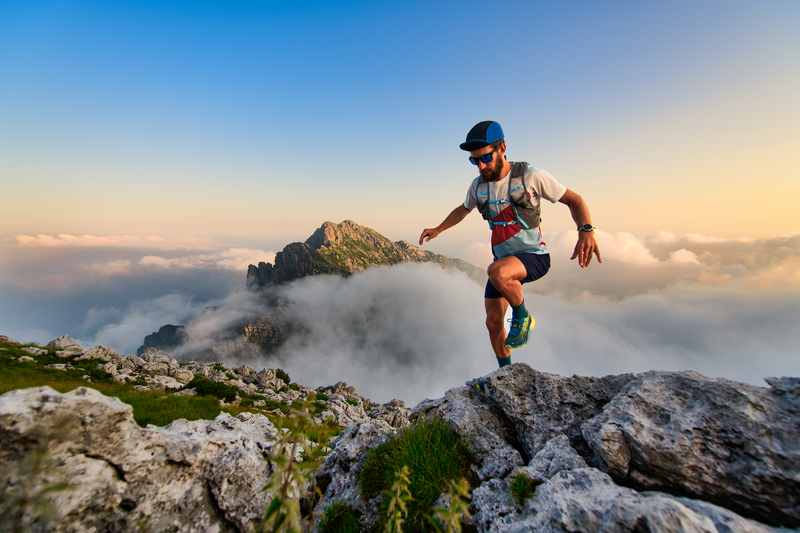
95% of researchers rate our articles as excellent or good
Learn more about the work of our research integrity team to safeguard the quality of each article we publish.
Find out more
REVIEW article
Front. Oncol. , 31 January 2020
Sec. Cancer Genetics
Volume 10 - 2020 | https://doi.org/10.3389/fonc.2020.00038
This article is part of the Research Topic PVT1 in Cancer View all 8 articles
Whole genome and transcriptome sequencing technologies have led to the identification of many long non-coding RNAs (lncRNAs) and stimulated the research of their role in health and disease. LncRNAs participate in the regulation of critical signaling pathways including cell growth, motility, apoptosis, and differentiation; and their expression has been found dysregulated in human tumors. Thus, lncRNAs have emerged as new players in the initiation, maintenance and progression of tumorigenesis. PVT1 (plasmacytoma variant translocation 1) lncRNA is located on chromosomal 8q24.21, a large locus frequently amplified in human cancers and predictive of increased cancer risk in genome-wide association studies (GWAS). Combined, colorectal and gastric adenocarcinomas are the most frequent tumor malignancies and also the leading cause of cancer-related deaths worldwide. PVT1 expression is elevated in gastrointestinal tumors and correlates with poor patient prognosis. In this review, we discuss the mechanisms of action underlying PVT1 oncogenic role in colorectal and gastric cancer such as MYC upregulation, miRNA production, competitive endogenous RNA (ceRNA) function, protein stabilization, and epigenetic regulation. We also illustrate the potential role of PVT1 as prognostic biomarker and its relationship with resistance to current chemotherapeutic treatments.
According to the latest data released by the International Agency for Research on Cancer (IARC), cancer of the digestive tract is the leading cause of cancer and cancer-related death worldwide (1–3) (Table 1). Digestive tract tumors include malignancies arising in the oral cavity, esophagus, stomach, small, and large intestines, rectum and anus. Briefly, oral cancer is a subgroup of head and neck cancers, characterized by the growth of tumor cells in the lining of the lips, mouth, and upper throat. Oral squamous cell carcinoma represents the most frequent oral neoplasm and arises from epithelial cells. The greatest risk factor for oral cancer is the tobacco and/or alcohol use. However, the exposure to chemical carcinogens, ultraviolet/ionizing radiation and viral infections, such as Human Papilloma virus (HPV), Epstein-Barr Virus (EBV) or Hepatitis C virus (HCV), are also known to increase oral cancer incidence rate (4). Esophageal cancer occurs when malignant cells arise in the tissue of the esophagus, the tube that transports the food from the mouth to the stomach. There are two major subtypes of esophageal cancer, which are epidemiologically and biologically very distinct. Esophageal squamous cell carcinomas occur in the epithelial cells of the mucosa, are frequently found in the upper and middle sections of the esophagus and associate with risk factors leading to recurrent chemical or physical insults to mucosa, i.e., tobacco, alcohol, or hot drinks use. Conversely, esophageal adenocarcinomas arise from the glandular cells in the mucosa, are more abundant in the lower section of the esophagus, and are associated with gastro-esophageal reflux and obesity as the main risk factors (5, 6). In turn, gastric cancer occurs when malignant cancer cells grow and colonize the wall of the stomach. The most common form of stomach cancer is the gastric adenocarcinoma, which originates in the epithelial cells from the mucosa. Other less common types of stomach cancer include gastro-intestinal stromal tumors, which develop in the connective tissue from the stomach wall, squamous cell carcinomas and carcinoid tumors. Traditionally, gastric adenocarcinomas are divided into two main histological subtypes, namely intestinal and diffuse. These subtypes follow very different oncogenic programs and thus display different molecular profiles (7). The etiology of gastric cancer is multifactorial but dietary factors, such as high salt and nitrate intake, and Helicobacter pylori infection increase the risk of gastric cancer development and progression (8–10). And finally, colorectal cancer occurs when tumor cells grow in the colon or rectum inner lining. The normal intestinal epithelium is maintained by a tight balance of proliferation, migration and cell death. Tumorigenesis occurs when these mechanisms become deregulated resulting in cell hyperproliferation and loss of differentiation, evidenced by the formation of aberrant crypts evolving into adenomatous polyps and subsequently into adenocarcinomas. Only a small proportion of colorectal tumors display a mesenchymal origin. Alcohol consumption, smoking, high fat diet as well as obesity are well-known risk factors for colorectal cancer (11).
Among all tumor types in the digestive tract, colorectal, and gastric cancers exhibit the highest incidence and mortality rates. Specifically, colorectal cancer is the third most common cancer type in men after lung and prostate cancer; and the second most common in women after breast cancer. Stomach cancer, in turn, ranks fourth and seventh regarding its incidence in men and women, respectively. Only in 2018, 1.8 million colorectal and about 1 million stomach cancer cases were diagnosed worldwide, accounting for almost 900,000 and 800,000 deaths, respectively (Table 1). Moreover, it is expected that by 2040, the incidence of colorectal cancer will raise by 30% and mortality by 40%; whereas statistics for stomach cancer will both worriedly increase by 60% due to its high prevalence in Asian countries, whose population and economic growth rates are increasing. The prognosis of gastrointestinal cancer patients largely depends on tumor stage at the time of diagnosis (Table 2). Additionally, tumor staging strongly influences the clinical management (12–15). Thus, gastrointestinal cancer represents a major health and social issue requiring great investment from governments worldwide to cover the cost of prevention, diagnosis and treatment.
Efficient clinical management of cancer largely relies on the identification and study of key mediators of the tumorigenic process, both at onset and progression. Classically, most of the research efforts in tumor biology have been focused on protein-coding oncogenes, tumor-suppressor genes and DNA repair genes. But with the irruption of whole-transcriptome sequencing (RNAseq) technologies and computational sciences, we have gained great insights into non-coding RNAs (ncRNAs). These RNAs cover over 90% of the human genome and regulate a great variety of cellular processes including chromatin architecture and remodeling, transcription, post-transcriptional modification, epigenetic regulation, and signal transduction, both in physiological and pathological processes including cancer (16–18). An arbitrary 200-nucleotide length cut-off allows the classification of non-coding transcripts into two categories: short and long ncRNAs. Short ncRNAs are represented by microRNAs (miRNAs), PIWI-interacting RNAs (piRNAs), transcription initiation RNAs (tiRNAs), small nucleolar RNAs (snoRNAs), promoter-associated small RNAs (PASRs), promoter upstream transcripts (PROMPTs), and TSS (transcriptional start site)-associated RNAs (TSSa-RNAs). On the other hand, long ncRNAs (lncRNAs) group heterogeneous non-coding transcripts such as intergenic non-coding RNAs (lincRNAs), ultraconserved regions (T-UCRs) and other ncRNAs collectively named lncRNAs (17, 19). miRNAs have been the most widely studied class of ncRNAs in tumor biology. Since its discovery two and a half decades ago, many miRNAs have been implicated in the development of multiple human cancers through a wide range of mechanisms. Among all these mechanism, gene silencing has been predominant (20, 21). Mirroring what we have learned about cancer-associated coding genes, we can classify miRNAs into oncogenic or tumor suppressor (22). Moreover, miRNAs have become actionable targets for cancer treatment and several therapeutic agents are under development including some that have reached clinical trials (23–25). Currently, there is a growing interest in lncRNAs biology and their role in the tumorigenic process. These transcripts are generated by RNA polymerase II, can be capped, spliced and polyadenylated, but lack an obvious open reading frame. According to the latest release of the GENCODE project (GRCh38.p12), which aims to build an encyclopedia of genes and gene variants, the human genome contains 16,066 lncRNA genes encoding for 29,566 different lncRNA transcripts (26–29). This number represents 27% of all annotated human genes.
Next-generation sequencing of large numbers of tumor specimens has revealed thousands of lncRNAs aberrantly expressed in a broad spectrum of cancers (30, 31). Dysregulation of certain lncRNAs leads to the hyper- or hypoactivation of cellular pathways that promote and/or sustain tumor initiation and progression (32). LncRNAs are involved in a broad range of processes such as transcriptional regulation of neighboring protein-coding genes, interference of miRNAs via sequence complementary, protein decoy, protein stability control, post-transcriptional processing, epigenetic regulation, high-order chromosomal dynamics, telomere biology, and subcellular structural organization (32–35). LncRNAs mediate all these functions through the interaction with proteins, RNAs and lipids. It is remarkable that unlike other ncRNAs, the function of lncRNAs cannot be inferred from sequence or structure and thus, experimental evaluation needs to be conducted for a full and accurate biological annotation (30). Because of their undeniable role in cancer biology, the therapeutic targeting of oncogenic lncRNAs and lncRNAs involved in resistance to treatment has raised significant attention. The main strategies to inactivate oncogenic lnRNAs aim their post-transcriptional degradation with siRNAs, or the steric blockade of lncRNA-protein interactions with small molecules, morpholinos, or antisense oligonucleotides (36, 37). Noteworthy, some intrinsic features of lncRNAs make them very attractive as cancer diagnostic and prognostic biomarkers (38). First, lncRNAs are expressed in a more tissue-specific manner than protein-coding genes. It has been estimated that 78% of all lncRNAs are tissue-specific, while protein-coding genes barely reach 19% of specificity (39). Additionally, lncRNAs dysregulation in primary tumors and metastasis is observed in body fluids, i.e., whole blood, plasma, urine, saliva, and gastric juice (40). And despite the high abundance of ribonucleases in most of these fluids, lncRNAs are easily detected due to its high stability. This represents a clear advantage to patients as it allows cancer diagnosis and follow-up using minimally invasive methodologies.
Cancer cells show a plethora of chromosomal abnormalities, including translocations, amplifications, and deletions. The plasmacytoma variant translocation 1 (PVT1) gene encodes a lncRNA that was first identified when studying a recurrent translocation breakpoint in the Igκ locus found in murine plamacytomas (41). One year later, a homologous human sequence was identified when studying immunoglobulin translocations in Burkitt lymphoma (42). We now know that PVT1 gene fusions occur in additional hematologic malignancies, such as non-Hodgkin lymphoma and advanced multiple myeloma (10, 43, 44). PVT1 gene fusions are also found in solid tumors although at much lower rates (10, 43, 44). PVT1 exon 1 and intron 1 are most often involved in these DNA rearrangements (45). In addition, human PVT1 is a target of genetic gains and amplifications in a large variety of cancers, including those of the digestive tract (46, 47). Moreover, genome-wide association studies (GWAS) identified single nucleotide polymorphisms (SNPs) in the PVT1 locus (8q24) that are associated with increased colorectal cancer risk (48). Of interest, PVT1 locus leads to the production of a cluster of four annotated miRNAs, namely miR-1204, miR-1205, miR-1206, and miR-1207 (-5p and -3p), being some of them important in the tumorigenic process of colorectal and gastric cancer (49–52). It is of note that although PVT1 has been mostly studied in the context of cancer, this lncRNA is related to multiple and diverse pathologies (Table 3).
In this review, we will discuss the current knowledge of PVT1 alteration/dysregulation, as well as its contribution to gastrointestinal cancer.
Most lncRNAs exhibit a great number of isoforms and PVT1 is not an exception (53). Human PVT1 locus resides in chromosome 8q24.21 and contains 21 exons leading to 25 annotated transcript variants (54). These variants arise as a consequence of alternative splicing mechanisms mediating exon skipping and the use of unconventional donor and acceptor splice sites. Thanks to consortia such as The Genotype-Tissue Expression Project (GTEx) and The Cancer Genome Atlas (TCGA), we know that among all these PVT1 transcripts, 14 are present in tissues at detectable levels (7, 55–58). Specifically, 11 PVT1 transcripts have been detected in the normal gastrointestinal mucosa and adenocarcinomas of the colon and stomach (Figure 1). Among them, PVT1-217 (ENST00000523190) containing only four exons, is the most abundant PVT1 transcript in the digestive tract. This heterogeneity in isoform expression needs to be considered when studying the role of PVT1 in carcinogenesis, as the biology of each isoform might have a different impact on tumor initiation/progression and patient survival. Several oncogenic mechanisms have been attributed to PVT1, i.e., as a ceRNA (competing endogenous RNA) for several miRNAs and as a source of miRNAs itself (49, 51, 59–65). As a non-coding gene, all of them rely into PVT1 RNA primary and secondary structure. Accordingly, the biological activity of PVT1 depends on the sequence of the specific PVT1 transcripts expressed in a given tissue at a given time. Unfortunately, very few reports have taken into consideration the large heterogeneity in PVT1 isoform expression and consequently, some of the results described in this review require further investigation. Nonetheless, multiple studies assessing PVT1 expression by means of microarray technology (66–68) or RT-PCR using oligonucleotides amplifying several PVT1 isoforms, have shown a general overexpression of PVT1 in colorectal tumors compared to paired normal tissue samples (66, 68–74). Only He et al. have examined the expression of individual PVT1 splice variants (Sv) in colorectal cancer (68). They found PVT1 Sv-214, Sv-205, Sv-209, Sv-208, Sv-206, Sv-207, Sv-213, Sv-219, Sv-201, and Sv-215 upregulated in colorectal cancer vs. normal samples (variants ranked by decreasing overexpression fold) (68). Similarly, increased PVT1 expression has been described in primary gastric tumors compared to the normal gastric mucosa (75–78). PVT1 isoform Sv-214 overexpression was found in primary gastric tumor samples compared to adjacent normal gastric tissue (79). In good agreement, increased PVT1 expression in COAD (colorectal adenocarcinoma), READ (rectal adenocarcinoma) and STAD (stomach adenocarcinoma) samples compared to the corresponding normal tissue was revealed using transcriptomic expression data available at the TCGA repository (57, 80). Interestingly, the tumorigenic process does not seem to affect the relative abundance of PVT1 isoforms (Figure 1), being PVT1 Sv-217 the isoform also more abundant in tumors. Unfortunately, the studies investigating the expression and role of PVT1 in gastrointestinal malignancies have not addressed directly or indirectly the expression of this isoform (66, 68–72, 75–78). All these observations have been extended to human cancer cell lines. Precisely, CACO2, SW480, SW620, HT29, and HCT116 cells derived from human colorectal tumors displayed higher levels of total PVT1 compared to NCM460, FHC and HCoEpiC normal colonic epithelial cells (67, 70, 72, 81). Likewise, AGS, MKN45, SGC7901, and BGC823 gastric cancer cell lines have higher PVT1 expression compared to GES1 normal gastric epithelium cell line (76, 79).
Figure 1. Expression of PVT1 isoforms in normal and tumor tissue from the gastrointestinal tract. Eleven of the twenty-five predicted PVT1 isoforms are expressed in the gastrointestinal tract. Transcripts variants are ranked from top to bottom according to their expression abundance (%) both in colon and stomach. Purple histograms illustrate the expression in normal tissue samples from GTEX database (colon n = 204 and stomach n = 204). Blue histograms illustrate the expression in tumor tissue samples from TCGA repository (colon adenocarcinoma n = 723 and stomach adenocarcinoma n = 453). Exon display for each PVT1 transcript variant is shown. Data was extracted using UCSC Xena Browser.
PVT1 expression in stomach cancer is directly regulated by FOXM1 (Forkhead Box M1) transcription factor (75). FOXM1 controls the expression of genes such as MYC, CCNB1, AURKB, and SKP2, which are essential for cell cycle progression at DNA replication and mitosis, and therefore important for tumor initiation and progression (82–85). Additionally, FOXM1 plays a key role in DNA damage checkpoint participating in the repair of DNA strand breaks (86). PVT1 transcript levels changes upon manipulation of FOXM1 protein expression in gastric cancer cells. Specifically, PVT1 expression is reduced upon FOXM1 silencing and increases after FOXM1 overexpression (75). This regulation occurs through a direct interaction of FOXM1 with two independent binding sites in the PVT1 promoter that enhances the transcriptional activation of this lncRNA (75). In addition, STAT3 (Signal Transducer and Activator of Transcription 3) has also been shown to regulate the expression of PVT1 (77). Persistent STAT3 activation in tumor cells results in increased cell proliferation, survival, and invasion; and at the same time, STAT3 exerts non-cell autonomous effects in the tumor microenvironment by boosting tumor-promoting inflammation and suppressing anti-tumor immunity (87). STAT3 overexpression in gastric cancer cell lines leads to increased PVT1 levels, while STAT3 knockdown results in PVT1 transcriptional downregulation. These effects are explained by the presence of three canonical STAT3 binding motifs within the PVT1 promoter, which were confirmed to control the transcription of this lncRNA (77) (Figure 2).
Figure 2. Chromosome 8q24.21 amplification and PVT1 gene structure and regulation. 8q24.21 chromosomal region is frequently amplified in colorectal and gastric cancers (upper). This region contains MYC as the only protein-coding gene (yellow boxes) and several lncRNAs. Those lncRNA involved in MYC or WNT regulation have been depicted (blue and black boxes) (middle). PVT1 lncRNA expression is regulated by FOXM1, STAT3, and p53 transcription factors. PVT1 promoter exhibits four enhancer regions that in normal conditions (WT) boost its own expression. Due to PVT1 promoter mutations and rearrangements (MUT), which are frequently found in cancers, enhancer elements preferentially interact with MYC promoter enhancing the transcription of the oncogene. PVT1 encodes for four miRNAs. miR-1204 has been show to stabilize p53 protein, while miR-1207 has been proven to modulate TERT protein at the post-transcriptional level and expression of stemness and EMT genes by mechanisms currently unknown (lower). Pictograms in purple and green illustrate evidences obtained in colorectal and gastric cancer, respectively. Dark gray boxes indicate gene promoters. Dark blue boxes indicate enhancer regions. Green and red arrows indicate up- and down-regulation, respectively.
In colorectal cancer, PVT1 transcription is influenced by p53 (50). This tumor suppressor is involved in the cell cycle regulation by transactivating a plethora of protein-coding but also non-protein-coding genes, that ultimately prevent cell division by inducing cell cycle arrest, senescence, or apoptosis (88, 89). Importantly, p53 is the most frequently mutated gene in human cancers (90, 91). The promoter of PVT1 harbors a functional p53 response element that enhances the transcription of this lncRNA in colorectal cancer cell lines exposed to the DNA damaging agent daunorubicin, or upon increased p53 protein levels achieved by the use of Nutlin-3a (50). It is important to mention that PVT1 upregulation upon these conditions was only monitored in p53 wild-type colorectal cancer cell lines and thus, it remains unknown the ability of mutant p53 proteins to exert an equivalent role. Although most p53 mutations result in expression of dominant-negative forms, certain mutations are known to confer oncogenic functions (92) (Figure 2).
High-resolution analyses of somatic copy-number alterations indicate that 8q24.21 region is one of the most frequently amplified regions across human cancers, including colorectal and gastric cancer, and are often associated with poor prognosis and/or drug resistance (7, 58, 93). Interestingly, although the amplified region spans almost 2 Mb the only protein coding gene in this locus is the MYC oncogene. This fact led to nickname this region as the gene “dessert” locus, and attribute to MYC the tumor promoting effects of gaining supernumerary copies of 8q24 (66, 94–96). Conversely, 8q24.21 has been shown to be an “oasis” for lncRNAs. Up to 12 lncRNAs have been identified in this locus, and very importantly for colorectal cancer, many of them regulate Wnt signaling pathway and in turn Myc activity (35, 69) (Figure 2). Wnt signaling is crucial for the initiation and maintenance of colorectal cancer and consistently, this pathway is altered in more than 90% of these malignancies according to the TCGA data (58). Wnt dysregulation in gastric tumorigenesis is less frequent but affects 10–30% tumors (7, 97). Accordingly, PVT1 and MYC coamplification (66, 78, 98) and coexpression (71, 98, 99) has been observed in colorectal and gastric primary tumors and cell lines (Figure 3).
Figure 3. PVT1 and MYC expression in gastrointestinal tumors and cell lines. PVT1 and MYC copy number variation (A,B) and RNAseq expression (C,D) in tumor samples from colorectal (n = 616) and stomach (n = 441) adenocarcinomas (A,C) and established cell lines from colorectal (n = 58) and gastric tumors (n = 38) (B,D). The name of cell lines used in studies investigating the role of PVT1 in gastrointestinal cancer are shown. Gray boxes indicate no alteration. Light blue boxes indicate gene amplification. Dark blue boxes indicate gene deletion. RNAseq expression units: RPKM (Reads Per Kilobase Million). Pearson and Spearman correlation coefficients and associated p-value (Spearman) are shown.
The first evidence of PVT1 in human solid malignancies, and also its relationship with MYC, came from the study of COLO320, a colorectal cancer cell line that was known to be MYC amplified. Two variants of the cell line were isolated: COLO320-HSR (homogeneous stained region) with MYC allele intrachromosomically amplified; and COLO320-DM, with a rearranged and amplified MYC allele that is carried on small fragments of extrachromosomal DNA named double minutes (100). By studying MYC amplification in COLO320-DM, the authors noticed that intron 1 from MYC contained an ectopic DNA fragment which they characterized and described as PVT1 in humans (101). However, the architecture of the MYC-PVT1 locus in COLO320-DM was later realized to be unique, since PTV1 locus normally lies 57 kb downstream of MYC gene. This chromosomal organization is conserved in other species such as mouse and rat, with syntenic 15qD1 and 7q33 regions, respectively (99).
The expression of PVT1 and MYC in tumors are correlated not only because of their genetic coamplification. The 8q24 region displays a very strong enhancer activity creating feedback loops controlling MYC expression (102). Precisely, lncRNAs within 8q24 region are efficiently transcribed due to the presence of abundant transcriptional factors and mediator proteins, and these lncRNAs in turn, increase the enhancer activity by forming chromatin loops and protein bridges to promote the transcription of MYC (71, 98, 103). Interestingly, PVT1 promoter is recurrently rearranged in human tumors, including those affecting the digestive tract (10, 44, 104). It has been demonstrated experimentally that insertions or deletions in the PVT1 promoter lead to Myc overexpression and consequently, enhanced growth in cancer cells (104). Chromatin is organized into self-interacting units called topologically associating domains (TADs). Contacts between promoter and enhancer regions take place within the TADs, leading to an additional layer of gene expression regulation (105). As a consequence, genomic DNA structural variations, i.e., deletions, duplications, insertions, inversions, and translocations, are able to modify the three-dimensional chromatin topology and promote or suppress promoter-enhancer interactions within the TADs (104, 106). PVT1 gene harbors four intragenic enhancer elements that under normal conditions establish strong interactions with the PVT1 promoter and sustain expression of the non-coding transcript. However, under the PVT1 promoter mutational rearrangements found in tumor cells, and even epigenetic inactivation, PVT1 intragenic enhancer elements interact preferentially with MYC promoter, thus boosting the transcription of MYC oncogene and its oncogenic activity of tumor cells (104) (Figure 2).
LncRNAs carry out their biological functions through multiple and diverse mechanisms. Several research groups have demonstrated the overall ability of PVT1 to sustain in vitro and in vivo cell growth, clonogenicity, migration, and invasion, both in colorectal (66–68, 71, 72, 107) and gastric cancer epithelial cells (75–77, 79). The capacity of PVT1 to negatively regulate apoptosis through the inhibition of the TGFβ pathway, is more controversial, as it has not been observed systematically (66, 70–72, 78, 79, 107, 108). PVT1 lncRNA has been shown to exert its protumorigenic activity also in a non-cell autonomous manner. Precisely, PVT1 expression in gastric tumors enhances microvessel formation, both in vitro and in vivo, through a mechanism that involves vascular endothelial growth factor A (VEGFA) expression in a STAT3-dependent manner (77). Formation of new vessels is a crucial step for tumors to maintain the supply of oxygen and nutrients, and VEGFA is a master regulator of this process (109). Consistently, antiangiogenic agents are routinely used in the clinical practice to treat cancer patients (110, 111). Antiangiogenics are used in combination with chemotherapy in the first line of treatment of colorectal cancer, and several compounds targeting tumor vasculature have been recently approved for the management of gastric cancer (13, 14, 112). In fact, expression of PVT1 and VEGFA in combination predicts poor survival in gastric cancer, further supporting the use of antiangiogenic drugs in these patients (77).
All these results have been obtained upon genetic manipulation of PVT1 levels in cell line models, and it is important to mention that these manipulations have not been restricted to a single PVT1 isoform (68). The siRNAs often used to achieve downregulation of PVT1 target multiple transcripts, and not always those predominantly expressed in colorectal and gastric tumors. On the other hand, models of PVT1 overexpression do not adequately report the specific isoforms used. The only exception is the study of He et al., where they convincingly demonstrated in HCT116 and SW480 colon cancer cell lines, the ability of PVT1 Sv-214 to promote proliferation, stemness, migration and invasion in vitro; as well as in vivo by establishing tumor xenografts and liver metastasis mouse models in immunodeficient nude mice (68). Importantly, differences in the PVT1 isoforms downregulated in different studies might explain the discrepancies reported regarding the role of PVT1 to regulate apoptosis. In this line, the suitability of certain PVT1 knockdown cellular models exhibiting apoptosis to assess invasion and/or migration properties should be reconsidered.
PVT1 locus leads to the production of a cluster of four annotated miRNAs, namely miR-1204, miR-1205, miR-1206, and miR-1207 (-5p and -3p) (49, 51) (Figure 2). Originally, miR-1208 was also mapped into PVT1 locus, but according to latest version of Ensemble Genome browser (v98) this miRNA lies outside the lncRNA sequence (54). All these miRNAs are conserved in the mouse Pvt1 gene (51). As depicted in Figure 4, most of the miRNAs contained within the PVT1 transcript variants expressed in the colon and stomach are found in intronic regions. Spatiotemporal organization of the RNA splicing has important implications to fully understand the PVT1 loss-of-expression experiments found in the literature (113). Gene silencing using exogenous siRNA against exonic regions does not necessary entail that the miRNAs encoded within introns will also be silenced. Upon splicing in the nucleus, introns are available for microRNA processing while the spliced transcript is exported to the cytoplasm where it may be targeted by exogenous siRNAs. This might explain why the use of siRNAs against PVT1 in HCT116 and RKO cells did not have any effect on the expression of miR-1204, miR-1205, and miR-1207-5p/3p (66). Contrary, expression of all PVT1-encoded miRNAs was enhanced upon exposure of the cells lines to the DNA damaging agent daunorubicin, or upon increased p53 protein levels achieved through Nutlin-3a stimulation (50). This, effect is likely to be indirect because, as mentioned before, PVT1 increases as consequence of p53 stabilization. Among the four PVT1-encoded miRNAs, only miR-1204 seems to engage a positive feedback loop to sustain stabilization of p53 at protein level (50).
Figure 4. miRNAs encoded by PVT1 transcript variants. PTV1 isoforms are ranked according to expression abundance (%) in gastrointestinal normal tissues and tumors. Gray and blue boxes indicate absence and presence of the indicated miRNA, respectively. Nucleotide sequence, length, and location within PVT1 introns or exons is indicated for all miRNAs.
The role of PVT1-encoded miRNAs in the initiation and/or progression of malignancies in the stomach or colon is uncertain due to the lack of literature. Only miR-1207 is reported to have an effect in gastric cancer proliferation and invasion, or in the stemness properties of colon cancer cells (52, 81). Specifically, it has been shown how ectopic expression of miR-1207-5p in the SGC7901 gastric tumor cell line is able to reduce proliferation and invasion in vitro and in vivo by targeting the catalytic subunit of the telomerase complex hTERT (52). Aberrant expression of hTERT is associated with the metastatic ability of gastric tumors (114, 115). In turn, miR-1207-5p overexpression in the HCoEpiC normal colon epithelial cell line enables the formation of primary and secondary spheres by a mechanism involving the upregulation at the mRNA level of TGFβ, CTNNB1, MMP2 and several colorectal stem cell markers such as CD44, CD133, and CD166 (81). Consistent with the tight relationship between stemness and epithelial-mesenchymal transition (EMT), Slug, Snail, and vimentin were also found upregulated (81, 116) (Figure 2). Interestingly, miR-1207-5p miRNA seems to exhibit oncogenic activity in colorectal cancer while having tumor suppressor effects in gastric cancer cells (contrary to the oncogenic role of PVT1).
LncRNAs have the ability to modulate gene expression indirectly by impairing miRNA activity through sequestration/sponging, which leads to an effective de-repression of targets of these miRNAs (33, 34). This function, named competitive endogenous RNA (ceRNA), has encountered certain skepticism due to the fact that the physiological expression levels of an individual lncRNA may not be sufficient to completely suppress the activity of miRNAs (117). However, regulation by modestly expressed lncRNAs could be magnified through downstream processes, particularly through the upregulation of transcription factors that by transactivating multiple targets contribute to outcome amplification. This might be the case for PVT1, which as shown here maintains a close relationship with Myc, p53 and STAT3, all of them key transcription factors in the tumorigenic process.
miR-152 suppresses the proliferation and motility of gastric cancer cells targeting CD151 and FGF2, which are cell surface receptors well-known to participate in the spreading, migration and invasion of tumors (118–120) (Figure 5). miR-152 expression is downregulated in gastric cancer tissues, most likely as a result of the interaction with PVT1 which has been shown in vitro to effectively sponge this miRNA (60, 118). In good agreement, PVT1 is negatively correlated with miR-152 expression in tumors from gastric cancer patients, and its genetic manipulation in SGC7901 and BGC823 gastric cell lines influences miR-152 levels and ultimately, CD151 and FGF2 expression (60). It is noteworthy that three independent binding sites for miR-152 have been identified in PVT1, however not all of them are present in the transcripts more frequently expressed in the gastric tissue (Figure 6). No reports are found in the literature regarding the role of PVT1 in sponging miR-152 in colorectal tumors. However, as observed for gastric tumorigenesis, this miRNA acts as a tumor suppressor in colorectal cancer cell lines and its expression is downregulated in primary colorectal tumors (121, 122).
Figure 5. PVT1 interaction with miRNAs and proteins. PVT1 interacts with multiple miRNAs and proteins in cancer cells, leading to post-transcriptional (1) and post-translational (2) regulation of gene expression. PVT1-miRNA interactions promote efficient de-repression of miRNA targets. Specifically, PVT1 hinders miRNAs binding in the 3′ untranslated region of target transcripts, preventing their degradation by the RISC complex and thus increasing protein levels. PVT1-protein interactions stabilize proteins and prevent their degradation. Pictograms in purple and green illustrate PVT1-miRNAs/proteins interactions identified in colorectal and gastric cancer, respectively.
Figure 6. Predicted sponge capacity of miRNAs by PVT1 transcript variants. PTV1 isoforms are ranked according to their relative expression abundance (%) in gastrointestinal normal tissues and tumors. Gray and solid blue boxes indicate absence and presence of the nucleotide sequence complementary to the different miRNA, respectively. Patterned blue boxes indicate complementarity with miR-128 principal binding motif, but lack of the additional contact sites and/or difference in length from the main interacting core. Nucleotide sequence of the miRNA binding site is specified.
This tumor suppressor miRNA negatively regulates the proliferation, invasion and migration of gastric cancer cells by impairing the EMT process through TWIST1 targeting and degradation (123). miR-186 regulates the expression of hypoxia inducible factor 1α (HIF-1α) and blocks aerobic glycolysis in gastric cancer cell lines (124). HIF-1α is a key protein regulating the response of cells to low levels of oxygen (125). In cancer, HIF-1α is significantly associated with metastasis, stemness and poor prognosis and thus, tumors engage sophisticated mechanisms to keep its expression at high levels (126). Gastric cancer cell lines with PVT1 overexpression show increased HIF-1α mRNA and protein levels, that are reduced with a miR-186 mimic. Conversely, the decreased HIF-1α levels achieved by PVT1 downregulation are rescued upon usage of a miR-186 inhibitor (124) (Figure 5). PVT1 binding to miR-186 occurs through a single interacting site, which according to in silico analysis is present exclusively in PVT1-Sv203 (Figure 6). miR-186 role in colorectal cancer pathogenesis is not so clear. Some reports have identified miR-186 [5p] as a tumor suppressor due to its ability to inhibit proliferation, metastasis and EMT of colorectal cancer cells by targeting ZEB1, while others point out in the opposite direction (127–129).
PVT1 acts as a ceRNA to negatively regulate the expression of miR-455 in HT29 and SW480 colon cancer cell lines (72). Hsa-miR-455 encodes for two miRNAs: miR-455-5p and miR-455-3p. PVT1 seems to specifically bind to miR-455-5p (Figure 6). This miRNA is frequently downregulated in colorectal tumors and acts predominantly as a tumor suppressor element by targeting phosphoinositide-3-kinase regulatory subunit 1 (PIK3R1) and Runt-related transcription factor 2 (RUNX2), both crucial for the development of tumors (72, 130, 131) (Figure 5). An equivalent tumor suppressor role has been attributed to miR-455 in gastric tumorigenesis (132, 133).
In silico analysis identified PVT1 Sv-214 as ceRNA for miR-128, and the initial observation was confirmed experimentally in colorectal cancer cell lines through gain and loss-of-function experiments against PVT1 Sv-214 isoform and further extended to gastric cancer cell line model (68, 79). Moreover, the expression of miR-128 showed a significant inverse correlation with the expression of PVT1 Sv-214 in colorectal and gastric primary tumor samples. As depicted in Figure 6, the central interacting motif between PVT1 Sv-214 and miR-128 is present in several transcript variants. However, the additional contact sites between the lncRNA and the miRNA pointed out by the authors are not always present in these variants or alternatively differ in length from the central motif. Therefore, the capacity of these PVT1 isoforms to sponge miR-128 should be confirmed experimentally. miR-128 targets the Lin28 transcript for degradation in colorectal cancer cell lines (68). Lin28 is an RNA-binding protein that inhibits the processing of let-7 family of miRNAs. Let-7 regulates the translation of mRNAs important for the embryo development, cell pluripotency, metabolism, and tumor progression (68, 134). In line with this observation, the expression of PTV1 Sv-214 and let7a negatively correlated in colorectal cancer patient samples (Figure 5). Notably, Lin28 cooperates with Wnt signaling and Snail to drive growth and invasiveness in colorectal and gastric tumors, respectively (135–137). Conversely, in gastric cancer cell line systems, miR-128 was shown to target TrkC (79) (Figure 5). TrkC/NTRK3 is a member of the NTRK neurotrophin tyrosine kinase receptor family and functions as a tumor suppressor. TrkC is considered a dependence receptor, which is characterized by its ability to induce opposing biological effects depending on the availability of the ligand, NT-3. In the presence of the TrkC ligand, a survival signal is transduced, whereas its absence results in cleavage of a death-domain peptide and induction of apoptosis. NT-3 levels are reduced in CRC and consistently with the tumor suppressor activity of the receptor, tumors tend to accumulate inactivating point mutations or silence protein expression by means of promoter methylation (138, 139). Interestingly, gastric tumors seem to achieve TrkC inactivation through miR128-mediated transcript degradation.
Bioinformatics analysis also allowed the identification of PVT1 as a sponge for miR-214-3p. This miRNA negatively regulates the expression of IRS1 (Insulin receptor substrate 1) in the colon cancer cell line HCT116 (107). IRS1 is a key signaling mediator of the insulin/insulin-like growth factor (IGF) system controlling cellular proliferation, differentiation, EMT, and apoptosis in colorectal cancer (140, 141). Upon phosphorylation by the insulin receptor, IRS1 binds specifically to cellular proteins containing SH2 domains such as phosphatidylinositol 3-kinase (PI3K) regulatory subunit (p85) or GRB2, leading to activation of PI3K and MAPK signaling pathways, respectively (142, 143) (Figure 5). Accordingly, both PVT1 and miR-214-3p sustained the expression and activation of PI3K catalytic subunit (p110) and Akt in colon cancer cell line models (107). No reports have described so far PVT1 binding to miR-214 in gastric cancer. However, this might not contribute for stomach carcinogenesis, as miR-214 displays an oncogenic role rather than tumor suppressor in gastric epithelial tumor cells. Specifically, miR-214 was been found upregulated in gastric tumors and sustains cell proliferation, migration and invasion by targeting GSK3β, PTEN, PRDM16, and A2AR (144, 145).
LncRNA can also interact with proteins and regulate their stability by preventing post-translational modifications associated with protein degradation. Specifically, PVT1 has been shown to bind and stabilize some proteins that are relevant in the gastrointestinal tumorigenesis, such as STAT3 and Lin28.
PVT1 has been shown to interact with transcription factor STAT3 in gastric cancer cell lines. Binding of PVT1 to STAT3 restricted STAT3 ubiquitin-proteasomal degradation leading to sustained phosphorylation and nuclear translocation of the transcription factor (77) (Figure 5). As described before, STAT3 has a strong role supporting tumor progression and therefore, significant efforts have been aimed at the development of specific and non-toxic inhibitors for cancer treatment (146, 147).
RNA immunoprecipitation followed by mass spectrometry identified Lin28 as a protein interacting directly with PVT1 Sv-214 (68). Lin28-PVT1 complex formation prevented Lin28 degradation by the proteasome, increasing the steady-state levels of this RNA-binding protein. Consistently, Lin28 protein levels were reduced in vitro and in vivo when PVT1 was knocked down using siRNAs technology, and increased when PVT1 was overexpressed (Figure 5). The interaction between Lin28 and PVT1 is known to take place in the cytoplasm and to involve the 3′ region of PVT1 Sv-214. Although the specific binding site has not been mapped, it is known to be contained within nucleotides ranging from position 672 and 922 in this PVT1 isoform and encoded by a small portion of exon 17 and full exons 18 and 19. Therefore, besides PVT1 Sv-214, only PVT1 Sv-217 could potentially interact with Lin28. Altogether, Lin28 is regulated by PVT1 through two independent mechanisms: (1) post-transcriptionally, preventing Lin28 transcript targeting by mir-128, as described above, and; (2) post-translationally, reducing Lin28 protein degradation by the proteasome machinery (68).
LncRNAs are important epigenetic regulators and in turn, perturbations of epigenetic regulation are thought to be a key feature of many cancers and even driver events (148). Twenty percent of all human lncRNAs have been shown to physically associate with Polycomb Repressive Complex 2 (PRC2 complex), which operates as a transcriptional repressor (149, 150). PVT1 has been shown to recruit Enhancer of Zeste homolog 2 (EZH2) to epigenetically negatively regulate the expression of p15 and p16 in gastric cancer cell lines (78). Interestingly, in primary gastric tumors PVT1 correlates positively with EZH2 and negatively with p15/p16 protein levels (78) (Figure 7).
Figure 7. Epigenetic regulation by PVT1. PVT1 recruits EZH2 polycomb group protein leading to promoter methylation and transcriptional repression of CDKN2A (p16) and CDKN2B (p15).
PVT1 is clearly dysregulated in gastric and colorectal cancer, and experimental mechanistic studies convincingly demonstrate that elevated levels of this lncRNA promote proliferation, angiogenesis and metastasis in human malignancies. Consistently, possible associations between PVT1 levels and patient prognosis have been investigated by several independent groups. However, as mentioned before, PVT1 exhibits a significant variability in transcript expression and this has not been rigorously considered when interrogating PVT1 levels in primary tumors. Most of the studies have determined PVT1 expression by means of qPCR-based techniques, and surprisingly none of them directly assessed the expression of PVT1 Sv-217, which according to datasets available in public repositories such as TCGA, is the isoform predominantly expressed in colorectal and stomach tumors (Figure 1) (151). Additionally, primers targeting multiple isoforms have been used in most of the studies, which might account for the differences observed in the clinicopathological features correlating with PVT1 expression.
In colorectal cancer, the expression of PVT1 isoform Sv-214 was found to be positively associated with tumor size, advanced stage (III-IV), distant metastasis, and reduced overall and disease-free survival (70). PVT1 Sv-203 when amplified with qPCR primers that could co-amplify Sv-202 and Sv-211 (66) (two isoforms that according to genome-wide RNAseq profiling have no detectable expression in colorectal malignancies) correlates with advanced stage (III-IV), lymph node metastasis, venous invasion, and decreased overall survival (66, 70). However, the expression of the same PVT1 isoform Sv-203, when determined together with the expression of Sv-206 and Sv-212 (two isoforms that are moderately expressed in colorectal tumors), was not associated with the clinicopathological features described above (69). Additional studies assessing PVT1 overall levels by means of high-throughput technologies such as microarrays or RNA sequencing, observed that high expression values were associated with shorter patient overall survival (67, 152). Similarly, in gastric cancer, elevated PVT1 expression was found to be associated with high tumor stage (III–IV) (75–78), lymph node metastasis (76) and overall/disease-free survival (78). PVT1 Sv-214 specific evaluation in gastric cancer patients was associated with larger tumor size (5 cm cut off), advanced stage (III-IV) and reduced overall survival (79). Collectively, the available literature supports the contention that elevated expression of PVT1 is associated with poor prognosis of gastrointestinal cancer patients, but additional isoform-specific studies are warranted.
Current treatment for locally advanced colorectal and stomach cancer involves surgical resection of the primary tumor followed by adjuvant chemotherapy to lower the risk of tumor recurrence. Certain patients are also eligible for preoperative or neoadjuvant therapy, being radiation-based treatment the best option for rectal cancer. Several drugs are approved for the treatment of gastrointestinal cancer, but nowadays ECF chemotherapy (epirubicin, cisplatin, and 5-fluorouracil) and FOLFOX/FOLFIRI/FOLFOXIRI regimens (5-fluorouracil, leucovorin, oxaliplatin, and/or irinotecan) constitute the standard of care for stomach and colorectal cancer, respectively (12–15). Intrinsic and acquired resistance to therapeutic agents is the first cause of treatment failure in cancer patients (153). Therefore, identification of the molecular pathways driving the resistance to chemotherapeutic drugs and the screening of drug response biomarkers in cancer patients is of paramount importance to maximize response rates (154–156).
Several studies have investigated the expression of PVT1 in colorectal and gastric cancer patients treated with different regimens. PVT1 was found upregulated using quantitative RT-PCR in colorectal cancer patients refractory to 5-fluorouracil (5-FU) in neoadyuvancy (primary resistance), and also in colorectal and gastric cancer patients receiving but not benefiting from cisplatin (70, 108, 157). These findings were consistent with the results obtained using cellular models of acquired drug resistance in vitro. HCT116 colon cancer cells resistant to the combination of 5-FU and radiation displayed increased levels of PVT1 compared to the parental cell line when evaluated using a human lncRNA Expression Array (158). Assessment of PVT1 transcript levels by quantitative RT-PCR in LOVO and RKO colon cancer cell lines with acquired resistance to cisplatin, or HCT8 and HCT116 with secondary resistance to 5-FU also evidenced the transcriptional upregulation of PVT1 lncRNA (70, 157). Likewise, BGC823 and SGC7901 gastric cancer cell line models resistant to cisplatin exposure, exhibited increased levels of PVT1 (108). Of note, all the cell lines used in these studies were all MYC amplified, and in the case of the colorectal cancer cell lines, also PVT1 amplified. It might have been very interesting to investigate to what extent elevated PVT1 levels by copy-number gain, are required for drug resistance. Interference of PVT1 expression using siRNA technology in cisplatin resistant cellular models resulted in a reduction of cell proliferation and engagement of apoptosis (70, 108, 157). Mechanistically, some of these effects could be attributed to MDR1 (Multidrug resistance protein 1B) and MRP1 (Multidrug resistance-associated protein 1), which have been shown in vitro to modify their expression at mRNA and protein level in a PTV1-dependent manner (70, 108, 157). Both proteins are plasma membrane efflux pumps controlling the intracellular bioavailability of substrates at the expense of ATP hydrolysis, and are well-known chemotherapy-resistance mediators in cancer (159).
PVT1 is an oncogenic lncRNA in many cancer types, including colorectal and stomach cancer. This lncRNA is involved in multifaceted aspects of cancer biology such tumor growth, metastasis, and response to therapeutic agents through a complex signaling network that involves interactions with DNA, RNA, and proteins. PVT1 complexity starts at the transcriptional level. Twenty-five splice variants have been described for PVT1 according the latest release of Ensembl database, being 11 of them detectable in colorectal and gastric normal/tumor tissues with the current sequencing platforms. Surprisingly, this complexity has been largely neglected when addressing PVT1 expression different in tissues. This is of special relevance if as postulated by some authors, PVT1 is intended to be used as a biomarker for diagnosis, personalized therapeutic treatment, or even a new therapeutic target. Currently, there are no reports associating PVT1 up-regulation with well-established and clinically relevant molecular features of colorectal and gastric tumors such as microsatellite stability (MSI/MSS), CpG island methylator phenotype (CIMP+/CIMP−), consensus molecular subtypes (CMS1, CMS2, CMS3, and CMS4 for colorectal cancer, and MSI, EBV, CIN, GS for gastric cancer) or mutations in key driver genes (APC, CTNNB1, SMAD4, TGFB1, TP53, KRAS, PI3K, CDKN2A, CDH1, ERBB2, or RHOA).
Experimentally, more efforts should be invested in the study of the primary, secondary, and higher-order structure of PVT1 transcripts and PVT1 promoter region, which is crucial for understanding the function of this lncRNA at a molecular level. The role of PVT1 in colorectal and gastric cancer has been addressed using gain-and-loss of function experiments using gene overexpression and RNA interference approaches in cell line systems, respectively. PVT1 encodes multiple miRNAs in intronic regions. The nuclear localization of spliced intronic sequences prevents optimal targeting by the RNAi machinery, which is mainly localized to the cytoplasm. Alternative oligo-mediated RNA knockdown strategies, such as modified antisense oligonucleotides or gapmers, should be used to block PVT1 miRNA-mediated effects. Ideally, knock-out/knock-in mouse models should be used to better assess the role of PVT1 in cancer biology. However, compared to protein coding genes, very few animal models have been generated to assess the function of lncRNAs in vivo. Unfortunately, many lncRNAs are not highly conserved hindering the identification of mouse orthologs and full function evaluation still represents a challenge.
AM-B, DA, and HD wrote the manuscript.
This study was partially funded by grants of the Spanish Ministry for Economy and Competitiveness (PI16/00540, AC15/00066, AC19/00095, and PI19/00993) and the Spanish Association Against Cancer (AECC GCA15152966ARAN) to DA.
The authors declare that the research was conducted in the absence of any commercial or financial relationships that could be construed as a potential conflict of interest.
1. International Agency for Research on Cancer. Global Cancer Observatory. (2018). Available online at: https://gco.iarc.fr/ (accessed April 18, 2019).
2. Bray F, Ferlay J, Soerjomataram I, Siegel RL, Torre LA, Jemal A. Global cancer statistics 2018: GLOBOCAN estimates of incidence and mortality worldwide for 36 cancers in 185 countries. Cancer J Clin. (2018) 68:394–424. doi: 10.3322/caac.21492
3. Ferlay J, Colombet M, Soerjomataram I, Mathers C, Parkin DM, Pineros M, et al. Estimating the global cancer incidence and mortality in 2018: GLOBOCAN sources and methods. Int J Cancer. (2019) 144:1941–53. doi: 10.1002/ijc.31937
4. Rivera C, Venegas B. Histological and molecular aspects of oral squamous cell carcinoma (Review). Oncol Lett. (2014) 8:7–11. doi: 10.3892/ol.2014.2103
5. Smyth EC, Lagergren J, Fitzgerald RC, Lordick F, Shah MA, Lagergren P, et al. Oesophageal cancer. Nat Rev Dis Primers. (2017) 3:17048. doi: 10.1038/nrdp.2017.48
6. Lagergren J, Smyth E, Cunningham D, Lagergren P. Oesophageal cancer. Lancet. (2017) 390:2383–96. doi: 10.1016/S0140-6736(17)31462-9
7. Cancer Genome Atlas Research N. Comprehensive molecular characterization of gastric adenocarcinoma. Nature. (2014) 513:202–9. doi: 10.1038/nature13480
8. Nagini S. Carcinoma of the stomach: a review of epidemiology, pathogenesis, molecular genetics and chemoprevention. World J Gastrointest Oncol. (2012) 4:156–69. doi: 10.4251/wjgo.v4.i7.156
9. Ashktorab H, Kupfer SS, Brim H, Carethers JM. Racial disparity in gastrointestinal cancer risk. Gastroenterology. (2017) 153:910–23. doi: 10.1053/j.gastro.2017.08.018
10. Kim HP, Cho GA, Han SW, Shin JY, Jeong EG, Song SH, et al. Novel fusion transcripts in human gastric cancer revealed by transcriptome analysis. Oncogene. (2014) 33:5434–41. doi: 10.1038/onc.2013.490
11. Kuipers EJ, Grady WM, Lieberman D, Seufferlein T, Sung JJ, Boelens PG, et al. Colorectal cancer. Nat Rev Dis Primers. (2015) 1:15065. doi: 10.1038/nrdp.2015.65
12. Smyth EC, Verheij M, Allum W, Cunningham D, Cervantes A, Arnold D, et al. Gastric cancer: ESMO clinical practice guidelines for diagnosis, treatment and follow-up. Ann Oncol. (2016) 27(Suppl 5):v38–49. doi: 10.1093/annonc/mdw350
13. Labianca R, Nordlinger B, Beretta GD, Mosconi S, Mandala M, Cervantes A, et al. Early colon cancer: ESMO clinical practice guidelines for diagnosis, treatment and follow-up. Ann Oncol. (2013) 24(Suppl 6):vi64–72. doi: 10.1093/annonc/mdt354
14. Van Cutsem E, Cervantes A, Nordlinger B, Arnold D, Group EGW. Metastatic colorectal cancer: ESMO clinical practice guidelines for diagnosis, treatment and follow-up. Ann Oncol. (2014) 25(Suppl 3):iii1–9. doi: 10.1093/annonc/mdu260
15. Glynne-Jones R, Wyrwicz L, Tiret E, Brown G, Rodel C, Cervantes A, et al. Rectal cancer: ESMO clinical practice guidelines for diagnosis, treatment and follow-up. Ann Oncol. (2018) 29(Suppl 4):iv263. doi: 10.1093/annonc/mdy161
16. Anastasiadou E, Jacob LS, Slack FJ. Non-coding RNA networks in cancer. Nat Rev Cancer. (2018) 18:5–18. doi: 10.1038/nrc.2017.99
17. Morris KV, Mattick JS. The rise of regulatory RNA. Nat Rev Genet. (2014) 15:423–37. doi: 10.1038/nrg3722
18. Consortium EP. An integrated encyclopedia of DNA elements in the human genome. Nature. (2012) 489:57–74. doi: 10.1038/nature11247
19. Esteller M. Non-coding RNAs in human disease. Nat Rev Genet. (2011) 12:861–74. doi: 10.1038/nrg3074
20. Huntzinger E, Izaurralde E. Gene silencing by microRNAs: contributions of translational repression and mRNA decay. Nat Rev Genet. (2011) 12:99–110. doi: 10.1038/nrg2936
21. Jonas S, Izaurralde E. Towards a molecular understanding of microRNA-mediated gene silencing. Nat Rev Genet. (2015) 16:421–33. doi: 10.1038/nrg3965
22. Croce CM. Causes and consequences of microRNA dysregulation in cancer. Nat Rev Genet. (2009) 10:704–14. doi: 10.1038/nrg2634
23. Rupaimoole R, Slack FJ. MicroRNA therapeutics: towards a new era for the management of cancer and other diseases. Nat Rev Drug Discov. (2017) 16:203–22. doi: 10.1038/nrd.2016.246
24. van Rooij E, Kauppinen S. Development of microRNA therapeutics is coming of age. EMBO Mol Med. (2014) 6:851–64. doi: 10.15252/emmm.201100899
25. Kong YW, Ferland-McCollough D, Jackson TJ, Bushell M. microRNAs in cancer management. Lancet Oncol. (2012) 13:e249–58. doi: 10.1016/S1470-2045(12)70073-6
26. Harrow J, Frankish A, Gonzalez JM, Tapanari E, Diekhans M, Kokocinski F, et al. GENCODE: the reference human genome annotation for The ENCODE project. Genome Res. (2012) 22:1760–74. doi: 10.1101/gr.135350.111
27. Frankish A, Diekhans M, Ferreira AM, Johnson R, Jungreis I, Loveland J, et al. GENCODE reference annotation for the human and mouse genomes. Nucleic Acids Res. (2019) 47:D766–73. doi: 10.1093/nar/gky955
28. Uszczynska-Ratajczak B, Lagarde J, Frankish A, Guigo R, Johnson R. Towards a complete map of the human long non-coding RNA transcriptome. Nat Rev Genet. (2018) 19:535–48. doi: 10.1038/s41576-018-0017-y
29. GENCODE. Available from: https://www.gencodegenes.org.
30. Huarte M. The emerging role of lncRNAs in cancer. Nat Med. (2015) 21:1253–61. doi: 10.1038/nm.3981
31. Sanchez Calle A, Kawamura Y, Yamamoto Y, Takeshita F, Ochiya T. Emerging roles of long non-coding RNA in cancer. Cancer Sci. (2018) 109:2093–100. doi: 10.1111/cas.13642
32. Lin C, Yang L. Long noncoding RNA in cancer: wiring signaling circuitry. Trends Cell Biol. (2018) 28:287–301. doi: 10.1016/j.tcb.2017.11.008
33. Mercer TR, Dinger ME, Mattick JS. Long non-coding RNAs: insights into functions. Nat Rev Genet. (2009) 10:155–9. doi: 10.1038/nrg2521
34. Wang KC, Chang HY. Molecular mechanisms of long noncoding RNAs. Mol Cell. (2011) 43:904–14. doi: 10.1016/j.molcel.2011.08.018
35. Shen P, Pichler M, Chen M, Calin GA, Ling H. To Wnt or lose: the missing non-coding linc in colorectal cancer. Int J Mol Sci. (2017) 18:E2003. doi: 10.3390/ijms18092003
36. Arun G, Diermeier SD, Spector DL. Therapeutic targeting of long non-coding RNAs in cancer. Trends Mol Med. (2018) 24:257–77. doi: 10.1016/j.molmed.2018.01.001
37. Qi P, Du X. The long non-coding RNAs, a new cancer diagnostic and therapeutic gold mine. Mod Pathol. (2013) 26:155–65. doi: 10.1038/modpathol.2012.160
38. Bhan A, Soleimani M, Mandal SS. Long noncoding RNA and cancer: a new paradigm. Cancer Res. (2017) 77:3965–81. doi: 10.1158/0008-5472.CAN-16-2634
39. Cabili MN, Trapnell C, Goff L, Koziol M, Tazon-Vega B, Regev A, et al. Integrative annotation of human large intergenic noncoding RNAs reveals global properties and specific subclasses. Genes Dev. (2011) 25:1915–27. doi: 10.1101/gad.17446611
40. Bolha L, Ravnik-Glavac M, Glavac D. Long noncoding RNAs as biomarkers in cancer. Dis Markers. (2017) 2017:7243968. doi: 10.1155/2017/7243968
41. Cory S, Graham M, Webb E, Corcoran L, Adams JM. Variant (6;15) translocations in murine plasmacytomas involve a chromosome 15 locus at least 72 kb from the c-myc oncogene. EMBO J. (1985) 4:675–81. doi: 10.1002/j.1460-2075.1985.tb03682.x
42. Graham M, Adams JM. Chromosome 8 breakpoint far 3' of the c-myc oncogene in a Burkitt's lymphoma 2;8 variant translocation is equivalent to the murine pvt-1 locus. EMBO J. (1986) 5:2845–51. doi: 10.1002/j.1460-2075.1986.tb04578.x
43. Boerma EG, Siebert R, Kluin PM, Baudis M. Translocations involving 8q24 in Burkitt lymphoma and other malignant lymphomas: a historical review of cytogenetics in the light of todays knowledge. Leukemia. (2009) 23:225–34. doi: 10.1038/leu.2008.281
44. Nagoshi H, Taki T, Hanamura I, Nitta M, Otsuki T, Nishida K, et al. Frequent PVT1 rearrangement and novel chimeric genes PVT1-NBEA and PVT1-WWOX occur in multiple myeloma with 8q24 abnormality. Cancer Res. (2012) 72:4954–62. doi: 10.1158/0008-5472.CAN-12-0213
45. Cui M, You L, Ren X, Zhao W, Liao Q, Zhao Y. Long non-coding RNA PVT1 and cancer. Biochem Biophys Res Commun. (2016) 471:10–4. doi: 10.1016/j.bbrc.2015.12.101
46. Cerami E, Gao J, Dogrusoz U, Gross BE, Sumer SO, Aksoy BA, et al. The cBio cancer genomics portal: an open platform for exploring multidimensional cancer genomics data. Cancer Discov. (2012) 2:401–4. doi: 10.1158/2159-8290.CD-12-0095
47. cBioPortal for Cancer Genomics. Available from: http://www.cbioportal.org/.
48. Yao L, Tak YG, Berman BP, Farnham PJ. Functional annotation of colon cancer risk SNPs. Nat Commun. (2014) 5:5114. doi: 10.1038/ncomms6114
49. Huppi K, Volfovsky N, Runfola T, Jones TL, Mackiewicz M, Martin SE, et al. The identification of microRNAs in a genomically unstable region of human chromosome 8q24. Mol Cancer Res. (2008) 6:212–21. doi: 10.1158/1541-7786.MCR-07-0105
50. Barsotti AM, Beckerman R, Laptenko O, Huppi K, Caplen NJ, Prives C. p53-Dependent induction of PVT1 and miR-1204. J Biol Chem. (2012) 287:2509–19. doi: 10.1074/jbc.M111.322875
51. Beck-Engeser GB, Lum AM, Huppi K, Caplen NJ, Wang BB, Wabl M. Pvt1-encoded microRNAs in oncogenesis. Retrovirology. (2008) 5:4. doi: 10.1186/1742-4690-5-4
52. Chen L, Lu MH, Zhang D, Hao NB, Fan YH, Wu YY, et al. miR-1207-5p and miR-1266 suppress gastric cancer growth and invasion by targeting telomerase reverse transcriptase. Cell Death Dis. (2014) 5:e1034. doi: 10.1038/cddis.2013.553
53. Volders PJ, Anckaert J, Verheggen K, Nuytens J, Martens L, Mestdagh P, et al. LNCipedia 5: towards a reference set of human long non-coding RNAs. Nucleic Acids Res. (2019) 47:D135–9. doi: 10.1093/nar/gky1031
54. Ensembl Genome Browser. Available from: https://www.ensembl.org/
55. Genotype-Tissue Expression (GTEx) project. Available from: https://gtexportal.org.
56. Consortium GT. The genotype-tissue expression (GTEx) project. Nat Genet. (2013) 45:580–5. doi: 10.1038/ng.2653
57. The Cancer Genome Atlas (TCGA). Available from: https://cancergenome.nih.gov
58. Cancer Genome Atlas N. Comprehensive molecular characterization of human colon and rectal cancer. Nature. (2012) 487:330–7. doi: 10.1038/nature11252
59. Zheng J, Yu F, Dong P, Wu L, Zhang Y, Hu Y, et al. Long non-coding RNA PVT1 activates hepatic stellate cells through competitively binding microRNA-152. Oncotarget. (2016) 7:62886–97. doi: 10.18632/oncotarget.11709
60. Li T, Meng XL, Yang WQ. Long noncoding RNA PVT1 acts as a “sponge” to inhibit microRNA-152 in gastric cancer cells. Digest Dis Sci. (2017) 62:3021–8. doi: 10.1007/s10620-017-4508-z
61. Zhou Q, Chen F, Zhao J, Li B, Liang Y, Pan W, et al. Long non-coding RNA PVT1 promotes osteosarcoma development by acting as a molecular sponge to regulate miR-195. Oncotarget. (2016) 7:82620–33. doi: 10.18632/oncotarget.13012
62. Wu D, Li Y, Zhang H, Hu X. Knockdown of Lncrna PVT1 enhances radiosensitivity in non-small cell lung cancer by sponging Mir-195. Cell Physiol Biochem. (2017) 42:2453–66. doi: 10.1159/000480209
63. Shen CJ, Cheng YM, Wang CL. LncRNA PVT1 epigenetically silences miR-195 and modulates EMT and chemoresistance in cervical cancer cells. J Drug Target. (2017) 25:637–44. doi: 10.1080/1061186X.2017.1307379
64. Kong F, Ma J, Yang H, Yang D, Wang C, Ma X. Long non-coding RNA PVT1 promotes malignancy in human endometrial carcinoma cells through negative regulation of miR-195-5p. Biochim Biophys Acta Mol Cell Res. (2018) 1865:1479–90. doi: 10.1016/j.bbamcr.2018.07.008
65. Li PD, Hu JL, Ma C, Ma H, Yao J, Chen LL, et al. Upregulation of the long non-coding RNA PVT1 promotes esophageal squamous cell carcinoma progression by acting as a molecular sponge of miR-203 and LASP1. Oncotarget. (2017) 8:34164–76. doi: 10.18632/oncotarget.15878
66. Takahashi Y, Sawada G, Kurashige J, Uchi R, Matsumura T, Ueo H, et al. Amplification of PVT-1 is involved in poor prognosis via apoptosis inhibition in colorectal cancers. Br J Cancer. (2014) 110:164–71. doi: 10.1038/bjc.2013.698
67. Wang C, Zhu X, Pu C, Song X. Upregulated plasmacytoma variant translocation 1 promotes cell proliferation, invasion and metastasis in colorectal cancer. Mol Med Rep. (2018) 17:6598–604. doi: 10.3892/mmr.2018.8669
68. He F, Song Z, Chen H, Chen Z, Yang P, Li W, et al. Long noncoding RNA PVT1-214 promotes proliferation and invasion of colorectal cancer by stabilizing Lin28 and interacting with miR-128. Oncogene. (2018) 38:164–79. doi: 10.1038/s41388-018-0432-8
69. Ozawa T, Matsuyama T, Toiyama Y, Takahashi N, Ishikawa T, Uetake H, et al. CCAT1 and CCAT2 long noncoding RNAs, located within the 8q.24.21 'gene desert', serve as important prognostic biomarkers in colorectal cancer. Ann Oncol. (2017) 28:1882–8. doi: 10.1093/annonc/mdx248
70. Ping G, Xiong W, Zhang L, Li Y, Zhang Y, Zhao Y. Silencing long noncoding RNA PVT1 inhibits tumorigenesis and cisplatin resistance of colorectal cancer. Am J Transl Res. (2018) 10:138–49.
71. Guo K, Yao J, Yu Q, Li Z, Huang H, Cheng J, et al. The expression pattern of long non-coding RNA PVT1 in tumor tissues and in extracellular vesicles of colorectal cancer correlates with cancer progression. Tumour Biol. (2017) 39:1010428317699122. doi: 10.1177/1010428317699122
72. Chai J, Guo D, Ma W, Han D, Dong W, Guo H, et al. A feedback loop consisting of RUNX2/LncRNA-PVT1/miR-455 is involved in the progression of colorectal cancer. Am J Cancer Res. (2018) 8:538–50.
73. Zhang R, Li J, Yan X, Jin K, Li W, Liu X, et al. Long noncoding RNA plasmacytoma variant translocation 1 (PVT1) promotes colon cancer progression via endogenous sponging mir-26b. Med Sci Monitor. (2018) 24:8685–92. doi: 10.12659/MSM.910955
74. Gharib E, Anaraki F, Baghdar K, Ghavidel P, Sadeghi H, Nasrabadi PN, et al. Investigating the diagnostic performance of HOTTIP, PVT1, and UCA1 long noncoding RNAs as a predictive panel for the screening of colorectal cancer patients with lymph node metastasis. J Cell Biochem. (2019) 120:14780–90. doi: 10.1002/jcb.28739
75. Xu MD, Wang Y, Weng W, Wei P, Qi P, Zhang Q, et al. A positive feedback loop of lncRNA-PVT1 and FOXM1 facilitates gastric cancer growth and invasion. Clin Cancer Res. (2017) 23:2071–80. doi: 10.1158/1078-0432.CCR-16-0742
76. Huang T, Liu HW, Chen JQ, Wang SH, Hao LQ, Liu M, et al. The long noncoding RNA PVT1 functions as a competing endogenous RNA by sponging miR-186 in gastric cancer. Biomed Pharmacother. (2017) 88:302–8. doi: 10.1016/j.biopha.2017.01.049
77. Zhao J, Du P, Cui P, Qin Y, Hu C, Wu J, et al. LncRNA PVT1 promotes angiogenesis via activating the STAT3/VEGFA axis in gastric cancer. Oncogene. (2018) 37:4094–109. doi: 10.1038/s41388-018-0250-z
78. Kong R, Zhang EB, Yin DD, You LH, Xu TP, Chen WM, et al. Long noncoding RNA PVT1 indicates a poor prognosis of gastric cancer and promotes cell proliferation through epigenetically regulating p15 and p16. Mol Cancer. (2015) 14:82. doi: 10.1186/s12943-015-0355-8
79. Zhao S, Fan NF, Chen XH, Zhuo CH, Xu CW, Lin RB. Long noncoding RNA PVT1-214 enhances gastric cancer progression by upregulating TrkC expression in competitively sponging way. Euro Rev Med Pharmacol Sci. (2019) 23:4173–84. doi: 10.26355/eurrev_201905_17920
80. Zeng Y, Wang T, Liu Y, Su Z, Lu P, Chen X, et al. LncRNA PVT1 as an effective biomarker for cancer diagnosis and detection based on transcriptome data and meta-analysis. Oncotarget. (2017) 8:75455–66. doi: 10.18632/oncotarget.20634
81. Farhana L, Antaki F, Anees MR, Nangia-Makker P, Judd S, Hadden T, et al. Role of cancer stem cells in racial disparity in colorectal cancer. Cancer Med. (2016) 5:1268–78. doi: 10.1002/cam4.690
82. Wierstra I, Alves J. FOXM1, a typical proliferation-associated transcription factor. Biol Chem. (2007) 388:1257–74. doi: 10.1515/BC.2007.159
83. Katoh M, Igarashi M, Fukuda H, Nakagama H, Katoh M. Cancer genetics and genomics of human FOX family genes. Cancer Lett. (2013) 328:198–206. doi: 10.1016/j.canlet.2012.09.017
84. Raychaudhuri P, Park HJ. FoxM1: a master regulator of tumor metastasis. Cancer Res. (2011) 71:4329–33. doi: 10.1158/0008-5472.CAN-11-0640
85. Laissue P. The forkhead-box family of transcription factors: key molecular players in colorectal cancer pathogenesis. Mol Cancer. (2019) 18:5. doi: 10.1186/s12943-019-0938-x
86. Zona S, Bella L, Burton MJ, Nestal de Moraes G, Lam EW. FOXM1: an emerging master regulator of DNA damage response and genotoxic agent resistance. Biochim Biophys Acta. (2014) 1839:1316–22. doi: 10.1016/j.bbagrm.2014.09.016
87. Yu H, Pardoll D, Jove R. STATs in cancer inflammation and immunity: a leading role for STAT3. Nat Rev Cancer. (2009) 9:798–809. doi: 10.1038/nrc2734
88. Pfister NT, Prives C. Transcriptional regulation by wild-type and cancer-related mutant forms of p53. Cold Spring Harb Perspect Med. (2017) 7:26054. doi: 10.1101/cshperspect.a026054
89. Li XL, Zhou J, Chen ZR, Chng WJ. P53 mutations in colorectal cancer - molecular pathogenesis and pharmacological reactivation. World J Gastroenterol. (2015) 21:84–93. doi: 10.3748/wjg.v21.i1.84
90. Martincorena I, Raine KM, Gerstung M, Dawson KJ, Haase K, Van Loo P, et al. Universal patterns of selection in cancer and somatic tissues. Cell. (2017) 171:1029–41.e21. doi: 10.1016/j.cell.2017.09.042
91. Bailey MH, Tokheim C, Porta-Pardo E, Sengupta S, Bertrand D, Weerasinghe A, et al. Comprehensive characterization of cancer driver genes and mutations. Cell. (2018) 174:1034–5. doi: 10.1016/j.cell.2018.07.034
92. Muller PA, Vousden KH. p53 mutations in cancer. Nat Cell Biol. (2013) 15:2–8. doi: 10.1038/ncb2641
93. Beroukhim R, Mermel CH, Porter D, Wei G, Raychaudhuri S, Donovan J, et al. The landscape of somatic copy-number alteration across human cancers. Nature. (2010) 463:899–905. doi: 10.1038/nature08822
94. Huppi K, Pitt JJ, Wahlberg BM, Caplen NJ. The 8q24 gene desert: an oasis of non-coding transcriptional activity. Front Genet. (2012) 3:69. doi: 10.3389/fgene.2012.00069
95. Jin DH, Park SE, Lee J, Kim KM, Kim S, Kim DH, et al. Copy number gains at 8q24 and 20q11-q13 in gastric cancer are more common in intestinal-type than diffuse-type. PLoS ONE. (2015) 10:e0137657. doi: 10.1371/journal.pone.0137657
96. Grisanzio C, Freedman ML. Chromosome 8q24-associated cancers and MYC. Genes Cancer. (2010) 1:555–9. doi: 10.1177/1947601910381380
97. Flanagan DJ, Vincan E, Phesse TJ. Winding back Wnt signalling: potential therapeutic targets for treating gastric cancers. Br J Pharmacol. (2017) 174:4666–83. doi: 10.1111/bph.13890
98. Tseng YY, Moriarity BS, Gong W, Akiyama R, Tiwari A, Kawakami H, et al. PVT1 dependence in cancer with MYC copy-number increase. Nature. (2014) 512:82–6. doi: 10.1038/nature13311
99. Colombo T, Farina L, Macino G, Paci P. PVT1: a rising star among oncogenic long noncoding RNAs. BioMed Res Int. (2015) 2015:304208. doi: 10.1155/2015/304208
100. Schwab M, Klempnauer KH, Alitalo K, Varmus H, Bishop M. Rearrangement at the 5′ end of amplified c-myc in human COLO 320 cells is associated with abnormal transcription. Mol Cell Biol. (1986) 6:2752–5. doi: 10.1128/MCB.6.7.2752
101. Shtivelman E, Bishop JM. The PVT gene frequently amplifies with MYC in tumor cells. Mol Cell Biol. (1989) 9:1148–54. doi: 10.1128/MCB.9.3.1148
102. Ahmadiyeh N, Pomerantz MM, Grisanzio C, Herman P, Jia L, Almendro V, et al. 8q24 prostate, breast, and colon cancer risk loci show tissue-specific long-range interaction with MYC. Proc Natl Acad Sci USA. (2010) 107:9742–6. doi: 10.1073/pnas.0910668107
103. Sotelo J, Esposito D, Duhagon MA, Banfield K, Mehalko J, Liao H, et al. Long-range enhancers on 8q24 regulate c-Myc. Proc Natl Acad Sci USA. (2010) 107:3001–5. doi: 10.1073/pnas.0906067107
104. Cho SW, Xu J, Sun R, Mumbach MR, Carter AC, Chen YG, et al. Promoter of lncRNA gene PVT1 is a tumor-suppressor DNA boundary element. Cell. (2018) 173:1398–412.e22. doi: 10.1016/j.cell.2018.03.068
105. Pombo A, Dillon N. Three-dimensional genome architecture: players and mechanisms. Nat Rev Mol Cell Biol. (2015) 16:245–57. doi: 10.1038/nrm3965
106. Parolia A, Cieslik M, Chinnaiyan AM. Competing for enhancers: PVT1 fine-tunes MYC expression. Cell Res. (2018) 28:785–6. doi: 10.1038/s41422-018-0064-0
107. Shang AQ, Wang WW, Yang YB, Gu CZ, Ji P, Chen C, et al. Knockdown of long noncoding RNA PVT1 suppresses cell proliferation and invasion of colorectal cancer via upregulation of microRNA-214-3p. Am J Physiol Gastrointest Liver Physiol. (2019) 317:G222–32. doi: 10.1152/ajpgi.00357.2018
108. Zhang XW, Bu P, Liu L, Zhang XZ, Li J. Overexpression of long non-coding RNA PVT1 in gastric cancer cells promotes the development of multidrug resistance. Biochem Biophys Res Commun. (2015) 462:227–32. doi: 10.1016/j.bbrc.2015.04.121
109. Carmeliet P. VEGF as a key mediator of angiogenesis in cancer. Oncology. (2005) 69(Suppl 3):4–10. doi: 10.1159/000088478
110. Sitohy B, Nagy JA, Dvorak HF. Anti-VEGF/VEGFR therapy for cancer: reassessing the target. Cancer Res. (2012) 72:1909–14. doi: 10.1158/0008-5472.CAN-11-3406
111. Lupo G, Caporarello N, Olivieri M, Cristaldi M, Motta C, Bramanti V, et al. Anti-angiogenic therapy in cancer: downsides and new pivots for precision medicine. Front Pharmacol. (2016) 7:519. doi: 10.3389/fphar.2016.00519
112. Nienhuser H, Schmidt T. Angiogenesis and anti-angiogenic therapy in gastric cancer. Int J Mol Sci. (2017) 19:43. doi: 10.3390/ijms19010043
113. Han J, Xiong J, Wang D, Fu XD. Pre-mRNA splicing: where and when in the nucleus. Trends Cell Biol. (2011) 21:336–43. doi: 10.1016/j.tcb.2011.03.003
114. Nandakumar J, Cech TR. Finding the end: recruitment of telomerase to telomeres. Nat Rev Mol Cell Biol. (2013) 14:69–82. doi: 10.1038/nrm3505
115. Lu MH, Deng JQ, Cao YL, Fang DC, Zhang Y, Yang SM. Prognostic role of telomerase activity in gastric adenocarcinoma: a meta-analysis. Exp Ther Med. (2012) 3:728–34. doi: 10.3892/etm.2012.471
116. Fabregat I, Malfettone A, Soukupova J. New insights into the crossroads between EMT and stemness in the context of cancer. J Clin Med. (2016) 5:E37. doi: 10.3390/jcm5030037
117. Balas MM, Johnson AM. Exploring the mechanisms behind long noncoding RNAs and cancer. Noncoding RNA Res. (2018) 3:108–17. doi: 10.1016/j.ncrna.2018.03.001
118. Zhai R, Kan X, Wang B, Du H, Long Y, Wu H, et al. miR-152 suppresses gastric cancer cell proliferation and motility by targeting CD151. Tumour Biol. (2014) 35:11367–73. doi: 10.1007/s13277-014-2471-2
119. Sadej R, Grudowska A, Turczyk L, Kordek R, Romanska HM. CD151 in cancer progression and metastasis: a complex scenario. Lab Invest. (2014) 94:41–51. doi: 10.1038/labinvest.2013.136
120. Akl MR, Nagpal P, Ayoub NM, Tai B, Prabhu SA, Capac CM, et al. Molecular and clinical significance of fibroblast growth factor 2 (FGF2 /bFGF) in malignancies of solid and hematological cancers for personalized therapies. Oncotarget. (2016) 7:44735–62. doi: 10.18632/oncotarget.8203
121. Li B, Xie Z, Li B. miR-152 functions as a tumor suppressor in colorectal cancer by targeting PIK3R3. Tumour Biol. (2016) 37:10075–84. doi: 10.1007/s13277-016-4888-2
122. Ghazanchaei A, Mansoori B, Mohammadi A, Biglari A, Baradaran B. Restoration of miR-152 expression suppresses cell proliferation, survival, and migration through inhibition of AKT-ERK pathway in colorectal cancer. J Cell Physiol. (2018) 234:769–76. doi: 10.1002/jcp.26891
123. Cao C, Sun D, Zhang L, Song L. miR-186 affects the proliferation, invasion and migration of human gastric cancer by inhibition of Twist1. Oncotarget. (2016) 7:79956–63. doi: 10.18632/oncotarget.13182
124. Liu L, Wang Y, Bai R, Yang K, Tian Z. MiR-186 inhibited aerobic glycolysis in gastric cancer via HIF-1alpha regulation. Oncogenesis. (2016) 5:e224. doi: 10.1038/oncsis.2016.35
125. Majmundar AJ, Wong WJ, Simon MC. Hypoxia-inducible factors and the response to hypoxic stress. Mol Cell. (2010) 40:294–309. doi: 10.1016/j.molcel.2010.09.022
126. Yoshida Y, Takahashi K, Okita K, Ichisaka T, Yamanaka S. Hypoxia enhances the generation of induced pluripotent stem cells. Cell Stem Cell. (2009) 5:237–41. doi: 10.1016/j.stem.2009.08.001
127. Li J, Xia L, Zhou Z, Zuo Z, Xu C, Song H, et al. MiR-186-5p upregulation inhibits proliferation, metastasis and epithelial-to-mesenchymal transition of colorectal cancer cell by targeting ZEB1. Arch Biochem Biophys. (2018) 640:53–60. doi: 10.1016/j.abb.2018.01.002
128. Islam F, Gopalan V, Vider J, Wahab R, Ebrahimi F, Lu CT, et al. MicroRNA-186-5p overexpression modulates colon cancer growth by repressing the expression of the FAM134B tumour inhibitor. Exp Cell Res. (2017) 357:260–70. doi: 10.1016/j.yexcr.2017.05.021
129. Mazeh H, Mizrahi I, Ilyayev N, Halle D, Brucher B, Bilchik A, et al. The diagnostic and prognostic role of microRNA in colorectal cancer - a comprehensive review. J Cancer. (2013) 4:281–95. doi: 10.7150/jca.5836
130. Wang J, Lu Y, Zeng Y, Zhang L, Ke K, Guo Y. Expression profile and biological function of miR-455-5p in colorectal carcinoma. Oncol Lett. (2019) 17:2131–40. doi: 10.3892/ol.2018.9862
131. Zhang J, Roberts TM, Shivdasani RA. Targeting PI3K signaling as a therapeutic approach for colorectal cancer. Gastroenterology. (2011) 141:50–61. doi: 10.1053/j.gastro.2011.05.010
132. Ning T, Peng Z, Li S, Qu Y, Zhang H, Duan J, et al. miR-455 inhibits cell proliferation and migration via negative regulation of EGFR in human gastric cancer. Oncol Rep. (2017) 38:175–82. doi: 10.3892/or.2017.5657
133. Liu J, Zhang J, Li Y, Wang L, Sui B, Dai D. MiR-455-5p acts as a novel tumor suppressor in gastric cancer by down-regulating RAB18. Gene. (2016) 592:308–15. doi: 10.1016/j.gene.2016.07.034
134. Balzeau J, Menezes MR, Cao S, Hagan JP. The LIN28/let-7 pathway in cancer. Front Genet. (2017) 8:31. doi: 10.3389/fgene.2017.00031
135. Tu HC, Schwitalla S, Qian Z, LaPier GS, Yermalovich A, Ku YC, et al. LIN28 cooperates with WNT signaling to drive invasive intestinal and colorectal adenocarcinoma in mice and humans. Genes Dev. (2015) 29:1074–86. doi: 10.1101/gad.256693.114
136. Zhang H, Zong Y, Qiu G, Jia R, Xu X, Wang F, et al. Silencing Lin28 promotes apoptosis in colorectal cancer cells by upregulating let7c targeting of antiapoptotic BCL2L1. Mol Med Rep. (2018) 17:5143–9. doi: 10.3892/mmr.2018.8483
137. Yu WW, Jiang H, Zhang CT, Peng Y. The SNAIL/miR-128 axis regulated growth, invasion, metastasis, and epithelial-to-mesenchymal transition of gastric cancer. Oncotarget. (2017) 8:39280–95. doi: 10.18632/oncotarget.16849
138. Bardelli A, Parsons DW, Silliman N, Ptak J, Szabo S, Saha S, et al. Mutational analysis of the tyrosine kinome in colorectal cancers. Science. (2003) 300:949. doi: 10.1126/science.1082596
139. Luo Y, Kaz AM, Kanngurn S, Welsch P, Morris SM, Wang J, et al. NTRK3 is a potential tumor suppressor gene commonly inactivated by epigenetic mechanisms in colorectal cancer. PLoS Genet. (2013) 9:e1003552. doi: 10.1371/journal.pgen.1003552
140. Vigneri PG, Tirro E, Pennisi MS, Massimino M, Stella S, Romano C, et al. The insulin/IGF system in colorectal cancer development and resistance to therapy. Front Oncol. (2015) 5:230. doi: 10.3389/fonc.2015.00230
141. Li H, Batth IS, Qu X, Xu L, Song N, Wang R, et al. IGF-IR signaling in epithelial to mesenchymal transition and targeting IGF-IR therapy: overview and new insights. Mol Cancer. (2017) 16:6. doi: 10.1186/s12943-016-0576-5
142. Taniguchi CM, Emanuelli B, Kahn CR. Critical nodes in signalling pathways: insights into insulin action. Nat Rev Mol Cell Biol. (2006) 7:85–96. doi: 10.1038/nrm1837
143. Metz HE, Houghton AM. Insulin receptor substrate regulation of phosphoinositide 3-kinase. Clin Cancer Res. (2011) 17:206–11. doi: 10.1158/1078-0432.CCR-10-0434
144. Yang TS, Yang XH, Wang XD, Wang YL, Zhou B, Song ZS. MiR-214 regulate gastric cancer cell proliferation, migration and invasion by targeting PTEN. Cancer Cell Int. (2013) 13:68. doi: 10.1186/1475-2867-13-68
145. Li HL, Liang S, Cui JH, Han GY. Targeting of GSK-3beta by miR-214 to facilitate gastric cancer cell proliferation and decrease of cell apoptosis. Euro Rev Med Pharmacol Sci. (2018) 22:127–34. doi: 10.26355/eurrev_201801_14109
146. Yu H, Lee H, Herrmann A, Buettner R, Jove R. Revisiting STAT3 signalling in cancer: new and unexpected biological functions. Nat Rev Cancer. (2014) 14:736–46. doi: 10.1038/nrc3818
147. Furqan M, Akinleye A, Mukhi N, Mittal V, Chen Y, Liu D. STAT inhibitors for cancer therapy. J Hematol Oncol. (2013) 6:90. doi: 10.1186/1756-8722-6-90
148. Morlando M, Fatica A. Alteration of epigenetic regulation by long noncoding RNAs in cancer. Int J Mol Sci. (2018) 19:E570. doi: 10.3390/ijms19020570
149. Khalil AM, Guttman M, Huarte M, Garber M, Raj A, Rivea Morales D, et al. Many human large intergenic noncoding RNAs associate with chromatin-modifying complexes and affect gene expression. Proc Natl Acad Sci USA. (2009) 106:11667–72. doi: 10.1073/pnas.0904715106
150. Comet I, Riising EM, Leblanc B, Helin K. Maintaining cell identity: PRC2-mediated regulation of transcription and cancer. Nat Rev Cancer. (2016) 16:803–10. doi: 10.1038/nrc.2016.83
151. The UCSC Xena Browser. Available from: https://xenabrowser.net/transcripts/.
152. Ansari H, Shahrisa A, Birgani YT, Birgani MT, Hajjari M, Asl JM. Long noncoding RNAs in colorectal adenocarcinoma; an in silico analysis. Pathol Oncol Res. (2018) 25:1387–94. doi: 10.1007/s12253-018-0428-2
153. Holohan C, Van Schaeybroeck S, Longley DB, Johnston PG. Cancer drug resistance: an evolving paradigm. Nat Rev Cancer. (2013) 13:714–26. doi: 10.1038/nrc3599
154. Hammond WA, Swaika A, Mody K. Pharmacologic resistance in colorectal cancer: a review. Ther Adv Med Oncol. (2016) 8:57–84. doi: 10.1177/1758834015614530
155. Van der Jeught K, Xu HC, Li YJ, Lu XB, Ji G. Drug resistance and new therapies in colorectal cancer. World J Gastroenterol. (2018) 24:3834–48. doi: 10.3748/wjg.v24.i34.3834
156. Shi WJ, Gao JB. Molecular mechanisms of chemoresistance in gastric cancer. World J Gastrointest Oncol. (2016) 8:673–81. doi: 10.4251/wjgo.v8.i9.673
157. Fan H, Zhu JH, Yao XQ. Knockdown of long noncoding RNA PVT1 reverses multidrug resistance in colorectal cancer cells. Mol Med Rep. (2018) 17:8309–15. doi: 10.3892/mmr.2018.8907
158. Chen Z, Cai X, Chang L, Xia Y, Wang L, Hou Y, et al. LINC00152 is a potential biomarker involved in the modulation of biological characteristics of residual colorectal cancer cells following chemoradiotherapy. Oncol Lett. (2018) 15:4177–84. doi: 10.3892/ol.2018.7833
Keywords: PVT1, lncRNA, siRNA, ceRNA, Myc, colorectal/gastric cancer
Citation: Martínez-Barriocanal Á, Arango D and Dopeso H (2020) PVT1 Long Non-coding RNA in Gastrointestinal Cancer. Front. Oncol. 10:38. doi: 10.3389/fonc.2020.00038
Received: 18 April 2019; Accepted: 09 January 2020;
Published: 31 January 2020.
Edited by:
Ihab Younis, Carnegie Mellon University in Qatar, QatarReviewed by:
Yuchen Liu, Shenzhen University, ChinaCopyright © 2020 Martínez-Barriocanal, Arango and Dopeso. This is an open-access article distributed under the terms of the Creative Commons Attribution License (CC BY). The use, distribution or reproduction in other forums is permitted, provided the original author(s) and the copyright owner(s) are credited and that the original publication in this journal is cited, in accordance with accepted academic practice. No use, distribution or reproduction is permitted which does not comply with these terms.
*Correspondence: Águeda Martínez-Barriocanal, YWd1ZWRhLm1hcnRpbmV6QHZoaXIub3Jn; Diego Arango, ZGllZ28uYXJhbmdvQHZoaXIub3Jn; Higinio Dopeso, ZG9wZXNvZ2pAbXNrY2Mub3Jn
Disclaimer: All claims expressed in this article are solely those of the authors and do not necessarily represent those of their affiliated organizations, or those of the publisher, the editors and the reviewers. Any product that may be evaluated in this article or claim that may be made by its manufacturer is not guaranteed or endorsed by the publisher.
Research integrity at Frontiers
Learn more about the work of our research integrity team to safeguard the quality of each article we publish.