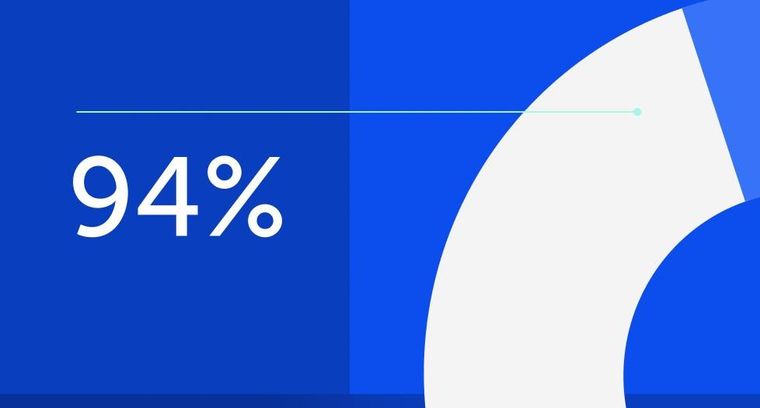
94% of researchers rate our articles as excellent or good
Learn more about the work of our research integrity team to safeguard the quality of each article we publish.
Find out more
REVIEW article
Front. Oncol., 24 October 2019
Sec. Molecular and Cellular Oncology
Volume 9 - 2019 | https://doi.org/10.3389/fonc.2019.01091
This article is part of the Research TopicNeovascularization, Angiogenesis and Vasculogenic Mimicry in CancerView all 25 articles
Angiogenesis is a crucial process for organ morphogenesis and growth during development, and it is especially relevant during the repair of wounded tissue in adults. It is coordinated by an equilibrium of pro- and anti-angiogenic factors; nevertheless, when affected, it promotes several diseases. Lately, a growing body of evidence is indicating that non-coding RNAs (ncRNAs), such as miRNAs, circRNAs, and lncRNAs, play critical roles in angiogenesis. These ncRNAs can act in cis or trans and alter gene transcription by several mechanisms including epigenetic processes. In the following pages, we will discuss the functions of ncRNAs in the regulation of angiogenesis and neovascularization, both in normal and disease contexts, from an epigenetic perspective. Additionally, we will describe the contribution of Next-Generation Sequencing (NGS) techniques to the discovery and understanding of the role of ncRNAs in angiogenesis.
In the vascular network, blood vessels act as channels for nutrients, oxygen delivery, and metabolic waste evacuation. The growth of new capillary vessels, known as angiogenesis, plays key roles in embryonic development and in tissue homeostasis and remodeling in adults, as well as in cancer initiation and progression (1, 2). The balance between pro- and anti-angiogenic factors (such as VEGF, PDGF, and TSP-1/2) coordinates angiogenesis and other neovascularization mechanisms such as intussusceptive angiogenesis, vasculogenesis, lymphangiogenesis, vessel co-option, and vasculogenic mimicry (3–5).
Over the last few decades, the study of angiogenesis has helped researchers to understand vascular physiology and its implications for several diseases. For instance, in atherosclerosis, ischemia, and retinopathy, excessive or insufficient vascular growth can affect the behavior of endothelial and smooth muscle cells (6, 7). Studies of the neovascularization processes have also provided molecular targets for the development of therapies to delay cancer progression, since it is well-known that angiogenesis is an essential process that is altered in tumors (8).
Nowadays, the study of the molecular mechanisms involved in angiogenesis is being built on different experimental approaches, such as cell migration, proliferation, and metabolic assays or histological and tri-dimensional models, that approach specific stages of angiogenesis; however, only pieces of the puzzle have been elucidated (9). With advances in high-throughput genomic technologies such as microarrays, next-generation sequencing (NGS), and bioinformatic analyses, a genome-wide perspective of the elements involved in the angiogenic process is now being taken. Some of the newest players revealed by these approaches are non-coding RNAs (ncRNAs), which have gained relevance in the field of epigenetics (10–12). Therefore, in this review, we will describe the epigenetic regulatory functions of ncRNAs in physiological angiogenesis and vascular diseases, as well as the contribution of NGS technologies to the discovery of new roles for ncRNAs that are associated with angiogenesis.
In 1939, the term “epigenetics” was coined by Conrad Hal Waddington (13). Today, one of the most accepted definitions of the term explains that “epigenetics is the study of the heritable changes in gene expression that cannot be explained by alterations in the DNA sequence” (14). Among the epigenetic components that coordinate nucleus organization and gene transcription are DNA methylation, histone post-translational modifications (PTMs), and histone positioning, but recently, ncRNAs have been incorporated as epigenetic modifiers, because many of these can function as scaffolding elements to transport proteins with epigenetic functions (15). Each of these processes is stimulated by the signals derived from a dynamic epigenetic code that is established on the chromatin depending on the physiological and extracellular context. The writers, readers, and erasers of this code are proteins that place, recognize, or remove chemical modifications of DNA nucleotides and within the amino-terminal regions of histones. Most chromatin “writers” are methyltransferases that catalyze the transfer of methyl groups. DNA methylation occurs predominantly in regions enriched in CpG sites. The occurrence of methylation at the promoter regions of genes is associated with gene silencing. PTMs alter the regulation of gene transcription by changing the structure of chromatin depending on the particular residue that is modified (16, 17). The “readers” are proteins that recognize and associate with the epigenetic modifications, interpret them, and, in many cases, promote the assembly of protein complexes. The erasers remove the modifications and, therefore, alter signaling components that contribute to the regulation of gene expression. Recently, it has been reported that ncRNAs can mediate the binding of epigenetic proteins to their target sequences. Though they do not function alone as “classic” epigenetic modifiers, they play a vital role in both the recruitment and transcriptional regulation of epigenetic modifiers (18). In fact, multiple chromatin-remodeling enzymes have been shown to directly contact ncRNAs, including Enhancer of Zeste Homolog 2 (EZH2) and Suppressor of Zeste 12 Protein Homolog (SUZ12) (writer and eraser within the Polycomb repressive complex 2/PRC2, respectively), and nuclear architectural proteins like Yin Yang 1 and CTCF, among others (19–22). The incorporation of ncRNAs as epigenetic elements has opened up new fields of study in which they have been shown to regulate gene expression. In the following pages, we will provide an overview of the ncRNAs involved in angiogenesis, focusing on those involved in epigenetic processes.
MicroRNAs (miRNAs) are short ncRNAs with a length of 19–23 nucleotides that are conserved in animals, plants, and some viruses (23–25). MiRNAs are transcribed as long pri-microRNAs (pri-miRNA) and are subsequently processed to ~70-nucleotide precursor hairpins (pre-miRNA) by the RNase Drosha (26). Pre-miRNAs are then exported to the cytoplasm and recognized by the RNase DICER, which removes the loop linking the 3′ and 5′ ends of the hairpin, producing a ~20-nucleotide miRNA duplex (27). Later, one of these strands is fused into the RNA Induced Silencing Complex (RISC), where both the miRNA and its messenger RNA (mRNA) target interact (28).
MiRNAs have two main functions: post-transcriptional gene regulation and RNA silencing. They act by pairing bases with a complementary sequence located in the 3′UTR region of target mRNA (29, 30). Consequently, these mRNAs are regulated by one or more mechanisms that include the inhibition of mRNA translation to proteins by ribosomes and by mRNA strand cleavage into two fragments and poly(A) tail shortening that results in mRNA disruption (29, 31). In the last 10 years, the field of miRNA biology has ignited, revealing amazing functions in angiogenesis. These miRNAs have been termed angiomiRs, and they target key angiogenesis molecular drivers, such as metalloproteinases, hypoxia inducible factor 1 (HIF1), cytokines, and growth factors, such as EGFL7, FGF11, PDGFRB, and the vascular endothelial growth factor (VEGF) family (32–34).
MiRNAs are not considered epigenetic components, but some of them are modulated by epigenetic mechanisms. This mainly affects their regulatory region through the incorporation of DNA methylation, repressive histone marks, or the loss of transcriptional factors, as has been reported for miR-125b1 and miR-124 (35, 36). Others, known as Epi-miRNAs, can also regulate the gene expression of epigenetic elements, DNA methyltransferases (DNMTs) (such as miR-152, miR-30, and miR-148a/b), histone deacetylases (HDACs) (such as miR-140, miR-1, and miR-449a), and the Polycomb Group of genes (such as miR-101 and miR-26a) (37–44), and some of them have been considered angiomiRs (39, 40). MiRNAs and their identified epigenetic targets in angiogenesis are listed in Table 1.
Transforming Growth Factor (TGF-β) is a relevant cytokine that functions in the process of vascular homeostasis and is involved in the vascular development of endothelial cells. It has been reported that the administration of TGF-β to endothelial cells leads to decreased miR-30a-3p expression. The absence of this microRNA results in increased levels of methyl-CpG-binding protein 2 (MeCP2), a protein associated with silencing of SIRT1 (45). SIRT1 is necessary for the migration of endothelial cells to occur throughout sprouting angiogenesis, and the loss of this enzyme induces abnormal angiogenesis in vivo (52). Conversely, increased levels of miR-30a-3p expression lead to the activation of SIRT1 expression (Figure 1A). Further experiments revealed that MeCP2 enhanced the methylation status of the SIRT1 promoter, probably by DNMT1 recruitment, leading to a reduction in SIRT1 expression and endothelial angiogenic defects (53).
Figure 1. The chromatin regulatory role of non-coding RNAs in angiogenesis. The miRNAs can interfere with the expression of key epigenetic players, leading to the (A) induction or (B) inhibition of angiogenesis. The lncRNAs regulate (C) the activity and (D) recruitment of chromatin-modifying complexes. MiRNAs are represented in blue panels, and lncRNAs are represented in green.
The microRNA miR-101 acts as a tumor suppressor, promoting apoptosis and inhibiting cell proliferation, angiogenesis, invasion, and metastasis. MiR-101 performs its regulatory functions by targeting an abundant range of epigenetic molecular effectors, such as DNMT3A, EZH2, and HDAC9 (54, 55). In endothelial cells, high levels of VEGF are associated with the downregulation of miR-101, allowing an increase in EZH2 (46). EZH2 is associated with the formation of heterochromatin and can affect multiple target genes such as Vasohibin 1 (VASH1), which functions as a negative feedback modulator of angiogenesis in vascular endothelial cells (56, 57) (Figure 1B). The overexpression miR-101 leads to EZH2 repression and the activation of VASH1 transcription. This evidence, taken together, suggests that miR-101 is involved in multiple processes such as cellular growth attenuation, migration, and invasion mechanisms and the ability of endothelial cells to form capillary-like structures in glioblastomas (47).
MiR-20a belongs to the miR-17-92 cluster and has been linked to breast cancer cells with a high angiogenic profile. High levels of miR-20a are correlated with complex vascular structures and larger vessels, suggesting that miR-20a could be used as a potential new angiogenic target (58). Additionally, overexpression of miR-20a affects the mRNA stability of the lysine acetyltransferase, p300. In mouse myocardium cells, p300 is a key factor that regulates angiogenic and hypertrophic programs, influencing the expression of many related genes, such as Hif,1 Vegfc, Vegfa, Angpt1, and Egln3. Interestingly, high p300 levels induce an increase in the expression of miR-20a, providing a feedback inhibition loop for p300 that prevents its pro-angiogenic effects (48).
MiR-137 has a tumor suppressor gene function that has been reported for several neoplasms (49, 59, 60). It was also reported that this miRNA can inhibit angiogenesis and cell proliferation by EZH2 downregulation in glioblastomas. Overexpression of miR-137 reduces the mRNA and protein levels of EZH2, while downregulation of miR-137 is associated with poor prognosis in affected patients (49).
The miRNA miR-124 is highly conserved, from nematodes to humans. Three human genes encoding miR-124 have previously been characterized (miR124a-1, miR-124a-2, and miR-124a-3) and the majority have been shown to be deregulated in neoplasms (61). Also, it has been shown that expression of miR-124 is elevated after treatment with certain drugs such as niclosamide. In this case, it is associated with the inhibition of vasculogenic mimicry formation, particularly by reducing levels of phosphorylated STAT3 (62).
Some reports propose that miR-124 suppresses the E3 ubiquitin ligase with PHD and RING finger domain 1 (UHRF1) expression, a factor involved in the recruitment of epigenetic components in bladder cancer tissues. Also, UHRF1 is known to enhance malignancy, inducing cellular proliferation, migration, and angiogenesis (63). MiR-124 overexpression resulted in UHRF1 suppression through the competitive binding of its 3'-UTR region. In addition, miR-124 overexpression attenuated tumor growth and cell proliferation in vivo and invasion, migration, and vasculogenic mimicry in vitro. Further, it reduced VEGF protein levels and levels of the matrix metalloproteinases MMP-2 and MMP-9. A matrigel assay in a three-dimensional culture revealed reductions in tubular channel formation when miR-124 was over-expressed in bladder cancer cell lines compared to the control group, suggesting that miR-124 indirectly regulates vasculogenic mimicry in bladder cancer (44).
Originating from intron 14 of the Dynamina-3 gene (DNM3), the primary transcript of miR-214 produces four different miRNAs (miR-199-3p, miR-199-5p, miR214-3p, and miR-214-5p) (64). During the endothelial differentiation of embryonic stem cells, the Brain-Derived Neurotrophic Factor (BDNF) promotes angiogenesis, in vitro and in vivo, by increasing levels of miR-214. The miR-214 inhibits EZH2 at the post-transcriptional level, leading to reductions in EZH2 occupancy at the NOS3 promoter (50). Also, miR-214 controls the BDNF-mediated upregulation of neuropilin 1, VEGF-R, and Crk-associated substrate kinase (50, 65). Thus, miR-214 is a downstream player within the BDNF signaling pathway that regulates important angiogenic targets.
miR-200b is part of the miR-200 family, which is organized into two main groups according to seed sequence. The miRNAs of group A are miR-141 and miR−200a, while the miRNAs in group B are miR-200b, miR−200c, and miR−429 (66). Particularly, miR-200b has been indicated to have a role in the process of angiogenesis. Studies of malignant neoplasms demonstrated that miR-200b controls the epithelial to mesenchymal transition by downregulating p300 (67–70). In addition, p300 activates HIF1, which is a transcriptional regulator of VEGF-A, and triggers the development of abundant blood vessels (71–73). Since miR-200b negatively regulates p300, this miRNA has antiangiogenic properties (51).
In sum, these studies suggest that miRNAs have the capacity to indirectly affect epigenetic pathways in endothelial cells and influence the angiogenic response. This opens up the possibility of considering miRNAs as therapeutic targets or biomarkers, an exciting prospect since therapies for both vascular diseases and cancer are needed. In several diseases, miRNAs have proven to be excellent biomarkers as a result of their high circulating levels. Indeed, analysis of oncogenic and suppressor miRNAs that are found in primary tumors against non-neoplastic cells revealed exosome-mediated sorting mechanisms related to cancer progression (74, 75). It is unknown whether similar mechanisms could be utilized by Epi-miRNAs during the evolution of vascular diseases. Recently, the attention of the scientific community has been focused on other, widely-studied ncRNAs known as long non-coding RNAs (lncRNAs), which have master regulatory functions in angiogenesis.
LncRNAs are all ncRNAs larger than 200 nucleotides and are classified according to their proximity to protein-coding genes as intergenic, intronic, bidirectional, sense, and antisense lncRNAs. Massive analyses have revealed that lncRNAs are originated using the same mechanisms as protein-coding genes; however, contrary to protein-coding genes, lncRNAs show a preference for having two-exon transcripts, and most of them lack any protein coding-potential. Also, lncRNAs show tissue-specific expression patterns and are predominantly located in the nucleus rather than the cytoplasm. In fact, there are several lines of evidence that suggest that lncRNAs are significantly more enriched in chromatin than miRNAs (76).
LncRNAs can indirectly modulate DNA methylation at CpG sites, which in turn regulates gene transcription. For example, Tsix recruits DNMT3a to methylate and silence the XIST promoter. XIST is an important effector involved in the inactivation of the X chromosome (77). Likewise, the lncRNA Kcnq1ot1 recruits the de novo DNA demethylase DNMT1 to control the methylation status of ubiquitously imprinted genes during mouse development (78). LncRNAs can act as guides or scaffolds, facilitating interaction between several proteins, such as those that are part of chromatin-modifying complexes, causing gene activation or repression, depending on the interaction partners involved (79, 80). The polycomb repressive complexes PRC1 and PRC2, the transcriptional repressor element-1 silencing transcription factor REST, its cofactor (REST/CoREST), other epigenetic components like the mixed lineage leukemia protein and the H3K9 methyltransferase G9a, physically interact with lncRNAs (78, 80, 81). In addition, many lncRNAs such as HOTAIR, Xist, Kcnq1ot1, and Breaveheart interact with PRC2, implying that these ncRNAs play a role in recruiting this complex through its subunits (EZH2, SUZ12, EED, RBBP4, and AEBP2) or through a bridging protein (such as JARID2) to their target genes (82, 83). Likewise, the expression of many angiogenesis-related genes involved in the VEGF signaling pathway is regulated through lncRNAs (such as H19, MEG3, and HOTAIR), and recently, researchers discovered that some of them perform their regulatory function by influencing the expression and activity of several epigenetic modulators (20, 22). LncRNAs and their identified epigenetic targets in angiogenesis are listed in Table 2.
MANTIS is a recently discovered lncRNA required for endothelial cell function and proper angiogenesis. MANTIS is induced in the endothelium of glioblastoma tumors and is overexpressed during vascular regeneration in atherosclerosis regression. It alters angiogenic sprouting, tube formation, and epithelial cell migration. Loss of MANTIS expression is reported during pulmonary arterial hypertension, and its downregulation also led to the reduced expression of many angiogenesis-related mRNAs (80).
In endothelial cells, MANTIS is upregulated following the knockdown of the histone demethylase JARID1B. JARID1B loss triggers increased H3K4me3 levels at transcription start sites (TSS) of the MANTIS gene, facilitating gene expression. Interestingly, in patients with idiopathic pulmonary arterial hypertension, a disease characterized by endothelial dysfunction, MANTIS expression is downregulated, while JARID1B is upregulated (80).
Novel studies have revealed that MANTIS functions as a scaffold and regulates the activity of Brahma related gene-1 (BRG1), the catalytic subunit of the SWI/SNF chromatin remodeling complex. The MANTIS-BRG1 interaction allows for increased binding of BAF155, which is a core component of the SWI/SNF complex, enhancing BRG1 ATPase activity and chromatin relaxation at the TSS of the transcription factor COUP-TFII, which, in turn, recruits RNA Pol II binding and transcription of the pro-angiogenic genes SOX18 and SMAD6. The knockdown of MANTIS reduces BRG1 ATPase activity (80) (Figure 1C).
ANRIL is an antisense lncRNA from the INK4 locus. It encodes two cyclin-dependent kinase inhibitors, p15 (INK4b) and p16 (INK4a), and a protein known as ARF. All of the genes cooperate in tumor suppressor networks. When these genes are silenced, proatherosclerotic cellular mechanisms are enhanced, such as increased adhesion and diminished apoptosis (86). In fact, ANRIL expression is correlated with the risk of some vascular diseases such as coronary atherosclerosis and carotid arteriosclerosis (87).
It has been shown that ANRIL recruits PRC2 or PRC1 to different target genes by directly interacting with their subunits EZH2, SUZ12, and CBX7 (86, 88, 89). In a diabetic retinopathy cellular model, high glucose levels upregulated ANRIL and VEGF expression. In turn, ANRIL positively regulated EZH2, EED, and p300 levels. Furthermore, ANRIL recruits EZH2 and histone acetyl-transferase p300 to the VEGF promoter, enhancing its expression and angiogenic effects. It was shown that ANRIL silencing prevented the formation of capillary-like structures in spite of the angiogenic influence of high glucose levels (84) (Figure 1D). Moreover, ANRIL silencing also promoted miR-200b expression, a previously described miRNA that has been shown to be involved in regulating VEGF (90).
GATA6-AS is the hypoxia-regulated long non-coding antisense transcript of GATA6 and promotes angiogenesis by negatively regulating lysyl oxidase-like 2 (LOXL2). LOXL2 catalyzes the oxidative deamination of lysines and hydroxylysines, which results in the generation of non-methylated H3K4 and gene silencing. Thus, GATA6-AS silencing leads to increased LOXL2 activity and transcriptional repression. In the nucleus, the physical interaction between GATA6-AS and LOXL2 positively regulates the expression of several angiogenesis- and hypoxia-related genes, such as periostin and cyclooxygenase-2. It has been shown that GATA6-AS silencing in epithelial cells significantly prevented TGF-β2-induced endothelial to mesenchymal transition and augmented angiogenic sprouting in xenograft models in vivo (85).
Like epi-miRNAs, the epi-lncRNAs are excellent candidates biomarkers due to their easy collection and tissue specificity. Although there are few examples of epi-lncRNAs in angiogenesis, the implications behind these interactions provide an interesting view of the mechanisms in which lncRNAs regulate not only the recruitment but also the activity of chromatin modifiers. Another layer of complexity is added if we consider that lncRNAs have many alternative splice forms, including the non-linear, circular RNAs (circRNAs).
Circular RNAs (circRNA) are single-stranded RNAs that are widely conserved in all life domains and form a covalent closed loop (91). The discovery of this type of RNA has occurred fairly recently, and before their discovery, the RNAs were considered the result of errors within the process of gene transcription. These circRNAs are produced by a back-splicing process of pre-mRNA, in which a downstream splice donor is linked to an upstream acceptor (92, 93). The splice forms can circularize from exonic, intronic, or a combination of both regions (EIciRNAs) (94).
In cancer-derived cell lines, it has been reported that changes in DNMTs and the hypermethylation of the CpG islands of some genes that host circRNA can induce gene silencing of both linear RNA and circRNA, suggesting an epigenetic mechanism that produces two molecular “hits” (95). Because circRNA lack 5′ and 3′ ends, these cannot be degraded by exoribonucleases. Instead, circRNA levels may be regulated by endonucleases and exosomal deportation (96). These molecules are stable, abundant and specific to certain cell types, having distinct transcriptional patterns for specific tissues and multiple isoforms in eukaryotic cells (97). CircRNAs have been linked to different biological processes, including cell proliferation, senescence, and apoptosis, among others. The study of circRNA has increased in recent years, since they have been shown to be related to both physiological and pathological processes (98). In fact, circRNAs have been proposed as potential biomarkers for neurological disorders, infectious diseases, cancer, and preeclampsia as a result of their availability in circulating body fluids (99–102).
The circRNAs have transcriptional and post-transcriptional regulatory functions. EIciRNAs such as EIF3J associate with ribonucleoproteins like U1 and the Pol II at the promoters of their parental genes to enhance their own expression (94). Similar to EIciRNAs, some circRNAs (such as ciANKRD52) can positively regulate their own expression through interaction with the Pol II complex (103). Other circRNAs regulate alternative splicing or serve as sponges to bind, store, or sequester miRNAs and other protein complexes containing transcription factors and RNA binding proteins (94, 104, 105). Due to the ability of cirRNA to bind to miRNAs, they have been referred to as miRNA sponges (106). Despite their recent discovery, some evidence suggests that circRNAs are implicated in angiogenesis (e.g., circRNA-MYLK) and many cardiovascular diseases, such as atherosclerosis (e.g., circR-284), myocardial infarction (e.g., ciRS-7), and coronary artery disease (CAD) (e.g., circ_0124644), among others (107, 108). However, to our knowledge, no study has shown that circRNAs have an epigenetic regulatory role in angiogenesis. Similar to the lncRNA ANRIL, a circularized and anti-sense splice variant of the INK4/ARF locus (cANRIL) has been associated with atherosclerotic vascular disease (109). Moreover, in the cytoplasm, the binding of circANRIL to the rRNA-processing machinery impairs its function and causes nucleolar fragmentation and stress signaling (110). These findings suggest that, just like their longer-sized isoform, the variant cANRIL may have a role in the epigenetic regulation of vascular disease.
The study of ncRNA has opened up a new research field, and this has been extended to the genome scale. This type of experimental approach has become common practice in both the research laboratory and at the clinical level. Therefore, along with a growing array of genomic analysis machinery, bioinformatics platforms have also been developed, thus generating a new set of tools for the study and analysis of ncRNA.
In recent years, increasing quantities of data have been obtained from NGS technologies such as mass RNA sequencing (RNA-seq), small RNA-seq (smRNA-seq), and single-cell RNA-seq, among others. These technologies have revealed that the human genome encodes for more than 90,000 non-coding RNAs and that these play an important role in several diseases (111). Using publicly available genomic information, it is now possible to discover and characterize novel disease-associated ncRNAs. In the next section, we will describe some of the key discoveries that have been made thanks to NGS data, in which ncRNAs are shown to have roles in angiogenesis and neovascularization processes.
The study of the ncRNAs involved in molecular processes associated with neovascularization and angiogenesis in several diseases can be carried out by using RNA-seq approaches, especially where angiogenesis or neovascularization is one of the causes, risk factors, or consequences of the disorders. Some of the diseases studied in this manner have been ischemia stroke, CAD, hemangioma, and heart failure (HF). Furthermore, angiogenesis and neovascularization are strongly related to endothelial functioning and the transcriptional programming of endothelial progenitor cells (EPCs). Thus, the study of the molecular mechanisms involved in the regulation of EPCs is of great interest. Nevertheless, only a few studies have been conducted on human umbilical vein endothelial cells (HUVEC) or other endothelial models to understand the role of ncRNAs using NGS technologies. In this section, we will provide a compilation of some studies aiming to identify or characterize ncRNAs involved in vascular processes.
First, in 2012 Cheng et al. performed smRNA-seq on umbilical cord blood EPCs (UC-EPCs), which was known for its enrichment in EPCs, and compared the expression profiles against EPCs derived from peripheral blood in adults (PB-EPCs) to understand the underlying mechanisms involved the functional differences between these two models. They identified specific patterns of miRNAs (miRNome) in UC-EPCs and PB-EPCs in which 54 miRNAs were overexpressed in UC-EPC and 50 miRNAs were overexpressed in PB-EPCs. For instance, UC-EPCs expressed miRNAs involved in angiogenesis such as miR-31 and mir-18a, while PB-EPCs are enriched in tumor-suppressive miRNA expression such as that of miR-10a and mir-26a (112).
A study performed by Wang and colleagues in 2014 revealed that there was cooperation between VEGF and miRNAs in CAD progression. They performed smRNA-seq and identified EPC-specific miRNome that was related to angiogenic processes, which suggests that miRNAs in EPCs with a poor capacity to enhance angiogenesis might have higher levels of miRNAs targeting VEGF. Indeed, they identified anti-VEGF miRNAs such as miR-361-5p that were enriched in EPCs and in the plasma of patients with CAD (113).
Also, atherosclerosis appears to be one of the factors leading to CAD. In 2018, Mao and colleagues conducted a study to identify miRNAs linked with carotid atherosclerosis. They performed a differential expression analysis to identify genes that were specifically associated with either primary or advanced atherosclerotic plaque tissues. Using public databases, they predicted 23 miRNAs that targeted the differentially expressed genes, such as miR-126, miR-155, miR-19A, and miR-19B, which can play a regulating role in neovascularization and angiogenesis (114).
Furthermore, a study from Liu et al. (115) identified differentially expressed ncRNAs that were predicted to be involved in the regulation of high-density lipoprotein (HDL) metabolism, the deregulation of which is believed to be one of the main causes of CAD. To this end, they treated HUVEC cells with HDL from healthy subjects and patients with CAD and hypercholesterolemia. After RNA-seq analysis, 41 ncRNAs were identified, and researchers were able to show that the ncRNAs, along with protein-coding genes such as DGKA and UBE2V1, have critical functions in vascular cells (115).
Additionally, it is well-known that endothelial cell metabolism is sensitive to hypoxia, which is an adverse effect of atherosclerotic lesions in humans. In 2018, Moreau et al. investigated the lncRNA profiles of HUVEC cells using global run-on sequencing (GRO-Seq). GRO-seq is a sequencing method that measures active transcription, identifying newly synthetized RNA, and providing sufficient resolution to map the position and orientation of transcripts detected. This group aimed to discover changes in the expression patterns of lncRNAs in HUVEC cells exposed to hypoxia and demonstrated that hypoxia affects the transcription of ~1,800 lncRNAs. Among the most relevant lncRNAs identified were MALAT1, HYMAI, LOC730101, KIAA1656, and LOC339803, which were differentially expressed in human atherosclerotic lesions compared to normal vascular tissue (116).
In contrast, heart and circulatory system diseases often involve changes in vascular smooth muscle or cardiac cells. In 2018, Cheng et al. used RNA-seq to identify circRNAs in human aortic valves. They recognized 1,412 specific circRNAs, most of which originated from exons of their host genes. Furthermore, after performing a gene ontology enrichment analysis, they found that the host genes were associated with pathways regulating aortic valve function (ECM-receptor interaction pathway, ErbB signaling pathway, and vascular smooth muscle contraction pathway) (117). In addition, Bell et al. identified novel lncRNAs in human vascular smooth muscle cells in 2014. This work expanded our knowledge of the relevance of lncRNAs in the control of smooth muscle cells. The researchers performed an RNA-seq experiment examining expression patterns in human coronary artery smooth muscle cells. Their analysis revealed 31 novel lncRNAs. They discovered and characterized a novel vascular cell-enriched lncRNA that they named SENCR. They performed RNA-seq after knockdown of SENCR and observed that expression of Myocardin and genes involved in the contraction of smooth muscle were reduced, while expression of other promigratory genes was enhanced (118). These results have enhanced our understanding of vascular cells and should be further studied in order to discern lncRNAs in vascular diseases. Finally, in 2015, Di Salvo et al. analyzed the expression profiles of cells derived from 22 human hearts from patients with Heart Failure (HF) vs. non-HF donor hearts. Initially, they discovered 2,085 lncRNAs, and subsequent analyses revealed 48 differentially expressed lncRNAs in HF patients. Among these, AP000783.2, RP11-403B2.6, and RP11-60A24.3 were identified (119).
Angiogenesis and neovascularization processes affect the prognosis of patients who have suffered from brain stroke ischemia. Thus, the identification of ncRNAs involved in these processes might be useful for their further use as drug targets or biomarkers for the disease. Therefore, Zhang et al. (120) aimed to uncover which ncRNAs have altered expression profiles after cerebrovascular dysfunction in ischemic stroke. Using bulk RNA-seq, they profiled lncRNA signatures in primary brain microvascular endothelial cells after oxygen-glucose deficiency. This approach allowed for the identification of 362 differentially expressed lncRNAs. The top three lncRNAs that were upregulated were Snhg12, Malat1, and lnc-OGD 1006, while the top three down-regulated lncRNAs were 281008D09Rik, Peg13, and lnc-OGD 3916 (120).
Another disease model that has been studied in order to identify ncRNAs involved in angiogenesis and neovascularization is infantile hemangioma (IH), which is a type of vascular tumor in infants. Li et al. investigated whether ncRNAs have a role in IH pathogenesis in 2018. The researchers used a bulk RNA-seq approach to examine global ncRNAs expression profiles in IH patients compared to their matched, normal-skin controls. In this study, researchers identified 256 lncRNAs and 142 miRNAs that were differentially expressed. They also found more than a thousand sponge modulators involved in miRNA-, lncRNA-, and mRNA-mediated interactions. These findings suggest the presence of an endogenous ncRNA regulatory network associated with the development of IH and other vascular diseases (121).
Overall, the studies described above have shown that NGS technologies can be very effective in identifying and characterizing ncRNAs. This type of technology has helped researchers to understand the regulatory role of ncRNAs in angiogenic and neovascularization processes. However, studies in this field are just emerging, and additional research will be required to expand our knowledge and translated into clinical use.
After the development of NGS technologies, ncRNAs have been discovered, and multiple efforts have been made to organize, collect, provide, and unify all available information regarding ncRNAs so that it can be accessed by the research community. Furthermore, new methods have developed to predict and identify novel ncRNAs. Here we present some of the cutting-edge bioinformatics approaches currently being used to study ncRNAs and give some examples of how they are used in the study of neovascularization processes (Figure 2). For a detailed explanation, see the following reference (122).
Figure 2. Schematic representation of an ncRNA analysis workflow using databases. The central panel shows the available analyses provided by the databases; letters in black indicate the available analyses for miRNAs and lncRNAs; blue letters indicate analysis for miRNAs, and green letters the analyses for lncRNAs. Blue and green panels show the names of the available databases for miRNAs and lncRNAs, respectively.
Transcriptome-wide association studies can be performed to identify expression-trait associations where ncRNAs might be involved. This method can identify single-nucleotide polymorphisms (SNPs) located in transcribed regions of ncRNA genes that can be related to a specific phenotype. A second bioinformatic approach is the use of tools for the prediction of primary, secondary, and tertiary ncRNA structures to obtain information about their potential function. This method has been used for circRNAs, smRNAs, and lncRNAs. The third approach to studying ncRNAs is the use of biological networks. These types of analyses enhance our understanding of the function of ncRNAs by integrating expression, regulatory, and protein–protein interaction networks. NcRNAs are highly connected in these networks and can influence more than one target gene in order to produce a specific phenotype. These approaches can identify disease-specific regulatory modules where ncRNAs play an important role (122).
Though the effective methods described above can be used to discover and understand the biological functions of ncRNAs, they have not been adequately exploited to reveal the roles of ncRNAs in angiogenesis or neovascularization. So far, only a few studies have used advanced bioinformatics tools for this purpose. For example, in 2018, Li et al. detected novel circRNAs related with IH using RNA-seq data. The best experimental approach for the detection of circRNAs is the use of deep sequencing of RNA treated with RNase R (which leaves a circRNA-enriched sample). The availability of tools to predict novel circRNAs from RNA-seq data is of great value, given that RNA-seq data are much more highly available (122). Thus, Li et al. used circRNAFinder, a tool able to predict circRNAs from bulk RNA-seq experiments, and identified 249 circRNA candidates differentially expressed between IH and matched normal skin samples. The circRNAs hsa_circRNA001885 and hsa_circRNA006612 where further investigated by this group, providing novel insights about the disease (123).
As shown previously, the development of tools used to predict and identify novel ncRNAs is invaluable. The increasing number of RNA-seq experiments and access to databases will increasingly facilitate the discovery of novel ncRNAs, and the characterization of ncRNAs will become increasingly straightforward. For instance, ANGIOGENES is a database that has been created to store information related to angiogenic processes. It depicts experimental data obtained from RNA-seq experiments in endothelial cells. This allows for the in-silico detection of genes expressed in several endothelial cell types from different tissues. ANGIOGENES uses publicly-available RNA-seq experiments and identifies endothelial cell-specific ncRNAs in human, mouse, and zebrafish. The database facilitates further analyses using GO enrichment terms and is available online (124). In addition to ANGIOGENES, EndoDB is another database that retrieves information about endothelial cells from different platforms for several species (125). Other databases are available for the study of ncRNAs; nevertheless, these are not specialized in angiogenesis or neovascular processes. Databases and tools used for the study of ncRNAs are listed in Table 3.
We know that endothelial cells are heterogeneous; for instance, they function differently depending on vessel type (162). To uncover the molecular mechanisms controlling this heterogeneity, single-cell RNA sequencing analyses (scRNA-seq) have the potential to enhance our understanding of vascular biology. ScRNA-seq is currently being used to study and assess cellular heterogeneity. Particularly with respect to cancer research, this approach has proved to be valuable (163–165); nevertheless, its use in vascular research is just beginning. Recently published studies have mostly focused on protein-coding genes (166, 167). The participation of ncRNAs, along with epigenetic factors, in regulating the metabolic activities of endothelial cells from a single-cell perspective in vascular development and diseases is not yet clear.
ncRNAs comprise a new frontier in genetic regulation that has impacts on several research areas. Undoubtedly, the study of angiogenesis and neovascularization has been enhanced through the integration of the study of ncRNAs and epigenetics. Further, ncRNAs are involved in the regulation of several angiogenic targets through epigenetic mechanisms. On the basis of this relationship, a new field of opportunity has emerged in which biomarkers and specific therapies may be identified that can improve the treatment of different vascular diseases and cancers. NGS platforms allow for the global analysis of ncRNA expression and can be used to compare different physiological and pathological processes. Most of the pathways and mechanisms controlling the ncRNA-mediated regulation of angiogenesis remain unexplored. It is likely that new research strategies implementing an epigenetic perspective will facilitate future discoveries.
IH-R, LG-C, MS-A, TM-H, and ES-R wrote the manuscript. MS-A and IH-R did the artwork. TM-H and LG-C combined their information to make the tables. All authors contributed to manuscript revision and read and approved the submitted version.
This work was supported by the Consejo Nacional de Ciencia y Tecnología (CONACyT) through the Fondo Sectorial de Investigación en Salud y Seguridad Social (FOSISS, Grant No. 0261181), Fondo CB-SEP-CONACyT (284748), and UAM-PTC-704. ES-R was supported by the Natural Science Department at UAM Cuajimalpa Unit.
The authors declare that the research was conducted in the absence of any commercial or financial relationships that could be construed as a potential conflict of interest.
IH-R and TM-H are masters students and LG-C and MS-A are doctoral students from Programa de Maestría y Doctorado en Ciencias Bioquímicas, UNAM and received a fellowship from CONACyT (IH-R: CVU 886138, TM-H: CVU 924685, LG-C: CVU 588391, and MS-A: CVU 659273). MS-A was also a beneficiary of the German Academic Exchange Service (DAAD Grant No. 91693321).
BDNF, Brain-Derived Neurotrophic Factor; BRG1, Brahma related gene-1; CAD, Coronary Artery Disease; circRNA, circular RNAs; DEGs, Differentially Expressed Genes; DNMT, DNA methyltransferase; EIciRNAs, Exonintron circular RNAs; EPCs, Endothelial Progenitor Cells; EZH2, Enhancer of Zeste Homolog 2; GRO-Seq, Global run-on sequencing; HDAC, Histone deacetylase; HDL, High-density lipoprotein; HF, Heart Failure; HIF1, Hypoxia Inducible Factors 1; HUVEC, Human Umbilical Vein Endothelial Cells; IH, Infantile hemangioma; lincRNAs, intergenic lncRNAs; lncRNAs, long non-coding RNAs; LOXL2, Lysyl oxidase-like 2; MeCP2, Methyl-CpG-binding protein 2; miRNAs, microRNAs; MMP, Matrix metalloproteinase; mRNA, messenger RNA; ncRNAs, non-coding RNAs; NGS, Next-Generation Sequencing; PBEPCs, Peripheral Blood EPCs; PRC, Polycomb Repressive Complex; pre-miRNA, precursor hairpin miRNA; REST, Repressor Element-1 Silencing Transcription; RNA-seq, RNA sequencing; SIRT1, NAD-dependent deacetylase sirtuin1; smRNA-seq, small RNA-seq; SNPs, Single Nucleotide Polymorphisms; SUZ12, Suppressor of Zeste 12 Protein Homolog 2; TF, Transcription Factor; TGF-β, Transforming Growth Factor; TSS, Transcription Start Sites; UC-EPCs, Umbilical Cord EPCs; UHRF1, E3 ubiquitin ligase with PHD and RING finger domain 1; VASH1, Angiogenesis inhibitor vasohibin 1; VEGF, Vascular Endothelial Growth Factor.
1. Carmeliet P, Jain RK. Molecular mechanisms and clinical applications of angiogenesis. Nature. (2011) 473:298–307. doi: 10.1038/nature10144
2. Song X, Shan D, Chen J, Jing Q. miRNAs and lncRNAs in vascular injury and remodeling. Sci China Life Sci. (2014) 57:826–35. doi: 10.1007/s11427-014-4698-y
3. Helkin A, Maier KG, Gahtan V. Thrombospondin-1,−2 and−5 have differential effects on vascular smooth muscle cell physiology. Biochem Biophys Res Commun. (2015) 464:1022–7. doi: 10.1016/j.bbrc.2015.07.044
4. Xie H, Cui Z, Wang L, Xia Z, Hu Y, Xian L, et al. PDGF-BB secreted by preosteoclasts induces angiogenesis during coupling with osteogenesis. Nat Med. (2014) 20:1270–8. doi: 10.1038/nm.3668
5. Xu X, Zong Y, Gao Y, Sun X, Zhao H, Luo W, Jia S. VEGF induce vasculogenic mimicry of choroidal melanoma through the PI3k Signal Pathway. Biomed Res Int. (2019) 2019:3909102. doi: 10.1155/2019/3909102
6. Carmeliet P, Jain RK. Angiogenesis in cancer and other diseases. Nature. (2000) 407:249–57. doi: 10.1038/35025220
7. Tímár J, Döme B, Fazekas K, Janovics A, Paku S. Angiogenesis-dependent diseases and angiogenesis therapy. Pathol Oncol Res. (2001) 7:85–94. doi: 10.1007/BF03032573
8. Bieniasz-Krzywiec P, Martín-Pérez R, Ehling M, García-Caballero M, Pinioti S, Pretto S, et al. Podoplanin-expressing macrophages promote lymphangiogenesis and lymphoinvasion in breast cancer. Cell Metab. (2019) 30:1–20. doi: 10.1016/j.cmet.2019.07.015
9. Nowak-Sliwinska P, Alitalo K, Allen E, Anisimov A, Aplin AC, Auerbach R, et al. Consensus guidelines for the use and interpretation of angiogenesis assays. Angiogenesis. (2018) 21:425–532. doi: 10.1007/s10456-018-9613-x
10. Celletti FL, Waugh JM, Amabile PG, Brendolan A, Hilfiker PR, Dake MD. Vascular endothelial growth factor enhances atherosclerotic plaque progression. Nat Med. (2001) 7:425–9. doi: 10.1038/86490
11. Li M, Liu C, Bin J, Wang Y, Chen J, Xiu J, et al. Mutant hypoxia inducible factor-1α improves angiogenesis and tissue perfusion in ischemic rabbit skeletal muscle. Microvasc Res. (2011) 81:26–33. doi: 10.1016/j.mvr.2010.09.008
12. Xue K, Zhao X, Zhang Z, Qiu B, Tan QSW, Ong KH, et al. Sustained delivery of anti-VEGFs from thermogel depots inhibits angiogenesis without the need for multiple injections. Biomater Sci. (2019). doi: 10.1039/C9BM01049A. [Epub ahead of print].
13. Nicoglou A. Waddington's epigenetics or the pictorial meetings of development and genetics. Hist Philos Life Sci. (2018) 40:61. doi: 10.1007/s40656-018-0228-8
14. Felsenfeld G. A brief history of epigenetics. Cold Spring Harb Perspect Biol. (2014) 6:a018200. doi: 10.1101/cshperspect.a018200
15. Richard JLC, Eichhorn PJA. Deciphering the roles of lncRNAs in breast development and disease. Oncotarget. (2018) 9:20179–212. doi: 10.18632/oncotarget.24591
16. Felsenfeld G, Groudine M. Controlling the double helix. Nature. (2003) 421:448–53. doi: 10.1038/nature01411
17. Jenuwein T, Allis CD. Translating the histone code. Science. (2001) 293:1074–80. doi: 10.1126/science.1063127
18. Biswas S, Rao CM. Epigenetic tools. (The Writers, The Readers and The Erasers) and their implications in cancer therapy. Eur J Pharmacol. (2018) 837:8–24. doi: 10.1016/j.ejphar.2018.08.021
19. Iliopoulos D, Lindahl-Allen M, Polytarchou C, Hirsch HA, Tsichlis PN, Struhl K. Loss of miR-200 inhibition of Suz12 leads to polycomb-mediated repression required for the formation and maintenance of cancer stem cells. Mol Cell. (2010) 39:761–72. doi: 10.1016/j.molcel.2010.08.013
20. Song Y, Wang R, Li L-W, Liu X, Wang Y-F, Wang Q-X, et al. Long non-coding RNA HOTAIR mediates the switching of histone H3 lysine 27 acetylation to methylation to promote epithelial-to-mesenchymal transition in gastric cancer. Int J Oncol. (2019) 54:77–86. doi: 10.3892/ijo.2018.4625
21. Tang W, Zhou W, Xiang L, Wu X, Zhang P, Wang J, et al. The p300/YY1/miR-500a-5p/HDAC2 signalling axis regulates cell proliferation in human colorectal cancer. Nat Commun. (2019) 10:663. doi: 10.1038/s41467-018-08225-3
22. Wang F, Tang Z, Shao H, Guo J, Tan T, Dong Y, et al. Long noncoding RNA HOTTIP cooperates with CCCTC-binding factor to coordinate HOXA gene expression. Biochem Biophys Res Commun. (2018) 500:852–9. doi: 10.1016/j.bbrc.2018.04.173
23. Bartel DP. MicroRNAs: genomics, biogenesis, mechanism, and function. Cell. (2004) 116:281–97. doi: 10.1016/S0092-8674(04)00045-5
24. Esteller M. Non-coding RNAs in human disease. Nat Rev Genet. (2011) 12:861–74. doi: 10.1038/nrg3074
25. Fromm B, Billipp T, Peck LE, Johansen M, Tarver JE, King BL, et al. A uniform system for the annotation of vertebrate microRNA Genes and the Evolution of the Human microRNAome. Annu Rev Genet. (2015) 49:213–42. doi: 10.1146/annurev-genet-120213-092023
26. Krol J, Loedige I, Filipowicz W. The widespread regulation of microRNA biogenesis, function and decay. Nat Rev Genet. (2010) 11:597–610. doi: 10.1038/nrg2843
27. Lund E, Dahlberg JE. Substrate selectivity of exportin 5 and Dicer in the biogenesis of microRNAs. Cold Spring Harb Symp Quant Biol. (2006) 71:59–66. doi: 10.1101/sqb.2006.71.050
28. Catalanotto C, Cogoni C, Zardo G. MicroRNA in control of gene expression: an overview of nuclear functions. Int J Mol Sci. (2016) 17:1712. doi: 10.3390/ijms17101712
29. Bartel DP. MicroRNAs: target recognition and regulatory functions. Cell. (2009) 136:215–33. doi: 10.1016/j.cell.2009.01.002
30. Wang X-J, Reyes JL, Chua N-H, Gaasterland T. Prediction and identification of Arabidopsis thaliana microRNAs and their mRNA targets. Genome Biol. (2004) 5:R65. doi: 10.1186/gb-2004-5-9-r65
31. Fabian MR, Sonenberg N, Filipowicz W. Regulation of mRNA translation and stability by microRNAs. Annu Rev Biochem. (2010) 79:351–79. doi: 10.1146/annurev-biochem-060308-103103
32. Chen H-X, Xu X-X, Tan B-Z, Zhang Z, Zhou X-D. MicroRNA-29b inhibits angiogenesis by targeting VEGFA through the MAPK/ERK and PI3K/Akt signaling pathways in endometrial carcinoma. Cell Physiol Biochem. (2017) 41:933–46. doi: 10.1159/000460510
33. Tzeng H-E, Chang A-C, Tsai C-H, Wang S-W, Tang C-H. Basic fibroblast growth factor promotes VEGF-C-dependent lymphangiogenesis via inhibition of miR-381 in human chondrosarcoma cells. Oncotarget. (2016) 7:38566–78. doi: 10.18632/oncotarget.9570
34. Hua Z, Lv Q, Ye W, Wong C-KA, Cai G, Gu D, et al. MiRNA-directed regulation of VEGF and other angiogenic factors under hypoxia. PLoS ONE. (2006) 1:e116. doi: 10.1371/journal.pone.0000116
35. Soto-Reyes E, González-Barrios R, Cisneros-Soberanis F, Herrera-Goepfert R, Pérez V, Cantú D, et al. Disruption of CTCF at the miR-125b1 locus in gynecological cancers. BMC Cancer. (2012) 12:40. doi: 10.1186/1471-2407-12-40
36. Lujambio A, Ropero S, Ballestar E, Fraga MF, Cerrato C, Setién F, et al. Genetic unmasking of an epigenetically silenced microRNA in human cancer cells. Cancer Res. (2007) 67:1424–29. doi: 10.1158/0008-5472.CAN-06-4218
37. Amodio N, Rossi M, Raimondi L, Pitari MR, Botta C, Tagliaferri P, et al. miR-29s: a family of epi-miRNAs with therapeutic implications in hematologic malignancies. Oncotarget. (2015) 6:12837–61. doi: 10.18632/oncotarget.3805
38. Chen Z, Wang X, Liu R, Chen L, Yi J, Qi B, et al. KDM4B-mediated epigenetic silencing of miRNA-615-5p augments RAB24 to facilitate malignancy of hepatoma cells. Oncotarget. (2016) 8:17712–25. doi: 10.18632/oncotarget.10832
39. Garzon R, Liu S, Fabbri M, Liu Z, Heaphy CEA, Callegari E, et al. MicroRNA-29b induces global DNA hypomethylation and tumor suppressor gene reexpression in acute myeloid leukemia by targeting directly DNMT3A and 3B and indirectly DNMT1. Blood. (2009) 113:6411–8. doi: 10.1182/blood-2008-07-170589
40. Malumbres M. miRNAs and cancer: an epigenetics view. Mol Aspects Med. (2013) 34:863–74. doi: 10.1016/j.mam.2012.06.005
41. Tuddenham L, Wheeler G, Ntounia-Fousara S, Waters J, Hajihosseini MK, Clark I, et al. The cartilage specific microRNA-140 targets histone deacetylase 4 in mouse cells. FEBS Lett. (2006) 580:4214–7. doi: 10.1016/j.febslet.2006.06.080
42. Varambally S, Cao Q, Mani R-S, Shankar S, Wang X, Ateeq B, et al. Genomic Loss of microRNA-101 leads to overexpression of histone methyltransferase EZH2 in cancer. Science. (2008) 322:1695–9. doi: 10.1126/science.1165395
43. Verrando P, Capovilla M, Rahmani R. Trans-nonachlor decreases miR-141-3p levels in human melanocytes in vitro promoting melanoma cell characteristics and shows a multigenerational impact on miR-8 levels in Drosophila. Toxicology. (2016) 368–369:129–41. doi: 10.1016/j.tox.2016.09.003
44. Wang X, Wu Q, Xu B, Wang P, Fan W, Cai Y, et al. MiR-124 exerts tumor suppressive functions on the cell proliferation, motility and angiogenesis of bladder cancer by fine-tuning UHRF1. FEBS J. (2015) 282:4376–88. doi: 10.1111/febs.13502
45. Volkmann I, Regalla K, Nils P, Jan F, Seema D, Angelika H, et al. MicroRNA-mediated epigenetic silencing of sirtuin1 contributes to impaired angiogenic responses. Circulation Res. (2013) 113:997–1003. doi: 10.1161/CIRCRESAHA.113.301702
46. Smits M, Mir SE, Nilsson RJA, van der Stoop PM, Niers JM, Marquez VE, et al. Down-regulation of miR-101 in endothelial cells promotes blood vessel formation through reduced repression of EZH2. PLoS ONE. (2011) 6:e16282. doi: 10.1371/journal.pone.0016282
47. Smits M, Nilsson J, Mir SE, van der Stoop PM, Hulleman E, Niers JM, et al. miR-101 is down-regulated in glioblastoma resulting in EZH2-induced proliferation, migration, and angiogenesis. Oncotarget. (2010) 1:710–20. doi: 10.18632/oncotarget.205
48. Shehadeh LA, Sharma S, Pessanha M, Wei JQ, Liu J, Yuan H, et al. MicroRNA-20a constrains p300-driven myocardial angiogenic transcription by direct targeting of p300. PLoS ONE. (2013) 8:e79133. doi: 10.1371/journal.pone.0079133
49. Sun J, Zheng G, Gu Z, Guo Z. MiR-137 inhibits proliferation and angiogenesis of human glioblastoma cells by targeting EZH2. J Neurooncol. (2015) 122:481–9. doi: 10.1007/s11060-015-1753-x
50. Descamps B, Saif J, Benest AV, Biglino G, Bates DO, Chamorro-Jorganes A, et al. BDNF. (Brain-Derived Neurotrophic Factor) promotes embryonic stem cells differentiation to endothelial cells via a molecular pathway, including microRNA-214, EZH2. (Enhancer of Zeste Homolog 2), and eNOS. (Endothelial Nitric Oxide Synthase). Arterioscler Thromb Vasc Biol. (2018) 38:2117–25. doi: 10.1161/ATVBAHA.118.311400
51. McArthur K, Feng B, Wu Y, Chen S, Chakrabarti S. MicroRNA-200b regulates vascular endothelial growth factor-mediated alterations in diabetic retinopathy. Diabetes. (2011) 60:1314–23. doi: 10.2337/db10-1557
52. Potente M, Ghaeni L, Baldessari D, Mostoslavsky R, Rossig L, Dequiedt F, Haendeler J, et al. SIRT1 controls endothelial angiogenic functions during vascular growth. Genes Dev. (2007) 21:2644–58. doi: 10.1101/gad.435107
53. Wang C, Wang F, Li Z, Cao Q, Huang L, Chen S. MeCP2-mediated epigenetic regulation in senescent endothelial progenitor cells. Stem Cell Res Ther. (2018) 9:87. doi: 10.1186/s13287-018-0828-y
54. Jin Q, He W, Chen L, Yang Y, Shi K, You Z. MicroRNA-101-3p inhibits proliferation in retinoblastoma cells by targeting EZH2 and HDAC9. Exp Ther Med. (2018) 16:1663–70. doi: 10.3892/etm.2018.6405
55. Wei X, Xiang T, Ren G, Tan C, Liu R, Xu X, et al. miR-101 is down-regulated by the hepatitis B virus x protein and induces aberrant DNA methylation by targeting DNA methyltransferase 3A. Cell Signal. (2013) 25:439–46. doi: 10.1016/j.cellsig.2012.10.013
56. Huang W, Ren Y, Liu H. Vasohibin 1 inhibits Adriamycin resistance in osteosarcoma cells via the protein kinase B signaling pathway. Oncol Lett. (2018) 15:5983–8. doi: 10.3892/ol.2018.8074
57. Lu C, Han HD, Mangala LS, Ali-Fehmi R, Newton CS, Ozbun L, et al. Regulation of tumor angiogenesis by EZH2. Cancer Cell. (2010) 18:185–97. doi: 10.1016/j.ccr.2010.06.016
58. Luengo-Gil G, Gonzalez-Billalabeitia E, Perez-Henarejos SA, Navarro Manzano E, Chaves-Benito A, Garcia-Martinez E, et al. Angiogenic role of miR-20a in breast cancer. PLoS ONE. (2018) 13:e0194638. doi: 10.1371/journal.pone.0194638
59. Zhang W, Chen J-H, Shan T, Aguilera-Barrantes I, Wang L-S, Huang TH-M, et al. miR-137 is a tumor suppressor in endometrial cancer and is repressed by DNA hypermethylation. Lab Invest. (2018) 98:1397–407. doi: 10.1038/s41374-018-0092-x
60. Ding F, Zhang S, Gao S, Shang J, Li Y, Cui N, et al. MiR-137 functions as a tumor suppressor in pancreatic cancer by targeting MRGBP. J Cell Biochem. (2018) 119:4799–807. doi: 10.1002/jcb.26676
61. Ben Gacem R, Ben Abdelkrim O, Ziadi S, Ben Dhiab M, Trimeche M. Methylation of miR-124a-1, miR-124a-2, and miR-124a-3 genes correlates with aggressive and advanced breast cancer disease. Tumour Biol. (2014) 35:4047–56. doi: 10.1007/s13277-013-1530-4
62. Li X, Yang Z, Han Z, Wen Y, Ma Z, Wang Y. Niclosamide acts as a new inhibitor of vasculogenic mimicry in oral cancer through upregulation of miR-124 and downregulation of STAT3. Oncol Rep. (2018) 39:827–33. doi: 10.3892/or.2017.6146
63. Gao S-P, Sun H-F, Li L-D, Fu W-Y, Jin W. UHRF1 promotes breast cancer progression by suppressing KLF17 expression by hypermethylating its promoter. Am J Cancer Res. (2017) 7:1554–65. doi: 10.1158/1538-7445.SABCS16-P1-08-11
64. Stevens HC, Deng L, Grant JS, Pinel K, Thomas M, Morrell NW, et al. Regulation and function of miR-214 in pulmonary arterial hypertension. Pulm Circ. (2016) 6:109–17. doi: 10.1086/685079
65. Namba T, Koike H, Murakami K, Aoki M, Makino H, Hashiya N, et al. Angiogenesis induced by endothelial nitric oxide synthase gene through vascular endothelial growth factor expression in a rat hindlimb ischemia model. Circulation. (2003) 108:2250–7. doi: 10.1161/01.CIR.0000093190.53478.78
66. Sinha M, Ghatak S, Roy S, Sen CK. microRNA−200b as a switch for inducible adult angiogenesis. Antioxid Redox Signal. (2015) 22:1257–72. doi: 10.1089/ars.2014.6065
67. Adam L, Zhong M, Choi W, Qi W, Nicoloso M, Arora A, et al. miR-200 expression regulates epithelial-to-mesenchymal transition in bladder cancer cells and reverses resistance to epidermal growth factor receptor therapy. Clin Cancer Res. (2009) 15:5060–72. doi: 10.1158/1078-0432.CCR-08-2245
68. Li Y, VandenBoom TG, Kong D, Wang Z, Ali S, Philip PA, et al. Up-regulation of miR-200 and let-7 by natural agents leads to the reversal of epithelial-to-mesenchymal transition in gemcitabine-resistant pancreatic cancer cells. Cancer Res. (2009) 69:6704–12. doi: 10.1158/0008-5472.CAN-09-1298
69. Mees ST, Mardin WA, Wendel C, Baeumer N, Willscher E, Senninger N, et al. EP300–a miRNA-regulated metastasis suppressor gene in ductal adenocarcinomas of the pancreas. Int J Cancer. (2010) 126:114–24. doi: 10.1002/ijc.24695
70. Feng B, Cao Y, Chen S, Chu X, Chu Y, Chakrabarti S. miR-200b mediates endothelial-to-mesenchymal transition in diabetic cardiomyopathy. Diabetes. (2016) 65:768–79. doi: 10.2337/db15-1033
71. Arany Z, Huang LE, Eckner R, Bhattacharya S, Jiang C, Goldberg MA, et al. An essential role for p300/CBP in the cellular response to hypoxia. Proc Natl Acad Sci USA. (1996) 93:12969–73. doi: 10.1073/pnas.93.23.12969
72. Detmar M, Brown LF, Berse B, Jackman RW, Elicker BM, Dvorak HF, et al. Hypoxia regulates the expression of vascular permeability factor/vascular endothelial growth factor. (VPF/VEGF) and its receptors in human skin. J Invest Dermatol. (1997) 108:263–8. doi: 10.1111/1523-1747.ep12286453
73. Liu Y, Cox SR, Morita T, Kourembanas S. Hypoxia regulates vascular endothelial growth factor gene expression in endothelial cells. Identification of a 5' enhancer. Circ Res. (1995) 77:638–43. doi: 10.1161/01.RES.77.3.638
74. Al-Mulla F. Vesicular control of metastasis: entrap the “virtuous” and free the “wicked” microRNA. Transl Cancer Res. (2017) 6:S999-S1002–S1002. doi: 10.21037/tcr.2017.06.49
75. Teng Y, Ren Y, Hu X, Mu J, Samykutty A, Zhuang X, et al. MVP-mediated exosomal sorting of miR-193a promotes colon cancer progression. Nat Commun. (2017) 8:14448. doi: 10.1038/ncomms14448
76. Derrien T, Johnson R, Bussotti G, Tanzer A, Djebali S, Tilgner H, et al. The GENCODE v7 catalog of human long noncoding RNAs: Analysis of their gene structure, evolution, and expression. Genome Res. (2012) 22:1775–89. doi: 10.1101/gr.132159.111
77. Sun BK, Deaton AM, Lee JT. A Transient heterochromatic state in Xist preempts X inactivation choice without RNA stabilization. Mol Cell. (2006) 21:617–28. doi: 10.1016/j.molcel.2006.01.028
78. Mohammad F, Mondal T, Guseva N, Pandey GK, Kanduri C. Kcnq1ot1 noncoding RNA mediates transcriptional gene silencing by interacting with Dnmt1. Development. (2010) 137:2493–9. doi: 10.1242/dev.048181
79. Ding X, Jia X, Wang C, Xu J, Gao S-J, Lu C. A DHX9-lncRNA-MDM2 interaction regulates cell invasion and angiogenesis of cervical cancer. Cell Death Differ. (2019) 26:1750–65. doi: 10.1038/s41418-018-0242-0
80. Leisegang MS, Fork C, Josipovic I, Richter FM, Preussner J, Hu J, et al. Long Noncoding RNA MANTIS facilitates endothelial angiogenic function. Circulation. (2017) 136:65–79. doi: 10.1161/CIRCULATIONAHA.116.026991
81. Miao H, Wang L, Zhan H, Dai J, Chang Y, Wu F, et al. A long noncoding RNA distributed in both nucleus and cytoplasm operates in the PYCARD-regulated apoptosis by coordinating the epigenetic and translational regulation. PLoS Genet. (2019) 15:e1008144. doi: 10.1371/journal.pgen.1008144
82. Davidovich C, Cech TR. The recruitment of chromatin modifiers by long noncoding RNAs: lessons from PRC2. RNA. (2015) 21:2007–22. doi: 10.1261/rna.053918.115
83. Brockdorff N. Noncoding RNA and polycomb recruitment. RNA. (2013) 19:429–42. doi: 10.1261/rna.037598.112
84. Thomas AA, Feng B, Chakrabarti S. ANRIL: a regulator of VEGF in diabetic retinopathy. Invest Ophthalmol Vis Sci. (2017) 58:470–80. doi: 10.1167/iovs.16-20569
85. Neumann P, Jaé N, Knau A, Glaser SF, Fouani Y, Rossbach O, et al. The lncRNA GATA6-AS epigenetically regulates endothelial gene expression via interaction with LOXL2. Nat Commun. (2018) 9:237. doi: 10.1038/s41467-017-02431-1
86. Nie F, Sun M, Yang J, Xie M, Xu T, Xia R, et al. Long noncoding RNA ANRIL promotes non-small cell lung cancer cell proliferation and inhibits apoptosis by silencing KLF2 and P21 expression. Mol Cancer Ther. (2015) 14:268–77. doi: 10.1158/1535-7163.MCT-14-0492
87. Congrains A, Kamide K, Oguro R, Yasuda O, Miyata K, Yamamoto E, et al. Genetic variants at the 9p21 locus contribute to atherosclerosis through modulation of ANRIL and CDKN2A/B. Atherosclerosis. (2012) 220:449–55. doi: 10.1016/j.atherosclerosis.2011.11.017
88. Kotake Y, Nakagawa T, Kitagawa K, Suzuki S, Liu N, Kitagawa M, et al. Long non-coding RNA ANRIL is required for the PRC2 recruitment to and silencing of p15INK4B tumor suppressor gene. Oncogene. (2011) 30:1956–62. doi: 10.1038/onc.2010.568
89. Yap KL, Li S, Muñoz-Cabello AM, Raguz S, Zeng L, Mujtaba S, et al. Molecular interplay of the noncoding RNA ANRIL and methylated histone H3 lysine 27 by polycomb CBX7 in transcriptional silencing of INK4a. Mol Cell. (2010) 38:662–74. doi: 10.1016/j.molcel.2010.03.021
90. Ruiz MA, Feng B, Chakrabarti S. Polycomb repressive complex 2 regulates MiR-200b in retinal endothelial cells: potential relevance in diabetic retinopathy. PLoS ONE. (2015) 10:e0123987. doi: 10.1371/journal.pone.0123987
91. Danan M, Schwartz S, Edelheit S, Sorek R. Transcriptome-wide discovery of circular RNAs in Archaea. Nucleic Acids Res. (2012) 40:3131–42. doi: 10.1093/nar/gkr1009
92. Barrett SP, Salzman J. Circular RNAs: analysis, expression and potential functions. Development. (2016) 143:1838–47. doi: 10.1242/dev.128074
93. Lei K, Bai H, Wei Z, Xie C, Wang J, Li J, Chen Q. The mechanism and function of circular RNAs in human diseases. Exp Cell Res. (2018) 368:147–58. doi: 10.1016/j.yexcr.2018.05.002
94. Li Z, Huang C, Bao C, Chen L, Lin M, Wang X, et al. Exon-intron circular RNAs regulate transcription in the nucleus. Nat Struct Mol Biol. (2015) 22:256–64. doi: 10.1038/nsmb.2959
95. Ferreira HJ, Davalos V, de Moura MC, Soler M, Perez-Salvia M, Bueno-Costa A, et al. Circular RNA CpG island hypermethylation-associated silencing in human cancer. Oncotarget. (2018) 9:29208–19. doi: 10.18632/oncotarget.25673
96. Dou Y, Cha DJ, Franklin JL, Higginbotham JN, Jeppesen DK, Weaver AM, et al. Circular RNAs are down-regulated in KRAS mutant colon cancer cells and can be transferred to exosomes. Sci Rep. (2016) 6:37982. doi: 10.1038/srep37982
97. Li X, Yang L, Chen L-L. The biogenesis, functions, and challenges of circular RNAs. Mol Cell. (2018) 71:428–42. doi: 10.1016/j.molcel.2018.06.034
98. Dang R-Y, Liu F-L, Li Y. Circular RNA hsa_circ_0010729 regulates vascular endothelial cell proliferation and apoptosis by targeting the miR-186/HIF-1α axis. Biochem Biophys Res Commun. (2017) 490:104–110. doi: 10.1016/j.bbrc.2017.05.164
99. Bai Y, Rao H, Chen W, Luo X, Tong C, Qi H. Profiles of circular RNAs in human placenta and their potential roles related to preeclampsia. Biol Reprod. (2018) 98:705–12. doi: 10.1093/biolre/ioy034
100. Lü L, Sun J, Shi P, Kong W, Xu K, He B, et al. Identification of circular RNAs as a promising new class of diagnostic biomarkers for human breast cancer. Oncotarget. (2017) 8:44096–107. doi: 10.18632/oncotarget.17307
101. Qian Z, Liu H, Li M, Shi J, Li N, Zhang Y, et al. Potential diagnostic power of blood circular RNA expression in active pulmonary tuberculosis. EBioMed. (2018) 27:18–26. doi: 10.1016/j.ebiom.2017.12.007
102. Yang P, Qiu Z, Jiang Y, Dong L, Yang W, Gu C, et al. Silencing of cZNF292 circular RNA suppresses human glioma tube formation via the Wnt/β-catenin signaling pathway. Oncotarget. (2016) 7:63449–55. doi: 10.18632/oncotarget.11523
103. Zhang Y, Zhang X-O, Chen T, Xiang J-F, Yin Q-F, Xing Y-H, et al. Circular intronic long noncoding RNAs. Mol Cell. (2013) 51:792–806. doi: 10.1016/j.molcel.2013.08.017
104. Ashwal-Fluss R, Meyer M, Pamudurti NR, Ivanov A, Bartok O, Hanan M, et al. circRNA biogenesis competes with pre-mRNA splicing. Mol Cell. (2014) 56:55–66. doi: 10.1016/j.molcel.2014.08.019
105. Schneider T, Hung L-H, Schreiner S, Starke S, Eckhof H, Rossbach O, et al. CircRNA-protein complexes: IMP3 protein component defines subfamily of circRNPs. Sci Rep. (2016) 6:31313. doi: 10.1038/srep31313
106. Memczak S, Jens M, Elefsinioti A, Torti F, Krueger J, Rybak A, et al. Circular RNAs are a large class of animal RNAs with regulatory potency. Nature. (2013) 495:333–8. doi: 10.1038/nature11928
107. He R, Liu P, Xie X, Zhou Y, Liao Q, Xiong W, et al. circGFRA1 and GFRA1 act as ceRNAs in triple negative breast cancer by regulating miR-34a. J Exp Clin Cancer Res. (2017) 36:145. doi: 10.1186/s13046-017-0614-1
108. Zhong Z, Huang M, Lv M, He Y, Duan C, Zhang L, et al. Circular RNA MYLK as a competing endogenous RNA promotes bladder cancer progression through modulating VEGFA/VEGFR2 signaling pathway. Cancer Lett. (2017) 403:305–17. doi: 10.1016/j.canlet.2017.06.027
109. Burd CE, Jeck WR, Liu Y, Sanoff HK, Wang Z, Sharpless NE. Expression of linear and novel circular forms of an INK4/ARF-associated non-coding RNA correlates with atherosclerosis risk. PLoS Genet. (2010) 6:e1001233. doi: 10.1371/journal.pgen.1001233
110. Holdt LM, Stahringer A, Sass K, Pichler G, Kulak NA, Wilfert W, et al. Circular non-coding RNA ANRIL modulates ribosomal RNA maturation and atherosclerosis in humans. Nat Commun. (2016) 7:12429. doi: 10.1038/ncomms12429
111. Fang S, Zhang L, Guo J, Niu Y, Wu Y, Li H, et al. NONCODEV5: a comprehensive annotation database for long non-coding RNAs. Nucleic Acids Res. (2018) 46:D308–14. doi: 10.1093/nar/gkx1107
112. Cheng C-C, Lo H-H, Huang T-S, Cheng Y-C, Chang S-T, Chang S-J, et al. Genetic module and miRNome trait analyses reflect the distinct biological features of endothelial progenitor cells from different anatomic locations. BMC Genom. (2012) 13:447. doi: 10.1186/1471-2164-13-447
113. Wang H-W, Lo H-H, Chiu Y-L, Chang S-J, Huang P-H, Liao K-H, et al. Dysregulated miR-361-5p/VEGF axis in the plasma and endothelial progenitor cells of patients with coronary artery disease. PLOS ONE. (2014) 9:e98070. doi: 10.1371/journal.pone.0098070
114. Mao Z, Wu F, Shan Y. Identification of key genes and miRNAs associated with carotid atherosclerosis based on mRNA-seq data. Medicine. (2018) 97:e9832. doi: 10.1097/MD.0000000000009832
115. Liu X, Wang TT, Li Y, Shi MM, Li HM, Yuan HX, et al. High density lipoprotein from coronary artery disease patients caused abnormal expression of long non-coding RNAs in vascular endothelial cells. Biochem Biophys Res Commun. (2017) 487:552–9. doi: 10.1016/j.bbrc.2017.04.082
116. Moreau PR, Örd T, Downes NL, Niskanen H, Bouvy-Liivrand M, Aavik E, et al. Transcriptional profiling of hypoxia-regulated non-coding RNAs in human primary endothelial cells. Front Cardiovasc Med. (2018) 5:159. doi: 10.3389/fcvm.2018.00159
117. Chen J, Wang J, Jiang Y, Gu W, Ni B, Sun H, et al. Identification of circular RNAs in human aortic valves. Gene. (2018) 642:135–44. doi: 10.1016/j.gene.2017.10.016
118. Bell RD, Long X, Lin M, Bergmann JH, Nanda V, Cowan SL, et al. Identification and initial functional characterization of a human vascular cell-enriched long noncoding RNA. Arterioscler Thromb Vasc Biol. (2014) 34:1249–59. doi: 10.1161/ATVBAHA.114.303240
119. Di Salvo TG, Guo Y, Su YR, Clark T, Brittain E, Absi T, et al. Right ventricular long noncoding RNA expression in human heart failure. Pulm Circ. (2015) 5:135–61. doi: 10.1086/679721
120. Zhang J, Yuan L, Zhang X, Hamblin MH, Zhu T, Meng F, et al. Altered long non-coding RNA transcriptomic profiles in brain microvascular endothelium after cerebral ischemia. Exp Neurol. (2016) 277:162–70. doi: 10.1016/j.expneurol.2015.12.014
121. Li J, Li Q, Chen L, Gao Y, Zhou B, Li J. Competitive endogenous RNA networks: integrated analysis of non-coding RNA and mRNA expression profiles in infantile hemangioma. Oncotarget. (2018) 9:11948–63. doi: 10.18632/oncotarget.23946
122. Wolfien M, Brauer DL, Bagnacani A, Wolkenhauer O. Workflow development for the functional characterization of ncRNAs. Methods Mol Biol. (2019) 1912:111–32. doi: 10.1007/978-1-4939-8982-9_5
123. Wang J, Liu K, Liu Y, Lv Q, Zhang F, Wang H. Evaluating the bias of circRNA predictions from total RNA-Seq data. Oncotarget. (2017) 8:110914–21. doi: 10.18632/oncotarget.22972
124. Müller R, Weirick T, John D, Militello G, Chen W, Dimmeler S, et al. ANGIOGENES: knowledge database for protein-coding and noncoding RNA genes in endothelial cells. Sci Rep. (2016) 6:32475. doi: 10.1038/srep32475
125. Khan S, Taverna F, Rohlenova K, Treps L, Geldhof V, de Rooij L, et al. EndoDB: a database of endothelial cell transcriptomics data. Nucleic Acids Res. (2019) 47:D736–44. doi: 10.1093/nar/gky997
126. Kozomara A, Birgaoanu M, Griffiths-Jones S. miRBase: from microRNA sequences to function. Nucleic Acids Res. (2019) 47:D155–62. doi: 10.1093/nar/gky1141
127. Van Peer G, Lefever S, Anckaert J, Beckers A, Rihani A, Van Goethem A, et al. miRBase tracker: keeping track of microRNA annotation changes. Database. (2014) 2014:bau080. doi: 10.1093/database/bau080
128. Volders P-J, Anckaert J, Verheggen K, Nuytens J, Martens L, Mestdagh P, et al. LNCipedia 5: towards a reference set of human long non-coding RNAs. Nucleic Acids Res. (2019) 47:D135–9. doi: 10.1093/nar/gky1031
129. Xu J, Bai J, Zhang X, Lv Y, Gong Y, Liu L, et al. A comprehensive overview of lncRNA annotation resources. Brief Bioinform. (2017) 18:236–49. doi: 10.1093/bib/bbw015
130. Sethupathy P, Corda B, Hatzigeorgiou AG. TarBase: a comprehensive database of experimentally supported animal microRNA targets. RNA. (2006) 12:192–7. doi: 10.1261/rna.2239606
131. Chou C-H, Shrestha S, Yang C-D, Chang N-W, Lin Y-L, Liao K-W, et al. miRTarBase update 2018: a resource for experimentally validated microRNA-target interactions. Nucleic Acids Res. (2018) 46:D296–302. doi: 10.1093/nar/gkx1067
132. Andrés-León E, González Peña D, Gómez-López G, Pisano DG. miRGate: a curated database of human, mouse and rat miRNA-mRNA targets. Database. (2015) 2015:bav035. doi: 10.1093/database/bav035
133. Bruno AE, Li L, Kalabus JL, Pan Y, Yu A, Hu Z. miRdSNP: a database of disease-associated SNPs and microRNA target sites on 3'UTRs of human genes. BMC Genom. (2012) 13:44. doi: 10.1186/1471-2164-13-44
134. Bonome T, Levine DA, Shih J, Randonovich M, Pise-Masison CA, Bogomolniy F, et al. A gene signature predicting for survival in suboptimally debulked patients with ovarian cancer. Cancer Res. (2008) 68:5478–86. doi: 10.1158/0008-5472.CAN-07-6595
135. Wu W-S, Tu B-W, Chen T-T, Hou S-W, Tseng JT. CSmiRTar: Condition-specific microRNA targets database. PLoS ONE. (2017) 12:e0181231. doi: 10.1371/journal.pone.0181231
136. Xiao F, Zuo Z, Cai G, Kang S, Gao X, Li T. miRecords: an integrated resource for microRNA-target interactions. Nucleic Acids Res. (2009) 37:D105–110. doi: 10.1093/nar/gkn851
137. Naeem H, Küffner R, Csaba G, Zimmer R. miRSel: automated extraction of associations between microRNAs and genes from the biomedical literature. BMC Bioinform. (2010) 11:135. doi: 10.1186/1471-2105-11-135
138. Dweep H, Gretz N. miRWalk2.0: a comprehensive atlas of microRNA-target interactions. Nat Methods. (2015) 12:697. doi: 10.1038/nmeth.3485
139. Backes C, Kehl T, Stöckel D, Fehlmann T, Schneider L, Meese E, et al. miRPathDB: a new dictionary on microRNAs and target pathways. Nucleic Acids Res. (2017) 45:D90–6. doi: 10.1093/nar/gkw926
140. Gennarino VA, Sardiello M, Mutarelli M, Dharmalingam G, Maselli V, Lago G, et al. HOCTAR database: a unique resource for microRNA target prediction. Gene. (2011) 480:51–8. doi: 10.1016/j.gene.2011.03.005
141. Hsu JB-K, Chiu C-M, Hsu S-D, Huang W-Y, Chien C-H, Lee T-Y, et al. miRTar: an integrated system for identifying miRNA-target interactions in human. BMC Bioinform. (2011) 12:300. doi: 10.1186/1471-2105-12-300
142. Wong N, Wang X. miRDB: an online resource for microRNA target prediction and functional annotations. Nucleic Acids Res. 43:D146–52. doi: 10.1093/nar/gku1104
143. Paraskevopoulou MD, Vlachos IS, Karagkouni D, Georgakilas G, Kanellos I, Vergoulis T, et al. DIANA-LncBase v2: indexing microRNA targets on non-coding transcripts. Nucleic Acids Res. (2016) 44:D231–8. doi: 10.1093/nar/gkv1270
144. Cheng L, Wang P, Tian R, Wang S, Guo Q, Luo M, et al. LncRNA2Target v2.0: a comprehensive database for target genes of lncRNAs in human and mouse. Nucleic Acids Res. (2019) 47:D140–4. doi: 10.1093/nar/gky1051
145. Juzenas S, Venkatesh G, Hübenthal M, Hoeppner MP, Du ZG, Paulsen M, et al. A comprehensive, cell specific microRNA catalogue of human peripheral blood. Nucleic Acids Res. (2017) 45:9290–301. doi: 10.1093/nar/gkx706
146. Barupal JK, Saini AK, Chand T, Meena A, Beniwal S, Suthar JR, et al. ExcellmiRDB for translational genomics: a curated online resource for extracellular microRNAs. OMICS. (2015) 19:24–30. doi: 10.1089/omi.2014.0106
147. Russo F, Bella SD, Nigita G, Macca V, Laganà A, Giugno R, et al. miRandola: extracellular circulating MicroRNAs database. PLOS ONE. (2012) 7:e47786. doi: 10.1371/journal.pone.0047786
148. Yang Q, Qiu C, Yang J, Wu Q, Cui Q. miREnvironment database: providing a bridge for microRNAs, environmental factors and phenotypes. Bioinformatics. (2011) 27:3329–30. doi: 10.1093/bioinformatics/btr556
149. Gong J, Wu Y, Zhang X, Liao Y, Sibanda VL, Liu W, et al. Comprehensive analysis of human small RNA sequencing data provides insights into expression profiles and miRNA editing. RNA Biol. (2014) 11:1375–85. doi: 10.1080/15476286.2014.996465
150. Yang Z, Wu L, Wang A, Tang W, Zhao Y, Zhao H, Teschendorff AE. dbDEMC 2.0: updated database of differentially expressed miRNAs in human cancers. Nucleic Acids Res. (2017) 45:D812–8. doi: 10.1093/nar/gkw1079
151. Mooney C, Becker BA, Raoof R, Henshall DC. EpimiRBase: a comprehensive database of microRNA-epilepsy associations. Bioinformatics. (2016) 32:1436–8. doi: 10.1093/bioinformatics/btw008
152. Huang Z, Shi J, Gao Y, Cui C, Zhang S, Li J, et al. HMDD v3.0: a database for experimentally supported human microRNA-disease associations. Nucleic Acids Res. (2019) 47:D1013–7. doi: 10.1093/nar/gky1010
153. Wang D, Gu J, Wang T, Ding Z. OncomiRDB: a database for the experimentally verified oncogenic and tumor-suppressive microRNAs. Bioinformatics. (2014) 30:2237–8. doi: 10.1093/bioinformatics/btu155
154. Bao Z, Yang Z, Huang Z, Zhou Y, Cui Q, Dong D. LncRNADisease 2.0: an updated database of long non-coding RNA-associated diseases. Nucleic Acids Res. (2019) 47:D1034–7. doi: 10.1093/nar/gky905
155. Gao Y, Wang P, Wang Y, Ma X, Zhi H, Zhou D, et al. Lnc2Cancer v2.0: updated database of experimentally supported long non-coding RNAs in human cancers. Nucleic Acids Res. (2019) 47:D1028–33. doi: 10.1093/nar/gky1096
156. Miao Y-R, Liu W, Zhang Q, Guo A-Y. lncRNASNP2: an updated database of functional SNPs and mutations in human and mouse lncRNAs. Nucleic Acids Res. (2018) 46:D276–80. doi: 10.1093/nar/gkx1004
157. Ning S, Yue M, Wang P, Liu Y, Zhi H, Zhang Y, et al. LincSNP 2.0: an updated database for linking disease-associated SNPs to human long non-coding RNAs and their TFBSs. Nucleic Acids Res. (2017) 45:D74–8. doi: 10.1093/nar/gkw945
158. Dai E, Yu X, Zhang Y, Meng F, Wang S, Liu X, et al. EpimiR: a database of curated mutual regulation between miRNAs and epigenetic modifications. Database. (2014) 2014:bau023. doi: 10.1093/database/bau023
159. Vergoulis T, Kanellos I, Kostoulas N, Georgakilas G, Sellis T, Hatzigeorgiou A, et al. mirPub: a database for searching microRNA publications. Bioinformatics. (2015) 31:1502–4. doi: 10.1093/bioinformatics/btu819
160. Szcześniak MW, Makałowska I. miRNEST 2.0: a database of plant and animal microRNAs. Nucleic Acids Res. (2014) 42:D74–7. doi: 10.1093/nar/gkt1156
161. Gerlach D, Kriventseva EV, Rahman N, Vejnar CE, Zdobnov EM. miROrtho: computational survey of microRNA genes. Nucleic Acids Res. (2009) 37:D111–7. doi: 10.1093/nar/gkn707
162. Potente M, Mäkinen T. Vascular heterogeneity and specialization in development and disease. Nat Rev Mol Cell Biol. (2017) 18:477–94. doi: 10.1038/nrm.2017.36
163. Liu SJ, Nowakowski TJ, Pollen AA, Lui JH, Horlbeck MA, Attenello FJ, et al. Single-cell analysis of long non-coding RNAs in the developing human neocortex. Genome Biol. (2016) 17:67. doi: 10.1186/s13059-016-0932-1
164. Wagner J, Rapsomaniki MA, Chevrier S, Anzeneder T, Langwieder C, Dykgers A, et al. A single-cell atlas of the tumor and immune ecosystem of human breast cancer. Cell. (2019) 177:1330–45.e18. doi: 10.1016/j.cell.2019.03.005
165. Xiao Z, Dai Z, Locasale JW. Metabolic landscape of the tumor microenvironment at single cell resolution. Nat Commun. (2019) 10:3763. doi: 10.1038/s41467-019-11738-0
166. Lukowski SW, Patel J, Andersen SB, Sim S-L, Wong HY, Tay J, et al. Single-cell transcriptional profiling of aortic endothelium identifies a hierarchy from endovascular progenitors to differentiated cells. Cell Rep. (2019) 27:2748–58.e3. doi: 10.1016/j.celrep.2019.04.102
Keywords: angiogenesis, non-coding RNA, epigenetics, neovascularization, next generation sequencing, miRNAs, lncRNAs, circRNA
Citation: Hernández-Romero IA, Guerra-Calderas L, Salgado-Albarrán M, Maldonado-Huerta T and Soto-Reyes E (2019) The Regulatory Roles of Non-coding RNAs in Angiogenesis and Neovascularization From an Epigenetic Perspective. Front. Oncol. 9:1091. doi: 10.3389/fonc.2019.01091
Received: 20 June 2019; Accepted: 03 October 2019;
Published: 24 October 2019.
Edited by:
Erika Ruiz-Garcia, National Institute of Cancerology (INCan), MexicoCopyright © 2019 Hernández-Romero, Guerra-Calderas, Salgado-Albarrán, Maldonado-Huerta and Soto-Reyes. This is an open-access article distributed under the terms of the Creative Commons Attribution License (CC BY). The use, distribution or reproduction in other forums is permitted, provided the original author(s) and the copyright owner(s) are credited and that the original publication in this journal is cited, in accordance with accepted academic practice. No use, distribution or reproduction is permitted which does not comply with these terms.
*Correspondence: Ernesto Soto-Reyes, esotoreyes@correo.cua.uam.mx
†These authors have contributed equally to this work
Disclaimer: All claims expressed in this article are solely those of the authors and do not necessarily represent those of their affiliated organizations, or those of the publisher, the editors and the reviewers. Any product that may be evaluated in this article or claim that may be made by its manufacturer is not guaranteed or endorsed by the publisher.
Research integrity at Frontiers
Learn more about the work of our research integrity team to safeguard the quality of each article we publish.