 10.1002/hep.26195
194. Sun Y, Daemen A, Hatzivassiliou G, Arnott D, Wilson C, Zhuang G, et al. Metabolic and transcriptional profiling reveals pyruvate dehydrogenase kinase 4 as a mediator of epithelial-mesenchymal transition and drug resistance in tumor cells. Cancer Metab. (2014) 2:20. doi: 10.1186/2049-3002-2-20
195. Wang Y, Liu Z, Yao B, Dou C, Xu M, Xue Y, et al. Long non-coding RNA TUSC7 acts a molecular sponge for miR-10a and suppresses EMT in hepatocellular carcinoma. Tumor Biol. (2016) 37:11429–41. doi: 10.1007/s13277-016-4892-6
196. Lv D, Wang Y, Zhang Y, Cui P, Xu Y. Downregulated long non-coding RNA DREH promotes cell proliferation in hepatitis B virus-associated hepatocellular carcinoma. Oncol Lett. (2017) 14:2025–32. doi: 10.3892/ol.2017.6436
197. Farooqi AA, Attar R, Qureshi MZ, Fayyaz S, Sohail MI, Sabitaliyevich UY, et al. Interplay of long non-coding RNAs and TGF/SMAD signaling in different cancers. Cell Mol Biol. (2018) 64:1–6. doi: 10.14715/cmb/2017.64.15.1
198. Li J, Wang J, Chen Y, Li S, Jin M, Wang H, et al. LncRNA MALAT1 exerts oncogenic functions in lung adenocarcinoma by targeting miR-204. Am J Cancer Res. (2016) 6:1099−107.
199. Beltran M, Puig I, Peña C, García JM, Álvarez AB, Peña R, Bonilla F, et al. A natural antisense transcript regulates Zeb2/Sip1 gene expression during Snail1-induced epithelial-mesenchymal transition. Genes Dev. (2008) 22:756–69. doi: 10.1101/gad.455708
200. Liu C, Lin J. Long noncoding RNA ZEB1-AZS1 acts as an oncogene in osteosarcoma by epigenetically activating ZEB1. Am J Transl Res. (2016) 8:4095−105.
201. Xiao C, Wu CH, Hu HZ. LncRNA UCA1 promotes epithelial-mesenchymal transition (EMT) of breast cancer cells via enhancing Wnt/beta-catenin signaling pathway. Eur Rev Med Pharmacol Sci. (2016) 20:2819−24.
202. Matouk IJ, Raveh E, Abu-lail R, Mezan S, Gilon M, Gershtain E, et al. Oncofetal H19 RNA promotes tumor metastasis. Biochim Biophys Acta Mol Cell Res. (2014) 1843:1414–26. doi: 10.1016/j.bbamcr.2014.03.023
203. Liang WC, Fu WM, Wong CW, Wang Y, Wang WM, Hu GX, et al. The LncRNA H19 promotes epithelial to mesenchymal transition by functioning as MiRNA sponges in colorectal cancer. Oncotarget. (2015) 6:22513–25. doi: 10.18632/oncotarget.4154
204. Zhao XY, Lin JD. Long noncoding RNAs: a new regulatory code in metabolic control. Trends Biochem Sci. (2015) 40:586–96. doi: 10.1016/j.tibs.2015.08.002
205. Rupaimoole R, Lee J, Haemmerle M, Ling H, Previs RA, Pradeep S, et al. Long noncoding RNA ceruloplasmin promotes cancer growth by altering glycolysis. Cell Rep. (2015) 13:2395–402. doi: 10.1016/j.celrep.2015.11.047
206. Liberti MV, Locasale JW. The Warburg Effect: how does it benefit cancer cells? Trends Biochem Sci. (2016) 41:211–8. doi: 10.1016/j.tibs.2015.12.001
207. Yu L, Lu M, Jia D, Ma J, Ben-Jacob E, Levine H, et al. Modeling the genetic regulation of cancer metabolism: interplay between glycolysis and oxidative phosphorylation. Cancer Res. (2017) 77:1564–74. doi: 10.1158/0008-5472.CAN-16-2074
208. Jia D, Lu M, Jung KH, Park JH, Yu L, Onuchic JN, et al. Elucidating cancer metabolic plasticity by coupling gene regulation with metabolic pathways. Proc Natl Acad Sci USA. (2019) 116:3909–18. doi: 10.1073/pnas.1816391116
209. LeBleu VS, O'Connell JT, Gonzalez Herrera KN, Wikman H, Pantel K, Haigis MC, et al. PGC-1alpha mediates mitochondrial biogenesis and oxidative phosphorylation in cancer cells to promote metastasis. Nat Cell Biol. (2014) 16:992–1003:1–15. doi: 10.1038/ncb3039
210. Porporato PE, Payen VL, Perez-Escuredo J, De Saedeleer CJ, Danhier P, Copetti T, et al. A mitochondrial switch promotes tumor metastasis. Cell Rep. (2014) 8:754–66. doi: 10.1016/j.celrep.2014.06.043
211. Shaul YD, Freinkman E, Comb WC, Cantor JR, Tam WL, Thiru P, et al. Dihydropyrimidine accumulation is required for the epithelial-mesenchymal transition. Cell. (2014) 158:1094–109. doi: 10.1016/j.cell.2014.07.032
212. Dong C, Yuan T, Wu Y, Wang Y, T.Fan WM, Miriyala S, et al. Loss of FBP1 by snail-mediated repression provides metabolic advantages in basal-like breast cancer. Cancer Cell. (2013) 23:316–31. doi: 10.1016/j.ccr.2013.01.022
213. Li J, Wang Y, Li QG, Xue JJ, Wang Z, Yuan X, et al. Downregulation of FBP1 promotes tumor metastasis and indicates poor prognosis in gastric cancer via regulating epithelial-mesenchymal transition. PLoS ONE. (2016) 11:e0167857. doi: 10.1371/journal.pone.0167857
214. Son B, Lee S, Kim H, Kang H, Jeon J, Jo S, et al. Decreased FBP1 expression rewires metabolic processes affecting aggressiveness of glioblastoma. Oncogene. (2019) 39:36–49. doi: 10.1038/s41388-019-0974-4.
215. Marin-Hernandez A, Gallardo-Perez J, Ralph S, Rodriguez-Enriquez S, Moreno-Sanchez R. HIF-1alpha modulates energy metabolism in cancer cells by inducing over-expression of specific glycolytic isoforms. Mini Rev Med Chem. (2009) 9:1084–101. doi: 10.2174/138955709788922610
216. Semenza GL. HIF-1 mediates metabolic responses to intratumoral hypoxia and oncogenic mutations. J Clin Invest. (2013)123:3664–71. doi: 10.1172/JCI67230
217. Huang S, Pan Y, Zhang Q, Sun W. Role of CD200/CD200R signaling pathway in regulation of CD4+T cell subsets during thermal ablation of hepatocellular carcinoma. Med Sci Monitor. (2019) 25:1718–28. doi: 10.12659/MSM.913094
218. Patra KC, Wang Q, Bhaskar PT, Miller L, Wang Z, Wheaton W, et al. Hexokinase 2 is required for tumor initiation and maintenance and its systemic deletion is therapeutic in mouse models of cancer. Cancer Cell. (2013) 24:213–28. doi: 10.1016/j.ccr.2013.06.014
219. DeWaal D, Nogueira V, Terry AR, Patra KC, Jeon SM, Guzman G, et al. Hexokinase-2 depletion inhibits glycolysis and induces oxidative phosphorylation in hepatocellular carcinoma and sensitizes to metformin. Nat Commun. (2018) 9:446. doi: 10.1038/s41467-018-04182-z
220. Chen G, Zhang Y, Liang J, Li W, Zhu Y, Zhang M, et al. Deregulation of hexokinase II is associated with glycolysis, autophagy, and the epithelial-mesenchymal transition in tongue squamous cell carcinoma under hypoxia. BioMed Res Int. (2018) 2018:1–15. doi: 10.1155/2018/8480762
221. Pudova EA, Kudryavtseva AV, Fedorova MS, Zaretsky AR, Shcherbo DS, Lukyanova EN, et al. HK3 overexpression associated with epithelial-mesenchymal transition in colorectal cancer. BMC Genomics. (2018) 19:113. doi: 10.1186/s12864-018-4477-4
222. Leclerc D, D.Pham NT, Lévesque N, Truongcao M, Foulkes WD, Sapienza C, et al. Oncogenic role of PDK4 in human colon cancer cells. Br J Cancer. (2017) 116:930–6. doi: 10.1038/bjc.2017.38
223. Woolbright BL, Choudhary D, Mikhalyuk A, Trammel C, Shanmugam S, Abbott E, et al. The role of pyruvate dehydrogenase kinase-4 (PDK4) in bladder cancer and chemoresistance. Mol Cancer Ther. (2018) 17:2004–12. doi: 10.1158/1535-7163.MCT-18-0063
224. Wong N, Ojo D, Yan J, Tang D. PKM2 contributes to cancer metabolism. Cancer Lett. (2015) 356:184–91. doi: 10.1016/j.canlet.2014.01.031
225. Hamabe A, Konno M, Tanuma N, Shima H, Tsunekuni K, Kawamoto K, et al. Role of pyruvate kinase M2 in transcriptional regulation leading to epithelial-mesenchymal transition. Proc Natl Acad Sci USA. (2014) 111:15526–31. doi: 10.1073/pnas.1407717111
226. Li C, Zhao Z, Zhou Z, Liu R. PKM2 promotes cell survival and invasion under metabolic stress by enhancing warburg effect in pancreatic ductal adenocarcinoma. Dig Dis Sci. (2016) 61:767–73. doi: 10.1007/s10620-015-3931-2
227. Chang GC, Liu KJ, Hsieh CL, Hu TS, Charoenfuprasert S, Liu HK, et al. Identification of α-enolase as an autoantigen in lung cancer: its overexpression is associated with clinical outcomes. Clin Cancer Res. (2006) 12:5746–54. doi: 10.1158/1078-0432.CCR-06-0324
228. Tsai ST, Chien IH, Shen WH, Kuo YZ, Jin YT, Wong TY, et al. ENO1, a potential prognostic head and neck cancer marker, promotes transformation partly via chemokine CCL20 induction. Eur J Cancer. (2010) 46:1712–23. doi: 10.1016/j.ejca.2010.03.018
229. Zhou J, Zhang S, Chen Z, He Z, Xu Y, Li Z. CircRNA-ENO1 promoted glycolysis and tumor progression in lung adenocarcinoma through upregulating its host gene ENO1. Cell Death Dis. (2019) 10:885. doi: 10.1038/s41419-019-2127-7
230. Yan GR, Xu SH, Tan ZL, Yin XF, He QY. Proteomics characterization of gastrokine 1-induced growth inhibition of gastric cancer cells. Proteomics. (2011) 11:3657–64. doi: 10.1002/pmic.201100215
231. Niinaka Y, Paku S, Haga A, Watanabe H, Raz A. Expression and secretion of neuroleukin/phosphohexose isomerase/maturation factor as autocrine motility factor by tumor cells. Cancer Res. (1998) 58:2667–74.
232. Funasaka T, Hu A, Yanagawa T, Hogan V, Raz A. Down-regulation of phosphoglucose isomerase/autocrine motility factor results in mesenchymal-to-epithelial transition of human lung fibrosarcoma cells. Cancer Res. (2007) 67:4236–43. doi: 10.1158/0008-5472.CAN-06-3935
233. Chang YC, Yang YC, Tien CP, Yang CJ, Hsiao M. Roles of aldolase family genes in human cancers and diseases. Trends Endocrinol Metab. (2018) 29:549–59. doi: 10.1016/j.tem.2018.05.003
234. Du S, Guan Z, Hao L, Song Y, Wang L, Gong L, et al. Fructose-bisphosphate aldolase a is a potential metastasis-associated marker of lung squamous cell carcinoma and promotes lung cell tumorigenesis and migration. PLoS ONE. (2014) 9:e85804. doi: 10.1371/journal.pone.0085804
235. Ye F, Chen Y, Xia L, Lian J, Yang S. Aldolase A overexpression is associated with poor prognosis and promotes tumor progression by the epithelial-mesenchymal transition in colon cancer. Biochem Biophys Res Commun. (2018) 497:639–45. doi: 10.1016/j.bbrc.2018.02.123
236. Ji S, Zhang B, Liu J, Qin Y, Liang C, Shi S, et al. ALDOA functions as an oncogene in the highly metastatic pancreatic cancer. Cancer Lett. (2016) 374:127–35. doi: 10.1016/j.canlet.2016.01.054
237. Li J, Wang F, Gao H, Huang S, Cai F, Sun J. ALDOLASE A regulates invasion of bladder cancer cells via E-cadherin-EGFR signaling. J Cell Biochem. (2019) 120:13694–705. doi: 10.1002/jcb.28642
238. Krzeslak A, Wojcik-Krowiranda K, Forma E, Jozwiak P, Romanowicz H, Bienkiewicz A, et al. Expression of GLUT1 and GLUT3 glucose transporters in endometrial and breast cancers. Pathol Oncol Res. (2012) 18:721–8. doi: 10.1007/s12253-012-9500-5
239. Yu M, Yongzhi H, Chen S, Luo X, Lin Y, Zhou Y, et al. The prognostic value of GLUT1 in cancers: a systematic review and meta-analysis. Oncotarget. (2017) 8:43356–67. doi: 10.18632/oncotarget.17445
240. Zuo J, Wen J, Lei M, Wen M, Li S, Lv X, et al. Hypoxia promotes the invasion and metastasis of laryngeal cancer cells via EMT. Med Oncol. (2016) 33:1–9. doi: 10.1007/s12032-015-0716-6
241. Masin M, Vazquez J, Rossi S, Groeneveld S, Samson N, Schwalie PC, et al. GLUT3 is induced during epithelial-mesenchymal transition and promotes tumor cell proliferation in non-small cell lung cancer. Cancer Metab. (2014) 2:11. doi: 10.1186/2049-3002-2-11
242. Alam NA, Olpin S, Leigh IM. Fumarate hydratase mutations and predisposition to cutaneous leiomyomas, uterine leiomyomas and renal cancer. Br J Dermatol. (2005) 153:11–7. doi: 10.1111/j.1365-2133.2005.06678.x
243. Stratakis CA, Carney JA. The triad of paragangliomas, gastric stromal tumours and pulmonary chondromas (Carney triad), and the dyad of paragangliomas and gastric stromal sarcomas (Carney-Stratakis syndrome): molecular genetics and clinical implications. J Intern Med. (2009) 266:43–52. doi: 10.1111/j.1365-2796.2009.02110.x
244. Letouzé E, Martinelli C, Loriot C, Burnichon N, Abermil N, Ottolenghi C, et al. SDH mutations establish a hypermethylator phenotype in paraganglioma. Cancer Cell. (2013) 23:739–52. doi: 10.1016/j.ccr.2013.04.018
245. Loriot C, Burnichon N, Gadessaud N, Vescovo L, Amar L, Libé R, et al. Epithelial to mesenchymal transition is activated in metastatic pheochromocytomas and paragangliomas caused by SDHB gene mutations. J Clin Endocrinol Metab. (2012) 97:E954–62. doi: 10.1210/jc.2011-3437
246. Li J, Liang N, Long X, Zhao J, Yang J, Du X, et al. SDHC-related deficiency of SDH complex activity promotes growth and metastasis of hepatocellular carcinoma via ROS/NFκB signaling. Cancer Lett. (2019) 461:44–55. doi: 10.1016/j.canlet.2019.07.001
247. Røsland GV, Dyrstad SE, Tusubira D, Helwa R, Tan TZ, Lotsberg ML, et al. Epithelial to mesenchymal transition (EMT) is associated with attenuation of succinate dehydrogenase (SDH) in breast cancer through reduced expression of SDHC. Cancer Metab. (2019) 7:6. doi: 10.1186/s40170-019-0197-8
248. Reitman ZJ, Yan H. Isocitrate dehydrogenase 1 and 2 mutations in cancer: alterations at a crossroads of cellular metabolism. Natl Cancer Inst. (2010) 102:932–41. doi: 10.1093/jnci/djq187
249. Yan H, Parsons DW, Jin G, McLendon R, Rasheed BA, Yuan W, et al. IDH1 and IDH2 mutations in gliomas. N Engl J Med. (2009) 360:765–73. doi: 10.1056/NEJMoa0808710
250. Mardis ER, Ding L, Dooling DJ, Larson DE, McLellan MD, Chen K, et al. Recurring mutations found by sequencing an acute myeloid leukemia genome. N Engl J Med. (2009) 361:1058–66. doi: 10.1056/NEJMoa0903840
251. Colvin H, Nishida N, Konno M, Haraguchi N, Takahashi H, Nishimura J, et al. Oncometabolite D-2-hydroxyglurate directly induces epithelial-mesenchymal transition and is associated with distant metastasis in colorectal cancer. Sci Rep. (2016) 6:36289. doi: 10.1038/srep36289
252. Swinnen JV, Brusselmans K, Verhoeven G. Increased lipogenesis in cancer cells: new players, novel targets. Curr Opin Clin Nutr Metab Care. (2006) 9:358–65. doi: 10.1097/01.mco.0000232894.28674.30
253. Milgraum LZ, Witters LA, Pasternack GR, Kuhajda FP. Enzymes of the fatty acid synthesis pathway are highly expressed in in situ breast carcinoma. Clin Cancer Res. (1997) 3:2115–20.
254. Ye B, Yin L, Wang Q, Cunshuan XU. ACC1 is overexpressed in liver cancers and contributes to the proliferation of human hepatoma Hep G2 cells and the rat liver cell line BRL 3A. Mol Med Rep. (2019) 49:3431–40. doi: 10.3892/mmr.2019.9994
255. Kim J, Deberardinis RJ. Blocking fatty acid synthesis reduces lung tumor growth in mice. Nat Med. (2016) 22:1077–8. doi: 10.1038/nm.4195
256. Rios Garcia M, Steinbauer B, Srivastava K, Singhal M, Mattijssen F, Maida A, et al. Acetyl-CoA carboxylase 1-dependent protein acetylation controls breast cancer metastasis and recurrence. Cell Metab. (2017) 26:842−55.e5. doi: 10.1016/j.cmet.2017.09.018
257. Ye Q, Chung LW, Li S, Zhau HE. Identification of a novel FAS/ER-alpha fusion transcript expressed in human cancer cells. Biochim Biophys Acta. (2000) 1493:373–7. doi: 10.1016/S0167-4781(00)00202-5
258. Alo PL, Visca P, Marci A, Mangoni A, Botti C, Di Tondo U. Expression of fatty acid synthase (FAS) as a predictor of recurrence in stage I breast carcinoma patients. Cancer. (1996) 77:474–82. doi: 10.1002/(SICI)1097-0142(19960201)77:3<474::AID-CNCR8>3.0.CO
259. Gansler TS, Hardman W, Hunt DA, Schaffel S, Hennigar RA. Increased expression of fatty acid synthase (OA-519) in ovarian neoplasms predicts shorter survival. Human Pathol. (1997) 28:686–92. doi: 10.1016/S0046-8177(97)90177-5
260. Visca P, Sebastiani V, Botti C, Diodoro MG, Lasagni RP, Romagnoli F, et al. Fatty Acid Synthase (FAS) is a marker of increased risk of recurrence in lung carcinoma. Anticancer Res. (2004) 24:4169−73.
261. Li J, Dong L, Wei D, Wang X, Zhang S, Li H. Fatty acid synthase mediates the epithelial-mesenchymal transition of breast cancer cells. Int J Biol Sci. (2014) 10:171–80. doi: 10.7150/ijbs.7357
262. Huang J, Fan XX, He J, Pan H, Li RZ, Huang L, et al.Leung LH, He JX. SCD1 is associated with tumor promotion, late stage and poor survival in lung adenocarcinoma. Oncotarget. (2016) 7:39970–9. doi: 10.18632/oncotarget.9461
263. Mauvoisin D, Charfi C, Lounis AM, Rassart E, Mounier C. Decreasing stearoyl-CoA desaturase-1 expression inhibits β-catenin signaling in breast cancer cells. Cancer Sci. (2013) 104:36–42. doi: 10.1111/cas.12032
264. Hatzivassiliou G, Zhao F, Bauer DE, Andreadis C, Shaw AN, Dhanak D, et al. ATP citrate lyase inhibition can suppress tumor cell growth. Cancer Cell. (2005) 8:311–21. doi: 10.1016/j.ccr.2005.09.008
265. Zaidi N, Swinnen JV, Smans K. ATP-citrate lyase: a key player in cancer metabolism. Cancer Res. (2012) 72:3709–14. doi: 10.1158/0008-5472.CAN-11-4112
266. Wen J, Min X, Shen M, Hua Q, Han Y, Zhao L, et al. ACLY facilitates colon cancer cell metastasis by CTNNB1. J Exp Clin Cancer Res. (2019) 38:401. doi: 10.1186/s13046-019-1391-9
267. Fu Y, Lu R, Cui J, Sun H, Yang H, Meng Q, et al. Inhibition of ATP citrate lyase (ACLY) protects airway epithelia from PM 2.5 -induced epithelial-mesenchymal transition. Ecotoxicol Environ Safety. (2019) 167:309–16. doi: 10.1016/j.ecoenv.2018.10.033
268. Hanai JI, Doro N, Sasaki AT, Kobayashi S, Cantley LC, Seth P, et al. Inhibition of lung cancer growth: ATP citrate lyase knockdown and statin treatment leads to dual blockade of mitogen-activated protein Kinase (MAPK) and Phosphatidylinositol-3-kinase (PI3K)/AKT pathways. J Cell Physiol. (2012) 227:1709–20. doi: 10.1002/jcp.22895
269. Chen WC, Wang CY, Hung YH, Weng TY, Yen MC, Lai MD. Systematic analysis of gene expression alterations and clinical outcomes for long-chain acyl-coenzyme A synthetase family in cancer. PLoS ONE. (2016) 11:e0155660. doi: 10.1371/journal.pone.0155660
270. Wilson KE, Bachawal SV, Tian L, Willmann JK. Multiparametric spectroscopic photoacoustic imaging of breast cancer development in a transgenic mouse model. Theranostics. (2014) 4:1062–71. doi: 10.7150/thno.9922
271. Migita T, Takayama KI, Urano T, Obinata D, Ikeda K, Soga T, et al. ACSL3 promotes intratumoral steroidogenesis in prostate cancer cells. Cancer Sci. (2017) 108:2011–21. doi: 10.1111/cas.13339
272. Sebastiano MR, Konstantinidou G. Targeting long chain acyl-coa synthetases for cancer therapy. Int J Mol Sci. (2019) 20:3624. doi: 10.3390/ijms20153624
273. Cruz-Gil S, Sanchez-Martinez R, De Cedron MG, Martin-Hernandez R, Vargas T, Molina S, et al. Targeting the lipid metabolic axis ACSL/SCD in colorectal cancer progression by therapeutic miRNAs: MiR-19b-1 role. J Lipid Res. (2018) 59:14–24. doi: 10.1194/jlr.M076752
274. Sánchez-Martínez R, Cruz-Gil S, García-Álvarez MS, Reglero G, De Molina AR. Complementary ACSL isoforms contribute to a non-Warburg advantageous energetic status characterizing invasive colon cancer cells. Sci Rep. (2017) 7:11143. doi: 10.1038/s41598-017-11612-3
275. Kim SJ, Chung TW, Choi HJ, Kwak CH, Song KH, Suh SJ, et al. Ganglioside GM3 participates in the TGF-β1-induced epithelial-mesenchymal transition of human lens epithelial cells. Biochem J. (2013) 449:241–51. doi: 10.1042/BJ20120189
276. Lukey MJ, Katt WP, Cerione RA. Targeting amino acid metabolism for cancer therapy. Drug Discov Today. (2017) 22:796–804. doi: 10.1016/j.drudis.2016.12.003
277. Jain M, Nilsson R, Sharma S, Madhusudhan N, Kitami T, Souza AL, et al. Metabolite profiling identifies a key role for glycine in rapid cancer cell proliferation. Science. (2012) 336:1040–4. doi: 10.1126/science.1218595
278. Yang L, Venneti S, Nagrath D. Glutaminolysis: a hallmark of cancer metabolism. Ann Rev Biomed Eng. (2017) 19:163–94. doi: 10.1146/annurev-bioeng-071516-044546
279. Lee SY, Jeon HM, Ju MK, Jeong EK, Kim CH, Park HG, et al. Dlx-2 and glutaminase upregulate epithelial-mesenchymal transition and glycolytic switch. Oncotarget. (2016) 7:7925–39. doi: 10.18632/oncotarget.6879
280. Ramirez-Peña E, Arnold J, Shivakumar V, Joseph R, Vidhya Vijay G, den Hollander P, et al. The epithelial to mesenchymal transition promotes glutamine independence by suppressing GLS2 expression. Cancers. (2019) 11:1610. doi: 10.3390/cancers11101610
281. S.Knott RV, Wagenblast E, Khan S, Kim SY, Soto M, Wagner M, et al. Asparagine bioavailability governs metastasis in a model of breast cancer. Nature. (2018) 554:378–81. doi: 10.1038/nature25465
282. Marty-Double CH, Pignodel CH. [Juvenile aponeurotic fibroma (Keasbey's tumor) may have an unfavorable course]. Presse Med. (1985) 14:1971.
283. Combs JA, Denicola GM. The non-essential amino acid cysteine becomes essential for tumor proliferation and survival. Cancers. (2019) 11:678. doi: 10.3390/cancers11050678
284. Tang X, Ding CK, Wu J, Sjol J, Wardell S, Spasojevic I, et al. Cystine addiction of triple-negative breast cancer associated with EMT augmented death signaling. Oncogene. (2017) 36:4235–42. doi: 10.1038/onc.2016.394
285. Revenco T, Nicodème A, Pastushenko I, Sznurkowska MK, Latil M, Sotiropoulou PA, et al. Context dependency of epithelial-to-mesenchymal transition for metastasis. Cell Rep. (2019) 29:1458−68.e3. doi: 10.1016/j.celrep.2019.09.081
286. Shintani Y, Okimura A, Sato K, Nakagiri T, Kadota Y, Inoue M, et al. Epithelial to mesenchymal transition is a determinant of sensitivity to chemoradiotherapy in non-small cell lung cancer. Ann Thorac Surg. (2011) 92:1794–804. doi: 10.1016/j.athoracsur.2011.07.032
287. Smith B, Bhowmick N. Role of EMT in metastasis and therapy resistance. J Clin Med. (2016) 5:17. doi: 10.3390/jcm5020017
288. da Fonseca LM, da Silva VA, Freire-de-Lima L, Previato JO, Mendonça-Previato L, Capella MAM. Glycosylation in cancer: Interplay between multidrug resistance and epithelial-to-mesenchymal transition? Front Oncol. (2016) 6:158. doi: 10.3389/fonc.2016.00158
289. Halder SK, Beauchamp RD, Datta PK. A specific inhibitor of TGF-β receptor kinase, SB-431542, as a potent antitumor agent for human cancers. Neoplasia. (2005) 7:509–21. doi: 10.1593/neo.04640
290. Lo HW, Hsu SC, Xia W, Cao X, Shih JY, Wei Y, et al. Epidermal growth factor receptor cooperates with signal transducer and activator of transcription 3 to induce epithelial-mesenchymal transition in cancer cells via up-regulation of TWIST gene expression. Cancer Res. (2007) 67:9066–76. doi: 10.1158/0008-5472.CAN-07-0575
291. Holland SJ, Pan A, Franci C, Hu Y, Chang B, Li W, et al. R428, a selective small molecule inhibitor of Axl kinase, blocks tumor spread and prolongs survival in models of metastatic breast cancer. Cancer Res. (2010) 70:1544–54. doi: 10.1158/0008-5472.CAN-09-2997
292. Morris JC, Tan AR, Olencki TE, Shapiro GI, Dezube BJ, Reiss M, et al. Phase I study of GC1008 (Fresolimumab): a human anti-transforming growth factor-beta (TGFβ) monoclonal antibody in patients with advanced malignant melanoma or renal cell carcinoma. PLoS ONE. (2014) 9:e90353. doi: 10.1371/journal.pone.0090353
293. Lacouture ME, Morris JC, Lawrence DP, Tan AR, Olencki TE, Shapiro GI, et al. Cutaneous keratoacanthomas/squamous cell carcinomas associated with neutralization of transforming growth factor β by the monoclonal antibody fresolimumab (GC1008). Cancer Immunol Immunother. (2015) 64:437–46. doi: 10.1007/s00262-015-1653-0
294. Cho ES, Kang HE, Kim NH, Yook JI. Therapeutic implications of cancer epithelial-mesenchymal transition (EMT). Arch Pharm Res. (2019) 42:14–24. doi: 10.1007/s12272-018-01108-7
295. Palagani V, El Khatib M, Kossatz U, Bozko P, Müller MR, Manns MP, et al. Epithelial mesenchymal transition and pancreatic tumor initiating CD44+/EpCAM+ cells are inhibited by γ-secretase inhibitor IX. PLoS ONE. (2012) 7:e46514. doi: 10.1371/journal.pone.0046514
296. Thaiparambil JT, Bender L, Ganesh T, Kline E, Patel P, Liu Y, et al. Withaferin A inhibits breast cancer invasion and metastasis at sub-cytotoxic doses by inducing vimentin disassembly and serine 56 phosphorylation. Int J Cancer. (2011) 129:2744–55. doi: 10.1002/ijc.25938
297. Tanaka H, Kono E, Tran CP, Miyazaki H, Yamashiro J, Shimomura T, et al. Monoclonal antibody targeting of N-cadherin inhibits prostate cancer growth, metastasis and castration resistance. Nat Med. (2010) 16:1414–21. doi: 10.1038/nm.2236
298. Li Y, Vandenboom TG, Kong D, Wang Z, Ali S, Philip PA, et al. Up-regulation of miR-200 and let-7 by natural agents leads to the reversal of epithelial-to-mesenchymal transition in gemcitabine-resistant pancreatic cancer cells. Cancer Res. (2009) 69:6704–12. doi: 10.1158/0008-5472.CAN-09-1298
299. Shi Z, Zhang J, Qian X, Han L, Zhang K, Chen L, et al. AC1MMYR2, an inhibitor of dicer-mediated biogenesis of oncomir miR-21, reverses epithelial-mesenchymal transition and suppresses tumor growth and progression. Cancer Res. (2013) 73:5519–31. doi: 10.1158/0008-5472.CAN-13-0280
300. Xu Q, Deng F, Qin Y, Zhao Z, Wu Z, Xing Z, et al. Long non-coding rna regulation of epithelial- mesenchymal transition in cancer metastasis. Cell Death Dis. (2016) 7:e2254. doi: 10.1038/cddis.2016.149
301. Morandi A, Taddei ML, Chiarugi P, Giannoni E. Targeting the metabolic reprogramming that controls epithelial-to-mesenchymal transition in aggressive tumors. Front Oncol. (2017) 7:40. doi: 10.3389/fonc.2017.00040
302. Luengo A, Gui DY, Vander Heiden MG. Targeting metabolism for cancer therapy. Cell Chem Biol. (2017) 24:1161–80. doi: 10.1016/j.chembiol.2017.08.028
303. Ganapathy-Kanniappan S. Taming tumor glycolysis and potential implications for immunotherapy. Front Oncol. (2017) 7:36. doi: 10.3389/fonc.2017.00036
304. Akins NS, Nielson TC, Le HV. Inhibition of glycolysis and glutaminolysis: an emerging drug discovery approach to combat cancer. Curr Topics Med Chem. (2018) 18:494–504. doi: 10.2174/1568026618666180523111351
305. Wood TE, Dalili S, Simpson CD, Hurren R, Mao X, Saiz FS, et al. A novel inhibitor of glucose uptake sensitizes cells to FAS-induced cell death. Mol Cancer Ther. (2008) 7:3546–55. doi: 10.1158/1535-7163.MCT-08-0569
306. Abdel-Wahab AF, Mahmoud W, Al-Harizy RM. Targeting glucose metabolism to suppress cancer progression: prospective of anti-glycolytic cancer therapy. Pharmacol Res. (2019) 150:104511. doi: 10.1016/j.phrs.2019.104511
307. Hsieh IS, Gopula B, Chou CC, Wu HY, Chang GD, Wu WJ, et al. Development of novel irreversible pyruvate kinase M2 inhibitors. J Med Chem. (2019) 62:8497–510. doi: 10.1021/acs.jmedchem.9b00763
308. Liu X, Gong Y. Isocitrate dehydrogenase inhibitors in acute myeloid leukemia. Biomark Res. (2019) 7:22. doi: 10.1186/s40364-019-0173-z
309. Mizojiri R, Asano M, Sasaki M, Satoh Y, Yamamoto Y, Sumi H, et al. The identification and pharmacological evaluation of potent, selective and orally available ACC1 inhibitor. Bioorg Med Chem Lett. (2019) 29:126749. doi: 10.1016/j.bmcl.2019.126749
310. Tracz-Gaszewska Z, Dobrzyn P. Stearoyl-CoA desaturase 1 as a therapeutic target for the treatment of cancer. Cancers (Basel). (2019) 11:pii: E948. doi: 10.3390/cancers11070948
311. Wei J, Leit S, Kuai J, Therrien E, Rafi S, Harwood HJ, et al. An allosteric mechanism for potent inhibition of human ATP-citrate lyase. Nature. (2019) 568:566–70. doi: 10.1038/s41586-019-1094-6
312. Steinway SN, J.Zanudo GT, Michel PJ, Feith DJ, Loughran TP, Albert R. Combinatorial interventions inhibit TGFbeta-driven epithelial-to-mesenchymal transition and support hybrid cellular phenotypes. NPJ Syst Biol Appl. (2015) 1:15014. doi: 10.1038/npjsba.2015.14
313. Huang B, Lu M, Jia D, Ben-Jacob E, Levine H, Onuchic JN. Interrogating the topological robustness of gene regulatory circuits by randomization. PLoS Comput Biol. (2017) 13:e1005456. doi: 10.1371/journal.pcbi.1005456
314. Font-Clos F, Zapperi S, C.La Porta AM. Topography of epithelial-mesenchymal plasticity. Proc Natl Acad Sci USA. (2018) 115:5902–7. doi: 10.1073/pnas.1722609115
Keywords: EMT, metabolic pathways, transcription factors, non-coding RNAs, cancer metabolism
Citation: Georgakopoulos-Soares I, Chartoumpekis DV, Kyriazopoulou V and Zaravinos A (2020) EMT Factors and Metabolic Pathways in Cancer. Front. Oncol. 10:499. doi: 10.3389/fonc.2020.00499
Received: 07 January 2020; Accepted: 19 March 2020;
Published: 07 April 2020.
Edited by:
Katarína Smolková, Institute of Physiology (ASCR), CzechiaReviewed by:
Joanna Maria Boncela, Institute for Medical Biology (PAN), PolandCopyright © 2020 Georgakopoulos-Soares, Chartoumpekis, Kyriazopoulou and Zaravinos. This is an open-access article distributed under the terms of the Creative Commons Attribution License (CC BY). The use, distribution or reproduction in other forums is permitted, provided the original author(s) and the copyright owner(s) are credited and that the original publication in this journal is cited, in accordance with accepted academic practice. No use, distribution or reproduction is permitted which does not comply with these terms.
*Correspondence: Apostolos Zaravinos, YXphcmF2aW5vc0BxdS5lZHUucWE=
Disclaimer: All claims expressed in this article are solely those of the authors and do not necessarily represent those of their affiliated organizations, or those of the publisher, the editors and the reviewers. Any product that may be evaluated in this article or claim that may be made by its manufacturer is not guaranteed or endorsed by the publisher.
Research integrity at Frontiers
Learn more about the work of our research integrity team to safeguard the quality of each article we publish.